- CATMAT Lab, Department of Molecular Sciences and Nanosystems, Ca’ Foscari University Venice and INSTM-RU, Venezia Mestre, Italy
Among many guidelines issued by the World Health Organization to prevent contagion from novel coronavirus (SARS-CoV-2), disinfection of animate and inanimate surfaces has emerged as a key issue. One effective approach to prevent its propagation can be achieved by disinfecting air, skin, or surfaces. A thorough and rational application of an Environmental Protection Agent for disinfection of surfaces, as well as a good personal hygiene, including cleaning hands with appropriate products (e.g., 60–90% alcohol-based product) should minimize transmission of viral respiratory pathogens such as SARS-CoV-2. Critical issues, associated with the potential health hazard of chemical disinfectants and the ineffective duration of most of the treatments, have fostered the introduction of innovative and alternative disinfection approaches. The present review aims to provide an outline of methods currently used for inanimate surface disinfection with a look to the future and a focus on the development of innovative and effective disinfection approaches (e.g., metal nanoparticles, photocatalysis, self-cleaning, and self-disinfection) with particular focus on SARS-CoV-2. The research reviews are, usually, focused on a specific category of disinfection methods, and therefore they are limited. On the contrary, a panoramic review with a wider focus, as the one here proposed, can be an added value for operators in the sector and generally for the scientific community.
Introduction
Historically, disease outbreaks have damaged humanity, sometimes changing the course of history and, at times, marking the extinction of entire populations. In December 2019, a new coronavirus (SARS-CoV-2) arises from China generating a worldwide public health emergency; therefore, the unavailability of effective antiviral therapies made things worse. Preventive care is currently the sole concrete option to limit the virus spreading ability. Generally, infections related to a virus and in particular to coronavirus may spread with physical contact and droplets transmission mechanism. Animate and inanimate surfaces play a key role within the diffusion of Covid-19 and more generally of virus and bacterial infections. Depending upon the surface features, pH, temperature, and relatively humidity (RH), viruses (particularly human coronaviruses) can remain active on inanimate surfaces from 1 to 9 days above 30 °C (Kampf et al., 2020).
Surface disinfection (in healthcare environment but also in domiciliary environment) plays a central role on infection inhibition and control strategies adopted in response to transmissible disease outbreaks. A typical definition of disinfectant may be a “substance” or an approach that reduces microbial contamination on the surfaces to standards that can be considered secure from the general public health standpoint. Disinfectant or sanitizing (this word is employed in food industry, particularly in the United States) products are complex formulations where stability, organoleptic and sensory features, and safety for environment and consumers are as valuable as their microbiocidal efficacy.
Professor Tamar Kohn (Sigstam et al., 2013) and her team highlighted that not all disinfectants work the same way. She stated “A virus is a genome and some proteins. We discovered that each disinfectant has totally different effects, attacking one or several of the virus’ functions. Even though the outcome is the same, the eradication methods are different.” Kohn’s research allows to better understand the disinfectants’ action and the parameters that mostly affect their behavior. The erroneous application of the disinfection instructions can even cause greater contamination problems with high impact, especially in hospital environments. It is crucial in this sense to understand how microbial agents are structured.
Viruses are microbial agents of small dimensions made of two main components: the genetic material, DNA or RNA, single-stranded or double-stranded, and a protein coat, called capsid, which protects and encapsulates the genome (Golin et al., 2020). Some viruses have an envelope formed by a double layer of glycoproteins and lipids called pericapsid or peplos. Nonenveloped viruses are more resistant to high temperatures, acids, disinfectants, and drying, while enveloped viruses survive longer in a humid environment but are less resistant. Virus SARS-CoV-2 is an enveloped virus characterized by four different structural proteins: nucleocapsid (N), spike (S), membrane (M), and envelope (E). The S, E, and M proteins build viral envelope, while N protein holds the RNA genome (Wu et al., 2020). SARS-CoV-2 transmission is mainly associated with the spike protein that promotes the virus sticking on different surfaces. The antimicrobial disinfection approaches that are able to attach and to destroy proteins and lipids can be effective toward the new Coronovirus that, therefore, it is more sensitive to inactivation by most chemical disinfectants which compromise its integrity and lower its infectious capacity. Chemical disinfectants (such as chlorines, peroxides, quaternary amines, and alcohols) active against a large spectrum of microbial agents, among that enveloped viruses, have been effectively used for disinfection and sterilization of surfaces and personal protective equipment (Fathizadeh et al., 2020) (Figure 1). Despite the well demonstrated efficacy of chemical disinfectants, they are often associated with several disadvantages such as high concentration requirements to get 100% viral inhibition, time depending effectiveness, and potential hazards to human health and environment (Liu et al., 2012; Wang et al., 2019). The optimization of disinfection approaches that can remain longer on surfaces, begin resistant to washing and friction, with good safety requisites is a challenge. A new perspective is offered by nanotechnology, self-sterilizing surfaces, and light-driven self-disinfections. Metallic nanoparticles, NPs (for example, silver, copper, and titanium dioxide nanoparticles), are considered valuable alternative due to their broad range antiviral activities, persistence, and efficiency at much lower dosage (Sportelli et al., 2020). NPs are often used in synergy with chemical and physical modification of surfaces, for example, by using polymeric functionalization and antiadhesive coatings with improved antimicrobial features (Knetsch and Koole, 2011; Li et al., 2017). Functionalization with opportune semiconductors is another green and very promising method to prevent bacterial contamination by using light-driven approaches. Despite the promising performances, the potentiality of these approaches has not yet been extensively investigated and exploited. For metal NPs, safety issue is a critical point; in fact, the lacks and disagreement about biotoxicities of nanostructured materials toward environment and human health are the principal issues discouraging their massive application.
This review proposes a general overview on the products and approaches currently used for inanimate surface disinfection with a look to the long run and in particular on the development of innovative and effective disinfection approaches (e.g., antimicrobial metals, antimicrobial surfaces, self-cleaning surfaces, and photocatalysis). The attention will be focused on approaches effective toward viruses with particular reference to SARS-CoV-2.
Traditional Disinfection Approaches: Chemical Biocides
Chemical biocides are substances with antiseptic, disinfectant, or preservative activity characterized by significative differences in terms of structure, features, and spectrum of action (Maillard and McDonnell, 2012). The effectiveness of a biocide depends not only on the nature of the agent (e.g., toxicity, corrosiveness, spectrum of action, and stability) but also on the conditions of use (equipment, target pathogen, and level of microbial contamination). Chemical biocides used as disinfectants can be classified into eight distinct classes: alcohol, chlorine, iodophors, quaternary ammonium compounds (QACs), organic acids, anionic sanitizers, carboxylic acid, and oxidant compounds. Ionic, nonionic, and amphoteric surfactants show microbicidal activity and at the same time a good surfactant features and consequently a primary role in the formulation optimization of biocidal products. In Table 1, a list of the principal European approved chemical biocides is reported (European Chemical Agency, 2020).
Disinfectant products are complex formulations where stability, cleaning, safety, and organoleptic properties are important as their microbiocidal effectiveness (Figure 2). An effective disinfectant must have a significant antiviral and antibacterial activity within a low contact time.
An ideally contact times should be greater or equal to kill time; it is based on the results of microbiological testing using U.S. Environmental Protection Agency (EPA)-approved methods. Contact times for disinfectants range from 15 s to 10 min, the maximum time authorized by the EPA (Becker’s Healthcare, 2018; West et al., 2018). Operation instructions of disinfectant products must ensure that the surface is sufficiently wet for the contact time. The minimum effective contact time for a specific biocide is strictly related to the type of pathogen to be countered, to the environmental working conditions, and to the minimum concentration of biocide that can be used in the final formulation of a disinfectant product. It is, therefore, hard to give univocal values of optimal contact time for each biocidal; these values are established from time to time according to the parameters previously highlighted, and they are indicated by the manufacturer. In anyway, the optimal effectiveness of a disinfectant product is, generally, achieved by the right synergy of several ingredients. Alcohols, e.g., ethanol (78–95%) and isopropanol (70–100%), are widely used as effective antimicrobial agents; they exhibit high disinfectant activity with a negligible toxic effect on human skin. Surface protein precipitation of the outer shell of viruses and bacteria is considered crucial in the biocidal activity of alcohols based disinfectants. Through this action, they kill pathogen and prevent infections. They are effective toward virus with envelope, such as Coronaviruses, but not toward viruses, such as norovirus, that do not have the outer layer (known as an envelope). Besides alcohol, other potent biocides widely integrated into surface disinfectant formulations are quaternary ammonium compounds (QACs), membrane activating agents that directly interact with the cytoplasmatic membrane of bacteria and yeast (Wieczorek et al., 2017). The antimicrobial activity is closely correlated with several factors, among which nuclear size, basicity, and chain length play a key role. Chain length from C12–C16 shows the best antimicrobial activity. The long nonpolar tails make QACs active toward a lipid-containing virus like SARS-CoV-2. Long chain hydrocarbons enhance the permeability by affecting the surface-active features whereas the cationic fraction bonded to the charged nucleic acids condenses within the capsid, leading to the virucidal activity (Requião et al., 2020).
An action like QACs is explicated from surfactants. Surfactants and detergents play an important role in disinfection and sterilization protocols to operate in synergy with other antimicrobial agents (Falk, 2019). When surfactants meet the phospholipidic outside layer of SARS-CoV-2, they dissolve it and damage the virus apart. Moreover, the use of surfactant in concert with other microbial agents can improve the disinfection capacity. In a recent study (Jahromia et al., 2020), the effect of surfactant (sodium dodecylbenzenesulfonate (SDBS), sodium laureth sulfate (SLS) commercial dish soap, and liquid hand soap) on coronaviruses and the virucidal efficiency of sanitizing fluids were investigated with particular attention toward evaporation rate and surface contact time. Twelve fluids composed of ethanol, isopropanol, SDBS, SLS, glycerin, and water of standardized hardness (WSH) were tested for their evaporation time and virucidal efficiency. Evaporation time grew by 17–63% in the presence of surfactant agents. In addition, surfactant improved the virucidal efficiency between 15 and 27% (according to the 4-field test in the EN 16615:2015 European Standard method) by providing a synergistic effect with alcohols to inactivate the SARS-CoV-2 virus.
Chlorine-based biocides represent another class of disinfectants effective toward a wide range of bacteria and viruses; moreover, they are generally inexpensive and widely available. A further benefit of chlorine-derived compound is its wide microbiocidal spectrum, while drawbacks include corrosive and irritant features at high concentrations, potential surface damage, and inactivation by organic matter (Iqbal et al., 2016; Centres for Disease Control and Prevention, 2020). The widely used chlorine-based disinfectants are made from a mix of acid (HOCl) and hypochlorite (NaOCl). HOCl effectiveness is superior to that of NaOCl because it selectively binds with unsaturated bonds of lipid layering and destroys cellular integrity. Moreover, acid may be a better oxidant than hypochlorite. The standard formulation is obtained with a dilution of 1:100 of a 5% hypochlorite water solution leading to a final concentration of 0.05% (World Health Organization, 2014). The dynamic equilibrium of the mixture, and consequently its biocidal activity, is significantly affected by the pH of the medium. Alkaline pH fosters the formation of sodium hypochlorite, whereas acid pH favors hypochlorous acid. Moreover, surface texture, in particular the porosity, significantly affects the effectiveness of chlorine-based biocides (Fraise et al., 2013). Another class of disinfectants is represented by peroxides and peracids that induce the formation of free radicals such as superoxide ion, hydroxyl ion, peroxyl ion, hydrogen peroxide radicals that oxidize proteins, nucleic acids, and lipids, by exercising the biocidal activity. Peroxides and peracids based antimicrobial products are used as effective alternative to formaldehyde that is correlated with severe neuro and systemic toxicity (Songur at al., 2010).
It is clear from this brief overview that, as well as chemical biocide, it must always be considered that other parameters influence the disinfection efficacy (Fraise et al., 2013): 1) the target microorganism’s resistance to disinfection; 2) the surface features, for example, the texture and the presence of organic matter; 3) the disinfectant composition (i.e., ingredients and concentration); and 4) the disinfection protocol, such as precleaning, disinfectant application mode, and exposure time. The suitable biocidal and disinfection protocol selection plays a key role in disinfection effectiveness. Recently, Kampf et al. (2020) reviewed the literature about the persistence of human and veterinary coronaviruses on inanimate surfaces and about the chemical disinfection approaches used in healthcare facilities. The analysis of twenty-two studies discloses that human coronaviruses like severe acute respiratory syndrome (SARS) coronavirus, middle east respiratory syndrome (MERS) coronavirus, or endemic human coronaviruses (HCoV) can survive on surfaces such as glass, metal, or plastic for up to 9 days but are deactivated by chemical based disinfection protocols using 62 and 71% ethanol, 0.5% peroxide, or 0.1% hypochlorite within 1 min. Benzalkonium chloride (0.05 and 0.2%) or 0.02% chlorhexidine digluconate is less active.
Most of the currently used chemical biocides are effective for surface disinfection, but the incorrect following of disinfection instructions (for example, biocidal solution concentration and time of exposure) can cause a lack of effectiveness and even greater contamination problems especially in health environments such as hospitals. Other constraints are harmfulness, corrosive nature, and bacterial resistance (Rutala and Weber, 2004). To overcome these issues, new approaches are being designated to limit the microorganism survival over surface and materials. The integration of different disinfection approaches can be a winning strategy to prevent and control infection spreading.
Antimicrobial Materials and Coatings
Antimicrobial Metals, Metal Oxide Nanoparticles, and Coatings
Some materials have intrinsic antimicrobial features. Silver (Ag), copper (Cu), copper oxide (CuO), zinc oxide (ZnO), and gold (Au) have been known and applied for centuries (Dizaj et al., 2014) (Table 2). Metal ions or metal NPs are potentially lethal for pathogens because they destroy microbes directly or through the formation of reactive oxygen species (ROS), by disrupting proteins, ribonucleic acid, intracellular organelles or cell wall, and cell membrane (Amini, 2019). The use of antimicrobial-derived metals can reduce the possibility of formation of harmful disinfection by-products related to traditional disinfection processes. Moreover, in comparison with traditionally used disinfectants, as chemical biocides, (Zhang et al., 2007) metal and metal-derived nanoparticles can exhibit antimicrobial activity at low concentration, and they are more stable in harshest working conditions. Additionally, metal-derived compounds and in particular metal NPs can explain their effectiveness toward a wide range of viruses. Viruses show a considerable heterogeneity in term of size, structure, properties, as well as the way in which they interact with the environment. Metal NPs are smaller than most viral particles, such as the SARS-CoV-2 which has an average size of 120 nm and can attack, facilely, the whole viral particle or the surface proteins and other structural components (as viral genome), leading to the viruses inactivation (Medical Plastics News, 2020; Sportelli et al., 2020) (Figure 3). Among metals, silver is the most used antimicrobial; its bactericidal mechanism is predicated on the binding of silver atoms with thiol (SH) and disulfide (S–S) groups present within the proteins of bacterial cell membranes, resulting in its disruption and eventual necrobiosis (Gordon et al., 2010). Silver can also have different effectiveness consistent with the category of bacteria. Gram-positive bacteria cell walls, contrary to Gram-negative bacteria, don't have an outer membrane, but they have a thicker cell membrane made of several layers of peptidoglycans (Silhavy et al., 2010); this, alongside with their charge, fosters silver ions (positively charged) trapping preventing their entrance into the bacteria (Kawahara et al., 2000) and, consequently, to influence the Ag effectiveness. In addition, Ag has been stated to be less toxic than many other disinfectants (Marabio-Jones and Hoek, 2010). In consideration of these interesting features, silver has been used for many applications on different sectors: plastic products, textiles, and coating (Egger et al., 2009). AGC Glass (AGS Glass United Kingdom Ltd., Rugby, Great Britain) developed an antibacterial glass doped with silver ions that seems to be able to eliminate 99% of bacteria and prevents fungi proliferation. This evidence was demonstrated by evaluating the performance of the glass on a bacteria and two fungi strains (Querido et al., 2019). Surfacine Development Company (Tyngsborough, MA, United States) industrialized an antimicrobial silver coating (Surfacine®) suitable for several materials, from medical devices to food packaging industry or water distribution systems (Querido et al., 2019). Silver, also, possesses a range of biomedical applications, especially when used as silver sulfadiazine in creams or wound dressings (Shao et al., 2015). More recently, silver NPs have been greatly studied to make potential antimicrobial materials such as catheters and surgery sutures (Dhas et al., 2015; Wu et al., 2015) and are widely used to obtain antibacterial and superhydrophobic coatings (Heinonen et al., 2013). Moreover, many reports have proved the antiviral effectiveness of Ag NPs against several human pathogenic viruses such as respiratory syncytial virus (RSV), influenza virus, norovirus, hepatitis B virus (HBV), human immunodeficiency virus (HIV), and SARS-CoV (Han et al., 2005). Silver NPs can carry out their antiviral activity in several ways (Kampf et al., 2020) by interaction with viral envelope and/or viral surface proteins; (Sigstam et al., 2013) by inhibiting the cellular pathways of viral entry; (Golin et al., 2020) by interaction with viral genome; (Wu et al., 2020) by altering the mechanism responsible for the viral replication; and (Fathizadeh et al., 2020) by blocking receptor (Rai et al., 2016). To date, there are no reported research studies demonstrating definitively the effectiveness of Ag NPs on SARS-CoV-2, but affinities with virus SARS-CoV have paved the way to several studies and several disinfectants formulation, and solution based on Ag NPs has been proposed to contrast the Covid-19 pandemic. Ag NPs can be used on a variety of inanimate surfaces. Preliminary studies show that silver nanocluster/silica composite coating on facial masks exhibits virucidal effects against SARS-CoV-2 and could potentially be active when used on the air filters of air conditioners and medical devices (Balagna et al., 2020). In another example, polycotton fabrics containing Ag NPs have been proven to inhibit SARS-CoV-2 (Tremiliosi et al., 2020). At the same time, several Ag-based sanitizers and disinfectants have been proposed on the market for disinfection of hands and inanimate surfaces, respectively (Jeremiah et al., 2020). NanoTechSurface, Italy, developed a durable and self-sterilizing disinfectant surface formula made of titanium dioxide and silver ions (StatNano, 2020). However, there are some unresolved issues concerning the Ag NPs safety in relation with their influence on microbial life when released in the environment (Wei et al., 2015). Similar to silver, copper has been used since ancient times as an antimicrobial agent for water treatment and transportation. The biocidal activity of this metal involves the release of copper ions which destroy the bacterial envelop by a consequent leakage of the cell content and copper ions penetration into the bacteria. Copper ions produce toxic radicals causing oxidative injury to cellular components and DNA degradation (Luo et al., 2017). Recently copper has been exploited successfully in hospital surfaces and medical equipment as an effective medium for preventing hospital acquired infections (Casey et al., 2010). Souli et al. (2017) compared two rooms in a hospital medical care unit: one with copper coated equipment (beds, side table, intravenous pole stands, and so on) and the other with regular equipment. Copper NP equipment promotes a significative reduction of percentage of colonized surfaces (55.6%) compared with the regular compartment (72.5%). Collected experimental data showed reduction both in colonization by Gram-negative and Gram-positive bacteria and in total bioburden (2.9 vs. 7.6 CFU/100 cm2). Usman et al. (2013) investigated the antimicrobial activities of Cu-chitosan NPs (2–350 nm) toward several microorganisms, including methicillin-resistant S. aureus, B. subtilis, P. eruginosa, Salmonella choleraesuis, and C. albicans. Their results indicated the high potential of these nanoparticles as antimicrobial agents. Recently Behzadinasab et al. (2020) designed a copper-based coating to reduce the permanence of SARS-CoV-2 on solids. The coating is made of a composite based on cuprous oxide (Cu2O) particles bound with polyurethane. After 1 h on coated glass or stainless steel, the viral titer was reduced by about 99.9% on average compared with the uncoated sample. The coating performs well in the cross-hatch durability test and retains its activity after 13 days of being immersed in water or after multiple cycles of exposure to the virus and disinfection. As part of the research for increasingly effective methods to improve the antibacterial and antiviral activity of metal NPs, a growing number of studies are focused on the optimization of green and sustainable synthetic approaches. In a recent work, Cu NPs were synthesized by using plant leaf extracts of E. camaldulensis, A. indica, M. koenigii, A. marina, R. rubiginosa, and D. stramonium. The antimicrobial effectiveness of the obtained CuNPs was tested against various human pathogenic microorganisms such as S. aureus, S. mutans, E. coli, K. pneumoniae, S. typhi, and P. eruginosa. CuNPs prepared from A. indica exhibited good chemical and physical features and excellent antibacterial activity against highly resistant clinical isolates (Asghar, 2020). A similar synthetic biological route is reported by Maruthupandy et al. (2020) for the synthesis of CuO and ZnO NPs by using a leaf extract from a Camellia japonica plant. This study showed that CuO and ZnO NPs are promising antimicrobial agent against bacterial and fungal pathogens. The antibacterial test demonstrated that both Gram-positive (B. subtilis and S. pneumoniae) and Gram-negative (P. eruginosa and S. typhimurium) bacterial strains are more prone to ZnO NPs than CuO NPs. As highlighted, ZnO exhibits excellent and generally greatest antimicrobial activity than other antimicrobial-derived metals. In addition, ZnO safety and its compatibility with human skin justify its widespread use as an antibacterial agent and make it a suitable additive for textiles and surfaces that get in contact with the human body (Saraf, 2013). In the last years, the attention was focused on nanostructured zinc oxide with the development of several physical, chemical, and eco-friendly (“green chemistry”) approaches to obtain ZnO NPs and nanocomposites with different morphologies that show significant growth inhibition of a wide spectrum of bacterial species (Roselli et al., 2003; Buzea et al., 2007; Raghupathi et al., 2011). The exact mechanisms of action of ZnO are not completely clear yet, but several models are conceived. One of the proposed mechanisms is based on the electrostatic interactions between ZnO NPs and cell walls resulting in destroying bacterial cell integrity, with the delivery of antimicrobial Zn2+ ions, their accumulation into bacteria cells, and ROS formation (Król et al., 2017). ZnO NP effectiveness is closely related with concentration and surface area; higher concentrations and larger surface area displayed better antibacterial activity (Buzea et al., 2007). Hosseinkhani et al. (2011) investigated the antibacterial features of ZnO nanoparticle against Shigella dysenteriae; a considerable antimicrobial improvement was observed as a result of particle size reduction. Emami-Karvani and Chehrazi (2011) studied, instead, the effects of concentration and particle size reduction on the antibacterial activity of ZnO nanoparticles against Gram-negative (E. coli) and Gram-positive (S. aureus) bacteria. They found that the antibacterial activity of ZnO NPs increased with decreasing particle size and enhancing powder concentration; on the other hand, ZnO bulk powder showed no significant antibacterial activity (Emami-Karvani and Chehrazi, 2011). As previously reported, ZnO is used in a wide range of applications from cosmetic and pharmaceutical products of everyday use to textile and packaging applications. In a recent study, ZnO NPs were deposited onto cotton and starched cotton fabrics (El-Nahhal et al., 2020). Starch improves the adhesion of zinc oxide nanoparticles on cotton and decreases the leaching of ZnO NPs, ensuring antibacterial effectiveness even after intensive laundry regimes that are used in hospitals. ZnO NPs coated cotton materials were functionalized with silver NPs and curcumin, ZnO-Ag nanohybrid, and Zn(II) curcumin complex. ZnO-Ag/cotton material showed synergistic and enhanced antimicrobial efficacy for both Gram-positive and Gram-negative bacteria, thanks to the strong interaction between ZnO and metallic Ag. These features can be well exploited in different textile applications, e.g., medical clothes fabrication to minimize the chance of nosocomial infections. ZnO NPs have shown promising antimicrobial properties and potential applications in food preservation. In fact, ZnO NPs have been successfully incorporated in several polymeric matrices, as LDPE (low density polyethylene/polypropylene) and PP (polypropylene) in order to provide antimicrobial activity and at the same time to improve packaging properties (Espitia et al., 2012). The antimicrobial effectiveness of Zn explains not only toward bacteria but also toward viruses. Hybrid coating (Rai et al., 2020) made of silver, copper, and zinc cations has great virucidal effects against enveloped viruses such as HIV-1, human herpesvirus 1, influenza H1N1, and dengue type 2 viruses, making them excellent virucides to be applied in a wide range of surfaces. The uses of these nanoparticles in different surface and especially in biodegradable polymeric matrices, although recently, promise to expand since the incorporation of ZnO would allow for improvements in material performance, enhancing mechanical, thermal, and barrier properties. Moreover, additional research is required to identify toxicological effects of ZnO nanoparticles and to determine the impact on consumers. Biotoxicities of nanostructured materials toward environment and even humans are the major concerns discouraging their extensive application of metal NPs, especially in a health environment (Parveen et al., 2016). Safety issues related with metal NPs involve also the formulation step, and developing sustainable and safe methods is essential in this field. Green approaches were introduced to synthesize metallic nanostructures with minimum environmental impact and high antiseptic efficacy. Various biological raw materials including plant and algae extracts, as well as different microorganisms such as bacteria, fungi, yeast, and phytochemical (plant derived secondary metabolites) have been utilized for preparing metallic nanomaterials by biological and green chemistry approaches (Dizaj et al., 2014; Su and Chang, 2018). These methods have been successfully exploited for the preparation of Au NPs that are widely used for medical, diagnostic (Fatemi et al., 2017), and therapeutic applications (Amini et al., 2017). Au NPs are also well known for their antibacterial activity. Even ROS generation is the main cause of antibacterial and antibiotic effectiveness for most nanomaterials, antimicrobial activity of Au nanoparticles does not induce any ROS-generation process. Cui et al. (2012) demonstrated that Au NPs attack bacterial membrane inducing a successive membrane modification and ATP level decrease and inhibition of tRNA binding to the ribosome (El-Nahhal et al., 2020). Tiwari et al. (2011) investigated the antibacterial and antifungal activities of Au NPs functionalized with 5-fluorouracil against Micrococcus luteus, S. aureus, P. eruginosa, E. coli, Aspergillus fumigates (A. fumigates), and Aspergillus niger (A. niger). The authors concluded that Au NPs are more effective toward Gram-negative bacteria than Gram-positive ones due to their easier access into Gram-negative bacteria wall. In the light of the very promising antibacterial features of Au NPs in the last years, as previously highlighted, several biological approaches by using plant extract and phytochemical have been developed to make antimicrobial Au NPs or to prepare gold-coated surfaces (Tettey et al., 2012; El-Batal et al., 2013; Kim et al., 2017). Au NPs prepared by using Gallic acid show discrete antimicrobial activity toward Escherichia coli and Staphylococcus aureus (Su and Chang, 2018). In another study, cinnamaldehyde-coated Au NPs with an average diameter of 11 ± 3 nm were prepared with a direct one-pot synthesis approach, and the obtained NPs were effective against both classes of Gram bacteria. Interestingly, cinnamaldehyde-coated Au NPs interfere with the hyphae formation of Candida albicans and decrease pathogenicity of the organism (Ramasamy et al., 2017).
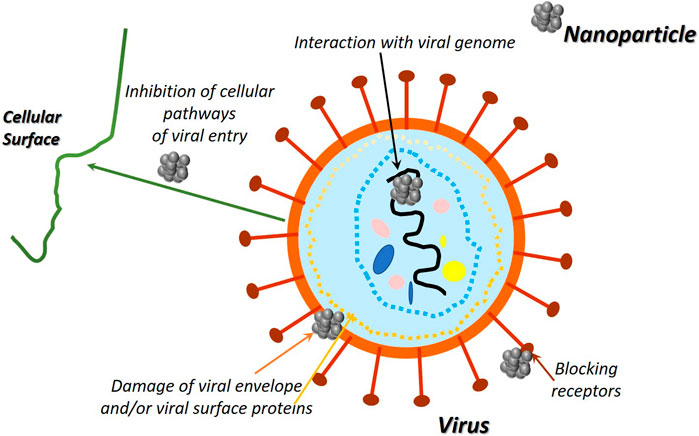
FIGURE 3. Picture of enveloped and nonenveloped virus structure and of principal chemical biocides showing activity toward enveloped virus deactivation.
Concluding, metal and metal oxide NP exploitation could be considered as a suitable way for the development of effective antimicrobial approaches. Metal NPs (especially metal oxide nanoparticles) show not only good antimicrobial effects but also good resistance to strong environmental conditions.
Moreover, metal-derived compounds and coating can explain their effectiveness toward a wide range of viruses, especially toward enveloped viruses as SARS-CoV-2. It may be concluded that metal oxide and metal-based NPs may be extensively used in the near future in synergy with other antimicrobial products and technologies to develop effective disinfection approaches. For example, the integration of metal antimicrobial NPs and antiadhesive technologies can be a good way to prevent bacterial contamination and to improve the effectiveness of sanitation protocols.
Antiadhesive and Antimicrobial Surfaces
Biofilm formation on surfaces, especially in public and hospital environments, is a serious issue (Abdallah et al., 2014; Centres for Disease Control and Prevention, 2016; Khelissa et al., 2017), and it is considered from the World Organization Health (WHO) as one of the main causes of infection propagation. Surface modification to prevent or reduce surface contamination and bacterial growth is a challenge. Surfaces with antiadhesive features or functionalized with antimicrobial substances or with biological active metals are some of the strategies recently proposed.
Numerous natural or biological surfaces have been subjected to diverse evolutionary processes that make them resistant to colonization of microorganisms (Sifri et al., 2016). Several plants, insect species, and animals have developed superhydrophobic surfaces with water active properties and mimesis that consent to them an improved survival in their environment (Darmanin and Guittard, 2015) (Figure 4). Natural superhydrophobic layers can prevent water accumulation, inhibit adhesion to external substances, and make easy droplet rolling to capture contaminants at their surface and sometimes to inhibit microbial growth. For example, plants can use their self-cleaning properties to remove solid and liquid contaminants that can hinder the photosynthesis (Watson et al., 2017); on the other hand, the superhydrophobic layer on the surface of mosquito eyes works as antifogging. Some of these natural antiadhesive or self-cleaning surfaces have been investigated for their potential application on micro/nanostructured surfaces development. It is also possible to give antiadhesive properties to a surface by modifying the material, namely, using self-assembled monolayers or polymer brushes immobilized on the surface (Gao et al., 2011b; Junter et al., 2016).
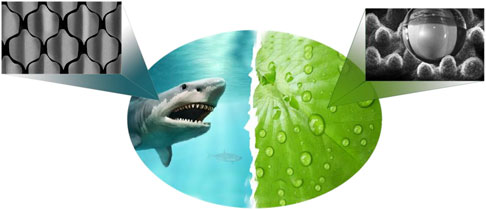
FIGURE 4. Examples of hydrophilic and hydrophobic surface coatings obtained by polymeric coating and by shark skin mimicking, respectively.
There are several chemical ways to obtain materials with antifouling properties. For instance, highly negative charged surfaces, i.e., polyanionic surfaces, could repulse the bacteria containing polyanionic lycocalice (most of Gram-positive bacteria) through electrostatic interactions. However, Gram-negative bacteria have policationic glycocalices, so this surface modification mechanism is only effective against some bacterial species and sometimes only against specific strain types (Campoccia et al., 2013). Zwitterionic materials, such as carboxybetaine, sulfobetaine, and phosphobetaine, also exhibit antifouling properties. Zwitterionic polymers are ultra-hydrophilic materials made with an equal number of anionic and cationic units that confer great hydration ability (Mi and Jiang, 2014) (Figure 3). Studies regarding surface modification with zwitterionic polymers have proved to be a promising strategy to reduce microorganisms’ adhesion (Han et al., 2017). A recent report (Peng et al., 2020) proposes an innovative approach to obtain a zwitterionic/active ester copolymer coating via a two-step process based on a reversible addition-fragmentation chain transfer polymerization (RAFT). Zwitterionic sulfobetaine/active ester block copolymers (pSBMA-b-pNHSMA) with different zwitterionic segment lengths (repeat units of 20 and 90) were prepared. SR substrates (silicone rubber catheter) were modified with a stable polymeric coating anchored on the surface through bonding between the active ester pendant from the copolymer and the amino residues on the activated SR surface. The composition of block polymers determines the ability to prevent biofilm formation, inflammation, blood coagulation, and cell/tissue adhesion. It was highlighted, in particular a correlation between the length of zwitterionic sulfobetaine segment and bacterial adhesion. In a very recent work (Tang et al., 2020), the effectiveness of a novel zwitterionic material was evaluated. It was obtained by reaction of iodopropionic acid with a copolymer of styrene and 4-vinylpyrridine to mitigate biofouling on commercial PVDF membranes. Resistance to biofouling was considerably improved in various conditions (static and dynamic) and by using a large variety of biofoulants (proteins, bacteria, and human cells). Another important highlight of this research is the material resistance to steam sterilization that determines the membranes reusability without losing the antifouling property.
Another effective approach that can be used to hinder microorganisms’ adhesion is surface coating with polymeric brushes. These polymers are usually hydrophilic, so water will be attracted into the brush making a repellent layer in aqueous environment. The water adsorbed in the brush and the elasticity of the polymer chains can repel by steric hindrance proteins and microorganisms encountering the brush surface (Gao et al., 2011a). In a recent study, Hadjesfandiari et al. (2014) demonstrated that brushes coatings, obtained by using polyethylene oxide (PEO) covalently immobilized, reduced up to 98% the surface adhesion of Staphylococci and E. coli.
The antiadhesive features of a surface can be modulated, also, by acting on the surface texture. An example is the superficial functionalization by nanostructured materials (zeolites, nanoparticles, nanofibers, or nanotubes) reducing the area available for microorganisms’ adhesion (Knetsch and Koole, 2011; Yee et al., 2015; Fagan et al., 2016; Li et al., 2017).
Moreover, as previously said, mimicking the topography of antiadhesive natural surfaces is an innovative and effective approach to obtain self-cleaning and self-disinfecting surfaces. Many plants possess unusual surface properties, namely, wettability. Lotus leaves have micropapillae covered by nanostructures with fine branch-like shape that give the lotus superhydrophobic surface and antifouling properties. Water droplets slip away due to lotus leaf hydrophobicity dragging out the foreign agents and keeping its surface clean (Yamamoto et al., 2015).
Shark skin is another valuable example of antiadhesive surface. Sharks have a unique scale micropattern made of denticles organized in a distinct diamond pattern with millions of tiny ribs (Figure 2). The ribbed texture justifies the antibiofouling, self-cleaning, hydrophobic, and drag reducing properties of shark skin (Jaggerssar et al., 2017). Due to these features, shark skin is one of the most mimicked surfaces. The replication of shark skin topography to obtain different substrates with antibacterial adhesion and antifouling features is the core business of an US company (Sharklet Technologies, Alachua, FL, United States) (Sullivan and Regan, 2011). Sharklet micropattern surfaces compared with regular surfaces in a clinical simulated scenario demonstrated that shark skin features decreased the bacterial contamination by about five fold (Reddy, 2014).
Functionalization of surfaces with antibacterial coatings further reduces the bacterial propagation. Therefore, the development of controlled release approaches is a key factor to regulate the biological activity of the coating (Cloutier et al., 2015).
In a recent study, Pejman et al. (2020) investigated the antifouling, antibiofouling, and antimicrobial activity of a thin-film composite forward osmosis membrane functionalized with silver-based metal organic frameworks (Ag-MOFs) to strengthen the membrane. The antifouling and antibiofouling features of the membranes are tested using sodium alginate and E. coli, respectively. Experimental data confirm an excellent antifouling and antibiofouling behavior of functionalized membranes, with high resilience against flux decline. He et al. (2016) designed contact-active antibacterial surfaces by using a quaternary ammonium salt waterborne polyurethane (GWPU) made with an antifouling polyethylene glycol/lysine agent. The coating showed an excellent biological activity to contrast microbial adhesion on inert surfaces, thanks to a reversed surface structure integrated with the antibacterial upper-layer and the antifouling sublayer. The antibacterial activity of the upper-layer and antifouling attributes of the sublayer led to an enhancement of the coating surface features with good antimicrobial activity toward both Gram-positive and Gram-negative bacteria. Recently, an effective method to prepare multifunctional silk coated with a superhydrophobic layer made with a water repellent compounds and silica nanoparticles has been developed. The multifunctional coating proves superhydrophobic and superoleophobic behavior (contact angle > 150°) against water, and it protects silk from bacterial contamination (Aslanidou and Karapanagiotis, 2018).
As reported, there are several successful chemical and physical strategies to give a surface antiadhesive and to prevent biofilm growth. Functionalization with opportune metal semiconductors is a green and very promising approach to prevent bacterial contamination by using light-driven approaches. In the next section, a brief overview concerning this topic will be outlined.
Light-Driven Self-Sterilizing Surfaces
Solar disinfection (SODIS) has emerged in the last decades as cheap and effective technology to sterilize freshwater by only using solar radiation, which is abundant, cheap, and widespread worldwide, making this an appealing disinfection approach especially in poor countries (McGuigan et al., 2012). SODIS works by photoexcitation of microorganism chromophores by UVA light (315–400 nm), leading to an in situ generation of reactive oxygen species (ROS), such as superoxide (O2−), hydroperoxyl (HO2∙), hydroxyl (HO∙) radicals as well as hydrogen peroxide (H2O2). ROS species induce several chemical damaging effects within the microorganism, affording in their death (Castro-Alférez et al., 2016). Besides solar radiation, UVC light (100–280 nm) from artificial sources (i.e., LED) can be involved in disinfection (Lee et al., 2018), by a direct damaging of microorganism DNA without any ROS, through photochemical reaction occurring on pyrimidines (McGuigan et al., 2012). The main disadvantage of both mechanisms is the strong dependence on the photochemical properties of microorganism chromophores, which cannot be tuned a priori. Furthermore, the UVC approach requires special equipment due to their absence in solar light and their harmfulness for human being, losing the key advantages of sunlight.
Photocatalysis (PC) is a competitive process in which ROS are generated on the surface of a selected semiconductor, acting as light-activated catalyst or photocatalyst (Robertson et al., 2012). The key aspect of such approach is that, like materials used for photocatalytic pollutant degradation, the photocatalyst can be specifically designed to maximize the ROS generation and to exploit longer wavelength radiation (i.e., visible light) (Boyjoo et al., 2017), abundant in sunlight but less effective in SODIS. The general mechanism of photocatalysis is reported in Figure 5. From an electronic point of view, a semiconductor (SC) is composed of two sets of molecular orbitals: a low-energy called valence band (VB) and completely occupied by electrons in equilibrium conditions (dark) and a high-energy set called conduction band (CB) empty in dark. The energetic gap between those bands is called bandgap (Eg). When a photon of suitable energy (Eph), namely, Eph > Eg, strikes a surface, an electron can be promoted from the valence band to the conduction band, leading to the formation of a photoexcited electron in the CB and an electronic hole in the VB (process 1). Such species are very reactive and can react each other, leading to a nonradiative or radiative recombination process (process 2, Figure 4), losing the absorbed energy. If these charge carriers, electrons, and holes reach the catalyst surface, they can react with a suitable electron acceptor, like oxygen (process 3, Figure 4) and donor like water or organic compounds (process 4, Figure 4), respectively, affording in several ROS and other radical species (Mills and Hunte, 1997). The absolute position of CB and VB can be either reported as absolute energy value vs. vacuum (eV) (Xu and Schoonen, 2000) or as potential vs. a reference electrode (Sivula and van de Krol, 2016), the latter more useful for comparing standard redox potential of acceptor/donor species.
The formation of ROS is a complex phenomenon, dealing with different pathways, side reactions as well as strongly dependent on the type of SC, as extensively reviewed by Nosaka and Nosaka (2017). The formation reaction, with the corresponding potentials, and other features are summarized in Table 3.

TABLE 3. ROS generation reactions and other properties (Boyjoo et al., 2017). Standard redox reduction potential (E°) is given respect to the reversible hydrogen electrode (RHE). E° vs. RHE = (E° vs. NHE) + 0.059×pH, where NHE is the normal hydrogen electrode.
The utilization of PC in disinfection has been widely reported for water disinfection (Fujishima et al., 2000); however, it has been also proposed as an effective tool for self-sterilization of different surfaces in mild conditions, competitive with harsh treatment (i.e., hot steam or aggressive chemicals such as NaClO) (Fujishima et al., 2000). TiO2 is by far the most reported PC material for several applications, among them also PC self-sterilizing surfaces (Fujishima et al., 2008). ZnO has been also proposed for self-sterilizing surfaces (Saif et al., 2013), while other materials such as WO3 (Zhu et al., 2016), BiVO4 (Wang et al., 2012), or CeO2 (Zhou et al., 2018) have been observed to be active in photocatalytic sterilization in aqueous-base medium though no application on light-induced self-sterilizing surfaces has been proposed. Ag NPs have been often implemented on the photocatalyst to both improve the visible-light harvesting through the localized surface plasmon resonance (LSPR) and improve the reduction ability of photoexcited electrons (Zhu et al., 2016; Zhou et al., 2018). Finally, it is worth to mention an interesting phenomenon related to WO3: the PC energy-storage systems. TiO2-WO3 composites have been observed to be able to store the photoexcited electrons generated within TiO2, by their injection in WO3, with the partial reduction of W6+ and charge compensation by intercalation of a cation (H+ or Na+) arising from either humidity wetting the surface or a liquid electrolyte in which the material is immersed (Tatsuma et al., 2002). The light-accumulated electrons within WO3 can be then discharged by reacting with O2 in dark for several hours, thus releasing the previously light-accumulated chemical energy (Tatsuma et al., 2001). These energy-storage systems have been also used in self-sterilizing surfaces, allowing the PC material to be active, by generating ROS, even in dark (Tatsuma et al., 2003). The overall mechanism of charge-accumulation is reported in Figure 6.
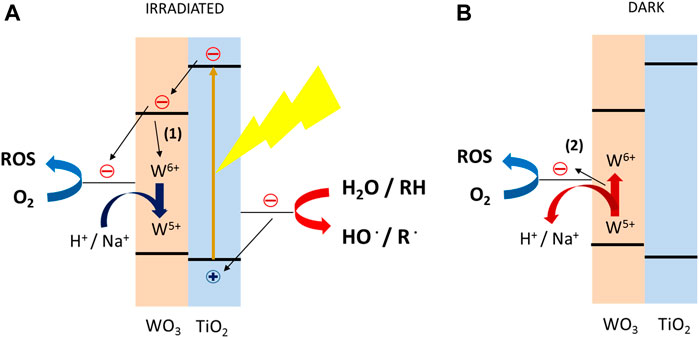
FIGURE 6. Mechanism of reductive energy storage of TiO2-WO2 photocatalyst (Wang et al., 2012).
Besides the sole role of PC-generated ROS, an interesting synergistic effect between PC and antimicrobial cations such as Cu2+, specifically a Cu2+/TiO2 PC system, has been reported to improve the sterilization efficiency, allowing a deeper penetration of Cu2+ within the microorganism (Sunada et al., 2003). Cu+ ions have been observed to perform better than Cu2+ in PC sterilization with TiO2 and, remarkably, even in dark conditions (Qiu et al., 2012b). The utilization of Cu2O as Cu+ source is however hindered by its poor chemical stability in water and air (Hua et al., 2011). An interesting air-stable Cu+ oxide is represented by the copper iron delafossite (CuFeO2) which has been observed to be stable up to 500 °C (Xiong et al., 2015). This material has been observed to be effective on both virus (Qiu et al., 2012a) and fungi (Abdelhamid et al., 2016) inactivation.
Furthermore, being this material is a p-type semiconductor (SC), it has been recently addressed as promising candidate in photoelectrochemical (PEC) water reduction due to its stability in water, narrow bandgap (1.47 eV), and high-lying CB (Prévot et al., 2015). The presence of CuO overlayer was observed to improve electron extraction from CuFeO2 (heterojunction) and allow the reduction of a very thermodynamically stable molecule such as CO2 (Kangab and Park, 2017). CuFeO2 has been also used as photocatalyst for Cr(VI) (Xu et al., 2017) and Cd2+ (Bassaid et al., 2009) removal; however, no example in photocatalytic self-cleaning or self-sterilizing surfaces has been reported yet.
It has been also observed to be active as heterogeneous catalyst in the Fenton reaction (dark process), affording in the generation of radical ROS, HO∙ in particular, from H2O2 either from Cu(I) or Fe(III) composing the CuFeO2 (Zhang et al., 2014), (Figure 7):
All these features make CuFeO2 a potential candidate in development of light and dark self-sterilizing surfaces.
Finally, Zn-based oxides are another class of potential bifunctional material. In fact, besides the already mentioned PC sterilization effect, ZnO is known to possess biocidal activity even in dark conditions due to a slow release of Zn2+ ions within the microorganism (Joe et al., 2017). Ca(OH)2-ZnO materials used as coating for both glass and limestone were also observed to be active in fungi deactivation on light-dark conditions though the study was not explicitly related to PC sterilization (Gómez-Ortíz et al., 2013).
PC materials have been widely implemented as coating on several inorganic surfaces, such as stones (Quagliarini et al., 2012), ceramics (Määttä et al., 2007), or alloys (Boroujeny et al., 2012), either using low-temperature process (Qiu et al., 2012b) or high-temperature treatments (Qiu et al., 2012a). Inorganic surfaces have the key advantage of being stable against the chemical attack of both ROS and photoexcited holes. On the contrary, organic materials such as cellulose, that compose organic surfaces such as paper, cotton fabric, or wood, are sensitive to PC degradation (Wakerley et al., 2017). Nevertheless, immobilization on organic surfaces such as cotton fibers has been also reported, either through direct PC NPs (NPs)-cellulose interaction (Afzal et al., 2013) or by cellulose functionalization with molecular linking groups (i.e., COOH), to improve the interaction with metal oxide PC NPs (Wijesena et al., 2015).
Low-temperature immobilization processes are appealing for both inorganic and organic surfaces functionalization, as well as for the mild operative conditions, allowing a safer, cheaper, and more eco-friendly process. However, stability of the coating is an issue that has to be properly addressed, especially on a long-term timescale (Munafò et al., 2014). Goffredo et al. (2017) reported a spray-coating method of TiO2 NP sols (from Salentec s.r.l.) on travertine (limestone) affording on a good stability after prolonged UVA aging, though a previous work with lab-made TiO2 NP sols, and the same coating technique on the same surface afforded a remarkable decrease in photocatalytic self-cleaning activity (Xu et al., 2017). From these results, it appears that the choice of a proper PC NP material is essential to afford a stable coating. More detailed studies on process conditions, both as physico-chemical features of PC NP and spray-coating parameters, are likely to be important in order to get a comprehensive knowledge about the stability of such PC layers. Veltri et al. (2019) reported another simple low-temperature coating technique, avoiding the requirement of spray-coating equipment and relying on a simple wet impregnation of a TiO2 NP aqueous sol; the photocatalytic biocidal activity on fungi was observed to be retained even after prolonged washing procedure because of the deeper penetration of TiO2 NPs within travertine pores.
Concerning coating on organic surfaces, like cotton fibers, an interesting result was obtained by Afzal et al. (2013) by using a dip-pad-dry-cure process from a TiO2 NP aqueous sol and then functionalized with a Cu-porphyrin for visible-light harvesting. The cotton-coated fabric was observed to retain the self-cleaning ability after 30 h of visible-light irradiation or by washing with different liquids. This result is promising since no cotton functionalization was required to bind the TiO2 NPs.
As general perspective, the immobilization of a selected PC onto both inorganic or organic surfaces is something still challenging, requiring both the optimization of an active PC and an effective immobilization technique that allows a stable surface-to-PC linkage. Finally, please note that despite an ideal PC for the degradation of harmful substances should afford on a complete mineralization to CO2, H2O, and inorganic ions (Keller et al., 2003; Kaneco et al., 2004), PC sterilization requires only a partial degradation of cellular component of microorganisms to get their inactivation (Castro-Alférez et al., 2016). This means that a less active PC could be still enough for photo-induced sterilization while limiting the ROS generation which can afford in the damaging process of the surface, especially the organic ones (i.e., cotton).
Conclusions
Surface disinfection (in healthcare and domiciliary environment) plays a key role in environmental infection prevention and control strategies adopted to contrast infectious disease outbreaks. In most cases, the application of correct disinfection protocol is the only concrete option left to restrict virus and bacterial spread ability. The integration of different products and approaches is mandatory. Chemical disinfectants (such as chlorines, peroxides, quaternary amines, and alcohols) efficient against a large spectrum of pathogens, among that enveloped viruses, have been successfully used for disinfection and sterilization of personal protective equipment and surfaces. Despite the proven efficacy of chemical disinfectants, they exhibit different disadvantages such as high concentration requirements for 100% viral inhibition, limited effectiveness over time, and possible hazards concerns. In this review, a collection of the principal innovative surface disinfectants was proposed, with particular attention to metal NPs and self-sterilizing approaches. Metallic NPs have been proposed as effective alternatives due to their broad range antiviral activities, persistence, and ability to be effective at lower dosage. Copper, silver, and titanium in particular showed activity toward SARS-CoV-2 and have been used in a wide range of applications. Surface coating with self-sterilizing features is another very powerful and promising approach against bacterial and viral contamination. In this context, a sustainable, relatively cheap, and effective approach is the use of light-induced disinfection from photocatalysis (PC). The utilization of PC, by using pristine or promoted semiconductors, has been proposed as an effective tool for self-sterilization of different surfaces in mild conditions, competitive with harsh treatment (i.e., hot steam or aggressive chemicals such as NaClO). By improving the antifouling and antimicrobial features of the surfaces, it is possible to assure the maximum effectiveness of traditional and innovative approaches. Surface functionalization with polymeric brushes or zwitterionic polymers in synergy with silver and copper NPs appears a successful method to contrast microbial surface adhesion and growth.
Despite the promising performances, the potentiality of these approaches has not yet been extensively investigated and exploited. For metal NPs, safety issue is a critical point; in fact, the disagreement about biotoxicities of nanostructured materials toward environment and even human health is the major concerns; several approaches, among that biological, green synthesis, and NPs coatings, are actually studied to face these issues. On the other hand, the effectiveness of self-cleaning and self-sterilizing surfaces against viruses is not fully proven.
Concluding, effective and integrate disinfection approaches supported from appropriate and reliable protocol to verify their effectiveness and safety are the right route to control and combat infection propagations, and today more than ever this is an issue of primary importance.
Author Contributions
MS conceptualized the study and involved in project administration, supervision, and resources. MS wrote, reviewed, and edited the manuscript. EG performed investigation, developed methodology, wrote and reviewing the manuscript. LL performed investigation, developed methodology, and wrote and reviewed the manuscript. MP performed investigation, developed methodology, and wrote and reviewed the manuscript. DZ developed methodology. DZ wrote, reviewed, and edited the manuscript. FM developed methodology and wrote and reviewed the manuscript.
Conflict of Interest
The authors declare that the research was conducted in the absence of any commercial or financial relationships that could be construed as a potential conflict of interest.
References
Abdallah, M., Benoliel, C., Drider, D., Dhulster, P., and Chihib, N. E. (2014). Biofilm formation and persistence on abiotic surfaces in the context of food and medical environments. Arch. Microbiol. 196, 453–472. doi:10.1007/s00203-014-0983-1
Abdelhamid, H. N., Kumarana, S., and Wu, H. F. (2016). One-pot synthesis of CuFeO2 nanoparticles capped with glycerol and proteomic analysis of their nanocytotoxicity against fungi. RSC Adv. 6, 97629–97635. doi:10.1039/C6RA13396G
Afzal, S., Daoud, W. A., and Langford, S. J. (2013). Photostable self-cleaning cotton by a copper(II) porphyrin/TiO2 visible-light photocatalytic system. ACS Appl. Mater. Interfaces 5, 4753–4759. doi:10.1021/am400002k
Amini, S. M. (2019). Preparation of antimicrobial metallic nanoparticles with bioactive compounds. Mater. Sci. Eng. C. 103, 109809. doi:10.1016/j.msec.2019.109809
Amini, S. M., Kharrazi, S., and Jaafari, M. R. (2017). Radio frequency hyperthermia of cancerous cells with gold nanoclusters: an in vitro investigation. Gold Bull. 50, 43–50. doi:10.1007/s13404-016-0192-6
Asghar, M. A. (2020). Green synthesized and characterized copper nanoparticles using various new plants extracts aggravate microbial cell membrane damage after interaction with lipopolysaccharide. Int. J. Biol. Macromol. 160, 1168–1176. doi:10.1016/j.ijbiomac.2020.05.198
Aslanidou, D., and Karapanagiotis, I. (2018). Superhydrophobic, Superoleophobic and antimicrobial coatings for the protection of silk textiles. Coatings 8, 101. doi:10.3390/coatings8030101
Balagna, C., Perero, S., Percivalle, E., Nepita, E. V., and Ferraris, M. (2020). Virucidal effect against coronavirus SARS-CoV-2 of a silver nanocluster/silica composite sputtered coating. Open Ceram. 1, 100006. doi:10.1016/j.oceram.2020.100006
Bassaid, S., Chaib, M., Omeiri, S., Bouguelia, A., and Trari, M. (2009). Photocatalytic reduction of cadmium over CuFeO2 synthesized by sol–gel. J. Photochem. Photobiol. A. 201, 62–68. doi:10.1016/j.jphotochem.2008.09.015
Becker’s Healthcare (2018). The importance of contact time and visible wetness to ensure effective disinfection. Available at: https://www.beckershospitalreview.com/quality/the-importance-of-contact-time-and-visible-wetness-to-ensure-effective-disinfection.html (Accessed October 22, 2020).
Behzadinasab, S., Chin, A., Hosseini, M., Poon, L., and Ducker, W. A. (2020). A surface coating that rapidly inactivates SARS-CoV-2. ACS Appl. Mater. Interfaces 12, 34723–34727. doi:10.1021/acsami.0c11425
Boroujeny, B. S., Afshar, A., and Dolati, A. (2012). Photoactive and self-cleaning TiO2–SiO2 thin films on 316L stainless steel. Thin Solid Films 520, 6355–6360. doi:10.1016/j.tsf.2012.03.051
Boyjoo, Y., Sun, H., Liu, J., Pareek, V. K., and Wang, S. (2017). A review on photocatalysis for air treatment: from catalyst development to reactor design. Chem. Eng. J. 310, 537–559. doi:10.1016/j.cej.2016.06.090
Buzea, C., Pacheco, I. I., and Robbie, K. (2007). Nanomaterials and nanoparticles: sources and toxicity. Biointerphases 2, 17–71. doi:10.1116/1.2815690
Campoccia, D., Montanaro, L., and Arciola, C. R. (2013). A review of the biomaterials technologies for infection-resistant surfaces. Biomaterials 34, 8533–8554. doi:10.1016/j.biomaterials.2013.07.089
Casey, A. L., Adams, D., Karpanen, T. J., Lambert, P. A., Cookson, B. D., Nightingale, P., et al. (2010). Role of copper in reducing hospital environment contamination. J. Hosp. Infect. 74, 72–77. doi:10.1016/j.jhin.2009.08.018
Castro-Alférez, M., Polo-López, M. I., and Fernández-Ibáñez, P. (2016). Intracellular mechanisms of solar water disinfection. Sci. Rep. 6, 38145. doi:10.1038/srep38145
Centers for Disease Control and Prevention (2016). Annual report. Available at: https://www.cdc.gov/globalhealth/resources/reports/annual/2016/index.html (Accessed October 23, 2020).
Centers for Disease Control and Prevention (2020). Guideline for disinfection and sterilization in healthcare facilities. Available at: https://www.cdc.gov/infectioncontrol/guidelines/disinfection/ (Accessed June 8, 2020).
Cloutier, M., Mantovani, D., and Rosei, F. (2015). Antibacterial coatings: challenges, perspectives, and opportunities. Trends Biotechnol. 33, 637–652. doi:10.1016/j.tibtech.2015.09.002
Cui, Y., Zhao, Y., Tian, Y., Zhang, W., Lü, X., and Jiang, X. (2012). The molecular mechanism of action of bactericidal gold nanoparticles on Escherichia coli. Biomaterials 33, 2327–2333. doi:10.1016/j.biomaterials.2011.11.057
Darmanin, T., and Guittard, F. (2015). Superhydrophobic and superoleophobic properties in nature. Mater. Today 18, 273–285. doi:10.1016/j.mattod.2015.01.001
Dhas, S. P., Anbarasan, S., Mukherjee, A., and Chandrasekaran, N. (2015). Biobased silver nanocolloid coating on silk fibers for prevention of post-surgical wound infections. Int. J. Nanomed. 10, 159–170. doi:10.2147/IJN.S82211
Dizaj, S., Lotfipour, F., Barzegar-Jalali, M., Zarrintan, M., and Adibkia, K. (2014). Antimicrobial activity of the metals and metal oxide nanoparticles. Mater. Sci. Eng. C Mater. Biol. Appl. 44, 278–284. doi:10.1016/j.msec.2014.08.031
Egger, S., Lehmann, R. P., Height, M. J., Loessner, M. J., and Schuppler, M. (2009). Antimicrobial properties of a novel silver-silica nanocomposite material. Appl. Environ. Microbiol. 75, 2973–2976. doi:10.1128/AEM.01658-08
El-Batal, A. I., Hashem, A. A., and Abdelbaky, N. M. (2013). Gamma radiation mediated green synthesis of gold nanoparticles using fermented soybean-garlic aqueous extract and their antimicrobial activity. SpringerPlus 2, 129. doi:10.1186/2193-1801-2-129
El-Nahhal, I. M., Salem, J., Anbar, R., Kodeh, F. S., and Elmanama, A. (2020). Preparation and antimicrobial activity of ZnO-NPs coated cotton/starch and their functionalized ZnO-Ag/cotton and Zn(II) curcumin/cotton materials. Sci. Rep. 10, 5410. doi:10.1038/s41598-020-61306-6
Emami-Karvani, Z., and Chehrazi, P. (2011). Antibacterial activity of ZnO nanoparticle on Gram positive and gram-negative bacteria. Afr. J. Microbiol. Res. 5, 1368–1373. doi:10.5897/AJMR10.159
Espitia, P. J. P., Soares, N. F. F., Coimbra, J. S. R., Andrade, N. J., Cruz, R. S., and Medeiros, E. A. A. (2012). Zinc oxide nanoparticles: synthesis, antimicrobial activity and food packaging applications. Food Bioprocess Technol. 5, 1447–1464. doi:10.1007/s11947-012-0797-6
European Chemical Agency (2020). Biocidal active substances. Available at: https://echa.europa.eu/it/information-on-chemicals/biocidal-active-substances (Accessed July 25, 2020).
Fagan, R., McCormack, D. E., Dionysiou, D. D., and Pillai, S. C. (2016). A review of solar and visible light active TiO2 photocatalysis for treating bacteria, cyanotoxins and contaminants of emerging concern. Mater. Sci. Semicond. Process 42, 2–14. doi:10.1016/j.mssp.2015.07.052
Falk, N. A. (2019). Surfactants as antimicrobials: a brief overview of microbial interfacial chemistry and surfactant antimicrobial activity. J. Surfactants Deterg. 22, 1119–1127. doi:10.1002/jsde.12293
Fatemi, F., Amini, S. M., Kharrazi, S., Rasaee, M. J., Mazlomi, M. A., Asadi-Ghalehni, M., et al. (2017). Construction of genetically engineered M13K07 helper phage for simultaneous phage display of gold binding peptide 1 and nuclear matrix protein 22 ScFv antibody. Colloids Surf. B Biointerfaces 159, 770–780. doi:10.1016/j.colsurfb.2017.08.034
Fathizadeh, H., Maroufi, P., Momen-Heravi, M., Dao, S., Köse, Ş., Ganbarov, K., et al. (2020). Protection and disinfection policies against SARS-CoV-2 (COVID-19). Inf. Med. 28, 185–191.
Fraise, A. P., Maillard, J. Y., and Sattar, S. (2013). Russell, hugo and ayliffe's principles and practice of disinfection, preservation and sterilization. 5th Edn. Oxford, United Kingdom: Wiley-Blackwell.
Fujishima, A., Rao, T. N., and Tryk, D. A. (2000). Titanium dioxide photocatalysis. J. Photoch. Photobio. C 1, 1–21. doi:10.1016/S1389-5567(00)00002-2
Fujishima, A., Zhang, X., and Tryk, D. A. (2008). TiO2 photocatalysis and related surface phenomena. Surf. Sci. Rep. 63, 515–582. doi:10.1016/j.surfrep.2008.10.001
Gao, G., Lange, D., Hilpert, K., Kindrachuk, J., Zou, Y., Cheng, J. T., et al. (2011a). The biocompatibility and biofilm resistance of implant coatings based on hydrophilic polymer brushes conjugated with antimicrobial peptides. Biomaterials 32, 3899–3909. doi:10.1016/j.biomaterials.2011.02.013
Gao, G., Yu, K., Kindrachuk, J., Brooks, D. E., Hancock, R. E., and Kizhakkedathu, J. N. (2011b). Antibacterial surfaces based on polymer brushes: investigation on the influence of brush properties on antimicrobial peptide immobilization and antimicrobial activity. Biomacromolecules 12, 3715–3727. doi:10.1021/bm2009697
Goffredo, G. B., Terlizzi, V., and Munafò, P. (2017). Multifunctional TiO2-based hybrid coatings on limestone: initial performances and durability over time. J. Build. Eng. 14, 134–149. doi:10.1016/j.jobe.2017.10.006
Golin, A. P., Choi, D., and Ghahary, A. (2020). Hand sanitizers: a review of ingredients, mechanisms of action, modes of delivery, and efficacy against coronaviruses. Am. J. Infect. Contr. 48, 1062–1067. doi:10.1016/j.ajic.2020.06.182
Gordon, O., Vig Slenters, T., Brunetto, P. S., Villaruz, A. E., Sturdevant, D. E., Otto, M., et al. (2010). Silver coordination polymers for prevention of implant infection: thiol interaction, impact on respiratory chain enzymes, and hydroxyl radical induction. Antimicrob. Agents Chemother. 54, 4208–4218. doi:10.1128/AAC.01830-09
Gómez-Ortíz, N., De la Rosa-García, S., González-Gómez, W., Soria-Castro, M., Quintana, P., Oskam, G., et al. (2013). Antifungal coatings based on Ca(OH)2 mixed with ZnO/TiO2 nanomaterials for protection of limestone monuments. ACS Appl. Mater. Interfaces 5, 1556–1565. doi:10.1021/am302783h
Hadjesfandiari, N., Yu, K., Mei, Y., and Kizhakkedathu, J. N. (2014). Polymer brush-based approaches for the development of infection-resistant surfaces. J. Mater. Chem. B 2, 4968–4978. doi:10.1039/c4tb00550c
Han, J., Chen, L., Duan, S. M., Yang, Q. X., Yang, M., Gao, C., et al. (2005). Efficient and quick inactivation of SARS coronavirus and other microbes exposed to the surfaces of some metal catalysts. Biomed. Environ. Sci. 18, 176–180.
Han, Q., Li, B., Zhou, X., Ge, Y., Wang, S., Li, M., et al. (2017). Anti-caries effects of dental adhesives containing quaternary ammonium methacrylates with different chain lengths. Materials 10, 643. doi:10.3390/ma10060643
He, W., Zhang, Y., Li, J., Gao, Y., Luo, F., Tan, H., et al. (2016). A novel surface structure consisting of contact-active antibacterial upper-layer and antifouling sub-layer derived from gemini quaternary ammonium salt polyurethanes. Sci. Rep. 6, 32140. doi:10.1038/srep32140
Heinonen, S., Nikkanen, J. P., Laakso, J., Raulio, M., Priha, O., and Levänen, E. (2013). Bacterial growth on a superhydrophobic surface containing silver nanoparticles. IOP Conf. Ser. Mater. Sci. Eng. 47, 012064. doi:10.1088/1757-899X/47/1/012064
Hosseinkhani, P., Zand, A., Imani, S., Rezayi, M., and Rezaei Zarchi, S. (2011). Determining the antibacterial effect of ZnO nanoparticle against the pathogenic bacterium, Shigella dysenteriae (type 1). Int. J. Nano Dimens. 1, 279–285. doi:10.7508/IJND.2010.04.006
Hua, Q., Shang, D., Zhang, W., Chen, K., Chang, S., Ma, Y., et al. (2011). Morphological evolution of Cu2O nanocrystals in an acid solution: stability of different crystal planes. Langmuir. 27, 665–671. doi:10.1021/la104475s
Iqbal, Q., Lubeck-Schricker, M., Wells, E., Wolfe, M. K., and Lantagne, D. (2016). Shelf-life of chlorine solutions recommended in ebola virus disease response. PloS One 11, e0156136. doi:10.1371/journal.pone.0156136
Jaggessar, A., Shahali, H., Mathew, A., and Yarlagadda, P. K. D. V. (2017). Bio-mimicking nano and micro-structured surface fabrication for antibacterial properties in medical implants. J. Nanobiotechnol. 15, 64. doi:10.1186/s12951-017-0306-1
Jahromi, R., Mogharab, V., Jahromi, H., and Avazpour, A. (2020). Synergistic effects of anionic surfactants on coronavirus (SARS-CoV-2) virucidal efficiency of sanitizing fluids to fight COVID-19. Food Chem. Toxicol. 145, 111702. doi:10.1016/j.fct.2020.111702
Jeremiah, S. S., Miyakawa, K., Morita, T., Yamaoka, Y., and Ryo, A. (2020). Potent antiviral effect of silver nanoparticles on SARS-CoV-2. Biochem. Biophys. Res. Commun. 533, 195–200. doi:10.1016/j.bbrc.2020.09.018
Joe, A., Park, H.S., Shim, K. D., Kim, D. J., Jhee, K. H., et al. (2017). Antibacterial mechanism of ZnO nanoparticles under dark conditions. J. Ind. Eng. Chem. 45, 430–439. doi:10.1016/j.jiec.2016.10.013
Junter, G. A., Thébault, P., and Lebrun, L. (2016). Polysaccharide-based antibiofilm surfaces. Acta Biomater. 30, 13–25. doi:10.1016/j.actbio.2015.11.010
Kampf, G., Dodt, D., Pfaender, S., and Steinmann, E. (2020). Potential role of inanimate surfaces for the spread of coronaviruses and their inactivation with disinfectant agents. Infect. Prev. Prac. 2, 100044. doi:10.1016/j.infpip.2020.100044
Kaneco, S., Rahman, M. A., Suzuki, T., Katsumata, H., and Ohta, K. (2004). Optimization of solar photocatalytic degradation conditions of bisphenol A in water using titanium dioxide. J. Photochem. Photobiol. 163, 419–424. doi:10.1016/j.jphotochem.2004.01.012
Kangab, U., and Park, H. (2017). A facile synthesis of CuFeO2 and CuO composite photocatalyst films for the production of liquid formate from CO2 and water over a month. J. Mater. Chem. 5, 2123–2131. doi:10.1039/c6ta09378g
Kawahara, K., Tsuruda, K., Morishita, M., and Uchida, M. (2000). Antibacterial effect of silver-zeolite on oral bacteria under anaerobic conditions. Dent. Mater. 16, 452–455. doi:10.1016/s0109-5641(00)00050-6
Keller, V., Bernhardt, P., and Garin, F. (2003). Photocatalytic oxidation of butyl acetate in vapor phase on TiO2, Pt/TiO2 andWO3/TiO2 catalysts. J. Catal. 215, 129–138. doi:10.1016/S0021-9517(03)00002-2
Khelissa, S. O., Abdallah, M., Jama, C., Faille, C., and Chihib, N.-E. (2017). Bacterial contamination and biofilm formation on abiotic surfaces and strategies to overcome their persistence. JMES 8, 3326–3346.
Kim, D. Y., Kim, M., Shinde, S., Sung, J. S., and Ghodake, G. (2017). Cytotoxicity and antibacterial assessment of gallic acid capped gold nanoparticles. Colloids Surf. B Biointerfaces 149, 162–167. doi:10.1016/j.colsurfb.2016.10.017
Knetsch, M. L. W., and Koole, L. H. (2011). New strategies in the development of antimicrobial coatings: the example of increasing usage of silver and silver nanoparticles. Polymers 3, 340–366. doi:10.3390/polym3010340
Król, A., Pomastowski, P., Rafińska, K., Railean-Plugaru, V., and Buszewski, B. (2017). Zinc oxide nanoparticles: synthesis, antiseptic activity and toxicity mechanism. Adv. Colloid Interfac. 249, 37–52. doi:10.1016/j.cis.2017.07.033
Lee, Y. W., Yoon, H. D., Park, J., and Ryu, U. (2018). Application of 265-nm UVC LED lighting to sterilization of typical gram negative and positive bacteria. J. Korean Phys. Soc. 72, 1174–1178. doi:10.3938/jkps.72.1174
Li, H., Williams, G. R., Wu, J., Lv, Y., Sun, X., Wu, H., et al. (2017). Thermosensitive nanofibers loaded with ciprofloxacin as antibacterial wound dressing materials. Int. J. Pharm. 517, 135–147. doi:10.1016/j.ijpharm.2016.12.008
Liu, J., Chamakura, K., Perez-Ballestero, R., and Bashir, S. (2012). “Historical overview of the first two waves of bactericidal agents and development of the third wave of potent disinfectants,” in Nanomaterials for Biomedicine. Editor R. Nagarajan (Washington, United States: Oxford University Press), 129–154.
Luo, J., Hein, C., Mücklich, F., and Solioz, M. (2017). Killing of bacteria by copper, cadmium, and silver surfaces reveals relevant physicochemical parameters. Biointerphases 12, 020301. doi:10.1116/1.4980127
Määttä, J., Piispanen, M., Kymäläinen, H. R., Uusi-Rauva, A., Hurme, K. R., Areva, S., et al. (2007). Effects of UV-radiation on the cleanability of titanium dioxide-coated glazed ceramic tiles. J. Eur. Ceram. Soc. 27, 4569–4574. doi:10.1016/j.jeurceramsoc.2007.03.026
Maillard, J., and McDonnell, G. (2012). Selection and use of disinfectants. In Pract. 34, 292–299. doi:10.1136/inp.e2741
Marambio-Jones, C., and Hoek, E. M. V. (2010). A review of the antibacterial effects of silver nanomaterials and their implications for human health and environment. J. Nanopart. Res. 12, 1531–1551. doi:10.1007/s11051-010-9900-y
Maruthupandy, M., Muneeswaran, T., Rajivgandhi, G., Quero, F., Anand, M., and Song, J. M. (2020). Biologically synthesized copper and zinc oxide nanoparticles for important biomolecules detection and antimicrobial applications. Mater. Today Commun. 22, 100766. doi:10.1016/j.mtcomm.2019.100766
McGuigan, K. G., Conroy, R. M., Mosler, H. J., du Preez, M., Ubomba-Jaswa, E., and Fernandez-Ibañez, P. (2012). Solar water disinfection (SODIS): a review from bench-top to roof-top. J. Hazard Mater. 235-236, 29–46. doi:10.1016/j.jhazmat.2012.07.053
Medical Plastics News (2020). The journey to developing a coating effective against Covid-19. Available at: https://www.medicalplasticsnews.com/mpn-north-america/the-journey-to-developing-a-coating-effective-against-covid-/ (Accessed October 22, 2020).
Mi, L., and Jiang, S. (2014). Integrated antimicrobial and nonfouling zwitterionic polymers. Angew Chem. Int. Ed. Engl. 53, 1746–1754. doi:10.1002/anie.201304060
Mills, A., and Hunte, S. L. (1997). An overview of semiconductor photocatalysis. J. Photochem. Photobiol. 108, 1–35. doi:10.1016/S1010-6030(97)00118-4
Munafò, P., Quagliarini, E., Goffredo, G. B., Bondioli, F., and Licciulli, A. (2014). Durability of nano-engineered TiO2 self-cleaning treatments on limestone. Construct. Build. Mater. 65, 218–231. doi:10.1016/j.conbuildmat.2014.04.112
Nosaka, Y., and Nosaka, A. Y. (2017). Generation and detection of reactive oxygen species in photocatalysis. Chem. Rev. 117, 11302–11336. doi:10.1021/acs.chemrev.7b00161
Parveen, K., Banse, V., and Ledwani, L. (2016). Green synthesis of nanoparticles: their advantages and disadvantages. AIP Conf. Proc. 1724, 1. doi:10.1063/1.4945168
Pejman, M., Firouzjaei, M. D., Aktij, S. A., Das, P., Zolghadr, E., Jafariang, H., et al. (2020). Improved antifouling and antibacterial properties of forward osmosis membranes through surface modification with zwitterions and silver-based metal organic frameworks. J. Membr. Sci. 611, 118352. doi:10.1016/j.memsci.2020.118352
Peng, W., Liu, P., Zhang, X., Peng, J., Gu, J., Dong, X., et al. (2020). Multi-functional zwitterionic coating for silicone-based biomedical devices. Chem. Eng. J. 398, 125663. doi:10.1016/j.cej.2020.125663
Prévot, M. S., Guijarro, N., and Sivula, K. (2015). Enhancing the performance of a robust sol–gel-processed p-type delafossite CuFeO2 photocathode for solar water reduction. Chem. Sus. Chem. 8, 1359–1367. doi:10.1002/cssc.201403146
Qiu, X., Liu, M., Sunada, K., Miyauchi, M., and Hashimoto, K. (2012a). A facile one-step hydrothermal synthesis of rhombohedral CuFeO2 crystals with antivirus property. Chem. Commun. 48, 7365–7367. doi:10.1039/c2cc33475e
Qiu, X., Miyauchi, M., Sunada, K., Minoshima, M., Liu, M., Lu, Y., et al. (2012b). Hybrid Cu(x)O/TiO₂ nanocomposites as risk-reduction materials in indoor environments. ACS Nano. 6, 1609–1618. doi:10.1021/nn2045888
Quagliarini, E., Bondioli, F., Goffredo, G. B., Cordoni, C., and Munafò, P. (2012). Self-cleaning and de-polluting stone surfaces: TiO2 nanoparticles for limestone. Construct. Build. Mater. 37, 51–57. doi:10.1016/j.conbuildmat.2012.07.006
Querido, M. M., Aguiar, L., Neves, P., Pereira, C., and Teixeira, J. P. (2019). Self-disinfecting surfaces and infection control. Colloids Surf. B. Biointerfaces 178, 8–21. doi:10.1016/j.colsurfb.2019.02.009
Raghupathi, K. R., Koodali, R. T., and Manna, A. C. (2011). Size-dependent bacterial growth inhibition and mechanism of antibacterial activity of zinc oxide nanoparticles. Langmuir 27, 4020–4028. doi:10.1021/la104825u
Rai, M., Deshmukh, S. D., Ingle, A. P., Gupta, I. R., Galdiero, M., and Galdiero, S. (2016). Metal nanoparticles: the protective nanoshield against virus infection. Crit. Rev. Microbiol. 42 (1), 46–56. doi:10.3109/1040841X.2013.879849
Rai, P. K., Usmani, Z., Thakur, V. K., Gupta, V. K., and MishraTackling, Y. K. (2020). Tackling COVID-19 pandemic through nanocoatings: confront and exactitude. Curr. Res. Green Sustain. Chem. 3, 100011. doi:10.1016/j.crgsc.2020.100011
Ramasamy, M., Lee, J. H., and Lee, J. (2017). Direct one-pot synthesis of cinnamaldehyde immobilized on gold nanoparticles and their antibiofilm properties. Colloids Surf. B. Biointerfaces 160, 639–648. doi:10.1016/j.colsurfb.2017.10.018
Reddy, S. T. (2014). Surface micropattern resists bacterial contamination transferred by healthcare practitioners. J. Microbiol. Exp. 1, 1–7. doi:10.15406/jmen.2014.01.00032
Requião, R. D., Carneiro, R. L., Moreira, M. H., Ribeiro-Alves, M., Rossetto, S., Palhano, F. L., et al. (2020). Viruses with different genome types adopt a similar strategy to pack nucleic acids based on positively charged protein domains. Sci. Rep. 10, 5470. doi:10.1038/s41598-020-62328-w
Robertson, P. K., Robertson, J. M., and Bahnemann, D. W. (2012). Removal of microorganisms and their chemical metabolites from water using semiconductor photocatalysis. J. Hazard Mater. 211-212, 161–171. doi:10.1016/j.jhazmat.2011.11.058
Roselli, M., Finamore, A., Garaguso, I., Britti, M. S., and Mengheri, E. (2003). Zinc oxide protects cultured enterocytes from the damage induced by Escherichia coli. J. Nutr. 133, 4077–4082. doi:10.1093/jn/133.12.4077
Rutala, W. A., and Weber, D. J. (2004). Disinfection and sterilization in health care facilities: what clinicians need to know. Clin. Infect. Dis. 39, 702–709. doi:10.1086/423182
Saif, M., Hafez, H., and Nabeel, A. I. (2013). Photo-induced self-cleaning and sterilizing activity of Sm3+ doped ZnO nanomaterials. Chemosphere 90, 840–847. doi:10.1016/j.chemosphere.2012.09.095
Saraf, R. (2013). Cost effective and monodispersed zinc oxide nanoparticles synthesis and their characterization. Int. J. Adv. Appl. Sci. 2, 85–88. doi:10.11591/ijaas.v2i2.1614
Shao, W., Liu, H., Liu, X., Wang, S., Wu, J., Zhang, R., et al. (2015). Development of silver sulfadiazine loaded bacterial cellulose/sodium alginate composite films with enhanced antibacterial property. Carbohydr. Polym. 132, 351–358. doi:10.1016/j.carbpol.2015.06.057
Sifri, C. D., Burke, G. H., and Enfield, K. B. (2016). Reduced health care-associated infections in an acute care community hospital using a combination of self-disinfecting copper-impregnated composite hard surfaces and linens. Am. J. Infect. Contr. 44, 1565–1571. doi:10.1016/j.ajic.2016.07.007
Sigstam, T., Gannon, G., Cascella, M., Pecson, B. M., Wigginton, K. R., and Kohn, T. (2013). Subtle differences in virus composition affect disinfection kinetics and mechanisms. Appl. Environ. Microbiol. 79, 3455–3467. doi:10.1128/AEM.00663-13
Silhavy, T. J., Kahne, D., and Walker, S. (2010). The bacterial cell envelope. Cold Spring Harb. Perspect. Biol. 2, a000414. doi:10.1101/cshperspect.a000414
Sivula, K., and van de Krol, R. (2016). Semiconducting materials for photoelectrochemical energy conversion. Nat. Rev. Mater. 1, 15010. doi:10.1038/natrevmats.2015.10
Songur, A., Ozen, O. A., and Sarsilmaz, M. (2010). The toxic effects of formaldehyde on the nervous system. Rev. Environ. Contam. Toxicol. 203, 105–118. doi:10.1007/978-1-4419-1352-4_3
Souli, M., Antoniadou, A., Katsarolis, I., Mavrou, I., Paramythiotou, E., Papadomichelakis, E., et al. (2017). Reduction of environmental contamination with multidrug-resistant bacteria by copper-alloy coating of surfaces in a highly endemic setting. Infect. Control Hosp. Epidemiol. 38, 765–771. doi:10.1017/ice.2017.52
Sportelli, M. C., Izzi, M., Kukushkina, E. A., Hossain, S., Picca, R. A., Ditaranto, N., et al. (2020). Can nanotechnology and materials science help the fight against SARS-CoV-2? Nanomaterials 10, 802–814. doi:10.3390/nano10040802
StatNano (2020). Nanotechnology in battle against coronavirus. Available at: https://statnano.com/nanotechnology-in-battle-against-coronavirus.
Su, S. S., and Chang, I. (2018). “Review of production routes of nanomaterials,” in Commercialization of nanotechnologies–a case study approach. Editors D. Brabazon, E. Pellicer, F. Zivic, J. Sort, M. D. Baró, N. Grujovicet al. (Geneva, Switzerland: Springer), 15–29.
Sullivan, T., and Regan, F. (2011). The characterization, replication and testing of dermal denticles of Scyliorhinus canicula for physical mechanisms of biofouling prevention. Bioinspiration Biomimetics 6, 046001. doi:10.1088/1748-3182/6/4/046001
Sunada, K., Watanabe, T., and Hashimoto, K. (2003). Bactericidal activity of copper-deposited TiO2 thin film under weak UV light illumination. Environ. Sci. Technol. 37, 4785–4789. doi:10.1021/es034106g
Tang, S. H., Venault, A., Hsieh, C., Dizon, G. V., Lo, C. T., and Chang, Y. (2020). A bio-inert and thermostable zwitterionic copolymer for the surface modification of PVDF membranes. J. Membr. Sci. 598, 117655. doi:10.1016/j.memsci.2019.117655
Tatsuma, T., Saitoh, S., Ngaotrakanwiwat, P., Ohko, Y., and Fujishima, A. (2002). Energy storage of TiO2-WO3 photocatalysis systems in the gas phase. Langmuir 18, 7777–7779. doi:10.1021/la026011i
Tatsuma, T., Saitoh, S., Ohko, Y., and Fujishima, A. (2001). TiO2-WO3 photoelectrochemical anticorrosion system with an energy storage ability. Chem. Materials 13, 2838–2842. doi:10.1021/cm010024k
Tatsuma, T., Takeda, S., Saitoh, S., Ohko, Y., and Fujishima, A. (2003). Bactericidal effect of an energy storage TiO2–WO3 photocatalyst in dark. Electrochem. Commun. 5, 793–796. doi:10.1016/j.elecom.2003.07.003
Tettey, C., Nagajyothi, P., Lee, S., Ocloo, A., Minh An, T., Sreekanth, T. V., et al. (2012). Anti-melanoma, tyrosinase inhibitory and anti-microbial activities of gold nanoparticles synthesized from aqueous leaf extracts of Teraxacum officinale. Int. J. Cosmet. Sci. 34, 150–154. doi:10.1111/j.1468-2494.2011.00694.x
Tiwari, P. M., Vig, K., Dennis, V. A., and Sing, S. R. (2011). Functionalized gold nanoparticles and their biomedical applications. Nanomaterials. 1, 31–63. doi:10.3390/nano101003
Tremiliosi, G. C., Simoes, L. G. P., Minozzi, D. T., Santos, R. I., Vilela, D. C. B., Durigon, E. L., et al. (2020). Ag nanoparticles-based antimicrobial polycotton fabrics to prevent the transmission and spread of SARS-CoV-2. BioRxiv [Preprint]. Available at https://europepmc.org/article/ppr/ppr180897 (Accessed October 23, 2020).
Usman, M. S., El Zowalaty, M. E., Shameli, K., Zainuddin, N., Salama, M., and Ibrahim, N. A. (2013). Synthesis, characterization, and antimicrobial properties of copper nanoparticles. Int. J. Nanomed. 8, 4467–4479. doi:10.2147/IJN.S50837
Veltri, S., Palerma, A. M., De Filpo, G., and Xu, F. (2019). Subsurface treatment of TiO2 nanoparticles for limestone: prolonged surface photocatalytic biocidal activities. Build. Environ. 149, 655–661. doi:10.1016/j.buildenv.2018.10.038
Wakerley, D. W., Kuehnel, M. F., Orchard, K. L., Ly, K. H., Rosser, T. E., and Reisner, E. (2017). Solar-driven reforming of lignocellulose to H2 a CdS/CdOx photocatalyst. Nat. Energy 2, 17021. doi:10.1038/nenergy.2017.21
Wang, J., Shen, J., Ye, D., Yan, X., Zhang, Y., Yang, W., et al. (2020). Disinfection technology of hospital wastes and wastewater: suggestions for disinfection strategy during coronavirus Disease 2019 (COVID-19) pandemic in China. Environ. Pollut. 262, 114665. doi:10.1016/j.envpol.2020.114665
Wang, W., Yu, Y., An, T., Li, G., Yip, H. Y., Yu, J. C., et al. (2012). Visible-light-driven photocatalytic inactivation of E. coli K-12 by bismuth vanadate nanotubes: bactericidal performance and mechanism. Environ. Sci. Technol. 46, 4599–4606. doi:10.1021/es2042977
Watson, G. S., Green, D. W., Cribb, B. W., Brown, C. L., Meritt, C. R., Tobin, M. J., et al. (2017). Insect analogue to the lotus leaf: a planthopper wing membrane incorporating a low-adhesion, nonwetting, superhydrophobic, bactericidal, and biocompatible surface. ACS Appl. Mater. Interfaces 9, 24381–24392. doi:10.1021/acsami.7b08368
Wei, L., Lu, J., Xu, H., Patel, A., Chen, Z. S., and Chen, G. (2015). Silver nanoparticles: synthesis, properties, and therapeutic applications. Drug Discov. Today 20, 595–601. doi:10.1016/j.drudis.2014.11.014
West, A. M., Teska, P. J., Lineback, C. B., and Oliver, H. F. (2018). Strain, disinfectant, concentration, and contact time quantitatively impact disinfectant efficacy. Antimicrob. Resist. Infect. Contr. 7, 49. doi:10.1186/s13756-018-0340-2
Wieczorek, D., Dobrowolski, A., Staszak, K., Kwaśniewska, D., and Dubyk, P. (2017). Synthesis, surface and antimicrobial activity of piperidine-based sulfobetaines. J. Surfactants Deterg. 20, 151–158. doi:10.1007/s11743-016-1906-8
Wijesena, R. N., Tissera, N. D., Perera, R., Nalin de Silva, K. M., and Amaratunga, G. A. J. (2015). Slightly carbomethylated cotton supported TiO2 nanoparticles as self-cleaning fabrics. J. Mol. Catal. Chem. 398, 107–114. doi:10.1016/j.molcata.2014.11.012
World Health Organization (2014). “Annex G Use of disinfectants: alcohol and bleach”, in Infection prevention and control of epidemic and pandemic prone acute respiratory infections in health care. Geneva, Switzerland: WHO Press, 65–66.
Wu, C., Liu, Y., Yang, Y., Zhang, P., Zhong, W., Wang, Y., et al. (2020). Analysis of therapeutic targets for SARS-CoV-2 and discovery of potential drugs by computational methods. Acta Pharm. Sin. B. 10, 766–788. doi:10.1016/j.apsb.2020.02.008
Wu, K., Yang, Y., Zhang, Y., Deng, J., and Lin, C. (2015). Antimicrobial activity and cytocompatibility of silver nanoparticles coated catheters via a biomimetic surface functionalization strategy. Int. J. Nanomed. 10, 7241–7252. doi:10.2147/IJN.S92307
Xiong, D., Qi, Y., Li, X., Liu, X., Tao, H., Chen, W., et al. (2015). Hydrothermal synthesis of delafossite CuFeO2 crystals at 100°C. RSC Adv. 5, 49280–49286. doi:10.1039/C5RA08227G
Xu, Q., Li, R., Wang, C., and Yuan, D. (2017). Visible-light photocatalytic reduction of Cr(VI) using nano-sized delafossite (CuFeO2) synthesized by hydrothermal method. J. Alloys Compd. 723, 441–447. doi:10.1016/j.jallcom.2017.06.243
Xu, Y., and Schoonen, M. A. A. (2000). The absolute energy positions of conduction and valence bands of selected semiconducting minerals. Am. Mineral. 85, 543–556. doi:10.2138/am-2000-0416
Yamamoto, M., Nishikawa, N., Mayama, H., Nonomura, Y., Yokojima, S., Nakamura, S., et al. (2015). Theoretical explanation of the lotus effect: superhydrophobic property changes by removal of nanostructures from the surface of a lotus leaf. Langmuir 31, 7355–7363. doi:10.1021/acs.langmuir.5b00670
Yee, M. S. L., Khiew, P. S., Tan, Y. F., Chiu, W. S., and Leong, C. O. (2015). Low temperature, rapid solution growth of antifouling silver-zeolite nanocomposite clusters. Microporous Mesoporous Mater. 218, 69–78. doi:10.1016/j.micromeso.2015.07.004
Zhang, L., Jiang, Y., Ding, Y., Povey, M., and York, D. (2007). Investigation into the antibacterial behaviour of suspensions of ZnO nanoparticles (ZnO nanofluids). J. Nanopart. Res. 9, 479–489. doi:10.1007/s11051-006-9150-1
Zhang, X., Ding, Y., Tang, H., Han, X., Zhu, L., and Wang, N. (2014). Degradation of bisphenol A by hydrogen peroxide activated with CuFeO2 microparticles as a heterogeneous fenton-like catalyst: efficiency, stability and mechanism. Chem. Eng. J. 236, 251–262. doi:10.1016/j.cej.2013.09.051
Zhou, Q., Ma, S., and Zhan, S. (2018). Superior photocatalytic disinfection effect of Ag-3D ordered mesoporous CeO2 under visible light. Appl. Catal. B. Environ. 224, 27–37. doi:10.1016/j.apcatb.2017.10.032
Keywords: disinfection, chemical biocides, metal-nanoparticles, anti-adhesive surfaces, self-cleaning
Citation: Ghedini E, Pizzolato M, Longo L, Menegazzo F, Zanardo D and Signoretto M (2021) Which Are the Main Surface Disinfection Approaches at the Time of SARS-CoV-2?. Front. Chem. Eng. 2:589202. doi: 10.3389/fceng.2020.589202
Received: 20 August 2020; Accepted: 27 November 2020;
Published: 10 February 2021.
Edited by:
Fengqi You, Cornell University, United StatesReviewed by:
Lai Lyu, Guangzhou University, ChinaPaolo S. Calabrò, Mediterranea University of Reggio Calabria, Italy
Farooq Sher, Coventry University, United Kingdom
Haitao Wang, Nankai University, China
Copyright © 2021 Ghedini, Pizzolato, Longo, Menegazzo, Zanardo and Signoretto. This is an open-access article distributed under the terms of the Creative Commons Attribution License (CC BY). The use, distribution or reproduction in other forums is permitted, provided the original author(s) and the copyright owner(s) are credited and that the original publication in this journal is cited, in accordance with accepted academic practice. No use, distribution or reproduction is permitted which does not comply with these terms.
*Correspondence: Michela Signoretto, bWlreUB1bml2ZS5pdA==