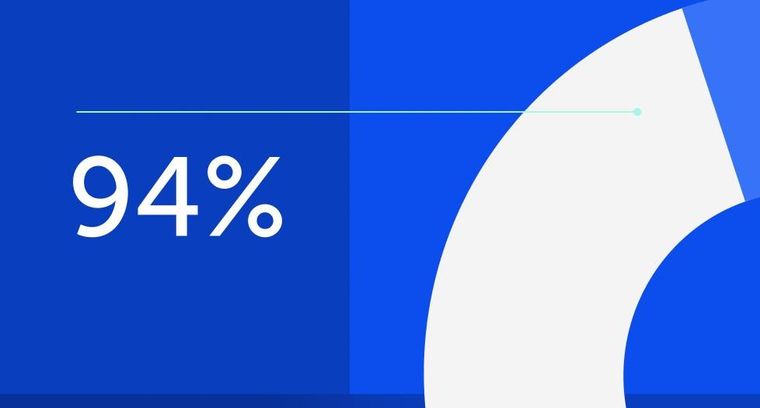
94% of researchers rate our articles as excellent or good
Learn more about the work of our research integrity team to safeguard the quality of each article we publish.
Find out more
MINI REVIEW article
Front. Chem. Biol., 28 February 2025
Sec. Molecular Sciences
Volume 4 - 2025 | https://doi.org/10.3389/fchbi.2025.1549003
This article is part of the Research TopicSTING Signaling in Cancer ImmunotherapyView all 4 articles
Since the discovery of Stimulator of Interferon Genes (STING) as a key element in recognizing cytosolic DNA and initiating interferon (IFN) production, substantial research has been conducted to comprehend the precise molecular process of its activation for the treatment of tumor and immune-related diseases. However, new research has enhanced our understanding of STING biology and shown additional major functions of STING that go beyond its capacity to stimulate (Interferon-Stimulated Genes)(ISGs). This mini-review article highlights important details of the established STING biology, including TBK1-IRF3 activation, the NF-κB and MAPK pathway, endoplasmic reticulum (ER) stress and autophagy, and lysosome-induced degradation. We also provide an overview of the independent functions of cGAS and STING, unresolved questions that need to be addressed, and directions for future research.
Cancer is commonly described as a chronic condition that does not heal. The concept was first suggested by Rudolph Virchow and highlights the parallels between the progression of cancer and inflammation (Byun and Gardner, 2013). In the typical physiological response to injury, various mechanisms are in place to ensure the prompt resolution of wound-healing processes. However, the growing body of data indicates that chronic inflammation plays a substantial role in the formation of malignancies (Morrison, 2012).
The identification and protection against external genetic material are essential and fundamental functions of biological systems. The STING pathway is a crucial mediator of inflammation, connecting the recognition of cytosolic DNA to the synthesis of type I interferons and pro-inflammatory cytokines. Effective control of this system is essential for sustaining immunological homeostasis and avoiding pathological inflammation (Wang et al., 2023). Type I interferons are a recent addition in vertebrates, whereas cGAS-STING and related systems are ancient and can be observed in primitive eukaryotes and even prokaryotes (Ou et al., 2021). The interaction of cGAS with chromosomal DNA in the nucleus raises significant questions regarding its level of activation, its control, and its ability to distinguish between chromatin and infection-related DNA indicating the finely tuned regulation of this route. Understanding these pathways is crucial for developing therapeutics aimed at cGAS-associated autoimmune disorders and cancer.
A sequence-independent interaction with double-stranded DNA (dsDNA) mediates the activation of cGAS. The DNA ligands bind to cGAS in a 2:2 complex, inducing structural changes that allow cGAS to catalyze the conversion of ATP and GTP into 2′,3′-cyclic GMP-AMP (cGAMP) (Blest and Chauveau, 2023). Significantly, DNA with a greater length exhibits a higher capacity to activate cGAS and promote the formation of liquid-like droplets (Wang et al., 2024). The droplets act as a concentrated environment for cGAS and dsDNA, which improves the efficiency of cGAMP synthesis. Afterwards, STING activation at the endoplasmic reticulum (ER) is stimulated by the second messenger, cGAMP (Ou et al., 2021). This results in the creation of tetramers by a process known as higher-orderoligomerization. The recruitment of TANK binding kinase 1 (TBK1) and interferon regulatory factor 3 (IRF3) has been proposed as a consequence of palmitoylation of STING at the Golgi (King et al., 2017). TBK1 phosphorylates the C-terminal regions of STING in order to recruit IRF3 for activation. Upon activation, IRF3 translocates to the nucleus and performs its function in transcribing ISG and type 1 IFNs (Lin et al., 2023). In addition, STING concurrently activates IκB kinase (IKK), facilitating the start of nuclear factor kappa b (NF-κB)-driven inflammatory gene expression (Chen and Xu, 2023). STING is transported to endo-lysosomes for destruction upon activation.
Unlike normal cells, cancer cells usually contain a large amount of cytosolic double-stranded DNA (Talbot et al., 2024). DNA can be derived from a variety of sources, including exogenous, mitochondrial, and genetic sources. The precise effects of mislocated cytosolic double-stranded DNA (dsDNA) on cancer are not yet fully understood. Research suggests that, depending on the specific conditions and stage of tumor growth, it may have both tumor-inhibiting and tumor-promoting effects (Wang et al., 2024). In the early phases of transformation, cytosolic DNA is likely to initiate immune surveillance as well as intrinsic senescence of cancer cells. Nevertheless, the lack of crucial regulators of the cell cycle and immunological checkpoints may enable cytosolic dsDNA to initiate persistent inflammatory signals associated with cell survival and the metastasis of cancer.
Recent research suggests that chromosomal instability (CIN) has a significant role in the occurrence of cytosolic double-stranded DNA (dsDNA) (Targa and Rancati, 2018). Cancer cells that have unstable genomes are prone to experiencing errors in the distribution of chromosomes during the process of cell division known as mitosis (Targa and Rancati, 2018). One consequence of these segregation issues is the formation of micronuclei, which act as a repository of genetic material originating from the nucleus. The activation of cGAS-STING in cancer cells is triggered by cytosolic DNA that is produced by similar processes in response to high genomic stressors induced by radiation, cisplatin, and intrinsic DNA damage.
Although bound to cGAS, not all DNAs have the ability to activate cGAS. This is particularly true for the vast majority of short DNA segments. Recent research suggests that the bonding of cGAS molecules by short DNAs is not very strong. Active cGAS-DNA complexes are fully activated and stabilized by forming oligomeric structures or condensates. DNA greatly enhances this process after it reaches a certain length threshold, allowing two or more cGAS molecules to attach to the same DNA. Regarding cGAS, it was observed that its activation resulted in the creation of condensates driven by liquid-liquid phase separation (Serpico et al., 2023). Proteins and nucleic acids are highly concentrated within the condensate, while they are found in low quantities elsewhere. Oligomers and condensates play crucial roles in establishing substrate selectivity and enabling a prompt response to infections within the framework of cGAS and STING. This is due to their preference for the existence of long and easily accessible DNA (Luecke et al., 2017; Andreeva et al., 2017), unhindered by nucleosomes, hence facilitating the creation of substantial assemblies.
The activation of cGAS, which depends on the length of DNA, can be elucidated by the recent revelation that cGAS dimers assemble into bigger structures known as oligomers (Luecke et al., 2017). These oligomers possess distinct physicochemical characteristics that resemble liquid droplets and potentially have a significant impact on the regulation of cGAS activation. The structural investigation of cGAS bound to 39-bp DNA provided the initial insight into the formation of oligomeric assemblies by cGAS. The studies demonstrated that two cGAS dimers can form a ladder-like structure (Andreeva et al., 2017), mimicking steps along the two DNA arms (Figure 1). This phase change from liquid to solid depends on the amounts of DNA and cGAS present, which suggests that cGAS must be activated above a particular minimum DNA content threshold. In this configuration, the neighboring cGAS dimers can enhance each other’s presence along the DNA through multivalent interactions. It has been proposed that this could be a way to stabilize cGAS by encouraging cooperative effects, leading to higher levels of cGAS in the nearby region. Subsequently, it was proven in cellular experiments that the catalytic domain and the N-terminal domain of cGAS form multiple contacts, leading to the formation of condensed structures with liquid-like properties (Wang et al., 2022).
Figure 1. Liquid phase separation in cGAS activation. Effective cGAS involves the attachment of DNA that exceeds specific length and quantity thresholds, enabling cGAS to undergo active conformational alterations, dimerization, oligomerization, and ultimately phase separation. The phase separation of DNA-induced cGAS is significantly influenced by the quantity of cGAS and the cellular DNA contents. Allosteric or cooperative stimulation specifically induces CGAS clustering and liquid phase separation. The assembly of cGAS dimers occurs through the interaction of cGAS with non-agonistic short DNA; whereas nucleosomes can associate with cGAS, they do not activate it; the exact mechanism of binding between cGAS and chromatin remains unclear. Prolonged DNA facilitates cluster oligomerization and various DNA arrangement techniques, resulting in a ladder-like structure. The cGAS dimers are organized along the DNA, whereby the DNA engages with the charged plaques situated on the N-terminus of cGAS and the DNA network. The DNA-bending proteins alter the DNA, leading to a more active and durable dimer configuration, which promotes the development of substantial liquid-liquid phase separation condenses. In these condensates, cGAS is significantly concentrated and sustained for its catalytic functions.
The identification and defense systems within cells against foreign genetic material are an ancient and fundamental characteristic of living systems. The innate immune system serves as the initial line of defense in mammals, interaction with cGAMP activates STING, which then translocates to the Golgi and activates TANK-binding kinase 1 (TBK1) (Fang et al., 2021). Localization takes place in the nucleus as well as at the cell membrane. The interaction between cGAS and chromosomal DNA in the nucleus gives rise to significant issues regarding its level of activation, regulation, and its capacity to distinguish between chromatin and infection-related DNA. It has been demonstrated that oligomeric structures are produced with both the activation of STING and cGAS. In the following sections, we provide a concise overview of four distinct roles of STING (Figure 2): activation of IRF3, activation of NF-κB and MAPK, regulation of autophagy, and induction of lysosomal cell death (LCD) through STING-mediated lysosomal trafficking.
Figure 2. Molecular Mechanisms of the cGAS-STING pathways. cGAS can detect DNA from many cytoplasmic origins and facilitate the synthesis of cGAMP, which then attaches to and triggers the adaptor STING in the endoplasmic reticulum, or ER membrane. cGAS can detect pathogenic microbes, defective mitochondria, and micronuclei as DNA resources. cGAMP can activate STING in neighboring cells by utilizing cGAMP transporters or gap junctions present on the plasma membrane. Upon exiting the endoplasmic reticulum, STING oligomers penetrate the endoplasmic reticulum-Golgi intermediate compartment (ERGIC). a The STING oligomer engages with the enzyme known as TANK binding kinase 1 (TBK1). Active TBK1 phosphorylates Ser366 in the C-terminal tail (CTT) of STING, thus improving its attachment of interferon regulatory factor 3 (IRF3). b Activation of TBK1 can also influence the activation of NF-κB. In conjunction with IRF3, NF-κB translocates to the nucleus and stimulates an increase in type I interferon and various other pro-inflammatory genes. c In the ERGIC, STING can facilitate the onset of autophagy, which has been shown to be an inherent function of cGAS-STING in relation to TBK1 activation. Mechanically, ERGIC containing STING acts as the outer membrane origin for autophagosome biogenesis, while cGAMP facilitates the formation of the central autophagosome molecule LC3. It is crucial to acknowledge that STING-induced autophagy is unrelated to its CTT or TBK1 action, and the precise mechanism remains unidentified. d STING can be transported to the lysosome through late endosomes, resulting in the buildup of STING that causes lysosomal membrane permeabilization. This kind of cell death, characterized by rupture, is termed lysosomal-dependent cell death and incites a subsequent robust inflammatory response.
STING is activated through the binding of cGAMP, which triggers signal transduction (Blest and Chauveau, 2023). This pathway is mostly recognized for its capacity to trigger phosphorylation of IRF3, leading to the synthesis of several antiviral genes. TKB1 phosphorylates itself, STING, and subsequently the interferon regulatory factor 3 (IRF3) transcription factor (King et al., 2017). The activation of IRF3, which has been intensively investigated, is in fact the most recent evolutionary advancement in the downstream signaling of STING. In addition, STING carries out other functions that are more ancient in terms of evolution and can be differentiated from IRF3 activation based on their processes and roles.
Activation of the STING pathway in both humans and mice leads to in activation of the NF-κB and MAPK (Zhou et al., 2023) signaling pathways. The mammalian STING protein utilizes an atypical signaling pathway to stimulate the activation of transcription factors, including MAPK and NF-κB. STING employs an alternative method, instead of forming TRAF-dependent signalosomes to attract TAK and IKK complexes. The mechanisms behind the activation of STING-dependent NF-κB and MAPK are not yet fully comprehended, and there are opposing hypotheses proposed. Research has shown that TBK1 plays a role in the activation of NF-κB (Yum et al., 2021). Nevertheless, there is compelling evidence indicating that the C-terminal tail (CTT) of STING is unnecessary for this particular activity, hence eliminating the role of TBK1 as well (Dorta-Estremera et al., 2019). Studies have demonstrated that STING can induce NF-κB activation in the process of DNA damage signaling (Zhang et al., 2023), independent of cGAS. It is possible that STING-dependent NF-κB activation is regulated by a signal originating from the ligand-binding domain of oligomerized STING. Notably, ray-finned fish have developed a C-terminal extension of the STING CTT, which functions to recruit TRAF6. This supplementary module leads to significantly enhanced NF-κB activation relative to mammalian STING variants. Ultimately, it has been noted that STING can induce NF-κB activation downstream of DNA damage signaling, independent of cGAS. This response was demonstrated to form an atypical STING signaling complex, leading to TRAF6-dependent NF-κB activation. The mechanism by which STING activates the MAPK pathway remains to be clarified (Lin et al., 2024).
Following departure from the endoplasmic reticulum (ER), STING triggers autophagy from the ER-Golgi intermediate compartment (ERGIC) (Zhang et al., 2021). This phenomenon can be discerned by examining the process of lipidation of LC3 (Gui et al., 2019), also referred to as microtubule-associated proteins 1A/1B light chain 3B, and the subsequent formation of autophagosomes. Autophagy, which functions independently of its CTT and does not require TBK1 and IRF3 activation, is one important outcome of STING-dependent signaling. Concurrently, the inhibition of IKK activity does not impede autophagy. The autophagy process initiates with the activation of the ULK1 complex, which then phosphorylates components of the PI3KC3 complex I, leading to their activation. The complex then triggers the local synthesis of phosphatidylinositol 3-phosphate (PtdIns3P) molecules, leading to the formation of the phagophore. The residues serve as binding sites for PtdIns3P effector proteins, which then recruit and activate the ubiquitin-like conjugation machinery that is responsible for the formation and functionalization of autophagic membranes. Within the framework of STING, the ULK1 and PI3KC3 complexes, which are components of the standard autophagy initiation mechanism, do not contribute to STING-dependent autophagy. Nevertheless, the downstream components, including the PtdIns3P effector protein WIPI2 and ATG5 (Yamazaki et al., 2021), are common to both pathways. Autophagy has been found in STING from the sea anemone, despite the absence of the CTT. These findings indicate that autophagy may be a fundamental and primordial role of STING. Additionally, by enabling cancer cells to thrive and survive in harsh conditions, the activation of the ER stress response and autophagy pathways can promote the growth of advanced malignancies. Endoplasmic reticulum (ER) stress response has been shown to be a mechanism by which cancer cells use their latent and immune-evading capacities to spread. The disturbance of ER homeostasis has the potential to contribute to the advancement of metastasis.
The tumor microenvironment can exploit these metabolic pathways to facilitate immune suppression, in addition to the fundamental importance of ER stress in fostering malignancy inside tumor cells. The tumor microenvironment was proposed to impede the effective uptake of nutrients, such as glucose, by T cells within the tumor. As a consequence, the occurrence of endoplasmic reticulum (ER) stress leads to the subsequent activation of the IRE1α–XBP1 unfolded protein response (UPR) pathway. Continual activation of the unfolded protein response (UPR) induces a reprogramming of T cells (Wu et al., 2019), resulting in the acquisition of a dysfunctional state marked by diminished antitumor functions.
STING activation can trigger cell death through several mechanisms. Thus, apart from its capacity to stimulate antiviral genes via IRF3, STING also incites the synthesis of several substances that facilitate the pathways leading to programmed cell death, such as necroptosis and apoptosis. Activation of STING in some cellular types can also initiate lysosome-induced cell death (LCD), which is a specific type of cell death observed in human myeloid cells. The relocation of STING to the lysosome was found to be essential for its proper functioning. STING is carried to the lysosomes, triggering the lysosomal membrane to become permeable and leading to the release of lysosomal hydrolases, ultimately culminating in the death of the cell. However, the STING-induced LCD and subsequent extensive inflammation act as the final mechanism that prevents the proliferation of microorganisms. Nevertheless, it is critical to investigate conditions where autophagy and LCD may be distinguished in order to test this theory. STING’s activity duration is mostly controlled by the lysosomal system, rather than by proteasomal degradation or autophagy. The process of lysosomal degradation of STING leads to the termination of its signaling. Imaging studies demonstrate that STING translocates to the lysosome following its departure from the Golgi via the late endosome pathway (Gentili et al., 2023). Nevertheless, the specific elements that govern this transit process remain unidentified. STING translocation to the lysosome during ER export occurs independent of the phosphorylation of TBK1 (Hancock-Cerutti et al., 2022). It is hypothesized that there are two conserved regions in the LBDα3 that govern the transport of STING to the lysosome. Nevertheless, additional research is required to figure out the precise mechanism involved.
The vital function of the cGAS-STING pathway in stimulating both innate and adaptive immune responses highlights the therapeutic significance of DNA-damaging therapies. Emerging evidence indicates that medical treatments such as radiation, PARP inhibitors (PARPi), and etoposide, promote the production of cytosolic DNA and trigger the activation of STING-dependent IFN, hence boosting the body’s immune response against tumors. For example, radiation therapy has been linked to the inhibition of tumor growth via the cGAS-STING pathway. Furthermore, research has shown that blocking PARP triggers the cGAS-STING signaling pathway in both cancerous cells and immune cells, resulting in increased eradication of tumors, intensified proinflammatory signaling, and higher infiltration of immune cells. It is crucial to recognize that different types of DNA damage triggers might initiate various pathways to activate STING signaling. Contrary to the effects of PARP inhibition and radiation, the presence of cytosolic DNA resulting from etoposide can trigger the release of IFNs via the STING pathway, without requiring cGAS. These findings collectively provide evidence that cancers with inherent deficits in DNA repair mechanisms are vulnerable to exposure of DNA in the cytoplasm. Subsequently, this contact can activate an immunological reaction through the cGAS-STING-IRF3 pathway. The association between DNA damage and cGAS-STING activation offers an interesting opportunity to employ integrated strategies in order to target these pathways and bolster the immune system’s capacity to eradicate cancers.
Nevertheless, even in the therapeutic setting, persistent activation of the cGAS-STING pathway could lead to resistance (Raghava Kurup et al., 2022). Prolonged radiation exposure triggers the activation of interferon (IFN) through the action of STING. This, in turn, causes the recruitment of myeloid-derived suppressor cells to MC38 adenocarcinoma cells, ultimately leading to the creation of an immunosuppressive environment (Hines et al., 2023).
The development of pharmacological agents that activate STING has been prompted by the impact of the cGAS-STING signaling pathway on altering anticancer effects. Based on the discovery that human STING is stimulated by 2′3′-cGAMP, which results in robust downstream IFN signaling, the majority of chemicals that activate STING are synthetic derivatives of 2′3′-cGAMP. These analogues have undergone chemical modifications to increase their effectiveness in fighting tumors by making them resistant to hydrolysis. Pharmacologically stimulating STING has been shown to restrict tumor growth and enhance immunogenicity in different mouse models of cancer. When cGAMP is injected directly into a tumor in mice models of colorectal cancer and melanoma with an active immune system (Ge et al., 2023), it triggers the STING pathway, which causes dendritic cells (DCs) to produce interferon (IFN) (Yu et al., 2022). Subsequently, these dendritic cells present tumor-specific antigens on major histocompatibility complexes, thereby stimulating CD8+ T lymphocytes to eliminate the tumor (Li et al., 2020). In some cancer types, such as B-cell malignancies, STING can be utilized as a possible therapeutic target. This is because apoptosis induction by STING agonists has been shown to promote the activation of antitumor T lymphocytes by releasing tumor antigens.
Pharmacologic intervention in immunotherapy has made significant progress by targeting STING, resulting in the development of human STING agonists for clinical trials. Since distinct forms of the human STING gene respond differently to 3′–5′ phosphodiester-linked cGAMP, precise design of these analogues is essential to attaining therapeutic efficacy. STING agonists (ADU-S100 and MK-1454) are currently being injected directly into solid human tumors and lymphomas in two phase I clinical trials. The escalation in dosage was well endured, and there was indication of infiltration by CD8+ immune cells at the tumor sites that were injected, even in patients who had previously had checkpoint inhibitors. However, preliminary results indicate that the treatment alone had a limited clinical response and did not demonstrate significant success. Therefore, the combination of STING agonists with immune checkpoint inhibitors is a viable approach for activating both the innate and adaptive immune systems to combat cancer. This method has the ability to surmount resistance, enhance efficacy, and target non-immunogenic malignancies. Several preclinical research and clinical trials have validated this proof of concept. In murine models, the combination of STING agonists with immune checkpoint inhibitors (anti-PD-1 or anti-CTLA-4 treatments) has demonstrated enhanced tumor remission and prolonged survival relative to monotherapy (de Oliveira et al., 2019). Numerous clinical trials, such as MK-1454 combined with Pembrolizumab and ADU-S100 combined with Spartalizumab, are evaluating STING agonists with immune checkpoint inhibitors to augment immune responses in refractory malignancies. Preliminary findings indicate potential in stimulating both innate and adaptive immunity, especially in “cold” cancers (Cohen et al., 2022; Meric-Bernstam et al., 2023).
Recent research has uncovered additional functional functions of cGAS-STING in cancer, in addition to its well-established involvement in antiviral immunity. Tumors have the ability to regulate a diverse array of molecular processes in order to either enhance or inhibit malignancy by manipulating the cGAS-STING pathway. STING expression levels may be precisely regulated as a potential approach. In a recent study, it was shown that the activation of apoptotic programs in macrophages and T lymphocytes was directly influenced by the intensity of STING signaling, which supported this concept. This implies that the activation of specific downstream effector programs could result from the regulation of STING’s activity. In order to comprehend the precise molecular factors and circumstances that determine whether the cGAS-STING cascade promotes or inhibits metastasis, further research is required.
Therefore, the meticulous screening of patients based on genomic and phenotypic indicators of chromosomal instability (CIN) should provide valuable information regarding which patients could potentially benefit from the inhibition or activation of the STING pathway.
AH: Validation, Visualization, Writing–original draft, Writing–review and editing. LW: Supervision, Writing–review and editing. RW: Conceptualization, Funding acquisition, Supervision, Writing–original draft, Writing–review and editing.
The author(s) declare that financial support was received for the research, authorship, and/or publication of this article. This research was supported by Suqian Science and Technology Program (grant no. K202410), Jiangsu Science and Technology Association (grant no. JSTJ-2022-004), China Scholarship Council (grant no. 202206920039).
The authors declare that the research was conducted in the absence of any commercial or financial relationships that could be construed as a potential conflict of interest.
The author(s) declare that no Generative AI was used in the creation of this manuscript.
All claims expressed in this article are solely those of the authors and do not necessarily represent those of their affiliated organizations, or those of the publisher, the editors and the reviewers. Any product that may be evaluated in this article, or claim that may be made by its manufacturer, is not guaranteed or endorsed by the publisher.
Andreeva, L., Hiller, B., Kostrewa, D., Lässig, C., de Oliveira Mann, C. C., Jan Drexler, D., et al. (2017). cGAS senses long and HMGB/TFAM-bound U-turn DNA by forming protein-DNA ladders. Nature 549 (7672), 394–398. doi:10.1038/nature23890
Blest, H., and Chauveau, L. (2023). cGAMP the travelling messenger. Front. Immunol. 14, 1150705. doi:10.3389/fimmu.2023.1150705
Byun, J. S., and Gardner, K. (2013). Wounds that will not heal: pervasive cellular reprogramming in cancer. Am. J. Pathology 182 (4), 1055–1064. doi:10.1016/j.ajpath.2013.01.009
Chen, C., and Xu, P. (2023). Cellular functions of cGAS-STING signaling. Trends Cell Biol. 33 (8), 630–648. doi:10.1016/j.tcb.2022.11.001
Cohen, E., Nabell, L., Wong, D. J., Day, T., Daniels, G. A., Milhem, M., et al. (2022). Intralesional SD-101 in combination with Pembrolizumab in anti-PD-1 treatment-naive head and neck squamous cell carcinoma: results from a multicenter, phase II trial. Clin. Cancer Res. 28 (6), 1157–1166. doi:10.1158/1078-0432.ccr-21-1411
de Oliveira, M. C., Orzalli, M. H., King, D. S., Kagan, J. C., Lee, A. S. Y., and Kranzusch, P. J. (2019). Modular architecture of the STING C-terminal tail allows interferon and NF-kappaB signaling adaptation. Cell Rep. 27 (4), 1165–1175.e5. doi:10.1016/j.celrep.2019.03.098
Dorta-Estremera, S., Hegde, V. L., Slay, R. B., Sun, R., Yanamandra, A. V., Nicholas, C., et al. (2019). Targeting interferon signaling and CTLA-4 enhance the therapeutic efficacy of anti-PD-1 immunotherapy in preclinical model of HPV(+) oral cancer. J. Immunother. Cancer 7 (1), 252. doi:10.1186/s40425-019-0728-4
Fang, R., Jiang, Q., Guan, Y., Gao, P., Zhang, R., Zhao, Z., et al. (2021). Golgi apparatus-synthesized sulfated glycosaminoglycans mediate polymerization and activation of the cGAMP sensor STING. Immunity 54 (5), 962–975.e8. doi:10.1016/j.immuni.2021.03.011
Ge, H., Dan, Q., and Yang, Y. (2023). cGAS-STING pathway as the target of immunotherapy for lung cancer. Curr. Cancer Drug Targets 23 (5), 354–362. doi:10.2174/1568009623666221115095114
Gentili, M., Liu, B., Papanastasiou, M., Dele-Oni, D., Schwartz, M. A., Carlson, R. J., et al. (2023). ESCRT-dependent STING degradation inhibits steady-state and cGAMP-induced signalling. Nat. Commun. 14 (1), 611. doi:10.1038/s41467-023-36132-9
Gui, X., Yang, H., Li, T., Tan, X., Shi, P., Li, M., et al. (2019). Autophagy induction via STING trafficking is a primordial function of the cGAS pathway. Nature 567 (7747), 262–266. doi:10.1038/s41586-019-1006-9
Hancock-Cerutti, W., Wu, Z., Xu, P., Yadavalli, N., Leonzino, M., Tharkeshwar, A. K., et al. (2022). ER-lysosome lipid transfer protein VPS13C/PARK23 prevents aberrant mtDNA-dependent STING signaling. J. Cell Biol. 221 (7), e202106046. doi:10.1083/jcb.202106046
Hines, J. B., Kacew, A. J., and Sweis, R. F. (2023). The development of STING agonists and emerging results as a cancer immunotherapy. Curr. Oncol. Rep. 25 (3), 189–199. doi:10.1007/s11912-023-01361-0
King, K. R., Aguirre, A. D., Ye, Y. X., Sun, Y., Roh, J. D., Ng, R. P., et al. (2017). IRF3 and type I interferons fuel a fatal response to myocardial infarction. Nat. Med. 23 (12), 1481–1487. doi:10.1038/nm.4428
Li, W., Lu, L., Lu, J., Wang, X., Yang, C., Jin, J., et al. (2020). cGAS-STING-mediated DNA sensing maintains CD8(+) T cell stemness and promotes antitumor T cell therapy. Sci. Transl. Med. 12 (549), eaay9013. doi:10.1126/scitranslmed.aay9013
Lin, C., Kuffour, E. O., Fuchs, N. V., Gertzen, C. G., Kaiser, J., Hirschenberger, M., et al. (2023). Regulation of STING activity in DNA sensing by ISG15 modification. Cell Rep. 42 (11), 113277. doi:10.1016/j.celrep.2023.113277
Lin, W., Szabo, C., Liu, T., Tao, H., Wu, X., and Wu, J. (2024). STING trafficking activates MAPK-CREB signaling to trigger regulatory T cell differentiation. Proc. Natl. Acad. Sci. U. S. A. 121 (29), e2320709121. doi:10.1073/pnas.2320709121
Luecke, S., Holleufer, A., Christensen, M. H., Jønsson, K. L., Boni, G. A., Sørensen, L. K., et al. (2017). cGAS is activated by DNA in a length-dependent manner. EMBO Rep. 18 (10), 1707–1715. doi:10.15252/embr.201744017
Meric-Bernstam, F., Sweis, R. F., Kasper, S., Hamid, O., Bhatia, S., Dummer, R., et al. (2023). Combination of the STING agonist MIW815 (ADU-S100) and PD-1 inhibitor spartalizumab in advanced/metastatic solid tumors or lymphomas: an open-label, multicenter, phase Ib study. Clin. Cancer Res. 29 (1), 110–121. doi:10.1158/1078-0432.ccr-22-2235
Morrison, W. B. (2012). Inflammation and cancer: a comparative view. J. Veterinary Intern. Med. 26 (1), 18–31. doi:10.1111/j.1939-1676.2011.00836.x
Ou, L., Zhang, A., Cheng, Y., and Chen, Y. (2021). The cGAS-STING pathway: a promising immunotherapy target. Front. Immunol. 12, 795048. doi:10.3389/fimmu.2021.795048
Raghava Kurup, R., Oakes, E. K., Vadlamani, P., Nwosu, O., Danthi, P., and Hundley, H. A. (2022). ADAR3 activates NF-κB signaling and promotes glioblastoma cell resistance to temozolomide. Sci. Rep. 12 (1), 13362. doi:10.1038/s41598-022-17559-4
Serpico, A. F., Pisauro, C., and Grieco, D. (2023). cGAS-dependent proinflammatory and immune homeostatic effects of the microtubule-targeting agent paclitaxel. Front. Immunol. 14, 1127623. doi:10.3389/fimmu.2023.1127623
Talbot, E. J., Joshi, L., Thornton, P., Dezfouli, M., Tsafou, K., Perkinton, M., et al. (2024). cGAS-STING signalling regulates microglial chemotaxis in genome instability. Nucleic Acids Res. 52 (3), 1188–1206. doi:10.1093/nar/gkad1184
Targa, A., and Rancati, G. (2018). Cancer: a CINful evolution. Curr. Opin. Cell Biol. 52, 136–144. doi:10.1016/j.ceb.2018.03.007
Wang, L., Li, S., Wang, K., Wang, N., Liu, Q., Sun, Z., et al. (2022). DNA mechanical flexibility controls DNA potential to activate cGAS-mediated immune surveillance. Nat. Commun. 13 (1), 7107. doi:10.1038/s41467-022-34858-6
Wang, R., Hussain, A., Guo, Q., and Ma, M. (2024). cGAS-STING at the crossroads in cancer therapy. Crit. Rev. Oncology/Hematology 193, 104194. doi:10.1016/j.critrevonc.2023.104194
Wang, X., Zhang, H., Wang, Y., Bramasole, L., Guo, K., Mourtada, F., et al. (2023). DNA sensing via the cGAS/STING pathway activates the immunoproteasome and adaptive T-cell immunity. EMBO J. 42 (8), e110597. doi:10.15252/embj.2022110597
Wu, J., Chen, Y. J., Dobbs, N., Sakai, T., Liou, J., Miner, J. J., et al. (2019). STING-mediated disruption of calcium homeostasis chronically activates ER stress and primes T cell death. J. Exp. Med. 216 (4), 867–883. doi:10.1084/jem.20182192
Yamazaki, T., Bravo-San Pedro, J. M., Galluzzi, L., Kroemer, G., and Pietrocola, F. (2021). Autophagy in the cancer-immunity dialogue. Adv. Drug Deliv. Rev. 169, 40–50. doi:10.1016/j.addr.2020.12.003
Yu, R., Zhu, B., and Chen, D. (2022). Type I interferon-mediated tumor immunity and its role in immunotherapy. Cell Mol. Life Sci. 79 (3), 191. doi:10.1007/s00018-022-04219-z
Yum, S., Li, M., Fang, Y., and Chen, Z. J. (2021). TBK1 recruitment to STING activates both IRF3 and NF-κB that mediate immune defense against tumors and viral infections. Proc. Natl. Acad. Sci. U. S. A. 118 (14), e2100225118. doi:10.1073/pnas.2100225118
Zhang, L., Wei, X., Wang, Z., Liu, P., Hou, Y., Xu, Y., et al. (2023). NF-κB activation enhances STING signaling by altering microtubule-mediated STING trafficking. Cell Rep. 42 (3), 112185. doi:10.1016/j.celrep.2023.112185
Zhang, R., Kang, R., and Tang, D. (2021). The STING1 network regulates autophagy and cell death. Signal Transduct. Target. Ther. 6 (1), 208. doi:10.1038/s41392-021-00613-4
Keywords: CGAS, STING, immune checkpoint inhibitors (ICI), tumor microenvironment, immune therapy
Citation: Hussain A, Wen L and Wang R (2025) Multifaceted roles of STING in tumors: from molecular mechanisms to therapeutic strategies. Front. Chem. Biol. 4:1549003. doi: 10.3389/fchbi.2025.1549003
Received: 20 December 2024; Accepted: 04 February 2025;
Published: 28 February 2025.
Edited by:
Neelkanth Bardhan, Massachusetts Institute of Technology, United StatesReviewed by:
Euna Yoo, National Cancer Institute at Frederick (NIH), United StatesCopyright © 2025 Hussain, Wen and Wang. This is an open-access article distributed under the terms of the Creative Commons Attribution License (CC BY). The use, distribution or reproduction in other forums is permitted, provided the original author(s) and the copyright owner(s) are credited and that the original publication in this journal is cited, in accordance with accepted academic practice. No use, distribution or reproduction is permitted which does not comply with these terms.
*Correspondence: Rui Wang, MTU4OTYzNDE2NjJAMTYzLmNvbQ==; Linchun Wen, d2VubGluY2h1bkAxMjYuY29t
Disclaimer: All claims expressed in this article are solely those of the authors and do not necessarily represent those of their affiliated organizations, or those of the publisher, the editors and the reviewers. Any product that may be evaluated in this article or claim that may be made by its manufacturer is not guaranteed or endorsed by the publisher.
Research integrity at Frontiers
Learn more about the work of our research integrity team to safeguard the quality of each article we publish.