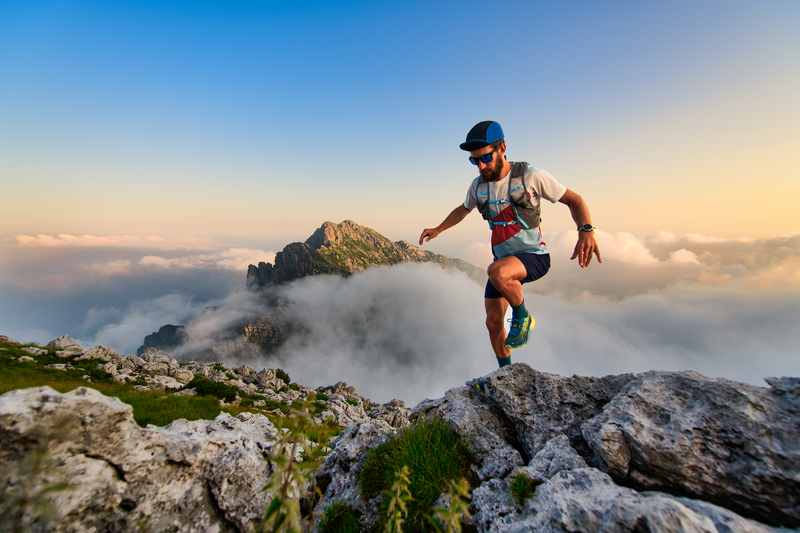
95% of researchers rate our articles as excellent or good
Learn more about the work of our research integrity team to safeguard the quality of each article we publish.
Find out more
MINI REVIEW article
Front. Chem. Biol. , 05 April 2024
Sec. Molecular Sciences
Volume 3 - 2024 | https://doi.org/10.3389/fchbi.2024.1386220
This article is part of the Research Topic STING Signaling in Cancer Immunotherapy View all 4 articles
Engaging the immune sensing Stimulator of Interferon Genes (STING) pathway has emerged as a potentially powerful approach to cancer therapy. However, current STING agonists lack stability and specificity, resulting in toxic adverse effects and disappointing patient outcomes. Therefore, novel delivery vehicles are needed to mitigate negative results and improve the efficacy of STING agonists. Here we discuss innovative particle-based strategies and how they have increased the therapeutic results seen with STING agonists. We review ultrasound-responsive vehicles, pH-responsive particles, inorganic particles, carriers for extended release, and particles that act as both STING agonists and/or drug carriers. Further optimization of these strategies can potentially enable the clinical use of STING agonists for cancer therapy.
As early as ancient Egypt, there have been anecdotal reports detailing the regression or spontaneous eradication of tumorous growths following an infection or episode of high fever (Dobosz and Dzieciatkowski, 2019). This led to the belief that the immunological processes used to eliminate infectious diseases could also be exploited in the fight against cancer. Over time this belief became reality, especially when it was elucidated that the cyclic GMP-AMP synthase–stimulator of interferon genes (cGAS-STING) pathway mediates innate immune recognition of tumors (Woo et al., 2014; Corrales and Gajewski, 2016). The cGAS-STING pathway, a crucial component of innate immune defense against foreign pathogens, is initiated by the recognition of irregular cytosolic DNA (Sun et al., 2013). The resulting cascade of effector responses culminates in the production of proinflammatory type I interferons (IFNs) that are responsible for adequate T cell priming (Ishikawa and Barber, 2008; Crouse et al., 2015). These immunostimulatory attributes associated with STING make it an attractive target for anticancer applications.
Even before the STING pathway was fully elucidated in 2013, its anticancer potential was already established, as the chemotherapeutic 5,6-dimethylxanthenone-4-acetic acid (DMXAA) had been discovered to be a STING agonist, albeit in mouse models only (Prantner et al., 2012; Conlon et al., 2013). Nevertheless, the potential that STING could promote antitumor responses led to the development of many synthetic STING agonists (Amouzegar et al., 2021). Since then, studies have revealed that the efficacy of other clinically used cancer treatments, such as radiotherapy and immune checkpoint inhibitors (ICIs), are also dependent on STING activation (Deng et al., 2014; Wang et al., 2017). In light of these results, numerous clinical trials have been initiated to explore the use of STING agonists for the treatment of a variety of cancer indications or to improve current treatments (Ding et al., 2020; Le Naour et al., 2020; Vasiyani et al., 2023).
And yet, FDA approval has remained elusive due to a multitude of stability, efficacy, and safety issues (Motedayen Aval et al., 2020). The repeated failed clinical results, however, have gleaned insight into the shortcomings of STING agonists. Endogenous STING agonists such as 2′3′-cGAMP are cyclic dinucleotides that are degraded by circulating and cell-bound esterases such as ENPP1 (Li et al., 2014). This results in a low serum half-life and consequently limited efficacy after systemic administration (Lioux et al., 2016). As such, synthetic STING agonists with improved serum stability were developed for clinical application (Chen et al., 2024). Off-target toxicity is still an issue, however, as STING activation in T-cells and B-cells can trigger lymphocyte cell death and apoptosis, facilitating tumor immune evasion and further hindering clinical translation (Wu et al., 2020; Li S. et al., 2022). Intratumoral injection has been used as a strategy to overcome the risks of off-target toxicity associated with systemic administration by concentrating the therapeutic dose at the tumor site (Melero et al., 2021). Unfortunately, due to their low molecular weight and high water solubility, STING agonists rapidly clear out of the tumoral space and reach systemic circulation after intratumoral injection (Sivick et al., 2018; Meric-Bernstam et al., 2019). Finally, the negative charge present on most STING agonists also limits their intracellular delivery across the negatively charged plasma cell membrane (Koshy et al., 2017). Given the therapeutic potential of STING activation, the pressing need for novel strategies to overcome associated technical and clinical challenges is evident. In this review, we will discuss innovative delivery vehicles for advancing STING agonists towards clinical use.
Originally used in the clinic as contrast agents for ultrasound (US) imaging, microbubbles (MBs) now show promise for drug delivery in clinically translatable immunotherapeutic applications (Ho et al., 2020; Navarro-Becerra and Borden, 2023). Comprised of a gas core and a lipid, protein, or polymer shell, these 1–10 µm particles are elastic and able to oscillate in an acoustic field (Tinkov et al., 2009). At high acoustic pressures, MBs will collapse and transiently permeabilize cell membranes within their vicinity, allowing for the delivery of any incorporated payload directly into the cell’s cytoplasm (Bouakaz and Michel Escoffre, 2024). This phenomenon, termed sonoporation, bypasses endocytosis and facilitates the use of MBs in drug delivery. Furthermore, MBs are known to have a favorable safety profile due to their decades of use as contrast agents (Jakobsen et al., 2005). US scanners also have the added benefit of being inexpensive, widely available, and noninvasive while allowing for both spatial and temporal control over delivery of payloads on MBs (Sharma et al., 2022). In a recent example, nanocomplex-conjugated MBs were loaded with cGAMP and targeted to antigen-presenting cells (APCs) (Li X. et al., 2022). Through US-meditated release, cGAMP was delivered directly into the cytosol of tumor-associated APCs, resulting in activation of STING and downstream inflammatory effects (Figure 1A). This strategy, termed MUSIC, bridged innate and adaptive immunities by increasing cytokine production and T cell infiltration. Furthermore, MUSIC led to greater tumor growth inhibition and increased survival in metastatic murine breast cancer and a 60% complete response rate in primary breast cancer. Rechallenging the complete responders led to a 100% survival rate, suggesting that MUSIC could act as an in situ vaccine. The specificity of MUSIC was also validated, as using non-targeted MBs resulted in a complete loss of efficacy. This work provides convincing evidence of the therapeutic potential of US-mediated STING activation and offers a clinically translatable strategy to overcome the limitations of free STING agonists.
Figure 1. Novel drug delivery strategies for enhanced STING activation. (A) Ultrasound and microbubbles can be used to transiently permeabilize cell membranes, allowing for the direct delivery of STING agonist into the cell’s cytosol. (B) The environment of the tumor and cell cytosol will cause hydrogels to expand, thus releasing their STING agonist cargo for an extended period. (C) Drug delivery vehicles can be designed to bind and activate STING themselves, serving as both agonist and drug carrier. (D) Particles exhibiting pH-responsiveness can facilitate endosome dissociation to improve endosomal release of their STING agonist cargo. (E) Manganese-based particles will degrade in response to the tumor microenvironment, causing the release of manganese ions that have a direct effect on STING activation. Credit: Siloa Willis.
Hydrogels are an appealing drug delivery vehicle because of their stability and sustained release properties (Vigata et al., 2020). In addition, many physical properties of hydrogels, such as their pore size, rate of degradation, and drug-network interactions, are tunable based on the desired therapeutic application (Li and Mooney, 2016). Owing to these unique properties, hydrogel nanoparticles were one of the first published vehicles for delivery of STING agonists (Figure 1B) (Lee et al., 2016). A more recent example reported a supramolecular hydrogel system for the intratumoral codelivery of STING agonists and chemotherapeutic drugs (Wang et al., 2020). The hydrogel consisted of a peptide-drug conjugate of iRGD and camtothecin that spontaneously self-assembled into nanotubes. The STING agonist cyclic-di-AMP (CDA) was then condensed on the surface of the nanotubes, which then formed hydrogels in physiological conditions. The designed hydrogel system demonstrated sustainable prolonged release over several weeks for both therapeutic agents, was able to degrade in vivo over 45 days, and showed enhanced tumor tissue penetration through iRGD-mediated targeting. The localized administration of this hydrogel in various tumor models, including brain tumors, colon cancer, and breast cancer, led to near-complete tumor regression, prolonged survival, and prevention of tumor recurrence and metastasis. Evaluation of the immune landscape after treatment exhibited increased infiltration of natural killer cells, dendritic cells (DCs), and antigen-specific T cells. These results suggest that STING agonist-loaded hydrogels can promote immune stimulation and induce robust antitumor immune responses by extending the local release of drugs compared with their solution form.
In another study, STING agonist and pancreatic cancer neoantigens were embedded into a hyaluronic acid hydrogel for implantation at the tumor site after incomplete resection surgery (Delitto et al., 2021). The loaded components, termed PancVax, were selected after exhibiting enhanced tumor regression and survival benefit when combined with ICIs in a mouse model of pancreatic adenocarcinoma (Kinkead et al., 2018). Compared to PancVax alone, however, PancVax gel did not require additional ICIs to induce tumor regression. PancVax gel prevented local tumor recurrence and demonstrated no evidence of residual tumors even at 2 months after incomplete tumor resection, suggesting complete regression of the remnant tumor. Comparatively, surgery-only resulted in uniform regrowth only a week after resection. PancVax gel also led to T cell activation in the lymph nodes and expansion of antigen-specific T cells, demonstrating vaccine efficacy. Other immune landscape changes associated with the PancVax gel included an influx of neutrophils, macrophages, and dendritic cells 1 week after implantation, confirming innate immune activation and continued adjuvant activity. These results demonstrate that STING-loaded hydrogel vaccines can help modulate the local immunity at the time of surgery to eliminate residual tumor.
In addition to encapsulating payload, some drug delivery vehicles have been developed to intrinsically bind to and activate STING (Zhao et al., 2022). In one example, a polymeric micelle consisting of an amphiphilic PEG-polymethacrylate block copolymer was found to act as a STING agonist on its own (Figure 1C) (Luo et al., 2017). This polymer, termed PC7A, contained repeating cyclic seven-membered tertiary amine sidechains that conferred ultra-pH sensitivity through a cooperative effect (Zhou et al., 2012). Loading PC7A micelles with tumor-associated antigens led to potent tumor growth inhibition in murine melanoma, colon cancer, and human papilloma virus (HPV)-induced tumor models. These results were determined to be dependent on the cyclic seven-membered tertiary amine side chain on PC7A as well as the number of repeating units on the polymer (Li et al., 2021). A higher degree of polymerization was found to increase the number of polyvalent interactions with STING protein, thus inducing a phase condensation mechanism for STING activation. Further experiments confirmed a noncompetitive binding site for PC7A that was distinct from the natural cGAMP binding site, which formed the basis for the combination of PC7A with cGAMP to obtain prolonged and synergistic STING activation. Treatment with cGAMP-loaded PC7A nanoparticles (NPs) showed significantly improved tumor growth inhibition and long-term survival compared with either treatment alone in colon and HPV-induced tumor models. As such, using polyvalent interactions to activate STING in a condensation manner offers a robust strategy to boost cancer immunotherapy.
A separate example confirmed the STING activity of cyclic tertiary amines by evaluating a library of lipids composed of alkyl and alkylene ketone lipid tails, isocyanide linkers, and amine head groups (Miao et al., 2019). Preliminary experiments demonstrated that, although similar levels of protein expression were induced, mRNA-loaded lipid nanoparticles (LNPs) that were formulated with lipids containing heterocyclic amine head groups stimulated a greater adaptive immune response than their linear-amine counterparts. Further exploration into structure-function relationships determined that lipids containing heterocyclic amino head groups were able to facilitate APC maturation in a STING-dependent manner, thus acting as STING agonists. Empty LNPs that were formulated with these lipids were found to increase expression of DC activation markers (CD86/CD40), secretion of IFN-γ, and activation of interferon regulatory factor compared to LNPs formulated with linear amine head groups. Cyclic amine LNPs were then loaded with antigen-expressing mRNA and assessed for efficacy in a variety of tumor models, including OVA-expressing B16F10 (B16-OVA), B16F10 melanoma, and TC-1 HPV. The cyclic amine LNPs were able to deliver tumor-specific antigens for each model, slow tumor growth, and increase survival. The LNPs also increased CD8+ T cell infiltration in all three tumor models, confirming that they can induce a strong adaptive immune response in vivo. These results suggest that the incorporation of a STING agonist as a lipid component of an LNP system not only facilitates mRNA delivery for vaccination, but also provides adjuvant stimulation via STING activation.
During endocytosis, the pH of the endolysosomal lumen decreases from 6.5 to 4.5 as compared to 7.4 in the extracellular environment (Hu et al., 2015). Therefore, use of materials that will cause endosome dissociation in response to pH changes is a potentially effective strategy to enhance therapeutic efficacy and reduce toxic effects (Figure 1D) (Manchun et al., 2012; Su et al., 2022). In one study, polymersomes were formulated with amphiphilic diblock copolymers containing chains of moieties that confer pH-sensitivity and facilitate endosomal escape (Manganiello et al., 2012; Shae et al., 2019). In response to the acidic pH of the endolysosomal environment, the polymersomes disassembled to reveal the membrane-destabilizing polymer segments that promoted endosomal escape of encapsulated cargo. Using these endosomolytic polymersomes to deliver cGAMP enhanced potency by 240-610-fold over free cGAMP depending on the cell line. In the B16F10 melanoma model, cGAMP-encapsulated polymersomes resulted in an 11-fold decrease in the tumor growth rate and a significant increase in survival relative to cGAMP only, with one-third of treated mice completely rejecting tumors. Rechallenging the complete responders led to five out of seven mice completely resisting tumor growth, suggesting the establishment of systemic antitumor immune memory.
In a follow-up study, the polymersomes were co-loaded with both cGAMP and peptide antigens to yield a cancer vaccine termed nanoSTING-vax (Shae et al., 2020). The co-delivery of both cGAMP and peptide antigens into the cytosol of APCs using nanoSTING-vax elicited inflammatory cytokine production, co-stimulatory marker expression, and antigen cross-presentation to a greater extent than controls. NanoSTING-vax was also able to enhance antigen-specific CD8+ T cell proliferation in vivo against several epitopes, including the OVA model antigen as well as two antigens associated with MC38 colon adenocarcinoma. As a functional validation of the CD8+ T cell response, mice were subcutaneously immunized with nanoSTING-vax first and then challenged with B16-OVA, which resulted in significant inhibition of tumor growth. NanoSTING-vax was also able to synergize with ICIs in the treatment of MC38 and B16F10, resulting in 70% and 30% complete response rates respectively. Overall, these results indicate that delivery of STING agonists with polymersomes can synergize with tumor antigens, resulting in personalized cancer vaccines that improve anticancer responses.
Manganese (Mn2+) has been shown to be essential for host defense by increasing the sensitivity of cGAS to double stranded DNA as well as enhancing cGAMP’s binding affinity to STING (Wang et al., 2018). Further studies revealed that Mn2+ is also essential in the innate immune sensing of tumors by inducing type I IFN production in a STING-dependent manner, thus promoting DC maturation and T cell-mediated antitumor effects (Lv et al., 2020). The same study also showed that Mn2+ could revive patient responses to anti-PD1 therapy and exhibit type I IFN induction, thereby exhibiting adjuvant potential. Groups are now working on delivery of both Mn2+ and STING agonist for improved immunotherapy (Sun et al., 2021). A recent study explored the use of theranostic manganese oxide nanoparticles coated with mesoporous silica and conjugated with the tumor-homing peptide iRGD as a potential Mn2+ delivery vehicle and imaging agent (Sun et al., 2022). In response to the acidic tumor microenvironment, the NPs degraded and released Mn2+ (Figure 1E) to act as both a STING agonist and a catalyst for the generation of hydroxyl radicals from endogenous H2O2. Simultaneously, the NPs were able to also enhance T1 contrast in MRI of the tumor, suggesting tumor-specific uptake. In vivo, these NPs synergized with anti-PD1 to augment cytotoxic T lymphocyte infiltration and pro-inflammatory cytokine production in the tumor. These immune effects led to enhanced tumor inhibition as well as reduced lung metastases in the B16F10 melanoma tumor model. Furthermore, these NPs were biodegradable and excreted from the body after 14 days, indicating a favorable safety profile.
A separate study evaluated the use of manganese oxide-coated hollow mesoporous silica nanoparticles (MnOx@HMSN) as a platform for the co-delivery of antigens and STING agonist with Mn2+ as an adjuvant for vaccination against either cancer or SARS-CoV-2 (Xu et al., 2023). MnOx@HMSNs released Mn2+ at a greater rate in a tumor-mimicking environment, suggesting tumor specific release of cargo. MnOx@HMSNs alone also activated STING and the downstream IFN pathway, which was synergistically enhanced upon inclusion of the STING agonist CDA. Furthermore, treatment of DCs using CDA-loaded MnOx@HMSNs led to higher upregulation of CD80 and CD86, indicating DC activation and maturation. As rapid clearance is an issue with STING agonists, MnOx@HMSNs were shown to retain CDA in the tumor microenvironment for over 144 h, a significant improvement over CDA alone. In CT26 and MC38 murine colon cancer tumors, MnOx@HMSNs demonstrated potent antitumor effects in both local and untreated distal tumors when co-loaded with CDA and tumor antigens, thus exhibiting antigen-specific T cell responses and abscopal effects. Lastly, MnOx@HMSNs were co-loaded with CDA and the SARS-CoV-2 spike receptor binding domain (RBD) protein to investigate their potential as a COVID-19 vaccine. After two immunizations, vaccination with MnOx@HMSNs resulted in 50-fold higher RBD-specific serum IgG titers compared with the soluble CDA + RBD + Mn2+ group, demonstrating strong humoral and cellular immune responses against SARS-CoV-2. As such, these studies establish the role of Mn2+ as an effective STING agonist and confirm that their incorporation into NPs can result in tumor-specific imaging and immunotherapy.
The cGAS-STING pathway, a key regulator of innate immune defense, has emerged as a promising target in immunotherapy due to its ability to bridge the innate and adaptive immune systems. By triggering the release of proinflammatory cytokines, STING activation is directly responsible for APC maturation and T cell priming. The potential of STING agonists in cancer treatment is only further fueled by the growing evidence suggesting that STING activation has a direct impact on the efficacy of many popular cancer therapies, such as radiotherapy and ICI therapy. As such, many pharmaceutical companies have begun clinical trials for their proprietary agonists. However, despite the established potential and preclinical results, the journey to FDA approval for STING agonists has been disappointing. The multiple clinical failures of STING agonists have illuminated previously unknown barriers, such as low serum stability, off-target activation, and poor membrane permeability. If STING agonists are to become applicable in a clinical setting, they will require the use of novel delivery strategies.
This review sought to examine many innovative delivery vehicles currently in development that present promising solutions to the challenges associated with STING agonists (Table 1). These vehicles have been shown to not only enhance the delivery efficiency of these agonists, but also protect them from degradation, exhibit responsiveness to the tumor microenvironment, extend release time, increase cell specificity, or even act as STING agonists themselves. By converging engineering principles with immunological insights, these delivery strategies collectively address many of the limitations of current STING agonist administration. Moving forward, future research endeavors should be directed towards optimization of these delivery systems for clinical translation. By ensuring their safety and efficacy across diverse cancer indications, these strategies will become well equipped for clinical use. As these strategies progress through development, the prospect of incorporating STING agonists into clinical workflows inches closer to reality, thus ushering in a new era for immuno-oncology.
SK: Conceptualization, Investigation, Visualization, Writing–original draft, Writing–review and editing. KH: Conceptualization, Investigation, Project administration, Validation, Writing–review and editing. JL: Conceptualization, Funding acquisition, Investigation, Project administration, Resources, Supervision, Validation, Writing–review and editing.
The author(s) declare that no financial support was received for the research, authorship, and/or publication of this article. This work was supported in part by the Cancer Prevention and Research Institute of Texas (CPRIT) grant RP210199, the Department of Defense grants W81XWH-21-1-0332/0333, the UT Southwestern Innovation Days Award, and the department of Radiology at UT Southwestern.
The authors would like to thank Siloa Willis for illustration of the figure.
SK and JL are cofounders of MusiQ Bio.
The remaining author declares that the research was conducted in the absence of any commercial or financial relationships that could be construed as a potential conflict of interest.
The author KH declared that they were an editorial board member of Frontiers, at the time of submission. This had no impact on the peer review process and the final decision.
All claims expressed in this article are solely those of the authors and do not necessarily represent those of their affiliated organizations, or those of the publisher, the editors and the reviewers. Any product that may be evaluated in this article, or claim that may be made by its manufacturer, is not guaranteed or endorsed by the publisher.
APC, antigen-presenting cell; CDA, cyclic-di-AMP; cGAMP, cyclic GMP-AMP; cGAS-STING, cyclic GMP-AMP synthase–stimulator of interferon genes; DC, dendritic cell; HPV, human papilloma virus; ICI, immune checkpoint inhibitor; IFN, interferon; MB, microbubble; Mn2+, manganese; MnOx@HMSN, manganese oxide-coated hollow mesoporous silica nanoparticle; MUSIC, microbubble-assisted ultrasound-guided immunotherapy of cancer; LNP, lipid nanoparticles; NP, nanoparticles; OVA, ovalbumin; RBD, receptor binding domain; STING, stimulator of interferon genes; US, ultrasound.
Amouzegar, A., Chelvanambi, M., Filderman, J. N., Storkus, W. J., and Luke, J. J. (2021). STING agonists as cancer therapeutics. Cancers 13, 2695. doi:10.3390/cancers13112695
Bouakaz, A., and Michel Escoffre, J. (2024). From concept to early clinical trials: 30 years of microbubble-based ultrasound-mediated drug delivery research. Adv. Drug Deliv. Rev. 206, 115199. doi:10.1016/j.addr.2024.115199
Chen, X., Xu, Z., Li, T., Thakur, A., Wen, Y., Zhang, K., et al. (2024). Nanomaterial-encapsulated STING agonists for immune modulation in cancer therapy. Biomark. Res. 12, 2. doi:10.1186/s40364-023-00551-z
Conlon, J., Burdette, D. L., Sharma, S., Bhat, N., Thompson, M., Jiang, Z., et al. (2013). Mouse, but not human STING, binds and signals in response to the vascular disrupting agent 5,6-dimethylxanthenone-4-acetic acid. J. Immunol. 190, 5216–5225. doi:10.4049/jimmunol.1300097
Corrales, L., and Gajewski, T. F. (2016). Endogenous and pharmacologic targeting of the STING pathway in cancer immunotherapy. Cytokine 77, 245–247. doi:10.1016/j.cyto.2015.08.258
Crouse, J., Kalinke, U., and Oxenius, A. (2015). Regulation of antiviral T cell responses by type I interferons. Nat. Rev. Immunol. 15, 231–242. doi:10.1038/nri3806
Delitto, D., Zabransky, D. J., Chen, F., Thompson, E. D., Zimmerman, J. W., Armstrong, T. D., et al. (2021). Implantation of a neoantigen-targeted hydrogel vaccine prevents recurrence of pancreatic adenocarcinoma after incomplete resection. Oncoimmunology 10, 2001159. doi:10.1080/2162402X.2021.2001159
Deng, L., Liang, H., Xu, M., Yang, X., Burnette, B., Arina, A., et al. (2014). STING-dependent cytosolic DNA sensing promotes radiation-induced type I interferon-dependent antitumor immunity in immunogenic tumors. Immunity 41, 843–852. doi:10.1016/j.immuni.2014.10.019
Ding, C., Song, Z., Shen, A., Chen, T., and Zhang, A. (2020). Small molecules targeting the innate immune cGAS‒STING‒TBK1 signaling pathway. Acta Pharm. Sin. B 10, 2272–2298. doi:10.1016/j.apsb.2020.03.001
Dobosz, P., and Dzieciatkowski, T. (2019). The intriguing history of cancer immunotherapy. Front. Immunol. 10, 2965. doi:10.3389/fimmu.2019.02965
Ho, Y. J., Li, J. P., Fan, C. H., Liu, H. L., and Yeh, C. K. (2020). Ultrasound in tumor immunotherapy: current status and future developments. J. Control. Release 323, 12–23. doi:10.1016/j.jconrel.2020.04.023
Hu, Y. B., Dammer, E. B., Ren, R. J., and Wang, G. (2015). The endosomal-lysosomal system: from acidification and cargo sorting to neurodegeneration. Transl. Neurodegener. 4, 18. doi:10.1186/s40035-015-0041-1
Ishikawa, H., and Barber, G. N. (2008). STING is an endoplasmic reticulum adaptor that facilitates innate immune signalling. Nature 455, 674–678. doi:10.1038/nature07317
Jakobsen, J., Oyen, R., Thomsen, H. S., and Morcos, S. K.Members of Contrast Media Safety Committee of European Society of Urogenital Radiology (2005). Safety of ultrasound contrast agents. Eur. Radiol. 15, 941–945. doi:10.1007/s00330-004-2601-0
Kinkead, H. L., Hopkins, A., Lutz, E., Wu, A. A., Yarchoan, M., Cruz, K., et al. (2018). Combining STING-based neoantigen-targeted vaccine with checkpoint modulators enhances antitumor immunity in murine pancreatic cancer. JCI Insight 3, e122857. doi:10.1172/jci.insight.122857
Koshy, S. T., Cheung, A. S., Gu, L., Graveline, A. R., and Mooney, D. J. (2017). Liposomal delivery enhances immune activation by STING agonists for cancer immunotherapy. Adv. Biosyst. 1, 1600013. doi:10.1002/adbi.201600013
Lee, E., Jang, H. E., Kang, Y. Y., Kim, J., Ahn, J. H., and Mok, H. (2016). Submicron-sized hydrogels incorporating cyclic dinucleotides for selective delivery and elevated cytokine release in macrophages. Acta Biomater. 29, 271–281. doi:10.1016/j.actbio.2015.10.025
Le Naour, J., Zitvogel, L., Galluzzi, L., Vacchelli, E., and Kroemer, G. (2020). Trial watch: STING agonists in cancer therapy. Oncoimmunology 9, 1777624. doi:10.1080/2162402x.2020.1777624
Li, J., and Mooney, D. J. (2016). Designing hydrogels for controlled drug delivery. Nat. Rev. Mater 1, 16071. doi:10.1038/natrevmats.2016.71
Li, L. Y., Yin, Q., Kuss, P., Maliga, Z., Millán, J. L., Wu, H., et al. (2014). Hydrolysis of 2′3′-cGAMP by ENPP1 and design of nonhydrolyzable analogs. Nat. Chem. Biol. 10, 1043–1048. doi:10.1038/NChemBio.1661
Li, S., Luo, M., Wang, Z., Feng, Q., Wilhelm, J., Wang, X., et al. (2021). Prolonged activation of innate immune pathways by a polyvalent STING agonist. Nat. Biomed. Eng. 5, 455–466. doi:10.1038/s41551-020-00675-9
Li, S., Mirlekar, B., Johnson, B. M., Brickey, W. J., Wrobel, J. A., Yang, N., et al. (2022a). STING-induced regulatory B cells compromise NK function in cancer immunity. Nature 610, 373–380. doi:10.1038/s41586-022-05254-3
Li, X., Khorsandi, S., Wang, Y., Santelli, J., Huntoon, K., Nguyen, N., et al. (2022b). Cancer immunotherapy based on image-guided STING activation by nucleotide nanocomplex-decorated ultrasound microbubbles. Nat. Nanotechnol. 17, 891–899. doi:10.1038/s41565-022-01134-z
Lioux, T., Mauny, M. A., Lamoureux, A., Bascoul, N., Hays, M., Vernejoul, F., et al. (2016). Design, synthesis, and biological evaluation of novel cyclic adenosine-inosine monophosphate (cAIMP) analogs that activate stimulator of interferon genes (STING). J. Med. Chem. 59, 10253–10267. doi:10.1021/acs.jmedchem.6b01300
Luo, M., Wang, H., Wang, Z., Cai, H., Lu, Z., Li, Y., et al. (2017). A STING-activating nanovaccine for cancer immunotherapy. Nat. Nanotechnol. 12, 648–654. doi:10.1038/nnano.2017.52
Lv, M. Z., Chen, M. X., Zhang, R., Zhang, W., Wang, C. G., Zhang, Y., et al. (2020). Manganese is critical for antitumor immune responses via cGAS-STING and improves the efficacy of clinical immunotherapy. Cell Res. 30, 966–979. doi:10.1038/s41422-020-00395-4
Manchun, S., Dass, C. R., and Sriamornsak, P. (2012). Targeted therapy for cancer using pH-responsive nanocarrier systems. Life Sci. 90, 381–387. doi:10.1016/j.lfs.2012.01.008
Manganiello, M. J., Cheng, C., Convertine, A. J., Bryers, J. D., and Stayton, P. S. (2012). Diblock copolymers with tunable pH transitions for gene delivery. Biomaterials 33, 2301–2309. doi:10.1016/j.biomaterials.2011.11.019
Melero, I., Castanon, E., Alvarez, M., Champiat, S., and Marabelle, A. (2021). Intratumoural administration and tumour tissue targeting of cancer immunotherapies. Nat. Rev. Clin. Oncol. 18, 558–576. doi:10.1038/s41571-021-00507-y
Meric-Bernstam, F., Sandhu, S. K., Hamid, O., Spreafico, A., Kasper, S., Dummer, R., et al. (2019). Phase Ib study of MIW815 (ADU-S100) in combination with spartalizumab (PDR001) in patients (pts) with advanced/metastatic solid tumors or lymphomas. J. Clin. Oncol. 37, 2507. doi:10.1200/JCO.2019.37.15_suppl.2507
Miao, L., Li, L., Huang, Y., Delcassian, D., Chahal, J., Han, J., et al. (2019). Delivery of mRNA vaccines with heterocyclic lipids increases anti-tumor efficacy by STING-mediated immune cell activation. Nat. Biotechnol. 37, 1174–1185. doi:10.1038/s41587-019-0247-3
Motedayen Aval, L., Pease, J. E., Sharma, R., and Pinato, D. J. (2020). Challenges and opportunities in the clinical development of STING agonists for cancer immunotherapy. J. Clin. Med. 9, 3323. doi:10.3390/jcm9103323
Navarro-Becerra, J. A., and Borden, M. A. (2023). Targeted microbubbles for drug, gene, and cell delivery in therapy and immunotherapy. Pharmaceutics 15, 1625. doi:10.3390/pharmaceutics15061625
Prantner, D., Perkins, D. J., Lai, W., Williams, M. S., Sharma, S., Fitzgerald, K. A., et al. (2012). 5,6-Dimethylxanthenone-4-acetic acid (DMXAA) activates stimulator of interferon gene (STING)-dependent innate immune pathways and is regulated by mitochondrial membrane potential. J. Biol. Chem. 287, 39776–39788. doi:10.1074/jbc.M112.382986
Shae, D., Baljon, J. J., Wehbe, M., Christov, P. P., Becker, K. W., Kumar, A., et al. (2020). Co-Delivery of peptide neoantigens and stimulator of interferon genes agonists enhances response to cancer vaccines. ACS Nano 14, 9904–9916. doi:10.1021/acsnano.0c02765
Shae, D., Becker, K. W., Christov, P., Yun, D. S., Lytton-Jean, A. K. R., Sevimli, S., et al. (2019). Endosomolytic polymersomes increase the activity of cyclic dinucleotide STING agonists to enhance cancer immunotherapy. Nat. Nanotechnol. 14, 269–278. doi:10.1038/s41565-018-0342-5
Sharma, D., Leong, K. X., and Czarnota, G. J. (2022). Application of ultrasound combined with microbubbles for cancer therapy. Int. J. Mol. Sci. 23, 4393. doi:10.3390/ijms23084393
Sivick, K. E., Desbien, A. L., Glickman, L. H., Reiner, G. L., Corrales, L., Surh, N. H., et al. (2018). Magnitude of therapeutic STING activation determines CD8+ T cell-mediated anti-tumor immunity. Cell Rep. 25, 3074–3085.e5. doi:10.1016/j.celrep.2018.11.047
Su, T., Cheng, F. R., Qi, J. L., Zhang, Y., Zhou, S. R., Mei, L., et al. (2022). Responsive multivesicular polymeric nanovaccines that codeliver STING agonists and neoantigens for combination tumor immunotherapy. Adv. Sci. 9, e2201895. doi:10.1002/advs.202201895
Sun, L., Wu, J., Du, F., Chen, X., and Chen, Z. J. (2013). Cyclic GMP-AMP synthase is a cytosolic DNA sensor that activates the type I interferon pathway. Science 339, 786–791. doi:10.1126/science.1232458
Sun, X., Zhang, Y., Li, J., Park, K. S., Han, K., Zhou, X., et al. (2021). Amplifying STING activation by cyclic dinucleotide-manganese particles for local and systemic cancer metalloimmunotherapy. Nat. Nanotechnol. 16, 1260–1270. doi:10.1038/s41565-021-00962-9
Sun, Z., Wang, Z., Wang, T., Wang, J., Zhang, H., Li, Z., et al. (2022). Biodegradable MnO-based nanoparticles with engineering surface for tumor therapy: simultaneous fenton-like ion delivery and immune activation. ACS Nano 16, 11862–11875. doi:10.1021/acsnano.2c00969
Tinkov, S., Bekeredjian, R., Winter, G., and Coester, C. (2009). Microbubbles as ultrasound triggered drug carriers. J. Pharm. Sci. 98, 1935–1961. doi:10.1002/jps.21571
Vasiyani, H., Wadhwa, B., and Singh, R. (2023). Regulation of cGAS-STING signalling in cancer: approach for combination therapy. Biochimica Biophysica Acta (BBA) - Rev. Cancer 1878, 188896. doi:10.1016/j.bbcan.2023.188896
Vigata, M., Meinert, C., Hutmacher, D. W., and Bock, N. (2020). Hydrogels as drug delivery systems: a review of current characterization and evaluation techniques. Pharmaceutics 12, 1188. doi:10.3390/pharmaceutics12121188
Wang, C. G., Guan, Y. K., Lv, M. Z., Zhang, R., Guo, Z. Y., Wei, X. M., et al. (2018). Manganese increases the sensitivity of the cGAS-STING pathway for double-stranded DNA and is required for the host defense against DNA viruses. Immunity 48, 675–687.e7. doi:10.1016/j.immuni.2018.03.017
Wang, F., Su, H., Xu, D., Dai, W., Zhang, W., Wang, Z., et al. (2020). Tumour sensitization via the extended intratumoural release of a STING agonist and camptothecin from a self-assembled hydrogel. Nat. Biomed. Eng. 4, 1090–1101. doi:10.1038/s41551-020-0597-7
Wang, H., Hu, S., Chen, X., Shi, H., Chen, C., Sun, L., et al. (2017). cGAS is essential for the antitumor effect of immune checkpoint blockade. Proc. Natl. Acad. Sci. 114, 1637–1642. doi:10.1073/pnas.1621363114
Woo, S. R., Fuertes, M. B., Corrales, L., Spranger, S., Furdyna, M. J., Leung, M. Y., et al. (2014). STING-dependent cytosolic DNA sensing mediates innate immune recognition of immunogenic tumors. Immunity 41, 830–842. doi:10.1016/j.immuni.2014.10.017
Wu, J., Dobbs, N., Yang, K., and Yan, N. (2020). Interferon-independent activities of mammalian STING mediate antiviral response and tumor immune evasion. Immunity 53, 115–126 e5. doi:10.1016/j.immuni.2020.06.009
Xu, C., Dobson, H. E., Yu, M., Gong, W., Sun, X., Park, K. S., et al. (2023). STING agonist-loaded mesoporous manganese-silica nanoparticles for vaccine applications. J. Control. Release 357, 84–93. doi:10.1016/j.jconrel.2023.03.036
Zhao, J., Xu, Y., Ma, S., Wang, Y., Huang, Z., Qu, H., et al. (2022). A minimalist binary vaccine carrier for personalized postoperative cancer vaccine therapy. Adv. Mater. 34, e2109254. doi:10.1002/adma.202109254
Keywords: STING, immunotherapy, cancer therapy, drug delivery, particles
Citation: Khorsandi S, Huntoon K and Lux J (2024) Putting the sting back in STING therapy: novel delivery vehicles for improved STING activation. Front. Chem. Biol 3:1386220. doi: 10.3389/fchbi.2024.1386220
Received: 14 February 2024; Accepted: 11 March 2024;
Published: 05 April 2024.
Edited by:
Efstratios Stratikos, National and Kapodistrian University of Athens, GreeceReviewed by:
Alexiane Decout, Imperial College London, United KingdomCopyright © 2024 Khorsandi, Huntoon and Lux. This is an open-access article distributed under the terms of the Creative Commons Attribution License (CC BY). The use, distribution or reproduction in other forums is permitted, provided the original author(s) and the copyright owner(s) are credited and that the original publication in this journal is cited, in accordance with accepted academic practice. No use, distribution or reproduction is permitted which does not comply with these terms.
*Correspondence: Jacques Lux, SmFjcXVlcy5MdXhAVVRTb3V0aHdlc3Rlcm4uZWR1
Disclaimer: All claims expressed in this article are solely those of the authors and do not necessarily represent those of their affiliated organizations, or those of the publisher, the editors and the reviewers. Any product that may be evaluated in this article or claim that may be made by its manufacturer is not guaranteed or endorsed by the publisher.
Research integrity at Frontiers
Learn more about the work of our research integrity team to safeguard the quality of each article we publish.