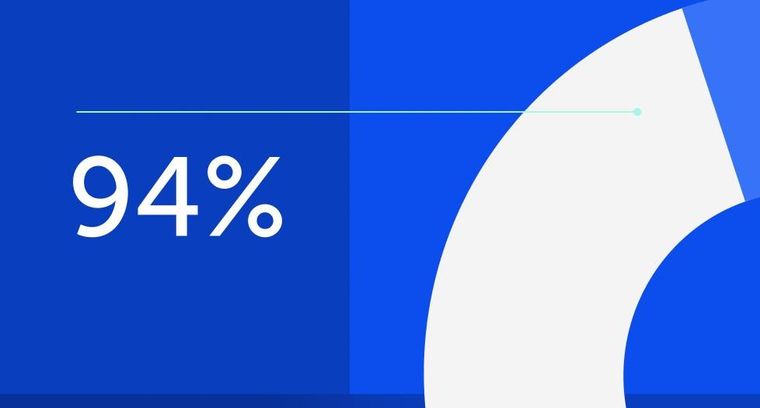
94% of researchers rate our articles as excellent or good
Learn more about the work of our research integrity team to safeguard the quality of each article we publish.
Find out more
MINI REVIEW article
Front. Cell. Neurosci., 28 March 2025
Sec. Cellular Neuropathology
Volume 19 - 2025 | https://doi.org/10.3389/fncel.2025.1552032
This article is part of the Research TopicCell-type-specific transcriptional and epigenetic regulation in substance use disorderView all 3 articles
Substance use disorder (SUD) is a chronic and relapse-prone neuropsychiatric disease characterized by impaired brain circuitry within multiple cell types and neural circuits. Recent advancements in single-cell transcriptomics, epigenetics, and neural circuit research have unveiled molecular and cellular alterations associated with SUD. These studies have provided valuable insights into the transcriptional and epigenetic regulation of neuronal and non-neuronal cells, particularly in the context of drug exposure. Critical factors influencing the susceptibility of individuals to SUD include the regulation of gene expression during early developmental stages, neuroadaptive responses to psychoactive substances, and gene–environment interactions. Here we briefly review some of these mechanisms underlying SUD, with an emphasis on their crucial roles in in neural plasticity and maintenance of addiction and relapse in neuronal and non-neuronal cell-types. We foresee the possibility of integrating multi-omics technologies to devise targeted and personalized therapeutic strategies aimed at both the prevention and treatment of SUD. By utilizing these advanced methodologies, we can gain a deeper understanding of the fundamental biology of SUD, paving the way for more effective interventions.
Substance use disorder (SUD) is a complex and highly predisposing neuropsychiatric disease, characterized by excessive drug use, tolerance, and chronic relapse (Koob and Volkow, 2010). SUD is associated with persistent and recurrent disturbances within multiple brain circuits, for example, the mesolimbic dopamine system (Koob, 1992). Exposure to drugs of abuse changes transcriptional and epigenetic regulation of neuronal and non-neuronal (e.g., Microglia) cells (Nogueira et al., 2019; Facciol et al., 2020; Bestry et al., 2024; Gonzalez-Flores et al., 2024). These are further perturbed and damaged during the development of chronic SUD and are linked to differences in predisposition to develop SUD as defined below. Here, we review transcriptional and epigenetic adaptations in different cell types in SUD in animal models and humans. We discuss how adaptations occur during different phases of SUD and are linked to individual differences in how early developmental gene expression regulation and gene–environment interactions contribute to SUD susceptibility. Finally, we present a perspective on the potential future clinical applications and therapeutic potentials.
Substance use disorder is a multifactorial disease influenced by a combination of genetic and environmental factors. Transcriptional mechanisms act to create messenger RNA (mRNA) from DNA which are then translated into proteins in cells (Dynan and Tjian, 1985). Transcription factors (TF) are proteins which regulate the expression of specific genes (Yusuf et al., 2012), but can be altered resulting from SUD. ΔFosB, for example, is a TF infamously described as a ‘molecular switch’ in SUD by overexpressing in the brain’s reward system (Nestler et al., 2001). ΔFosB is associated with drug craving and an elevated risk of relapse (Winstanley et al., 2007) and is associated with long term plastic adaptations of reward circuits which exacerbate addictive behaviors (Gajewski et al., 2019). SUD alters expression of hundreds of genes, leading to maladaptive changes at the molecular, cellular, and circuit levels, which in turn promote drug seeking behaviors (Walker et al., 2018). Early exposure to addictive substances enhances the sensitivity of reward system in the brain (Caster and Kuhn, 2009). Dopaminergic neurons exhibit distinct transcriptional responses to drug exposure during childhood and adolescence compared to adulthood, which are crucial in the development of drug dependence (Caster and Kuhn, 2009). Environmental stressors and drug exposure act synergistically to enhance the reward response to addictive substances by altering the transcriptional responses of neurons involved in stress regulation, intensifying drug cravings and dependence (Jiang et al., 2023).
Epigenetics is defined by a broad range of molecular mechanisms that regulate gene expression without altering the actual DNA sequence itself (Allis and Jenuwein, 2016; Sartor, 2019). This includes factors from the environment and individual behaviors that can alter chromatin dynamics and capabilities of DNA transcription (Conaway, 2012), which includes DNA methylation and post-translational modifications of histones (such as acetylation, methylation, and phosphorylation) (Allis and Jenuwein, 2016; Farrelly et al., 2019; Sartor, 2019). In the nervous system, DNA methylation and histone modifications are of critical importance with regard to the development and maintenance of neuronal networks, learning and memory processes, and behavioral responses to environmental stimuli (Conaway, 2012). Studies have implicated epigenetic alterations linked to persistent pathophysiological changes associated with neuronal function and disease susceptibility (Houston et al., 2013). Chronic exposure to drugs of abuse induces long-term epigenetic modifications in the reward circuit in the brain (Cheron et al., 2023), which in turn give rise to enduring functional alterations in neural circuits (Kaplan et al., 2022; Koijam et al., 2024). These modifications play a critical role in reinforcing drug-related behaviors and promote drug dependence (Hamilton and Nestler, 2019). Epigenetic adaptations play a pivotal role not only in the progression of SUD but also in determining an individual’s susceptibility to addiction. For example, gene expression regulation during early developmental stages, such as DNA methylation and histone modifications, could persist into adulthood, making individuals more susceptible to drug addiction (Browne et al., 2020). After drug exposure, epigenetic marks may leave persistent traces in the transcriptional regulation of individual neurons and influence physiological responses in subsequent stages (Nogueira et al., 2019). For example, the upregulation of certain transcription factors (such as FosB) and neurotransmitter receptor genes closely associated with mesolimbic reward plasticity changes after drug exposure (Nestler et al., 2001; Winstanley et al., 2007; Grueter et al., 2013; Robison and Nestler, 2022). These findings support theories of transgenerational ‘heritability’ of SUD (Deak and Johnson, 2021) and suggest it is both genetic and epigenetic factors that influence individual predisposition to develop SUD (Winstanley et al., 2007; Walker et al., 2018; Hamilton and Nestler, 2019).
The mesolimbic dopamine system functions to promote reward seeking in naturalistic settings, for example, for food (Parada et al., 1990; Carelli et al., 2000; Wise, 2006; Nicola, 2016), water (Parada et al., 1990; Carelli et al., 2000), and sexual reward (Fibiger, 1993; Hull et al., 2004). Drugs of abuse are thought to overwhelm these processes, and cause long term plastic adaptations in these same reward-associated brain regions (Self, 2004; Koob, 2008; Tan et al., 2024). Consequently, this results in drugs hijacking these systems and obsession with drugs despite negative associations and consequences (Volkow, 2005; Koob and Volkow, 2010; Koob, 2013b; Pickard and Ahmed, 2016). The onset and progression of SUD involves dysregulation across multiple neurotransmitter systems, which are closely linked to transcriptional and epigenetic changes in various neural cell types (Hamilton and Nestler, 2019; Nestler and Lüscher, 2019; Anderson and Taniguchi, 2022; Koijam et al., 2024). Exposure to addictive drugs leads to the transcriptional adaptations of key genes such as Brain-Derived Neurotrophic Factor (BDNF) and Activity-Regulated Cytoskeletal-associated Protein (ARC), which directly influence the synaptic strength and neural plasticity (Huggett and Stallings, 2020; Chen et al., 2024). We describe a sample of these epigenetic and transcriptional adaptations in crucial brain regions and cell-type specific changes of neurons unique to those systems.
Motivated movements are theorized to arise by way of spiraling ascending limbic projections (Haber et al., 2000). These projection systems are thought to be ‘gated’ by deep brain neurons such as those in the nucleus accumbens (NAc) (Morrison et al., 2017). NAc receives input from a multitude of regions associated with SUD (Britt et al., 2012) and are predominantly made of inhibitory GABAergic medium spiny neurons (MSNs) (Gerfen et al., 1990) thought to disinhibit downstream projections to premotor and motor cortices and guide motivated movements (Morrison et al., 2017). Drugs of abuse can alter this dynamic and cause changes to this aspect of the projection system (MacAskill et al., 2014). This results in situations where NAc neurons signal drug availability even during ‘drug-free’ states (Estrin et al., 2023). Individuals are thought to maintain a ‘preferred’ micromolar brain level of certain drugs (e.g., cocaine) by self-administering in predictable patterns to achieve a desired ‘satiety’ state (Zimmer et al., 2013). This floods the brain with drug and induces changes to the transcriptional, and epigenetic mechanisms regulating these cells (Huggett and Stallings, 2020; Chen et al., 2024).
Drug use induces a wide range of changes to the NAc transcriptional mechanisms (Nestler and Lüscher, 2019; Mews et al., 2024) including CREB (Carlezon et al., 1998; Dong et al., 2006) and FosB (Grueter et al., 2013). Epigenetic mechanisms in NAc are also disrupted during drug use (Anderson and Taniguchi, 2022). For example, DNA methylation is typically induced by DNA methyltransferases (DNMTs) (Bestor, 2000). Administration of cocaine increases DNMT3A and DNMT3B induced hypermethylation in NAc (Anier et al., 2010) and enhances behavioral sensitization but is reversed by DNMT inhibition (Anier et al., 2010). This coincides with transcriptional downregulation of protein phosphatase-1 catalytic (PP1c) promoter and upregulation of NAcΔFosB (Anier et al., 2010). Further, histone modifications in NAc (Anderson et al., 2019; Werner et al., 2021) are involved in drug-induced NAc plasticity (Maze et al., 2010; Damez-Werno et al., 2016) and are associated with heightened risk of drug relapse (Walker et al., 2015). Long-term drug use also alters NAc chromatin structure (Mews et al., 2024). MSNs exist subtypes D1 and D2 (Gerfen et al., 1990; Lobo and Nestler, 2011). Inhibition of transcription of DMNT3A2 in NAc-D1 MSNs inhibits cocaine seeking reduces inductions of genes such as Arc, FosB, and Egr2 (Bisagno and Cadet, 2019; Koijam et al., 2024). Suppression of histone methyltransferase G9a of D1-MSNs results in reduced cocaine-seeking, while D2 G9a suppression enhances cocaine seeking (Maze et al., 2014; Peña et al., 2014). Histone deacetylase-3 (HDAC3) is hyper-expressed in NAc-D1 MSNs following cocaine exposure and acts to promote cocaine seeking (Campbell et al., 2021). Conversely, downregulation of histone methylation in D2-MSNs is thought to act as a ‘break’ for development of addiction (Damez-Werno et al., 2016). Removal of BDNF receptor TrkB in D2-MSN reduces cocaine-seeking while the opposite holds true for NAc D1-MSNs (Lobo et al., 2010). Opposing D1 and D2 findings are also present regarding egr3 (a cocaine-associated gene) and cocaine-seeking behaviors (Chandra et al., 2017). These changes and adaptations are well documented and suggest drug use of any kind disturbs ‘typical’ functioning of genetic and epigenetic regulation in NAc MSNs.
The basolateral amygdala (BLA) is thought to encode a host of cognitive processes including fear (Rogan et al., 1997; Gale et al., 2004), affect (Grace and Rosenkranz, 2002), and reinforcement (Gale et al., 2004; Tye et al., 2008; Namburi et al., 2015). The BLA is also associated with motivation (Floresco and Ghods-Sharifi, 2007; Grabenhorst et al., 2012), drug-seeking (Everitt and Wolf, 2002; See, 2002; Stefanik and Kalivas, 2013), and drug craving (Bonson et al., 2002). Neurons in BLA respond to cues predictive of reward (Sugase-Miyamoto and Richmond, 2005). Excitatory glutamatergic principle output neurons are the predominant NAc-projecting neurons in the BLA (McDonald, 1992; Grace and Rosenkranz, 2002; Russo and Nestler, 2013) while BLA inhibitory GABAergic neurons are thought to modulate the activity of glutamatergic neurons (Ahmed and Paré, 2023). It is thought that BLA projects to NAc and PFC via excitatory projections (Shinonaga et al., 1994; Ambroggi et al., 2008; Stuber et al., 2011; Britt et al., 2012; Janak and Tye, 2015) theorized to be necessary for “cue-induced” reward seeking (Di Ciano and Everitt, 2004; Ambroggi et al., 2008; Shiflett and Balleine, 2010). Epigenetic influences of BLA have been shown via inhibition of DNMT reduces cue-induced heroin seeking (Qian et al., 2022), while inhibition of histone deacetylase (HDAC) enhances morphine-seeking and enhances activation of BDNF, ΔFosB, and CREB (Wang et al., 2015). In general, BLA gene expression is ‘globally’ altered in humans suffering from alcoholism resulting from histone modification and DNA hypomethylation (Ponomarev et al., 2012). Further, heightened BLA c-FOS and mRNA changes associated with pyramidal cells have been modeled in rats exposed to alcohol during adolescence (Vázquez-Ágredos et al., 2024).
The prefrontal cortex (PFC) is associated with drug seeking behavior (Glanzberg et al., 2024). The PFC is a crucial component involved in feed-forward limbic processing. For instance, PFC receives BLA signals (Burgos-Robles et al., 2017; Crofton et al., 2022) and transmits excitatory projections with BLA and NAc (Ambroggi et al., 2008; Stuber et al., 2011; Otis et al., 2017; Li et al., 2023) during reward seeking. The neural circuit dynamics between these critical brain regions have been demonstrated to facilitate motivated responding (Ambroggi et al., 2008; Ghazizadeh et al., 2012; Tomasi and Volkow, 2013; Thomas et al., 2020). Early-life cocaine exposure alters a host of PFC gene expression that appeared as early as day 1 (e.g., Egr1/2, and Jmjd1a), while some genes (and histone methylation) endured into adulthood (Black et al., 2006). Chronic cocaine addiction promotes PFC DNA methylation at BDNF promoter regions in humans (Poisel et al., 2023), and histone acetylation in rat DNA increases BDNF transcription in the PFC (Sadri-Vakili et al., 2010). Morphine withdrawal is associated with epigenetic regulation of BDNF via chromatin remodeling (Wang et al., 2012). Early alcohol exposure increases histone acetylation at the PFC BDNF promotor and transcription sites (Montesinos et al., 2016). Neurons in the PFC include the excitatory projection pyramidal cells (Krimer and Goldman-Rakic, 2001) and the inhibitory interneurons that act to modulate the local activity of pyramidal cell signaling (Pouille and Scanziani, 2001; Isaacson and Scanziani, 2011; Hu et al., 2014; Ferguson and Gao, 2018). Morphine administration disinhibits these inhibitory neurons and enhances reward (Jiang et al., 2021). Interestingly, direct infusion of BDNF into PFC reduces drug seeking by modulating pyramidal glutamatergic signals to NAc (Berglind et al., 2009; Whitfield et al., 2011). Supporting this notion, ‘knockdown’ of PFC BDNF amplifies cocaine seeking via the same glutamatergic process (Sadri-Vakili et al., 2010). Heroin use in humans is associated with altered DNA methylation and glutamatergic plasticity in the PFC (Kozlenkov et al., 2017). Finally, human genome-wide ‘addiction-risk’ assessments found chromatin modulations and enhanced susceptibility for initiation of cannabis use and cigarette smoking among excitatory, but not inhibitory, neurons in PFC (Kozlenkov et al., 2017; Barker and Hines, 2020; Huggett and Stallings, 2020; Sundar et al., 2021; Chen et al., 2024).
In addition to neurons, glia is an additional subtype of cells involved in typical mammalian neurophysiology. In the central nervous system, major glia cell-types typically include astrocytes, microglia, and oligodendrocytes (Silver et al., 2015). They are essential for a host of critical supportive neurological processes and are impacted by drugs of abuse. We present a brief summary of epigenetic and transcriptional changes in glia below.
Astrocytes maintain homeostasis in the brain by maintaining the blood brain barrier, regulating synaptic transmission, neurotransmitter concentrations, energy metabolism (Lee et al., 2022). Following drug exposure, astrocytes exhibit significant transcriptional and epigenetic adaptations that affect the plasticity and function of neural circuits, which highlights the complexity and multifaceted nature of SUD (Bachtell et al., 2017). When exposed to pharmacological agents, astrocytes undergo transcriptional adaptations primarily related to glutamate transport, energy metabolism, and inflammatory responses (Holt and Nestler, 2024). Prolonged exposure to pharmacological agents reduces in the expression of the glutamate transporter (GLT-1, also known as EAAT2), leading to the elevated extracellular glutamate concentrations, which induce neurotoxicity and disrupt synaptic plasticity (Kruyer and Kalivas, 2021). Astrocytes support neuronal energy demands through the lactate-glucose-glutamate metabolic cycle (Petit and Magistretti, 2015). Drug exposure may exert an influence on this process through transcriptional regulation (Mahna et al., 2018). For instance, following alcohol exposure, the expression of monocarboxylate transporters (MCT1 and MCT2) in astrocytes is downregulated, which results in an imbalance in lactate metabolism and may relate to the development of drug dependence (Tan et al., 2022).
Microglia are seen as the principal immune cells in the central nervous system (Aloisi, 2001). Microglia maintain brain homeostasis by clearing damaged tissue, releasing inflammatory factors in response to injury and infection, and by facilitating neural circuit repair (Aloisi, 2001; Borst et al., 2021). However, chronic drug exposure induces excessive microglia activation (Li et al., 2024). This is theorized to lead to a reinforcing inflammatory state characterized by release of inflammatory factors and recruitment of additional immune cells (i.e., chronic inflammation) (Loftis et al., 2011; Chan et al., 2015; Piepenbrink et al., 2016; Prakash et al., 2017) associated with sickness behavior which mirrors human reports of depression (Dantzer et al., 2008). People suffering from SUD report high rates of depression (Koob, 2013a; Sullivan, 2018) and drugs of abuse temporarily suppress the activation of certain pro-inflammatory cytokines (Bayer et al., 1995; Baldwin et al., 1998), theoretically providing short-term relief. However, these result in long-term hyperactive responses to sickness (Buchanan et al., 2010; Loftis et al., 2011) and immune activation in response to drug withdrawal (Wang et al., 2021). Microglial activation is frequently accompanied by a substantial upregulation of the NFκB signaling pathway, which regulates the expression of a number of genes involved in the inflammatory response, including IL-6 and TNF-α (Jones, 2020). In chronic opioid exposure models, an elevated expression of IL-6 in the microglia has been strongly correlated with the disruption of synaptic plasticity in the nucleus accumbens, which may represent an important molecular mechanism underlying drug craving (Guo et al., 2023). Therefore, it is likely that inflammation changes neuronal communication which leads to changes in behavior and mood. These changes can make it difficult for individuals to stop using opioids (Guo et al., 2023) and contributes to negative reinforcing aspects of addiction (Koob, 2013b; Jones, 2020; Guo et al., 2023).
Oligodendrocytes are thought to promote myelination of axons in the central nervous system (Stadelmann et al., 2019). Recent research indicates myelin integrity is closely associated with neuronal function in diseases such as multiple sclerosis (Steinman, 1996). Myelin integrity is linked to an array of neurobehavioral dysfunctions and compulsive behavioral disorders (including SUD) (Sequeira and Turecki, 2006; Nave and Ehrenreich, 2014). Binge-eating, for instance, results in an altered transcriptome and downregulation of oligodendrocytes (Kirkpatrick et al., 2017; Babbs et al., 2020) and impacts reward-related behavior (Babbs et al., 2020). Finally, alcoholism is associated with altered transcription factors and histone modifications resulting in gene expression alteration in oligodendrocytes (Miguel-Hidalgo, 2018).
In recent years, the application of single-cell transcriptomics has provided new opportunities to explore cell-type-specific transcriptional adaptations in substance use disorder (SUD). By conducting high-precision analysis of the transcriptomes of individual cells, researchers are now able to reveal the dynamic changes in different cell populations after drug exposure and identify key genes and molecular pathways associated with addiction progression and susceptibility. Single-cell RNA sequencing (scRNA-seq) enables the differentiation of gene expression changes across heterogeneous cell populations, particularly in complex brain regions such as the nucleus accumbens, hippocampus, and dorsolateral prefrontal cortex, which are important in the pathogenesis of SUD (Tran et al., 2021).
Moreover, epigenetic research has provided new perspectives on the regulatory mechanisms underlying transcriptional adaptations. Drug exposure not only affects gene transcription levels but also influences transcriptional adaptations in specific cell types by altering epigenetic marks, including DNA methylation and histone modifications (Cheron et al., 2023),which adds complexity to our understanding of how drugs reshape the transcriptional landscape of the brain. Future studies can combine epigenetics with single-cell genomics to explore how epigenetic regulation affects cell-type-specific transcriptional adaptations, ultimately influencing the progression of SUD and individual susceptibility (Nohesara et al., 2024). This multidisciplinary approach holds the potential to unveil novel therapeutic targets and personalized intervention strategies for SUD.
A deeper understanding of how specific cell-type transcriptional and epigenetic adaptations to the progression and susceptibility of SUD will provide new therapeutic targets for precision medicine. For instance, by regulating the expression of addiction-related transcription factors (e.g., ΔFosB) and targeting epigenetic modifications in non-neuronal cells may facilitate the development of more precise drugs to reverse the molecular changes induced by drug exposure (Robison and Nestler, 2022). Additionally, treatment strategies based on non-coding RNAs (e.g., miRNAs) and the application of CRISPR technology has opened new avenues for the personalized treatment of addictive disorders (Tan et al., 2024).
Moving forward, multi-omics and cutting-edge molecular techniques to deeply probe will offer new insights into dynamic alterations in various cell types across the addiction cycle. It will pave the way for the development of more targeted strategies for precise intervention and individualized treatment of SUD.
In summary, SUD is a multifaceted neuropsychiatric disease resulting from complex interactions between genetic and epigenetic factors in neuronal and non-neuronal cells. The transcriptional and epigenetic adaptations in both neuronal and non-neuronal cells play a crucial role in the onset, progression, and relapse of SUD. Recent advancements in single-cell transcriptomics and epigenetics have provided valuable insights into the cell-type-specific molecular changes underlying these processes. Understanding the early developmental gene expression regulation, drug-induced neuroadaptive responses, and gene–environment interactions is essential for identifying new biomarkers and therapeutic targets. By integrating multi-omics technologies and advanced molecular tools, future research will lead to more effective, individualized approaches for preventing and treating SUD, paving the way for improved clinical outcomes.
BW: Writing – original draft, Writing – review & editing. JW: Writing – original draft, Writing – review & editing. NB: Writing – review & editing. D-TL: Writing – review & editing. YZ: Conceptualization, Supervision, Writing – review & editing.
The author(s) declare that financial support was received for the research and/or publication of this article. BW, JW, and YZ are supported by National Natural Science Foundation of China (82471512). NB and D-TL are supported by NIH NIDA IRP.
The authors declare that the research was conducted in the absence of any commercial or financial relationships that could be construed as a potential conflict of interest.
The author(s) declare that no Gen AI was used in the creation of this manuscript.
All claims expressed in this article are solely those of the authors and do not necessarily represent those of their affiliated organizations, or those of the publisher, the editors and the reviewers. Any product that may be evaluated in this article, or claim that may be made by its manufacturer, is not guaranteed or endorsed by the publisher.
Ahmed, N., and Paré, D. (2023). The basolateral amygdala sends a mixed (GABAergic and glutamatergic) projection to the mediodorsal thalamic nucleus. J. Neurosci. 43, 2104–2115. doi: 10.1523/JNEUROSCI.1924-22.2022
Allis, C. D., and Jenuwein, T. (2016). The molecular hallmarks of epigenetic control. Nat. Rev. Genet. 17, 487–500. doi: 10.1038/nrg.2016.59
Ambroggi, F., Ishikawa, A., Fields, H. L., and Nicola, S. M. (2008). Basolateral amygdala neurons facilitate reward-seeking behavior by exciting nucleus accumbens neurons. Neuron 59, 648–661. doi: 10.1016/j.neuron.2008.07.004
Anderson, E. M., Penrod, R. D., Barry, S. M., Hughes, B. W., Taniguchi, M., and Cowan, C. W. (2019). It is a complex issue: emerging connections between epigenetic regulators in drug addiction. Eur. J. Neurosci. 50, 2477–2491. doi: 10.1111/ejn.14170
Anderson, E. M., and Taniguchi, M. (2022). Epigenetic effects of addictive drugs in the nucleus accumbens. Front. Mol. Neurosci. 15:828055. doi: 10.3389/fnmol.2022.828055
Anier, K., Malinovskaja, K., Aonurm-Helm, A., Zharkovsky, A., and Kalda, A. (2010). DNA methylation regulates cocaine-induced behavioral sensitization in mice. Neuropsychopharmacology 35, 2450–2461. doi: 10.1038/npp.2010.128
Babbs, R. K., Beierle, J. A., Yao, E. J., Kelliher, J. C., Medeiros, A. R., Anandakumar, J., et al. (2020). The effect of the demyelinating agent cuprizone on binge-like eating of sweetened palatable food in female and male C57BL/6 substrains. Appetite 150:104678. doi: 10.1016/j.appet.2020.104678
Bachtell, R. K., Jones, J. D., Heinzerling, K. G., Beardsley, P. M., and Comer, S. D. (2017). Glial and neuroinflammatory targets for treating substance use disorders. Drug Alcohol Depend. 180, 156–170. doi: 10.1016/j.drugalcdep.2017.08.003
Baldwin, G. C., Roth, M. D., and Tashkin, D. P. (1998). Acute and chronic effects of cocaine on the immune system and the possible link to AIDS. J. Neuroimmunol. 83, 133–138. doi: 10.1016/S0165-5728(97)00229-4
Barker, J. S., and Hines, R. M. (2020). Regulation of GABA(a) receptor subunit expression in substance use disorders. Int. J. Mol. Sci. 21:4445. doi: 10.3390/ijms21124445
Bayer, B. M., Mulroney, S. E., Hernandez, M. C., and Ding, X. Z. (1995). Acute infusions of cocaine result in time-and dose-dependent effects on lymphocyte responses and corticosterone secretion in rats. Immunopharmacology 29, 19–28. doi: 10.1016/0162-3109(95)00040-Z
Berglind, W. J., Whitfield, T. W., Lalumiere, R. T., Kalivas, P. W., and Mcginty, J. F. (2009). A single intra-PFC infusion of BDNF prevents cocaine-induced alterations in extracellular glutamate within the nucleus accumbens. J. Neurosci. 29, 3715–3719. doi: 10.1523/JNEUROSCI.5457-08.2009
Bestor, T. H. (2000). The DNA methyltransferases of mammals. Hum. Mol. Genet. 9, 2395–2402. doi: 10.1093/hmg/9.16.2395
Bestry, M., Larcombe, A. N., Kresoje, N., Chivers, E. K., Bakker, C., Fitzpatrick, J. P., et al. (2024). Early moderate prenatal alcohol exposure and maternal diet impact offspring DNA methylation across species. eLife 12:RP92135.3. doi: 10.7554/eLife.92135.3
Bisagno, V., and Cadet, J. L. (2019). Expression of immediate early genes in brain reward circuitries: differential regulation by psychostimulant and opioid drugs. Neurochem. Int. 124, 10–18. doi: 10.1016/j.neuint.2018.12.004
Black, Y. D., Maclaren, F. R., Naydenov, A. V., Carlezon, W. A., Baxter, M. G., and Konradi, C. (2006). Altered attention and prefrontal cortex gene expression in rats after binge-like exposure to cocaine during adolescence. J. Neurosci. 26, 9656–9665. doi: 10.1523/JNEUROSCI.2391-06.2006
Bonson, K. R., Grant, S. J., Contoreggi, C. S., Links, J. M., Metcalfe, J., Weyl, H. L., et al. (2002). Neural systems and cue-induced cocaine craving. Neuropsychopharmacology 26, 376–386. doi: 10.1016/S0893-133X(01)00371-2
Borst, K., Dumas, A. A., and Prinz, M. (2021). Microglia: immune and non-immune functions. Immunity 54, 2194–2208. doi: 10.1016/j.immuni.2021.09.014
Britt, J. P., Benaliouad, F., Mcdevitt, R. A., Stuber, G. D., Wise, R. A., and Bonci, A. (2012). Synaptic and behavioral profile of multiple glutamatergic inputs to the nucleus accumbens. Neuron 76, 790–803. doi: 10.1016/j.neuron.2012.09.040
Browne, C. J., Godino, A., Salery, M., and Nestler, E. J. (2020). Epigenetic mechanisms of opioid addiction. Biol. Psychiatry 87, 22–33. doi: 10.1016/j.biopsych.2019.06.027
Buchanan, J. B., Sparkman, N. L., and Johnson, R. W. (2010). A neurotoxic regimen of methamphetamine exacerbates the febrile and neuroinflammatory response to a subsequent peripheral immune stimulus. J. Neuroinflammation 7, 82–89. doi: 10.1186/1742-2094-7-82
Burgos-Robles, A., Kimchi, E. Y., Izadmehr, E. M., Porzenheim, M. J., Ramos-Guasp, W. A., Nieh, E. H., et al. (2017). Amygdala inputs to prefrontal cortex guide behavior amid conflicting cues of reward and punishment. Nat. Neurosci. 20, 824–835. doi: 10.1038/nn.4553
Campbell, R., Kramár, E., Pham, L., Beardwood, J., Augustynski, A., López, A., et al. (2021). HDAC3 activity within the nucleus accumbens regulates cocaine-induced plasticity and behavior in a cell-type-specific manner. J. Neurosci. 41, 2814–2827. doi: 10.1523/JNEUROSCI.2829-20.2021
Carelli, R. M., Ijames, S. G., and Crumling, A. J. (2000). Evidence that separate neural circuits in the nucleus accumbens encode cocaine versus “natural” (water and food) reward. J. Neurosci. 20, 4255–4266. doi: 10.1523/JNEUROSCI.20-11-04255.2000
Carlezon, W. A. Jr., Thome, J., Olson, V. G., Lane-Ladd, S. B., Brodkin, E. S., Hiroi, N., et al. (1998). Regulation of cocaine reward by CREB. Science 282, 2272–2275. doi: 10.1126/science.282.5397.2272
Caster, J. M., and Kuhn, C. M. (2009). Maturation of coordinated immediate early gene expression by cocaine during adolescence. Neuroscience 160, 13–31. doi: 10.1016/j.neuroscience.2009.01.001
Chan, Y.-Y., Yang, S.-N., Lin, J.-C., Chang, J.-L., Lin, J.-G., and Lo, W.-Y. (2015). Inflammatory response in heroin addicts undergoing methadone maintenance treatment. Psychiatry Res. 226, 230–234. doi: 10.1016/j.psychres.2014.12.053
Chandra, R., Engeln, M., Francis, T. C., Konkalmatt, P., Patel, D., and Lobo, M. K. (2017). A role for peroxisome proliferator-activated receptor gamma coactivator-1α in nucleus accumbens neuron subtypes in cocaine action. Biol. Psychiatry 81, 564–572. doi: 10.1016/j.biopsych.2016.10.024
Chen, J., Zhou, Y., Lai, M., Zhang, Y., Hu, Y., Zhuang, D., et al. (2024). Antidepressant effects of activation of infralimbic cortex via upregulation of BDNF and beta-catenin in an estradiol withdrawal model. Psychopharmacology 241, 1923–1935. doi: 10.1007/s00213-024-06610-z
Cheron, J., Beccari, L., Hague, P., Icick, R., Despontin, C., Carusone, T., et al. (2023). USP7/Maged1-mediated H2A monoubiquitination in the paraventricular thalamus: an epigenetic mechanism involved in cocaine use disorder. Nat. Commun. 14:8481. doi: 10.1038/s41467-023-44120-2
Conaway, J. W. (2012). Introduction to theme “chromatin, epigenetics, and transcription”. Annu. Rev. Biochem. 81, 61–64. doi: 10.1146/annurev-biochem-090711-093103
Crofton, E. J., Zhu, M., Curtis, K. N., Nolan, G. W., O'buckley, T. K., Morrow, A. L., et al. (2022). Medial prefrontal cortex-basolateral amygdala circuit dysfunction in chronic alcohol-exposed male rats. Neuropharmacology 205:108912. doi: 10.1016/j.neuropharm.2021.108912
Damez-Werno, D. M., Sun, H., Scobie, K. N., Shao, N., Rabkin, J., Dias, C., et al. (2016). Histone arginine methylation in cocaine action in the nucleus accumbens. Proc. Natl. Acad. Sci. 113, 9623–9628. doi: 10.1073/pnas.1605045113
Dantzer, R., O'connor, J. C., Freund, G. G., Johnson, R. W., and Kelley, K. W. (2008). From inflammation to sickness and depression: when the immune system subjugates the brain. Nat. Rev. Neurosci. 9, 46–56. doi: 10.1038/nrn2297
Deak, J. D., and Johnson, E. C. (2021). Genetics of substance use disorders: a review. Psychol. Med. 51, 2189–2200. doi: 10.1017/S0033291721000969
Di Ciano, P., and Everitt, B. J. (2004). Direct interactions between the basolateral amygdala and nucleus accumbens core underlie cocaine-seeking behavior by rats. J. Neurosci. 24, 7167–7173. doi: 10.1523/JNEUROSCI.1581-04.2004
Dong, Y., Green, T., Saal, D., Marie, H., Neve, R., Nestler, E. J., et al. (2006). CREB modulates excitability of nucleus accumbens neurons. Nat. Neurosci. 9, 475–477. doi: 10.1038/nn1661
Dynan, W. S., and Tjian, R. (1985). Control of eukaryotic messenger RNA synthesis by sequence-specific DNA-binding proteins. Nature 316, 774–778. doi: 10.1038/316774a0
Estrin, D. J., Kulik, J. M., Beacher, N. J., Pawlak, A. P., Klein, S. D., and West, M. O. (2023). Acquired alterations in nucleus accumbens responsiveness to a cocaine-paired discriminative stimulus preceding rats’ daily cocaine consumption. Addict. Neurosci. 8:100121. doi: 10.1016/j.addicn.2023.100121
Everitt, B. J., and Wolf, M. E. (2002). Psychomotor stimulant addiction: a neural systems perspective. J. Neurosci. 22, 3312–3320. doi: 10.1523/JNEUROSCI.22-09-03312.2002
Facciol, A., Bailleul, C., Nguyen, S., Chatterjee, D., and Gerlai, R. (2020). Developmental stage-dependent deficits induced by embryonic ethanol exposure in zebrafish: a neurochemical analysis. Prog. Neuro-Psychopharmacol. Biol. Psychiatry 99:109859. doi: 10.1016/j.pnpbp.2020.109859
Farrelly, L. A., Thompson, R. E., Zhao, S., Lepack, A. E., Lyu, Y., Bhanu, N. V., et al. (2019). Histone serotonylation is a permissive modification that enhances TFIID binding to H3K4me3. Nature 567, 535–539. doi: 10.1038/s41586-019-1024-7
Ferguson, B. R., and Gao, W.-J. (2018). PV interneurons: critical regulators of E/I balance for prefrontal cortex-dependent behavior and psychiatric disorders. Front. Neural Circuits 12:37. doi: 10.3389/fncir.2018.00037
Fibiger, H. C. (1993). Mesolimbic dopamine: An analysis of its role in motivated behavior. Semin. Neurosci. 5, 321–327. doi: 10.1016/S1044-5765(05)80039-9
Floresco, S. B., and Ghods-Sharifi, S. (2007). Amygdala-prefrontal cortical circuitry regulates effort-based decision making. Cereb. Cortex 17, 251–260. doi: 10.1093/cercor/bhj143
Gajewski, P. A., Eagle, A. L., Williams, E. S., Manning, C. E., Lynch, H., Mccornack, C., et al. (2019). Epigenetic regulation of hippocampal Fosb expression controls behavioral responses to cocaine. J. Neurosci. 39, 8305–8314. doi: 10.1523/JNEUROSCI.0800-19.2019
Gale, G. D., Anagnostaras, S. G., Godsil, B. P., Mitchell, S., Nozawa, T., Sage, J. R., et al. (2004). Role of the basolateral amygdala in the storage of fear memories across the adult lifetime of rats. J. Neurosci. 24, 3810–3815. doi: 10.1523/JNEUROSCI.4100-03.2004
Gerfen, C. R., Engber, T. M., Mahan, L. C., Susel, Z., Chase, T. N., Monsma, F. J., et al. (1990). D1 and D2 dopamine receptor-regulated gene expression of striatonigral and striatopallidal neurons. Science 250, 1429–1432. doi: 10.1126/science.2147780
Ghazizadeh, A., Ambroggi, F., Odean, N., and Fields, H. L. (2012). Prefrontal cortex mediates extinction of responding by two distinct neural mechanisms in accumbens shell. J. Neurosci. 32, 726–737. doi: 10.1523/JNEUROSCI.3891-11.2012
Glanzberg, J. T., Denman, A. J., Beacher, N. J., Broomer, M. C., Liang, B., Li, Y., et al. (2024). Individual differences in prelimbic neural representation of food and cocaine seeking. Cell Rep. 43:115022. doi: 10.1016/j.celrep.2024.115022
Gonzalez-Flores, D., Marquez, A., and Casimiro, I. (2024). Oxidative effects in early stages of embryo development due to alcohol consumption. Int. J. Mol. Sci. 25:4100. doi: 10.3390/ijms25074100
Grabenhorst, F., Hernádi, I., and Schultz, W. (2012). Prediction of economic choice by primate amygdala neurons. Proc. Natl. Acad. Sci. 109, 18950–18955. doi: 10.1073/pnas.1212706109
Grace, A. A., and Rosenkranz, J. A. (2002). Regulation of conditioned responses of basolateral amygdala neurons. Physiol. Behav. 77, 489–493. doi: 10.1016/S0031-9384(02)00909-5
Grueter, B. A., Robison, A. J., Neve, R. L., Nestler, E. J., and Malenka, R. C. (2013). ∆ FosB differentially modulates nucleus accumbens direct and indirect pathway function. Proc. Natl. Acad. Sci. 110, 1923–1928. doi: 10.1073/pnas.1221742110
Guo, M. L., Roodsari, S. K., Cheng, Y., Dempsey, R. E., and Hu, W. (2023). Microglia NLRP3 Inflammasome and Neuroimmune signaling in substance use disorders. Biomol. Ther. 13:922. doi: 10.3390/biom13060922
Haber, S. N., Fudge, J. L., and Mcfarland, N. R. (2000). Striatonigrostriatal pathways in primates form an ascending spiral from the shell to the dorsolateral striatum. J. Neurosci. 20, 2369–2382. doi: 10.1523/JNEUROSCI.20-06-02369.2000
Hamilton, P. J., and Nestler, E. J. (2019). Epigenetics and addiction. Curr. Opin. Neurobiol. 59, 128–136. doi: 10.1016/j.conb.2019.05.005
Holt, L. M., and Nestler, E. J. (2024). Astrocytic transcriptional and epigenetic mechanisms of drug addiction. J. Neural Transm. 131, 409–424. doi: 10.1007/s00702-023-02716-4
Houston, I., Peter, C. J., Mitchell, A., Straubhaar, J., Rogaev, E., and Akbarian, S. (2013). Epigenetics in the human brain. Neuropsychopharmacology 38, 183–197. doi: 10.1038/npp.2012.78
Hu, H., Gan, J., and Jonas, P. (2014). Fast-spiking, parvalbumin+ GABAergic interneurons: from cellular design to microcircuit function. Science 345:1255263. doi: 10.1126/science.1255263
Huggett, S. B., and Stallings, M. C. (2020). Genetic architecture and molecular neuropathology of human cocaine addiction. J. Neurosci. 40, 5300–5313. doi: 10.1523/JNEUROSCI.2879-19.2020
Hull, E. M., Muschamp, J. W., and Sato, S. (2004). Dopamine and serotonin: influences on male sexual behavior. Physiol. Behav. 83, 291–307. doi: 10.1016/j.physbeh.2004.08.018
Isaacson, J. S., and Scanziani, M. (2011). How inhibition shapes cortical activity. Neuron 72, 231–243. doi: 10.1016/j.neuron.2011.09.027
Janak, P. H., and Tye, K. M. (2015). From circuits to behaviour in the amygdala. Nature 517, 284–292. doi: 10.1038/nature14188
Jiang, Z., Chen, Z., and Chen, X. (2023). Candidate gene-environment interactions in substance abuse: a systematic review. PLoS One 18:e0287446. doi: 10.1371/journal.pone.0287446
Jiang, C., Wang, X., Le, Q., Liu, P., Liu, C., Wang, Z., et al. (2021). Morphine coordinates SST and PV interneurons in the prelimbic cortex to disinhibit pyramidal neurons and enhance reward. Mol. Psychiatry 26, 1178–1193. doi: 10.1038/s41380-019-0480-7
Jones, J. D. (2020). Potential of glial cell modulators in the Management of Substance use Disorders. CNS Drugs 34, 697–722. doi: 10.1007/s40263-020-00721-9
Kaplan, G., Xu, H., Abreu, K., and Feng, J. (2022). DNA epigenetics in addiction susceptibility. Front. Genet. 13:806685. doi: 10.3389/fgene.2022.806685
Kirkpatrick, S. L., Goldberg, L. R., Yazdani, N., Babbs, R. K., Wu, J., Reed, E. R., et al. (2017). Cytoplasmic FMR1-interacting protein 2 is a major genetic factor underlying binge eating. Biol. Psychiatry 81, 757–769. doi: 10.1016/j.biopsych.2016.10.021
Koijam, A. S., Singh, K. D., Nameirakpam, B. S., Haobam, R., and Rajashekar, Y. (2024). Drug addiction and treatment: an epigenetic perspective. Biomed. Pharmacother. 170:115951. doi: 10.1016/j.biopha.2023.115951
Koob, G. F. (1992). Dopamine, addiction and reward. Semin. Neurosci. 4, 139–148. doi: 10.1016/1044-5765(92)90012-Q
Koob, G. F. (2008). Hedonic homeostatic dysregulation as a driver of drug-seeking behavior. Drug Discov. Today Dis. Model. 5, 207–215. doi: 10.1016/j.ddmod.2009.04.002
Koob, G. F. (2013a). Addiction is a reward deficit and stress surfeit disorder. Front. Psych. 4:72. doi: 10.3389/fpsyt.2013.00072
Koob, G. F. (2013b). Negative reinforcement in drug addiction: the darkness within. Curr. Opin. Neurobiol. 23, 559–563. doi: 10.1016/j.conb.2013.03.011
Koob, G. F., and Volkow, N. D. (2010). Neurocircuitry of addiction. Neuropsychopharmacology 35, 217–238. doi: 10.1038/npp.2009.110
Kozlenkov, A., Jaffe, A. E., Timashpolsky, A., Apontes, P., Rudchenko, S., Barbu, M., et al. (2017). DNA methylation profiling of human prefrontal cortex neurons in heroin users shows significant difference between genomic contexts of hyper-and hypomethylation and a younger epigenetic age. Genes 8:152. doi: 10.3390/genes8060152
Krimer, L. S., and Goldman-Rakic, P. S. (2001). Prefrontal microcircuits: membrane properties and excitatory input of local, medium, and wide arbor interneurons. J. Neurosci. 21, 3788–3796. doi: 10.1523/JNEUROSCI.21-11-03788.2001
Kruyer, A., and Kalivas, P. W. (2021). Astrocytes as cellular mediators of cue reactivity in addiction. Curr. Opin. Pharmacol. 56, 1–6. doi: 10.1016/j.coph.2020.07.009
Lee, H.-G., Wheeler, M. A., and Quintana, F. J. (2022). Function and therapeutic value of astrocytes in neurological diseases. Nat. Rev. Drug Discov. 21, 339–358. doi: 10.1038/s41573-022-00390-x
Li, H., Watkins, L. R., and Wang, X. (2024). Microglia in neuroimmunopharmacology and drug addiction. Mol. Psychiatry 29, 1912–1924. doi: 10.1038/s41380-024-02443-6
Li, J.-Y., Yu, Y.-J., Su, C.-L., Shen, Y.-Q., Chang, C.-H., and Gean, P.-W. (2023). Modulation of methamphetamine memory reconsolidation by neural projection from basolateral amygdala to nucleus accumbens. Neuropsychopharmacology 48, 478–488. doi: 10.1038/s41386-022-01417-y
Lobo, M. K., Covington Iii, H. E., Chaudhury, D., Friedman, A. K., Sun, H., Damez-Werno, D., et al. (2010). Cell type–specific loss of BDNF signaling mimics optogenetic control of cocaine reward. Science 330, 385–390. doi: 10.1126/science.1188472
Lobo, M. K., and Nestler, E. J. (2011). The striatal balancing act in drug addiction: distinct roles of direct and indirect pathway medium spiny neurons. Front. Neuroanat. 5:41. doi: 10.3389/fnana.2011.00041
Loftis, J. M., Choi, D., Hoffman, W., and Huckans, M. S. (2011). Methamphetamine causes persistent immune dysregulation: a cross-species, translational report. Neurotox. Res. 20, 59–68. doi: 10.1007/s12640-010-9223-x
Macaskill, A. F., Cassel, J. M., and Carter, A. G. (2014). Cocaine exposure reorganizes cell type–and input-specific connectivity in the nucleus accumbens. Nat. Neurosci. 17, 1198–1207. doi: 10.1038/nn.3783
Mahna, D., Puri, S., and Sharma, S. (2018). DNA methylation signatures: biomarkers of drug and alcohol abuse. Mutat. Res. Rev. Mutat. Res. 777, 19–28. doi: 10.1016/j.mrrev.2018.06.002
Maze, I., Chaudhury, D., Dietz, D. M., Von Schimmelmann, M., Kennedy, P. J., Lobo, M. K., et al. (2014). G9a influences neuronal subtype specification in striatum. Nat. Neurosci. 17, 533–539. doi: 10.1038/nn.3670
Maze, I., Covington Iii, H. E., Dietz, D. M., Laplant, Q., Renthal, W., Russo, S. J., et al. (2010). Essential role of the histone methyltransferase G9a in cocaine-induced plasticity. Science 327, 213–216. doi: 10.1126/science.1179438
McDonald, A. (1992). “Cell types and intrinsic connections of the amygdala” in The amygdala: neurobiological aspects of emotion, memory, and mental dysfunction. ed. J. P. Aggleton, 67–96. Available at: https://psycnet.apa.org/record/1992-97763-000.
Mews, P., Van Der Zee, Y., Gurung, A., Estill, M., Futamura, R., Kronman, H., et al. (2024). Cell type–specific epigenetic priming of gene expression in nucleus accumbens by cocaine. Sci. Adv. 10:eado3514. doi: 10.1126/sciadv.ado3514
Miguel-Hidalgo, J. J. (2018). Molecular neuropathology of astrocytes and oligodendrocytes in alcohol use disorders. Front. Mol. Neurosci. 11:78. doi: 10.3389/fnmol.2018.00078
Montesinos, J., Pascual, M., Rodríguez-Arias, M., Miñarro, J., and Guerri, C. (2016). Involvement of TLR4 in the long-term epigenetic changes, rewarding and anxiety effects induced by intermittent ethanol treatment in adolescence. Brain Behav. Immun. 53, 159–171. doi: 10.1016/j.bbi.2015.12.006
Morrison, S. E., Mcginty, V. B., Du Hoffmann, J., and Nicola, S. M. (2017). Limbic-motor integration by neural excitations and inhibitions in the nucleus accumbens. J. Neurophysiol. 118, 2549–2567. doi: 10.1152/jn.00465.2017
Namburi, P., Beyeler, A., Yorozu, S., Calhoon, G. G., Halbert, S. A., Wichmann, R., et al. (2015). A circuit mechanism for differentiating positive and negative associations. Nature 520, 675–678. doi: 10.1038/nature14366
Nave, K.-A., and Ehrenreich, H. (2014). Myelination and oligodendrocyte functions in psychiatric diseases. JAMA Psychiatry 71, 582–584. doi: 10.1001/jamapsychiatry.2014.189
Nestler, E. J., Barrot, M., and Self, D. W. (2001). ΔFosB: a sustained molecular switch for addiction. Proc. Natl. Acad. Sci. 98, 11042–11046. doi: 10.1073/pnas.191352698
Nestler, E. J., and Lüscher, C. (2019). The molecular basis of drug addiction: linking epigenetic to synaptic and circuit mechanisms. Neuron 102, 48–59. doi: 10.1016/j.neuron.2019.01.016
Nicola, S. M. (2016). Reassessing wanting and liking in the study of mesolimbic influence on food intake. Am. J. Phys. Regul. Integr. Comp. Phys. 311, R811–R840. doi: 10.1152/ajpregu.00234.2016
Nogueira, D. D. S., Merienne, K., and Befort, K. (2019). Neuroepigenetics and addictive behaviors: where do we stand? Neurosci. Biobehav. Rev. 106, 58–72. doi: 10.1016/j.neubiorev.2018.08.018
Nohesara, S., Mostafavi Abdolmaleky, H., and Thiagalingam, S. (2024). Substance-induced psychiatric disorders, epigenetic and microbiome alterations, and potential for therapeutic interventions. Brain Sci. 14:769. doi: 10.3390/brainsci14080769
Otis, J. M., Namboodiri, V. M., Matan, A. M., Voets, E. S., Mohorn, E. P., Kosyk, O., et al. (2017). Prefrontal cortex output circuits guide reward seeking through divergent cue encoding. Nature 543, 103–107. doi: 10.1038/nature21376
Parada, M. A., Hernandez, L., De Parada, M. P., Paez, X., and Hoebel, B. G. (1990). Dopamine in the lateral hypothalamus may be involved in the inhibition of locomotion related to food and water seeking. Brain Res. Bull. 25, 961–968. doi: 10.1016/0361-9230(90)90195-6
Peña, C. J., Bagot, R. C., Labonté, B., and Nestler, E. J. (2014). Epigenetic signaling in psychiatric disorders. J. Mol. Biol. 426, 3389–3412. doi: 10.1016/j.jmb.2014.03.016
Petit, J., and Magistretti, P. (2015). Regulation of neuron–ASTROCYTE metabolic coupling. Neuroscience 323, 135–156. doi: 10.1016/j.neuroscience.2015.12.007
Pickard, H., and Ahmed, S. H. (2016). “How do you know you have a drug problem? The role of knowledge of negative consequences in explaining drug choice in humans and rats,” in Addiction and choice: Rethinking the relationship. eds. N. Heather and G. Segal (Oxford University Press), p. 29–48.
Piepenbrink, M. S., Samuel, M., Zheng, B., Carter, B., Fucile, C., Bunce, C., et al. (2016). Humoral dysregulation associated with increased systemic inflammation among injection heroin users. PLoS One 11:e0158641. doi: 10.1371/journal.pone.0158641
Poisel, E., Zillich, L., Streit, F., Frank, J., Friske, M. M., Foo, J. C., et al. (2023). DNA methylation in cocaine use disorder–an epigenome-wide approach in the human prefrontal cortex. Front. Psych. 14:1075250. doi: 10.3389/fpsyt.2023.1075250
Ponomarev, I., Wang, S., Zhang, L., Harris, R. A., and Mayfield, R. D. (2012). Gene coexpression networks in human brain identify epigenetic modifications in alcohol dependence. J. Neurosci. 32, 1884–1897. doi: 10.1523/JNEUROSCI.3136-11.2012
Pouille, F., and Scanziani, M. (2001). Enforcement of temporal fidelity in pyramidal cells by somatic feed-forward inhibition. Science 293, 1159–1163. doi: 10.1126/science.1060342
Prakash, M. D., Tangalakis, K., Antonipillai, J., Stojanovska, L., Nurgali, K., and Apostolopoulos, V. (2017). Methamphetamine: effects on the brain, gut and immune system. Pharmacol. Res. 120, 60–67. doi: 10.1016/j.phrs.2017.03.009
Qian, S., Shi, C., Huang, S., Yang, C., and Luo, Y. (2022). DNA methyltransferase activity in the basolateral amygdala is critical for reconsolidation of a heroin reward memory. Front. Mol. Neurosci. 15:1002139. doi: 10.3389/fnmol.2022.1002139
Robison, A. J., and Nestler, E. J. (2022). DeltaFOSB: a potentially druggable master orchestrator of activity-dependent gene expression. ACS Chem. Neurosci. 13, 296–307. doi: 10.1021/acschemneuro.1c00723
Rogan, M. T., Stäubli, U. V., and Ledoux, J. E. (1997). Fear conditioning induces associative long-term potentiation in the amygdala. Nature 390, 604–607. doi: 10.1038/37601
Russo, S. J., and Nestler, E. J. (2013). The brain reward circuitry in mood disorders. Nat. Rev. Neurosci. 14, 609–625. doi: 10.1038/nrn3381
Sadri-Vakili, G., Kumaresan, V., Schmidt, H. D., Famous, K. R., Chawla, P., Vassoler, F. M., et al. (2010). Cocaine-induced chromatin remodeling increases brain-derived neurotrophic factor transcription in the rat medial prefrontal cortex, which alters the reinforcing efficacy of cocaine. J. Neurosci. 30, 11735–11744. doi: 10.1523/JNEUROSCI.2328-10.2010
Sartor, G. C. (2019). Epigenetic pharmacotherapy for substance use disorder. Biochem. Pharmacol. 168, 269–274. doi: 10.1016/j.bcp.2019.07.012
See, R. E. (2002). Neural substrates of conditioned-cued relapse to drug-seeking behavior. Pharmacol. Biochem. Behav. 71, 517–529. doi: 10.1016/S0091-3057(01)00682-7
Self, D. W. (2004). Regulation of drug-taking and-seeking behaviors by neuroadaptations in the mesolimbic dopamine system. Neuropharmacology 47, 242–255. doi: 10.1016/j.neuropharm.2004.07.005
Sequeira, A., and Turecki, G. (2006). Genome wide gene expression studies in mood disorders. Omics 10, 444–454. doi: 10.1089/omi.2006.10.444
Shiflett, M. W., and Balleine, B. W. (2010). At the limbic–motor interface: disconnection of basolateral amygdala from nucleus accumbens core and shell reveals dissociable components of incentive motivation. Eur. J. Neurosci. 32, 1735–1743. doi: 10.1111/j.1460-9568.2010.07439.x
Shinonaga, Y., Takada, M., and Mizuno, N. (1994). Topographic organization of collateral projections from the basolateral amygdaloid nucleus to both the prefrontal cortex and nucleus accumbens in the rat. Neuroscience 58, 389–397. doi: 10.1016/0306-4522(94)90045-0
Silver, J., Schwab, M. E., and Popovich, P. G. (2015). Central nervous system regenerative failure: role of oligodendrocytes, astrocytes, and microglia. Cold Spring Harb. Perspect. Biol. 7:a020602. doi: 10.1101/cshperspect.a020602
Stadelmann, C., Timmler, S., Barrantes-Freer, A., and Simons, M. (2019). Myelin in the central nervous system: structure, function, and pathology. Physiol. Rev. 99, 1381–1431. doi: 10.1152/physrev.00031.2018
Stefanik, M. T., and Kalivas, P. W. (2013). Optogenetic dissection of basolateral amygdala projections during cue-induced reinstatement of cocaine seeking. Front. Behav. Neurosci. 7:213. doi: 10.3389/fnbeh.2013.00213
Steinman, L. (1996). Multiple sclerosis: a coordinated immunological attack against myelin in the central nervous system. Cell 85, 299–302. doi: 10.1016/S0092-8674(00)81107-1
Stuber, G. D., Sparta, D. R., Stamatakis, A. M., Van Leeuwen, W. A., Hardjoprajitno, J. E., Cho, S., et al. (2011). Excitatory transmission from the amygdala to nucleus accumbens facilitates reward seeking. Nature 475, 377–380. doi: 10.1038/nature10194
Sugase-Miyamoto, Y., and Richmond, B. J. (2005). Neuronal signals in the monkey basolateral amygdala during reward schedules. J. Neurosci. 25, 11071–11083. doi: 10.1523/JNEUROSCI.1796-05.2005
Sullivan, M. D. (2018). Depression effects on long-term prescription opioid use, abuse, and addiction. Clin. J. Pain 34, 878–884. doi: 10.1097/AJP.0000000000000603
Sundar, M., Patel, D., Young, Z., and Leong, K. C. (2021). Oxytocin and addiction: potential glutamatergic mechanisms. Int. J. Mol. Sci. 22:2405. doi: 10.3390/ijms22052405
Tan, B., Browne, C. J., Nobauer, T., Vaziri, A., Friedman, J. M., and Nestler, E. J. (2024). Drugs of abuse hijack a mesolimbic pathway that processes homeostatic need. Science 384:eadk6742. doi: 10.1126/science.adk6742
Tan, X., Liu, X., Liu, E., Liu, M., Mu, S., Hang, Z., et al. (2022). Astrocyte-derived lactate/NADH alters methamphetamine-induced memory consolidation and retrieval by regulating neuronal synaptic plasticity in the dorsal hippocampus. Brain Struct. Funct. 227, 2681–2699. doi: 10.1007/s00429-022-02563-1
Thomas, C. M., Thrailkill, E. A., Bouton, M. E., and Green, J. T. (2020). Inactivation of the prelimbic cortex attenuates operant responding in both physical and behavioral contexts. Neurobiol. Learn. Mem. 171:107189. doi: 10.1016/j.nlm.2020.107189
Tomasi, D., and Volkow, N. D. (2013). Striatocortical pathway dysfunction in addiction and obesity: differences and similarities. Crit. Rev. Biochem. Mol. Biol. 48, 1–19. doi: 10.3109/10409238.2012.735642
Tran, M. N., Maynard, K. R., Spangler, A., Huuki, L. A., Montgomery, K. D., Sadashivaiah, V., et al. (2021). Single-nucleus transcriptome analysis reveals cell-type-specific molecular signatures across reward circuitry in the human brain. Neuron 109:e3085. doi: 10.1016/j.neuron.2021.09.001
Tye, K. M., Stuber, G. D., De Ridder, B., Bonci, A., and Janak, P. H. (2008). Rapid strengthening of thalamo-amygdala synapses mediates cue–reward learning. Nature 453, 1253–1257. doi: 10.1038/nature06963
Vázquez-Ágredos, A., Valero, M., Aparicio-Mescua, T., García-Rodríguez, R., Gámiz, F., and Gallo, M. (2024). Adolescent alcohol exposure modifies adult anxiety-like behavior and amygdala sensitivity to alcohol in rats: increased c-Fos activity and sex-dependent microRNA-182 expression. Pharmacol. Biochem. Behav. 238:173741. doi: 10.1016/j.pbb.2024.173741
Volkow, N. D. (2005). What do we know about drug addiction? Am. J. Psychiatry 162, 1401–1402. doi: 10.1176/appi.ajp.162.8.1401
Walker, D. M., Cates, H. M., Heller, E. A., and Nestler, E. J. (2015). Regulation of chromatin states by drugs of abuse. Curr. Opin. Neurobiol. 30, 112–121. doi: 10.1016/j.conb.2014.11.002
Walker, D. M., Cates, H. M., Loh, Y. E., Purushothaman, I., Ramakrishnan, A., Cahill, K. M., et al. (2018). Cocaine Self-administration alters transcriptome-wide responses in the Brain's reward circuitry. Biol. Psychiatry 84, 867–880. doi: 10.1016/j.biopsych.2018.04.009
Wang, W.-S., Kang, S., Liu, W.-T., Li, M., Liu, Y., Yu, C., et al. (2012). Extinction of aversive memories associated with morphine withdrawal requires ERK-mediated epigenetic regulation of brain-derived neurotrophic factor transcription in the rat ventromedial prefrontal cortex. J. Neurosci. 32, 13763–13775. doi: 10.1523/JNEUROSCI.1991-12.2012
Wang, Y., Lai, J., Cui, H., Zhu, Y., Zhao, B., Wang, W., et al. (2015). Inhibition of histone deacetylase in the basolateral amygdala facilitates morphine context-associated memory formation in rats. J. Mol. Neurosci. 55, 269–278. doi: 10.1007/s12031-014-0317-4
Wang, J., Lu, C., Zheng, L., and Zhang, J. (2021). Peripheral inflammatory biomarkers of methamphetamine withdrawal patients based on the neuro-inflammation hypothesis: the possible improvement effect of exercise. Front. Psych. 12:795073. doi: 10.3389/fpsyt.2021.795073
Werner, C. T., Altshuler, R. D., Shaham, Y., and Li, X. (2021). Epigenetic mechanisms in drug relapse. Biol. Psychiatry 89, 331–338. doi: 10.1016/j.biopsych.2020.08.005
Whitfield, T. W., Shi, X., Sun, W.-L., and Mcginty, J. F. (2011). The suppressive effect of an intra-prefrontal cortical infusion of BDNF on cocaine-seeking is Trk receptor and extracellular signal-regulated protein kinase mitogen-activated protein kinase dependent. J. Neurosci. 31, 834–842. doi: 10.1523/JNEUROSCI.4986-10.2011
Winstanley, C. A., Laplant, Q., Theobald, D. E., Green, T. A., Bachtell, R. K., Perrotti, L. I., et al. (2007). DeltaFosB induction in orbitofrontal cortex mediates tolerance to cocaine-induced cognitive dysfunction. J. Neurosci. 27, 10497–10507. doi: 10.1523/JNEUROSCI.2566-07.2007
Wise, R. A. (2006). Role of brain dopamine in food reward and reinforcement. Philos. Trans. R. Soc. Biol. Sci. 361, 1149–1158. doi: 10.1098/rstb.2006.1854
Yusuf, D., Butland, S. L., Swanson, M. I., Bolotin, E., Ticoll, A., Cheung, W. A., et al. (2012). The transcription factor encyclopedia. Genome Biol. 13, 1–25. doi: 10.1186/gb-2012-13-3-r24
Keywords: substance use disorder, epigenetic regulation, transcriptional adaptations, cell-type specific modifications, neuroadaptive mechanisms
Citation: Wang B, Wang J, Beacher NJ, Lin D-T and Zhang Y (2025) Cell-type specific epigenetic and transcriptional mechanisms in substance use disorder. Front. Cell. Neurosci. 19:1552032. doi: 10.3389/fncel.2025.1552032
Received: 27 December 2024; Accepted: 13 March 2025;
Published: 28 March 2025.
Edited by:
Qingyao Kong, The University of Chicago, United StatesReviewed by:
Jianjun Zhang, Shanxi University of Chinese Medicine, ChinaCopyright © 2025 Wang, Wang, Beacher, Lin and Zhang. This is an open-access article distributed under the terms of the Creative Commons Attribution License (CC BY). The use, distribution or reproduction in other forums is permitted, provided the original author(s) and the copyright owner(s) are credited and that the original publication in this journal is cited, in accordance with accepted academic practice. No use, distribution or reproduction is permitted which does not comply with these terms.
*Correspondence: Yan Zhang, eWFuemhhbmcxMDEyQGJqbXUuZWR1LmNu
Disclaimer: All claims expressed in this article are solely those of the authors and do not necessarily represent those of their affiliated organizations, or those of the publisher, the editors and the reviewers. Any product that may be evaluated in this article or claim that may be made by its manufacturer is not guaranteed or endorsed by the publisher.
Research integrity at Frontiers
Learn more about the work of our research integrity team to safeguard the quality of each article we publish.