- 1Division of Basic Biomedical Sciences, Sanford School of Medicine, University of South Dakota, Vermillion, SD, United States
- 2Department of Biotechnology, Mount Marty University, Yankton, SD, United States
- 3School of Health Sciences, University of South Dakota, Vermillion, SD, United States
- 4Department of Psychiatry, Department of Pharmacology, Sackler Institute for Developmental Psychobiology, Weill Cornell Medicine, New York, NY, United States
Autism spectrum disorder (ASD) is a group of neurodevelopmental disorders with strong genetic heterogeneity and more prevalent in males than females. We and others hypothesize that diminished activity-dependent neural signaling is a common molecular pathway dysregulated in ASD caused by diverse genetic mutations. Brain-derived neurotrophic factor (BDNF) is a key growth factor mediating activity-dependent neural signaling in the brain. A common single nucleotide polymorphism (SNP) in the pro-domain of the human BDNF gene that leads to a methionine (Met) substitution for valine (Val) at codon 66 (Val66Met) significantly decreases activity-dependent BDNF release without affecting basal BDNF secretion. By using mice with genetic knock-in of this human BDNF methionine (Met) allele, our previous studies have shown differential severity of autism-like social deficits in male and female BDNF+/Met mice. Pyramidal neurons are the principal neurons in the prefrontal cortex (PFC), a key brain region for social behaviors. Here, we investigated the impact of diminished activity-dependent BDNF signaling on the intrinsic excitability of pyramidal neurons in the PFC. Surprisingly, diminished activity-dependent BDNF signaling significantly increased the intrinsic excitability of pyramidal neurons in male mice, but not in female mice. Notably, significantly decreased thresholds of action potentials were observed in male BDNF+/Met mice, but not in female BDNF+/Met mice. Voltage-clamp recordings revealed that the sodium current densities were significantly increased in the pyramidal neurons of male BDNF+/Met mice, which were mediated by increased transcriptional level of Scn2a encoding sodium channel NaV 1.2. Medium after hyperpolarization (mAHP), another important parameter to determine intrinsic neuronal excitability, is strongly associated with neuronal firing frequency. Further, the amplitudes of mAHP were significantly decreased in male BDNF+/Met mice only, which were mediated by the downregulation of Kcnn2 encoding small conductance calcium-activated potassium channel 2 (SK2). This study reveals a sexually dimorphic signature of diminished activity-dependent BDNF signaling on the intrinsic neuronal excitability of pyramidal neurons in the PFC, which provides possible cellular and molecular mechanisms underpinning the sex differences in idiopathic ASD patients and human autism victims who carry BDNF Val66Met SNP.
1 Introduction
ASD is highly genetically heterogeneous and caused by both inherited and de novo gene mutations (Simons Foundation Autism Research Initiative, SFARI) (Satterstrom et al., 2020; Homozygosity Mapping Collaborative for Autism et al., 2014). We and others hypothesize that diminished activity-dependent neural signaling is a common molecular pathway dysregulated in ASD caused by diverse genetic mutations (Ebert and Greenberg, 2013; Yap and Greenberg, 2018). Brain-derived neurotrophic factor (BDNF) is synthesized and secreted in response to neuronal activity, which is a key molecule mediating activity-dependent neural signaling and has particular roles in synaptic transmission, synaptic connections, neurotransmitter release, and synaptic plasticity during postnatal neuronal development (Song et al., 2017; Greenberg et al., 2009; Chao, 2003). A common single nucleotide polymorphism (SNP) in the pro-domain of the human BDNF gene that leads to a methionine (Met) substitution for valine (Val) at amino acid 66 (Val66Met) significantly reduces dendritic trafficking, synaptic localization of the protein, and decreases up to 30% of activity-dependent BDNF release without affecting basal BDNF secretion (Chen et al., 2006; Egan et al., 2003). This human BDNF Val66Met SNP has been linked to psychiatric diseases, including anxiety (Chen et al., 2006; Egan et al., 2003; Notaras et al., 2015). Human studies showed that the BDNF Val66Met SNP was significantly associated with children with ASD in the Korean population (Yoo et al., 2014). Abnormal cortical developmental was observed in autism children harboring BDNF Val66Met SNP (Raznahan et al., 2009). By using mice with genetic knock-in of this human BDNF methionine (Met) allele, our recent studies have shown that diminished activity-dependent BDNF signaling differentially induces autism-like social deficits in males and females, and that males appear to be more severe than females (Ma et al., 2023).
In humans and rodents, the prefrontal cortex (PFC) is a hub brain region critical for “high-level” executive functions, including social behavior and cognition (Arnsten and Rubia, 2012; Davidson, 2002; Qin et al., 2019; Qin et al., 2018). Ours and others’ studies have demonstrated that Shank3-deficiency significantly diminished NMDA receptors- and AMPA (α-amino-3-hydroxy-5-methyl-4-isoxazolepropionic acid) receptors-mediated glutamatergic synaptic transmission in the PFC, which caused social deficits in mouse models (Duffney et al., 2015; Wang et al., 2016; Qin et al., 2018). Increased neural activity in the PFC is a key pathogenesis of social deficits in ASD (Lee et al., 2017; Gao and Penzes, 2015; Yizhar et al., 2011; Spratt et al., 2019). Pyramidal neurons are the principal neurons in the PFC, which send out long-distance glutamatergic excitation to the subcortical regions (Murugan et al., 2017; Zhong et al., 2022; Zhong et al., 2020). Intrinsic neuronal excitability is the capability of a neuron to generate action potentials in response to integrative synaptic inputs such as somatic current injections, which is determined primarily by the densities and functions of voltage-gated ion channels (Zhang and Linden, 2003). The changes in intrinsic neuronal excitability are thought to play an important role in learning and memory (Chen et al., 2020), and social deficits in autism mouse models (Spratt et al., 2019; Zhang et al., 2021; Tatsukawa et al., 2019; Eaton et al., 2021; Spratt et al., 2021; Khandelwal et al., 2021). However, little is known about the impact of activity-dependent BDNF signaling on the intrinsic neuronal excitability of pyramidal neurons in the PFC.
According to the latest data from the Autism and Developmental Disabilities Monitoring (ADDM) network (CDC: Centers for Diseases Control and Prevention), one in 36 8-year-old children have been identified with ASD in 2020. Males are four times more likely to be diagnosed with ASD than females. To highlight the need to investigate the neural mechanisms of ASD in both sexes (Shansky and Woolley, 2016), in this study, we characterized the impact of diminished activity-dependent BDNF signaling on the intrinsic neuronal excitability of pyramidal neurons in the PFC by using male and female BDNF+/+ and BDNF+/Met mice.
2 Materials and methods
2.1 Animal care and husbandry
The use of animals and procedures performed were approved by the Institutional Animal Care and Use Committee of Sanford School of Medicine, University of South Dakota. A mouse model with genetic knock-in of a human BDNF Met variant was created, and the procedures for heterozygote breeding and genotyping were described previously (Chen et al., 2006). These mice were backcrossed more than 12 generations into the C57BL/6 strain. Animals were group-housed (n = 4–5) in standard cages and were kept on a 12-h light–dark cycle in a temperature-controlled room. Food and water were available ad libitum. Experiments were performed in male and female BDNF+/Met mice and sex- and age- matched WT littermates BDNF+/+, which were derived from heterozygous BDNF+/Met breeding pairs.
2.2 Brain slice preparation
Coronal brain slices containing PFC were prepared from 2 months old male and female BDNF+/+ and BDNF+/Met mice as described previously (Qin et al., 2019; Qin et al., 2018; Qin et al., 2021). In brief, mice were anesthetized with isoflurane and rapidly decapitated. Brains were immediately removed, iced, and cut into 300 μm slices by a Vibratome (Leica VP1000S, Leica Microsystems Inc.). Brain slices were then incubated at 33°C in artificial cerebrospinal fluid (ACSF) (in mM: 130 NaCl, 26 NaHCO3, 3 KCl, 5 MgCl2, 1.25 NaH2PO4, 1 CaCl2, 10 glucose, pH 7.4, 300 mOsm) for 1 h and then kept for 1–4 h at room temperature (20–21°C) bubbling with 95% O2, 5% CO2.
2.3 Whole cell patch-clamp recordings
For recordings, the brain slice was positioned in a perfusion chamber attached to the fixed stage of an upright microscope (Olympus) and submerged in continuously flowing oxygenated ACSF (in mM: 130 NaCl, 26 NaHCO3, 1 CaCl2, 5 MgCl2, 3 KCl, 1.25 NaH2PO4, 10 glucose, pH 7.4, 300 mOsm). Layer V pyramidal neurons in the PFC were visualized with infrared differential interference contrast video microscopy. Recordings were performed with a multi-Clamp 700B amplifier (Molecular Devices), and data were acquired using pClamp 11.2 software, filtered at 1 kHz and sampling rate at 10 kHz with an Axon Digidata 1550B plus HumSilencer digitizer (Molecular Devices). Recording electrodes were pulled from borosilicate glass capillaries (1.5/0.86 mm OD/ID) with a micropipette puller (Sutter Instrument, model P-97, Novato, CA). The resistances of patch electrodes were 4–6 MΩ when filled with internal solution.
Whole-cell current-clamp recordings were used to measure action potentials. The brain slice was bathed in a modified ACSF with low (0.5 mM) MgCl2 to elevate neuronal activity, which more closely mimics the ionic composition of the brain interstitial fluid in situ. AMPA (α-amino-3-hydroxy-5-methyl-4-isoxazolepropionic acid) (20 mM CNQX) and GABAA (g-aminobutyric acid) receptors blockers (20 mM bicuculline) were added in action potentials recordings as in our previous studies (Qin et al., 2021). The pipettes were filled with an intracellular solution (in mM): 124 K-gluconate, 1 MgCl2, 6 KCl, 5 EGTA, 10 HEPES, 0.5 CaCl2, and 12 phosphocreatine, 5 MgATP, 0.5 Na2GTP, 0.2 Leupeptin, pH 7.2–7.3, 265–270 mOsm. To label the neurons under recording, the internal solution was supplemented with 0.05% sulforhodamine B. Slices were then fixed with 4% paraformaldehyde for 30 min, washed 3 times in PBS (pH 7.4). The neuronal images were acquired with a Leica TCS SP8 confocal microscope.
Seal formation and membrane rupture were done in a voltage-clamp mode at holding potential of −70 mV. Resting membrane potentials were measured immediately on break-in. A series of 250 ms current pulses (from −20 to 130 pA, 10 pA increments) were elicited to obtain action potential firing trains while the neurons were held at a fixed potential of −70 mV.
Rheobase was the minimal electric current required to elicit an action potential when current was injected into a neuron holding at −70 mV. The first spike latency was the time interval between the beginning of the current step and the occurrence of the first action potential elicited by minimal electric current. The voltage threshold of action potential was defined as the voltage where the value of dV/dt first exceeded 10 mV/ms at the first spike elicited by minimal electric current. The amplitude of medial after hyperpolarization (mAHP) was measured as the difference between the threshold and the peak of the most negative followed the action potentials after the first action potential (regular spiking neurons) or after short-bursts (intrinsic bursting neurons) elicited by 100 pA current injection (Wu et al., 2016).
The input resistance (r) was determined by injecting a − 100 pA, 250 ms hyperpolarizing current into the neuron holding at −70 mV. The membrane time constant (τ) was calculated using a single exponential fit of the voltage change in response to −100 pA hyperpolarizing current injection with 250 ms duration. The cell capacitance (c) was calculated under a current-clamp mode using the formula c = r/τ, where c was membrane capacitance, r was cell membrane resistance, and τ was membrane time constant (Sun et al., 2020).
Whole-cell voltage-clamp recordings were used to measure sodium currents. The brain slice was bathed in ACSF. Recording pipette contained the following internal solution (in mM: 100 CsCl, 10 tetraethylammonium chloride (TEA-Cl), 5 4-aminopyridine (4-AP), 10 HEPES, 4 NaCl, 1 MgCl2, 5 EGTA, 12 phosphocreatine, 5 MgATP, 0.5 Na2GTP, 0.2 Leupeptin, pH 7.2–7.3, 265–270 mOsm) (Milescu et al., 2010). Neurons were held at −70 mV and stepped to a range of potentials (−70 to +50 mV, 10 mV increments) for 100 ms each. Current densities (current/capacitance) were plotted as a function of depolarizing potential to generate current densities-voltage curves. All electrophysiological recordings were performed at room temperature (21–22°C). During recordings, neurons with leak currents >200 pA were discarded. Series resistance (Rs) was compensated to 80–90%. Cells having series resistance >10 MΩ or change above 20% throughout the experiments were excluded from analysis (Sontheimer and Ransom, 2002; Manz et al., 2021). The leakage current amplitudes were subtracted offline from the current peaks, and the capacitive transients were not cancelled (Surges et al., 2006). The amplitude of sodium current was measured as the difference between the onset of depolarization (after capacitive transient) and the peak of inward current.
2.4 Quantitative real-time RT-PCR
Total RNA was isolated from mouse PFC punches using Trizol reagent (Invitrogen) and treated with DNase I (Invitrogen) to remove genomic DNA. Then the iScriptTM cDNA synthesis Kit (Bio-Rad) was used to obtain cDNA from the tissue mRNA. Quantitative real time PCR was carried out using the iCycler iQ™ RealTime PCR Detection System and iQ™ Supermix (Bio-Rad) according to the manufacturer’s instructions. In brief, GAPDH was used as the housekeeping gene for quantitation of the expression of target genes in samples from male and female BDNF+/+ and BDNF+/Met mice. Fold changes in the target genes were determined by: Fold change = 2-∆(∆CT), where ∆CT = CT (target) - CT(GAPDH), and ∆(∆CT) = ∆CT (another group) - ∆CT (male BDNF+/+). CT (threshold cycle) is defined as the fractional cycle number at which the fluorescence reaches 10X of the standard deviation of the baseline. A total reaction mixture of 20 mL was amplified in a 96-well thin-wall PCR plate (Bio-Rad) using the following PCR cycling parameters: 95°C for 5 min followed by 40 cycles of 95°C for 45 s, 55°C for 45 s, and 72°C for 45 s. Primers for all target genes are listed in Table 1.
2.5 Statistical analysis
Data were analyzed with GraphPad Prism 10 (GraphPad) and Clampfit 11.2 (Molecular Devices, Sunnyvale, CA). For statistical significance, experiments with more than two groups were assessed with two-way or three-way ANOVA, followed by post hoc Bonferroni tests for multiple comparisons. All values were presented as mean ± SEM. p < 0.05 was considered statistically different.
3 Results
3.1 Diminished activity-dependent BDNF signaling significantly increases the intrinsic excitability of pyramidal neurons in the PFC of male mice, but not in female mice
To examine the impact of diminished activity-dependent BDNF signaling on the intrinsic excitability of pyramidal neurons in the PFC, we performed a whole-cell patch clamp to evoke action potentials by injecting a series of constant currents while holding the membrane potential at -70 mV. Given that deep layer glutamatergic pyramidal neurons in PFC showed the clearest deficits in autistic children (Stoner et al., 2014), pyramidal neurons in layer V were selected for electrophysiological measurements. As shown in Figure 1A, pyramidal neurons can be reliably identified by electrophysiological recordings according to our previous studies (Qin et al., 2018; Qin et al., 2021). Pyramidal neurons have been classified into different subclasses based upon their spiking patterns (Franceschetti et al., 1998; Griego et al., 2022). We identified regularly spiking (RS) and intrinsic bursting (IB) pyramidal neurons in male and female BDNF+/+ and BDNF +/Met mice, which is consistent with others’ studies (Franceschetti et al., 1998; Griego et al., 2022; Hedrick and Waters, 2011). In male BDNF+/+ mice, 50% (8/16 neurons) of the recorded pyramidal neurons exhibited a regular spiking (RS) pattern in response to a series of depolarizing current steps and 50% (8/16 neurons) of them exhibited short bursts (two to five closely-spaced action potentials) (intrinsic bursting, IB) pattern. The proportion of these two types’ pyramidal neurons remained similar in female BDNF+/+ (RS, 56%, 9/16 neurons; IB, 44, 7/16 neurons), male BDNF +/Met (RS, 50%, 8/16 neurons; IB, 50%, 8/16 neurons) and female BDNF+/Met mice (RS: 50%, 10/20 neurons; IB: 50%, 10/20 neurons). The input–output curve was conducted by gradually increasing the stimulus intensity of the depolarizing pulse (10 pA, 250 ms). The number of action potentials in regular spiking and intrinsic bursting pyramidal neurons was significantly increased with the incremented injected currents in male and female BDNF+/+ and BDNF+/Met mice. The regular spiking and intrinsic bursting pyramidal neurons from male BDNF+/Met mice displayed similar trends of higher number of evoked action potentials, but not significantly, compared to male and female BDNF+/+, and female BDNF+/Met mice (Supplementary Figure S1). Then, we combined the regular spiking and intrinsic bursting pyramidal neurons together in each group. The number of evoked action potentials was significantly higher in the total pyramidal neurons of PFC from male BDNF+/Met mice, but not female BDNF+/Met mice, compared to male and female BDNF+/+ mice (F Genotype (1, 64) = 3.7, p = 0.059, F Sex (1, 64) = 2.8, p = 0.099, F Genotype and Sex interaction (1, 64) = 5.4, p = 0.024, three-way ANOVA) (Figures 1B,C). Therefore, regular spiking and intrinsic bursting pyramidal neurons were pooled together in each group in this study. These results suggest that diminished activity-dependent BDNF signaling has a sexual dimorphic effect on the intrinsic excitability of pyramidal neurons in the PFC.
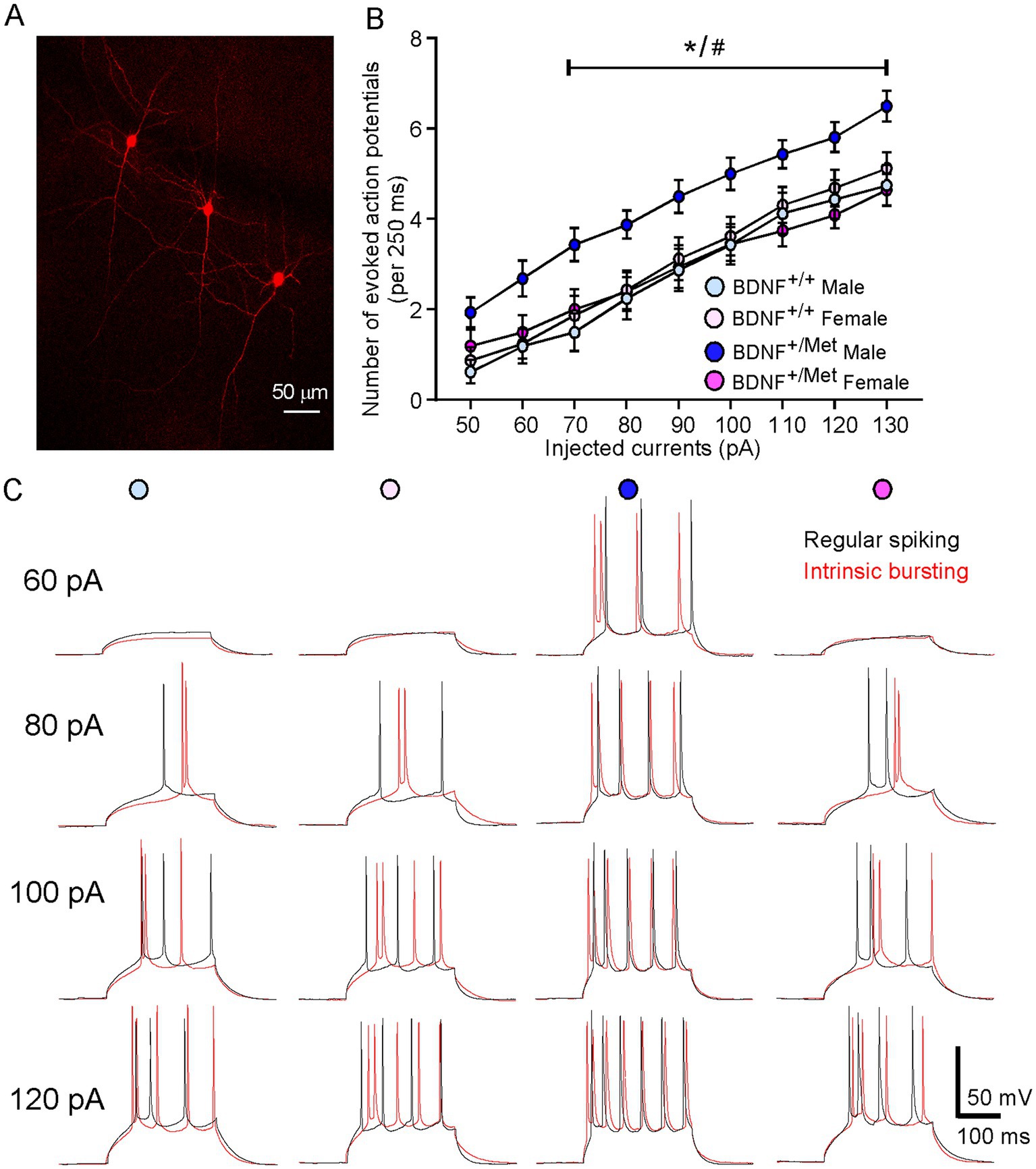
Figure 1. Diminished activity-dependent BDNF signaling significantly increases intrinsic neuronal excitability of pyramidal neurons in the PFC of male mice, but not in female mice. (A) A representative confocal image showing layer V pyramidal neurons used for recording. (B) Quantification of the number of evoked action potentials in response to injected currents in the pyramidal neurons of PFC from BDNF+/+ and BDNF+/Met mice. n = 16–20 neurons/4–5 mice/group. (C) Representative action potential traces from regular spiking (black) and intrinsic bursting (red) pyramidal neurons. *p < 0.05, BDNF+/Met versus BDNF+/+; #p < 0.05, male versus female, three-way ANOVA.
3.2 Diminished activity-dependent BDNF signaling significantly alters properties of action potential of pyramidal neurons in male mice, but not in female mice
To further determine the mechanisms of diminished activity-dependent BDNF signaling increased intrinsic excitability of pyramidal neurons in male mice, we examined the key parameters reflecting the action potential properties of pyramidal neurons in male and female BDNF+/+ and BDNF+/Met mice. As shown in Figures 2A–E, diminished activity-dependent BDNF signaling significantly decreased rheobase in male, but not in female mice, the minimal electric current required to elicit an action potential when current was injected into a neuron (male BDNF+/+: 72.5 ± 5.6 pA; female BDNF+/+: 71.6 ± 4.9 pA; male BDNF+/Met: 50.6 ± 3.3; female BDNF+/Met: 70.3 ± 3.9 pA. n = 16–20 neurons/4–5 mice/group. F Genotype (1, 64) = 6.7, p = 0.012; F Sex (1, 64) = 4.3, p = 0.042; F Genotype and Sex interaction (1, 64) = 5.2, p = 0.025, two-way ANOVA). Male BDNF+/Met mice showed significantly shortened first spike latency, the period from the beginning of stimulus to the occurrence of first action potential (male BDNF+/+: 180.2 ± 10 ms; female BDNF+/+: 187.1 ± 9.8 ms; male BDNF+/Met: 133.2 ± 12.4 ms; female BDNF+/Met: 181.3 ± 9.2 ms. n = 16–20 neurons/4–5 mice/group. F Genotype (1, 64) = 6.4, p = 0.01, F Sex (1, 64) = 7.0, p = 0.01, F Genotype and Sex interaction (1, 64) = 3.9, p = 0.05, two-way ANOVA). The threshold of action potential reflects how easily a neuron turns synaptic inputs into an action potential. The thresholds of action potentials were significantly lower in the pyramidal neurons from male BDNF+/Met mice, but not from female mice, compared to male and female BDNF+/+ mice (male BDNF+/+: −44.6 ± 1.1 mV; female BDNF+/+: −45.0 ± 0.6 mV; male BDNF+/Met: −48.5 ± 0.5 mV; female BDNF+/Met: −45.7 ± 0.5 mV. n = 16–20 neurons/4–5 mice/group. F Genotype (1, 64) = 10.4, p = 0.002, F Sex (1, 64) = 2.9, p = 0.09, F Genotype and Sex interaction (1, 64) = 5.2, p = 0.03, two-way ANOVA). Medium afterhyperpolarization (mAHP) affects the threshold of action potential and neuronal firing activity (Bean, 2007; Dwivedi and Bhalla, 2021). The amplitudes of mAHP were significantly smaller in the pyramidal neurons from male BDNF+/Met mice, but not from female mice, compared to male and female BDNF+/+ mice (male BDNF+/+: −6.2 ± 0.4 mV; female BDNF+/+: −6.6 ± 0.3 mV; male BDNF+/Met: −4.5 ± 0.2 s; female BDNF+/Met: −6.3 ± 0.3 s. n = 16–20 neurons/4–5 mice/group. F Genotype (1, 64) = 9.7, p = 0.003, F Sex (1, 64) = 13.2, p = 0.0006, F Genotype and Sex interaction (1, 64) = 4.7, p = 0.03, two-way ANOVA). These results demonstrate that diminished activity-dependent BDNF signaling differentially alters the properties of action potential in the pyramidal neurons from male and female mice, which mediates the sex effect of diminished activity-dependent BDNF signaling on the intrinsic excitability of pyramidal neurons.
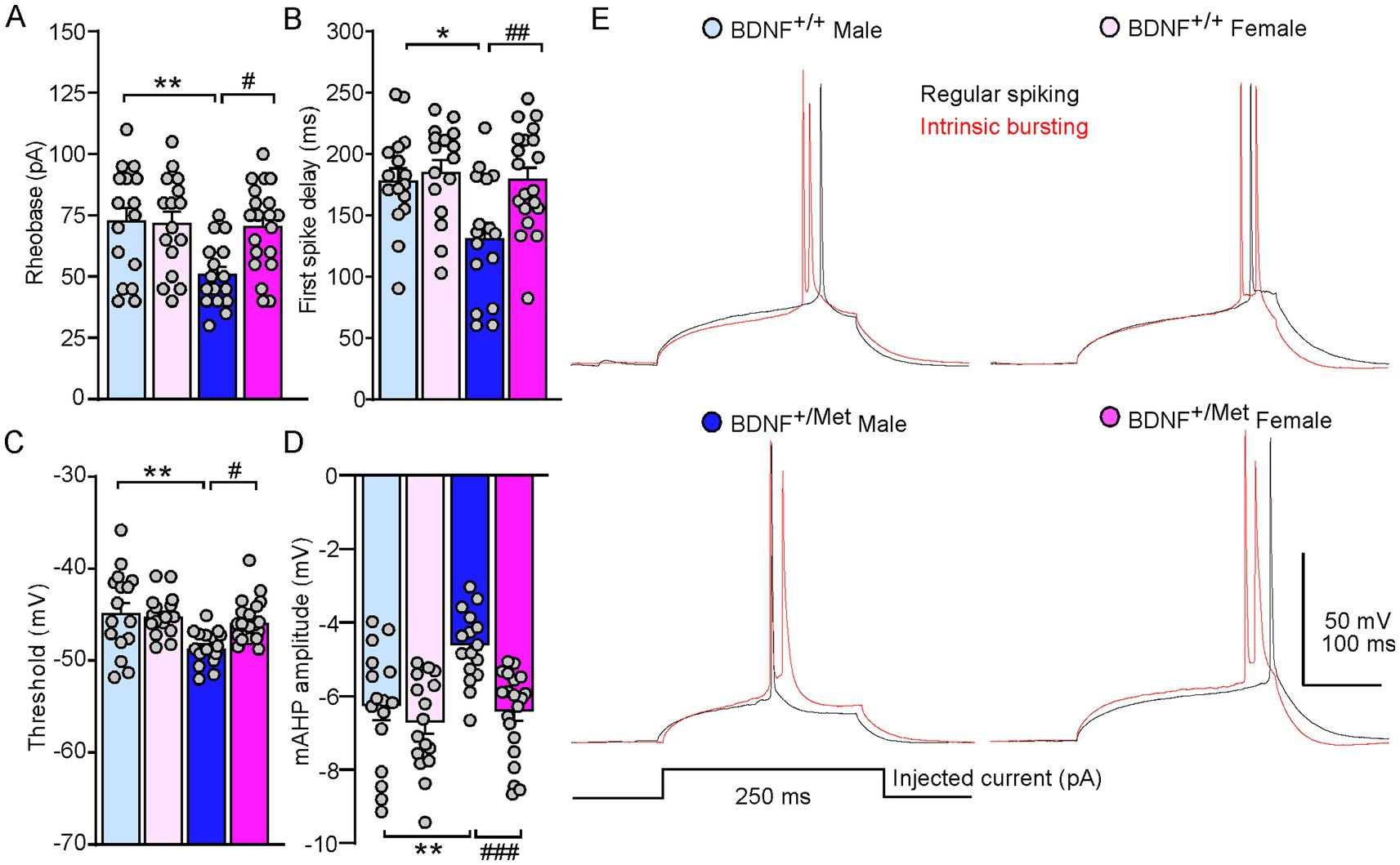
Figure 2. Diminished activity-dependent BDNF signaling differentially alters properties of action potential of pyramidal neurons in the PFC of males and females. Bar graphs showing rheobase (A), first spike delay (B), threshold (C), and mAHP (D) in the pyramidal neurons of PFC from male and female BDNF+/+ and BDNF+/Met mice. *p < 0.05, **p < 0.01, BDNF+/Met versus BDNF+/+; #p < 0.05, ##p < 0.01, ###p < 0.001, male versus female, n = 16–20 neurons/4–5 mice/group, two-way ANOVA. (E) Representative traces of the first spike elicited with minimum electric current in regular spiking (black) and intrinsic bursting (red) pyramidal neurons.
3.3 Diminished activity-dependent BDNF signaling has no effects on passive membrane properties
The passive intrinsic properties of neurons are highly related to the ability of neurons to generate action potentials (Chen et al., 2020). Next, we examined the impact of diminished activity-dependent BDNF signaling on the passive membrane properties of pyramidal neurons in male and female mice. The passive membrane properties were determined by injecting a small hyperpolarizing current into the soma of neurons (100 pA). As shown in Supplementary Figure S2, there were no differences of resting membrane potentials, cell capacitance, input resistance, and Tau between male and female BDNF+/+ mice and BDNF+/Met mice. These results demonstrated that diminished activity-dependent BDNF signaling has no effects on the passive membrane properties of pyramidal neurons in male and female mice, which indicates there are no significant differences in morphology of layer V pyramidal neurons in the PFC of male and female BDNF+/+ and BDNF+/Met mice (Isokawa, 1997).
3.4 Diminished activity-dependent BDNF signaling increases the expression of Scn2a and voltage-gated sodium currents in male, but not in female mice
Voltage-gated sodium channels play an essential role in generation and propagation of action potentials (Goldin et al., 2000). To determine whether diminished activity-dependent BDNF signaling increases the sodium currents in male mice, we evaluated the amplitudes of sodium currents in the pyramidal neurons of male and female BDNF+/+ and BDNF+/Met mice in a voltage-clamp mode. The membrane potential was held at −70 mV. The total inward currents were recorded in response to voltage steps from −70 to +50 mV (10 mV step increase, 100 ms). As shown in current density curves and representative traces (Figures 3A–C), the peak inward currents were triggered at −50 mV. The inward currents were abolished in the presence of tetrodotoxin (TTX, 0.5 μM). The peak current densities were significantly increased in the pyramidal neurons from male BDNF+/Met mice, compared to female BDNF+/Met and BDNF+/+ mice (male BDNF+/+: −64.5 ± 1.5 pA/pF; female BDNF+/+: −68.9 ± 2.1 pA/pF; male BDNF+/Met: −92.5 ± 1.9 pA/pF; female BDNF+/Met: −71.4 ± 1.3 pA/pF, n = 16 neurons/4 mice/group, F Genotype (1, 60) = 76.0, p < 0.0001; F Sex (1, 60) = 22.9, p < 0.0001, F Genotype and Sex interaction (1, 60) = 52.9, p < 0.0001). Among the 9 known members, Nav1.1, Nav1.2, Nav 1.3, and Nav1.6 are highly expressed in the central nervous system (Lai and Jan, 2006), which are composed by α and β subunits. To determine which sodium channel contributes to the decreased thresholds of action potentials in the pyramidal neurons from male BDNF+/Met mice, we examined the transcriptional level of α subunits of these four sodium channels, given that the a subunit forms a pore that conducts sodium. As shown in Figure 3D, the mRNA levels of Scn2a (encoding Nav 1.2) were significantly higher in PFC lysates from male BDNF+/Met mice (F Genotype (1, 64) = 11.6, p = 0.002, F Sex (1, 64) = 14.6, p = 0.0007, F Genotype and Sex interaction (1, 64) = 15.4, p = 0.005), while the mRNA levels of Scn1a (encoding Nav 1.1), Scn3a (encoding Nav 1.3), and Scn8a (encoding Nav 1.6) were largely unchanged. These results suggest the increased Scn2a contributes to the higher sodium currents and decreased thresholds of action potentials in male BDNF+/Met mice.
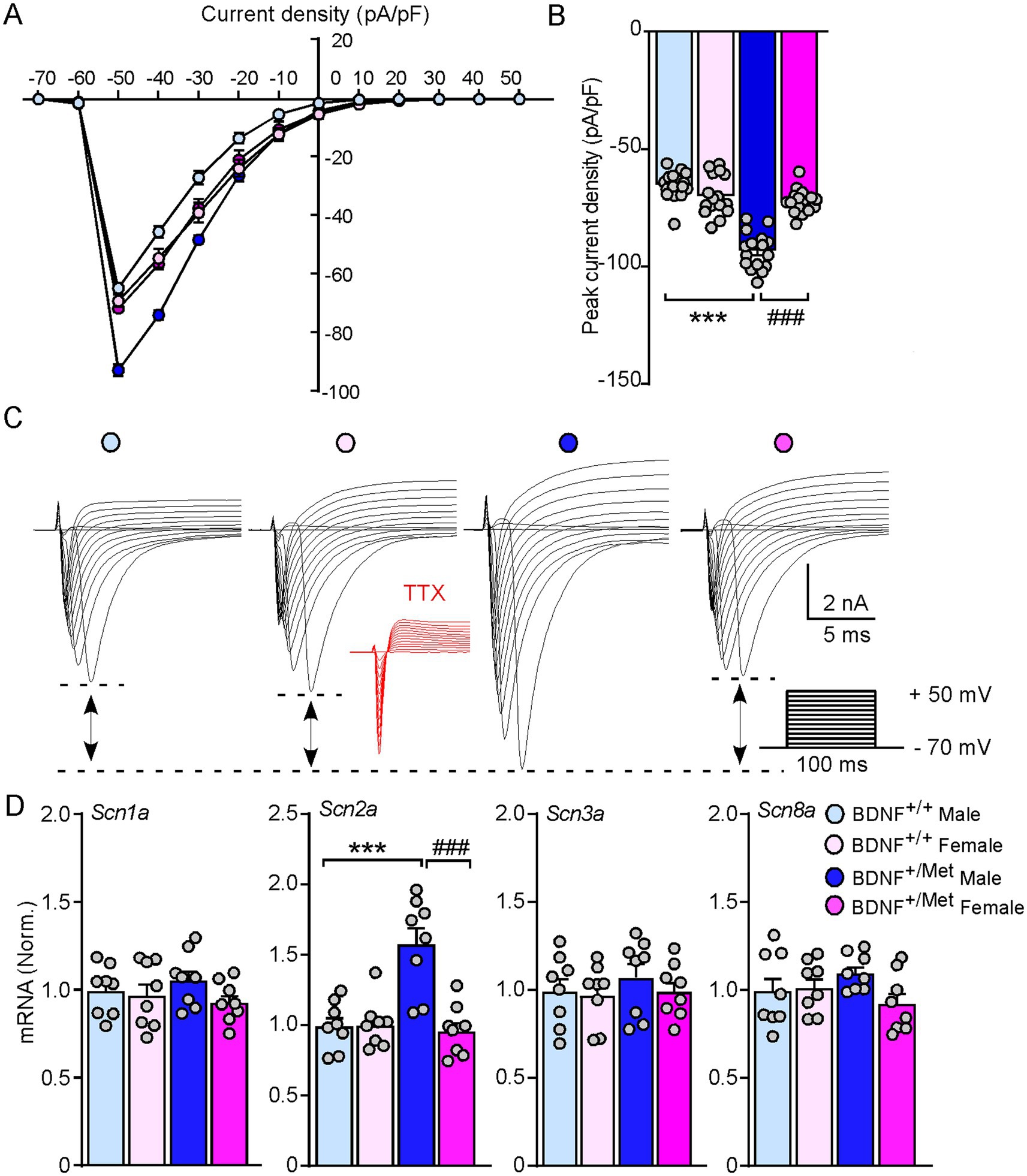
Figure 3. Diminished activity-dependent BDNF signaling significantly increases voltage-gated sodium currents and the expression of Scn2a in male mice, but not in female mice. (A) Current density-voltage relationship showing sodium current density in the pyramidal neurons from PFC of BDNF+/+ and BDNF+/Met mice. n = 16 neurons/4 mice/group. (B) Bar graph showing the peak current density in the pyramidal neurons from PFC of BDNF+/+ and BDNF+/Met mice. ***p < 0.001, BDNF+/Met versus BDNF+/+; ###p < 0.001, male versus female, n = 16 neurons/4 mice/group, two-way ANOVA. (C) Representative traces of voltage-gated sodium currents recorded in response to voltage steps from −70 to +50 mV in the pyramidal neurons from PFC of BDNF+/+ and BDNF+/Met mice. Inset: TTX (0.5 µM) abolished the inward currents. (D) Quantitative real-time PCR showing the mRNA levels of Scn1a, Scn2a, Scn3a, and Scn8a in the PFC of BDNF+/+ and BDNF+/Met mice. ***p < 0.001, BDNF+/Met versus BDNF+/+; ###p < 0.001, male versus female, n = 8 mice/group, two-way ANOVA.
3.5 Diminished activity-dependent BDNF signaling selectively decreases the transcriptional level of Kcnn2 in male mice, but not in female mice
Medium afterhyperpolarization (mAHP) is mainly mediated by small conductance calcium activated potassium channels (SK), voltage-gated potassium channels 7 (KCNQ), and hyperpolarization activated cyclic nucleotide (HCN) channels (Dwivedi and Bhalla, 2021; Mateos-Aparicio et al., 2014; Gu et al., 2005). To find out which ion channels mediate the smaller mAHP in pyramidal neurons from male BDNF+/Met mice (Figure 2E), we examined the transcriptional levels of Kcnn1-4 (encoding SK1-4 channels), Kcnq2-5 (encoding KCNQ channels), and Hcn1-4 (encoding HCN1-4 channels) in the PFC. As shown in Figure 4, the mRNA levels of kcnn2 (encoding SK2) were significantly decreased in PFC lysates from male BDNF+/Met mice (F Genotype (1, 28) = 12.5, p = 0.0015; F Sex (1, 28) = 5.6, p = 0.025), while the mRNA levels of Kcnn1, Kcnn3, Kcnn4, Kcnq2-5 and Hcn1-4 were largely unchanged. These results suggest that the decreased Kcnn2 is responsible for the smaller mAHP in male BDNF+/Met mice.
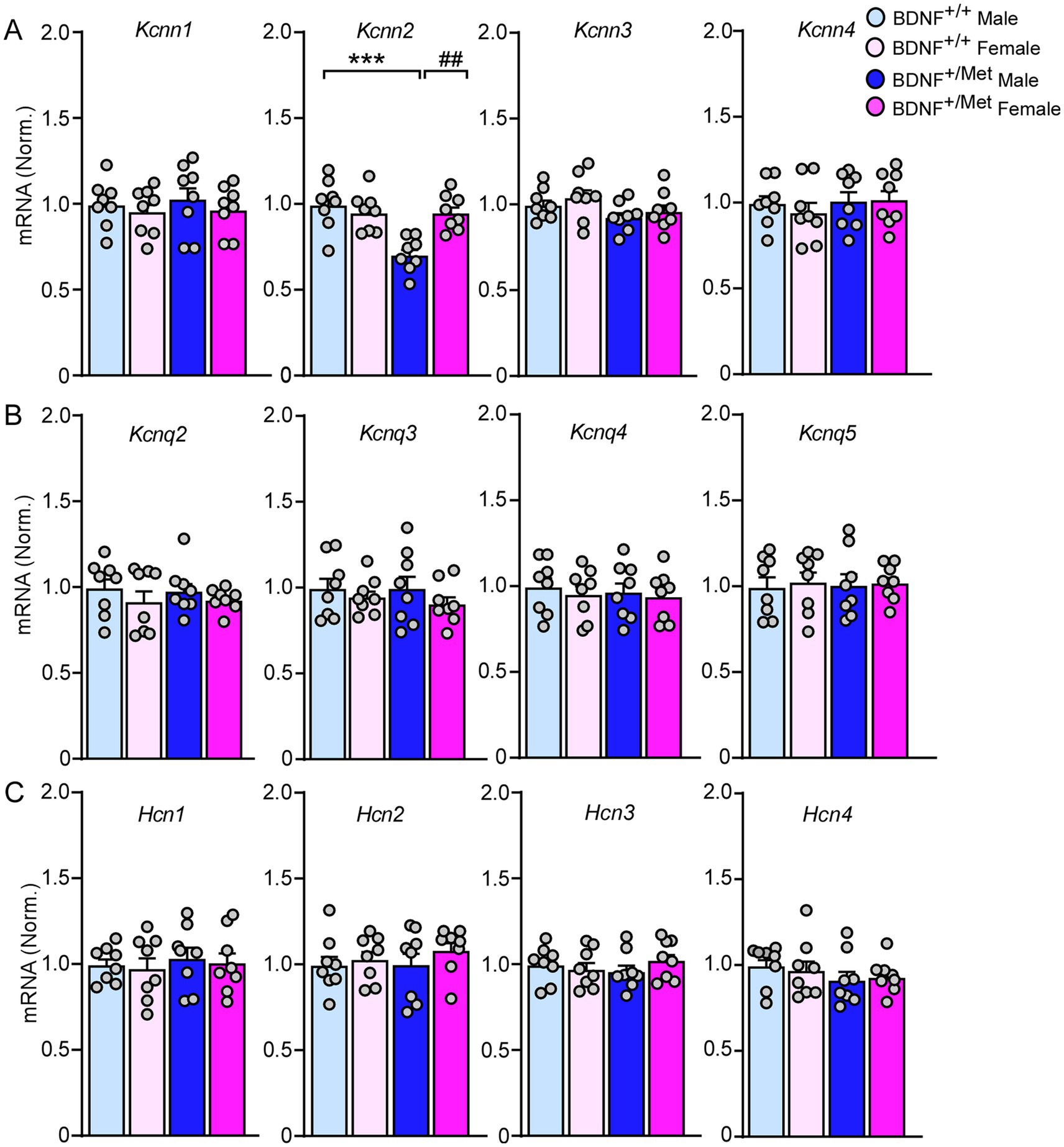
Figure 4. The impact of diminished activity-dependent BDNF signaling on the expression of SK channels, KCNQ channels, and HCN channels in the PFC. Quantitative real-time PCR showing the mRNA levels of SK channels (A), KCNQ channels (B), and HCN channels (C) in the PFC of BDNF+/+ and BDNF+/Met mice. ***p < 0.001, BDNF+/Met versus BDNF+/+; ##p < 0.01, male versus female, n = 8 mice/group, two-way ANOVA.
4 Discussion
In this study, we characterized the impact of diminished activity-dependent BDNF signaling on the intrinsic excitability of pyramidal neurons in the PFC by using mice with knock-in of a human BDNF Met allele. Our results showed that diminished activity-dependent BDNF signaling increased intrinsic excitability of pyramidal neurons in male mice, but not in female mice. Furthermore, diminished activity-dependent BDNF signaling significantly increased the expression of Scn2a and decreased the expression of Kcnn2 in male mice only, which decreased the thresholds of action potentials and facilitated the propagation of firing. We provide the first preclinical evidence that diminished activity-dependent BDNF signaling has a sexually dimorphic effect on the intrinsic excitability of pyramidal neurons in the PFC.
The high incidence of epilepsy in ASD patients indicates that the elevated excitability of pyramidal neurons is a major pathogenic factor in ASD (Blackmon et al., 2016; Sansa et al., 2011; Frye et al., 2013; Viscidi et al., 2013; Jeste and Tuchman, 2015). Increased intrinsic excitability of pyramidal neurons was found in the cortical neuronal culture by blocking neuronal activity by adding TTX, which was prevented by co-application of BDNF (Desai et al., 1999a; Desai et al., 1999b). In the present study, we found the evoked action potentials were significantly increased in the pyramidal neurons of PFC from male, but not female BDNF+/Met mice, indicating the critical role of activity-dependent BDNF in homeostatic regulation of intrinsic excitability of pyramidal neurons. Additionally, the thresholds and mAHP of action potentials were significantly lower in male BDNF+/Met mice. The higher AP frequencies, lower thresholds, and smaller mAHP of action potentials in male BDNF+/Met mice indicate that activity-dependent BDNF differentially regulates intrinsic neuronal excitability in males and females.
One critical question is the underlying molecular mechanisms. The increased intrinsic excitability of pyramidal neurons in male BDNF+/Met mice may occur through different ionic mechanisms. Voltage-gated sodium channels are one of the most important ion channels, which are crucial for the thresholds of action potentials. We found an upregulation of the sodium currents in pyramidal neurons of male BDNF+/Met mice. Consistent with others’ report (Sontheimer and Ransom, 2002), the sodium currents showed abruptly activated large inward currents with voltage steps to −50 mV, which indicated intrinsic signs of poor voltage control in brain slices recordings. Activity deprivation increased the sodium currents without affecting activation nor inactivation characteristics of sodium channels (Desai et al., 1999b), which indicates diminished activity-dependent BDNF signaling will not alter the kinetic characteristics of sodium channels in male mice, though a voltage-independent change on open-channel probability cannot be ruled out (Li et al., 1992). Further studies are needed via minimizing the uncontrolled space-clamp axonal currents by applying a depolarizing pre-pulse before each voltage step (Milescu et al., 2010; Griego et al., 2022) or dissociated pyramidal neurons from PFC, given that somatic sodium currents are under good voltage control. Nav1.2 (encoded by Scn2a) is one of several sodium channels involved in initiation and propagation of action potentials in neurons (Sanders et al., 2018) and expressed predominantly in pyramidal cells (Spratt et al., 2019). The transcription of Scn2a is selectively upregulated in the PFC of male BDNF+/Met mice. Nav1.2 is expressed in other cell types, such as interneurons (Yamagata et al., 2017), and glia cells (Pappalardo et al., 2016) in the PFC. The combined transcriptional and electrophysiological evidence strongly suggests the upregulated Nav1.2 mediates the increased sodium currents in the pyramidal neurons of male BDNF+/Met mice.
mAHP is another parameter used to determine the intrinsic neuronal excitability, which is a hyperpolarized phase after a single or a train of action potentials and lasts 50 ~ 300 ms (Chen et al., 2020). mAHP is predominantly mediated by small conductance calcium-activated potassium (SK) channels, although voltage-gated potassium channels 7 (Kv7) and hyperpolarization-activated cyclic nucleotide-gated (HCN) channels have also been shown to contribute to the mAHP (Dwivedi and Bhalla, 2021; Gu et al., 2005).
SK channels are small-conductance calcium-activated potassium channels that are widely expressed in neurons and influence neuronal firing frequency (Faber and Sah, 2007; Sun et al., 2020). There are four family members in the SK channels, which are SK1, SK2, SK3, and SK4, encoded by Kcnn1, Kcnn2, Kcnn3, and Kcnn4, respectively. Activation of SK channels by calcium influx can modulate the frequency of action potential through rapid potassium efflux, which leads membrane repolarization/hyperpolarization. Alterations in SK channels have also been reported in various brain diseases. Humans carrying loss-of-function KCNN2 mutations have been linked with ASD (Nam et al., 2023; Alonso-Gonzalez et al., 2019; Grove et al., 2019). Downregulation of SK2 was associated with augmented reticular thalamic bursting and seizures in Scn1a-Dravet syndrome (Ritter-Makinson et al., 2019). However, increased Kcnn2 decreased intrinsic excitability of cortical pyramidal neurons in a PTEN-associated autism mouse model (Garcia-Junco-Clemente et al., 2013). Intellectual disability and developmental delay symptoms have been reported in patients carrying KCNN3 variants (Bauer et al., 2019; Gripp et al., 2021; Schwarz et al., 2022). We found that diminished activity-dependent BDNF signaling selectively decreases Kcnn2, but not Kcnn1, Kcnn3, and Kcnn4.
Kcnq genes encode five family members of the Kv7 channels (Kv7.1–Kv7.5), which are a group of low-threshold voltage-gated potassium channels known as “M-channel” (Brown and Passmore, 2009). Four of them (Kcnq2-5) are expressed in the nervous system. Kv7.2 and Kv7.3 (encoded by Kcnq2/3) are the principal molecular components of M-channels, which are expressed on both excitatory and inhibitory neurons and constrain repetitive neuronal firing (Gu et al., 2005; Brown and Passmore, 2009; Delmas and Brown, 2005). Mutations of KCNQ2/3 cause ASD and benign familial neonatal epilepsy (Satterstrom et al., 2020; Homozygosity Mapping Collaborative for Autism et al., 2014; Piro et al., 2019; Soldovieri et al., 2014; Maljevic and Lerche, 2014; Wang et al., 2020). Kcnq2 deficiency in pyramidal neurons increased neuronal excitability, resulting in epilepsy (Peters et al., 2005; Soh et al., 2014). However, diminished activity-dependent BDNF signaling has no effect on the expressions of Kcnq2-5.
HCN channels are encoded by four genes (Hcn1–4), which can be activated typically at potentials below −50 mV and open at the resting membrane potential, conducting an inward cation current (Ih) (Biel et al., 2009; Mishra and Narayanan, 2023; Santoro and Shah, 2020). In the mouse brain, HCN1 and HCN2 are predominantly expressed in the presynaptic synapse, soma, dendrites, and axon initial segments of layer V pyramidal neurons in the frontal cortex (Santoro and Shah, 2020), where they regulate glutamate release (Huang and Trussell, 2014) and generation of action potentials (Ko et al., 2016). The hyperexcitability of layer V pyramidal neurons in the anterior cingulate cortex was associated with a decrease in Ih (Santello and Nevian, 2015). HCN1/2 are implicated in early infantile epileptic encephalopathy and absence seizures (Crunelli et al., 2023; Ludwig et al., 2003; Nava et al., 2014). In this study, there were no differences in the expressions of Hcn1-4 between BDNF+/+ and BDNF+/Met mice.
Therefore, diminished activity-dependent BDNF signaling selectively decreased the expression of Kcnn2, but not Kcnn1, Kcnn3, Kcnn4, Kv7 channels, and HCN channels, which indicates SK2 mediates the decreased mAHP in male BDNF+/Met mice.
There are several limitations in this study. First, the causal link between upregulated Scn2a, decreased Kccn2 and increased intrinsic excitatory in pyramidal neurons of male BDNF+/Met mice is unknown. Selectively knockdown Scn2a and overexpression of Kcnn2 in the pyramidal neurons of male BDNF+/Met mice will confirm this causal link in our future studies. Female C57Bl/6 J mice exhibited higher glutamatergic transmission in the PFC compared to males (Knouse et al., 2022). Second, the contribution of synaptic inputs on the neuronal excitability of pyramidal neurons in the PFC, and the impact of diminished activity-dependent BDNF signaling on the principle neurons in the other brain regions, need to be further investigated, which may mediate the anxiety, autism-like social deficits, and sex-specific effect of diminished activity-dependent BDNF signaling on spatial memory (Ma et al., 2023). Third, the molecular mechanisms underlying the increased Scn2a and decreased Kcnn2 in the PFC of male BDNF+/Met mice are difficult to decipher at this moment, which will be investigated in future studies.
In summary, using a knock-in mouse model of the human BDNF Val66 Met SNP, the present study demonstrates that the increased Scn2a and decreased Kcnn2 account for the sex effect of diminished activity-dependent BDNF signaling on the intrinsic excitability of pyramidal neurons in the PFC. The sex differences of intrinsic excitability of pyramidal neurons in the PFC of male and female BDNF+/Met mice may partially contribute to the severe autism-like behavioral deficits in male BDNF+/Met mice (Ma et al., 2023) and support that males are more vulnerable to having ASD (Giarelli et al., 2010; Werling and Geschwind, 2013). Targeting Scn2a and Kcnn2 is a potential therapy for male ASD patients with or without BDNF Val66Met SNP.
Data availability statement
The raw data supporting the conclusions of this article will be made available by the authors, without undue reservation.
Ethics statement
The animal study was approved by Institutional Animal Care and Use Committee of Sanford School of Medicine, University of South Dakota. The study was conducted in accordance with the local legislation and institutional requirements.
Author contributions
KMa: Conceptualization, Data curation, Formal analysis, Investigation, Methodology, Resources, Writing – review & editing. DZ: Formal analysis, Writing – review & editing. KMc: Formal analysis, Writing – review & editing. MW: Formal analysis, Writing – review & editing. SN: Funding acquisition, Writing – review & editing. FL: Funding acquisition, Writing – review & editing. LQ: Conceptualization, Funding acquisition, Data curation, Formal analysis, Investigation, Methodology, Resources, Project administration, Supervision, Validation, Writing – original draft, Writing – review & editing.
Funding
The author(s) declare that financial support was received for the research, authorship, and/or publication of this article. This work is supported by startup funding from Sanford School of Medicine, University of South Dakota (LQ), Sanford School of Medicine Faculty Research Grant (LQ), South Dakota Board of Regents Competitive Research Grant (LQ), NIH R21 MH134106 (LQ), NIH P20 GM103443 (KMc), NIH R01 MH106640 (SSN), and NIH R01 MH123154 (FL).
Acknowledgments
We are grateful to Dr. Sunghee Cho (Burke Neurological Institute, Weill Medical College of Cornell University) for the mice with knock-in of a human BDNF Met variant.
Conflict of interest
The authors declare that the research was conducted in the absence of any commercial or financial relationships that could be construed as a potential conflict of interest.
The author(s) declared that they were an editorial board member of Frontiers, at the time of submission. This had no impact on the peer review process and the final decision.
Publisher’s note
All claims expressed in this article are solely those of the authors and do not necessarily represent those of their affiliated organizations, or those of the publisher, the editors and the reviewers. Any product that may be evaluated in this article, or claim that may be made by its manufacturer, is not guaranteed or endorsed by the publisher.
Supplementary material
The Supplementary material for this article can be found online at: https://www.frontiersin.org/articles/10.3389/fncel.2024.1496930/full#supplementary-material
SUPPLEMENTARY FIGURE S1 | Quantification of the number of evoked action potentials in response to injected currents in the regular spiking pyramidal neurons (A) and intrinsic bursting pyramidal neurons (B) of PFC from BDNF+/+ and BDNF+/Met mice. A: n = 8-10 neurons/4-5 mice/group; B: n = 7-10 neurons/4-5 mice/group.
SUPPLEMENTARY FIGURE S2 | Diminished activity-dependent BDNF signaling has no effect on the passive membrane properties of pyramidal neurons in male and female mice. n = 16-20 neurons/4-5 mice per group. (A) Resting potential, (B) Capacitance, (C) Input resistance, (D) Membrane time constant (τ), and (E) Representative traces in response to -100 pA hyperpolarizing current injection with 250 ms duration in the pyramidal neurons from PFC of BDNF+/+ and BDNF+/Met mice.
References
Alonso-Gonzalez, A., Calaza, M., Rodriguez-Fontenla, C., and Carracedo, A. (2019). Novel gene-based analysis of ASD GWAS: insight into the biological role of associated genes. Front. Genet. 10:733. doi: 10.3389/fgene.2019.00733
Arnsten, A. F., and Rubia, K. (2012). Neurobiological circuits regulating attention, cognitive control, motivation, and emotion: disruptions in neurodevelopmental psychiatric disorders. J. Am. Acad. Child Adolesc. Psychiatry 51, 356–367. doi: 10.1016/j.jaac.2012.01.008
Bauer, C. K., Schneeberger, P. E., Kortüm, F., Altmüller, J., Santos-Simarro, F., Baker, L., et al. (2019). Gain-of-function mutations in KCNN3 encoding the small-conductance ca(2+)-activated K(+) channel SK3 cause Zimmermann-Laband syndrome. Am. J. Hum. Genet. 104, 1139–1157. doi: 10.1016/j.ajhg.2019.04.012
Bean, B. P. (2007). The action potential in mammalian central neurons. Nat. Rev. Neurosci. 8, 451–465. doi: 10.1038/nrn2148
Biel, M., Wahl-Schott, C., Michalakis, S., and Zong, X. (2009). Hyperpolarization-activated cation channels: from genes to function. Physiol. Rev. 89, 847–885. doi: 10.1152/physrev.00029.2008
Blackmon, K., Bluvstein, J., MacAllister, W. S., Avallone, J., Misajon, J., Hedlund, J., et al. (2016). Treatment resistant epilepsy in Autism Spectrum disorder: increased risk for females. Autism Res. 9, 311–320. doi: 10.1002/aur.1514
Brown, D. A., and Passmore, G. M. (2009). Neural KCNQ (Kv7) channels. Br. J. Pharmacol. 156, 1185–1195. doi: 10.1111/j.1476-5381.2009.00111.x
Chao, M. V. (2003). Neurotrophins and their receptors: a convergence point for many signalling pathways. Nat. Rev. Neurosci. 4, 299–309. doi: 10.1038/nrn1078
Chen, L., Cummings, K. A., Mau, W., Zaki, Y., Dong, Z., Rabinowitz, S., et al. (2020). The role of intrinsic excitability in the evolution of memory: significance in memory allocation, consolidation, and updating. Neurobiol. Learn. Mem. 173:107266. doi: 10.1016/j.nlm.2020.107266
Chen, Z. Y., Jing, D., Bath, K. G., Ieraci, A., Khan, T., Siao, C. J., et al. (2006). Genetic variant BDNF (Val66Met) polymorphism alters anxiety-related behavior. Science 314, 140–143. doi: 10.1126/science.1129663
Crunelli, V., David, F., Morais, T. P., and Lorincz, M. L. (2023). HCN channels and absence seizures. Neurobiol. Dis. 181:106107. doi: 10.1016/j.nbd.2023.106107
Davidson, R. J. (2002). Anxiety and affective style: role of prefrontal cortex and amygdala. Biol. Psychiatry 51, 68–80. doi: 10.1016/S0006-3223(01)01328-2
Delmas, P., and Brown, D. A. (2005). Pathways modulating neural KCNQ/M (Kv7) potassium channels. Nat. Rev. Neurosci. 6, 850–862. doi: 10.1038/nrn1785
Desai, N. S., Rutherford, L. C., and Turrigiano, G. G. (1999a). BDNF regulates the intrinsic excitability of cortical neurons. Learn. Mem. 6, 284–291. doi: 10.1101/lm.6.3.284
Desai, N. S., Rutherford, L. C., and Turrigiano, G. G. (1999b). Plasticity in the intrinsic excitability of cortical pyramidal neurons. Nat. Neurosci. 2, 515–520. doi: 10.1038/9165
Duffney, L. J., Zhong, P., Wei, J., Matas, E., Cheng, J., Qin, L., et al. (2015). Autism-like deficits in Shank3-deficient mice are rescued by targeting actin regulators. Cell Rep. 11, 1400–1413. doi: 10.1016/j.celrep.2015.04.064
Dwivedi, D., and Bhalla, U. S. (2021). Physiology and therapeutic potential of SK, H, and M medium AfterHyperPolarization ion channels. Front. Mol. Neurosci. 14:658435. doi: 10.3389/fnmol.2021.658435
Eaton, M., Zhang, J., Ma, Z., Park, A. C., Lietzke, E., Romero, C. M., et al. (2021). Generation and basic characterization of a gene-trap knockout mouse model of Scn2a with a substantial reduction of voltage-gated sodium channel Na(v) 1.2 expression. Genes Brain Behav. 20:e12725. doi: 10.1111/gbb.12725
Ebert, D. H., and Greenberg, M. E. (2013). Activity-dependent neuronal signalling and autism spectrum disorder. Nature 493, 327–337. doi: 10.1038/nature11860
Egan, M. F., Kojima, M., Callicott, J. H., Goldberg, T. E., Kolachana, B. S., Bertolino, A., et al. (2003). The BDNF val66met polymorphism affects activity-dependent secretion of BDNF and human memory and hippocampal function. Cell 112, 257–269. doi: 10.1016/S0092-8674(03)00035-7
Faber, E. S., and Sah, P. (2007). Functions of SK channels in central neurons. Clin. Exp. Pharmacol. Physiol. 34, 1077–1083. doi: 10.1111/j.1440-1681.2007.04725.x
Franceschetti, S., Sancini, G., Panzica, F., Radici, C., and Avanzini, G. (1998). Postnatal differentiation of firing properties and morphological characteristics in layer V pyramidal neurons of the sensorimotor cortex. Neuroscience 83, 1013–1024. doi: 10.1016/S0306-4522(97)00463-6
Frye, R. E., Rossignol, D., Casanova, M. F., Brown, G. L., Martin, V., Edelson, S., et al. (2013). A review of traditional and novel treatments for seizures in autism spectrum disorder: findings from a systematic review and expert panel. Front. Public Health 1:31. doi: 10.3389/fpubh.2013.00031
Gao, R., and Penzes, P. (2015). Common mechanisms of excitatory and inhibitory imbalance in schizophrenia and autism spectrum disorders. Curr. Mol. Med. 15, 146–167. doi: 10.2174/1566524015666150303003028
Garcia-Junco-Clemente, P., Chow, D. K., Tring, E., Lazaro, M. T., Trachtenberg, J. T., and Golshani, P. (2013). Overexpression of calcium-activated potassium channels underlies cortical dysfunction in a model of PTEN-associated autism. Proc. Natl. Acad. Sci. USA 110, 18297–18302. doi: 10.1073/pnas.1309207110
Giarelli, E., Wiggins, L. D., Rice, C. E., Levy, S. E., Kirby, R. S., Pinto-Martin, J., et al. (2010). Sex differences in the evaluation and diagnosis of autism spectrum disorders among children. Disabil. Health J. 3, 107–116. doi: 10.1016/j.dhjo.2009.07.001
Goldin, A. L., Barchi, R. L., Caldwell, J. H., Hofmann, F., Howe, J. R., Hunter, J. C., et al. (2000). Nomenclature of voltage-gated sodium channels. Neuron 28, 365–368. doi: 10.1016/S0896-6273(00)00116-1
Greenberg, M. E., Xu, B., Lu, B., and Hempstead, B. L. (2009). New insights in the biology of BDNF synthesis and release: implications in CNS function. J. Neurosci. 29, 12764–12767. doi: 10.1523/JNEUROSCI.3566-09.2009
Griego, E., Santiago-Jiménez, G., and Galván, E. J. (2022). Systemic administration of lipopolysaccharide induces hyperexcitability of prelimbic neurons via modulation of sodium and potassium currents. Neurotoxicology 91, 128–139. doi: 10.1016/j.neuro.2022.05.010
Gripp, K. W., Smithson, S. F., Scurr, I. J., Baptista, J., Majumdar, A., Pierre, G., et al. (2021). Syndromic disorders caused by gain-of-function variants in KCNH1, KCNK4, and KCNN3-a subgroup of K(+) channelopathies. Eur. J. Hum. Genet. 29, 1384–1395. doi: 10.1038/s41431-021-00818-9
Grove, J., Ripke, S., Als, T. D., Mattheisen, M., Walters, R. K., Won, H., et al. (2019). Identification of common genetic risk variants for autism spectrum disorder. Nat. Genet. 51, 431–444. doi: 10.1038/s41588-019-0344-8
Gu, N., Vervaeke, K., Hu, H., and Storm, J. F. (2005). Kv7/KCNQ/M and HCN/h, but not KCa2/SK channels, contribute to the somatic medium after-hyperpolarization and excitability control in CA1 hippocampal pyramidal cells. J. Physiol. 566, 689–715. doi: 10.1113/jphysiol.2005.086835
Hedrick, T., and Waters, J. (2011). Spiking patterns of neocortical L5 pyramidal neurons in vitro change with temperature. Front. Cell. Neurosci. 5:1. doi: 10.3389/fncel.2011.00001
Homozygosity Mapping Collaborative for Autismde Rubeis, S., He, X., Goldberg, A. P., Poultney, C. S., Samocha, K., et al. (2014). Synaptic, transcriptional and chromatin genes disrupted in autism. Nature 515, 209–215. doi: 10.1038/nature13772
Huang, H., and Trussell, L. O. (2014). Presynaptic HCN channels regulate vesicular glutamate transport. Neuron 84, 340–346. doi: 10.1016/j.neuron.2014.08.046
Isokawa, M. (1997). Membrane time constant as a tool to assess cell degeneration. Brain Res. Brain Res. Protoc. 1, 114–116. doi: 10.1016/S1385-299X(96)00016-5
Jeste, S. S., and Tuchman, R. (2015). Autism Spectrum disorder and epilepsy: two sides of the same coin? J. Child Neurol. 30, 1963–1971. doi: 10.1177/0883073815601501
Khandelwal, N., Cavalier, S., Rybalchenko, V., Kulkarni, A., Anderson, A. G., Konopka, G., et al. (2021). FOXP1 negatively regulates intrinsic excitability in D2 striatal projection neurons by promoting inwardly rectifying and leak potassium currents. Mol. Psychiatry 26, 1761–1774. doi: 10.1038/s41380-020-00995-x
Knouse, M. C., McGrath, A. G., Deutschmann, A. U., Rich, M. T., Zallar, L. J., Rajadhyaksha, A. M., et al. (2022). Sex differences in the medial prefrontal cortical glutamate system. Biol. Sex Differ. 13:66. doi: 10.1186/s13293-022-00468-6
Ko, K. W., Rasband, M. N., Meseguer, V., Kramer, R. H., and Golding, N. L. (2016). Serotonin modulates spike probability in the axon initial segment through HCN channels. Nat. Neurosci. 19, 826–834. doi: 10.1038/nn.4293
Lai, H. C., and Jan, L. Y. (2006). The distribution and targeting of neuronal voltage-gated ion channels. Nat. Rev. Neurosci. 7, 548–562. doi: 10.1038/nrn1938
Lee, E., Lee, J., and Kim, E. (2017). Excitation/inhibition imbalance in animal models of Autism Spectrum disorders. Biol. Psychiatry 81, 838–847. doi: 10.1016/j.biopsych.2016.05.011
Li, M., West, J. W., Lai, Y., Scheuer, T., and Catterall, W. A. (1992). Functional modulation of brain sodium channels by cAMP-dependent phosphorylation. Neuron 8, 1151–1159. doi: 10.1016/0896-6273(92)90135-Z
Ludwig, A., Budde, T., Stieber, J., Moosmang, S., Wahl, C., Holthoff, K., et al. (2003). Absence epilepsy and sinus dysrhythmia in mice lacking the pacemaker channel HCN2. EMBO J. 22, 216–224. doi: 10.1093/emboj/cdg032
Ma, K., Taylor, C., Williamson, M., Newton, S. S., and Qin, L. (2023). Diminished activity-dependent BDNF signaling differentially causes autism-like behavioral deficits in male and female mice. Front. Psych. 14:1182472. doi: 10.3389/fpsyt.2023.1182472
Maljevic, S., and Lerche, H. (2014). Potassium channel genes and benign familial neonatal epilepsy. Prog. Brain Res. 213, 17–53. doi: 10.1016/B978-0-444-63326-2.00002-8
Manz, K. M., Siemann, J. K., McMahon, D. G., and Grueter, B. A. (2021). Patch-clamp and multi-electrode array electrophysiological analysis in acute mouse brain slices. STAR Protoc. 2:100442. doi: 10.1016/j.xpro.2021.100442
Mateos-Aparicio, P., Murphy, R., and Storm, J. F. (2014). Complementary functions of SK and Kv7/M potassium channels in excitability control and synaptic integration in rat hippocampal dentate granule cells. J. Physiol. 592, 669–693. doi: 10.1113/jphysiol.2013.267872
Milescu, L. S., Bean, B. P., and Smith, J. C. (2010). Isolation of somatic Na+ currents by selective inactivation of axonal channels with a voltage prepulse. J. Neurosci. 30, 7740–7748. doi: 10.1523/JNEUROSCI.6136-09.2010
Mishra, P., and Narayanan, R. (2023). The enigmatic HCN channels: a cellular neurophysiology perspective. Proteins. 1–21. doi: 10.1002/prot.26643
Murugan, M., Jang, H. J., Park, M., Miller, E. M., Cox, J., Taliaferro, J. P., et al. (2017). Combined social and spatial coding in a descending projection from the prefrontal cortex. Cell 171, 1663–77.e16. doi: 10.1016/j.cell.2017.11.002
Nam, Y. W., Downey, M., Rahman, M. A., Cui, M., and Zhang, M. (2023). Channelopathy of small- and intermediate-conductance ca(2+)-activated K(+) channels. Acta Pharmacol. Sin. 44, 259–267. doi: 10.1038/s41401-022-00935-1
Nava, C., Dalle, C., Rastetter, A., Striano, P., de Kovel, C. G., Nabbout, R., et al. (2014). De novo mutations in HCN1 cause early infantile epileptic encephalopathy. Nat. Genet. 46, 640–645. doi: 10.1038/ng.2952
Notaras, M., Hill, R., and van den Buuse, M. (2015). The BDNF gene Val66Met polymorphism as a modifier of psychiatric disorder susceptibility: progress and controversy. Mol. Psychiatry 20, 916–930. doi: 10.1038/mp.2015.27
Pappalardo, L. W., Black, J. A., and Waxman, S. G. (2016). Sodium channels in astroglia and microglia. Glia 64, 1628–1645. doi: 10.1002/glia.22967
Peters, H. C., Hu, H., Pongs, O., Storm, J. F., and Isbrandt, D. (2005). Conditional transgenic suppression of M channels in mouse brain reveals functions in neuronal excitability, resonance and behavior. Nat. Neurosci. 8, 51–60. doi: 10.1038/nn1375
Piro, E., Nardello, R., Gennaro, E., Fontana, A., Taglialatela, M., Donato Mangano, G., et al. (2019). A novel mutation in KCNQ3-related benign familial neonatal epilepsy: electroclinical features and neurodevelopmental outcome. Epileptic Disord. 21, 87–91. doi: 10.1684/epd.2019.1030
Qin, L., Ma, K., Wang, Z. J., Hu, Z., Matas, E., Wei, J., et al. (2018). Social deficits in Shank3-deficient mouse models of autism are rescued by histone deacetylase (HDAC) inhibition. Nat Neurosci. 21, 564–575. doi: 10.1038/s41593-018-0110-8
Qin, L., Ma, K., and Yan, Z. (2019). Chemogenetic activation of prefrontal cortex in Shank3-deficient mice ameliorates social deficits, NMDAR hypofunction, and Sgk2 downregulation. iScience 17, 24–35. doi: 10.1016/j.isci.2019.06.014
Qin, L., Williams, J. B., Tan, T., Liu, T., Cao, Q., Ma, K., et al. (2021). Deficiency of autism risk factor ASH1L in prefrontal cortex induces epigenetic aberrations and seizures. Nat. Commun. 12:6589. doi: 10.1038/s41467-021-26972-8
Raznahan, A., Toro, R., Proitsi, P., Powell, J., Paus, T., Bolton, P., et al. (2009). A functional polymorphism of the brain derived neurotrophic factor gene and cortical anatomy in autism spectrum disorder. J. Neurodev. Disord. 1, 215–223. doi: 10.1007/s11689-009-9012-0
Ritter-Makinson, S., Clemente-Perez, A., Higashikubo, B., Cho, F. S., Holden, S. S., Bennett, E., et al. (2019). Augmented reticular thalamic bursting and seizures in Scn1a-Dravet syndrome. Cell Rep. 26, 54–64.e6. doi: 10.1016/j.celrep.2018.12.018
Sanders, S. J., Campbell, A. J., Cottrell, J. R., Moller, R. S., Wagner, F. F., Auldridge, A. L., et al. (2018). Progress in understanding and treating SCN2A-mediated disorders. Trends Neurosci. 41, 442–456. doi: 10.1016/j.tins.2018.03.011
Sansa, G., Carlson, C., Doyle, W., Weiner, H. L., Bluvstein, J., Barr, W., et al. (2011). Medically refractory epilepsy in autism. Epilepsia 52, 1071–1075. doi: 10.1111/j.1528-1167.2011.03069.x
Santello, M., and Nevian, T. (2015). Dysfunction of cortical dendritic integration in neuropathic pain reversed by serotoninergic neuromodulation. Neuron 86, 233–246. doi: 10.1016/j.neuron.2015.03.003
Santoro, B., and Shah, M. M. (2020). Hyperpolarization-activated cyclic nucleotide-gated channels as drug targets for neurological disorders. Annu. Rev. Pharmacol. Toxicol. 60, 109–131. doi: 10.1146/annurev-pharmtox-010919-023356
Satterstrom, F. K., Kosmicki, J. A., Wang, J., Breen, M. S., De Rubeis, S., An, J. Y., et al. (2020). Large-scale exome Sequencing study implicates both developmental and functional changes in the neurobiology of Autism. Cell 180, 568–84.e23. doi: 10.1016/j.cell.2019.12.036
Schwarz, M., Ryba, L., Křepelová, A., Moslerová, V., Zelinová, M., Turnovec, M., et al. (2022). Zimmermann-Laband syndrome in monozygotic twins with a mild neurobehavioral phenotype lacking gingival overgrowth-a case report of a novel KCNN3 gene variant. Am. J. Med. Genet. A 188, 1083–1087. doi: 10.1002/ajmg.a.62616
Shansky, R. M., and Woolley, C. S. (2016). Considering sex as a biological variable will be valuable for neuroscience research. J. Neurosci. 36, 11817–11822. doi: 10.1523/JNEUROSCI.1390-16.2016
Soh, H., Pant, R., LoTurco, J. J., and Tzingounis, A. V. (2014). Conditional deletions of epilepsy-associated KCNQ2 and KCNQ3 channels from cerebral cortex cause differential effects on neuronal excitability. J. Neurosci. 34, 5311–5321. doi: 10.1523/JNEUROSCI.3919-13.2014
Soldovieri, M. V., Boutry-Kryza, N., Milh, M., Doummar, D., Heron, B., Bourel, E., et al. (2014). Novel KCNQ2 and KCNQ3 mutations in a large cohort of families with benign neonatal epilepsy: first evidence for an altered channel regulation by syntaxin-1A. Hum. Mutat. 35, 356–367. doi: 10.1002/humu.22500
Song, M., Martinowich, K., and Lee, F. S. (2017). BDNF at the synapse: why location matters. Mol. Psychiatry 22, 1370–1375. doi: 10.1038/mp.2017.144
Sontheimer, H., and Ransom, C. B. (2002). “Whole-cell patch-clamp recordings” in Patch-clamp analysis. eds. W. Walz, A. A. Boulton, and G. B. Baker (New Jersey: Humana Press), 35–68.
Spratt, P. W. E., Alexander, R. P. D., Ben-Shalom, R., Sahagun, A., Kyoung, H., Keeshen, C. M., et al. (2021). Paradoxical hyperexcitability from Na(V)1.2 sodium channel loss in neocortical pyramidal cells. Cell Rep. 36:109483. doi: 10.1016/j.celrep.2021.109483
Spratt, P. W. E., Ben-Shalom, R., Keeshen, C. M., Burke, K. J., Clarkson, R. L., Sanders, S. J., et al. (2019). The Autism-associated gene Scn2a contributes to dendritic excitability and synaptic function in the prefrontal cortex. Neuron 103, 673–85.e5. doi: 10.1016/j.neuron.2019.05.037
Stoner, R., Chow, M. L., Boyle, M. P., Sunkin, S. M., Mouton, P. R., Roy, S., et al. (2014). Patches of disorganization in the neocortex of children with autism. N. Engl. J. Med. 370, 1209–1219. doi: 10.1056/NEJMoa1307491
Sun, Q., Jiang, Y. Q., and Lu, M. C. (2020). Topographic heterogeneity of intrinsic excitability in mouse hippocampal CA3 pyramidal neurons. J. Neurophysiol. 124, 1270–1284. doi: 10.1152/jn.00147.2020
Sun, J., Liu, Y., Baudry, M., and Bi, X. (2020). SK2 channel regulation of neuronal excitability, synaptic transmission, and brain rhythmic activity in health and diseases. Biochim. Biophys. Acta, Mol. Cell Res. 1867:118834. doi: 10.1016/j.bbamcr.2020.118834
Surges, R., Brewster, A. L., Bender, R. A., Beck, H., Feuerstein, T. J., and Baram, T. Z. (2006). Regulated expression of HCN channels and cAMP levels shape the properties of the h current in developing rat hippocampus. Eur. J. Neurosci. 24, 94–104. doi: 10.1111/j.1460-9568.2006.04880.x
Tatsukawa, T., Raveau, M., Ogiwara, I., Hattori, S., Miyamoto, H., Mazaki, E., et al. (2019). Scn2a haploinsufficient mice display a spectrum of phenotypes affecting anxiety, sociability, memory flexibility and ampakine CX516 rescues their hyperactivity. Mol. Autism. 10:15. doi: 10.1186/s13229-019-0265-5
Viscidi, E. W., Triche, E. W., Pescosolido, M. F., McLean, R. L., Joseph, R. M., Spence, S. J., et al. (2013). Clinical characteristics of children with autism spectrum disorder and co-occurring epilepsy. PLoS One 8:e67797. doi: 10.1371/journal.pone.0067797
Wang, X., Bey, A. L., Katz, B. M., Badea, A., Kim, N., David, L. K., et al. (2016). Altered mGluR5-Homer scaffolds and corticostriatal connectivity in a Shank3 complete knockout model of autism. Nat. Commun. 7:11459. doi: 10.1038/ncomms11459
Wang, T., Hoekzema, K., Vecchio, D., Wu, H., Sulovari, A., Coe, B. P., et al. (2020). Large-scale targeted sequencing identifies risk genes for neurodevelopmental disorders. Nat. Commun. 11:4932. doi: 10.1038/s41467-020-18723-y
Werling, D. M., and Geschwind, D. H. (2013). Sex differences in autism spectrum disorders. Curr. Opin. Neurol. 26, 146–153. doi: 10.1097/WCO.0b013e32835ee548
Wu, X. B., Liang, B., and Gao, Y. J. (2016). The increase of intrinsic excitability of layer V pyramidal cells in the prelimbic medial prefrontal cortex of adult mice after peripheral inflammation. Neurosci. Lett. 611, 40–45. doi: 10.1016/j.neulet.2015.11.030
Yamagata, T., Ogiwara, I., Mazaki, E., Yanagawa, Y., and Yamakawa, K. (2017). Nav1.2 is expressed in caudal ganglionic eminence-derived disinhibitory interneurons: mutually exclusive distributions of Nav1.1 and Nav1.2. Biochem. Biophys. Res. Commun. 491, 1070–1076. doi: 10.1016/j.bbrc.2017.08.013
Yap, E. L., and Greenberg, M. E. (2018). Activity-regulated transcription: bridging the gap between neural activity and behavior. Neuron 100, 330–348. doi: 10.1016/j.neuron.2018.10.013
Yizhar, O., Fenno, L. E., Prigge, M., Schneider, F., Davidson, T. J., O'Shea, D. J., et al. (2011). Neocortical excitation/inhibition balance in information processing and social dysfunction. Nature 477, 171–178. doi: 10.1038/nature10360
Yoo, H. J., Yang, S. Y., Cho, I. H., Park, M., and Kim, S. A. (2014). Polymorphisms of BDNF gene and autism spectrum disorders: family based association study with korean trios. Psychiatry Investig. 11, 319–324. doi: 10.4306/pi.2014.11.3.319
Zhang, J., Chen, X., Eaton, M., Wu, J., Ma, Z., Lai, S., et al. (2021). Severe deficiency of the voltage-gated sodium channel Na(V)1.2 elevates neuronal excitability in adult mice. Cell Rep. 36:109495. doi: 10.1016/j.celrep.2021.109495
Zhang, W., and Linden, D. J. (2003). The other side of the engram: experience-driven changes in neuronal intrinsic excitability. Nat. Rev. Neurosci. 4, 885–900. doi: 10.1038/nrn1248
Zhong, P., Cao, Q., and Yan, Z. (2022). Selective impairment of circuits between prefrontal cortex glutamatergic neurons and basal forebrain cholinergic neurons in a tauopathy mouse model. Cereb. Cortex 32, 5569–5579. doi: 10.1093/cercor/bhac036
Keywords: activity-dependent neural signaling, BDNF, Val66Met polymorphism, intrinsic neuronal excitability, sex
Citation: Ma K, Zhang D, McDaniel K, Webb M, Newton SS, Lee FS and Qin L (2024) A sexually dimorphic signature of activity-dependent BDNF signaling on the intrinsic excitability of pyramidal neurons in the prefrontal cortex. Front. Cell. Neurosci. 18:1496930. doi: 10.3389/fncel.2024.1496930
Edited by:
Pierre Szepetowski, INSERM U901 Institut de Neurobiologie de la Méditerranée, FranceReviewed by:
Ernesto Griego, Albert Einstein College of Medicine, United StatesZhongjiao Jiang, University at Buffalo, United States
Igor Medina, Institut National de la Santé et de la Recherche Médicale (INSERM), France
Copyright © 2024 Ma, Zhang, McDaniel, Webb, Newton, Lee and Qin. This is an open-access article distributed under the terms of the Creative Commons Attribution License (CC BY). The use, distribution or reproduction in other forums is permitted, provided the original author(s) and the copyright owner(s) are credited and that the original publication in this journal is cited, in accordance with accepted academic practice. No use, distribution or reproduction is permitted which does not comply with these terms.
*Correspondence: Luye Qin, THV5ZS5RaW5AdXNkLmVkdQ==