- 1Department of Neurosciences, Université de Montréal, Montréal, QC, Canada
- 2Centre Interdisciplinaire de Recherche sur le Cerveau et l’Apprentissage (CIRCA), Montréal, QC, Canada
- 3Department of Stomatology, Université de Montréal, Montréal, QC, Canada
Information storage and transfer in the brain require a high computational power. Neuronal network display various local or global mechanisms to allow information storage and transfer in the brain. From synaptic to intrinsic plasticity, the rules of input–output function modulation have been well characterized in neurons. In the past years, astrocytes have been suggested to increase the computational power of the brain and we are only just starting to uncover their role in information processing. Astrocytes maintain a close bidirectional communication with neurons to modify neuronal network excitability, transmission, axonal conduction, and plasticity through various mechanisms including the release of gliotransmitters or local ion homeostasis. Astrocytes have been significantly studied in the context of long-term or short-term synaptic plasticity, but this is not the only mechanism involved in memory formation. Plasticity of intrinsic neuronal excitability also participates in memory storage through regulation of voltage-gated ion channels or axonal morphological changes. Yet, the contribution of astrocytes to these other forms of non-synaptic plasticity remains to be investigated. In this review, we summarized the recent advances on the role of astrocytes in different forms of plasticity and discuss new directions and ideas to be explored regarding astrocytes-neuronal communication and regulation of plasticity.
Introduction
The rules governing changes in synaptic and intrinsic plasticity are diverse and complex, sometimes synergistic and sometimes not (Debanne et al., 2019). Most studies have been neuro-centric, despite growing evidence that astrocytes can intervene or interact to modify or modulate synaptic transmission (Araque et al., 1998; Jourdain et al., 2007; Bonansco et al., 2011), input integration, neuronal excitability (Tan et al., 2017), spike waveform or axonal conductivity (Sasaki et al., 2011; Lezmy et al., 2021). Astrocytes can detect neuronal activity, and depending on the firing rate of action potentials (APs), they can not only release gliotransmitters such as adenosine or glutamate (Hamilton et al., 2008; Lezmy et al., 2021), but also trigger intracellular calcium ([Ca2+]i) oscillations at different frequencies (Pasti et al., 1997). Thanks to recent technical advances these [Ca2+]i responses can now be measured with great precision, such as in the recent approach published by the group of Volterra allowing for 3D calcium imaging (Savtchouk et al., 2018). In this review, we focus on the forms of synaptic and intrinsic plasticity that have not received much attention in relation to astrocyte involvement. Foremost, there is a more physiological form of synaptic plasticity known as spike timing-dependent plasticity (STDP), demonstrated both in vivo and in vitro across a range of species from rodents to humans. STDP is particularly relevant because it is believed to underlie spatial and episodic memory in the hippocampus. STDP is known to be affected by factors such as neuromodulation (Brzosko et al., 2019), extracellular calcium levels (Inglebert et al., 2020; Inglebert and Debanne, 2021), and activity patterns, all of which can be influenced by astrocytes through various mechanisms discussed below. Since synaptic plasticity is not the only mechanism involved in memory formation, we also discuss the often-overlooked potential role of astrocytes in intrinsic excitability. Plasticity of intrinsic excitability can act through numerous mechanisms such as voltage-gated ion channels (VGIC) regulation or the plasticity of excitable axonal domains. Synaptic and intrinsic plasticity are closely related, as changes in one will inevitably impact the other.
Astrocytes and spike timing-dependent plasticity
Spike timing-dependent plasticity is a form of synaptic plasticity where changes in synaptic strength are influenced by the precise timing of activity between pre- and post-synaptic neurons. Generally, when pre-synaptic activity precedes post-synaptic activity, it results in long-term potentiation (t-LTP). Conversely, when post-synaptic activity comes before pre-synaptic activity, it leads to long-term depression (t-LTD). Only a few studies, listed in Table 1, have begun exploring the role of astrocytes in this process. One pioneering study examining inputs from layer 4 to layer 2/3 (L4-2/3) in the barrel cortex demonstrated that astrocytes are required for t-LTD (Min and Nevian, 2012). During t-LTD induction, the level of intracellular calcium increases in astrocytes due to activation of cannabinoid-1 receptors (CB1Rs). This calcium increase prompts the release of glutamate, which then activates presynaptic NMDA receptors (pre-NMDARs) to induce t-LTD (see Figure 1H). In addition, electrical stimulation of astrocytes with a series of depolarizing pulses alone can induce long-term depression (LTD) in nearby pyramidal neurons. Interestingly, at corticostriatal synapses, STDP is controlled by endocannabinoid (eCB) levels (Cui et al., 2016). One might speculate whether astrocytic CB1Rs are also implicated in this process. Following this study, astrocytes’ involvement in STDP through pre-NMDARs has been reported in other brain regions. At hippocampal CA3-CA1 synapses, a developmental switch from t-LTD to a presynaptic form of t-LTP involving adenosine and glutamate release from astrocytes was shown to occur (Falcón-Moya et al., 2020; see Figure 1H). A similar observation has been recently made at L4-2/3 synapses in the somatosensory cortex (Martínez-Gallego et al., 2022). Interestingly, the loss of adenosine receptors is associated with lack of t-LTD in the hippocampus (Pérez-Rodríguez et al., 2019), highlighting the importance of adenosine in synaptic plasticity, which has been recently reviewed here (Martínez-Gallego and Rodríguez-Moreno, 2024). The role of astrocytes in glutamate clearance has also been shown to be essential for STDP. In the striatum, blockade of the astrocytic excitatory amino-acid transporter type-2 (EAAT2) leads to a form of timing-independent LTP while its overexpression prevents any plasticity from occurring (Valtcheva and Venance, 2016). Despite these recent advances, the role of astrocytes in STDP at most of the synapses is still overlooked. For example, a recent study has identified two new forms of t-LTD at synapses between the entorhinal cortex and dentate gyrus, both of which require astrocyte activity (Cuaya et al., 2024). In addition, in the hippocampus, spontaneous glutamate release from astrocytes has been proposed to control the threshold of t-LTP (Bonansco et al., 2011). Noteworthy, specialized hippocampal astrocytes able to rapidly (on the subsecond scale) release glutamate at precise hotspots have been recently identified (De Ceglia et al., 2023). STDP has been shown to exist at the scale of the single spine (Tazerart et al., 2020) and astrocytes could easily be involved in the local control of plasticity at the level of a single synapse. This calls to revisit past work in the light of this recent evidence. Moving forward, it is crucial to always take into account the potential role of astrocytes while aiming to develop a proper plasticitome (Sjöström, 2021). In addition, all the work mentioned previously (and summarized in Table 1) has been focused on excitatory to excitatory synapses, but astrocytes are known to respond to inhibitory activity (Perea et al., 2016). STDP exists in different forms depending on the synapse considered (Feldman, 2012) and astrocytes participation will likely be different as well.
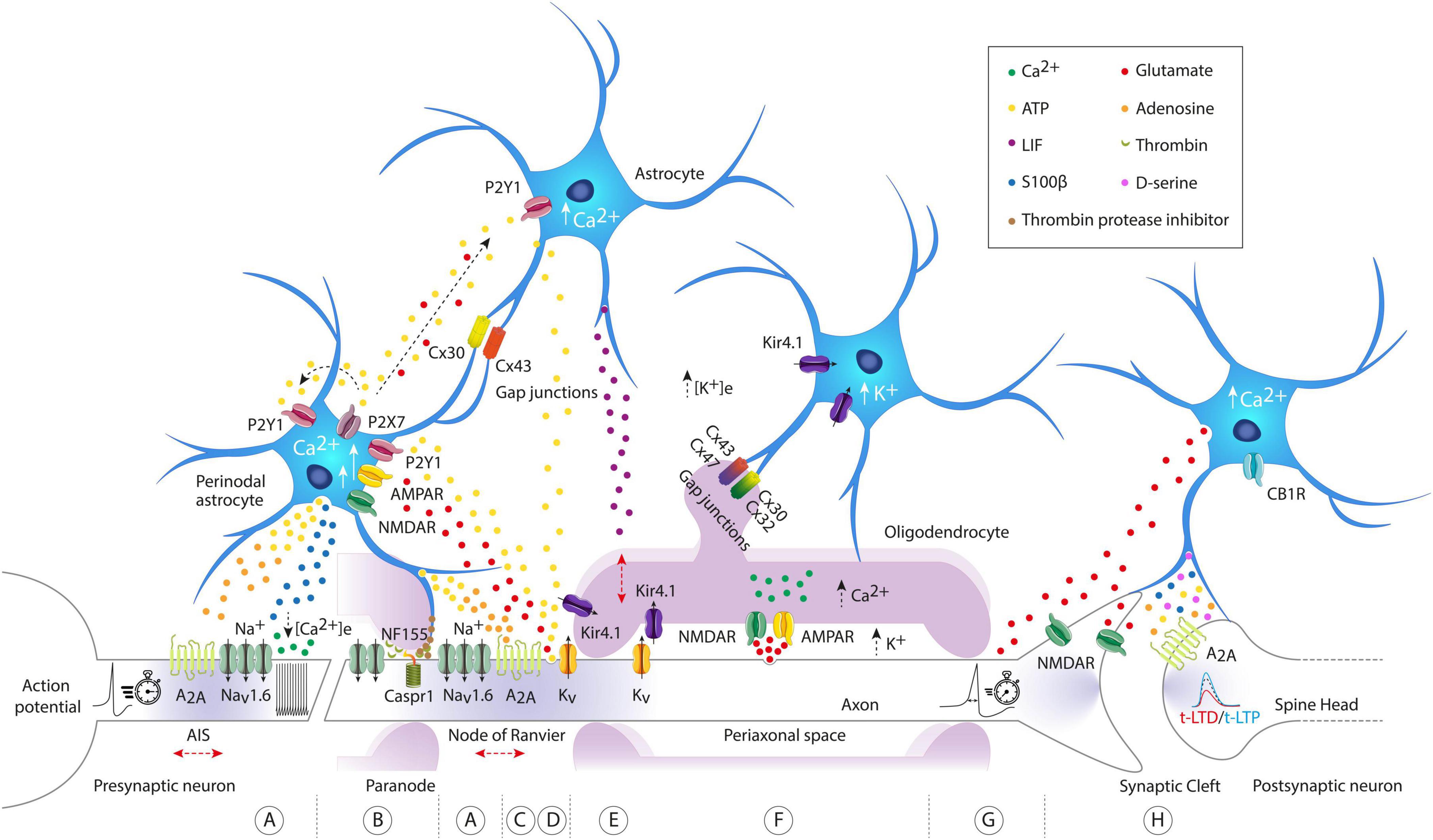
Figure 1. Molecular architecture of axon-astrocyte-oligodendrocyte communication pathways regulating intrinsic excitability and synaptic plasticity. (A) Astrocyte activated by arrival of action potentials (APs), can release ATP/adenosine to activate A2A axonal receptors, regulating both neuronal excitability at the axon initial segment (AIS) and the conduction velocity (CV) of the AP at the nodes of Ranvier. They can also release S100β, decrease the concentration of extracellular calcium ([Ca2+]e), and modify neuronal firing patterns. (B) Through the vesicular release of protease thrombin inhibitors, perinodal astrocytes can regulate the binding of myelin to the axon, precisely at the binding site of the cell adhesion molecule NF155 and Caspr1, interfering with the detachment of the outer paranodal loops and the widening of the nodal space. The widening of the nodal space causes lateral diffusion of voltage-gated Na+ channels and a slowing of AP CV. (C,D) Astrocytic [Ca2+]i signaling is evoked by axonal release of ATP and glutamate driven by APs. Axonal glutamate evokes small astroglial Ca2+ signals directly by acting on NMDA and AMPA receptors (short arrow). In contrast, axonal ATP induces large Ca2+ signals in astrocytes (long arrow), further stimulating the release of ATP and glutamate, which act as gliotransmitters in an autocrine and paracrine manner, propagating the signal to neighboring astrocytes. Note that astrocytes are highly interconnected through gap junction channels composed of connexin 30 (Cx30) and connexin 43 (Cx43). Axonal ATP release induces astrocytic secretion of LIF, which subsequently enhances myelination. (E,F) Oligodendrocytes can detect and respond to axonal activity either through axonal K+ release or through vesicular axonal glutamate release. In the first case, the axonal K+ influx pathway exits from the juxtaparanodal axolemma to be transported to the astrocytic syncytium, first through oligodendroglial Kir4.1 channels, then crossing successive paranodal loops, and finally reaching through astrocyte/oligodendrocyte gap junctions. Note that the uptake of K+ released by the axons is mostly performed by astroglial Kir4.1 channels. In the second case, APs can induce vesicular release of axonal glutamate, activating internodal NMDA and AMPA receptors, leading to an increase in [Ca2+]i in oligodendrocytes. (G) The release of astroglial glutamate can modify the AP waveform. (H) Astrocytes exhibit elevated [Ca2+]i levels through activation of CB1R. This calcium increase triggers glutamate release, which then activates presynaptic NMDA receptors to induce t-LTD (time-dependent long-term depression). Note that astrocytes can also spontaneously release glutamate and induce slow inward currents (SICs) in adjacent neurons, mediated by extrasynaptic NMDA receptors. Other gliotransmitters such as D-serine, ATP, adenosine, and S100β have been illustrated for their impact and modulation of synaptic plasticity. Observe that the red arrows indicate potential structural changes in excitable axonal domains. AIS, axon initial segment; ATP, adenosine triphosphate; A2A, adenosine A2a receptor; NF155, neurofascin 155; Caspr1, contactin-associated protein-1; Kir4.1, inward rectifier potassium channels; CB1R, cannabinoid receptor-1; LIF, leukemia inhibitory factor; P2Y1-P2X7, two types of purinergic receptors.
Astrocytes ion homeostasis on neuronal activity
Synaptic plasticity (including t-LTP and t-LTD) require post-synaptic calcium elevation to occur (Lisman, 1989; Nevian and Sakmann, 2006). One important function of astrocytes is to regulate local ion homeostasis such as extracellular calcium ([Ca2+]e) or potassium ([K+]e). Astrocytes can tightly control extracellular Ca2+ through S100β release (Morquette et al., 2015). S100β is often used as a specific astrocytic marker (Zhang et al., 2019) but is also a calcium-binding protein (Hermann et al., 2012). For example, optogenetic activation of astrocytes in layer 5 of the visual cortex has been shown to decrease [Ca2+]e specifically through the release of S100β (Ryczko et al., 2021). Interestingly, [Ca2+]e has been shown to be critical for normal expression of LTP and LTD in the hippocampus and the cortex (Forsberg et al., 2019; Inglebert et al., 2020; Inglebert and Debanne, 2021; Chindemi et al., 2022). At CA3-CA1 synapses, reduction of [Ca2+]e to physiological levels (1.3–1.8 mM) can prevent t-LTP, promote t-LTD, or even block the induction of plasticity (Inglebert et al., 2020). Then, S100β could locally modulate synaptic plasticity. In line with this idea, mutant mice lacking S100β display increased LTP and S100β level is increased following tetanization in the CA1 region of the hippocampus (Nishiyama et al., 2002; Pustylnyak et al., 2011). Further evidence from in vivo studies revealed that S100β overexpression is associated with impaired performance on various spatial memory tests, indicating hippocampal dysfunction (Gerlai and Roder, 1993; Gerlai et al., 1994, 1995). S100β has also been shown to support and regulate cognitive flexibility (the ability to switch between cognitively demanding tasks) in the medial prefrontal cortex (mPFC) (Brockett et al., 2018). Moreover, local infusion of S100β in the mPFC enhanced task performance. However, the mechanisms through which S100β regulates synaptic plasticity remain poorly understood. All indications are that a local reduction in [Ca2+]e will significantly affect the post-synaptic Ca2+ elevation necessary to trigger LTP or LTD (Lisman, 1989). Still, S100β might also influence synaptic plasticity through other targets. Ionic conductance, such as the A-type current (IA) carried by the potassium channel Kv 4.2, counteract the backpropagation of AP (bAP) (Hoffman and Johnston, 1998; Goldberg et al., 2003) and its inhibition facilitate LTP (Ramakers and Storm, 2002; Watanabe et al., 2002). Interestingly, S100β has been shown to inhibit A-type current in dopaminergic neurons (Bancroft et al., 2022). On the other hand, it also increases L-type voltage-gated Ca2+ channels (VGCCs) which have been described as required for long-lasting synaptic plasticity (Huang and Malenka, 1993; Kim et al., 2015). Reduction of [Ca2+]e by S100β release also shifts the activation threshold of NaV 1.6 channels (Morquette et al., 2015; Ryczko et al., 2021; Figure 1A) which have been shown to promote Ca2+ entry in dendritic spines (Stuart and Sakmann, 1995; Araya et al., 2007). More than just a simple Ca2+-binding protein, S100β could exert multiple antagonistic or synergistic effects on synaptic plasticity. It might even transcend this role, aligning with the concept that other gliotransmitters act as local controllers of neuronal excitability and firing patterns (Morquette et al., 2015; Ryczko et al., 2021). Its role in excitability has also been demonstrated in vivo as S100β-knockout mice (S100β KO) exhibit more epileptic seizures than wild-type (WT) mice (Dyck et al., 2002) and enhanced kainate-induced gamma oscillations, while the latter effect is reproduced by applications of anti-S100β antibodies in WT (Sakatani et al., 2007, 2008). Nonetheless, additional studies will be required to better understand the role of S100β and its spatiotemporal release dynamics in response to neuronal activity.
Given the importance of [K+]e in neuronal excitability and the classical role of astrocytes as active regulators of extracellular K+ homeostasis, astrocytes can be inferred to exert primary control over neuronal activity. However, many studies on astrocytic signaling have primarily focused on gliotransmitters, with very few investigating extracellular K+ measurements and almost none examining axonal physiology, both of which are key elements in intrinsic neuronal excitability. Astrocytic [Ca2+]i signaling has been linked to an increase in K+ uptake, resulting in a transient decrease in [K+]o, particularly in response to modest increases in extracellular K+ (5 mM), but not when K+ rises to pathological concentrations (Wang et al., 2012). Indeed, massive elevations of [K+]o have been shown to cause axonal conduction failures (Meeks and Mennerick, 2004) or induce astrocyte swelling, reducing the size of the extracellular space and significantly increasing neuronal excitability (Walch et al., 2022). Under normal physiological levels of axonal activity (stimulation at 1–10 Hz), the uptake of K+ released by axons is mainly carried out by astroglial inward rectifier potassium channels (Kir4.1) (see Figure 1F). These receptors are important for both K+ uptake and redistribution back to the extracellular space for subsequent uptake by axons (Djukic et al., 2007; Bay and Butt, 2012). The importance of astroglial Kir4.1 in K+ clearance at higher activity frequencies (up to 20 Hz) is also crucial for signal transmission fidelity (Bay and Butt, 2012). Moreover, Kir4.1 knockout mice specifically in astrocytes exhibited seizures, indicating Kir4.1 involvement in K+ spatial buffering and neuronal excitability control (Djukic et al., 2007).
In addition to regulating [Ca2+]e and [K+]e, it is important to note a new area of exploration emerging where recent studies are examining lactate transport between astrocytes and neurons. These studies show how lactate can influence excitability, synaptic plasticity (Dembitskaya et al., 2022), and neuronal activity through various mechanisms (see Cauli et al., 2023 for review).
Gliotransmitters and STDP
Synaptic plasticity has been extensively shown to be impacted and modulated by gliotransmission (see Araque et al., 2014 for review). As demonstrated in vivo, selectively expressing tetanus neurotoxin (TeNT) in astrocytes to inhibit gliotransmission led to impaired behavioral and cognitive performance (Lee et al., 2014). One of the most studied, D-serine, has been extensively shown to impact LTP in the hippocampus and the cortex (Yang et al., 2003; Henneberger et al., 2010; Fossat et al., 2012). Noteworthy, D-serine is required for t-LTD at CA3-CA1 synapses and for t-LTP at hippocampal mossy fiber synapses (Rebola et al., 2011; Andrade-Talavera et al., 2016). Similarly, adenosine triphosphate (ATP) has been shown to mediate striatal t-LTP through postsynaptic A2a receptors (see Figure 1H) and to increase LTP amplitude through A1 receptors in the visual cortex (Shen et al., 2008; Bannon et al., 2017). Despite recent advances, additional studies are still required to better understand the glial control of STDP.
Gliotransmitters and neuronal excitability
Adenosine triphosphate and glutamate can both be released from axons propagating APs or as gliotransmitters from astroglia (see Figure 1C). Glutamate released from the axonal compartment evokes only small astroglial Ca2+ signals directly, acting on NMDA and AMPA receptors to stimulate ATP release as a gliotransmitter, thereby indirectly inducing astroglial Ca2+ signals. Conversely, axonal ATP induces large Ca2+ signals in astrocytes, further stimulating the release of ATP and glutamate in an autocrine and paracrine manner, amplifying the axonal signal and propagating it to neighboring astrocytes (Hamilton et al., 2008). Astrocytes are highly interconnected through gap junction channels composed of connexin 30 (Cx30) and connexin 43 (Cx43), regulated by extracellular and intracellular signals that allow information exchange (see Giaume et al., 2010 for review). These astroglial networks can influence neuronal activity. One study showed that the selective elimination of Cx30 and Cx43 in astrocytes reduced the excitability of CA1 hippocampal neurons (Hösli et al., 2022), while another only removed astroglial Cx43 also observed a decrease in the excitability of orexin neurons located in the lateral hypothalamic area (Clasadonte et al., 2017). At the physiological level, astroglial ATP/adenosine release can activate axonal A2A receptors, regulating both neuronal excitability and AP conduction velocity (CV) (Lezmy et al., 2021), while astroglial glutamate release can modify the AP waveform (Sasaki et al., 2011; see Figures 1A, G). Independently of neuronal activity, astrocytes also exhibit certain forms of intrinsic activity, modulating the excitability, and synchronization of neighboring neurons. For instance, it has been shown that even in the absence of neuronal activity and neuronal vesicular transmitter release, astrocytes can spontaneously release glutamate and excite adjacent pyramidal neurons in acute hippocampal slices (Angulo et al., 2004). Furthermore, slow synchronized inward currents (SIC) were observed in neighboring neurons with processes close to the gliotransmitter release source (Angulo et al., 2004). These SICs are mediated by extrasynaptic NMDA receptors mainly composed of the NR1/NR2B complex (Fellin et al., 2004; see Figure 1H). However, the current challenge lies in determining the spatial extent of this non-synaptic excitatory mechanism. Specifically, it is difficult to establish how many neurons are affected by a single event, and whether the same neuron is activated by multiple events originating from different astrocytes.
Astrocytes, neuromodulation, and STDP
In addition to gliotransmitters, STDP is sensitive to neuromodulators such as dopamine (DA) and noradrenaline (NA), among others (see Brzosko et al., 2019 for recent review). For example, in the hippocampus, both DA and NA can extend the STDP timescale for t-LTP (Lin et al., 2003; Zhang et al., 2009). Like neurons, astrocytes have been shown to be responsive to neuromodulators. For instance, they express DA receptors, whose activation leads to changes in intracellular calcium (Corkrum and Araque, 2021). Interestingly, in the hippocampus, astrocytic D1R or D2R activation has been associated with an increase or decrease in astrocytes cytosolic calcium, respectively (Jennings et al., 2017). In the nucleus accumbens (NAc), DA-evoked astrocytes calcium signaling are associated with a decrease of excitatory synaptic transmission (Corkrum et al., 2020). However, in other brain regions, the effects on synaptic transmission and, ultimately, on synaptic plasticity remain unclear. In the hippocampus, D1R activation exerts multiple effects on t-LTP: it converts t-LTD into t-LTP, reduces t-LTP threshold, and extends t-LTP time window. In layer 5 of the prefrontal cortex, DA regulates t-LTP timing window through D1R activation and enables t-LTP through D2R activation on GABAergic interneurons to decrease inhibition. It is unknown whether astrocytes are partially or fully involved in some of these effects. Furthermore, adding to the complexity, it has been reported that astrocytes in the prefrontal cortex (PFC) respond to dopamine (DA) via α-adrenergic receptors (α-ARs), resulting in the release of ATP and likely regulating synaptic transmission (Fellin et al., 2004; Pittolo et al., 2022). The same receptor is also responsive to NA which triggers as well astrocytic calcium increases (Paukert et al., 2014; Slezak et al., 2019; Oe et al., 2020). In the visual cortex, α-ARs activation promotes an LTD-only STDP but the coactivation of α-ARs and β-ARs allows the conventional bidirectional STDP curve (Huang et al., 2013, 2014). β-ARs activation alone is sufficient to induce a robust t-LTP (Seol et al., 2007). Astrocytes express and respond as well to β-ARs activation through intracellular calcium increases (Oe et al., 2020). Interestingly, β-ARs activation has been shown to inhibit A-type current which could explain the effect on t-LTP (Yuan et al., 2002). In astrocytes, α-ARs activation has been linked to increased glutamate uptake (Hansson and Rönnbäck, 1989, 1992), reduced [K+]e clearance (Åkerman et al., 1988; Wotton et al., 2020) and even secretion of brain-derived neurotrophic factor (BDNF) (Juric et al., 2008; Koppel et al., 2018). On the contrary, β-ARs activation has been linked to increased GABA uptake (Hansson and Rönnbäck, 1989, 1992), increased [K+]e clearance (Wotton et al., 2020) and higher BDNF secretion as well. Interestingly, similar to what is observed in neurons, the level of NA exerts antagonistic effects because α-ARs have highest affinity compared to β-ARs. In the hippocampus, t-LTP can be BDNF-dependent or -independent depending respectively on high or low postsynaptic activities (Edelmann et al., 2015). This discussion could be extended to other neuromodulators such as acetylcholine or serotonin which are known to affect astrocytes calcium dynamics (Pacholko et al., 2020) and have been shown to greatly influence STDP (Seol et al., 2007; Brzosko et al., 2017). This underscores the importance of not overlooking astrocytes when studying the effects of neuromodulation on synaptic plasticity. It also invites a reconsideration of previous findings. The dynamics of neuromodulation and their impact on synaptic plasticity should be further explored within the tripartite synapse model.
Astrocytes and axonal plasticity
Hildebrand (1971), based on electron microscopy studies in the feline spinal cord, demonstrated that certain astrocytic processes are closely located at the nodes of Ranvier. Subsequently, these observations were confirmed in other animals in the following years (Waxman and Swadlow, 1976; Berthold and Carlstedt, 1977; Sims et al., 1985). These authors named these glial cells as perinodal astrocytes. Other quantitative measurements observed that the axon initial segment (AIS) of Purkinje cells is densely covered by glial processes, covering 74.2% of the total surface in cats and 85.6% in rats (Somogyu and Hámori, 1976). More recently, with the help of more advanced technology, it was confirmed that these glial processes were astrocytic (Iwakura et al., 2012). Additionally, it has also been revealed that the AIS of ganglion cells in the optic nerve receive contacts mainly from astrocytic processes (Stone et al., 1995). Today, it is widely accepted that the AIS and the nodes of Ranvier, far from being rigid structures, can undergo remodeling depending on neuronal activity. Several reviews have been published on experimental findings demonstrating the structural plasticity of these excitable axonal domains and their functional implications (see Grubb et al., 2011; Susuki and Kuba, 2016; Yamada and Kuba, 2016; Petersen et al., 2017 for review). However, the field remains immature, and periaxonal astrocytes have barely been included as key agents in this type of axonal plasticity. The first to do so was the group of Douglas Fields, pioneers in demonstrating how myelin-forming glial cells can detect neuronal impulses to optimize the speed and synchrony of information. They unexpectedly detected a link between astrocytes, myelination, and neuronal activity. They reported that ATP released by DRG neuron axons firing APs induces the release of leukemia inhibitory factor (LIF) by astrocytes, which in turn promotes myelination in late stages, beyond development (Ishibashi et al., 2006; see Figure 1D). This discovery shed light on existing hints of a possible link between astrocytic function and myelin structure, in GFAP-deficient mice that exhibited abnormalities in myelination (Liedtke et al., 1996). More recently, this same group provided a mechanism involving perinodal astrocytes as responsible for remodeling the myelin sheath and nodal structure. Specifically, perinodal astrocytes regulate myelin attachment to the axon through vesicular release of thrombin protease inhibitors, just at the binding site of neurofascin 155 (NF155) and Caspr1, interfering with the detachment of outer paranodal loops and widening of the nodal space (Dutta et al., 2018; see Figure 1B). These anatomical changes can have electrophysiological and functional consequences if astrocytes do not prevent thrombin-dependent NF155 (axoglial contact) cleavage. In this case, widening of the nodal space allowed lateral diffusion of voltage-gated Na+ channels and slowed down the CV of the AP. Additionally, it is noteworthy that neural AIS excitability was altered, and CV of APs in the nodes of Ranvier was also reduced when periaxonal astrocytes responded to the activity of myelinated neurons in layer 5 of the cerebral cortex by releasing vesicles containing ATP, subsequently converted into adenosine (Lezmy et al., 2021; see Figure 1). Consequently, these findings suggest that perinodal astrocytes could contribute to establishing appropriate myelin thickness and nodal properties essential for optimizing conduction latency in neuronal circuits. However, their involvement in AIS remodeling remains largely unexplored.
Astrocytes and oligodendrocytes: new pathways in axonal plasticity
Accumulating evidence indicates that astrocytes may be coupled to oligodendrocytes via gap junctions, forming extensive syncytia known as panglial networks (see Stephan et al., 2021 for review). As mentioned previously, astrocytes can express Cx30 and Cx43, whereas oligodendrocytes express different connexins, namely Cx47, Cx32, and Cx29, with Cx29 contributing minimally to intercellular communication (Altevogt et al., 2002; Nagy et al., 2003). Therefore, astrocyte–oligodendrocyte (A/O) coupling might predominantly be mediated by heterotypic channels: Cx43/Cx47, Cx30/Cx32, Cx43/Cx32, or Cx30/Cx47 (Orthmann-Murphy et al., 2007; Griemsmann et al., 2015). The primary function attributed to panglial networks is the modulation of neuronal excitability through the spatial buffering of K+ (Kamasawa et al., 2005; Battefeld et al., 2016). Similar to astrocytes, oligodendrocytes can detect and respond to axonal activity, either via the release of axonal K+ (Kamasawa et al., 2005; Looser et al., 2024) or through vesicular release of axonal glutamate (Micu et al., 2016; see Figures 1E, F). In the first case, following an AP, the pathway for axonal K+ flow not only exits the nodes of Ranvier toward the astrocytic endfeet, as was initially thought when myelin was considered to play a passive role (Waxman and Black, 1984), but predominantly occurs in the juxtaparanodal axolemma, the area enclosed within the myelin sheaths. Once released, the influx of K+ is transported then to the astrocytic syncytium, first through oligodendroglial Kir4.1 channels (Looser et al., 2024), then crossing successive paranodal loops, and finally through A/O junctions. This is presumably driven by an electrochemical force due to the high difference in electrical potential (+40 to +74 mV in the internodal compartment versus −85 mV in the coupled astrocytic syncytium). Another portion of the axonal K+ influx returns to the axon (Kamasawa et al., 2005). In the second case, high-resolution two-photon microscopy has shown that APs induce vesicular release of axonal glutamate, activating internodal NMDA receptors (containing GluN2D and GluN3A) and leading to an increase in intracellular Ca2+ in oligodendrocytes (Micu et al., 2016). Several studies have demonstrated that these axon-oligodendrocyte-astrocyte signaling mechanisms can produce biophysical changes in the fine structure of myelin, as well as modulate neuronal excitability and axonal conduction (Yamazaki et al., 2010; Battefeld et al., 2016; Micu et al., 2016). Additionally, there has been speculation that certain abnormalities in these intercellular interactions may trigger pathologies such as schizophrenia, multiple sclerosis, or epilepsy (Lundgaard et al., 2013; Bedner et al., 2015; Micu et al., 2016). Despite these promising advances, much work remains to be done for identifying which elements can locally modulate axonal plasticity for physiological purposes and which may play a more pathological role.
Astrocytes in others forms of plasticity
While our review focuses on astrocyte interactions with synaptic plasticity (STDP) and intrinsic plasticity, it is important to note that astrocytes also play roles in other forms of plasticity, such as homeostatic plasticity and synaptic scaling, both crucial for maintaining brain activity homeostasis in response to prolonged internal or external disturbances and ensuring a proper excitatory–inhibitory (E/I) balance (Turrigiano, 2007; Perez-Catalan et al., 2021). Several gliotransmitters, including SPARC (secreted protein acidic and rich in cysteine) and the cytokine IL-33, have been implicated in normal synaptic scaling. Both are essential for enhancing activity following deprivation induced by TTX (Jones et al., 2011; Wang et al., 2021). Considerable research has also focused on tumor necrosis factor α (TNF-α), whose secretion is essential for synaptic scaling (Stellwagen and Malenka, 2006; Heir and Stellwagen, 2020). In hippocampal cultures, astrocytes—but not microglia—produce TNF-α to support homeostatic plasticity in response to chronic TTX treatment (Heir et al., 2024). Additionally, astrocytes contribute to maintaining network homeostasis by participating in activity-dependent synapse remodeling, which involves both the elimination and formation of synapses through various mechanisms, as recently reviewed by Kim and Chung (2023).
In vivo consideration
Although this review primarily focused on in vitro studies, a significant amount of in vivo research was also conducted, particularly regarding the role of astrocytes in brain rhythms. Logically, since brain oscillations arise from synchronized neuronal activity, the previously described effect of astrocytes could influence them. In line with this idea, gliotransmitters have been associated to the modulation of brain rhythms. For instance, in a mouse model with impaired astrocyte-specific exocytosis, theta synchronization between the dorsal hippocampus and prefrontal cortex was disrupted, resulting in decreased performance in spatial learning and reference memory (Sardinha et al., 2017). In contrast, gamma activity in the somatosensory cortex is enhanced in a mouse model with impaired astrocyte calcium signaling, while it decreases during the pharmacological activation of astrocytes (Lines et al., 2020). Specifically, the genetic ablation of GABAB receptors on astrocytes resulted in a decline in decision-making performance and a reduction in low-gamma power (Mederos et al., 2021). Here, we focused on astrocytes, but it is worth noting that oligodendrocytes, through the modulation of myelin sheaths, and microglia, through ion buffering, also play important roles in modulating brain oscillations (Lewen et al., 2020; Dubey et al., 2022). The role of glial cells in brain rhythms and their implications for memory storage and processing in health and diseases have been excellently reviewed recently (Phatnani and Maniatis, 2015; Andrade-Talavera et al., 2023; Brandebura et al., 2023).
Conclusion
The role of astrocytes in various forms of plasticity is only beginning to be understood, and there is much more to uncover. Previous studies could be revisited to reassess the potential role of astrocytes, and future research should always consider their involvement. From synaptic to intrinsic or even axonal plasticity, astrocytes appear to have a significant influence (see Figure 1). Our discussion could extend to other forms, such as homeostatic plasticity, and to other glial cells, such as oligodendrocytes, which we briefly mentioned. One thing is certain: ideas, projects, and experiments will never cease for those intrigued by neuron–glia communication, as it now appears that they are not mere conjectures, nor are we so unequipped to fathom the function of glial cells, echoing the sentiments of Santiago Ramón y Cajal over a century ago.
Author contributions
RS-G: Writing – original draft, Writing – review & editing. DF: Writing – original draft, Writing – review & editing. AK: Project administration, Resources, Writing – review & editing. YI: Conceptualization, Project administration, Supervision, Validation, Writing – original draft, Writing – review & editing.
Funding
The author(s) declare financial support was received for the research, authorship, and/or publication of this article. This work was supported by the Canadian Institutes of Health Research (165900), Natural Sciences and Engineering Research Council of Canada (RGPIN/05255-2020), CIRCA post-doctoral fellowship to YI, and FRQNT doctoral scholarship to DF.
Acknowledgments
We thank Dr. Dorly Verdier for critically reading a preliminary version of the manuscript and members of AK Laboratories for comments on the manuscript. Parts of Figure 1 were used or adapted from Servier Medical Art, licensed under a CC BY 4.0 (https://creativecommons.org/licenses/by/4.0/).
Conflict of interest
The authors declare that the research was conducted in the absence of any commercial or financial relationships that could be construed as a potential conflict of interest.
The author(s) declared that they were an editorial board member of Frontiers, at the time of submission. This had no impact on the peer review process and the final decision.
Publisher’s note
All claims expressed in this article are solely those of the authors and do not necessarily represent those of their affiliated organizations, or those of the publisher, the editors and the reviewers. Any product that may be evaluated in this article, or claim that may be made by its manufacturer, is not guaranteed or endorsed by the publisher.
References
Åkerman, K. E. O., Enkvist, M. O. K., and Holopainen, I. (1988). Activators of protein kinase C and phenylephrine depolarize the astrocyte membrane by reducing the K+ permeability. Neurosci. Lett. 92, 265–269. doi: 10.1016/0304-3940(88)90600-3
Altevogt, B. M., Kleopa, K. A., Postma, F. R., Scherer, S. S., and Paul, D. L. (2002). Connexin29 is uniquely distributed within myelinating glial cells of the central and peripheral nervous systems. J. Neurosci. 22, 6458–6470. doi: 10.1523/JNEUROSCI.22-15-06458.2002
Andrade-Talavera, Y., Duque-Feria, P., Paulsen, O., and Rodríguez-Moreno, A. (2016). Presynaptic spike timing-dependent long-term depression in the mouse hippocampus. Cereb. Cortex 26, 3637–3654. doi: 10.1093/cercor/bhw172
Andrade-Talavera, Y., Fisahn, A., and Rodríguez-Moreno, A. (2023). Timing to be precise? An overview of spike timing-dependent plasticity, brain rhythmicity, and glial cells interplay within neuronal circuits. Mol. Psychiatry 28, 2177–2188. doi: 10.1038/s41380-023-02027-w
Angulo, M. C., Kozlov, A. S., Charpak, S., and Audinat, E. (2004). Glutamate released from glial cells synchronizes neuronal activity in the hippocampus. J. Neurosci. 24, 6920–6927. doi: 10.1523/JNEUROSCI.0473-04.2004
Araque, A., Carmignoto, G., Haydon, P. G., Oliet, S. H. R., Robitaille, R., and Volterra, A. (2014). Gliotransmitters travel in time and space. Neuron 81, 728–739. doi: 10.1016/j.neuron.2014.02.007
Araque, A., Parpura, V., Sanzgiri, R. P., and Haydon, P. G. (1998). Glutamate-dependent astrocyte modulation of synaptic transmission between cultured hippocampal neurons. Eur. J. Neurosci. 10, 2129–2142. doi: 10.1046/j.1460-9568.1998.00221.x
Araya, R., Nikolenko, V., Eisenthal, K. B., and Yuste, R. (2007). Sodium channels amplify spine potentials. Proc. Natl. Acad. Sci. U.S.A. 104, 12347–12352. doi: 10.1073/pnas.0705282104
Bancroft, E. A., De La Mora, M., Pandey, G., Zarate, S. M., and Srinivasan, R. (2022). Extracellular S100B inhibits A-type voltage-gated potassium currents and increases L-type voltage-gated calcium channel activity in dopaminergic neurons. Glia 70, 2330–2347. doi: 10.1002/glia.24254
Bannon, N. M., Chistiakova, M., Chen, J.-Y., Bazhenov, M., and Volgushev, M. (2017). Adenosine shifts plasticity regimes between associative and homeostatic by modulating heterosynaptic changes. J. Neurosci. 37, 1439–1452. doi: 10.1523/JNEUROSCI.2984-16.2016
Battefeld, A., Klooster, J., and Kole, M. H. P. (2016). Myelinating satellite oligodendrocytes are integrated in a glial syncytium constraining neuronal high-frequency activity. Nat. Commun 7:11298. doi: 10.1038/ncomms11298
Bay, V., and Butt, A. M. (2012). Relationship between glial potassium regulation and axon excitability: A role for glial Kir4.1 channels. Glia 60, 651–660. doi: 10.1002/glia.22299
Bedner, P., Dupper, A., Hüttmann, K., Müller, J., Herde, M. K., Dublin, P., et al. (2015). Astrocyte uncoupling as a cause of human temporal lobe epilepsy. Brain 138, 1208–1222. doi: 10.1093/brain/awv067
Berthold, C. H., and Carlstedt, T. (1977). Observations on the morphology at the transition between the peripheral and the central nervous system in the cat. III. Myelinated fibres in S1 dorsal rootlets. Acta Physiol. Scand. Suppl. 446, 43–60.
Bonansco, C., Couve, A., Perea, G., Ferradas, C. Á, Roncagliolo, M., and Fuenzalida, M. (2011). Glutamate released spontaneously from astrocytes sets the threshold for synaptic plasticity. Eur. J. Neurosci. 33, 1483–1492. doi: 10.1111/j.1460-9568.2011.07631.x
Brandebura, A. N., Paumier, A., Onur, T. S., and Allen, N. J. (2023). Astrocyte contribution to dysfunction, risk and progression in neurodegenerative disorders. Nat. Rev. Neurosci. 24, 23–39. doi: 10.1038/s41583-022-00641-1
Brockett, A. T., Kane, G. A., Monari, P. K., Briones, B. A., Vigneron, P.-A., Barber, G. A., et al. (2018). Evidence supporting a role for astrocytes in the regulation of cognitive flexibility and neuronal oscillations through the Ca2+ binding protein S100β. PLoS One 13:e0195726. doi: 10.1371/journal.pone.0195726
Brzosko, Z., Mierau, S. B., and Paulsen, O. (2019). Neuromodulation of spike-timing-dependent plasticity: Past, present, and future. Neuron 103, 563–581. doi: 10.1016/j.neuron.2019.05.041
Brzosko, Z., Zannone, S., Schultz, W., Clopath, C., and Paulsen, O. (2017). Sequential neuromodulation of Hebbian plasticity offers mechanism for effective reward-based navigation. Elife 6:e27756. doi: 10.7554/eLife.27756
Cauli, B., Dusart, I., and Li, D. (2023). Lactate as a determinant of neuronal excitability, neuroenergetics and beyond. Neurobiol. Dis. 184:106207. doi: 10.1016/j.nbd.2023.106207
Chindemi, G., Abdellah, M., Amsalem, O., Benavides-Piccione, R., Delattre, V., Doron, M., et al. (2022). A calcium-based plasticity model for predicting long-term potentiation and depression in the neocortex. Nat. Commun. 13:3038. doi: 10.1038/s41467-022-30214-w
Clasadonte, J., Scemes, E., Wang, Z., Boison, D., and Haydon, P. G. (2017). Connexin 43-mediated astroglial metabolic networks contribute to the regulation of the sleep-wake cycle. Neuron 95:1365–1380.e5. doi: 10.1016/j.neuron.2017.08.022
Corkrum, M., and Araque, A. (2021). Astrocyte-neuron signaling in the mesolimbic dopamine system: The hidden stars of dopamine signaling. Neuropsychopharmacology 46, 1864–1872. doi: 10.1038/s41386-021-01090-7
Corkrum, M., Covelo, A., Lines, J., Bellocchio, L., Pisansky, M., Loke, K., et al. (2020). Dopamine-evoked synaptic regulation in the nucleus accumbens requires astrocyte activity. Neuron 105:1036–1047.e5. doi: 10.1016/j.neuron.2019.12.026
Cuaya, H. C., Martínez-Gallego, I., and Rodríguez-Moreno, A. (2024). Astrocytes mediate two forms of spike timing-dependent depression at entorhinal cortex-hippocampal synapses. Elife 13:31. doi: 10.7554/eLife.98031.1
Cui, Y., Prokin, I., Xu, H., Delord, B., Genet, S., Venance, L., et al. (2016). Endocannabinoid dynamics gate spike-timing dependent depression and potentiation. Elife 5:e13185. doi: 10.7554/eLife.13185
De Ceglia, R., Ledonne, A., Litvin, D. G., Lind, B. L., Carriero, G., Latagliata, E. C., et al. (2023). Specialized astrocytes mediate glutamatergic gliotransmission in the CNS. Nature 622, 120–129. doi: 10.1038/s41586-023-06502-w
Debanne, D., Inglebert, Y., and Russier, M. (2019). Plasticity of intrinsic neuronal excitability. Curr. Opin. Neurobiol. 54, 73–82. doi: 10.1016/j.conb.2018.09.001
Dembitskaya, Y., Piette, C., Perez, S., Berry, H., Magistretti, P. J., and Venance, L. (2022). Lactate supply overtakes glucose when neural computational and cognitive loads scale up. Proc. Natl. Acad. Sci.m U.S.A. 119, e2212004119. doi: 10.1073/pnas.2212004119
Djukic, B., Casper, K. B., Philpot, B. D., Chin, L.-S., and McCarthy, K. D. (2007). conditional knock-out of Kir4.1 leads to glial membrane depolarization, inhibition of potassium and glutamate uptake, and enhanced short-term synaptic potentiation. J. Neurosci. 27, 11354–11365. doi: 10.1523/JNEUROSCI.0723-07.2007
Dubey, M., Pascual-Garcia, M., Helmes, K., Wever, D. D., Hamada, M. S., Kushner, S. A., et al. (2022). Myelination synchronizes cortical oscillations by consolidating parvalbumin-mediated phasic inhibition. Elife 11:e73827. doi: 10.7554/eLife.73827
Dutta, D. J., Woo, D. H., Lee, P. R., Pajevic, S., Bukalo, O., Huffman, W. C., et al. (2018). Regulation of myelin structure and conduction velocity by perinodal astrocytes. Proc. Natl. Acad. Sci.m U.S.A. 115, 11832–11837. doi: 10.1073/pnas.1811013115
Dyck, R. H., Bogoch, I. I., Marks, A., Melvin, N. R., and Teskey, G. C. (2002). Enhanced epileptogenesis in S100B knockout mice. Mol. Brain Res. 106, 22–29. doi: 10.1016/S0169-328X(02)00406-0
Edelmann, E., Cepeda-Prado, E., Franck, M., Lichtenecker, P., Brigadski, T., and Leßmann, V. (2015). Theta burst firing recruits BDNF release and signaling in postsynaptic CA1 neurons in spike-timing-dependent LTP. Neuron 86, 1041–1054. doi: 10.1016/j.neuron.2015.04.007
Falcón-Moya, R., Pérez-Rodríguez, M., Prius-Mengual, J., Andrade-Talavera, Y., Arroyo-García, L. E., Pérez-Artés, R., et al. (2020). Astrocyte-mediated switch in spike timing-dependent plasticity during hippocampal development. Nat. Commun. 11:4388. doi: 10.1038/s41467-020-18024-4
Feldman, D. E. (2012). The spike-timing dependence of plasticity. Neuron 75, 556–571. doi: 10.1016/j.neuron.2012.08.001
Fellin, T., Pascual, O., Gobbo, S., Pozzan, T., Haydon, P. G., and Carmignoto, G. (2004). Neuronal synchrony mediated by astrocytic glutamate through activation of extrasynaptic NMDA receptors. Neuron 43, 729–743. doi: 10.1016/j.neuron.2004.08.011
Forsberg, M., Seth, H., Björefeldt, A., Lyckenvik, T., Andersson, M., Wasling, P., et al. (2019). Ionized calcium in human cerebrospinal fluid and its influence on intrinsic and synaptic excitability of hippocampal pyramidal neurons in the rat. J. Neurochem. 149, 452–470. doi: 10.1111/jnc.14693
Fossat, P., Turpin, F. R., Sacchi, S., Dulong, J., Shi, T., Rivet, J.-M., et al. (2012). Glial D-serine gates NMDA receptors at excitatory synapses in prefrontal cortex. Cereb. Cortex 22, 595–606. doi: 10.1093/cercor/bhr130
Gerlai, R., and Roder, J. (1993). Female specific hyperactivity in S100β transgenic mice does not habituate in open-field. Behav. Brain Res. 59, 119–124. doi: 10.1016/0166-4328(93)90157-L
Gerlai, R., Marks, A., and Roder, J. (1994). T-maze spontaneous alternation rate is decreased in S100β transgenic mice. Behav. Neurosci. 108, 100–106. doi: 10.1037/0735-7044.108.1.100
Gerlai, R., Wojtowicz, J. M., Marks, A., and Roder, J. (1995). Overexpression of a calcium-binding protein, S100 beta, in astrocytes alters synaptic plasticity and impairs spatial learning in transgenic mice. Learn. Mem. 2, 26–39. doi: 10.1101/lm.2.1.26
Giaume, C., Koulakoff, A., Roux, L., Holcman, D., and Rouach, N. (2010). Astroglial networks: A step further in neuroglial and gliovascular interactions. Nat. Rev. Neurosci. 11, 87–99. doi: 10.1038/nrn2757
Goldberg, J. H., Tamas, G., and Yuste, R. (2003). Ca2+ imaging of mouse neocortical interneurone dendrites: Ia-type K+ channels control action potential backpropagation. J. Physiol. 551, 49–65. doi: 10.1113/jphysiol.2003.042580
Griemsmann, S., Höft, S. P., Bedner, P., Zhang, J., von Staden, E., Beinhauer, A., et al. (2015). Characterization of panglial gap junction networks in the thalamus, neocortex, and hippocampus reveals a unique population of glial cells. Cereb. Cortex 25, 3420–3433. doi: 10.1093/cercor/bhu157
Grubb, M. S., Shu, Y., Kuba, H., Rasband, M. N., Wimmer, V. C., and Bender, K. J. (2011). Short- and long-term plasticity at the axon initial segment. J. Neurosci. 31, 16049–16055. doi: 10.1523/JNEUROSCI.4064-11.2011
Hamilton, N., Vayro, S., Kirchhoff, F., Verkhratsky, A., Robbins, J., Gorecki, D. C., et al. (2008). Mechanisms of ATP- and glutamate-mediated calcium signaling in white matter astrocytes. Glia 56, 734–749. doi: 10.1002/glia.20649
Hansson, E., and Rönnbäck, L. (1989). Regulation of glutamate and GABA transport by adrenoceptors in primary astroglial cell cultures. Life Sci. 44, 27–34. doi: 10.1016/0024-3205(89)90214-2
Hansson, E., and Rönnbäck, L. (1992). Adrenergic receptor regulation of amino acid neurotransmitter uptake in astrocytes. Brain Res. Bull. 29, 297–301. doi: 10.1016/0361-9230(92)90060-B
Heir, R., Abbasi, Z., Komal, P., Altimimi, H. F., Franquin, M., Moschou, D., et al. (2024). Astrocytes are the source of TNF mediating homeostatic synaptic plasticity. J. Neurosci. 44:e2278222024. doi: 10.1523/JNEUROSCI.2278-22.2024
Heir, R., and Stellwagen, D. (2020). TNF-mediated homeostatic synaptic plasticity: From in vitro to in vivo models. Front. Cell. Neurosci. 14:565841. doi: 10.3389/fncel.2020.565841
Henneberger, C., Papouin, T., Oliet, S. H. R., and Rusakov, D. A. (2010). Long-term potentiation depends on release of d-serine from astrocytes. Nature 463, 232–236. doi: 10.1038/nature08673
Hermann, A., Donato, R., Weiger, T. M., and Chazin, W. J. (2012). S100 calcium binding proteins and ion channels. Front. Pharmacol. 3:67. doi: 10.3389/fphar.2012.00067
Hildebrand, C. (1971). Ultrastructural and light-microscopic studies of the nodal region in large myelinated fibres of the adult feline spinal cord white matter. Acta Physiol. Scand. 82, 43–79. doi: 10.1111/j.1365-201X.1971.tb10978.x
Hoffman, D. A., and Johnston, D. (1998). Downregulation of transient K+ channels in dendrites of hippocampal CA1 pyramidal neurons by activation of PKA and PKC. J. Neurosci. 18, 3521–3528. doi: 10.1523/JNEUROSCI.18-10-03521.1998
Hösli, L., Binini, N., Ferrari, K. D., Thieren, L., Looser, Z. J., Zuend, M., et al. (2022). Decoupling astrocytes in adult mice impairs synaptic plasticity and spatial learning. Cell Rep. 38:110484. doi: 10.1016/j.celrep.2022.110484
Huang, S., Huganir, R. L., and Kirkwood, A. (2013). Adrenergic gating of Hebbian spike-timing-dependent plasticity in cortical interneurons. J. Neurosci. 33, 13171–13178. doi: 10.1523/JNEUROSCI.5741-12.2013
Huang, S., Rozas, C., Treviño, M., Contreras, J., Yang, S., Song, L., et al. (2014). Associative Hebbian synaptic plasticity in primate visual cortex. J. Neurosci. 34, 7575–7579. doi: 10.1523/JNEUROSCI.0983-14.2014
Huang, Y. Y., and Malenka, R. C. (1993). Examination of TEA-induced synaptic enhancement in area CA1 of the hippocampus: The role of voltage-dependent Ca2+ channels in the induction of LTP. J. Neurosci. 13, 568–576. doi: 10.1523/JNEUROSCI.13-02-00568.1993
Inglebert, Y., Aljadeff, J., Brunel, N., and Debanne, D. (2020). Synaptic plasticity rules with physiological calcium levels. Proc. Natl. Acad. Sci. U.S.A. 117, 33639–33648. doi: 10.1073/pnas.2013663117
Inglebert, Y., and Debanne, D. (2021). Calcium and spike timing-dependent plasticity. Front. Cell. Neurosci. 15:727336. doi: 10.3389/fncel.2021.727336
Ishibashi, T., Dakin, K. A., Stevens, B., Lee, P. R., Kozlov, S. V., Stewart, C. L., et al. (2006). Astrocytes promote myelination in response to electrical impulses. Neuron 49, 823–832. doi: 10.1016/j.neuron.2006.02.006
Iwakura, A., Uchigashima, M., Miyazaki, T., Yamasaki, M., and Watanabe, M. (2012). Lack of molecular-anatomical evidence for GABAergic influence on axon initial segment of cerebellar purkinje cells by the pinceau formation. J. Neurosci. 32, 9438–9448. doi: 10.1523/JNEUROSCI.1651-12.2012
Jennings, A., Tyurikova, O., Bard, L., Zheng, K., Semyanov, A., Henneberger, C., et al. (2017). Dopamine elevates and lowers astroglial Ca2+ through distinct pathways depending on local synaptic circuitry. Glia 65, 447–459. doi: 10.1002/glia.23103
Jones, E. V., Bernardinelli, Y., Tse, Y. C., Chierzi, S., Wong, T. P., and Murai, K. K. (2011). Astrocytes control glutamate receptor levels at developing synapses through SPARC–β-integrin interactions. J. Neurosci. 31, 4154–4165. doi: 10.1523/JNEUROSCI.4757-10.2011
Jourdain, P., Bergersen, L. H., Bhaukaurally, K., Bezzi, P., Santello, M., Domercq, M., et al. (2007). Glutamate exocytosis from astrocytes controls synaptic strength. Nat. Neurosci. 10, 331–339. doi: 10.1038/nn1849
Juric, D. M., Loncar, D., and Carman-Krzan, M. (2008). Noradrenergic stimulation of BDNF synthesis in astrocytes: Mediation via alpha1- and beta1/beta2-adrenergic receptors. Neurochem. Int. 52, 297–306. doi: 10.1016/j.neuint.2007.06.035
Kamasawa, N., Sik, A., Morita, M., Yasumura, T., Davidson, K. G. V., Nagy, J. I., et al. (2005). Connexin-47 and connexin-32 in gap junctions of oligodendrocyte somata, myelin sheaths, paranodal loops and Schmidt-Lanterman incisures: Implications for ionic homeostasis and potassium siphoning. Neuroscience 136, 65–86. doi: 10.1016/j.neuroscience.2005.08.027
Kim, N.-S., and Chung, W.-S. (2023). Astrocytes regulate neuronal network activity by mediating synapse remodeling. Neurosci. Res. 187, 3–13. doi: 10.1016/j.neures.2022.09.007
Kim, Y., Hsu, C.-L., Cembrowski, M. S., Mensh, B. D., and Spruston, N. (2015). Dendritic sodium spikes are required for long-term potentiation at distal synapses on hippocampal pyramidal neurons. Elife 4:e06414. doi: 10.7554/eLife.06414
Koppel, I., Jaanson, K., Klasche, A., Tuvikene, J., Tiirik, T., Pärn, A., et al. (2018). Dopamine cross-reacts with adrenoreceptors in cortical astrocytes to induce BDNF expression, CREB signaling and morphological transformation. Glia 66, 206–216. doi: 10.1002/glia.23238
Lee, H. S., Ghetti, A., Pinto-Duarte, A., Wang, X., Dziewczapolski, G., Galimi, F., et al. (2014). Astrocytes contribute to gamma oscillations and recognition memory. Proc. Natl. Acad. Sci. U.S.A. 111:1410893111. doi: 10.1073/pnas.1410893111
Lewen, A., Ta, T.-T., Cesetti, T., Hollnagel, J.-O., Papageorgiou, I. E., Chausse, B., et al. (2020). Neuronal gamma oscillations and activity-dependent potassium transients remain regular after depletion of microglia in postnatal cortex tissue. J. Neurosci. Res. 98, 1953–1967. doi: 10.1002/jnr.24689
Lezmy, J., Arancibia-Cárcamo, I. L., Quintela-López, T., Sherman, D. L., Brophy, P. J., and Attwell, D. (2021). Astrocyte Ca2+-evoked ATP release regulates myelinated axon excitability and conduction speed. Science 374:eabh2858. doi: 10.1126/science.abh2858
Liedtke, W., Edelmann, W., Bieri, P. L., Chiu, F.-C., Cowan, N. J., Kucherlapati, R., et al. (1996). GFAP is necessary for the integrity of CNS white matter architecture and long-term maintenance of myelination. Neuron 17, 607–615. doi: 10.1016/S0896-6273(00)80194-4
Lin, Y.-W., Min, M.-Y., Chiu, T.-H., and Yang, H.-W. (2003). Enhancement of associative long-term potentiation by activation of β-adrenergic receptors at CA1 synapses in rat hippocampal slices. J. Neurosci. 23, 4173–4181. doi: 10.1523/JNEUROSCI.23-10-04173.2003
Lines, J., Martin, E. D., Kofuji, P., Aguilar, J., and Araque, A. (2020). Astrocytes modulate sensory-evoked neuronal network activity. Nat. Commun. 11:3689. doi: 10.1038/s41467-020-17536-3
Lisman, J. (1989). A mechanism for the Hebb and the anti-Hebb processes underlying learning and memory. Proc. Natl. Acad. Sci. U.S.A. 86, 9574–9578. doi: 10.1073/pnas.86.23.9574
Looser, Z. J., Faik, Z., Ravotto, L., Zanker, H. S., Jung, R. B., Werner, H. B., et al. (2024). Oligodendrocyte–axon metabolic coupling is mediated by extracellular K+ and maintains axonal health. Nat. Neurosci. 27, 433–448. doi: 10.1038/s41593-023-01558-3
Lundgaard, I., Luzhynskaya, A., Stockley, J. H., Wang, Z., Evans, K. A., Swire, M., et al. (2013). Neuregulin and BDNF induce a switch to NMDA receptor-dependent myelination by oligodendrocytes. PLoS Biol. 11:e1001743. doi: 10.1371/journal.pbio.1001743
Martínez-Gallego, I., and Rodríguez-Moreno, A. (2024). Adenosine and cortical plasticity. Neuroscientist 18:10738584241236773. doi: 10.1177/10738584241236773
Martínez-Gallego, I., Pérez-Rodríguez, M., Coatl-Cuaya, H., Flores, G., and Rodríguez-Moreno, A. (2022). Adenosine and astrocytes determine the developmental dynamics of spike timing-dependent plasticity in the somatosensory cortex. J. Neurosci. 42, 6038–6052. doi: 10.1523/JNEUROSCI.0115-22.2022
Mederos, S., Sánchez-Puelles, C., Esparza, J., Valero, M., Ponomarenko, A., and Perea, G. (2021). GABAergic signaling to astrocytes in the prefrontal cortex sustains goal-directed behaviors. Nat. Neurosci. 24, 82–92. doi: 10.1038/s41593-020-00752-x
Meeks, J. P., and Mennerick, S. (2004). Selective effects of potassium elevations on glutamate signaling and action potential conduction in hippocampus. J. Neurosci. 24, 197–206. doi: 10.1523/JNEUROSCI.4845-03.2004
Micu, I., Plemel, J. R., Lachance, C., Proft, J., Jansen, A. J., Cummins, K., et al. (2016). The molecular physiology of the axo-myelinic synapse. Exp. Neurol. 276, 41–50. doi: 10.1016/j.expneurol.2015.10.006
Min, R., and Nevian, T. (2012). Astrocyte signaling controls spike timing–dependent depression at neocortical synapses. Nat. Neurosci. 15, 746–753. doi: 10.1038/nn.3075
Morquette, P., Verdier, D., Kadala, A., Féthière, J., Philippe, A. G., Robitaille, R., et al. (2015). An astrocyte-dependent mechanism for neuronal rhythmogenesis. Nat. Neurosci. 18, 844–854. doi: 10.1038/nn.4013
Nagy, J. I., Ionescu, A. V., Lynn, B. D., and Rash, J. E. (2003). Connexin29 and connexin32 at oligodendrocyte and astrocyte gap junctions and in myelin of the mouse central nervous system. J. Comp. Neurol. 464, 356–370. doi: 10.1002/cne.10797
Nevian, T., and Sakmann, B. (2006). Spine Ca2+ signaling in spike-timing-dependent plasticity. J. Neurosci. 26, 11001–11013. doi: 10.1523/JNEUROSCI.1749-06.2006
Nishiyama, H., Knöpfel, T., Endo, S., and Itohara, S. (2002). Glial protein S100B modulates long-term neuronal synaptic plasticity. Proc. Natl. Acad. Sci.m U.S.A. 99, 4037–4042. doi: 10.1073/pnas.052020999
Oe, Y., Wang, X., Patriarchi, T., Konno, A., Ozawa, K., Yahagi, K., et al. (2020). Distinct temporal integration of noradrenaline signaling by astrocytic second messengers during vigilance. Nat. Commun. 11:471. doi: 10.1038/s41467-020-14378-x
Orthmann-Murphy, J. L., Freidin, M., Fischer, E., Scherer, S. S., and Abrams, C. K. (2007). Two distinct heterotypic channels mediate gap junction coupling between astrocyte and oligodendrocyte connexins. J. Neurosci. 27, 13949–13957. doi: 10.1523/JNEUROSCI.3395-07.2007
Pacholko, A. G., Wotton, C. A., and Bekar, L. K. (2020). Astrocytes—the ultimate effectors of long-range neuromodulatory networks? Front. Cell. Neurosci. 14:581075. doi: 10.3389/fncel.2020.581075
Pasti, L., Volterra, A., Pozzan, T., and Carmignoto, G. (1997). Intracellular calcium oscillations in astrocytes: A highly plastic, bidirectional form of communication between neurons and astrocytes in situ. J. Neurosci. 17, 7817–7830. doi: 10.1523/JNEUROSCI.17-20-07817.1997
Paukert, M., Agarwal, A., Cha, J., Doze, V. A., Kang, J. U., and Bergles, D. E. (2014). Norepinephrine controls astroglial responsiveness to local circuit activity. Neuron 82, 1263–1270. doi: 10.1016/j.neuron.2014.04.038
Perea, G., Gómez, R., Mederos, S., Covelo, A., Ballesteros, J. J., Schlosser, L., et al. (2016). Activity-dependent switch of GABAergic inhibition into glutamatergic excitation in astrocyte-neuron networks. Elife 5:e20362. doi: 10.7554/eLife.20362
Perez-Catalan, N. A., Doe, C. Q., and Ackerman, S. D. (2021). The role of astrocyte-mediated plasticity in neural circuit development and function. Neural Dev. 16:1. doi: 10.1186/s13064-020-00151-9
Pérez-Rodríguez, M., Arroyo-García, L. E., Prius-Mengual, J., Andrade-Talavera, Y., Armengol, J. A., Pérez-Villegas, E. M., et al. (2019). Adenosine receptor-mediated developmental loss of spike timing-dependent depression in the hippocampus. Cereb. Cortex 29, 3266–3281. doi: 10.1093/cercor/bhy194
Petersen, A. V., Cotel, F., and Perrier, J.-F. (2017). Plasticity of the axon initial segment: Fast and slow processes with multiple functional roles. Neuroscientist 23, 364–373. doi: 10.1177/1073858416648311
Phatnani, H., and Maniatis, T. (2015). Astrocytes in neurodegenerative disease. Cold Spring Harb. Perspect. Biol. 7:a020628. doi: 10.1101/cshperspect.a020628
Pittolo, S., Yokoyama, S., Willoughby, D. D., Taylor, C. R., Reitman, M. E., Tse, V., et al. (2022). Dopamine activates astrocytes in prefrontal cortex via α1-adrenergic receptors. Cell Rep. 40:111426. doi: 10.1016/j.celrep.2022.111426
Pustylnyak, V. O., Lisachev, P. D., Shtark, M. B., and Epstein, O. I. (2011). Regulation of S100B gene in rat hippocampal CA1 area during long term potentiation. Brain Res. 1394, 33–39. doi: 10.1016/j.brainres.2011.04.025
Ramakers, G. M. J., and Storm, J. F. (2002). A postsynaptic transient K(+) current modulated by arachidonic acid regulates synaptic integration and threshold for LTP induction in hippocampal pyramidal cells. Proc. Natl. Acad. Sci. U.S.A. 99, 10144–10149. doi: 10.1073/pnas.152620399
Rebola, N., Carta, M., Lanore, F., Blanchet, C., and Mulle, C. (2011). NMDA receptor–dependent metaplasticity at hippocampal mossy fiber synapses. Nat. Neurosci. 14, 691–693. doi: 10.1038/nn.2809
Ryczko, D., Hanini-Daoud, M., Condamine, S., Bréant, B. J. B., Fougère, M., Araya, R., et al. (2021). S100β-mediated astroglial control of firing and input processing in layer 5 pyramidal neurons of the mouse visual cortex. J. Physiol. 599, 677–707. doi: 10.1113/JP280501
Sakatani, S., Seto-Ohshima, A., Itohara, S., and Hirase, H. (2007). Impact of S100B on local field potential patterns in anesthetized and kainic acid-induced seizure conditions in vivo. Eur. J. Neurosci. 25, 1144–1154. doi: 10.1111/j.1460-9568.2007.05337.x
Sakatani, S., Seto-Ohshima, A., Shinohara, Y., Yamamoto, Y., Yamamoto, H., Itohara, S., et al. (2008). Neural-activity-dependent release of S100B from astrocytes enhances kainate-induced gamma oscillations in vivo. J. Neurosci. 28, 10928–10936. doi: 10.1523/JNEUROSCI.3693-08.2008
Sardinha, V. M., Guerra-Gomes, S., Caetano, I., Tavares, G., Martins, M., Reis, J. S., et al. (2017). Astrocytic signaling supports hippocampal–prefrontal theta synchronization and cognitive function. Glia 65, 1944–1960. doi: 10.1002/glia.23205
Sasaki, T., Matsuki, N., and Ikegaya, Y. (2011). Action-potential modulation during axonal conduction. Science 331, 599–601. doi: 10.1126/science.1197598
Savtchouk, I., Carriero, G., and Volterra, A. (2018). Studying axon-astrocyte functional interactions by 3d two-photon Ca2+ imaging: A practical guide to experiments and “big data” analysis. Front. Cell. Neurosci. 12:98. doi: 10.3389/fncel.2018.00098
Seol, G. H., Ziburkus, J., Huang, S., Song, L., Kim, I. T., Takamiya, K., et al. (2007). neuromodulators control the polarity of spike-timing-dependent synaptic plasticity. Neuron 55, 919–929. doi: 10.1016/j.neuron.2007.08.013
Shen, W., Flajolet, M., Greengard, P., and Surmeier, D. J. (2008). Dichotomous dopaminergic control of striatal synaptic plasticity. Science 321, 848–851. doi: 10.1126/science.1160575
Sims, T. J., Waxman, S. G., Black, J. A., and Gilmore, S. A. (1985). Perinodal astrocytic processes at nodes of ranvier in developing normal and glial cell deficient rat spinal cord. Brain Res. 337, 321–331. doi: 10.1016/0006-8993(85)90069-1
Sjöström, P. J. (2021). Grand challenge at the Frontiers of synaptic neuroscience. Front. Synap. Neurosci. 13:748937. doi: 10.3389/fnsyn.2021.748937
Slezak, M., Kandler, S., Veldhoven, P. P. V., Haute, C. V., den, Bonin, V., et al. (2019). Distinct mechanisms for visual and motor-related astrocyte responses in mouse visual cortex. Curr. Biol. 29:3120. doi: 10.1016/j.cub.2019.07.078
Somogyu, P., and Hámori, J. (1976). A quantitative electron microscopic study of the Purkinje cell axon initial segment. Neuroscience 1:361. doi: 10.1016/0306-4522(76)90127-5
Stellwagen, D., and Malenka, R. C. (2006). Synaptic scaling mediated by glial TNF-α. Nature 440, 1054–1059. doi: 10.1038/nature04671
Stephan, J., Eitelmann, S., and Zhou, M. (2021). Approaches to study gap junctional coupling. Front. Cell. Neurosci. 15:640406. doi: 10.3389/fncel.2021.640406
Stone, J., Makarov, F., and Holländer, H. (1995). The glial ensheathment of the soma and axon hillock of retinal ganglion cells. Vis. Neurosci. 12, 273–279. doi: 10.1017/S0952523800007951
Stuart, G., and Sakmann, B. (1995). Amplification of EPSPs by axosomatic sodium channels in neocortical pyramidal neurons. Neuron 15, 1065–1076. doi: 10.1016/0896-6273(95)90095-0
Susuki, K., and Kuba, H. (2016). Activity-dependent regulation of excitable axonal domains. J. Physiol. Sci. 66, 99–104. doi: 10.1007/s12576-015-0413-4
Tan, Z., Liu, Y., Xi, W., Lou, H., Zhu, L., Guo, Z., et al. (2017). Glia-derived ATP inversely regulates excitability of pyramidal and CCK-positive neurons. Nat. Commun. 8:13772. doi: 10.1038/ncomms13772
Tazerart, S., Mitchell, D. E., Miranda-Rottmann, S., and Araya, R. (2020). A spike-timing-dependent plasticity rule for dendritic spines. Nat. Commun. 11:4276. doi: 10.1038/s41467-020-17861-7
Turrigiano, G. (2007). Homeostatic signaling: The positive side of negative feedback. Curr. Opin. Neurobiol. 17, 318–324. doi: 10.1016/j.conb.2007.04.004
Valtcheva, S., and Venance, L. (2016). Astrocytes gate Hebbian synaptic plasticity in the striatum. Nat. Commun. 7:13845. doi: 10.1038/ncomms13845
Walch, E., Bilas, A., Bebawy, V., Lam, A., Murphy, T. R., Sriram, S., et al. (2022). Contributions of astrocyte and neuronal volume to CA1 neuron excitability changes in elevated extracellular potassium. Front. Cell. Neurosci. 16:930384. doi: 10.3389/fncel.2022.930384
Wang, F., Smith, N. A., Xu, Q., Fujita, T., Baba, A., Matsuda, T., et al. (2012). Astrocytes modulate neural network activity by Ca2+-dependent uptake of extracellular K+. Sci. Signal. 5:ra26. doi: 10.1126/scisignal.2002334
Wang, Y., Fu, W.-Y., Cheung, K., Hung, K.-W., Chen, C., Geng, H., et al. (2021). Astrocyte-secreted IL-33 mediates homeostatic synaptic plasticity in the adult hippocampus. Proc. Natl. Acad. Sci. U.S.A. 118:e2020810118. doi: 10.1073/pnas.2020810118
Watanabe, S., Hoffman, D. A., Migliore, M., and Johnston, D. (2002). Dendritic K+ channels contribute to spike-timing dependent long-term potentiation in hippocampal pyramidal neurons. Proc. Natl. Acad. Sci. U.S.A. 99, 8366–8371. doi: 10.1073/pnas.122210599
Waxman, S. G., and Black, J. A. (1984). Freeze-fracture ultrastructure of the perinodal astrocyte and associated glial junctions. Brain Res. 308, 77–87. doi: 10.1016/0006-8993(84)90919-3
Waxman, S. G., and Swadlow, H. A. (1976). Ultrastructure of visual callosal axons in the rabbit. Exp. Neurol. 53, 115–127. doi: 10.1016/0014-4886(76)90287-9
Wotton, C. A., Cross, CD., and Bekar, L. K. (2020). Serotonin, norepinephrine, and acetylcholine differentially affect astrocytic potassium clearance to modulate somatosensory signaling in male mice. J. Neurosci. Res. 98, 964–977. doi: 10.1002/jnr.24597
Yamada, R., and Kuba, H. (2016). Structural and functional plasticity at the axon initial segment. Front. Cell. Neurosci. 10:250. doi: 10.3389/fncel.2016.00250
Yamazaki, Y., Hozumi, Y., Kaneko, K., Fujii, S., Goto, K., and Kato, H. (2010). Oligodendrocytes: Facilitating axonal conduction by more than myelination. Neuroscientist 16, 11–18. doi: 10.1177/1073858409334425
Yang, Y., Ge, W., Chen, Y., Zhang, Z., Shen, W., Wu, C., et al. (2003). Contribution of astrocytes to hippocampal long-term potentiation through release of d-serine. Proc. Natl. Acad. Sci.m U.S.A. 100, 15194–15199. doi: 10.1073/pnas.2431073100
Yuan, L.-L., Adams, J. P., Swank, M., Sweatt, J. D., and Johnston, D. (2002). Protein kinase modulation of dendritic K+ channels in hippocampus involves a mitogen-activated protein kinase pathway. J. Neurosci. 22, 4860–4868. doi: 10.1523/JNEUROSCI.22-12-04860.2002
Zhang, J.-C., Lau, P.-M., and Bi, G.-Q. (2009). Gain in sensitivity and loss in temporal contrast of STDP by dopaminergic modulation at hippocampal synapses. Proc. Natl. Acad. Sci. U.S.A. 106, 13028–13033. doi: 10.1073/pnas.0900546106
Keywords: astrocytes, synaptic plasticity, STDP, neuronal excitability, axonal plasticity
Citation: Sanz-Gálvez R, Falardeau D, Kolta A and Inglebert Y (2024) The role of astrocytes from synaptic to non-synaptic plasticity. Front. Cell. Neurosci. 18:1477985. doi: 10.3389/fncel.2024.1477985
Received: 08 August 2024; Accepted: 02 October 2024;
Published: 18 October 2024.
Edited by:
Fabiola M. Ribeiro, Federal University of Minas Gerais, BrazilReviewed by:
Juliana Alves Silva, Federal University of Minas Gerais, BrazilPablo Leal Cardozo, Federal University of Minas Gerais, Brazil
Copyright © 2024 Sanz-Gálvez, Falardeau, Kolta and Inglebert. This is an open-access article distributed under the terms of the Creative Commons Attribution License (CC BY). The use, distribution or reproduction in other forums is permitted, provided the original author(s) and the copyright owner(s) are credited and that the original publication in this journal is cited, in accordance with accepted academic practice. No use, distribution or reproduction is permitted which does not comply with these terms.
*Correspondence: Yanis Inglebert, eWFuaXMuaW5nbGViZXJ0QHVtb250cmVhbC5jYQ==