- 1Graduate Biomedical Sciences Program, School of Medicine, University of Alabama at Birmingham, Tuskegee, AL, United States
- 2Department of Psychiatry, School of Medicine, University of Alabama at Birmingham, Tuskegee, AL, United States
- 3Comprehensive Neuroscience Center, University of Alabama at Birmingham, Tuskegee, AL, United States
- 4Department of Psychology and Sociology, College of Arts and Sciences, Tuskegee University, Tuskegee, AL, United States
The microcircuitry within superficial layers of the dorsolateral prefrontal cortex (DLPFC), composed of excitatory pyramidal neurons and inhibitory GABAergic interneurons, has been suggested as the neural substrate of working memory performance. In schizophrenia, working memory impairments are thought to result from alterations of microcircuitry within the DLPFC. GABAergic interneurons, in particular, are crucially involved in synchronizing neural activity at gamma frequency, the power of which increases with working memory load. Alterations of GABAergic interneurons, particularly parvalbumin (PV) and somatostatin (SST) subtypes, are frequently observed in schizophrenia. Abnormalities of GABAergic neurotransmission, such as deficiencies in the 67 kDA isoform of GABA synthesis enzyme (GAD67), vesicular GABA transporter (vGAT), and GABA reuptake transporter 1 (GAT1) in presynaptic boutons, as well as postsynaptic alterations in GABAA receptor subunits further contribute to impaired inhibition. This review explores GABAergic abnormalities of the postmortem DLPFC in schizophrenia, with a focus on the roles of interneuron subtypes involved in cognition, and GABAergic neurotransmission within presynaptic boutons and postsynaptic alterations. Where available, comparisons between schizophrenia and affective disorders that share cognitive pathology such as bipolar disorder and major depressive disorder will be made. Challenges in directly measuring GABA levels are addressed, emphasizing the need for innovative techniques. Understanding GABAergic abnormalities and their implications for neural circuit dysfunction in schizophrenia is crucial for developing targeted therapies.
Introduction to GABA abnormalities in schizophrenia
Schizophrenia is a debilitating and complex neuropsychiatric disorder characterized by an altered perception of reality. The symptoms of schizophrenia have been divided into three subtypes: positive (hallucinations and delusions), negative (lack of emotion and social withdrawal), and cognitive (learning, attention, and memory deficits), with cognitive symptoms being indicative of a more severe prognosis (Green, 1996; Harvey et al., 1998). Cognitive deficits in schizophrenia are hypothesized to be caused by neural circuit dysfunction involving imbalances between excitatory and inhibitory synaptic transmission (Weinberger et al., 1986; Carter et al., 1998; Glahn et al., 2005; Van Snellenberg et al., 2006; Minzenberg et al., 2009; Buffalo et al., 2011; Michalareas et al., 2016; Bastos et al., 2018). Inhibitory interneurons release the inhibitory neurotransmitter GABA, tuning the firing frequency of local pyramidal neurons that release the excitatory neurotransmitter glutamate and, in turn, excite the interneurons to maintain appropriate circuit and network function (Figure 1) (Goldman-Rakic, 1995; Wang et al., 2013; Gonzalez-Burgos et al., 2015; Chen et al., 2017). In schizophrenia, GABAergic interneurons are thought to underlie disruptions in neuronal synchrony contributing to observed cognitive deficits (Gonzalez-Burgos and Lewis, 2008; Lewis and Gonzalez-Burgos, 2008; Jones, 2010; Gandal et al., 2012). Thus, alterations of specific subtypes of GABAergic interneurons contributing to imbalanced neural circuitry are thought to ultimately result in cognitive impairment in schizophrenia (Nguyen et al., 2014).
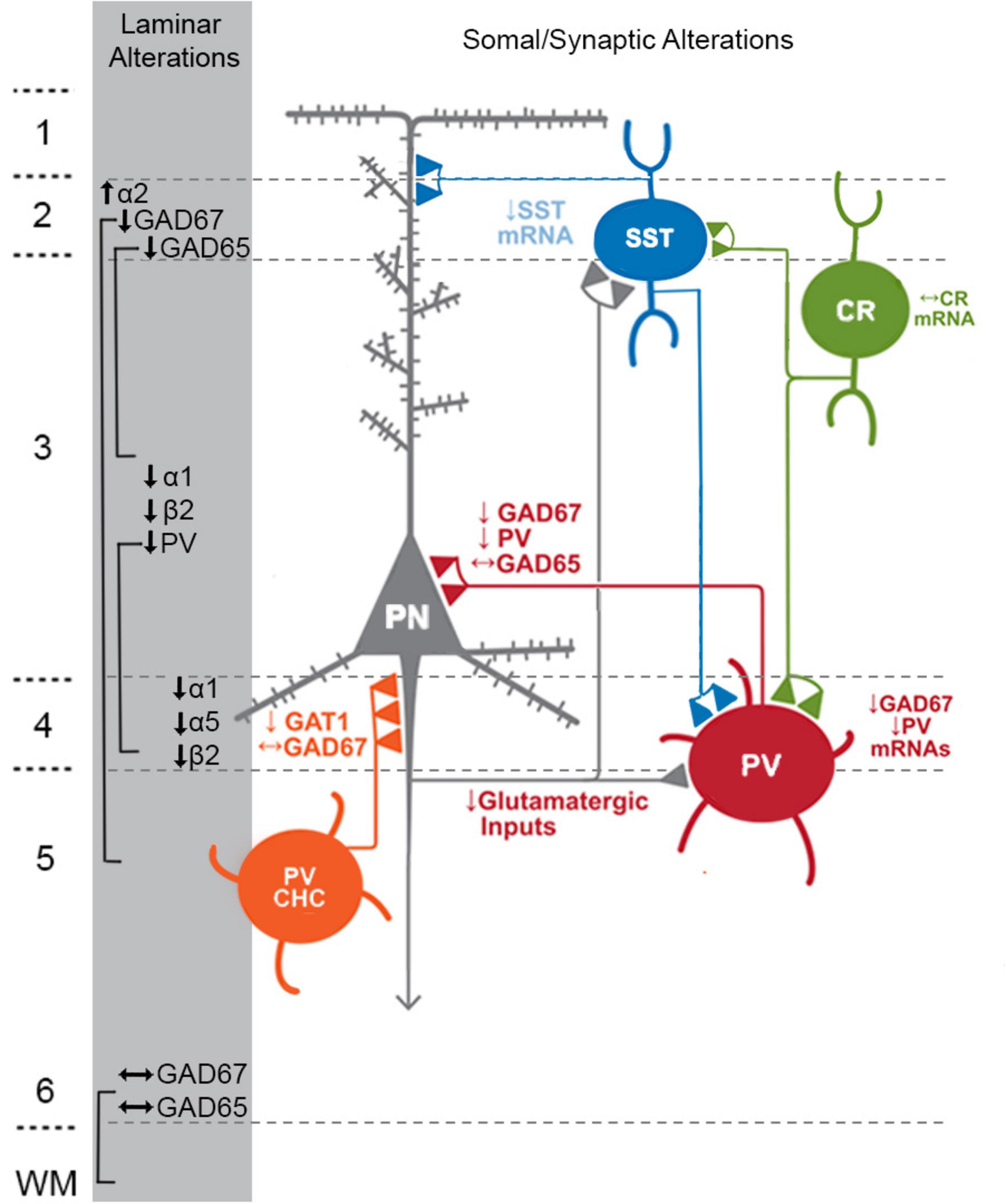
Figure 1. Schematic of components with consistent findings in schizophrenia of the DLPFC microcircuitry thought to be responsible for gamma oscillations and working memory. In the unaffected circuit, gamma oscillations are generated in supragranular layers via a microcircuit formed by connections between layer 3 pyramidal neurons (PNs), somatostatin neurons (SSTs), and parvalbumin-basket cells (PVBCs); SSTs dampen the excitation of L3PNs at their apical dendrites and also project inhibitory connections onto the cell body of PVBCs; the spiking of PVBCs synchronizes the firing of neighboring PNs at gamma frequency. Furthermore, SSTs project inhibitory connections onto PVBCs, regulating their spiking. In addition, the persistent firing of PNs during WM tasks arises, in part, from recurrent excitation among them and is shaped by dendritic inhibition and perisomatic inhibition thought to arise from SSTs and PVBCs, respectively. Calretinin (CR)-expressing interneurons target both PV and SST neurons, serving to disinhibit pyramidal neurons. PV chandelier cells (PV CHC) form connections directly onto the axon initial segment, and thus are poised to rapidly suppress the output of PNs. In schizophrenia, alterations of key transcripts by laminae are shown on the left. Lower PV and GAD67 in the PVBC terminals suggests that both presynaptic inhibition of PVBCs onto PNs is lower. Although the cell type-specific deficits in SST neurons are less well-defined, lower SST mRNA expression suggests these neurons may have an impaired ability to laterally inhibit other populations of PNs. CR cells do not exhibit lower levels of CR mRNA or protein in the illness, suggesting that these cells may not be affected in schizophrenia. PV CHCs express normal levels of GAD67, but lower GAT1 in their cartridges and higher levels of the GABAA receptor α2 subunit on the axon initial segment of the PN. Together, these alterations suggest that GABA signaling from PV CHCs might be higher in schizophrenia.
Thus, it is crucial to investigate how abnormalities of the GABA system contribute to cognitive deficits in schizophrenia. Furthermore, understanding the fundamental mechanisms governing GABAergic synaptic transmission will contribute to the creation of evidence-based interventions for other disorders that exhibit GABAergic dysfunction. Indeed, alterations of GABAergic signaling have also been associated with bipolar disorder (BPD) and major depressive disorder (MDD), leading to speculation that these disorders could exhibit a shared pathology that results in the cognitive impairments present in all three disorders (Barch, 2009; Reichenberg et al., 2009).
Therefore, here we focus on GABAergic abnormalities of the human postmortem dorsolateral prefrontal cortex (DLPFC) believed to be the neural substrate for cognitive and working memory deficits in hopes of providing insight for the development of better comorbid treatments beyond antipsychotics and antidepressants. We highlight the literature outlining DLPFC GABAergic abnormalities in schizophrenia and discuss gaps in the existing literature that preclude our understanding of how these deficits and subsequent circuit dysfunctions manifest.
Oscillation coherence in working memory deficits associated with schizophrenia
Many of the GABAergic neurons (80–90%) in the primate prefrontal cortex (PFC) consist of unique, non-overlapping subtypes based on the expression of one of three calcium-binding proteins—calbindin (CB; ∼20%), calretinin (CR; ∼45%), or parvalbumin (PV; ∼20%); (Conde et al., 1994; Gabbott and Bacon, 1996; del Río and DeFelipe, 1997a; Barinka and Druga, 2010). Additionally, each of the calcium-binding protein interneuron populations often co-express one of four neuropeptides— neuropeptide Y (NPY), vasoactive intestinal polypeptide (VIP), somatostatin (SST), and cholecystokinin (CCK). Indeed, CB-positive cells are often also SST-positive (Ang and Shul, 1995; Gonzalez-Albo et al., 2001; Suarez-Sola et al., 2009; Zaitsev et al., 2009), and CR-positive cells are often VIP-positive (Gabbott and Bacon, 1997). These interneurons contribute heavily to oscillations that synchronize circuit activity in the DLPFC and other brain regions (Dienel and Lewis, 2019). The synchronization of neuronal activity by oscillations is an innate mechanism that occurs to assist in proper cognitive function (Colgin and Moser, 2010) and functional connectivity between brain regions (Popescu et al., 2009).
Neuronal oscillations in the delta (0.5–3.5 Hz), theta (3.5–7 Hz), alpha (8–13 Hz), beta (18–25 Hz), and gamma (30–80 Hz) frequency ranges have distinctive roles in cognitive function. Slow-wave deep sleep and interconnected neuronal network connections at longer ranges are associated with delta oscillations (Harmony, 2013; Hiltunen et al., 2014), while rapid eye movement and shallow sleep as well as memory encoding involving the hippocampus are connected to theta oscillations (Cantero et al., 2003; Zielinski et al., 2020). When the brain is active yet relaxed or at rest, alpha waves are involved (Klimesch, 2012; Xavier et al., 2020), and awakened states, conscious thoughts, logical thinking, and attention are linked to beta oscillations (Espenhahn et al., 2019; Schmidt et al., 2019). These lower frequency oscillations can couple with each other to alter interneuron and pyramidal cell activity in cortical regions leading to changes in cognitive function altered in schizophrenia.
The coupling of oscillations between frequencies is referred to as neuronal coherence and is a fundamental feature of oscillatory activity across brain regions and within neural networks (Varela et al., 2001). Impaired coupling between frequencies where one or more oscillation ranges are altered has been linked to increased schizophrenia risk and susceptibility. For example, cross-frequency coupling between the theta and beta ranges are associated with working memory (WM) (Bygrave et al., 2019), and deficits in this coherence where theta is elevated, while beta is reduced can result in enhanced risk for psychosis associated with schizophrenia (Cousijn et al., 2015). Additionally, impaired alpha/beta coupling has been implicated during the consolidation period of WM in patients with schizophrenia compared with unaffected patients (Erickson et al., 2017). Furthermore, EEG recordings in individuals with schizophrenia that assessed delta/alpha oscillatory coherence have been shown to underlie physiological mechanisms associated with psychosis and could be associated with dysfunction of thalamocortical connectivity (Howells et al., 2018). Finally, several groups have provided evidence of coupling between theta and gamma oscillations, referred to as theta-gamma coupling (TGC), that underlies WM processes associated with schizophrenia (Engel et al., 2001; Canolty and Knight, 2010). GABAergic interneurons, particularly inhibition onto PV-positive ones, play a distinct role in regulating theta-gamma interactions (Wulff et al., 2009). In all, coherence between and within oscillation ranges impacts working memory in a variety of ways, thereby increasing susceptibility to schizophrenia.
Gamma oscillations and GABA neuron physiology
A large portion of research into WM impairments in schizophrenia, especially work completed in human postmortem tissue, has focused on the neural substrate of high-frequency gamma oscillations (30–80 Hz), as these oscillations are important for higher brain processes such as learning, memory, and cognition (Andersson et al., 2012). For gamma oscillations to generate, pyramidal neurons fire asynchronously leading to depolarization of GABAergic interneurons which ultimately causes feedback inhibition of connected principal cells (Dienel and Lewis, 2019). This cycle of asynchronous firing and feedback inhibition results in coordinated firing of pyramidal cells at a frequency within the gamma range (Constantinidis et al., 2002; Gonzalez-Burgos et al., 2015; Bastos et al., 2018). The power of gamma oscillations within the DLPFC are lower in individuals with schizophrenia (Uhlhaas and Singer, 2010; Dienel and Lewis, 2019), and fail to increase in power during a WM task (Cho et al., 2006), in contrast to that observed in unaffected comparison individuals (UCs).
Abnormalities of the GABAergic interneurons and excitatory pyramidal neurons within the superficial layers of the DLPFC are believed to be the neural substrate of altered gamma power and WM impairments in schizophrenia (Lewis et al., 1999; Lewis and Moghaddam, 2006; Lewis and Hashimoto, 2007), as well as other comorbid psychiatric disorders like BPD (Benes and Berretta, 2001; Knable et al., 2004; Benes et al., 2008) and MDD (Luscher et al., 2011). Many studies of schizophrenia have been dedicated to abnormalities of the GABAergic system (Uehara et al., 2015), with a focus on the specific alterations of the genetic expression and function of enzymes and proteins expressed in GABAergic interneurons and involved in inhibitory synaptic transmission (Howes and Shatalina, 2022). Indeed, schizophrenia genome-wide association studies (GWAS) have identified the gene SLC32A1, which encodes the vesicular GABA transporter (vGAT) and is involved in controlling the vesicular uptake of GABA (Ripke et al., 2011; Ikeda et al., 2019; Howes and Shatalina, 2022). Therefore, postmortem reports of alterations in GABAergic neurotransmission are of key importance to understanding schizophrenia pathology, and may also contribute to the shared pathology within affective disorders such as BPD and MDD.
Alterations of parvalbumin interneurons in schizophrenia DLPFC
PV interneurons are predominantly localized to layers 3–4 within the DLPFC (Chung et al., 2016b; Dienel et al., 2021), and compose approximately 25% of all cortical interneurons (Conde et al., 1994). PV cells can be divided into two different subtypes, basket cells (PVBCs) and chandelier cells (ChCs). PVBCs form synapses onto the somal bodies, proximal dendritic shafts, and spines of local layer 3 pyramidal neurons (Williams et al., 1992; Melchitzky et al., 1999; Zaitsev et al., 2009), whereas ChCs target the axon initial segment of pyramidal neurons (Somogyi, 1977; Lewis and Lund, 1990; Williams et al., 1992; Lund and Lewis, 1993; Melchitzky and Lewis, 2003). Although the unique populations of PV interneurons can be readily identified, methodological and technical limitations still exist that prohibit a clear distinction between PVBCs and ChCs. Therefore, for the purpose of this review, we will not distinguish between the PV interneuron subtypes unless otherwise specified.
Alterations of PV interneurons in the DLPFC of individuals with schizophrenia have been consistently reported (Hashimoto et al., 2003; Fung et al., 2010; Chung et al., 2016b; Volk et al., 2016; Enwright et al., 2018; Tsubomoto et al., 2019). Lower levels of DLPFC PV mRNA have been observed in total gray matter homogenates, layers 3–4, and within individual layer 4 PV neurons (Hashimoto et al., 2003; Dienel et al., 2023b). Such deficits in DLPFC PV mRNA translate to reductions in PV protein levels in cell bodies (Chung et al., 2016a) and axon terminals localized within the DLPFC (Glausier et al., 2014), without a reduction of PV-positive neurons (Woo et al., 1997; Hashimoto et al., 2003; Tooney and Chahl, 2004; Chung et al., 2016a; Enwright et al., 2016). At the level of the synapse, DLPFC PV-positive axon terminals exhibit lower levels of GAD67 (Curley et al., 2011) and PV protein levels (Glausier et al., 2014).
However, these alterations may be specific to PV interneuron subtype. Indeed, GAD67 protein expression is unaltered in the axon cartridges of ChCs within the prefrontal cortex (Rocco et al., 2016a). Although ChC cartridges do not appear to express alterations in GABA synthesis, the density of ChC cell cartridges identified by GABA reuptake transporter 1 (GAT1) mRNA has been reported to be lower in DLPFC layers 2–4 in individuals with schizophrenia (Woo et al., 1998; Pierri et al., 1999), although it is uncertain if these findings reflect a methodological limitation of detectability. Indeed, the density of cartridges identified by vGAT is unaltered in the illness (Rocco et al., 2017).
Fewer studies have been conducted examining PV interneurons within the PFC of individuals diagnosed with BPD or MDD. In total gray matter homogenate, Chung et al. (2016b) reported significantly lower levels of DLPFC PV mRNA in both BPD and MDD subjects compared to UC subjects, which were also simultaneously significantly higher than those diagnosed with schizophrenia. In contrast, utilizing whole tissue homogenate of the DLPFC, Sibille et al. (2011) observed a deficit only in those diagnosed with BPD. Although such differences could be attributed to cohort or tissue sampling differences, further testing is necessary to determine if distinct alterations can be differentially attributed to specific mood disorders.
Lack of schizophrenia-associated calretinin interneuron alterations
In primate DLPFC, CR interneurons represent approximately 50% of all DLPFC GABA neurons (Conde et al., 1994). Although the somata and dendritic and axonal processes are found in all cortical layers, the cell bodies of this interneuron subtype are most prevalent in layers 1–superficial 3 (Conde et al., 1994; Daviss and Lewis, 1995), and these observations are supported by findings of significantly higher levels of CR mRNA in layer 2 in comparison to layer 4 (Chung et al., 2016b). However, multiple types of CR-positive cells are present within each layer (Gabbott et al., 1997). Indeed, bipolar CR-positive cells within human PFC often co-express VIP (Gabbott and Bacon, 1997). Despite the high proportion of CR neurons to all GABAergic interneuron subtypes (Conde et al., 1994), CR axonal boutons comprise only about 16% of all GABAergic boutons in the DLPFC, but are present in all cortical layers (Rocco et al., 2016b). These boutons primarily target dendrites of PV (Donato et al., 2013; Pi et al., 2013) and SST neurons (Pfeffer et al., 2013; Pi et al., 2013), contributing to the spatial tuning of local microcircuits.
In schizophrenia PFC, no alterations of CR mRNA or protein have been observed (Hashimoto et al., 2003; Hashimoto et al., 2008; Chung et al., 2016b). However, these alterations may vary by cohort, brain region, or cortical layer: Volk et al. (2012) observed a cohort subset effect of a 9% upregulation in DLPFC of individuals with schizophrenia that did not exhibit significant downregulations in other inhibitory markers. Furthermore, Tsubomoto et al. (2019) observed a significant 41% upregulation of CR mRNA in primary visual cortex (V1) but not DLPFC, although the cohort was not subclustered in the same manner as Volk et al. (2012). Irrespective of CR mRNA or protein levels, studies of CR-immunoreactive somata have found no difference in CR cell density and soma size across all layers of the DLPFC (Daviss and Lewis, 1995; Reynolds and Beasley, 2001; Reynolds et al., 2001; Beasley et al., 2002; Reynolds et al., 2002; Hashimoto et al., 2003; Tooney and Chahl, 2004; Oh et al., 2012), suggesting that the identification of CR cells may not be affected by methodological limitations of detection thresholds like other interneuron types (e.g., SST cells, see below).
Even with the growing abundance of studies focused on CR cells in schizophrenia, there are limited and variable investigations of CR cells within postmortem cortical regions in individuals with mood disorders, such as BPD or MDD. A study of CR interneuronal density within the anterior cingulate cortex revealed no alterations in BPD subjects (Cotter et al., 2002), but a later study examining the density of CR neurons within layer 2 of the DLPFC of individuals with BPD revealed a higher density of large CR-immunoreactive cells (Sakai et al., 2008). Reports of CR interneuron density in MDD DLPFC have been varied (Beasley et al., 2002; Reynolds et al., 2002; Oh et al., 2012), but suggest a CR neuronal density deficit specifically in DLPFC layer 1 in MDD (Oh et al., 2012). However, a separate investigation of postmortem auditory cortex revealed a lower density of CR-positive cells without respect to cortical layer in individuals with MDD (Smiley et al., 2016). Thus, perhaps as in schizophrenia, CR alterations (or lack thereof) may differ by cortical region and layer in BPD and MDD.
Consistent and robust deficiencies of somatostatin neurons in schizophrenia
In the primate DLPFC, SST neurons account for approximately 30% of all interneurons (Rudy et al., 2011) and are found in the highest density within layer 2 (Lewis et al., 1986; Gabbott and Bacon, 1996; Dienel et al., 2021). SST interneurons can be further divided into unique subtypes. Those located within the superficial layers of the cortex often co-express calcium-binding protein CB (Gonzalez-Albo et al., 2001; Zaitsev et al., 2009) and primarily target the distal dendrites of local pyramidal neurons (Melchitzky and Lewis, 2008), temporally tuning the oscillations associated with WM capability (Abbas et al., 2018; Torres-Gomez et al., 2020; Froudist-Walsh et al., 2021). However, those within deep layer 6 and the superficial white matter typically lack CB mRNA (Ang and Shul, 1995; Suarez-Sola et al., 2009) and form long-range axonal projections (Tomioka and Rockland, 2007).
Due to their role in WM capability, SST neurons within superficial layers but not the deep layers of the cortex have been predominantly studied in schizophrenia. Therefore, here we focus on the findings of SST interneurons within cortical layers 1–3.
Downregulation of SST mRNA is perhaps the most highly replicated finding in schizophrenia. Indeed, lower levels of SST mRNA have been observed across multiple methodologies, subject cohorts, cortical regions, and laminae (Melchitzky and Lewis, 2008; Morris et al., 2008; Fung et al., 2010; Tsubomoto et al., 2019; Dienel et al., 2023a; Dienel et al., 2023b). Although a reduction of SST-positive interneuron density in schizophrenia has been previously reported (Morris et al., 2008), recent work illustrates that such findings were a result of a limited detection threshold of the methodologies available at the time; newer approaches with a higher level of detection sensitivity indicate a significant downregulation of SST mRNA per neuron without a loss of SST interneuron density in the illness (Dienel et al., 2023b).
In contrast to the consistency of findings in schizophrenia, findings of PFC SST levels in BPD or MDD differ substantially across studies. Individuals with MDD, but not BPD, exhibit lower SST mRNA levels (Sibille et al., 2011; Dienel et al., 2023a), but this data is contrasted by the work of Fung et al. (2014) who reported robust deficits of SST mRNA in BPD. However, a singular report investigating SST mRNA in deep layer 6/superficial white matter found no alterations within MDD or BPD (Dienel et al., 2023a), suggesting that there may be differential effects of both disease and cortical layer.
Inconsistent findings of calbindin-positive cells
Although CB and SST are often co-expressed within the superficial layers of the DLPFC (Gonzalez-Albo et al., 2001; Zaitsev et al., 2009), given the historical difficulty of identifying SST interneurons in schizophrenia (Dienel et al., 2023b), CB has often been used as an independent interneuron marker. However, not all SST-positive neurons are CB-positive (Ang and Shul, 1995; Suarez-Sola et al., 2009), meaning that CB-only investigations likely assess a population different than those identified solely with SST. Therefore, we thought it apt to include studies investigating CB-identified interneurons in a separate section. Indeed, findings of CB-positive interneurons in schizophrenia cortex differ from those of SST findings. In studies of CB-positive interneurons in schizophrenia, mRNA expression of CB within the DLPFC of individuals with schizophrenia was higher than that of matched comparison subjects (Fung et al., 2010). Assessments of CB density in schizophrenia have been varied. Three reports of CB density identified by immunohistochemistry within the DLPFC of individuals with schizophrenia have reported a higher number of neurons within layers 3 and 5/6 (Daviss and Lewis, 1995), unaltered density of CB neurons within any layer (Tooney and Chahl, 2004), and fewer medium-size CB neurons within layer 2 (Sakai et al., 2008). Investigations of other regions and/or disorders have not added clarity. The density of CB interneurons of any size identified by in situ hybridization in the anterior cingulate cortex did not differ in schizophrenia or BPD (Woo et al., 2008), whereas the density of large-size CB neurons within DLPFC layer 2 was higher in BPD (Sakai et al., 2008) but lower in MDD DLPFC (Rajkowska et al., 2007). If the inconsistency of the aforementioned results cannot be attributed to methodology or cohort, it may suggest unique subpopulations of CB cells by region, layer, and neuronal size.
Limited but consistent findings of vasoactive intestinal polypeptide neurons in schizophrenia
Approximately 80% of CR neurons also co-express VIP, and roughly 80% of VIP neurons contain CR (Gabbott and Bacon, 1997). VIP interneurons predominantly target the dendrites of PV and SST neurons (del Río and DeFelipe, 1997b; Rajkowska et al., 2007; Melchitzky and Lewis, 2008; Donato et al., 2013; Pfeffer et al., 2013; Pi et al., 2013), but also synapse onto the dendrites and pyramidal cell somata. By providing inhibition onto inhibitory PV and SST neurons, VIP interneurons mediate disinhibitory control of local pyramidal neurons, resulting in an upregulation of local excitatory activity (Pi et al., 2013). Therefore, VIP neurons play a crucial role in modulating the microcircuitry associated with WM capability. Despite their importance, VIP neurons have been infrequently studied in the postmortem PFC of schizophrenia subjects. However, findings of this neuronal type have been consistent in the illness, with reports of lower PFC VIP mRNA and protein (Gabriel et al., 1996; Fung et al., 2010; Fung et al., 2014). Furthermore, Gabriel et al. (1996) reported less VIP protein expression across multiple cortical regions involved in the visuospatial WM network. Lastly, these findings appear to be consistent with the singular study that has assessed VIP protein in BPD PFC (Fung et al., 2014), suggesting a potentially shared VIP neuron pathology between these two types of serious mental illness, but further work is needed.
Alterations of neuropeptide Y in cortical gray and white matter
NPY is often co-expressed alongside one of the calcium-binding proteins, and thus can be utilized to identify a particular subset of cortical interneuron. NPY-expressing cells are primarily localized in cortical layers 2/3 and 5/6 (Kuljis and Rakic, 1989). Relatively few, and virtually none, of these cells can be observed in layer 1 and layer 4, respectively (Kuljis and Rakic, 1989). However, NPY-positive cells are highly prevalent within the superficial white matter (Kuljis and Rakic, 1989). In schizophrenia, investigations of NPY expression within postmortem PFC have been varied. Indeed, many reports indicate lower mRNA and protein expression of this neuropeptide within PFC gray matter (Gabriel et al., 1996; Kuromitsu et al., 2001; Hashimoto et al., 2008; Mellios et al., 2009; Fung et al., 2010), although others report no change (Caberlotto and Hurd, 1999; Morris et al., 2009).
Investigations of NPY interneurons within superficial white matter have been limited, but report lower NPY mRNA within the DLPFC of individuals with schizoaffective disorder, but not a separate cohort of subjects with schizophrenia (Morris et al., 2009), suggesting NPY cells might be more perturbed in affective disorders. Indeed, the relatively few studies that have investigated this interneuron type in BPD or MDD PFC have reported lower levels of NPY mRNA expression in BPD, but not MDD (Caberlotto and Hurd, 1999; Kuromitsu et al., 2001), suggesting even further distinction of the NPY interneuron subtype within mood disorder pathology.
Differential expression of cholecystokinin interneurons in schizophrenia prefrontal cortex
In primate neocortex, CCK-positive interneuron somata are predominately observed in layers 2-superficial 3, whereas their axon terminals primarily innervate laminae 2, 4, and 6 (Oeth and Lewis, 1993; Eggan et al., 2010). These neurons, like PVBCs, target the perisomatic region of local pyramidal neurons and tune their firing rate. However, unlike PVBCs, CCK interneurons are suspected to tune cellular firing at a theta, not gamma, frequency (Traub et al., 1996; Klausberger et al., 2005; Klausberger and Somogyi, 2008), and therefore may be less directly involved in WM capability. Although a subpopulation of CCK-positive cells can be defined by its co-expression of other proteins such as cannabinoid 1 receptor (Eggan et al., 2010), for the purpose of this review we have limited discussion to studies using CCK as its primary identifying marker.
In schizophrenia PFC, lower levels of CCK transcript have been observed (Hashimoto et al., 2008; Fung et al., 2010). Although localized to the same laminae as findings of GAD67 deficits in schizophrenia, assessment of GAD67 within CCK cells in schizophrenia has yet to be conducted. However, investigation of GAD67 and GAD65 within cortical CCK-positive axon terminals indicates that CCK-positive cells may predominantly express GAD65 instead of GAD67 (Fish et al., 2011), and therefore may not contribute to the GAD67 deficit observed in the disease.
Investigations of CCK in BPD or MDD have been extremely limited. Indeed, zero studies were identified in BPD, and only two were identified with potential implications for MDD (Perry et al., 1981; Barde et al., 2024). However, the most recent study conducted by Barde et al. (2024) investigated MDD subjects who died by suicide, and reported elevated activity of the CCK systems in PFC, although these findings may be unique to those who completed suicide (Yin et al., 2016; Dowling et al., 2023).
GABA in presynaptic boutons in schizophrenia prefrontal cortex
Although difficult to assess directly in human postmortem tissue, measuring levels of mRNA transcripts and their cognate proteins that regulate GABA in presynaptic terminals can serve as an adequate proxy of the strength of GABAergic neurotransmission (Schoonover et al., 2020). Indeed, synaptic GABA is synthesized by the 67 and 65 kDA isoforms of the enzyme glutamic acid decarboxylase, known as GAD67 and GAD65, respectively. However, GAD67 is the enzyme predominantly responsible for the GABA synthesis load (Asada et al., 1997), whereas GAD65 appears to be active only during periods of high neuronal activity (Patel et al., 2006). In response to homeostatic signals of excessive network activity (Erickson et al., 2006; Hartmann et al., 2008), the strength of GABAergic neurotransmission can be upregulated by the increased accumulation of GABA into synaptic vesicles, mediated by vGAT. Lastly, the clearance of GABA from the synaptic cleft is largely regulated by GAT1, responsible for GABA neurotransmitter reuptake at the presynaptic terminal. Furthermore, elevation of synaptic GABA reuptake via GAT1 regulates inhibitory postsynaptic potentials of perisomatic GABA inhibition and prevents GABA spillover at both perisomatic and dendritic-targeting GABA inputs (Gonzalez-Burgos et al., 2009). Together, levels of these three presynaptic markers of GABA neurotransmission in the postmortem brain can provide insight into the integrity of GABAergic signaling in schizophrenia.
In schizophrenia, studies reporting prefrontal GAD67 deficits across multiple methodologies, anatomical levels of resolution, and cell types have been reported (Akbarian et al., 1995; Mirnics et al., 2000; Volk et al., 2000; Vawter et al., 2002; Hashimoto et al., 2005; Straub et al., 2007; Hashimoto et al., 2008; Duncan et al., 2010; Curley et al., 2011; Kimoto et al., 2014; Tao et al., 2018; Lanz et al., 2019; Enwright and Lewis, 2021). For example, two in situ hybridization studies revealed a GAD67 mRNA deficit across PFC layers 2–5, with pronounced deficits in layers 3–4 (Akbarian et al., 1995; Volk et al., 2000), and GAD67 protein levels measured by Western blot and immunofluorescence were also reported to be lower in the DLPFC of individuals with schizophrenia (Curley et al., 2011). In contrast, GAD65 mRNA and protein levels have been reported to be unaltered in PFC total gray matter homogenate in schizophrenia (Glausier et al., 2015). However, a recent study investigating levels of GAD67 and GAD65 mRNA in superficial (layers 2/superficial 3) and deep (deep layer 6/superficial white matter) zones of the DLPFC in schizophrenia found zone-specific alterations of both transcripts in subjects with schizophrenia, reporting alterations of GAD67 and GAD65 mRNA in the superficial, but not the deep, zone (Dowling et al., 2023). Together, these data suggest that potential alterations of GAD65 may be limited to specific cellular populations that are obfuscated during measures of broader whole tissue studies. Indeed, when examining GAD67 protein levels within vGAT-positive boutons of the prefrontal cortex, GAD67 levels are significantly lower in the disease (Rocco et al., 2016a), suggesting that GABA production is reduced in a subset of prefrontal boutons in schizophrenia.
In contrast to the relatively robust findings of alterations of GAD67 in schizophrenia DLPFC, vGAT and GAT1 mRNA levels appear to be unaltered or only modestly lower in total gray matter homogenates of PFC (Fung et al., 2011; Hoftman et al., 2015) or PFC layer 3 samples (Hoftman et al., 2018). Furthermore, no significant alterations of vGAT protein levels within prefrontal synaptic boutons, nor the number of vGAT-positive boutons, have been observed (Rocco et al., 2016a). However, these findings may be a result of a lack of cellular-specific investigation. Indeed, it has been suggested that a subset of neurons may be more severely affected in the disease. Volk et al. (2000) reported a ∼25% deficit in the density of GAT1 mRNA-positive neurons in schizophrenia, indicating cellular loss. However, recent advancements of in situ hybridization methodology may now allow detection of cells with a previously undetectable GAT1 mRNA level via older methods (Dienel et al., 2023b). Thus, investigation of GAT1 mRNA-positive neuronal density should be conducted utilizing new methodology.
Although studies of presynaptic GABAergic transcripts and proteins within the postmortem PFC in schizophrenia are relatively few given the importance of the system in cognition, investigations of the GABAergic system are even more sparse in mood disorders with similar cognitive impairments as schizophrenia. Indeed, only two studies have been conducted to date investigating GABA synthesis within the postmortem PFC from individuals with schizoaffective disorder (Glausier et al., 2015), BPD (Dowling et al., 2023), or MDD (Dowling et al., 2023). Both GAD65 mRNA and protein in PFC total gray matter may be lower in those with schizoaffective disorder (Glausier et al., 2015). In contrast, Dowling et al. (2023) examined GAD67 and GAD65 mRNA levels in superficial (layers 2/superficial 3) and deep (deep layer 6/white matter) zones of the DLPFC in BPD and MDD subjects, and reported no significant alterations of either transcript, regardless of laminar zone or diagnosis.
Postsynaptic GABAergic alterations in schizophrenia
In addition to deficits of presynaptic GABAergic function in the interneurons of the DLPFC microcircuitry, findings of postsynaptic alterations in apposition to inhibitory inputs in schizophrenia have been reported (Mohler, 2006; Glausier et al., 2010; Beneyto et al., 2011; Fish et al., 2018). Both the α1 subunit and the β2 subunit of the GABAA receptor are lower in schizophrenia DLPFC layers 3–4 (Beneyto et al., 2011). These subunits colocalize with the α1 subunit at PVBC inputs (Mohler, 2006). Furthermore, PVBC, but not PV ChC, terminals are highest in density within PFC layer 4 (Fish et al., 2018). Therefore, given that the local layer 3 pyramidal neurons likely to receive these inhibitory inputs also exhibit lower levels of α1 mRNA (Glausier et al., 2010), the data together suggest a dampening of the inhibitory signal received by pyramidal neurons from PVBCs.
Additionally, the postsynapse of ChC inputs have been reported to be altered in schizophrenia. As previously mentioned, ChCs are predominantly located within PFC layer 2 (Fish et al., 2018) and exhibit α2-enriched GABAA receptors postsynaptic to their inputs (Nusser et al., 1996; Loup et al., 1998). Within this cortical layer enriched in ChCs (Fish et al., 2018), the α2 mRNA expression and density of α2-positive cartridges have been reported to be upregulated in the illness, suggesting distinct alterations unique to PV interneuron subtype.
Alterations of postsynaptic SST inputs, although infrequently studied, have been reported in a laminar and cell-type specific manner. Indeed, lower levels of the α5 subunit of the GABAA receptor, often found in opposition to SST inputs (Klausberger et al., 2002; Silberberg and Markram, 2007), have been reported to be lower in DLPFC layer 4 (Beneyto et al., 2011). Such an alteration likely reflects abnormalities of the α5 subunit in the PV cells predominantly localized to layer 4 within the cortex, although this remains untested. Together, with the well-replicated downregulation of SST in schizophrenia, these data indicate lower activity of SST neurotransmission. However, a recent publication investigating key ionotropic receptor subunits across four cortical regions, including the DLPFC, in schizophrenia, did not report alterations of the α5 subunit within total gray matter homogenate of the DLPFC, but did report deficits of the subunit in caudal cortical regions (Schoonover et al., 2024), suggesting there could be region-specific alterations in the illness.
Assessment of postsynaptic GABAA receptor subunits in BPD or MDD have primarily been conducted within total gray matter or the somal body of pyramidal neurons in DLPFC, and not at the level of synaptic boutons. However, higher expression of the α1 subunit but lower expression of the β1 subunit within the DLPFC total gray matter has been reported in BPD (Fatemi et al., 2017). In contrast, when assessed at the level of pyramidal neuron soma, a second study reports unaltered protein levels of the α1 subunit, but elevated β2/3 protein levels, in BPD (Ishikawa et al., 2004). Lastly, a third study investigating β2 mRNA levels within total gray matter of the anterior cingulate cortex and DLPFC in elderly non-suicidal MDD patients reported lower expression within the anterior cingulate cortex, but not the DLPFC (Zhao et al., 2012).
Cortical interneuron pathology in other regions: implications for working memory
Although we have focused on interneuron pathology within human postmortem DLPFC as it pertains to WM impairments in schizophrenia, this does not suggest that there are no alterations within other regions that affect WM capability in the illness. Indeed, the inhibitory neurons within the deep cortical zone of the PFC are known to innervate pyramidal neurons that, in turn, are reciprocally connected to a variety of thalamic nuclei (Preuss and Goldman-Rakic, 1987), and provide inhibition to several other brain regions (Tomioka et al., 2005; Tomioka and Rockland, 2007; Tamamaki and Tomioka, 2010; Tomioka et al., 2015; Tremblay et al., 2016; Lim et al., 2018). Indeed, the thalamus, and particularly the mediodorsal nucleus of the thalamus (MD), has been implicated as crucially involved in the WM network (Niendam et al., 2012; Bolkan et al., 2017; Murray and Anticevic, 2017). The MD, like the PFC, exhibits sustained WM delay-related activity (Watanabe and Funahashi, 2012), and lesions to the MD in humans result in significant impairments in cognitive functions, particularly executive abilities and memory (Dagenbach et al., 2001; Kubat-Silman et al., 2002; Van der Werf et al., 2003).
Although the full complexity of PFC-thalamic connectivity in schizophrenia is beyond the scope of this review, altered signaling from the MD may substantially impact PV interneurons and their functional capability in WM. Indeed, the MD, which has been shown to exhibit reduced connectivity in patients with schizophrenia (Welsh et al., 2010; Woodward et al., 2012; Anticevic et al., 2014a; Anticevic et al., 2014b; Klingner et al., 2014; Tu et al., 2015; Wang et al., 2015; Lerman-Sinkoff and Barch, 2016) and to a lesser extent BPD (Anticevic et al., 2014a; Anticevic et al., 2014b; Woodward and Heckers, 2016), targets several types of interneurons as well as pyramidal neurons within cortical layers 1, 2–3, and 5 (Ferguson and Gao, 2018a; Lewis et al., 2022). Thus, reduced input from the MD to the PFC is likely to result in abnormal excitatory/inhibitory balance within the cortical microcircuitry associated with WM capability. Indeed, the activity of MD neurons is correlated with the spiking of PV interneurons in the medial PFC in mice (Schmitt et al., 2017; Ferguson and Gao, 2018b), indicating that a dampening of the MD activity selectively reduces inhibitory currents onto pyramidal neurons (Ferguson and Gao, 2018b), similar to the findings observed in postmortem tissue as previously discussed. However, postmortem assessments of MD cell counts and volume in schizophrenia have been inconsistent (Heckers, 1997; Pakkenberg et al., 2009; Dorph-Petersen and Lewis, 2017), although similar investigations within the pulvinar nuclei of the posterior thalamus suggest reduced pulvinar volume and cellular density (Dorph-Petersen and Lewis, 2017). Thus, further study is needed of the MD thalamic nuclei in schizophrenia postmortem tissue to identify the neural substrate of the observed in vivo MD alterations in the illness.
Taken together, these data indicate that WM capability is subserved by a multi-node network involving both cortical and subcortical regions, and investigations of PFC-only GABAergic neurotransmission likely represent only a part of the larger circuitry associated with WM.
Challenges in measuring GABA levels directly
This review has largely focused on alterations in molecular and neural circuit properties of GABAergic interneuron subtypes in nonhuman primate or human postmortem PFC from individuals with schizophrenia, which have been essential to our understanding of schizophrenia pathobiology. To continue to uncover the mechanistic mysteries underlying this illness for the creation of better pharmacotherapies, a large portion of research in this field has made use of preclinical techniques and model systems (Canetta et al., 2016; Kaneko et al., 2017; Smith et al., 2019; Arias et al., 2022). Preclinically, physiological techniques like electrophysiology combined with optogenetics have been widely used to study the electrical and physiological properties of cortical interneurons, both in vivo and in vitro, to electrically stimulate and record the transmission of neuronal signals (Sohal et al., 2009; Anastasiades et al., 2021; Maksymetz et al., 2021). The addition of pharmacological and molecular approaches allowed scientists the ability to decipher extracellular, intercellular, and intracellular signals within the brain to decipher the role of GABAergic interneurons, GABA receptors, ion channels, and certain neurotransmitters in specific neural circuits and behaviors associated with schizophrenia. For example, ketamine has been used to illustrate a dose-dependent effect on PV-positive interneuron physiology in a GAD1-knockdown model of schizophrenia resulting in molecular, physiological, and behavioral changes in mouse models (Brown et al., 2015). Thus, although using electrophysiology combined with pharmacology are classical and established methods of scientific investigation, we still rely heavily on these techniques to be able to infer the activity of neurotransmitters like GABA in preclinical models of aspects of schizophrenia.
Nevertheless, the aforementioned techniques do not directly measure GABA levels, which would be ideal for preclinical schizophrenia research based on the involvement of the GABA system in its underlying neural circuit processes. To guide future preclinical schizophrenia research involving GABA, there is a need for assessment of GABA levels that considers its multidimensional effects. Therefore, the utilization of genetically encoded fluorescent biosensors combined with pharmacology and physiological techniques allows for in vivo and potentially ex vivo optical assessment of pharmacological manipulation of GABA transients, providing novel understanding of the physiological mechanisms behind the role of GABA in schizophrenia pathology (Marvin et al., 2019; Guo et al., 2021).
In clinical research, the use of different types of electroencephalography (EEG) combined with neuroimaging tools is becoming more widespread in the investigation of GABAergic deficits associated with cognitive symptoms underlying schizophrenia pathophysiology. In relation to WM, several studies have used transcranial magnetic stimulation with EEG (TMS-EEG) to show connections between GABAergic neurotransmission and neural oscillations (Daskalakis et al., 2006; McClintock et al., 2011; Lett et al., 2014). In healthy subjects, single and paired-pulse variations of long interval cortical inhibition (LICI) in the DLPFC have been used with TMS-EEG to implicate GABAergic neurotransmission with performance in WM tasks (Daskalakis et al., 2008; Rogasch et al., 2015). Additionally, LICI and TMS-EEG have been applied in clinical studies of schizophrenia patients and healthy controls to implicate the contribution of GAD67 gene GAD1 in WM deficits associated with schizophrenia (Lett et al., 2016). Importantly, these studies do not directly assess the relationship between components of GABAergic neurotransmission with WM deficits in patients with schizophrenia specifically but can be used to infer neurophysiological processes for the development and testing of better treatments (Lett et al., 2016).
Sex differences remain understudied
Sex differences were rarely considered in the science and medicine until the last century (Yoon et al., 2014). Males were commonly used as a baseline of human body function, with hormones as a major perceived difference in females. Additionally, in the rare instances where both male and female subjects were assessed, the data was often not separated and analyzed by sex. We now know that all hormones, even those historically associated with one sex, are prevalent in both sexes and have large effects on the body and emotional processes of the brain (Lauretta et al., 2018; Miguel-Aliaga, 2022). In more modern times, sex differences have been identified in many mood disorders such as MDD and BPD (Douillard-Guilloux et al., 2017; Girgenti et al., 2019). For example, MDD was reported to have sex-specific differences in the number of SST interneurons, where fewer SST neurons were present in females compared to males, suggesting there is likely a neural substrate to the sex differences in the diagnosis of MDD (Douillard-Guilloux et al., 2017; Girgenti et al., 2019). However, even with the advancements made in expanding research into sex differences in serious mental illness, much progress has yet to be made.
After examining the relevant literature previously cited within this review, we found that approximately half of the reports investigated sex as a variable. Of those, approximately 20% reported sex-specific differences that were often complex (relying upon examination of specific cortical layer versus total gray matter) or no longer statistically significant following correction for multiple comparisons. Indeed, subunits of the GABAA receptor did not differ overall by sex in schizophrenia when compared to those unaffected by the disorder but did have higher expression of the GABAA receptor subunit α2 mRNA within the DLPFC layer 2 in males versus female individuals diagnosed with schizophrenia (Beneyto et al., 2011). Furthermore, GABAA receptor subunit α2 mRNA expression within layer 2 was higher in male subjects with schizophrenia than in male unaffected comparison subjects (Beneyto et al., 2011). Additionally, women expressed lower GAD67 and GABAA receptor subunit α4 mRNA (Duncan et al., 2010), but higher SST and lower PV mRNA (Mellios et al., 2009). Significant findings of GABAB receptor subunit protein expression by sex did not withstand correction for multiple comparisons in BPD (Fatemi et al., 2017).
It is important to note that in many studies, including some but not all examined here, subject cohorts are not well-powered enough to adequately assess the effect of sex as a biological variable, often having fewer than 50% female representation. It is crucial to investigate the possibility of sex differences in schizophrenia, given that close to half of patients with this disorder are female (McGrath et al., 2008). Expanding cohorts to include both sexes, comparing data based on sex, and investigating the effect of hormones would greatly improve our current understanding of schizophrenia and its underlying altered neural circuit mechanisms.
Conclusion
In summary, research into cortical interneurons in schizophrenia reveals a complex pattern of alterations, particularly in the DLPFC. PV interneurons, critical for local inhibitory control, show consistent deficits in mRNA and protein levels, with evidence suggesting reduced functionality rather than a reduction in cellular density. Notably, deficits are observed specifically in PVBCs, but not necessarily in ChCs, indicating subtype-specific effects. In contrast, CR interneurons appear largely unaffected, although regional and methodological variations exist. SST interneurons display robust downregulation of mRNA levels, suggesting impaired inhibitory signaling; however, this does not correspond to a reduction in cell density. Findings related to other interneuron types, such as CB and VIP, are more variable and less consistent, with some reports suggesting elevated or unchanged levels depending on the specific study and methodology. NPY and CCK interneurons also present mixed results, with some evidence of reduced expression in schizophrenia. Finally, the overall GABAergic system shows disrupted function, including reduced GAD67 levels and altered postsynaptic receptor subunit expression. These findings highlight the nuanced and region-specific nature of interneuron pathology in schizophrenia, which may contribute to the cognitive deficits observed in the disorder.
Lastly, research on interneurons in the PFC of individuals with BPD or MDD reveal inconsistent findings. Studies on PV, CR, and SST cells show mixed results, with some evidence of deficits in either BPD or MDD, and cortical layer-specific effects adding complexity. CB cell densities vary by region and layer, showing elevations in BPD and deficits in MDD, although VIP findings suggest possible shared pathology between the two disorders. NPY levels are lower in BPD but not MDD. Research on GAD65 and GAD67 mRNA and protein shows few changes in BPD and MDD. Studies on CCK and GABAA receptor subunits also present limited and variable results. Overall, these inconsistencies highlight the need for more research to better understand the role of these interneurons in cognitive function within affective disorder pathology.
Author contributions
HH: Conceptualization, Investigation, Writing – original draft, Writing – review & editing. LB: Conceptualization, Investigation, Resources, Supervision, Writing – original draft, Writing – review & editing. KS: Conceptualization, Investigation, Project administration, Resources, Supervision, Visualization, Writing – original draft, Writing – review & editing.
Funding
The authors declare that financial support was received for the research, authorship, and/or publication of this article. This article was supported by the Brain and Behavior Research Foundation Young Investigator Award (LB and KS), the National Institute on Drug Abuse of the National Institutes of Health (R00DA052641 to LB), and the National Institute of Health Faculty Institutional Recruitment for Sustainable Transformation (FIRST) award (U54CA267746 to KS).
Acknowledgments
None of the discussed work would be possible without the generous gifts from donors and their families.
Conflict of interest
The authors declare that the research was conducted in the absence of any commercial or financial relationships that could be construed as a potential conflict of interest.
Publisher’s note
All claims expressed in this article are solely those of the authors and do not necessarily represent those of their affiliated organizations, or those of the publisher, the editors and the reviewers. Any product that may be evaluated in this article, or claim that may be made by its manufacturer, is not guaranteed or endorsed by the publisher.
References
Abbas, A. I., Sundiang, M. J. M., Henoch, B., Morton, M. P., Bolkan, S. S., Park, A. J., et al. (2018). Somatostatin interneurons facilitate hippocampal-prefrontal synchrony and prefrontal spatial encoding. Neuron 100:926–939.e923. doi: 10.1016/j.neuron.2018.09.029
Akbarian, S., Kim, J. J., Potkin, S. G., Hagman, J. O., Tafazzoli, A., and Bunney, W. E. Jr., et al. (1995). Gene expression for glutamic acid decarboxylase is reduced without loss of neurons in prefrontal cortex of schizophrenics. Arch. Gen. Psychiatry 52, 258–266. doi: 10.1001/archpsyc.1995.03950160008002
Anastasiades, P. G., Collins, D. P., and Carter, A. G. (2021). Mediodorsal and ventromedial thalamus engage distinct L1 circuits in the prefrontal cortex. Neuron 109:314–330.e314. doi: 10.1016/j.neuron.2020.10.031
Andersson, R., Johnston, A., and Fisahn, A. (2012). Dopamine D4 receptor activation increases hippocampal gamma oscillations by enhancing synchronization of fast-spiking interneurons. PLoS One 7:e40906. doi: 10.1371/journal.pone.0040906
Ang, L. C., and Shul, D. D. (1995). Peptidergic neurons of subcortical white matter in aging and Alzheimer’s brain. Brain Res. 674, 329–335. doi: 10.1016/0006-8993(95)00034-n
Anticevic, A., Cole, M. W., Repovs, G., Murray, J. D., Brumbaugh, M. S., Winkler, A. M., et al. (2014a). Characterizing thalamo-cortical disturbances in schizophrenia and bipolar illness. Cereb. Cortex 24, 3116–3130. doi: 10.1093/cercor/bht165
Anticevic, A., Yang, G., Savic, A., Murray, J. D., Cole, M. W., Repovs, G., et al. (2014b). Mediodorsal and visual thalamic connectivity differ in schizophrenia and bipolar disorder with and without psychosis history. Schizophr. Bull. 40, 1227–1243. doi: 10.1093/schbul/sbu100
Arias, H. R., Germann, A. L., Pierce, S. R., Sakamoto, S., Ortells, M. O., Hamachi, I., et al. (2022). Modulation of the mammalian GABA(A) receptor by type I and type II positive allosteric modulators of the α7 nicotinic acetylcholine receptor. Br. J. Pharmacol. 179, 5323–5337.
Asada, H., Kawamura, Y., Maruyama, K., Kume, H., Ding, R. G., Kanbara, N., et al. (1997). Cleft palate and decreased brain gamma-aminobutyric acid in mice lacking the 67-kDa isoform of glutamic acid decarboxylase. Proc. Natl. Acad. Sci. U.S.A. 94, 6496–6499. doi: 10.1073/pnas.94.12.6496
Barch, D. M. (2009). Neuropsychological abnormalities in schizophrenia and major mood disorders: Similarities and differences. Curr. Psychiatry Rep. 11, 313–319.
Barde, S., Aguila, J., Zhong, W., Solarz, A., Mei, I., Prud’homme, J., et al. (2024). Substance P, NPY, CCK and their receptors in five brain regions in major depressive disorder with transcriptomic analysis of locus coeruleus neurons. Eur. Neuropsychopharmacol. 78, 54–63. doi: 10.1016/j.euroneuro.2023.09.004
Barinka, F., and Druga, R. (2010). Calretinin expression in the mammalian neocortex: A review. Physiol. Res. 59, 665–677.
Bastos, A. M., Loonis, R., Kornblith, S., Lundqvist, M., and Miller, E. K. (2018). Laminar recordings in frontal cortex suggest distinct layers for maintenance and control of working memory. Proc. Natl. Acad. Sci. U.S.A. 115, 1117–1122. doi: 10.1073/pnas.1710323115
Beasley, C. L., Zhang, Z. J., Patten, I., and Reynolds, G. P. (2002). Selective deficits in prefrontal cortical GABAergic neurons in schizophrenia defined by the presence of calcium-binding proteins. Biol. Psychiatry 52, 708–715.
Benes, F. M., and Berretta, S. (2001). GABAergic interneurons: Implications for understanding schizophrenia and bipolar disorder. Neuropsychopharmacology 25, 1–27.
Benes, F. M., Lim, B., Matzilevich, D., Subburaju, S., and Walsh, J. P. (2008). Circuitry-based gene expression profiles in GABA cells of the trisynaptic pathway in schizophrenics versus bipolars. Proc. Natl. Acad. Sci. U.S.A. 105, 20935–20940. doi: 10.1073/pnas.0810153105
Beneyto, M., Abbott, A., Hashimoto, T., and Lewis, D. A. (2011). Lamina-specific alterations in cortical GABAA receptor subunit expression in schizophrenia. Cereb. Cortex 21, 999–1011. doi: 10.1093/cercor/bhq169
Bolkan, S. S., Stujenske, J. M., Parnaudeau, S., Spellman, T. J., Rauffenbart, C., Abbas, A. I., et al. (2017). Thalamic projections sustain prefrontal activity during working memory maintenance. Nat. Neurosci. 20, 987–996.
Brown, J. A., Ramikie, T. S., Schmidt, M. J., Báldi, R., Garbett, K., Everheart, M. G., et al. (2015). Inhibition of parvalbumin-expressing interneurons results in complex behavioral changes. Mol. Psychiatry 20, 1499–1507.
Buffalo, E. A., Fries, P., Landman, R., Buschman, T. J., and Desimone, R. (2011). Laminar differences in gamma and alpha coherence in the ventral stream. Proc. Natl. Acad. Sci. U.S.A. 108, 11262–11267. doi: 10.1073/pnas.1011284108
Bygrave, A. M., Jahans-Price, T., Wolff, A. R., Sprengel, R., Kullmann, D. M., Bannerman, D. M., et al. (2019). Hippocampal-prefrontal coherence mediates working memory and selective attention at distinct frequency bands and provides A causal link between schizophrenia and its risk gene GRIA1. Transl. Psychiatry 9:142. doi: 10.1038/s41398-019-0471-0
Caberlotto, L., and Hurd, Y. L. (1999). Reduced neuropeptide Y mRNA expression in the prefrontal cortex of subjects with bipolar disorder. Neuroreport 10, 1747–1750. doi: 10.1097/00001756-199906030-00022
Canetta, S., Bolkan, S., Padilla-Coreano, N., Song, L. J., Sahn, R., Harrison, N. L., et al. (2016). Maternal immune activation leads to selective functional deficits in offspring parvalbumin interneurons. Mol. Psychiatry 21, 956–968. doi: 10.1038/mp.2015.222
Canolty, R. T., and Knight, R. T. (2010). The functional role of cross-frequency coupling. Trends Cogn. Sci. 14, 506–515.
Cantero, J. L., Atienza, M., Stickgold, R., Kahana, M. J., Madsen, J. R., and Kocsis, B. (2003). Sleep-dependent theta oscillations in the human hippocampus and neocortex. J. Neurosci. 23, 10897–10903.
Carter, C. S., Perlstein, W., Ganguli, R., Brar, J., Mintun, M., and Cohen, J. D. (1998). Functional hypofrontality and working memory dysfunction in schizophrenia. Am. J. Psychiatry 155, 1285–1287.
Chen, G., Zhang, Y., Li, X., Zhao, X., Ye, Q., Lin, Y., et al. (2017). Distinct inhibitory circuits orchestrate cortical beta and gamma band oscillations. Neuron 96:1403–1418.e1406. doi: 10.1016/j.neuron.2017.11.033
Cho, R. Y., Konecky, R. O., and Carter, C. S. (2006). Impairments in frontal cortical gamma synchrony and cognitive control in schizophrenia. Proc. Natl. Acad. Sci. U.S.A. 103, 19878–19883. doi: 10.1073/pnas.0609440103
Chung, D. W., Volk, D. W., Arion, D., Zhang, Y., Sampson, A. R., and Lewis, D. A. (2016b). Dysregulated ErbB4 splicing in schizophrenia: Selective effects on parvalbumin expression. Am. J. Psychiatry 173, 60–68. doi: 10.1176/appi.ajp.2015.15020150
Chung, D. W., Fish, K. N., and Lewis, D. A. (2016a). Pathological basis for deficient excitatory drive to cortical parvalbumin interneurons in schizophrenia. Am. J. Psychiatry 173, 1131–1139. doi: 10.1176/appi.ajp.2016.16010025
Colgin, L. L., and Moser, E. I. (2010). Gamma oscillations in the hippocampus. Physiology 25, 319–329.
Conde, F., Lund, J. S., Jacobowitz, D. M., Baimbridge, K. G., and Lewis, D. A. (1994). Local circuit neurons immunoreactive for calretinin, calbindin D-28k or parvalbumin in monkey prefrontal cortex: Distribution and morphology. J. Comp. Neurol. 341, 95–116.
Constantinidis, C., Williams, G. V., and Goldman-Rakic, P. S. (2002). A role for inhibition in shaping the temporal flow of information in prefrontal cortex. Nat. Neurosci. 5, 175–180. doi: 10.1038/nn799
Cotter, D., Landau, S., Beasley, C., Stevenson, R., Chana, G., Macmillan, L., et al. (2002). The density and spatial distribution of GABAergic neurons, labelled using calcium binding proteins, in the anterior cingulate cortex in major depressive disorder, bipolar disorder, and schizophrenia. Biol. Psychiatry 51, 377–386. doi: 10.1016/s0006-3223(01)01243-4
Cousijn, H., Tunbridge, E. M., Rolinski, M., Wallis, G., Colclough, G. L., Woolrich, M. W., et al. (2015). Modulation of hippocampal theta and hippocampal-prefrontal cortex function by a schizophrenia risk gene. Hum. Brain Mapp. 36, 2387–2395. doi: 10.1002/hbm.22778
Curley, A. A., Arion, D., Volk, D. W., Asafu-Adjei, J. K., Sampson, A. R., Fish, K. N., et al. (2011). Cortical deficits of glutamic acid decarboxylase 67 expression in schizophrenia: Clinical, protein, and cell type-specific features. Am. J. Psychiatry 168, 921–929. doi: 10.1176/appi.ajp.2011.11010052
Dagenbach, D., Kubat-Silman, A. K., and Absher, J. R. (2001). Human verbal working memory impairments associated with thalamic damage. Int. J. Neurosci. 111, 67–87.
Daskalakis, Z. J., Farzan, F., Barr, M. S., Rusjan, P. M., Favalli, G., Levinson, A. J., et al. (2008). Evaluating the relationship between long interval cortical inhibition, working memory and gamma band activity in the dorsolateral prefrontal cortex. Clin. EEG Neurosci. 39, 150–155. doi: 10.1177/155005940803900310
Daskalakis, Z. J., Möller, B., Christensen, B. K., Fitzgerald, P. B., Gunraj, C., and Chen, R. (2006). The effects of repetitive transcranial magnetic stimulation on cortical inhibition in healthy human subjects. Exp. Brain Res. 174, 403–412.
Daviss, S. R., and Lewis, D. A. (1995). Local circuit neurons of the prefrontal cortex in schizophrenia: Selective increase in the density of calbindin-immunoreactive neurons. Psychiatry Res. 59, 81–96. doi: 10.1016/0165-1781(95)02720-3
del Río, M. R., and DeFelipe, J. (1997a). Colocalization of parvalbumin and calbindin D-28k in neurons including chandelier cells of the human temporal neocortex. J. Chem. Neuroanat. 12, 165–173. doi: 10.1016/s0891-0618(96)00191-3
del Río, M. R., and DeFelipe, J. (1997b). Synaptic connections of calretinin-immunoreactive neurons in the human neocortex. J. Neurosci. 17, 5143–5154.
Dienel, S. J., and Lewis, D. A. (2019). Alterations in cortical interneurons and cognitive function in schizophrenia. Neurobiol. Dis. 131:104208.
Dienel, S. J., Ciesielski, A. J., Bazmi, H. H., Profozich, E. A., Fish, K. N., and Lewis, D. A. (2021). Distinct laminar and cellular patterns of GABA neuron transcript expression in monkey prefrontal and visual cortices. Cereb. Cortex 31, 2345–2363. doi: 10.1093/cercor/bhaa341
Dienel, S. J., Fish, K. N., and Lewis, D. A. (2023b). The nature of prefrontal cortical gaba neuron alterations in schizophrenia: Markedly lower somatostatin and parvalbumin gene expression without missing neurons. Am. J. Psychiatry 180, 495–507. doi: 10.1176/appi.ajp.20220676
Dienel, S. J., Dowling, K. F., Barile, Z., Bazmi, H. H., Liu, A., Vespoli, J. C., et al. (2023a). Diagnostic specificity and association with cognition of molecular alterations in prefrontal somatostatin neurons in schizophrenia. JAMA Psychiatry 80, 1235–1245. doi: 10.1001/jamapsychiatry.2023.2972
Donato, F., Rompani, S. B., and Caroni, P. (2013). Parvalbumin-expressing basket-cell network plasticity induced by experience regulates adult learning. Nature 504, 272–276. doi: 10.1038/nature12866
Dorph-Petersen, K. A., and Lewis, D. A. (2017). Postmortem structural studies of the thalamus in schizophrenia. Schizophr. Res. 180, 28–35.
Douillard-Guilloux, G., Lewis, D., Seney, M. L., and Sibille, E. (2017). Decrease in somatostatin-positive cell density in the amygdala of females with major depression. Depress. Anxiety 34, 68–78. doi: 10.1002/da.22549
Dowling, K. F., Dienel, S. J., Barile, Z., Bazmi, H. H., and Lewis, D. A. (2023). Localization and diagnostic specificity of glutamic acid decarboxylase transcript alterations in the dorsolateral prefrontal cortex in schizophrenia. Biol. Psychiatry 94, 322–331. doi: 10.1016/j.biopsych.2023.04.003
Duncan, C. E., Webster, M. J., Rothmond, D. A., Bahn, S., Elashoff, M., and Shannon Weickert, C. (2010). Prefrontal GABA(A) receptor alpha-subunit expression in normal postnatal human development and schizophrenia. J. Psychiatr. Res. 44, 673–681.
Eggan, S. M., Melchitzky, D. S., Sesack, S. R., Fish, K. N., and Lewis, D. A. (2010). Relationship of cannabinoid CB1 receptor and cholecystokinin immunoreactivity in monkey dorsolateral prefrontal cortex. Neuroscience 169, 1651–1661. doi: 10.1016/j.neuroscience.2010.06.011
Engel, A. K., Fries, P., and Singer, W. (2001). Dynamic predictions: Oscillations and synchrony in top-down processing. Nat. Rev. Neurosci. 2, 704–716.
Enwright, J. F. I., Huo, Z., Arion, D., Corradi, J. P., Tseng, G., and Lewis, D. A. (2018). Transcriptome alterations of prefrontal cortical parvalbumin neurons in schizophrenia. Mol. Psychiatry 23, 1606–1613.
Enwright, J. F., and Lewis, D. A. (2021). Similarities in cortical transcriptome alterations between schizophrenia and bipolar disorder are related to the presence of psychosis. Schizophr. Bull. 47, 1442–1451. doi: 10.1093/schbul/sbaa195
Enwright, J. F., Sanapala, S., Foglio, A., Berry, R., Fish, K. N., and Lewis, D. A. (2016). Reduced labeling of parvalbumin neurons and perineuronal nets in the dorsolateral prefrontal cortex of subjects with schizophrenia. Neuropsychopharmacology 41, 2206–2214. doi: 10.1038/npp.2016.24
Erickson, J. D., De Gois, S., Varoqui, H., Schafer, M. K., and Weihe, E. (2006). Activity-dependent regulation of vesicular glutamate and GABA transporters: A means to scale quantal size. Neurochem. Int. 48, 643–649. doi: 10.1016/j.neuint.2005.12.029
Erickson, M. A., Albrecht, M. A., Robinson, B., Luck, S. J., and Gold, J. M. (2017). Impaired suppression of delay-period alpha and beta is associated with impaired working memory in schizophrenia. Biol. Psychiatry Cogn. Neurosci. Neuroimaging 2, 272–279. doi: 10.1016/j.bpsc.2016.09.003
Espenhahn, S., Van Wijk, B. C. M., Rossiter, H. E., De Berker, A. O., Redman, N. D., Rondina, J., et al. (2019). Cortical beta oscillations are associated with motor performance following visuomotor learning. Neuroimage 195, 340–353.
Fatemi, S. H., Folsom, T. D., and Thuras, P. D. (2017). GABAA and GABAB receptor dysregulation in superior frontal cortex of subjects with schizophrenia and bipolar disorder. Synapse 71:73. doi: 10.1002/syn.21973
Ferguson, B. R., and Gao, W. J. (2018a). PV interneurons: Critical regulators of E/I balance for prefrontal cortex-dependent behavior and psychiatric disorders. Front. Neural Circuits 12:37. doi: 10.3389/fncir.2018.00037
Ferguson, B. R., and Gao, W. J. (2018b). Thalamic control of cognition and social behavior via regulation of gamma-aminobutyric acidergic signaling and excitation/inhibition balance in the medial prefrontal cortex. Biol. Psychiatry 83, 657–669. doi: 10.1016/j.biopsych.2017.11.033
Fish, K. N., Rocco, B. R., and Lewis, D. A. (2018). Laminar distribution of subsets of GABAergic axon terminals in human prefrontal cortex. Front. Neuroanat. 12:9. doi: 10.3389/fnana.2018.00009
Fish, K. N., Sweet, R. A., and Lewis, D. A. (2011). Differential distribution of proteins regulating GABA synthesis and reuptake in axon boutons of subpopulations of cortical interneurons. Cereb. Cortex 21, 2450–2460. doi: 10.1093/cercor/bhr007
Froudist-Walsh, S., Bliss, D. P., Ding, X., Rapan, L., Niu, M., Knoblauch, K., et al. (2021). A dopamine gradient controls access to distributed working memory in the large-scale monkey cortex. Neuron 109:3500–3520.e3513. doi: 10.1016/j.neuron.2021.08.024
Fung, S. J., Fillman, S. G., Webster, M. J., and Shannon Weickert, C. (2014). Schizophrenia and bipolar disorder show both common and distinct changes in cortical interneuron markers. Schizophr. Res. 155, 26–30. doi: 10.1016/j.schres.2014.02.021
Fung, S. J., Sivagnanasundaram, S., and Weickert, C. S. (2011). Lack of change in markers of presynaptic terminal abundance alongside subtle reductions in markers of presynaptic terminal plasticity in prefrontal cortex of schizophrenia patients. Biol. Psychiatry 69, 71–79. doi: 10.1016/j.biopsych.2010.09.036
Fung, S. J., Webster, M. J., Sivagnanasundaram, S., Duncan, C., Elashoff, M., and Weickert, C. S. (2010). Expression of interneuron markers in the dorsolateral prefrontal cortex of the developing human and in schizophrenia. Am. J. Psychiatry 167, 1479–1488.
Gabbott, P. L., and Bacon, S. J. (1996). Local circuit neurons in the medial prefrontal cortex (areas 24a,b,c, 25 and 32) in the monkey: I. Cell morphology and morphometrics. J. Comp. Neurol. 364, 567–608. doi: 10.1002/(SICI)1096-9861(19960122)364:4<567::AID-CNE1>3.0.CO;2-1
Gabbott, P. L., and Bacon, S. J. (1997). Vasoactive intestinal polypeptide containing neurones in monkey medial prefrontal cortex (mPFC): Colocalisation with calretinin. Brain Res. 744, 179–184. doi: 10.1016/s0006-8993(96)01232-2
Gabbott, P. L., Jays, P. R., and Bacon, S. J. (1997). Calretinin neurons in human medial prefrontal cortex (areas 24a,b,c, 32′, and 25). J. Comp. Neurol. 381, 389–410. doi: 10.1002/(sici)1096-9861(19970519)381:4<389::aid-cne1>3.0.co;2-z
Gabriel, S. M., Davidson, M., Haroutunian, V., Powchik, P., Bierer, L. M., Purohit, D. P., et al. (1996). Neuropeptide deficits in schizophrenia vs. Alzheimer’s disease cerebral cortex. Biol. Psychiatry 39, 82–91.
Gandal, M. J., Edgar, J. C., Klook, K., and Siegel, S. J. (2012). Gamma synchrony: Towards a translational biomarker for the treatment-resistant symptoms of schizophrenia. Neuropharmacology 62, 1504–1518. doi: 10.1016/j.neuropharm.2011.02.007
Girgenti, M. J., Wohleb, E. S., Mehta, S., Ghosal, S., Fogaca, M. V., and Duman, R. S. (2019). Prefrontal cortex interneurons display dynamic sex-specific stress-induced transcriptomes. Transl. Psychiatry 9:292. doi: 10.1038/s41398-019-0642-z
Glahn, D. C., Ragland, J. D., Abramoff, A., Barrett, J., Laird, A. R., Bearden, C. E., et al. (2005). Beyond hypofrontality: A quantitative meta-analysis of functional neuroimaging studies of working memory in schizophrenia. Hum. Brain Mapp. 25, 60–69. doi: 10.1002/hbm.20138
Glausier, J. R., Bazmi, H. H., and Lewis, D. A. (2010). GABA A alpha 1 subunit mRNA expression in pyramidal cells and interneurons in the dorsolateral prefrontal cortex of schizophrenia subjects. Biol. Psychiatry 67, 74S–74S.
Glausier, J. R., Fish, K. N., and Lewis, D. A. (2014). Altered parvalbumin basket cell inputs in the dorsolateral prefrontal cortex of schizophrenia subjects. Mol. Psychiatry 19, 30–36. doi: 10.1038/mp.2013.152
Glausier, J. R., Kimoto, S., Fish, K. N., and Lewis, D. A. (2015). Lower glutamic acid decarboxylase 65-kDa isoform messenger RNA and protein levels in the prefrontal cortex in schizoaffective disorder but not schizophrenia. Biol. Psychiatry 77, 167–176. doi: 10.1016/j.biopsych.2014.05.010
Gonzalez-Albo, M. C., Elston, G. N., and Defelipe, J. (2001). The human temporal cortex: Characterization of neurons expressing nitric oxide synthase, neuropeptides and calcium-binding proteins, and their glutamate receptor subunit profiles. Cereb. Cortex 11, 1170–1181. doi: 10.1093/cercor/11.12.1170
Gonzalez-Burgos, G., and Lewis, D. A. (2008). GABA neurons and the mechanisms of network oscillations: Implications for understanding cortical dysfunction in schizophrenia. Schizophr. Bull. 34, 944–961. doi: 10.1093/schbul/sbn070
Gonzalez-Burgos, G., Cho, R. Y., and Lewis, D. A. (2015). Alterations in cortical network oscillations and parvalbumin neurons in schizophrenia. Biol. Psychiatry 77, 1031–1040.
Gonzalez-Burgos, G., Rotaru, D. C., Zaitsev, A. V., Povysheva, N. V., and Lewis, D. A. (2009). GABA transporter GAT1 prevents spillover at proximal and distal GABA synapses onto primate prefrontal cortex neurons. J. Neurophysiol. 101, 533–547. doi: 10.1152/jn.91161.2008
Green, M. F. (1996). What are the functional consequences of neurocognitive deficits in schizophrenia? Am. J. Psychiatry 153, 321–330.
Guo, J., Ran, M., Gao, Z., Zhang, X., Wang, D., Li, H., et al. (2021). Cell-type-specific imaging of neurotransmission reveals a disrupted excitatory-inhibitory cortical network in isoflurane anaesthesia. EBioMedicine 65:103272. doi: 10.1016/j.ebiom.2021.103272
Harmony, T. (2013). The functional significance of delta oscillations in cognitive processing. Front. Integr. Neurosci. 7:83. doi: 10.3389/fnint.2013.00083
Hartmann, K., Bruehl, C., Golovko, T., and Draguhn, A. (2008). Fast homeostatic plasticity of inhibition via activity-dependent vesicular filling. PLoS One 3:e2979. doi: 10.1371/journal.pone.0002979
Harvey, P. D., Howanitz, E., Parrella, M., White, L., Davidson, M., Mohs, R. C., et al. (1998). Symptoms, cognitive functioning, and adaptive skills in geriatric patients with lifelong schizophrenia: A comparison across treatment sites. Am. J. Psychiatry 155, 1080–1086. doi: 10.1176/ajp.155.8.1080
Hashimoto, T., Arion, D., Unger, T., Maldonado-Avilés, J. G., Morris, H. M., Volk, D. W., et al. (2008). Alterations in GABA-related transcriptome in the dorsolateral prefrontal cortex of subjects with schizophrenia. Mol. Psychiatry 13, 147–161.
Hashimoto, T., Bergen, S. E., Nguyen, Q. L., Xu, B., Monteggia, L. M., Pierri, J. N., et al. (2005). Relationship of brain-derived neurotrophic factor and its receptor TrkB to altered inhibitory prefrontal circuitry in schizophrenia. J. Neurosci. 25, 372–383. doi: 10.1523/JNEUROSCI.4035-04.2005
Hashimoto, T., Volk, D. W., Eggan, S. M., Mirnics, K., Pierri, J. N., Sun, Z., et al. (2003). Gene expression deficits in a subclass of GABA neurons in the prefrontal cortex of subjects with schizophrenia. J. Neurosci. 23, 6315–6326. doi: 10.1523/JNEUROSCI.23-15-06315.2003
Heckers, S. (1997). Neuropathology of schizophrenia: Cortex, thalamus, basal ganglia, and neurotransmitter-specific projection systems. Schizophr. Bull. 23, 403–421. doi: 10.1093/schbul/23.3.403
Hiltunen, T., Kantola, J., Abou Elseoud, A., Lepola, P., Suominen, K., Starck, T., et al. (2014). Infra-slow EEG fluctuations are correlated with resting-state network dynamics in fMRI. J. Neurosci. 34, 356–362.
Hoftman, G. D., Dienel, S. J., Bazmi, H. H., Zhang, Y., Chen, K., and Lewis, D. A. (2018). Altered gradients of glutamate and gamma-aminobutyric acid transcripts in the cortical visuospatial working memory network in schizophrenia. Biol. Psychiatry 83, 670–679. doi: 10.1016/j.biopsych.2017.11.029
Hoftman, G. D., Volk, D. W., Bazmi, H. H., Li, S., Sampson, A. R., and Lewis, D. A. (2015). Altered cortical expression of GABA-related genes in schizophrenia: Illness progression vs developmental disturbance. Schizophr. Bull. 41, 180–191. doi: 10.1093/schbul/sbt178
Howells, F. M., Temmingh, H. S., Hsieh, J. H., Van Dijen, A. V., Baldwin, D. S., and Stein, D. J. (2018). Electroencephalographic delta/alpha frequency activity differentiates psychotic disorders: A study of schizophrenia, bipolar disorder and methamphetamine-induced psychotic disorder. Transl. Psychiatry 8:75. doi: 10.1038/s41398-018-0105-y
Howes, O. D., and Shatalina, E. (2022). Integrating the neurodevelopmental and dopamine hypotheses of schizophrenia and the role of cortical excitation-inhibition balance. Biol. Psychiatry 92, 501–513. doi: 10.1016/j.biopsych.2022.06.017
Ikeda, M., Takahashi, A., Kamatani, Y., Momozawa, Y., Saito, T., Kondo, K., et al. (2019). Genome-wide association study detected novel susceptibility genes for schizophrenia and shared trans-populations/diseases genetic effect. Schizophr. Bull. 45, 824–834. doi: 10.1093/schbul/sby140
Ishikawa, M., Mizukami, K., Iwakiri, M., Hidaka, S., and Asada, T. (2004). Immunohistochemical and immunoblot study of GABA(A) alpha1 and beta2/3 subunits in the prefrontal cortex of subjects with schizophrenia and bipolar disorder. Neurosci. Res. 50, 77–84. doi: 10.1016/j.neures.2004.06.006
Jones, M. W. (2010). Errant ensembles: Dysfunctional neuronal network dynamics in schizophrenia. Biochem. Soc. Trans. 38, 516–521. doi: 10.1042/BST0380516
Kaneko, G., Sanganahalli, B. G., Groman, S. M., Wang, H., Coman, D., Rao, J., et al. (2017). Hypofrontality and posterior hyperactivity in early schizophrenia: Imaging and behavior in a preclinical model. Biol. Psychiatry 81, 503–513. doi: 10.1016/j.biopsych.2016.05.019
Kimoto, S., Bazmi, H. H., and Lewis, D. A. (2014). Lower expression of glutamic acid decarboxylase 67 in the prefrontal cortex in schizophrenia: Contribution of altered regulation by Zif268. Am. J. Psychiatry 171, 969–978. doi: 10.1176/appi.ajp.2014.14010004
Klausberger, T., and Somogyi, P. (2008). Neuronal diversity and temporal dynamics: The unity of hippocampal circuit operations. Science 321, 53–57. doi: 10.1126/science.1149381
Klausberger, T., Marton, L. F., O’neill, J., Huck, J. H., Dalezios, Y., Fuentealba, P., et al. (2005). Complementary roles of cholecystokinin- and parvalbumin-expressing GABAergic neurons in hippocampal network oscillations. J. Neurosci. 25, 9782–9793. doi: 10.1523/JNEUROSCI.3269-05.2005
Klausberger, T., Roberts, J. D., and Somogyi, P. (2002). Cell type- and input-specific differences in the number and subtypes of synaptic GABA(A) receptors in the hippocampus. J. Neurosci. 22, 2513–2521. doi: 10.1523/JNEUROSCI.22-07-02513.2002
Klimesch, W. (2012). α-band oscillations, attention, and controlled access to stored information. Trends Cogn. Sci. 16, 606–617. doi: 10.1016/j.tics.2012.10.007
Klingner, C. M., Langbein, K., Dietzek, M., Smesny, S., Witte, O. W., Sauer, H., et al. (2014). Thalamocortical connectivity during resting state in schizophrenia. Eur. Arch. Psychiatry Clin. Neurosci. 264, 111–119.
Knable, M. B., Barci, B. M., Webster, M. J., Meador-Woodruff, J., and Torrey, E. F. (2004). Molecular abnormalities of the hippocampus in severe psychiatric illness: Postmortem findings from the Stanley neuropathology consortium. Mol. Psychiatry 9, 609–620. doi: 10.1038/sj.mp.4001471
Kubat-Silman, A. K., Dagenbach, D., and Absher, J. R. (2002). Patterns of impaired verbal, spatial, and object working memory after thalamic lesions. Brain Cogn. 50, 178–193. doi: 10.1016/s0278-2626(02)00502-x
Kuljis, R. O., and Rakic, P. (1989). Distribution of neuropeptide Y-containing perikarya and axons in various neocortical areas in the macaque monkey. J. Comp. Neurol. 280, 383–392. doi: 10.1002/cne.902800305
Kuromitsu, J., Yokoi, A., Kawai, T., Nagasu, T., Aizawa, T., Haga, S., et al. (2001). Reduced neuropeptide Y mRNA levels in the frontal cortex of people with schizophrenia and bipolar disorder. Brain Res. Gene Expr. Patterns 1, 17–21.
Lanz, T. A., Reinhart, V., Sheehan, M. J., Rizzo, S. J. S., Bove, S. E., James, L. C., et al. (2019). Postmortem transcriptional profiling reveals widespread increase in inflammation in schizophrenia: A comparison of prefrontal cortex, striatum, and hippocampus among matched tetrads of controls with subjects diagnosed with schizophrenia, bipolar or major depressive disorder. Transl. Psychiatry 9:151.
Lauretta, R., Sansone, M., Sansone, A., Romanelli, F., and Appetecchia, M. (2018). Gender in endocrine diseases: Role of sex gonadal hormones. Int. J. Endocrinol. 2018:4847376.
Lerman-Sinkoff, D. B., and Barch, D. M. (2016). Network community structure alterations in adult schizophrenia: Identification and localization of alterations. Neuroimage Clin. 10, 96–106. doi: 10.1016/j.nicl.2015.11.011
Lett, T. A., Kennedy, J. L., Radhu, N., Dominguez, L. G., Chakravarty, M. M., Nazeri, A., et al. (2016). Prefrontal white matter structure mediates the influence of GAD1 on working memory. Neuropsychopharmacology 41, 2224–2231. doi: 10.1038/npp.2016.14
Lett, T. A., Voineskos, A. N., Kennedy, J. L., Levine, B., and Daskalakis, Z. J. (2014). Treating working memory deficits in schizophrenia: A review of the neurobiology. Biol. Psychiatry 75, 361–370.
Lewis, D. A., and Gonzalez-Burgos, G. (2008). Neuroplasticity of neocortical circuits in schizophrenia. Neuropsychopharmacology 33, 141–165.
Lewis, D. A., and Hashimoto, T. (2007). Deciphering the disease process of schizophrenia: The contribution of cortical GABA neurons. Int. Rev. Neurobiol. 78, 109–131. doi: 10.1016/S0074-7742(06)78004-7
Lewis, D. A., and Lund, J. S. (1990). Heterogeneity of chandelier neurons in monkey neocortex: Corticotropin-releasing factor- and parvalbumin-immunoreactive populations. J. Comp. Neurol. 293, 599–615. doi: 10.1002/cne.902930406
Lewis, D. A., and Moghaddam, B. (2006). Cognitive dysfuntion in schizophrenia: Convergence of GABA and glutamate alterations. Arch. Neurol. 63, 1372–1376.
Lewis, D. A., Campbell, M. J., and Morrison, J. H. (1986). An immunohistochemical characterization of somatostatin-28 and somatostatin-281-12 in monkey prefrontal cortex. J. Comp. Neurol. 248, 1–18. doi: 10.1002/cne.902480102
Lewis, D. A., Pierri, J. N., Volk, D. W., Melchitzky, D. S., and Woo, T. U. (1999). Altered GABA neurotransmission and prefrontal cortical dysfunction in schizophrenia. Biol. Psychiatry 46, 616–626.
Lewis, E. M., Spence, H. E., Akella, N., and Buonanno, A. (2022). Pathway-specific contribution of parvalbumin interneuron NMDARs to synaptic currents and thalamocortical feedforward inhibition. Mol. Psychiatry 27, 5124–5134. doi: 10.1038/s41380-022-01747-9
Lim, L., Mi, D., Llorca, A., and Marín, O. (2018). Development and functional diversification of cortical interneurons. Neuron 100, 294–313.
Loup, F., Weinmann, O., Yonekawa, Y., Aguzzi, A., Wieser, H. G., and Fritschy, J. M. (1998). A highly sensitive immunofluorescence procedure for analyzing the subcellular distribution of GABAA receptor subunits in the human brain. J. Histochem. Cytochem. 46, 1129–1139. doi: 10.1177/002215549804601005
Lund, J. S., and Lewis, D. A. (1993). Local circuit neurons of developing and mature macaque prefrontal cortex: Golgi and immunocytochemical characteristics. J. Comp. Neurol. 328, 282–312. doi: 10.1002/cne.903280209
Luscher, B., Fuchs, T., and Kilpatrick, C. L. (2011). GABAA receptor trafficking-mediated plasticity of inhibitory synapses. Neuron 70, 385–409. doi: 10.1016/j.neuron.2011.03.024
Maksymetz, J., Byun, N. E., Luessen, D. J., Li, B., Barry, R. L., Gore, J. C., et al. (2021). mGlu(1) potentiation enhances prelimbic somatostatin interneuron activity to rescue schizophrenia-like physiological and cognitive deficits. Cell Rep. 37:109950. doi: 10.1016/j.celrep.2021.109950
Marvin, J. S., Shimoda, Y., Magloire, V., Leite, M., Kawashima, T., Jensen, T. P., et al. (2019). A genetically encoded fluorescent sensor for in vivo imaging of GABA. Nat. Methods 16, 763–770.
McClintock, S. M., Freitas, C., Oberman, L., Lisanby, S. H., and Pascual-Leone, A. (2011). Transcranial magnetic stimulation: A neuroscientific probe of cortical function in schizophrenia. Biol. Psychiatry 70, 19–27. doi: 10.1016/j.biopsych.2011.02.031
McGrath, J., Saha, S., Chant, D., and Welham, J. (2008). Schizophrenia: A concise overview of incidence, prevalence, and mortality. Epidemiol. Rev. 30, 67–76. doi: 10.1093/epirev/mxn001
Melchitzky, D. S., and Lewis, D. A. (2003). Pyramidal neuron local axon terminals in monkey prefrontal cortex: Differential targeting of subclasses of GABA neurons. Cereb. Cortex 13, 452–460. doi: 10.1093/cercor/13.5.452
Melchitzky, D. S., and Lewis, D. A. (2008). Dendritic-targeting GABA neurons in monkey prefrontal cortex: Comparison of somatostatin- and calretinin-immunoreactive axon terminals. Synapse 62, 456–465. doi: 10.1002/syn.20514
Melchitzky, D. S., Sesack, S. R., and Lewis, D. A. (1999). Parvalbumin-immunoreactive axon terminals in macaque monkey and human prefrontal cortex: Laminar, regional, and target specificity of type I and type II synapses. J. Comp. Neurol. 408, 11–22.
Mellios, N., Huang, H. S., Baker, S. P., Galdzicka, M., Ginns, E., and Akbarian, S. (2009). Molecular determinants of dysregulated GABAergic gene expression in the prefrontal cortex of subjects with schizophrenia. Biol. Psychiatry 65, 1006–1014.
Michalareas, G., Vezoli, J., Van Pelt, S., Schoffelen, J. M., Kennedy, H., and Fries, P. (2016). Alpha-beta and gamma rhythms subserve feedback and feedforward influences among human visual cortical areas. Neuron 89, 384–397. doi: 10.1016/j.neuron.2015.12.018
Minzenberg, M. J., Laird, A. R., Thelen, S., Carter, C. S., and Glahn, D. C. (2009). Meta-analysis of 41 functional neuroimaging studies of executive function in schizophrenia. Arch. Gen. Psychiatry 66, 811–822.
Mirnics, K., Middleton, F. A., Marquez, A., Lewis, D. A., and Levitt, P. (2000). Molecular characterization of schizophrenia viewed by microarray analysis of gene expression in prefrontal cortex. Neuron 28, 53–67. doi: 10.1016/s0896-6273(00)00085-4
Morris, H. M., Hashimoto, T., and Lewis, D. A. (2008). Alterations in somatostatin mRNA expression in the dorsolateral prefrontal cortex of subjects with schizophrenia or schizoaffective disorder. Cereb. Cortex 18, 1575–1587.
Morris, H. M., Stopczynski, R. E., and Lewis, D. A. (2009). NPY mRNA expression in the prefrontal cortex: Selective reduction in the superficial white matter of subjects with schizoaffective disorder. Schizophr. Res. 115, 261–269. doi: 10.1016/j.schres.2009.09.014
Murray, J. D., and Anticevic, A. (2017). Toward understanding thalamocortical dysfunction in schizophrenia through computational models of neural circuit dynamics. Schizophr. Res. 180, 70–77. doi: 10.1016/j.schres.2016.10.021
Nguyen, R., Morrissey, M. D., Mahadevan, V., Cajanding, J. D., Woodin, M. A., Yeomans, J. S., et al. (2014). Parvalbumin and GAD65 interneuron inhibition in the ventral hippocampus induces distinct behavioral deficits relevant to schizophrenia. J. Neurosci. 34, 14948–14960. doi: 10.1523/JNEUROSCI.2204-14.2014
Niendam, T. A., Laird, A. R., Ray, K. L., Dean, Y. M., Glahn, D. C., and Carter, C. S. (2012). Meta-analytic evidence for a superordinate cognitive control network subserving diverse executive functions. Cogn. Affect. Behav. Neurosci. 12, 241–268. doi: 10.3758/s13415-011-0083-5
Nusser, Z., Sieghart, W., Benke, D., Fritschy, J. M., and Somogyi, P. (1996). Differential synaptic localization of two major gamma-aminobutyric acid type A receptor alpha subunits on hippocampal pyramidal cells. Proc. Natl. Acad. Sci. U.S.A. 93, 11939–11944. doi: 10.1073/pnas.93.21.11939
Oeth, K. M., and Lewis, D. A. (1993). Postnatal development of the cholecystokinin innervation of monkey prefrontal cortex. J. Comp. Neurol. 336, 400–418. doi: 10.1002/cne.903360307
Oh, D. H., Son, H., Hwang, S., and Kim, S. H. (2012). Neuropathological abnormalities of astrocytes, GABAergic neurons, and pyramidal neurons in the dorsolateral prefrontal cortices of patients with major depressive disorder. Eur. Neuropsychopharmacol. 22, 330–338. doi: 10.1016/j.euroneuro.2011.09.001
Pakkenberg, B., Scheel-Krüger, J., and Kristiansen, L. V. (2009). Schizophrenia; From structure to function with special focus on the mediodorsal thalamic prefrontal loop. Acta Psychiatr. Scand. 120, 345–354. doi: 10.1111/j.1600-0447.2009.01447.x
Patel, A. B., De Graaf, R. A., Martin, D. L., Battaglioli, G., and Behar, K. L. (2006). Evidence that GAD65 mediates increased GABA synthesis during intense neuronal activity in vivo. J. Neurochem. 97, 385–396. doi: 10.1111/j.1471-4159.2006.03741.x
Perry, E. K., Tomlinson, B. E., Blessed, G., Perry, R. H., Cross, A. J., and Crow, T. J. (1981). Neuropathological and biochemical observations on the noradrenergic system in Alzheimer’s disease. J. Neurol. Sci. 51, 279–287.
Pfeffer, C. K., Xue, M., He, M., Huang, Z. J., and Scanziani, M. (2013). Inhibition of inhibition in visual cortex: The logic of connections between molecularly distinct interneurons. Nat. Neurosci. 16, 1068–1076. doi: 10.1038/nn.3446
Pi, H. J., Hangya, B., Kvitsiani, D., Sanders, J. I., Huang, Z. J., and Kepecs, A. (2013). Cortical interneurons that specialize in disinhibitory control. Nature 503, 521–524.
Pierri, J. N., Chaudry, A. S., Woo, T. U., and Lewis, D. A. (1999). Decreased GAT-1 -immunoreactive chandelier cell axon terminals in the prefrontal cortex in schizophrenia: Diagnostic and laminar specificity. Schizophr. Res. 36:83.
Popescu, A. T., Popa, D., and Paré, D. (2009). Coherent gamma oscillations couple the amygdala and striatum during learning. Nat. Neurosci. 12, 801–807.
Preuss, T. M., and Goldman-Rakic, P. S. (1987). Crossed corticothalamic and thalamocortical connections of macaque prefrontal cortex. J. Comp. Neurol. 257, 269–281. doi: 10.1002/cne.902570211
Rajkowska, G., O’dwyer, G., Teleki, Z., Stockmeier, C. A., and Miguel-Hidalgo, J. J. (2007). GABAergic neurons immunoreactive for calcium binding proteins are reduced in the prefrontal cortex in major depression. Neuropsychopharmacology 32, 471–482.
Reichenberg, A., Harvey, P. D., Bowie, C. R., Mojtabai, R., Rabinowitz, J., Heaton, R. K., et al. (2009). Neuropsychological function and dysfunction in schizophrenia and psychotic affective disorders. Schizophr. Bull. 35, 1022–1029.
Reynolds, G. P., and Beasley, C. L. (2001). GABAergic neuronal subtypes in the human frontal cortex – development and deficits in schizophrenia. J. Chem. Neuroanat. 22, 95–100.
Reynolds, G. P., Beasley, C. L., and Zhang, Z. J. (2002). Understanding the neurotransmitter pathology of schizophrenia: Selective deficits of subtypes of cortical GABAergic neurons. J. Neural Transm. 109, 881–889. doi: 10.1007/s007020200072
Reynolds, G. P., Zhang, Z. J., and Beasley, C. L. (2001). Neurochemical correlates of cortical GABAergic deficits in schizophrenia: Selective losses of calcium binding protein immunoreactivity. Brain Res. Bull. 55, 579–584. doi: 10.1016/s0361-9230(01)00526-3
Ripke, S., Sanders, A. R., Kendler, K. S., Levinson, D. F., Sklar, P., Holmans, P. A., et al. (2011). Genome-wide association study identifies five new schizophrenia loci. Nat. Genet. 43, 969–976.
Rocco, B. R., Dedionisio, A. M., Lewis, D. A., and Fish, K. N. (2017). Alterations in a unique class of cortical chandelier cell axon cartridges in schizophrenia. Biol. Psychiatry 82, 40–48. doi: 10.1016/j.biopsych.2016.09.018
Rocco, B. R., Lewis, D. A., and Fish, K. N. (2016a). Markedly lower glutamic acid decarboxylase 67 protein levels in a subset of boutons in schizophrenia. Biol. Psychiatry 79, 1006–1015. doi: 10.1016/j.biopsych.2015.07.022
Rocco, B. R., Sweet, R. A., Lewis, D. A., and Fish, K. N. (2016b). GABA-synthesizing enzymes in calbindin and calretinin neurons in monkey prefrontal cortex. Cereb. Cortex 26, 2191–2204. doi: 10.1093/cercor/bhv051
Rogasch, N. C., Daskalakis, Z. J., and Fitzgerald, P. B. (2015). Cortical inhibition of distinct mechanisms in the dorsolateral prefrontal cortex is related to working memory performance: A TMS-EEG study. Cortex 64, 68–77. doi: 10.1016/j.cortex.2014.10.003
Rudy, B., Fishell, G., Lee, S., and Hjerling-Leffler, J. (2011). Three groups of interneurons account for nearly 100% of neocortical GABAergic neurons. Dev. Neurobiol. 71, 45–61. doi: 10.1002/dneu.20853
Sakai, T., Oshima, A., Nozaki, Y., Ida, I., Haga, C., Akiyama, H., et al. (2008). Changes in density of calcium-binding-protein-immunoreactive GABAergic neurons in prefrontal cortex in schizophrenia and bipolar disorder. Neuropathology 28, 143–150. doi: 10.1111/j.1440-1789.2007.00867.x
Schmidt, R., Herrojo Ruiz, M., Kilavik, B. E., Lundqvist, M., Starr, P. A., and Aron, A. R. (2019). Beta oscillations in working memory, executive control of movement and thought, and sensorimotor function. J. Neurosci. 39, 8231–8238. doi: 10.1523/JNEUROSCI.1163-19.2019
Schmitt, L. I., Wimmer, R. D., Nakajima, M., Happ, M., Mofakham, S., and Halassa, M. M. (2017). Thalamic amplification of cortical connectivity sustains attentional control. Nature 545, 219–223.
Schoonover, K. E., Dienel, S. J., and Lewis, D. A. (2020). Prefrontal cortical alterations of glutamate and GABA neurotransmission in schizophrenia: Insights for rational biomarker development. Biomark. Neuropsychiatry 3:100015. doi: 10.1016/j.bionps.2020.100015
Schoonover, K. E., Dienel, S. J., Holly Bazmi, H., Enwright, J. F. III, and Lewis, D. A. (2024). Altered excitatory and inhibitory ionotropic receptor subunit expression in the cortical visuospatial working memory network in schizophrenia. Neuropsychopharmacology 49, 1183–1192. doi: 10.1038/s41386-024-01854-x
Sibille, E., Morris, H. M., Kota, R. S., and Lewis, D. A. (2011). GABA-related transcripts in the dorsolateral prefrontal cortex in mood disorders. Int. J. Neuropsychopharmacol. 14, 721–734. doi: 10.1017/S1461145710001616
Silberberg, G., and Markram, H. (2007). Disynaptic inhibition between neocortical pyramidal cells mediated by Martinotti cells. Neuron 53, 735–746.
Smiley, J. F., Hackett, T. A., Bleiwas, C., Petkova, E., Stankov, A., Mann, J. J., et al. (2016). Reduced GABA neuron density in auditory cerebral cortex of subjects with major depressive disorder. J. Chem. Neuroanat. 76, 108–121. doi: 10.1016/j.jchemneu.2015.10.008
Smith, M. R., Readhead, B., Dudley, J. T., and Morishita, H. (2019). Critical period plasticity-related transcriptional aberrations in schizophrenia and bipolar disorder. Schizophr. Res. 207, 12–21. doi: 10.1016/j.schres.2018.10.021
Sohal, V. S., Zhang, F., Yizhar, O., and Deisseroth, K. (2009). Parvalbumin neurons and gamma rhythms enhance cortical circuit performance. Nature 459, 698–702. doi: 10.1038/nature07991
Somogyi, P. (1977). A specific axo-axonal interneuron in the visual cortex of the rat. Brain Res. 136, 345–350. doi: 10.1016/0006-8993(77)90808-3
Straub, R. E., Lipska, B. K., Egan, M. F., Goldberg, T. E., Callicott, J. H., Mayhew, M. B., et al. (2007). Allelic variation in GAD1 (GAD67) is associated with schizophrenia and influences cortical function and gene expression. Mol. Psychiatry 12, 854–869. doi: 10.1038/sj.mp.4001988
Suarez-Sola, M. L., Gonzalez-Delgado, F. J., Pueyo-Morlans, M., Medina-Bolivar, O. C., Hernandez-Acosta, N. C., Gonzalez-Gomez, M., et al. (2009). Neurons in the white matter of the adult human neocortex. Front. Neuroanat. 3:7. doi: 10.3389/neuro.05.007.2009
Tamamaki, N., and Tomioka, R. (2010). Long-range GABAergic connections distributed throughout the neocortex and their possible function. Front. Neurosci. 4:202. doi: 10.3389/fnins.2010.00202
Tao, R., Davis, K. N., Li, C., Shin, J. H., Gao, Y., Jaffe, A. E., et al. (2018). GAD1 alternative transcripts and DNA methylation in human prefrontal cortex and hippocampus in brain development, schizophrenia. Mol. Psychiatry 23, 1496–1505. doi: 10.1038/mp.2017.105
Tomioka, R., and Rockland, K. S. (2007). Long-distance corticocortical GABAergic neurons in the adult monkey white and gray matter. J. Comp. Neurol. 505, 526–538. doi: 10.1002/cne.21504
Tomioka, R., Okamoto, K., Furuta, T., Fujiyama, F., Iwasato, T., Yanagawa, Y., et al. (2005). Demonstration of long-range GABAergic connections distributed throughout the mouse neocortex. Eur. J. Neurosci. 21, 1587–1600. doi: 10.1111/j.1460-9568.2005.03989.x
Tomioka, R., Sakimura, K., and Yanagawa, Y. (2015). Corticofugal GABAergic projection neurons in the mouse frontal cortex. Front. Neuroanat. 9:133. doi: 10.3389/fnana.2015.00133
Tooney, P. A., and Chahl, L. A. (2004). Neurons expressing calcium-binding proteins in the prefrontal cortex in schizophrenia. Prog. Neuropsychopharmacol. Biol. Psychiatry 28, 273–278.
Torres-Gomez, S., Blonde, J. D., Mendoza-Halliday, D., Kuebler, E., Everest, M., Wang, X. J., et al. (2020). Changes in the proportion of inhibitory interneuron types from sensory to executive areas of the primate neocortex: Implications for the origins of working memory representations. Cereb. Cortex 30, 4544–4562. doi: 10.1093/cercor/bhaa056
Traub, R. D., Whittington, M. A., Colling, S. B., Buzsaki, G., and Jefferys, J. G. (1996). Analysis of gamma rhythms in the rat hippocampus in vitro and in vivo. J. Physiol. 493, 471–484.
Tremblay, R., Lee, S., and Rudy, B. (2016). GABAergic interneurons in the neocortex: From cellular properties to circuits. Neuron 91, 260–292.
Tsubomoto, M., Kawabata, R., Zhu, X., Minabe, Y., Chen, K., Lewis, D. A., et al. (2019). Expression of transcripts selective for GABA neuron subpopulations across the cortical visuospatial working memory network in the healthy state and schizophrenia. Cereb. Cortex 29, 3540–3550. doi: 10.1093/cercor/bhy227
Tu, P. C., Lee, Y. C., Chen, Y. S., Hsu, J. W., Li, C. T., and Su, T. P. (2015). Network-specific cortico-thalamic dysconnection in schizophrenia revealed by intrinsic functional connectivity analyses. Schizophr. Res. 166, 137–143. doi: 10.1016/j.schres.2015.05.023
Uehara, T., Sumiyoshi, T., and Kurachi, M. (2015). New pharmacotherapy targeting cognitive dysfunction of schizophrenia via modulation of GABA neuronal function. Curr. Neuropharmacol. 13, 793–801. doi: 10.2174/1570159x13666151009120153
Uhlhaas, P. J., and Singer, W. (2010). Abnormal neural oscillations and synchrony in schizophrenia. Nat. Rev. Neurosci. 11, 100–113.
Van der Werf, Y. D., Scheltens, P., Lindeboom, J., Witter, M. P., Uylings, H. B., and Jolles, J. (2003). Deficits of memory, executive functioning and attention following infarction in the thalamus; a study of 22 cases with localised lesions. Neuropsychologia 41, 1330–1344. doi: 10.1016/s0028-3932(03)00059-9
Van Snellenberg, J. X., Torres, I. J., and Thornton, A. E. (2006). Functional neuroimaging of working memory in schizophrenia: Task performance as a moderating variable. Neuropsychology 20, 497–510.
Varela, F., Lachaux, J. P., Rodriguez, E., and Martinerie, J. (2001). The brainweb: Phase synchronization and large-scale integration. Nat. Rev. Neurosci. 2, 229–239. doi: 10.1038/35067550
Vawter, M. P., Crook, J. M., Hyde, T. M., Kleinman, J. E., Weinberger, D. R., Becker, K. G., et al. (2002). Microarray analysis of gene expression in the prefrontal cortex in schizophrenia: A preliminary study. Schizophr. Res. 58, 11–20.
Volk, D. W., Austin, M. C., Pierri, J. N., Sampson, A. R., and Lewis, D. A. (2000). Decreased glutamic acid decarboxylase67 messenger RNA expression in a subset of prefrontal cortical gamma-aminobutyric acid neurons in subjects with schizophrenia. Arch. Gen. Psychiatry 57, 237–245. doi: 10.1001/archpsyc.57.3.237
Volk, D. W., Matsubara, T., Li, S., Sengupta, E. J., Georgiev, D., Minabe, Y., et al. (2012). Deficits in transcriptional regulators of cortical parvalbumin neurons in schizophrenia. Am. J. Psychiatry 169, 1082–1091.
Volk, D. W., Sampson, A. R., Zhang, Y., Edelson, J. R., and Lewis, D. A. (2016). Cortical GABA markers identify a molecular subtype of psychotic and bipolar disorders. Psychol. Med. 46, 2501–2512. doi: 10.1017/S0033291716001446
Wang, H. L., Rau, C. L., Li, Y. M., Chen, Y. P., and Yu, R. (2015). Disrupted thalamic resting-state functional networks in schizophrenia. Front. Behav. Neurosci. 9:45. doi: 10.3389/fnbeh.2015.00045
Wang, M., Yang, Y., Wang, C. J., Gamo, N. J., Jin, L. E., Mazer, J. A., et al. (2013). NMDA receptors subserve persistent neuronal firing during working memory in dorsolateral prefrontal cortex. Neuron 77, 736–749. doi: 10.1016/j.neuron.2012.12.032
Watanabe, Y., and Funahashi, S. (2012). Thalamic mediodorsal nucleus and working memory. Neurosci. Biobehav. Rev. 36, 134–142.
Weinberger, D. R., Berman, K. F., and Zec, R. F. (1986). Physiologic dysfunction of dorsolateral prefrontal cortex in schizophrenia. I. Regional cerebral blood flow evidence. Arch. Gen. Psychiatry 43, 114–124.
Welsh, R. C., Chen, A. C., and Taylor, S. F. (2010). Low-frequency BOLD fluctuations demonstrate altered thalamocortical connectivity in schizophrenia. Schizophr. Bull. 36, 713–722. doi: 10.1093/schbul/sbn145
Williams, S. M., Goldman-Rakic, P. S., and Leranth, C. (1992). The synaptology of parvalbumin-immunoreactive neurons in the primate prefrontal cortex. J. Comp. Neurol. 320, 353–369. doi: 10.1002/cne.903200307
Woo, T. U., Miller, J. L., and Lewis, D. A. (1997). Schizophrenia and the parvalbumin-containing class of cortical local circuit neurons. Am. J. Psychiatry 154, 1013–1015.
Woo, T. U., Shrestha, K., Lamb, D., Minns, M. M., and Benes, F. M. (2008). N-methyl-D-aspartate receptor and calbindin-containing neurons in the anterior cingulate cortex in schizophrenia and bipolar disorder. Biol. Psychiatry 64, 803–809. doi: 10.1016/j.biopsych.2008.04.034
Woo, T. U., Whitehead, R. E., Melchitzky, D. S., and Lewis, D. A. (1998). A subclass of prefrontal gamma-aminobutyric acid axon terminals are selectively altered in schizophrenia. Proc. Natl. Acad. Sci. U.S.A. 95, 5341–5346.
Woodward, N. D., and Heckers, S. (2016). Mapping thalamocortical functional connectivity in chronic and early stages of psychotic disorders. Biol. Psychiatry 79, 1016–1025. doi: 10.1016/j.biopsych.2015.06.026
Woodward, N. D., Karbasforoushan, H., and Heckers, S. (2012). Thalamocortical dysconnectivity in schizophrenia. Am. J. Psychiatry 169, 1092–1099.
Wulff, P., Ponomarenko, A. A., Bartos, M., Korotkova, T. M., Fuchs, E. C., Bähner, F., et al. (2009). Hippocampal theta rhythm and its coupling with gamma oscillations require fast inhibition onto parvalbumin-positive interneurons. Proc. Natl. Acad. Sci. U.S.A. 106, 3561–3566. doi: 10.1073/pnas.0813176106
Xavier, G., Su Ting, A., and Fauzan, N. (2020). Exploratory study of brain waves and corresponding brain regions of fatigue on-call doctors using quantitative electroencephalogram. J. Occup. Health 62:e12121. doi: 10.1002/1348-9585.12121
Yin, H., Pantazatos, S. P., Galfalvy, H., Huang, Y. Y., Rosoklija, G. B., Dwork, A. J., et al. (2016). A pilot integrative genomics study of GABA and glutamate neurotransmitter systems in suicide, suicidal behavior, and major depressive disorder. Am. J. Med. Genet. B Neuropsychiatr. Genet. 171B, 414–426. doi: 10.1002/ajmg.b.32423
Yoon, D. Y., Mansukhani, N. A., Stubbs, V. C., Helenowski, I. B., Woodruff, T. K., and Kibbe, M. R. (2014). Sex bias exists in basic science and translational surgical research. Surgery 156, 508–516.
Zaitsev, A. V., Povysheva, N. V., Gonzalez-Burgos, G., Rotaru, D., Fish, K. N., Krimer, L. S., et al. (2009). Interneuron diversity in layers 2-3 of monkey prefrontal cortex. Cereb. Cortex 19, 1597–1615. doi: 10.1093/cercor/bhn198
Zhao, J., Bao, A. M., Qi, X. R., Kamphuis, W., Luchetti, S., Lou, J. S., et al. (2012). Gene expression of GABA and glutamate pathway markers in the prefrontal cortex of non-suicidal elderly depressed patients. J. Affect. Disord. 138, 494–502. doi: 10.1016/j.jad.2012.01.013
Keywords: bipolar disorder, gamma oscillations, interneuron, major depressive disorder, working memory, sex differences
Citation: Hughes H, Brady LJ and Schoonover KE (2024) GABAergic dysfunction in postmortem dorsolateral prefrontal cortex: implications for cognitive deficits in schizophrenia and affective disorders. Front. Cell. Neurosci. 18:1440834. doi: 10.3389/fncel.2024.1440834
Received: 30 May 2024; Accepted: 05 September 2024;
Published: 24 September 2024.
Edited by:
Claire-Marie Vacher, Columbia University, United StatesReviewed by:
Joanna Urban-Ciecko, Polish Academy of Sciences, PolandMengyu Tu, Johns Hopkins University, United States
Copyright © 2024 Hughes, Brady and Schoonover. This is an open-access article distributed under the terms of the Creative Commons Attribution License (CC BY). The use, distribution or reproduction in other forums is permitted, provided the original author(s) and the copyright owner(s) are credited and that the original publication in this journal is cited, in accordance with accepted academic practice. No use, distribution or reproduction is permitted which does not comply with these terms.
*Correspondence: Kirsten E. Schoonover, a3NjaG9vbm92ZXJAdWFibWMuZWR1