- 1Department of Pharmacy, University of Naples Federico II, Naples, Italy
- 2Task Force on Microbiome Studies, University of Naples Federico II, Naples, Italy
The Aryl hydrocarbon receptor (AHR) is a cytosolic receptor and ligand-activated transcription factor widely expressed across various cell types in the body. Its signaling is vital for host responses at barrier sites, regulating epithelial renewal, barrier integrity, and the activities of several types of immune cells. This makes AHR essential for various cellular responses during aging, especially those governing inflammation and immunity. In this review, we provided an overview of the mechanisms by which the AHR mediates inflammatory response at gut and brain level through signals from intestinal microbes. The age-related reduction of gut microbiota functions is perceived as a trigger of aberrant immune responses linking gut and brain inflammation to neurodegeneration. Thus, we explored gut microbiome impact on the nature and availability of AHR ligands and outcomes for several signaling pathways involved in neurodegenerative diseases and age-associated decline of brain functions, with an insight on Parkinson’s and Alzheimer’s diseases, the most common neurodegenerative diseases in the elderly. Specifically, we focused on microbial tryptophan catabolism responsible for the production of several AHR ligands. Perspectives for the development of microbiota-based interventions targeting AHR activity are presented for a healthy aging.
1 Introduction
The Aryl hydrocarbon receptor (AHR) has been identified as a crucial point of convergence among various cellular signaling pathways implicated in aging, as those governing inflammation and immunity in both the gut and the brain, as well as cell proliferation and differentiation. This is especially relevant in the context of inflammaging and neurodegenerative diseases. There is a growing recognition of the gut microbiota’s role in initiating chronic inflammation by disturbing intestinal balance. Numerous cross-sectional studies have highlighted alterations in microbiota composition among patients with neurodegenerative conditions such as Alzheimer’s (AD) and Parkinson’s diseases (PD), compared to healthy individuals. AD is a multifactorial disease where neuroinflammation, accumulation of beta-amyloid (Aβ) plaque, and neurofibrillary tau tangles in the brain are the causative agents of the progressive loss of memory, language, and cognitive ability in affected individuals (Alzheimer’s Association, 2024). In PD, the progressive loss of neurons in the substantia nigra pars compacta results in dopamine depletion in the dorsal aspect of the putamen, a part of the basal ganglia. This depletion gives rise to the most prominent signs and symptoms of PD, including unintended or uncontrollable movements, such as shaking, stiffness, and difficulties with balance and coordination (Simon et al., 2020). The specific mechanisms and molecular pathways linking gut microbiota alteration and neurodegenerative diseases still remain in their infancy in terms of comprehensive understanding. The AHR has been frequently implicated as a target and mediator of metabolites derived from commensal microbes, in particular those resulting from tryptophan (trp) catabolism (Barroso et al., 2021). Recent research studies evidence that the AHR might serve as a crucial link between dysfunctional microbiota and neurodegeneration both through its direct activation in CNS resident cells or by AHR contribution to peripheral modulation of immune cells and inflammatory responses.
The recognition of AHR pathways modulation upon microbial stimulation and its impact on cellular and tissue balance in processes encompassing neurodegenerative phenotype development may orientate the identification of effective and innovative microbiota-based interventions in the treatment of neurodegenerative diseases.
2 AHR in the regulation of immune/inflammatory response in gut and brain
The gut-brain axis has emerged as a critical area of study, shedding light on the intricate bidirectional communication between the gastrointestinal system and the central nervous system (CNS). This interaction is considered a key factor in the pathogenesis and progression of several neurological disorders, including neurodegenerative diseases. Within this context, the AHR has garnered significant attention due to its pivotal role in modulating immune responses, barrier function, and neurotransmitter production. CNS-resident cells harbor the AHR; immune and inflammatory pathways, triggered peripherally by AHR activation, can travel to the brain and affect its physiology. Here, we will describe the involvement of AHR in immune/inflammatory response along this axis.
The AHR is a cytosolic receptor and a ligand-activated transcription factor widely expressed by different cell types throughout the body. AHR signaling is considered a key component of the immune response at barrier sites, regulating epithelial renewal, barrier integrity, but also the activities of many immune cell types, as intraepithelial lymphocytes (IELs), T helper 17 cells (Th17), macrophages and others (Lamas et al., 2018). All these functions make AHR crucial for intestinal homeostasis, where it modulates physiological processes in response to environmental toxins (i.e., 2,3,7,8-tetrachlorodibenzo-p-dioxin and polycyclic aromatic hydrocarbon compounds), endogenous ligands (i.e., 6-formylindolo[3,2-b] carbazole and leukotrienes), and many trp metabolites including those processed by gut microorganisms (Safe et al., 2018).
AHR is present in the cytoplasm in a transcriptionally inactive state highly affine to its ligands, forming a complex with two heat shock protein 90 (HSP90), the X-associated protein 2 (XAP2), the cochaperone p23 and the protein kinase SRC. Upon ligand binding, AHR is activated and translocates to the nucleus where dimerizes with the AHR nuclear translocator (ARNT) forming a functional DNA-binding transcription factor (Figure 1). This heterodimer interacts with specific DNA sequences (dioxin/xenobiotic response element - DRE/XRE) in the regulation site of AHR-responsive genes leading, among the other events, to the degradation of AHR ligands (Figure 1). By interacting with other transcriptional factors and co-activators, AHR can also impact the transcription of genes that do not harbor DRE/XRE consensus sequences (recently reviewed by Sondermann et al., 2023). Additionally, AHR is also involved in epigenetic mechanisms, e.g., by controlling histone acetylation and methylation, long non-coding RNA, or microRNAs (Schnekenburger et al., 2007; Chang et al., 2014; Liu et al., 2018; Figure 1).
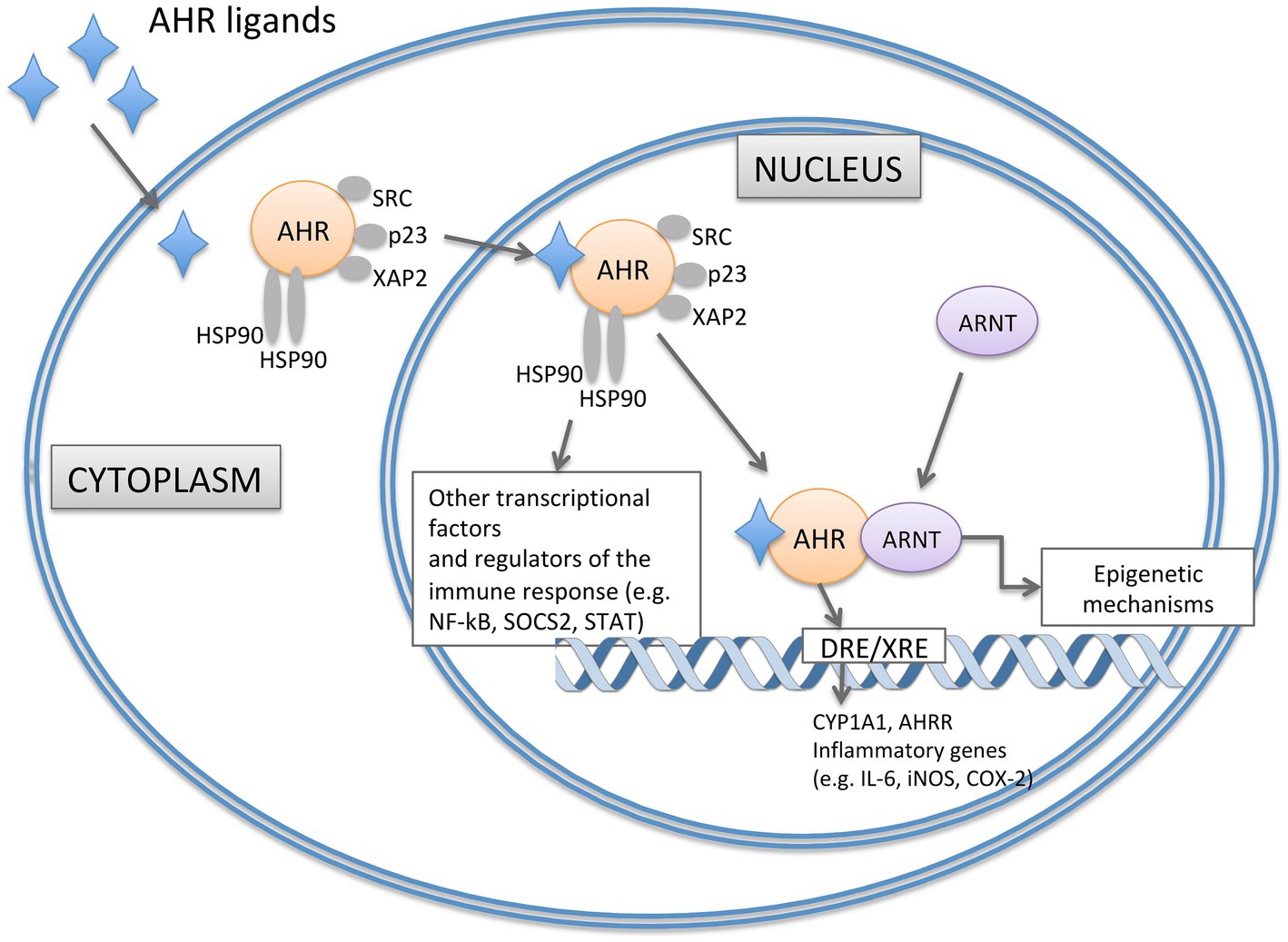
Figure 1. Representation of signaling pathways of AHR. In the absence of ligand, AHR is present in the cytoplasm in an inactive state forming a complex with two HSP90, XAP2, the cochaperone p23 and the protein kinase SRC. Upon ligand binding, AHR translocases to the nucleus and dimerizes with ARNT. The heterodimer interacts with specific DNA regions containing DRE sequences in the regulation site of AHR-responsive genes (such as CYP1A1, AHRR, IL-6, iNOS and COX-2). AHR can also impact the transcription of genes that do not harbour DRE/XRE consensus sequences by epigenetic mechanisms and the interaction with other transcriptional factors (such as NF-kB). AHRR, AHR Repressor; ARNT, AHR Nuclear Translocator; COX-2, Cyclooxygenase-2; CYP1A1, Cytochrome P450 1A1; DRE/XRE, Dioxin/Xenobiotic response element; HSP90, Heat Shock Protein 90; iNOS, Inducible Nitric Oxide Synthase; NF-κB, Nuclear Factor-κB; SOCS2, Suppressor of Cytokine Signaling 2; STAT, Signal Transducer and activator of Transcripton; XAP2, X-Associate Protein 2.
Various genes associated with inflammatory responses contain multiple DREs in their upstream sequences (Figure 1). Notable examples include the inflammatory mediators IL-6, the inducible nitric oxide synthase and cyclooxygenase-2 (Hollingshead et al., 2008; Lee Y. et al., 2015). Finally, AHR also interacts with the nuclear factorκB (NFκB) that is involved in the expression of pro-inflammatory and cell survival genes (Figure 1). AHR regulates NFκB signaling directly by interacting with RELA, RELB and other members of the NFκB pathway and indirectly through suppressor of cytokine signaling 2 (SOCS2)dependent mechanisms. Similarly, an intricate crosstalk of reciprocal control has been reported between AHR and the signal transducer and activator of transcription (STAT), crucially involved in the immunoregulation (Sondermann et al., 2023). Thus, AHR can switch from the pro-inflammatory to the anti-inflammatory activity playing different roles in the regulation of immune responses that are extremely important at epithelial barriers, such as the intestinal epithelial barrier (Esser and Rannug, 2015). In the context of the gastrointestinal tract, AHR is expressed in immune, epithelial, endothelial, and neuronal cells. Here, its role is to influence different aspects of intestinal barrier function, such as the intestinal epithelial cells (IECs) renewal and turnover, development, function and maintenance of mucosal immune system, and colonic peristalsis. Mice with genetic deletion of AHR show impaired proliferation of colonic crypt stem cells (Han et al., 2020); the specific deficiency of AHR at the intestinal epithelium results in enhanced apoptosis of epithelial cells in a mouse model of Dextran Sodium Sulfate (DSS)-induced intestinal inflammation (Chinen et al., 2015). The influence on intestinal epithelium can also derive from the expression and activation of AHR in immune cells residing in the gut, impacting on their ability to maintain barrier function and protection against infective insults. Different intestinal cells of the innate and adaptive immune response, such as IELs, Th17 cells, innate lymphoid cells (ILCs), macrophages, dendritic cells (DCs), and neutrophils, express AHR (Stockinger et al., 2021). Under homeostatic conditions, AHR activation through specific ligands from the diet or microbiota metabolism is crucial for preserving the integrity and functionality of the intestinal barrier. This signaling results in the production of IL-22, the induction of IL-10 receptor expression, reinforcement of tight junctions, and impacts on colonic neurons. IL-22, predominantly produced by type 3 innate lymphoid cells (ILC3s) under steady-state conditions, induces the secretion of antimicrobial peptides (for example, RegIIIbeta and RegIIIgamma) by IECs for barrier protection (Liang et al., 2006). AHR is also highly expressed in intestinal FoxP3+ Treg cells that balance the tissue inflammation by secreting IL-10 and TGF-beta. On contrary, a lack of AHR leads to an imbalance in immune cell populations, an increase in pro-inflammatory cytokines [tumor necrosis factor (TNF), IL-6, IL-17, and interferon-gamma (IFNγ)], vascular permeability, reduced mucus layer, and disruption of the barrier, including impaired tight junctions’ expression (Ye et al., 2017; Yu et al., 2018). Recently, AHR expression has also been identified in the colonic enteric nervous system (ENS) where the loss of Ahr results in delayed transit time that can lead to bacterial overgrowth and chronic constipation (Obata et al., 2020).
AHR is also widely expressed in the CNS from several neuronal cell types, including brain microvessels, neurons, astrocytes and microglia, where it modulates their activity during neurodevelopment and neuroinflammation (Juricek and Coumoul, 2018). Microglia and astrocytes play an important role in the control of the inflammation in the CNS by sensing and coordinating the reactions to endogenous and environmental stimuli, including AHR ligands. Thus, AHR can mediate pro-inflammatory and anti-inflammatory effects in the CNS depending on the availability of exogenous AHR ligands (Lee Y. et al., 2015). Noteworthy, deletion of AHR in both astrocytes and microglia is associated with dysregulated pro-inflammatory response and worsening of inflammatory demyelinating disease (Rothhammer et al., 2018). A central role in CNS inflammation is played by the complex interactions between the AHR and NF-κB that impact on the signaling between astrocytes and microglia. In both glial cells the activation of AHR by its ligands limits NF-κB activation in a SOCS2-dependent manner suppressing NF-κB control of glial responses, as demonstrated in a model of experimental autoimmune encephalomyelitis (EAE). Rothhammer et al. demonstrated that, in the EAE mouse model, deletion of AHR in microglia triggered exaggerated inflammatory responses in local astrocytes, thus microglial AHR expression limits pathogenic activities of astrocytes in chronic autoimmune inflammation (Rothhammer et al., 2018).
In summary, AHR plays a pivotal role in maintaining the integrity and function of the gut barrier, regulating immune responses, and modulating neuroinflammation in the brain. Peripheral homeostasis modulated by AHR can affect CNS resident cells and brain inflammatory processes, warning a critical mediator role for AHR in the gut-brain axis.
Altogether the activation of AHR at peripheral and central level upon different signals is a hotspot of modulation of inflammatory response as well as crucial to govern inflammatory route of the bidirectional communication between gut and brain.
3 Age-related microbiota changes and neurodegenerative diseases
Inflammaging is an excessive inflammatory process occurring during aging that significantly influences the development of various age-related diseases. At central level, persistent neuroinflammation can flow into the development of neurodegenerative disorders such as AD and PD (Kempuraj et al., 2015; Bostanciklioğlu, 2018). Several pathophysiological mechanisms have been considered for these neurodegenerative diseases, encompassing genetic, environmental, and endogenous factors that sustain persistent inflammatory responses in the brain, including peripheral inflammation triggered by gut dysbiosis. In fact, a growing body of evidence links the neuroinflammation observed in AD and PD with alteration in gut microbiota composition, suggesting a role for microbial-derived metabolites and host-related responses in the pathogenesis and progression of the diseases.
Changes in the composition of the gut microbiota occur in elderly and are associated with increased inflammation in various tissues and organs including brain tissue (Sarkar and Pitchumoni, 2017; Giau et al., 2018). Hallmarks of aging include genetic instability, cellular senescence, augmented oxidative stress, an imbalance of key messengers (as decline in growth factors), and finally, physiological changes in the gastrointestinal tract. In the intestine, these changes include hypochlorhydria, gastric motility disorders, malabsorption, diarrhea or constipation, degenerative changes in the ENS, all together eliciting dramatic effects on the composition and function of the gut microbiome, whose stability deteriorates in old age (Haran and McCormick, 2021). Many triggering factors as modified diets, use of drugs and reduced physical activity can impact on the gut microbiota of older individuals. A decreased diversity (Biagi et al., 2010), enrichment in pathobionts, and a reduction of bacteria with anti-inflammatory and immunomodulatory properties such as Bifidobacterium, Bacteroides, Lactobacillus, and Akkermansia have been reported (Biagi et al., 2016; Ruiz-Ruiz et al., 2019). From a functional perspective, a link between aging and the reduction of microbial pathways associated with trp, and indole production and metabolism has been proposed. Ruiz-Ruiz et al., by shotgun proteomics identified functional microbiome deficits associated with aging. They found that the synthesis of proteins involved in trp and indole production and the fecal concentrations of trp metabolites were progressively decreased with age (Ruiz-Ruiz et al., 2019). The trp and its metabolites are known to play fundamental roles in health and neuroprotection. Their production and catabolism have been found decreased in patients with a number of disorders, including neurodegenerative diseases, such as AD and PD (Favre et al., 2010; Vujkovic-Cvijin et al., 2013; Sandgren and Brummer, 2018; Platten et al., 2019; Chojnacki et al., 2020; Song et al., 2023).
3.1 Alteration of gut microbiota profiles in Alzheimer’s disease
The increase of specific bacterial species has been associated to AD dementia, including taxa known to cause inflammatory states, such as the gram negative Bacteroides vulgatus (Haran et al., 2019). Haran et al. identified a dysbiotic pattern among AD elders in comparison to those without dementia or with other dementia types. They reported a reduction in species producing short-chain fatty acids (SCFAs), with an increase in species known to have associations with either neurological disorders via inflammation or other colonic inflammatory states (Haran et al., 2019). Lower levels of SCFAs can affect intestinal barrier integrity and permeability allowing the entry of pro-inflammatory bacterial components, like lipopolysaccharide (LPS), into the systemic circulation. LPS, reaching the brain, activates microglia and can promote neuroinflammation and neurodegeneration.
Among the SCFAs, butyrate is the primary microbial metabolite in the gut, serving as a key energy source for colon cells. It binds to cell membrane receptors activating downstream signaling pathways or enters the cells and directly affects gene expression by inhibiting histone deacetylases. In addition to its local effects in the colon, such as maintaining gut barrier integrity and providing anti-inflammatory benefits, butyrate can also influence microbial signaling to the brain through various pathways. Butyrate impacts systemic inflammatory and gastrointestinal endocrine responses, can cross the blood–brain barrier (BBB), and communicates with the brain via vagal afferents (Stilling et al., 2016). By interacting with nearly all systems involved in gut-brain communication, reduced levels of butyrate can likely be linked to AD processes by affecting synaptic plasticity, amyloid-beta and tau pathologies, and neuroinflammation (Qian et al., 2022). Accordingly, the microbiota composition of AD elders is characterized by lower proportions of key butyrate-producing bacteria, such as members of Butyrivibrio (B. hungatei and B. proteoclasticus) and Eubacterium (E. eligens, E. hallii, and E. rectale) genera, as well as Clostridium sp. strain SY8519, Roseburia hominis, and Faecalibacterium prausnitzii. Moreover, decreased levels of Bifidobacterium, Bacteroides, Lachnospira, and Ruminiclostridium_9 and increased abundances of Prevotella have also been observed in patients with AD (Vogt et al., 2017; Guo et al., 2021). Experimental approaches modulating gut microbiota composition are defining functional roles for gut microbial communities in the progression of AD pathology but are also indicative of a possible therapeutic approach to ameliorate AD symptoms. Depletion of gut microbiota both in germ-free and antibiotic-treated transgenic AD mice induces reduction of cerebral Aβ amyloid pathology and neuroinflammation (Minter et al., 2016; Harach et al., 2017); in the ADLPAPT transgenic mouse model of AD, fecal microbiota transplantation from WT mice ameliorated the AD-like pathology in recipient mice, reversing also peripheral abnormalities related to intestinal macrophage activity and circulating blood inflammation (Kim M. S. et al., 2019). Multiple studies involving different AD rodent models have shown that probiotic interventions remodeled gut microbiota profile, SCFAs levels, inflammatory markers, and cognitive functioning (reviewed in de Rijke et al., 2022). Randomized, double blind, controlled trials with subjects diagnosed with AD found significant improvements in cognition after probiotic administration (Akbari et al., 2016; Tamtaji et al., 2019a). Beneficial effects of prebiotic and symbiotic consumption exerting healthy responses on gut microbiota and its metabolic products have also been recorded (Hoffman et al., 2019; Westfall et al., 2019; Wu et al., 2020; Xu et al., 2020; Deng et al., 2021; Gu et al., 2021; Lee et al., 2021; Liu et al., 2021).
3.2 Alteration of gut microbiota profiles in Parkinson’s disease
A pro-inflammatory gut microbiota, rich in gram negative bacteria, source of LPS, and deficient in anti-inflammatory SCFA-producing bacteria has been identified in patients with PD. Although there are conflicting results, the PD-associated microbial profile seems to be characterized by increased relative abundance of Enterobacteriaceae, Akkermansia, Lactobacillus, Bifidobacterium, Clostridium, and Ruminococcaceae, along with decreased abundance of Prevotellaceae, Lachnospiraceae and Faecalibacterium prausnitzii, the latter being a putative SCFA-producing species (Scheperjans et al., 2014; Keshavarzian et al., 2015; Hill-Burns et al., 2017; Aho et al., 2019; Li et al., 2019). On the other hand, probiotics interventions improve gastrointestinal symptoms of PD patients, such as abdominal pain, abdominal distension and constipation together with markers of inflammation and cognitive impairment (Barichella et al., 2016; Georgescu et al., 2016; Borzabadi et al., 2018; Tamtaji et al., 2019b; Tan et al., 2021). Several animal models of PD have also highlighted the important role of gut microbiota in influencing PD symptoms, progression, and treatment success. Bacteria of genus Desulfovibrio induced alpha-synuclein aggregation in the head region of a Caenorhabditis elegans model of PD. Noteworthy, some Desulfovibrio strains, isolated from PD patients, were more competent to induce alpha-synuclein aggregation compared to strains collected from healthy individual (Huynh et al., 2023). Recently, we have demonstrated that antibiotic-induced gut dysbiosis is able to worsen disease symptoms in a mouse model of PD. Reduction of bacterial diversity, of butyrate-producing bacteria and of fecal butyrate levels along with Ruminococcus lactaris increase were the main features of the gut microbiota of these mice. Notably, treatment with butyrate restored gut dysbiosis, gut permeability, positively impacting on motor symptoms and peripheral and central inflammation (Avagliano et al., 2022; Turco et al., 2023).
Collectively these findings, even though mostly based on preclinical and cross-sectional studies, indicate that reconfiguration of gut communities during aging instigate toward elevate peripheral and central inflammation that are associated to the development of neurodegeneration in AD and PD. This picture prompts future investigations on both specific mechanisms and molecular pathways underlying these associations and the consideration of microbiota-targeted approaches for the improvement of clinical outcomes.
4 Gut microbiota-derived tryptophan metabolites as ligands for AHR activation: relevance for gut-brain signaling
In the human body, the gut can accommodate an abundance of AHR ligands some of which are directly processed by commensal microorganisms. As such, there is much interest in the involvement of AHR as an integrator of microbial signals at intestinal and central levels to unveil gut-brain axis perturbation in neurodegenerative diseases. As mentioned above, AHR signaling participates in the gut-brain axis through multiple mechanisms ranging from the direct activation of AHR in CNS resident cells to AHR mediated peripheral modulation of inflammation. In the context of aging and neurodegenerative diseases, AHR ligands produced by gut microorganisms’ trp catabolism are under specific attention.
In the next paragraph, we will analyze the impact of microbiota-derived trp metabolites on gut-brain axis through AHR signaling. Specifically, we will focus our attention on some trp metabolites with specific neuroactive features, whose imbalance or deficiency could be involved in the pathophysiology of aging-related brain diseases in the context of AHR signaling pathways and regulatory functions (Table 1).
Dietary trp can be transformed into different metabolites following three major pathways in the gastrointestinal tract: the biotransformation of trp in indoles by gut microbiota, the kynurenine pathway, and the serotonin production pathway (reviewed in Agus et al., 2018).
4.1 Indole pathway
Through the indole pathway trp is transformed into several molecules, such as indole and its derivatives, many of which are ligands of the AHR able to induce AHR-dependent signaling for modulation of local inflammation or for neuromodulation (Table 1). Indole-producing species are gram negative and positive bacteria (e.g., members of Bacteroides and Clostridium genera, E. coli and Desulfovibrio vulgaris) that express the enzyme tryptophanase; as widely reviewed by Roager and Licht (2018), trp is also transformed by gut commensal in the AHR agonists tryptamine (by Clostridium sporogenes and Ruminococcus gnavus), indolelactic acid (ILA; by Anaerostipes spp., Bacteroides spp., Bifidobacterium spp., Lactobacillus spp. Among the others), indoleacrylic acid (IA; by Clostriudium sporogenes and Peptostreptococcus spp.), indole-3-propionic acid (IPA; by members of Clostriudium and Peptostreptococcus spp.), indole-3-aldehyde (IAld; by Lactobacillus spp.), indoleacetic acid (IAA; by Bacteroides spp., Bifidobacterium spp. and Clostridium spp. among the others), and the 3-methylindole (or skatole; by members of genera Clostriudium, Lactobacillus, Eubacterium, and Bacteroides thetaiotaomicron and Butyrivibrio fibrisolvens species). Commensal bacterialderived metabolites can be further metabolized by the host into other AHR agonists, such as the indoxyl3sulfate (I3S) formed in the liver starting from the bacterial indole (Banoglu and King, 2002). Evidence showed that indole-producers commensal bacteria might maintain the mucosal homeostasis and affect the immune system in the gut, as well as in systemic circulation and distal organs, through the AHR activation (Table 1). Indole derivatives produced by Lactobacillus spp., as ligands of AHR, reprogram naive CD4+ T helper cells into Treg cells (Cervantes-Barragan et al., 2017) and the polarization of Th17 cells (Wilck et al., 2017), keeping the Treg/Th17 balance whose alteration plays an important role in intestinal inflammatory diseases (Kim W. H. et al., 2019; Yan et al., 2020). The immunomodulatory benefits of indole and its derivatives are partly based on the AHR-driven mechanisms in intestinal DCs, IELs, and ILCs (Li et al., 2021) and AHR modulation of the T cell immunity through the alteration of Treg/Th17 cells with Treg dominance (Quintana et al., 2008). Thus, as AHR ligands, indole and some derivatives play a critical role in regulating epithelial integrity and the immune response, including intestinal stem cells (ISCs) and epithelial regeneration, intestinal barrier protection, and antimicrobial defense (Zelante et al., 2013; Cervantes-Barragan et al., 2017; Hendrikx et al., 2018; Hou et al., 2018; Busbee et al., 2020). Indole-3-ethanol, indole-3-pyruvate, and IAld ameliorate morbidity and inflammation of DSS-induced colitis in mice by maintaining the integrity of the apical junctional complex, a major regulator of intestinal permeability (Scott et al., 2020). Moreover, IA was reported to promote barrier function and immune tolerance in DSS-induced colitis mice by inducing the mRNA expression of the AHR target gene Cyp1a1 in the intestinal epithelium and immune cells (Wlodarska et al., 2017). By binding and activating AHR, indoles also promote the expression of IL-10 supporting barrier function (Powell et al., 2020). Maintenance of intestinal homeostasis and an anti-inflammatory state by gut microbes, through indole-dependent AHR activation, beneficially modulates bottom-up players of the gut-brain axis thus affecting brain functionality and behaviour. Gut indoles can also reach the brain, where, interacting with the AHR expressing cells, exert a potent neuroprotective effect (Table 1). For example, indole, I3S, IPA, IAld and tryptamine generated from dietary trp by the different pathways harbored in commensal bacteria can activate AHR signaling in CNS-resident cells, namely astrocytes and microglia, limiting neuroinflammation (Rothhammer et al., 2016, 2018; Dopkins et al., 2021). Recently, Wei et al. showed that microbiota-derived indole elicits also neurogenic effects in the adult mouse hippocampus by AHR activation, being neurogenesis not induced in AHR KO mice (Wei et al., 2021).
4.2 Kinurenine pathway
Dietary trp is mainly metabolized toward the kynurenine pathway (KP) by the host enzymes trp 2,3-dioxygenase (TDO) and indoleamine 2,3-dioxygenase (IDO), expressed in liver and other cells (such as intestinal cells, microglia, astrocytes, macrophages, and neuronal cells). The KP is induced in times of stress and/or immune activation, and it is associated with inflammatory response, cancer, and neurological disorders (AD, amyotrophic lateral sclerosis, Huntington disease, and PD), through the production of different neuroactive metabolites (Guillemin and Brew, 2002; Dehhaghi et al., 2019). This catabolism is mediated in the gut by the rate-limiting enzyme IDO1 and leads to the production of kynurenine, and the downstream products such as quinolonic acid (QA), niacin, nicotinamide adenine dinucleotide, and kynurenic acid (KA). Gut microbes encode enzymes homologous to those of the eukaryotic KP and can also produce kynurenine and downstream metabolites with neurotoxic effects, as the 3-hydroxyanthranilic acid (Vujkovic-Cvijin et al., 2013).
The KP is differently activated in the periphery and CNS. Microbial stimuli (as LPS), cytokines, amyloid peptides, and inflammatory molecules (IFNγ) can induce IDO1 in the gut to control host immunity. Elevated concentrations of kynurenines, as in chronic inflammatory diseases, regulate immune homeostasis by acting as AHR ligands and allow the generation of Treg cells, which protect from chronic hyperinflammatory responses (Romani et al., 2008). This can be protective against pathogens insult, where the activation of Treg cells via AHR prevented the infection and significantly reduced clinical signs of Streptococcus arthritis (Bessede et al., 2014). Moreover, at intestinal level, KA, xanthurenic acid, and cinnabarinic acid can bind the AHR and induce the expression of AHR-dependent genes that promote intestinal homeostasis (Chen et al., 2023; Table 1). Thus, a decreased production of KA can result in gut barrier imbalance and loss of integrity, with augmented local and systemic inflammation.
Within the CNS the KP is differentially compartmentalized within astrocytes and microglia. The trp uptake and metabolism in astrocytes lead to the production of the KA, reported to have neuroprotective properties in the CNS, due to its action as an antagonist at the N-methyl-D-aspartate receptor (NMDAR; Giil et al., 2017). In microglia, metabolism of trp gives rise to metabolites with reactive oxidative properties including 3-hydroxykynurenine, 3-hydroxyanthranilic acid, and QA that act as agonists at the NMDAR and may contribute to excitotoxicity and neurotoxicity (Table 1). The concentrations of QA in the human brain can increase drastically in inflamed tissues (O’Farrell and Harkin, 2017). QA causes neuronal degeneration, destabilizes the cytoskeleton, triggers pro-inflammatory responses and apoptosis of neurons and astrocytes, as well as disrupts the BBB (O’Farrell and Harkin, 2017). All these neurotoxic effects can be implicated in the pathology of neurodegenerative and neuropsychiatric disorders (Dehhaghi et al., 2019). The gut microbiota may indirectly influence the levels of circulating trp and kynurenine metabolism and consequently those KP metabolites that are AHR agonists. Germ-free and antibiotic treated animals, as result of lack of IDO stimulation upon bacterial TLRs activation, present higher serum trp and lower kynurenine levels along with a tendency for anxiety-like behavior and cognitive deficits (Campbell et al., 2014; Desbonnet et al., 2015). Moreover, as mentioned above, gut microbiota can synthesize kynurenine metabolites: some bacteria harbor an enzyme aspartic transaminase which transaminates kynurenine and 3-hydroxykynurenine to KA (Han et al., 2001); recently, Miyamoto and co-authors unraveled a bacterial trp metabolism gene (EC:1.13.11.11) that collaborates with intestinal cells in the biosynthesis of KA. This metabolite resulted a key element in the recruitment of pathogenic macrophages and the subsequent induction of Th17 cells promoting EAE in the CNS (Miyamoto et al., 2023). Other bacterial products influencing KP are several group B vitamins that are cofactors of some enzymes of KP, and the post-biotic butyrate that has been shown to reduce IDO transcription, thus blocking the kynurenine production (Więdłocha et al., 2021). Finally, lower kynurenine concentrations can also result from decreased trp availability associated with bacterial indole metabolism and serotonin synthesis.
4.3 Serotonine pathway
The serotonin (5-hydroxytryptamine, 5-HT) production pathway is the third route of trp metabolism that partially occurs in the brain, while for the 90% in the intestinal enterochromaffin cells via Trp hydroxylase 1 (TpH1) under the control of gut microbiota (Agus et al., 2018.). Gut-derived 5-HT is a key regulator of AHR ligand availability and receptor activation (Table 1). 5-HT is a CYP1A1 substrate that competes with AHR ligands for CYP1A1 degradation. Thus, via the inhibition of the enzymatic clearance of AHR ligands, 5-HT promotes sustained AHR signaling. In accordance, lack in the gut of 5-HT intracellular transport via the plasma membrane 5-HT transporter (SERT) impairs intestinal AHR activation (Manzella et al., 2020). Gut and brain 5-HT levels are correlated to specific members of the gut microbiota (Yano et al., 2015; Pirozzi et al., 2023), thus gut bacteria participate in several physiological functions that depend on 5-HT levels, such as intestinal motility and absorption of nutrients, but also in the underlying neurobiology of different behaviors. Gut microbial communities bidirectionally interact with host 5-HT (reviewed by Everett et al., 2022). Luminal 5-HT levels can favor certain species, some of those can increase 5-HT concentrations by changing the expression levels of genes involved in its synthesis, metabolism, secretion, or transport. Additionally, via the gut-brain axis, microbiota can affect brain 5-HT levels by modulating trp brain influx based on trp bacterial metabolism in the gut, but also influencing expression of 5-HT-related genes at central level. For example, bacterial extracellular vesicles derived from Akkermansia muciniphila cause an increase of colonic and hippocampal 5-HT levels, by up-regulating 5-HT synthesis enzymes both in the gut and, probably by crossing the BBB, in the brain. On these bases, changes in 5-HT induced by gut microbiota may contribute to various neurological conditions (Yaghoubfar et al., 2020).
Overall, a balance among the three pathways for trp catabolism is essential to maintain intestinal and central homeostasis and functionality. An excess or change in the ratio of trp pathways’ by-products could be responsible for several consequences, including neurological disturbances (Figure 2). For example, IDO1 pathway over-activation by intestinal mononuclear cells, which induces massive production of kynurenine, causes a deficiency of trp in brain and in 5-HT production, with consequent neurological disturbs. The over-activation of IDO1 may also decrease the gastrointestinal availability of trp, contributing to lower production of bacterial AHR agonists. In support of this evidence, the supplementation of trp or trp-derived agonists can limit CNS inflammation (Rothhammer et al., 2016). Based on the bacterial contribution to trp availability for each pathway, this balance strongly depends on gut microbiota membership. Neurodegeneration can be linked to alteration of microbiota structure and composition through modulation of several pathways involving gut microorganisms’ metabolic functions of trp catabolism (Figure 2).
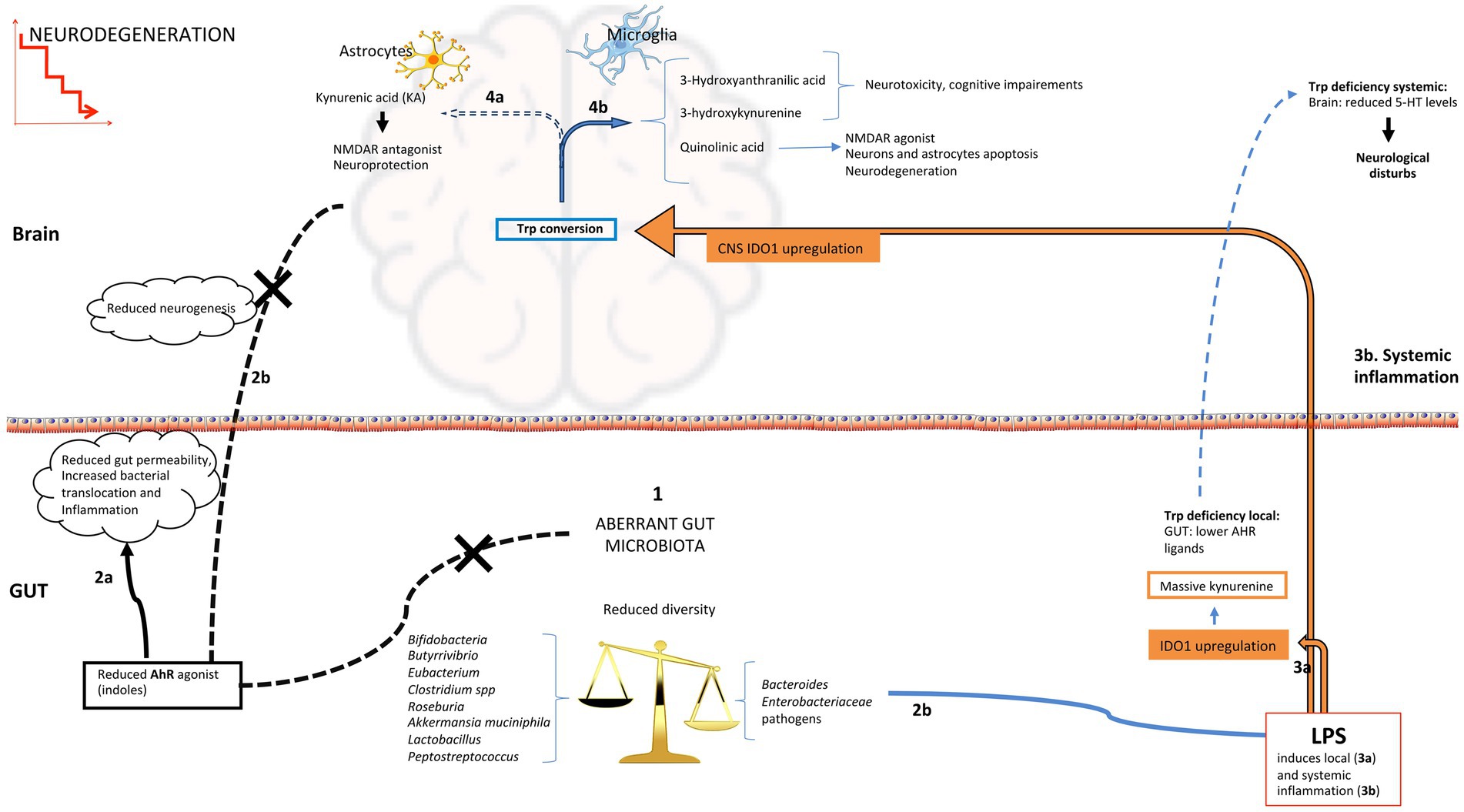
Figure 2. Possible routes of AHR activation in the gut and brain during neurodegeneration. (1) Aberrant microbiota profiles with diversity decline, enrichment in pathobionts and reduction of beneficial bacteria have been associated to neurodegenerative diseases; reduction in indole-producing bacteria induces low indoles levels that are AHR ligands, this can affect both intestinal function (2a; imbalance in immune cell populations, increase in proinflammatory cytokines and permeability), and the immunological state and neurogenesis at central level (2b); increased gut permeability could favour the translocation of inflammatory bacterial components like LPS into the systemic circulation, that reaching the brain and activating microglia can promote neuroinflammation (3b); (2d) gram-negative bacteria are source of LPS that can increase local inflammation and upregulate IDO1 activity increasing the kynurenine pathway of tryptophan degradation (3a) leading to indoles and 5-HT reduction; systemic LPS (3b) reaching the brain affects tryptophan conversion reducing the production of KA in astrocytes (4a) and increasing other downstream metabolites of kynurenine pathway with negative effects on neuronal homeostasis (4b). 5-HT, 5-hydroxytryptamine; IDO1, indoleamine 2,3-dioxygenase 1; KA, kynurenic acid.
5 Convergence of AHR signaling and aging-related brain diseases encompasses trp metabolism derivatives of gut microbiota
Despite a plethora of factors have been recognized as playing an important role in the onset of neurodegenerative diseases, AHR signaling is gaining attention due to the link with gut commensal trp derivatives and the control of CNS inflammation.
We will here consider the convergence of AHR signaling in PD and AD in which chronic inflammation represents a crucial cause of brain cell damage and death. Aberrant microbiota profiles described in patients with AD and PD could promote neuroinflammation by affecting local trp metabolism and AHR-dependent mechanisms that are critical regulators of inflammatory players at both gut and central level.
Abnormal levels of AHR have been found in post-mortem brains and circulation of AD patients (Rothhammer and Quintana, 2019; Ramos-García et al., 2020). Among the key features marking the gut microbiota alteration identified in patients with AD, reduction in species able to produce AHR ligands has also been described. Specifically, Bifidobacterium, Bacteroides, and several members of Firmicutes phylum, such as Lactobacillus spp. that are reduced in patients with AD, are indole-producing bacteria. Notably, long-term dietary supplementation of probiotics containing Lactobacillus and Bifidobacterium strains showed positive impact on cognitive function, learning and memory in a rat model of AD (Rezaeiasl et al., 2019). Moreover, fecal metabolomics profiling identified a disturbance of trp metabolism in AD patients, particularly related to metabolites processed by gut commensals, being the levels of trp host derivatives kynurenine and KA not changed. Interestingly, metabolites in the serotonin pathway, co-produced by host and gut microbiota, and some microbial indole derivatives, that are AHR ligands, were significantly decreased in AD (Wu et al., 2021). Decreased plasma levels of trp and ILA have also been associated with the progression of AD, suggesting enhanced trp degradation through the kynurenine pathway in AD (Shao et al., 2020). Recently, Sun et al. found abnormal levels of indole-producing bacteria in the APP/PS1 mouse model of AD; they demonstrated that treatment with indole and its derivatives (IAA and IPA) improved gut integrity and, by increasing the levels of AHR, reduced the inflammatory response and cognitive impairment of APP/PS1 mice (Sun et al., 2022). In another study, Pan and co-authors also demonstrated that high-trp diet ameliorates cognitive dysfunction and decreases Aβ deposition in APP/PS1 mice (Pan et al., 2023). Collectively, it is suggested that trp and its indoles derivatives could exert anti-neuroinflammatory effect by activating the AhR and restraining the NF-κB pathway in AD. Regarding KP by-products, whose levels can affect trp, 5-HT and indoles availability (Chatterjee et al., 2019), several evidences reported their abnormal levels both in patients and animal models of PD. Aberrant levels of QA, 3-hydroxykynurenine, and KA together with increased expression of IDO1 have been associated with AD hallmarks (Chatterjee et al., 2019). This increase in the KP intermediates is mainly observed in microglia and astrocytes, which are the mediators of neuroinflammation in AD. The increased secretion of inflammatory signaling molecules can both further trigger Aβ generation and immune responses, and activate IDO1 resulting in increased trp degradation and increased KP metabolites. Thus, the establishment of a vicious circle that encompasses a reduction of gut bacterial indole-producers can underlie the progression of neurodegeneration in AD.
Altered metabolism of trp and AHR activation has also been found in patients with PD and in animal models of the disease. The association between KP metabolites and PD has been extensively reviewed (Venkatesan et al., 2020). In addition, levels of neuroprotective trp metabolites, such as IAA and KA, have been found reduced in patients with PD (Chen and Lin, 2022). Interestingly, dietary trp significantly ameliorates impaired motor function, up-regulates tyrosine hydroxylase expression (the enzyme responsible for dopamine and L-DOPA production), inhibits NF-κB in substantia nigra, and down-regulates the serum levels of pro-inflammatory cytokines in rotenone-induced rat model of PD; these effects are reversed by antibiotic treatment with ampicillin and by the inhibition of AHR pathway (Wang et al., 2021), suggesting a concerted activity between microbial metabolism of trp by gut microbiota and host AHR activation that can protect from rotenone-induced neurotoxicity.
Thus, seminal evidence underlies that impaired production of AHR ligands by the gut microbiota represents an important factor in AD and PD. Given the influence of gut microbiota and its metabolites in preventing local intestinal as well as central inflammation, further investigation should explore how microbiota-based interventions could stimulate AHR to provide its critical functions and their impact on neurodegeneration.
6 Conclusions and future perspectives
The literature review emphasizes the significant role of the interaction between gut microbiota, trp metabolism and AHR in regulating both host health and disease. In elderly individuals, alterations in gut microbiota composition, including a decrease in indole-producing bacteria, may contribute to age-related reduction in systemic levels of indoles, affecting AHR signaling. All this can impact the host physiology leading to inflammaging, neuroinflammation, and compromised neuroprotective mechanisms. Thus, future microbiota-based interventions targeting AHR and its ligands show promise in reducing intestinal and CNS inflammation. Sustaining the production of indoles by the gut microbiota through dietary modifications (as dietary trp), prebiotics or probiotic therapies could benefit aging individuals by promoting adult neurogenesis. Additionally, administering peculiar postbiotics that are AHR ligands, namely indoles, could compensate for a suboptimal microbiota lacking indole-producing bacteria. This can represent a personalized therapy to counteract cognitive decline. Beside to be an important mediator in the interkingdom communication between gut bacteria and host by AHR signaling, indole is also an intra- and interspecies signaling molecule that, acting as signal of the quorum sensing, is able to influence the behavior of the gut microbe’s community members (Lee J. H. et al., 2015). This could favor the prevalence of bacterial species indole-responsive able themselves to produce AHR ligands helpful for immune modulation. Thus, indole may play a crucial role in reshaping disrupted gut microbial communities observed in patients with neurodegenerative diseases.
Author contributions
LC: Writing – original draft. EB: Writing – original draft, Writing – review & editing. FL: Writing – review & editing, Conceptualization.
Funding
The author(s) declare that financial support was received for the research, authorship, and/or publication of this article. This work was supported by #NEXTGENERATIONEU (NGEU) and funded by the Ministry of University and Research (MUR), NaEonal Recovery and Resilience Plan (NRRP), project MNESYS (PE0000006) – A MulEscale integrated approach to the study of the nervous system in health and disease [DN. 1553(DN. 11.10.2022) to LC].
Conflict of interest
The authors declare that the research was conducted in the absence of any commercial or financial relationships that could be construed as a potential conflict of interest.
The author(s) declared that they were an editorial board member of Frontiers, at the time of submission. This had no impact on the peer review process and the final decision.
Publisher’s note
All claims expressed in this article are solely those of the authors and do not necessarily represent those of their affiliated organizations, or those of the publisher, the editors and the reviewers. Any product that may be evaluated in this article, or claim that may be made by its manufacturer, is not guaranteed or endorsed by the publisher.
References
Agus, A., Planchais, J., and Sokol, H. (2018). Gut microbiota regulation of tryptophan metabolism in health and disease. Cell Host Microbe 23, 716–724. doi: 10.1016/j.chom.2018.05.003
Aho, V. T. E., Pereira, P. A. B., Voutilainen, S., Paulin, L., Pekkonen, E., Auvinen, P., et al. (2019). Gut microbiota in Parkinson’s disease: temporal stability and relations to disease progression. EBioMedicine 44, 691–707. doi: 10.1016/j.ebiom.2019.05.064
Akbari, E., Asemi, Z., Daneshvar Kakhaki, R., Bahmani, F., Kouchaki, E., Tamtaji, O. R., et al. (2016). Effect of probiotic supplementation on cognitive function and metabolic status in Alzheimer’s disease: A randomized, double-blind and controlled trial. Front. Aging Neurosci. 8:256. doi: 10.3389/fnagi.2016.00256
Alzheimer’s Association (2024). 2024 Alzheimer's disease facts and figures. Alzheimers Dement. 20, 3708–3821. doi: 10.1002/alz.13809
Avagliano, C., Coretti, L., Lama, A., Pirozzi, C., De Caro, C., De Biase, D., et al. (2022). Dual-hit model of Parkinson’s disease: impact of Dysbiosis on 6-Hydroxydopamine-insulted mice—neuroprotective and anti-inflammatory effects of butyrate. Int. J. Mol. Sci. 23:6367. doi: 10.3390/ijms23126367
Banoglu, E., and King, R. S. (2002). Sulfation of indoxyl by human and rat aryl (phenol) sulfotransferases to form indoxyl sulfate. Eur. J. Drug Metab. Pharmacokinet. 27, 135–140. doi: 10.1007/bf03190428
Barichella, M., Pacchetti, C., Bolliri, C., Cassani, E., Iorio, L., Pusani, C., et al. (2016). Probiotics and prebiotic fiber for constipation associated with Parkinson disease: an RCT. Neurology 87, 1274–1280. doi: 10.1212/WNL.0000000000003127
Barroso, A., Mahler, J. V., Fonseca-Castro, P. H., and Quintana, F. J. (2021). The aryl hydrocarbon receptor and the gut-brain axis. Cell. Mol. Immunol. 18, 259–268. doi: 10.1038/s41423-020-00585-5
Bessede, A., Gargaro, M., Pallotta, M. T., Matino, D., Servillo, G., Brunacci, C., et al. (2014). Aryl hydrocarbon receptor control of a disease tolerance defence pathway. Nature 511, 184–190. doi: 10.1038/nature13323
Biagi, E., Franceschi, C., Rampelli, S., Severgnini, M., Ostan, R., Turroni, S., et al. (2016). Gut microbiota and extreme longevity. Curr. Biol. 26, 1480–1485. doi: 10.1016/j.cub.2016.04.016
Biagi, E., Nylund, L., Candela, M., Ostan, R., Bucci, L., Pini, E., et al. (2010). Through ageing, and beyond: gut microbiota and inflammatory status in seniors and centenarians. PLoS One 5:e10667. doi: 10.1371/journal.pone.0010667
Borzabadi, S., Oryan, S., Eidi, A., Aghadavod, E., Daneshvar Kakhaki, R., Tamtaji, O. R., et al. (2018). The effects of probiotic supplementation on gene expression related to inflammation, insulin and lipid in patients with Parkinson's disease: a randomized, double-blind, placebocontrolled trial. Arch. Iran. Med. 21, 289–295
Bostanciklioğlu, M. (2018). Intestinal bacterial Flora and Alzheimer’s disease. Neurophysiology 50, 140–148. doi: 10.1007/s11062-018-9728-0
Busbee, P. B., Menzel, L., Alrafas, H., Dopkins, N., Becker, W., Miranda, K., et al. (2020). Indole-3-carbinol prevents colitis and associated microbial dysbiosis in an IL-22–dependent manner. JCI Insight 5:e127551. doi: 10.1172/jci.insight.127551
Campbell, B. M., Charych, E., Lee, A. W., and Möller, T. (2014). Kynurenines in CNS disease: regulation by inflammatory cytokines. Front. Neurosci. 8:12. doi: 10.3389/fnins.2014.00012
Cervantes-Barragan, L., Chai, J. N., Tianero, M., di Luccia, B., Ahern, P. P., Merriman, J., et al. (2017). Lactobacillus reuteri induces gut intraepithelial CD4 + CD8αα + T cells. Science 357, 806–810. doi: 10.1126/science.aah5825
Chang, C.-C., Sue, Y.-M., Yang, N.-J., Lee, Y.-H., and Juan, S.-H. (2014). 3-Methylcholanthrene, an AhR agonist, caused cell-cycle arrest by histone deacetylation through a RhoA-dependent recruitment of HDAC1 and pRb2 to E2F1 complex. PLoS One 9:e92793. doi: 10.1371/journal.pone.0092793
Chatterjee, P., Zetterberg, H., Goozee, K., Lim, C. K., Jacobs, K. R., Ashton, N. J., et al. (2019). Plasma neurofilament light chain and amyloid-β are associated with the kynurenine pathway metabolites in preclinical Alzheimer’s disease. J. Neuroinflammation 16:186. doi: 10.1186/s12974-019-1567-4
Chen, S.-J., and Lin, C.-H. (2022). Gut microenvironmental changes as a potential trigger in Parkinson’s disease through the gut–brain axis. J. Biomed. Sci. 29:54. doi: 10.1186/s12929-022-00839-6
Chen, Y., Wang, Y., Fu, Y., Yin, Y., and Xu, K. (2023). Modulating AHR function offers exciting therapeutic potential in gut immunity and inflammation. Cell Biosci. 13:85. doi: 10.1186/s13578-023-01046-y
Chinen, I., Nakahama, T., Kimura, A., Nguyen, N. T., Takemori, H., Kumagai, A., et al. (2015). The aryl hydrocarbon receptor/microRNA-212/132 axis in T cells regulates IL-10 production to maintain intestinal homeostasis. Int. Immunol. 27, 405–415. doi: 10.1093/intimm/dxv015
Chojnacki, C., Popławski, T., Chojnacki, J., Fila, M., Konrad, P., and Blasiak, J. (2020). Tryptophan intake and metabolism in older adults with mood disorders. Nutrients 12:3183. doi: 10.3390/nu12103183
de Rijke, T. J., Doting, M. H. E., van Hemert, S., De Deyn, P. P., van Munster, B. C., Harmsen, H. J. M., et al. (2022). A systematic review on the effects of different types of probiotics in animal Alzheimer’s disease studies. Front. Psychol. 13:879491. doi: 10.3389/fpsyt.2022.879491
Dehhaghi, M., Kazemi Shariat Panahi, H., and Guillemin, G. J. (2019). Microorganisms, tryptophan metabolism, and kynurenine pathway: A complex interconnected loop influencing human health status. Int. J. Tryptophan Res. 12:117864691985299. doi: 10.1177/1178646919852996
Deng, S., Chen, C., Lin, H., and Cheng, I. H. (2021). The beneficial effect of synbiotics consumption on Alzheimer’s disease mouse model via reducing local and systemic inflammation. IUBMB Life 74, 748–753. doi: 10.1002/iub.2589
Desbonnet, L., Clarke, G., Traplin, A., O’Sullivan, O., Crispie, F., Moloney, R. D., et al. (2015). Gut microbiota depletion from early adolescence in mice: implications for brain and behaviour. Brain Behav. Immun. 48, 165–173. doi: 10.1016/j.bbi.2015.04.004
Dopkins, N., Becker, W., Miranda, K., Walla, M., Nagarkatti, P., and Nagarkatti, M. (2021). Tryptamine attenuates experimental multiple sclerosis through activation of aryl hydrocarbon receptor. Front. Pharmacol. 11:619265. doi: 10.3389/fphar.2020.619265
Esser, C., and Rannug, A. (2015). The aryl hydrocarbon receptor in barrier organ physiology, immunology, and toxicology. Pharmacol. Rev. 67, 259–279. doi: 10.1124/pr.114.009001
Everett, B. A., Tran, P., and Prindle, A. (2022). Toward manipulating serotonin signaling via the microbiota–gut–brain axis. Curr. Opin. Biotechnol. 78:102826. doi: 10.1016/j.copbio.2022.102826
Favre, D., Mold, J., Hunt, P. W., Kanwar, B., Loke, P., Seu, L., et al. (2010). Tryptophan catabolism by Indoleamine 2,3-dioxygenase 1 alters the balance of T H 17 to regulatory T cells in HIV disease. Sci. Transl. Med. 2:32ra36. doi: 10.1126/scitranslmed.3000632
Georgescu, D., Ancusa, O. E., Georgescu, L. A., Ionita, I., and Reisz, D. (2016). Nonmotor gastrointestinal disorders in older patients with Parkinson's disease: is there hope? Clin. Interv. Aging 11, 1601–1608. doi: 10.2147/CIA.S106284
Giau, V., Wu, S., Jamerlan, A., An, S., Kim, S., and Hulme, J. (2018). Gut microbiota and their Neuroinflammatory implications in Alzheimer’s disease. Nutrients 10:1765. doi: 10.3390/nu10111765
Giil, L. M., Midttun, Ø., Refsum, H., Ulvik, A., Advani, R., Smith, A. D., et al. (2017). Kynurenine pathway metabolites in Alzheimer’s disease. J. Alzheimers Dis. 60, 495–504. doi: 10.3233/jad-170485
Gu, Y., Nishikawa, M., Brickman, A. M., Manly, J. J., Schupf, N., and Mayeux, R. P. (2021). Association of Dietary Prebiotic Consumption with reduced risk ofAlzheimer’s disease in a multiethnic population. Curr. Alzheimer Res. 18, 984–992. doi: 10.2174/1567205019666211222115142
Guillemin, G. J., and Brew, B. J. (2002). Implications of the kynurenine pathway and quinolinic acid in Alzheimer’s disease. Redox Rep. 7, 199–206. doi: 10.1179/135100002125000550
Guo, M., Peng, J., Huang, X., Xiao, L., Huang, F., and Zuo, Z. (2021). Gut microbiome features of Chinese patients newly diagnosed with Alzheimer’s disease or mild cognitive impairment. J. Alzheimers Dis. 80, 299–310. doi: 10.3233/jad-201040
Han, H., Davidson, L. A., Fan, Y., Goldsby, J. S., Yoon, G., Jin, U., et al. (2020). Loss of aryl hydrocarbon receptor potentiates FoxM1 signaling to enhance self-renewal of colonic stem and progenitor cells. EMBO J. 39:e104319. doi: 10.15252/embj.2019104319
Han, Q., Fang, J., and Li, J. (2001). Kynurenine aminotransferase and glutamine transaminase K of Escherichia coli: identity with aspartate aminotransferase. Biochem. J. 360, 617–623. doi: 10.1042/0264-6021:3600617
Harach, T., Marungruang, N., Duthilleul, N., Cheatham, V., Mc Coy, K. D., Frisoni, G., et al. (2017). Reduction of Abeta amyloid pathology in APPPS1 transgenic mice in the absence of gut microbiota. Sci. Rep. 7:46856. doi: 10.1038/srep46856
Haran, J. P., Bhattarai, S. K., Foley, S. E., Dutta, P., Ward, D. V., Bucci, V., et al. (2019). Alzheimer’s disease microbiome is associated with dysregulation of the anti-inflammatory P-glycoprotein pathway. MBio 10:e00632-19. doi: 10.1128/mbio.00632-19
Haran, J. P., and McCormick, B. A. (2021). Aging, frailty, and the microbiome-how Dysbiosis influences human aging and disease. Gastroenterology 160, 507–523. doi: 10.1053/j.gastro.2020.09.060
Hendrikx, T., Duan, Y., Wang, Y., Oh, J.-H., Alexander, L. M., Huang, W., et al. (2018). Bacteria engineered to produce IL-22 in intestine induce expression of REG3G to reduce ethanol-induced liver disease in mice. Gut 68, 1504–1515. doi: 10.1136/gutjnl-2018-317232
Hill-Burns, E. M., Debelius, J. W., Morton, J. T., Wissemann, W. T., Lewis, M. R., Wallen, Z. D., et al. (2017). Parkinson’s disease and Parkinson’s disease medications have distinct signatures of the gut microbiome. Mov. Disord. 32, 739–749. doi: 10.1002/mds.26942
Hoffman, J. D., Yanckello, L. M., Chlipala, G., Hammond, T. C., McCulloch, S. D., Parikh, I., et al. (2019). Dietary inulin alters the gut microbiome, enhances systemic metabolism and reduces neuroinflammation in an APOE4 mouse model. PLoS One 14:e0221828. doi: 10.1371/journal.pone.0221828
Hollingshead, B. D., Beischlag, T. V., DiNatale, B. C., Ramadoss, P., and Perdew, G. H. (2008). Inflammatory signaling and aryl hydrocarbon receptor mediate synergistic induction of interleukin 6 in MCF-7 cells. Cancer Res. 68, 3609–3617. doi: 10.1158/0008-5472.can-07-6168
Hou, Q., Ye, L., Liu, H., Huang, L., Yang, Q., Turner, J., et al. (2018). Lactobacillus accelerates ISCs regeneration to protect the integrity of intestinal mucosa through activation of STAT3 signaling pathway induced by LPLs secretion of IL-22. Cell Death Differ. 25, 1657–1670. doi: 10.1038/s41418-018-0070-2
Huynh, V. A., Takala, T. M., Murros, K. E., Diwedi, B., and Saris, P. E. J. (2023). Desulfovibrio bacteria enhance alpha-synuclein aggregation in a Caenorhabditis elegans model of Parkinson’s disease. Front. Cell. Infect. Microbiol. 13:1181315. doi: 10.3389/fcimb.2023.1181315
Juricek, L., and Coumoul, X. (2018). The aryl hydrocarbon receptor and the nervous system. Int. J. Mol. Sci. 19:2504. doi: 10.3390/ijms19092504
Kempuraj, D., Thangavel, R., Natteru, P. A., Selvakumar, G. P., Saeed, D., Zahoor, H., et al. (2015). Neuroinflammation induces neurodegeneration. J. Neurol. Neurosurg. Spine 1:1003.
Keshavarzian, A., Green, S. J., Engen, P. A., Voigt, R. M., Naqib, A., Forsyth, C. B., et al. (2015). Colonic bacterial composition in Parkinson’s disease. Mov. Disord. 30, 1351–1360. doi: 10.1002/mds.26307
Kim, M.-S., Kim, Y., Choi, H., Kim, W., Park, S., Lee, D., et al. (2019). Transfer of a healthy microbiota reduces amyloid and tau pathology in an Alzheimer’s disease animal model. Gut 69, 283–294. doi: 10.1136/gutjnl-2018-317431
Kim, W. H., Lillehoj, H. S., and Min, W. (2019). Indole treatment alleviates intestinal tissue damage induced by chicken coccidiosis through activation of the aryl hydrocarbon receptor. Front. Immunol. 10:560. doi: 10.3389/fimmu.2019.00560
Kurata, K., Ishii, K., Koto, Y., Naito, K., Yuasa, K., and Shimizu, H. (2023). Skatole-induced p38 and JNK activation coordinately upregulates, whereas AhR activation partially attenuates TNFα expression in intestinal epithelial cells. Biosci. Biotechnol. Biochem. 87, 611–619. doi: 10.1093/bbb/zbad030
Lamas, B., Natividad, J. M., and Sokol, H. (2018). Aryl hydrocarbon receptor and intestinal immunity. Mucosal Immunol. 11, 1024–1038. doi: 10.1038/s41385-018-0019-2
Lee, Y.-S., Lai, D.-M., Huang, H.-J., Lee-Chen, G.-J., Chang, C.-H., Hsieh-Li, H. M., et al. (2021). Prebiotic lactulose ameliorates the cognitive deficit in Alzheimer’s disease mouse model through macroautophagy and chaperone-mediated autophagy pathways. J. Agric. Food Chem. 69, 2422–2437. doi: 10.1021/acs.jafc.0c07327
Lee, Y., Lin, C., Hsu, P., Sun, Y., Huang, Y., Zhuo, J., et al. (2015). Aryl hydrocarbon receptor mediates both proinflammatory and anti-inflammatory effects in lipopolysaccharide-activated microglia. Glia 63, 1138–1154. doi: 10.1002/glia.22805
Lee, J.-H., Wood, T. K., and Lee, J. (2015). Roles of indole as an interspecies and Interkingdom signaling molecule. Trends Microbiol. 23, 707–718. doi: 10.1016/j.tim.2015.08.001
Li, C., Cui, L., Yang, Y., Miao, J., Zhao, X., Zhang, J., et al. (2019). Gut microbiota differs between Parkinson’s disease patients and healthy controls in Northeast China. Front. Mol. Neurosci. 12:171. doi: 10.3389/fnmol.2019.00171
Li, X., Zhang, B., Hu, Y., and Zhao, Y. (2021). New insights into gut-Bacteria-derived indole and its derivatives in intestinal and liver diseases. Front. Pharmacol. 12:769501. doi: 10.3389/fphar.2021.769501
Liang, S. C., Tan, X.-Y., Luxenberg, D. P., Karim, R., Dunussi-Joannopoulos, K., Collins, M., et al. (2006). Interleukin (IL)-22 and IL-17 are coexpressed by Th17 cells and cooperatively enhance expression of antimicrobial peptides. J. Exp. Med. 203, 2271–2279. doi: 10.1084/jem.20061308
Liu, Q., Xi, Y., Wang, Q., Liu, J., Li, P., Meng, X., et al. (2021). Mannan oligosaccharide attenuates cognitive and behavioral disorders in the 5xFAD Alzheimer’s disease mouse model via regulating the gut microbiota-brain axis. Brain Behav. Immun. 95, 330–343. doi: 10.1016/j.bbi.2021.04.005
Liu, C. C., Xia, M., Zhang, Y. J., Jin, P., Zhao, L., Zhang, J., et al. (2018). Micro124-mediated AHR expression regulates the inflammatory response of chronic rhinosinusitis (CRS) with nasal polyps. Biochem. Biophys. Res. Commun. 500, 145–151. doi: 10.1016/j.bbrc.2018.03.204
Manzella, C. R., Ackerman, M., Singhal, M., Ticho, A. L., Ceh, J., Alrefai, W. A., et al. (2020). Serotonin modulates AhR activation by interfering with CYP1A1-mediated clearance of AhR ligands. Cell. Physiol. Biochem. 54, 126–141. doi: 10.33594/000000209
Minter, M. R., Zhang, C., Leone, V., Ringus, D. L., Zhang, X., Oyler-Castrillo, P., et al. (2016). Antibiotic-induced perturbations in gut microbial diversity influences neuro-inflammation and amyloidosis in a murine model of Alzheimer’s disease. Sci. Rep. 6:30028. doi: 10.1038/srep30028
Miyamoto, K., Sujino, T., Harada, Y., Ashida, H., Yoshimatsu, Y., Yonemoto, Y., et al. (2023). The gut microbiota-induced kynurenic acid recruits GPR35-positive macrophages to promote experimental encephalitis. Cell Rep. 42:113005. doi: 10.1016/j.celrep.2023.113005
O’Farrell, K., and Harkin, A. (2017). Stress-related regulation of the kynurenine pathway: relevance to neuropsychiatric and degenerative disorders. Neuropharmacology 112, 307–323. doi: 10.1016/j.neuropharm.2015.12.004
Obata, Y., Castaño, Á., Boeing, S., Bon-Frauches, A. C., Fung, C., Fallesen, T., et al. (2020). Neuronal programming by microbiota regulates intestinal physiology. Nature 578, 284–289. doi: 10.1038/s41586-020-1975-8
Pan, S., Zhang, Y., Ye, T., Kong, Y., Cui, X., Yuan, S., et al. (2023). A high-tryptophan diet alleviated cognitive impairment and Neuroinflammation in APP/PS1 mice through activating aryl hydrocarbon receptor via the regulation of gut microbiota. Mol. Nutr. Food Res. 68:e2300601. doi: 10.1002/mnfr.202300601
Pirozzi, C., Coretti, L., Opallo, N., Bove, M., Annunziata, C., Comella, F., et al. (2023). Palmitoylethanolamide counteracts high-fat diet-induced gut dysfunction by reprogramming microbiota composition and affecting tryptophan metabolism. Front. Nutr. 10:1143004. doi: 10.3389/fnut.2023.1143004
Platten, M., Nollen, E. A. A., Röhrig, U. F., Fallarino, F., and Opitz, C. A. (2019). Tryptophan metabolism as a common therapeutic target in cancer, neurodegeneration and beyond. Nat. Rev. Drug Discov. 18, 379–401. doi: 10.1038/s41573-019-0016-5
Powell, D. N., Swimm, A., Sonowal, R., Bretin, A., Gewirtz, A. T., Jones, R. M., et al. (2020). Indoles from the commensal microbiota act via the AHR and IL-10 to tune the cellular composition of the colonic epithelium during aging. Proc. Natl. Acad. Sci. 117, 21519–21526. doi: 10.1073/pnas.2003004117
Qian, X. H., Xie, R. Y., Liu, X. L., Chen, S. D., and Tang, H. D. (2022). Mechanisms of short-chain fatty acids derived from gut microbiota in Alzheimer's disease. Aging Dis. 13, 1252–1266. doi: 10.14336/AD.2021.1215
Quintana, F. J., Basso, A. S., Iglesias, A. H., Korn, T., Farez, M. F., Bettelli, E., et al. (2008). Control of Treg and TH17 cell differentiation by the aryl hydrocarbon receptor. Nature 453, 65–71. doi: 10.1038/nature06880
Ramos-García, N. A., Orozco-Ibarra, M., Estudillo, E., Elizondo, G., Gómez Apo, E., Chávez Macías, L. G., et al. (2020). Aryl hydrocarbon receptor in post-mortem Hippocampus and in serum from young, elder, and Alzheimer’s patients. Int. J. Mol. Sci. 21:1983. doi: 10.3390/ijms21061983
Rezaeiasl, Z., Salami, M., and Sepehri, G. (2019). The effects of probiotic Lactobacillus and Bifidobacterium strains on memory and learning behavior, long-term potentiation (LTP), and some biochemical parameters in β-amyloid-induced Rat’s model of Alzheimer’s disease. Prevent. Nut. Food Sci. 24, 265–273. doi: 10.3746/pnf.2019.24.3.265
Roager, H. M., and Licht, T. R. (2018). Microbial tryptophan catabolites in health and disease. Nat. Commun. 9, 9:3294. doi: 10.1038/s41467-018-05470-4
Romani, L., Fallarino, F., De Luca, A., Montagnoli, C., D’Angelo, C., Zelante, T., et al. (2008). Defective tryptophan catabolism underlies inflammation in mouse chronic granulomatous disease. Nature 451, 211–215. doi: 10.1038/nature06471
Rothhammer, V., Borucki, D. M., Tjon, E. C., Takenaka, M. C., Chao, C.-C., Ardura-Fabregat, A., et al. (2018). Microglial control of astrocytes in response to microbial metabolites. Nature 557, 724–728. doi: 10.1038/s41586-018-0119-x
Rothhammer, V., Mascanfroni, I. D., Bunse, L., Takenaka, M. C., Kenison, J. E., Mayo, L., et al. (2016). Type I interferons and microbial metabolites of tryptophan modulate astrocyte activity and central nervous system inflammation via the aryl hydrocarbon receptor. Nat. Med. 22, 586–597. doi: 10.1038/nm.4106
Rothhammer, V., and Quintana, F. J. (2019). The aryl hydrocarbon receptor: an environmental sensor integrating immune responses in health and disease. Nat. Rev. Immunol. 19, 184–197. doi: 10.1038/s41577-019-0125-8
Ruiz-Ruiz, S., Sanchez-Carrillo, S., Ciordia, S., Mena, M. C., Méndez-García, C., Rojo, D., et al. (2019). Functional microbiome deficits associated with ageing: chronological age threshold. Aging Cell 19:e13063. doi: 10.1111/acel.13063
Safe, S., Han, H., Goldsby, J., Mohankumar, K., and Chapkin, R. S. (2018). Aryl hydrocarbon receptor (AhR) ligands as selective AhR modulators: genomic studies. Curre. Opin. Toxicol. 11-12, 10–20. doi: 10.1016/j.cotox.2018.11.005
Sandgren, A. M., and Brummer, R. J. M. (2018). ADHD-originating in the gut? The emergence of a new explanatory model. Med. Hypotheses 120, 135–145. doi: 10.1016/j.mehy.2018.08.022
Sarkar, A., and Pitchumoni, C. S. (2017). “Chapter 4 - Identification of the microbiota in the aging process,” in The microbiota in gastrointestinal pathophysiology. eds. M. H. Floch, Y. Ringel, and W. A. Walker (Academic Press), 37–56.
Scheperjans, F., Aho, V., Pereira, P. A. B., Koskinen, K., Paulin, L., Pekkonen, E., et al. (2014). Gut microbiota are related to Parkinson’s disease and clinical phenotype. Mov. Disord. 30, 350–358. doi: 10.1002/mds.26069
Schnekenburger, M., Peng, L., and Puga, A. (2007). HDAC1 bound to the Cyp1a1 promoter blocks histone acetylation associated with ah receptor-mediated trans-activation. Biochim. Biophy. Acta Gene Struct. Exp. 1769, 569–578. doi: 10.1016/j.bbaexp.2007.07.002
Scott, S. A., Fu, J., and Chang, P. V. (2020). Microbial tryptophan metabolites regulate gut barrier function via the aryl hydrocarbon receptor. Proc. Natl. Acad. Sci. 117, 19376–19387. doi: 10.1073/pnas.2000047117
Shao, Y., Ouyang, Y., Li, T., Liu, X., Xu, X., Li, S., et al. (2020). Alteration of metabolic profile and potential biomarkers in the plasma of Alzheimer’s disease. Aging Dis. 11:1459. doi: 10.14336/ad.2020.0217
Simon, D. K., Tanner, C. M., and Brundin, P. (2020). Parkinson disease epidemiology, pathology, genetics, and pathophysiology. Clin. Geriatr. Med. 36, 1–12. doi: 10.1016/j.cger.2019.08.002
Sondermann, N. C., Faßbender, S., Hartung, F., Hätälä, A. M., Rolfes, K. M., Vogel, C. F. A., et al. (2023). Functions of the aryl hydrocarbon receptor (AHR) beyond the canonical AHR/ARNT signaling pathway. Biochem. Pharmacol. 208:115371. doi: 10.1016/j.bcp.2022.115371
Song, Z., Wu, Z., Luo, R., He, C., Li, Z., Yang, M., et al. (2023). Identification of tryptophan metabolism-related genes in immunity and immunotherapy in Alzheimer’s disease. Aging 15, 13077–13099. doi: 10.18632/aging.205220
Stilling, R. M., van de Wouw, M., Clarke, G., Stanton, C., Dinan, T. G., and Cryan, J. F. (2016). The neuropharmacology of butyrate: the bread and butter of the microbiota-gut-brain axis? Neurochem. Int. 99, 110–132. doi: 10.1016/j.neuint.2016.06.011
Stockinger, B., Shah, K., and Wincent, E. (2021). AHR in the intestinal microenvironment: safeguarding barrier function. Nat. Rev. Gastroenterol. Hepatol. 18, 559–570. doi: 10.1038/s41575-021-00430-8
Sun, J., Zhang, Y., Kong, Y., Ye, T., Yu, Q., Kumaran Satyanarayanan, S., et al. (2022). Microbiota-derived metabolite indoles induced aryl hydrocarbon receptor activation and inhibited neuroinflammation in APP/PS1 mice. Brain Behav. Immun. 106, 76–88. doi: 10.1016/j.bbi.2022.08.003
Tamtaji, O. R., Heidari-soureshjani, R., Mirhosseini, N., Kouchaki, E., Bahmani, F., Aghadavod, E., et al. (2019a). Probiotic and selenium co-supplementation, and the effects on clinical, metabolic and genetic status in Alzheimer’s disease: A randomized, double-blind, controlled trial. Clin. Nutr. 38, 2569–2575. doi: 10.1016/j.clnu.2018.11.034
Tamtaji, O. R., Taghizadeh, M., Daneshvar Kakhaki, R., Kouchaki, E., Bahmani, F., Borzabadi, S., et al. (2019b). Clinical and metabolic response to probiotic administration in people with Parkinson's disease: a randomized, double-blind, placebo-controlled trial. Clin. Nutr. 38, 1031–1035. doi: 10.1016/j.clnu.2018.05.018
Tan, A. H., Lim, S. Y., Chong, K. K., A Manap, M. A. A., Hor, J. W., Lim, J. L., et al. (2021). Probiotics for constipation in Parkinson disease: a randomized placebo-controlled study. Neurology 96, e772–e782. doi: 10.1212/WNL.0000000000010998
Turco, L., Opallo, N., Buommino, E., De Caro, C., Pirozzi, C., Mattace Raso, G., et al. (2023). Zooming into gut Dysbiosis in Parkinson’s disease: new insights from functional mapping. Int. J. Mol. Sci. 24:9777. doi: 10.3390/ijms24119777
Venkatesan, D., Iyer, M., Narayanasamy, A., Siva, K., and Vellingiri, B. (2020). Kynurenine pathway in Parkinson’s disease—An update. eNeurologicalSci. 21:100270. doi: 10.1016/j.ensci.2020.100270
Vogt, N. M., Kerby, R. L., Dill-McFarland, K. A., Harding, S. J., Merluzzi, A. P., Johnson, S. C., et al. (2017). Gut microbiome alterations in Alzheimer’s disease. Sci. Rep. 7:13537. doi: 10.1038/s41598-017-13601-y
Vujkovic-Cvijin, I., Dunham, R. M., Iwai, S., Maher, M. C., Albright, R. G., Broadhurst, M. J., et al. (2013). Dysbiosis of the gut microbiota is associated with HIV disease progression and tryptophan catabolism. Sci. Transl. Med. 5:193ra91. doi: 10.1126/scitranslmed.3006438
Wang, Y., Chen, S., Tan, J., Gao, Y., Yan, H., Liu, Y., et al. (2021). Tryptophan in the diet ameliorates motor deficits in a rotenone-induced rat Parkinson’s disease model via activating the aromatic hydrocarbon receptor pathway. Brain Behav. 11:e2226. doi: 10.1002/brb3.2226
Wei, G. Z., Martin, K. A., Xing, P. Y., Agrawal, R., Whiley, L., Wood, T. K., et al. (2021). Tryptophan-metabolizing gut microbes regulate adult neurogenesis via the aryl hydrocarbon receptor. Proc. Natl. Acad. Sci. 118:e2021091118. doi: 10.1073/pnas.2021091118
Westfall, S., Lomis, N., and Prakash, S. (2019). A novel synbiotic delays Alzheimer’s disease onset via combinatorial gut-brain-axis signaling in Drosophila melanogaster. PLoS One 14:e0214985. doi: 10.1371/journal.pone.0214985
Więdłocha, M., Marcinowicz, P., Janoska-Jaździk, M., and Szulc, A. (2021). Gut microbiota, kynurenine pathway and mental disorders – review. Prog. Neuro-Psychopharmacol. Biol. Psychiatry 106:110145. doi: 10.1016/j.pnpbp.2020.110145
Wilck, N., Matus, M. G., Kearney, S. M., Olesen, S. W., Forslund, K., Bartolomaeus, H., et al. (2017). Salt-responsive gut commensal modulates TH17 axis and disease. Nature 551, 585–589. doi: 10.1038/nature24628
Wlodarska, M., Luo, C., Kolde, R., d’Hennezel, E., Annand, J. W., Heim, C. E., et al. (2017). Indoleacrylic acid produced by commensal Peptostreptococcus species suppresses inflammation. Cell Host Microbe 22, 25–37.e6. doi: 10.1016/j.chom.2017.06.007
Wu, L., Han, Y., Zheng, Z., Peng, G., Liu, P., Yue, S., et al. (2021). Altered gut microbial metabolites in amnestic mild cognitive impairment and Alzheimer's disease: signals in host-microbe interplay. Nutrients 13:228. doi: 10.3390/nu13010228
Wu, S., Zhang, J., Jiang, C., Wang, S., Que, R., and An, L. (2020). Up-regulation of neprilysin mediates the protection of fructo-oligosaccharides against Alzheimer’s disease. Food Funct. 11, 6565–6572. doi: 10.1039/d0fo00161a
Xu, M., Mo, X., Huang, H., Chen, X., Liu, H., Peng, Z., et al. (2020). Yeast β-glucan alleviates cognitive deficit by regulating gut microbiota and metabolites in Aβ1–42-induced AD-like mice. Int. J. Biol. Macromol. 161, 258–270. doi: 10.1016/j.ijbiomac.2020.05.180
Yaghoubfar, R., Behrouzi, A., Ashrafian, F., Shahryari, A., Moradi, H. R., Choopani, S., et al. (2020). Modulation of serotonin signaling/metabolism by Akkermansia muciniphila and its extracellular vesicles through the gut-brain axis in mice. Sci. Rep. 10:22119. doi: 10.1038/s41598-020-79171-8
Yan, J., Luo, M., Chen, Z., and He, B. (2020). The function and role of the Th17/Treg cell balance in inflammatory bowel disease. J Immunol Res 2020, 1–8. doi: 10.1155/2020/8813558
Yano, J. M., Yu, K., Donaldson, G. P., Shastri, G. G., Ann, P., Ma, L., et al. (2015). Indigenous Bacteria from the gut microbiota regulate host serotonin biosynthesis. Cell 163:258. doi: 10.1016/j.cell.2015.09.017
Ye, J., Qiu, J., Bostick, J. W., Ueda, A., Schjerven, H., Li, S., et al. (2017). The aryl hydrocarbon receptor preferentially Marks and promotes gut regulatory T cells. Cell Rep. 21, 2277–2290. doi: 10.1016/j.celrep.2017.10.114
Yu, M., Wang, Q., Ma, Y., Li, L., Yu, K., Zhang, Z., et al. (2018). Aryl hydrocarbon receptor activation modulates intestinal epithelial barrier function by maintaining tight junction integrity. Int. J. Biol. Sci. 14, 69–77. doi: 10.7150/ijbs.22259
Keywords: microbiota-gut-brain axis, neuroinflammation, AHR, Alzheimer’s disease, Parkinson’s disease, tryptophan metabolism
Citation: Coretti L, Buommino E and Lembo F (2024) The aryl hydrocarbon receptor pathway: a linking bridge between the gut microbiome and neurodegenerative diseases. Front. Cell. Neurosci. 18:1433747. doi: 10.3389/fncel.2024.1433747
Edited by:
Hayet Sellami, University of Sfax, TunisiaReviewed by:
Dorra Abdelmalek, Centre of Biotechnology of Sfax, TunisiaGreta Volpedo, University of Genoa, Italy
Copyright © 2024 Coretti, Buommino and Lembo. This is an open-access article distributed under the terms of the Creative Commons Attribution License (CC BY). The use, distribution or reproduction in other forums is permitted, provided the original author(s) and the copyright owner(s) are credited and that the original publication in this journal is cited, in accordance with accepted academic practice. No use, distribution or reproduction is permitted which does not comply with these terms.
*Correspondence: Francesca Lembo, ZnJsZW1ib0B1bmluYS5pdA==; Elisabetta Buommino, ZWxpc2FiZXR0YS5idW9tbWlub0B1bmluYS5pdA==