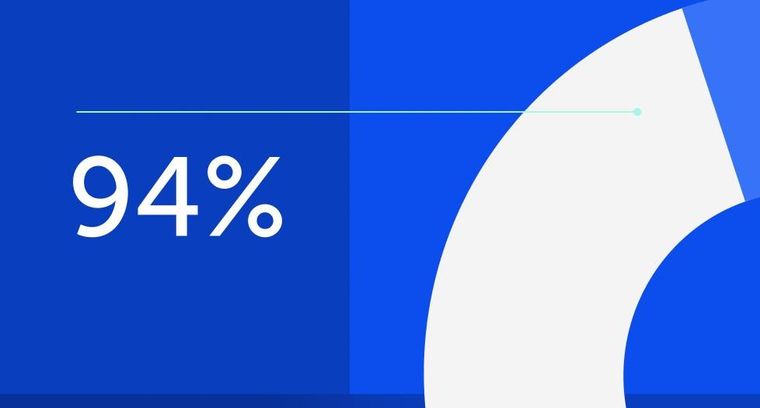
94% of researchers rate our articles as excellent or good
Learn more about the work of our research integrity team to safeguard the quality of each article we publish.
Find out more
MINI REVIEW article
Front. Cell. Neurosci., 30 September 2024
Sec. Cellular Neurophysiology
Volume 18 - 2024 | https://doi.org/10.3389/fncel.2024.1427515
This article is part of the Research TopicParadigm Shifts and Innovations in Cellular NeuroscienceView all 10 articles
The human primary visual cortex (V1) development is pivotal to understanding cortical maturation and neuroplasticity. Theories on V1 development range from early maturation models, which emphasize the early peak of synapses in infancy, to those suggesting an extended developmental timeline where key plasticity mechanisms continue to mature well into adulthood. Classic histological approaches have supported early development, while recent molecular studies highlight prolonged or multiple windows of plasticity, indicating that V1 remains susceptible to experience-dependent modifications beyond childhood. This review consolidates findings from both anatomical and molecular studies, tracing the development of V1 from prenatal stages through aging. The evidence reveals that human V1 develops across multiple timescales, with some aspects maturing early and others gradually changing across the lifespan. Reflecting on Cajal’s early work, this review underscores the importance of methodological advancements in revealing the intricate details of V1’s development.
The development of the human visual cortex (V1) has been a central theme in the two leading theories about cortical development: the system-by-system cascade, where V1 develops early (Huttenlocher and Dabholkar, 1997) and the integrated network, where V1 develops over a protracted period (Lidow et al., 1991). Classic histological investigations of V1 in infants, such as synapse counts, point to early development of human V1. Moreover, these studies suggest that the peak in synapse counts define the window of highest neuroplasticity in the human cortex (Huttenlocher, 1999; Petanjek et al., 2023). In contrast, studies using a variety of molecular approaches have shown that many of the mechanisms that regulate experience-dependent plasticity continue to mature past childhood into the second and third decades of life, suggesting that V1 has prolonged or multiple plasticity windows (Siu and Murphy, 2018). Furthermore, the protracted development of human V1 could provide a longer window for visual experience to shape its function and support plasticity-based treatments for visual disorders (Castaldi et al., 2020; Thompson et al., 2023).
In humans, more than 20 cortical areas process visual information, so the development of V1 can have a ripple effect on many cortical areas and perceptual functions. Animal models, especially those using mice, continue to reveal the unique impact of vision on the cellular landscape of the cortex (Chen et al., 2024). However, studying human V1 development using postmortem tissue is challenging because brain banks have limited cases at certain ages, and the samples are often delicate and tricky to work with. Over the past century, a growing body of anatomical and molecular research has used those rare and valuable samples to create a picture of how human V1 changes across the lifespan. This review consolidates the insights gained from classic cellular histology and modern molecular techniques, offering an overview of human V1 development covering prenatal and postnatal development and highlighting molecular mechanisms that regulate experience-dependent plasticity in V1.
The detailed cytoarchitecture of the human primary visual cortex (V1) has been known since the late 19th century, as shown by Cajal’s histological slides and drawing of neurons in V1 (Figure 1A; Cajal, 1899). Cajal’s precise drawings of human V1 using Nissl and Golgi stained sections primarily from infants led him to conclude that:
Figure 1. Human V1 cytoarchitecture. (A) Drawings by Santiago Ramón y Cajal showing the cytoarchitecture of human V1 through the full thickness of a Nissl stained section from (i) adult V1 and (ii-iv) smaller examples from Golgi stained sections of cells in layers 2/3, 4 and 5 from the infant V1. Even in the infant, the apical dendrites of pyramidal cells extend vertically across layers. Adapted from Figures 1, 4, 9 and 20 from the book “Comparative study of the sensory areas of the human cortex”, pages 314, 325, 337 and 353 (Cajal, 1899). Note: the original drawings do not include scale bars so these examples could not be matched for magnification; the book is available at: https://archive.org/details/comparativestud00cajagoog/. (B) Nissl-stained through the occipital pole of an adult human showing the unique laminar pattern of V1 and the different appearance of the neighboring extra-striate areas. In addition, variation in thickness of V1 is apparent through the depth of the calcarine fissure (asterisk). (C) The postnatal appearance of human V1 is shown in a series of Nissl-stained sections from 3 months to 17 years of age. All 6 layers are visible postnatally. Layer 1 is the outermost layer and is lightly stained because it has few cell bodies. Layers 2 & 3 have many darkly stained cells including smaller pyramidal at the top of layer 2 to medium sized pyramidal cells in layer 3. Layer 4 is the recipient zone for thalamocortical afferents and has 3 main sublaminae with larger stellate cells in 4A and smaller stellate cells, often called granule cells, through layers 4B, 4Cα and 4Cβ that give rise to the appearance of the stria of Gennari. Layer 5 appears lighter than layer 4 but is distinct because it has a band of large subcortically projecting pyramidal cells. Layer 6 has medium sized pyramidal cells at the top and a dense combination of smaller cells with a range of shapes in the deeper portion of this layer. The white matter under layer 6 has tiny round cells, the oligodendrocytes that form myelin, ensheathing the axons. In one case, the 13-year-old, some interstitial cells are visible in the white matter (Kostovic and Rakic, 1980, 1990; Judaš et al., 2010). Images of the Nissl-stained sections are from: © 2010 Allen Institute for Brain Science. Allen Human Brain Atlas. Available from: atlas.brain-map.org and © 2010 Allen Institute for Brain Science. BrainSpan Reference Atlas. Available from: www.brainspan.org/static/atlas.
The visual cortex of man and gyrencephalous mammals possesses a special structure very different from that of any other cortical area (Cajal, 1899).
The early stages of human cortical development have been reviewed in detail (Molnár et al., 2019), so here we highlight the development of V1. Changes in the first trimester of prenatal life include extending ventricular and outer radial glia fibers to the pial surface of the developing cortical plate (Bystron et al., 2008; Nowakowski et al., 2016; Arellano et al., 2021). The maturation of radial glia cells projecting to V1 lags behind those to the prefrontal cortex by about 3 weeks (Nowakowski et al., 2017). Next, neurogenesis starts (Bhardwaj et al., 2006; Clowry et al., 2010) along with peak levels of neurogenesis-specific gene expression (Zhu et al., 2018). Newly differentiated neurons migrate from the ventricular zone into the developing cortical plate along radial glia fibers (Rakic, 1972), and the cortical layers mature in an inside-out order: the neurons that will form layer 6 are born first, followed by neurons destined for layers 5 through 2 (Rakic, 1974). The supragranular neurons (layers 2/3) use outer radial glia to migrate through the cortical plate (Nowakowski et al., 2016). During the peak of neurogenesis, maturing excitatory neurons already express a rudimentary set of V1-specific marker genes (Nowakowski et al., 2017).
Throughout the second trimester, the morphological structure of the neurons begins to develop, and thalamocortical afferents grow into the transient subplate between 22 and 26 weeks of gestation and wait briefly before entering the cortical plate (Kostovic and Rakic, 1980). The cortical layers start to differentiate before thalamic afferents invade the cortical plate with deep layers apparent by 19 weeks of gestation (Takashima et al., 1980; Flower, 1985; Burkhalter, 1993; Burkhalter et al., 1993), followed by layer IV at 28 weeks and the superficial layers II/III at 28–35 weeks (Takashima et al., 1980). By the 24–25th week of gestation, the thalamocortical afferents begin to mature and express neuronal markers (Godovalova, 2010). Just a few weeks later (29th–31st weeks), functional connectivity between the thalamus and V1 begins to strengthen (Taymourtash et al., 2022), starting the period when thalamocortical activity can influence the development of V1 and a sharp increase in genes specific to neuronal differentiation (Zhu et al., 2018).
Both excitatory glutamatergic and inhibitory GABAergic neurons are found prenatally in the developing cortical plate (Yan et al., 1992; Andersen et al., 1995; Retz et al., 1996). Many of those cells, as well as synapses and thalamocortical afferents, form a transient arrangement in the subplate zone during the second and third trimesters (Kostović and Judaš, 2007). For example, dense parvalbumin (PV+) labeling is prominent in the subplate in the second trimester and, by birth, has shifted to a cortical laminar arrangement of PV+ inhibitory interneurons (Cao et al., 1996) that continues to mature until adolescence (Letinic and Kostovic, 1998).
In addition to neurons, markers for glia are found prenatally below or in the developing cortical plate of V1. Early in the second trimester, microglia cluster in the immature white matter among the visual radiations and by the middle of the second trimester are found throughout the thickness of the developing V1 (Monier et al., 2006, 2007). Astrocytes are also found in the developing V1 by the middle of the second trimester (Honig et al., 1996; Godovalova, 2010), and astrocyte-specific gene expression in human V1 continues to increase until early childhood (Zhu et al., 2018). Myelinating oligodendrocytes begin accumulating in the white matter below V1 but do not infiltrate the cortical plate before birth (Kostović et al., 2014), leaving V1 with the translucent appearance characteristic of the neonatal cortex.
The thickness of V1 grows from 100 μm to nearly 2 mm through the prenatal period and continues to grow postnatally (Zilles et al., 1986; Molnár et al., 2019). The volume and surface area of V1 has the greatest period of growth postnatally as dendrites and spines on pyramidal neurons contribute to the increased thickness of the cortex, with spines reaching a peak at 5 months before falling to adult levels by 2 years of age (Takashima et al., 1980; Becker et al., 1984; Michel and Garey, 1984). However, V1 does not have a uniform thickness and the gyral and sulcal pattern, especially deep in the calcarine fissure, leads to large variations in thickness. Furthermore, host factors such as sex and socio-economic status are known to affect the thickness of the developing cortex (Sowell et al., 2007; Mackey et al., 2015; Gennatas et al., 2017; Leonard et al., 2019; Alnćs et al., 2020; Tooley et al., 2021).
Other anatomical features, such as columnar patterns and horizontal connections, first appear in human V1 prenatally and continue to develop postnatally. Patchy cytochrome oxidase staining is visible prenatally, with distinct blobs apparent at birth (Burkhalter et al., 1993; Wong-Riley et al., 1993). The early pattern of blobs likely reflects the patchy arrangement and spontaneous activity of thalamocortical afferents projecting into the cortex. The plexus of fibers that form the stria of Gennari through layer IV of the human V1 is apparent at birth (Takashima et al., 1980), as are vertical connections between layers such as the apical dendrites of pyramidal cells drawn by Cajal (Figure 1Aii–iv). Horizontal connections emerge by layer, with long-range connections appearing in layers IV and V shortly before birth (Burkhalter et al., 1993). Those connections continue to mature postnatally, and the patchy arrangement of horizontal connections in superficial layers is found sometime after 4 months and becomes adult-like by 2 years (Burkhalter et al., 1993). Feedforward and feedback connections between V1 and V2 appear to mature sequentially, with feedforward developing by 4 months, but feedback connections continue to develop, similar to the maturation of horizontal connections in superficial layers (Burkhalter, 1993). Unfortunately, a limited number of anatomical studies with just a few cases have examined feedforward and feedback connections in human V1, so it is difficult to conclude how they develop.
Classic histological techniques have shown that anatomical structures of the human V1 are immature at birth. Still, some structures rapidly attain an adult-like appearance within the first few years of postnatal life (Conel, 1939, 1941, 1947, 1951, 1955, 1959, 1963, 1967; Huttenlocher et al., 1982; Burkhalter, 1993; Burkhalter et al., 1993). Cajal’s drawing of the infant V1 beautifully illustrates the cytoarchitecture of different laminae shortly after birth (Cajal, 1899). Although the infant (Figure 1Aii–iv) lacks the microanatomical complexity of the adult V1 (Figure 1Ai), it already has a dense network of neuronal processes that contribute to the appearance of the cortical layers. Nissl-stained sections through the occipital pole of the adult human cortex show the unique laminar appearance of V1, with the stria of Gennari in layer 4 distinguishing V1 from adjacent extra-striate areas (Figure 1B).
The adult-like appearance of layers in Nissl-stained sections from infants (Figure 1C) could be interpreted as evidence for early maturation of human V1. However, the primary function of Nissl-stained granular organelles in cells is the synthesis of proteins, which does not reflect all aspects of neuronal or glial maturity or plasticity. In contrast, molecular markers, especially for mechanisms that regulate experience-dependent plasticity, continue developing beyond the first few years of life.
Animal studies have shown that many neuronal and non-neuronal molecular mechanisms regulate experience-dependent plasticity in the visual system. It is beyond the scope of this mini-review to address all of those mechanisms, but there are excellent reviews that readers can use to gain a broader understanding (Huang et al., 1999; Berardi et al., 2003, 2004; Tropea et al., 2009; Takesian and Hensch, 2013; Pribiag and Stellwagen, 2014; Priebe and McGee, 2014; Sadahiro et al., 2016; Stephany et al., 2016; Hensch and Quinlan, 2018; Grieco et al., 2019; Benfey et al., 2022). Here, we focus on a set of glutamatergic and GABAergic mechanisms that are known to impact the role of excitatory and inhibitory neurotransmission in experience-dependent plasticity (Hensch, 2005; Hensch and Fagiolini, 2005; Maffei and Turrigiano, 2008; Smith et al., 2009; Tropea et al., 2009; Levelt and Hübener, 2012; Capogna et al., 2021) and have been well studied in human V1 across the lifespan (Murphy et al., 2005; Pinto et al., 2010, 2015; Williams et al., 2010; Siu et al., 2015, 2017; Siu and Murphy, 2018; Balsor et al., 2021).
This section reviews findings from animal studies to introduce the role of glutamatergic and GABAergic mechanisms in regulating experience-dependent plasticity in V1.
Most neurons and synapses in V1 are excitatory (glutamatergic), while only 20% of the neurons and an even smaller percentage of the synapses are inhibitory (GABAergic) (Beaulieu et al., 1992; Jones, 1993). The thalamocortical inputs to layer 4 are excitatory; however, many parvalbumin-positive (PV+) inhibitory interneurons are found in that layer (Balaram et al., 2014). Those PV+ neurons and processes modulate feedforward inputs to V1 (Pouille and Scanziani, 2001), thereby regulating experience-dependent plasticity (Sur et al., 2013; Capogna et al., 2021). This network of glutamatergic and GABAergic mechanisms contributes to shaping the maturation of V1 by guiding synapse development, transmitting visually-driven signals, and regulating experience-dependent plasticity to connect structure and function seamlessly.
Neurons expressing glutamatergic (NMDA and AMPA receptors) and GABAergic receptors are found in all layers of V1 (Albin et al., 1991; Hendry et al., 1994; Huntley et al., 1994; Trepel et al., 1998; Eickhoff et al., 2007, 2008), and developmental changes in those receptors contribute to experience-dependent plasticity. For example, NMDA receptors regulate how visual experience shapes the maturation of receptive field properties (e.g., ocular dominance, orientation tuning and direction selectivity) and the development of visual acuity (Kleinschmidt et al., 1987; Carmignoto and Vicini, 1992; Quinlan et al., 1999; Ramoa et al., 2001; Rivadulla et al., 2001; Fagiolini et al., 2003; Philpot et al., 2003, 2007; Smith et al., 2009). Also, GABAergic transmission and its receptors are essential for triggering the start and closure of the critical period and regulating the maturation of ocular dominance and orientation tuning in V1 (Tsumoto and Sato, 1985; Hensch et al., 1998; Huang et al., 1999; Fagiolini and Hensch, 2000; Morales et al., 2002; Fagiolini et al., 2004; Hensch, 2005). Together, glutamatergic and GABAergic mechanisms regulate the Excitatory-Inhibitory (E-I) balance. When GABAergic signaling is manipulated to shift that balance, it can either enhance or reduce visual plasticity depending on the age and in which GABAergic cells are engaged (Kirkwood and Bear, 1994; Iwai et al., 2003; Maffei et al., 2004; Hensch and Fagiolini, 2005). Importantly, even small changes in the E-I balance can dramatically alter plasticity in V1 (Fagiolini and Hensch, 2000).
NMDA receptors are key components regulating experience-dependent plasticity and are highly expressed prenatally in V1 when nascent synapses are functionally silent (Rumpel et al., 1998). NMDA receptors are tetrameric and contain two obligatory GluN1 subunits paired with two GluN2 (A-D) and/or GluN3 subunits (Monyer et al., 1994). The GluN2 subunits control the kinetics of the receptors. Nascent NMDA receptors have the slower GluN2B subunit, but visual experience drives the insertion of the faster GluN2A subunit into the receptor (Sheng et al., 1994). Furthermore, the GluN2A:GluN2B ratio controls the threshold for experience-dependent plasticity (Philpot et al., 2007; Yashiro and Philpot, 2008) and can be bidirectionally regulated by visual experience (Quinlan et al., 1999).
During the early stages of synapse development, few synapses express AMPA receptors, but visual-driven activity drives their insertion into nascent synapses, converting NMDAR-dominated silent synapses into active synapses (Rumpel et al., 1998). AMPA receptors are essential components of plasticity driven by long-term potentiation or homeostatic synaptic scaling (Gainey et al., 2009). Visual experience drives the accumulation of AMPA receptors at synapses to support visual responsiveness (Heynen et al., 2003; Lambo and Turrigiano, 2013), while deprivation leads to a loss of AMPA receptor expression in V1 (Williams et al., 2015).
Other glutamatergic mechanisms, such as the receptor scaffolding protein postsynaptic density 95 (PSD95), contribute to the experience-dependent refinement of V1. A mobile pool of PSD95 rapidly moves among active synapses, and that pool is dramatically reduced by sensory deprivation (Gray et al., 2006). Furthermore, a spike in PSD95 expression contributes to ending the critical period (Huang et al., 2015). Finally, PSD95, along with the scaffolding protein for GABAA receptors, gephyrin, mediate the E-I balance (Prange et al., 2004; Lardi-Studler et al., 2007; Keith and El-Husseini, 2008) that is crucial for regulating experience-dependent plasticity in V1 (Hensch, 2004; Hensch and Fagiolini, 2005; Hensch and Quinlan, 2018).
The GABAA receptor is the most abundant ionotropic GABA receptor in V1. More than 20 subunits combine to make a wide range of different GABAA receptors (Sieghart, 1995; Cherubini and Conti, 2001) and three of those subunits, α1, α2 and α3, are developmentally regulated (Laurie et al., 1992; Hendrickson et al., 1994; Chen et al., 2001; Heinen et al., 2004). Nascent GABAA receptors have more α2 and α3 subunits, but during development, there is a shift to more α1 and a faster inhibitory postsynaptic potential (IPSP) (Gingrich et al., 1995). The α1 subunit is the high-affinity interface for binding the neurotransmitter GABA and benzodiazepines, so its development has a crucial role in GABAergic neurotransmission (Pritchett et al., 1989; Smith and Olsen, 1995; Sigel, 2002). Abnormal visual experience during the critical period accelerates the developmental shift to more GABAAα1 (Beston et al., 2010; Balsor et al., 2019) while GABAergic synapses containing α2 receptors regulate neuronal firing (Fagiolini et al., 2004). However, only α1-containing receptors drive experience-dependent critical period plasticity (Fagiolini et al., 2004).
In the next section, we review the pattern of developmental changes in these glutamatergic and GABAergic mechanisms in human V1 and highlight insights from those developmental trajectories that may influence the potential for experience-dependent plasticity.
Animal studies have shown that the development of glutamatergic and GABAergic mechanisms regulates many aspects of experience-dependent plasticity in V1. Moreover, in human V1, magnetic resonance spectroscopy has been used to observe changes in glutamate and GABA linked to visual stimulation, age and plasticity (Lin et al., 2012; Lunghi et al., 2015; Pitchaimuthu et al., 2017; Wijtenburg et al., 2017; Kurcyus et al., 2018; Frank et al., 2022). Developmental transcriptome databases like the BrainSpan (Miller et al., 2014) contain information about those mechanisms, but only a few studies provide analyses of postnatal changes in human V1 (Zhu et al., 2018; Balsor et al., 2021; Natu et al., 2021). Our laboratory has a library of postmortem tissue samples from human V1 that we have used to explore the postnatal development of many glutamatergic, GABAergic proteins and a select few other plasticity-related proteins. Those studies are included in the review below.
In human V1, the development of glutamatergic and GABAergic molecular mechanisms proceeds in waves across multiple timescales that cover the lifespan (Murphy et al., 2005; Pinto et al., 2010, 2015; Williams et al., 2010; Siu et al., 2017; Siu and Murphy, 2018; Figure 2A). The obligatory subunit of NMDA receptors, GluN1, is highly expressed at birth in human V1, then quickly declines over the first 2 years of life (Murphy et al., 2005; Siu et al., 2017; Siu and Murphy, 2018). That loss is balanced by an increase in AMPA receptors (e.g., GluA2) and the maturational shift from more NMDA (GluN1) in infancy to more AMPA receptors in older children (Murphy et al., 2005; Siu et al., 2017; Siu and Murphy, 2018). The rapid response properties of AMPA receptors imbue synapses with strong feedforward responses to visually driven activity, while the slower NMDA receptors contribute to feedback modulation of visual processing in adult V1 (Self et al., 2012). So the early shift in the AMPA:NMDA (GluA2:GluN1) balance suggests the rapid establishment of feedforward connections to human V1, followed by an extended period when feedback and other connections are established (Figure 2B).
Figure 2. Development of plasticity proteins and balances in human V1. (A) The heatmap shows the development of a set of glutamatergic, GABAergic and a select few other plasticity-related proteins in human V1. The colors indicate the development of each protein across the lifespan, where yellow is the lowest and dark red is the highest expression level. There are waves of high expression (dark red) in each stage, highlighting multiple timescales for the development of these plasticity proteins. (B) The heatmap shows the development of a set of balances known to regulate experience-dependent plasticity in V1. The colors reflect maturation, with the purplish colors indicating that the earlier developing protein is dominant and greenish that the later developing protein dominates the balance. The proteins that develop earlier (GluN1, GluN2B, GABAAα2) dominate the balances in neonates and infants, but all of the balances shift toward the later developing proteins (GluA2, GluN2A, GABAAα1, PSD95) in childhood. The GluA2:GluN1 balance matures rapidly in childhood, but the other balances continue to mature slowly into teens, young adults and older adults. The time course of changes in these balances is significant as they indicate lifelong changes in the mechanisms regulating plasticity in human V1. The data used to create these figures are from the following papers (Murphy et al., 2005; Pinto et al., 2010, 2015; Williams et al., 2010; Siu et al., 2015, 2017).
Through infancy and young childhood, the GluN2B subunit dominates the composition of NMDA receptors in V1, and there is little expression of GluN2A or the receptor anchoring protein PSD95 (Murphy et al., 2005; Pinto et al., 2015; Siu et al., 2017; Siu and Murphy, 2018; Figure 2A). GluN2B declines through childhood to stable levels in adolescence while expression of PSD95 peaks in older childhood when the period for susceptibility to amblyopia ends. However, GluN2A does not dominate the GluN2A:GluN2B balance until teens to young adults (Siu et al., 2017; Siu and Murphy, 2018; Figure 2B). The gradual decline of GluN2B and subsequent increase of GluN2A suggests a prolonged period of NMDA-dependent plasticity in human V1 that may impact the threshold for synaptic modification (Philpot et al., 2007; Smith et al., 2009) and ocular dominance plasticity (Cho et al., 2009). Furthermore, in older adults, the loss of GluN2A triggers a shift in the GluN2A:GluN2B balance back to the pattern observed in childhood (Siu et al., 2017), but age-related changes in other mechanisms suggest this is not a return to child-like plasticity.
Developmental changes in GABAergic mechanisms over the first few years include loss of the presynaptic proteins VGAT and cannabinoid receptor CB1 and the postsynaptic receptor subunits GABAAα2 and GABAAα3. Over those same ages there is a gradual increase in expression of the postsynaptic receptor subunit GABAAα1 (Murphy et al., 2005; Pinto et al., 2010; Siu and Murphy, 2018; Figure 2A). The early loss of GABAAα2 causes a shift in favour of the α1 subunit (Figure 2B), which is important because the α1 subunit is necessary to engage experience-dependent plasticity in V1 (Fagiolini et al., 2004). In monkey V1, the shift from more α2 to more α1 is completed during early development (Hendrickson et al., 1994). However, in humans, the expression of α2 continues to decline across the lifespan, and the shift to α1 is more gradual (Pinto et al., 2010). Other GABAergic mechanisms also have prolonged development into older childhood, adolescence and adulthood. Gephyrin (GPHN) anchors GABAA receptors at the synapse, regulates the number of excitatory and inhibitory synapses, GABAergic synapse plasticity and the physiological E-I balance (Prange et al., 2004; Lardi-Studler et al., 2007; Keith and El-Husseini, 2008; Tyagarajan and Fritschy, 2014). Gephyrin peaks from adolescence to adulthood before declining into aging (Pinto et al., 2010). The enzyme that makes the basal pool of GABA, GAD67, is relatively constant, but the enzyme that makes the on-demand pool of GABA, GAD65, increases gradually until young adulthood before declining during aging of human V1 (Pinto et al., 2010). Together, these identify both pre- and post-synaptic losses of GABAergic signaling in aging human V1. These losses may contribute to changes in visual perception (Owsley, 2011) since the age-related loss of GABA in monkey V1 underlies poor orientation tuning (Leventhal et al., 2003).
There are lifelong changes in the expression of PSD95 and gephyrin (GPHN), two of the mechanisms regulating the E-I balance (Keith and El-Husseini, 2008). In infancy, gephyrin dominates the E-I balance (PSD95:GPHN), followed by a gradual shift to roughly equal expression of PSD95 and gephyrin in adults, then in aging, a shift back to more gephyrin (Figure 2B). The changes in the ratio of PSD95:gephyrin suggest that the E-I balance is continuously shifting and changing its contribution to plasticity in human V1 across the lifespan (Figure 2B).
Developmental changes in the presynaptic proteins synapsin (SYN1) and synaptophysin (SYP) and the dendritic spine marker drebrin A (DBN1) provide additional insights into synapse development in human V1. Both synapsin and synaptophysin change through infancy and childhood but in opposite ways; synapsin increases while synaptophysin decreases through infancy before rebounding in older childhood. However, neither of these synapse markers follows the trajectory described by electron microscopy studies that counted synapses (Huttenlocher et al., 1982). Furthermore, drebrin (DBN1), which is found in dendritic spines at excitatory synapses and supports homeostatic plasticity (Aoki et al., 2009; Takahashi and Naito, 2017), gradually increases across the lifespan, pointing to lifelong synaptic changes i human V1.
The development of a few other plasticity-related proteins has been studied in human V1. One of these mechanisms is the E3 ubiquitin ligase UBE3A, which controls ARC’s degradation and regulates AMPA receptor internalization (Greer et al., 2010). Knocking out UBE3A in mouse V1 leads to a loss of experience-dependent plasticity, leaving connections rigid and unable to be fine-tuned by visual experience (Yashiro et al., 2009). In human V1, the expression of UBE3A is highest in infancy, with a smaller peak in older childhood and adolescence, followed by a steady decline into aging (Williams et al., 2010). Another mechanism that regulates the expression of AMPA receptors, beta3 integrins (ITGB3) (Cingolani et al., 2008; McGeachie et al., 2011), has a pattern of expression similar to UBE3A, suggesting that age-related declines in these mechanisms may lead to endocytosis of AMPA receptors that reduce synaptic scaling plasticity. In addition, a glial mechanism known to reduce experience-dependent plasticity, cortical myelin (classic myelin basic protein MBP) (McGee et al., 2005), gradually increases in human V1 into the fourth decade of life (Siu et al., 2015). These 3 proteins contribute to the brakes on plasticity, and their prolonged changes suggest a gradual increase in the brakes and loss of the potential for experience-dependent plasticity in human V1.
Figure 2 summarizes the changes in the proteins and balances across the lifespan. It is clear that this collection of plasticity-related proteins has peaks at different ages across the lifespan (Figure 2A). The balances also show this pattern of both early (GluA2:GluN1) and prolonged changes [E-I (PSD95:GPHN)]. These findings underscore that multiple timescales and gradual shifts in plasticity mechanisms across the lifespan characterize human V1 development.
This review covers prenatal and postnatal development of anatomical and molecular features in human V1. Studies using various neurobiological techniques show that human V1 develops and changes over multiple timescales from the first trimester to aging, so whether human V1 is described as developing early or later depends on what is studied. Importantly, prolonged changes are found for many of the proteins that regulate plasticity, suggesting that some forms of experience-dependent plasticity in human V1 extend past childhood. Only a handful of plasticity mechanisms have been studied, so much is still unknown about the neural mechanisms that regulate plasticity in human V1.
One aspect of human V1 development that has not been explored is the impact of host factors, such as sex, genetic ancestry, and socioeconomic status. However, brain banks have small numbers of cases with limited diversity, making it challenging to do those studies. Still, it is crucial to understand the impact of host factor diversity on human V1 since those factors may account for developmental variability and the timing of plasticity.
The methods used to study the anatomy and molecular environment of human V1 continue to identify new features of its development. Molecular tools have revealed distinct aspects of human V1 (Kang et al., 2011; Carlyle et al., 2017; Jorstad et al., 2023; Wang et al., 2024) and its relationship to neurodevelopmental disorders (Gandal et al., 2022). The next wave of single-cell and spatial transcriptomic tools is poised to uncover new details about how the human cortex develops (Velmeshev et al., 2023; Qian et al., 2024). We end with a reminder from Cajal’s words about the importance of the methods for studying human V1:
The minute anatomy of the visual cortex (region of the calcarine fissure, sulcus lobulus lingualis) has already been explored by several investigators, among whom we may make particular mention of Meynert et al. But their very incomplete researches have been performed by such insufficient methods as staining with carmine, the Weigert-Pall method, or that of Nissl with basic anilines – methods which, as is well known, do not suffice at all to demonstrate the total morphology of the elements and the organization of the most delicate nerve plexuses (Cajal, 1899).
KM: Conceptualization, Visualization, Writing – original draft, Writing – review and editing. LM: Conceptualization, Visualization, Writing – original draft, Writing – review and editing.
The author(s) declare financial support was received for the research, authorship, and/or publication of this article. The research was funded by the NSERC Grant RGPIN-2020-06403, awarded to KM, and NSERC CGS-D, awarded to LM. The funder had no role in study design, data collection and analysis, publication decisions, or manuscript preparation.
We recognize that our McMaster University laboratory is located on the traditional territories of the Mississauga and Haudenosaunee nations and within the lands protected by the “Dish with One Spoon” wampum agreement. We thank Maheshwar Panday for help with figure design.
The authors declare that the research was conducted in the absence of any commercial or financial relationships that could be construed as a potential conflict of interest.
The author(s) declared that they were an editorial board member of Frontiers, at the time of submission. This had no impact on the peer review process and the final decision.
All claims expressed in this article are solely those of the authors and do not necessarily represent those of their affiliated organizations, or those of the publisher, the editors and the reviewers. Any product that may be evaluated in this article, or claim that may be made by its manufacturer, is not guaranteed or endorsed by the publisher.
Albin, R. L., Sakurai, S. Y., Makowiec, R. L., Higgins, D. S., Young, A. B., and Penny, J. B. (1991). Excitatory amino acid, GABAA, and GABAB binding sites in human striate cortex. Cereb. Cortex 1, 499–509. doi: 10.1093/cercor/1.6.499
Alnćs, D., Kaufmann, T., Marquand, A. F., Smith, S. M., and Westlye, L. T. (2020). Patterns of sociocognitive stratification and perinatal risk in the child brain. Proc. Natl. Acad. Sci. U.S.A. 117, 12419–12427. doi: 10.1073/pnas.2001517117
Andersen, D. L., Tannenberg, A. E. G., Burke, C. J., and Dodd, P. R. (1995). Developmental rearrangements of cortical glutamate-NMDA receptor binding sites in late human gestation. Dev. Brain Res. 88, 178–185. doi: 10.1016/0165-3806(95)00101-i
Aoki, C., Kojima, N., Sabaliauskas, N., Shah, L., Ahmed, T. H., Oakford, J., et al. (2009). Drebrin a knockout eliminates the rapid form of homeostatic synaptic plasticity at excitatory synapses of intact adult cerebral cortex. J. Comp. Neurol. 517, 105–121. doi: 10.1002/cne.22137
Arellano, J. I., Morozov, Y. M., Micali, N., and Rakic, P. (2021). Radial glial cells: New views on old questions. Neurochem. Res. 46, 2512–2524. doi: 10.1007/s11064-021-03296-z
Balaram, P., Kaas, J., and Young, N. (2014). Histological features of layers and sublayers in cortical visual areas V1 and V2 of chimpanzees, macaque monkeys, and humans. Eye Brain 6, 5–18. doi: 10.2147/eb.s51814
Balsor, J. L., Arbabi, K., Singh, D., Kwan, R., Zaslavsky, J., Jeyanesan, E., et al. (2021). A practical guide to sparse k-means clustering for studying molecular development of the human brain. Front. Neurosci. 15:668293. doi: 10.3389/fnins.2021.668293
Balsor, J. L., Jones, D. G., and Murphy, K. M. (2019). Classification of visual cortex plasticity phenotypes following treatment for amblyopia. Neural Plast. 2019, 2564018–2564023. doi: 10.1155/2019/2564018
Beaulieu, C., Kisvarday, Z., Somogyi, P., Cynader, M., and Cowey, A. (1992). Quantitative distribution of GABA-immunopositive and-immunonegative neurons and synapses in the monkey striate cortex (area 17). Cereb. Cortex 2, 295–309.
Becker, L. E., Armstrong, D. L., Chan, F., and Wood, M. M. (1984). Dendritic development in human occipital cortical neurons. Dev. Brain Res. 315, 117–124. doi: 10.1016/0165-3806(84)90083-x
Benfey, N., Foubert, D., and Ruthazer, E. S. (2022). Glia regulate the development, function, and plasticity of the visual system from retina to cortex. Front. Neural Circuits 16:826664. doi: 10.3389/fncir.2022.826664
Berardi, N., Pizzorusso, T., and Maffei, L. (2004). Extracellular matrix and visual cortical plasticity freeing the synapse. Neuron 44, 905–908. doi: 10.1016/j.neuron.2004.12.008
Berardi, N., Pizzorusso, T., Ratto, G. M., and Maffei, L. (2003). Molecular basis of plasticity in the visual cortex. Trends Neurosci. 26, 369–378. doi: 10.1016/s0166-2236(03)00168-1
Beston, B. R., Jones, D. G., and Murphy, K. M. (2010). Experience-dependent changes in excitatory and inhibitory receptor subunit expression in visual cortex. Front. Synapt. Neurosci. 2:138. doi: 10.3389/fnsyn.2010.00138
Bhardwaj, R. D., Curtis, M. A., Spalding, K. L., Buchholz, B. A., Fink, D., Björk-Eriksson, T., et al. (2006). Neocortical neurogenesis in humans is restricted to development. Proc. Natl. Acad. Sci. U.S.A. 103, 12564–12568. doi: 10.1073/pnas.0605177103
Burkhalter, A. (1993). Development of forward and feedback connections between areas V1 and V2 of human visual cortex. Cereb. Cortex 3, 476–487. doi: 10.1093/cercor/3.5.476
Burkhalter, A., Bernardo, K. L., and Charles, V. (1993). Development of local circuits in human visual cortex. J. Neurosci. 13, 1916–1931.
Bystron, I., Blakemore, C., and Rakic, P. (2008). Development of the human cerebral cortex: Boulder Committee revisited. Nat. Rev. Neurosci. 9, 110–122. doi: 10.1038/nrn2252
Cajal, S. R. (1899). Comparative study of the sensory areas of the human cortex. Worcester, MA: Clark University.
Cao, Q. L., Yan, X. X., Luo, X. G., and Garey, L. J. (1996). Prenatal development of parvalbumin immunoreactivity in the human striate cortex. Cereb. Cortex 6, 620–630. doi: 10.1093/cercor/6.4.620
Capogna, M., Castillo, P. E., and Maffei, A. (2021). The ins and outs of inhibitory synaptic plasticity: Neuron types, molecular mechanisms and functional roles. Eur. J. Neurosci. 54, 6882–6901. doi: 10.1111/ejn.14907
Carlyle, B. C., Kitchen, R. R., Kanyo, J. E., Voss, E. Z., Pletikos, M., Sousa, A. M. M., et al. (2017). A multiregional proteomic survey of the postnatal human brain. Nat. Neurosci. 20, 1–15. doi: 10.1038/s41593-017-0011-2
Carmignoto, G., and Vicini, S. (1992). Activity-dependent decrease in NMDA receptor responses during development of the visual cortex. Science 258, 1007–1011. doi: 10.1126/science.1279803
Castaldi, E., Lunghi, C., and Morrone, M. C. (2020). Neuroplasticity in adult human visual cortex. Neurosci. Biobehav. Rev. 112, 542–552. doi: 10.1016/j.neubiorev.2020.02.028
Chen, L. L., Yang, C. C., and Mower, G. D. G. (2001). Developmental changes in the expression of GABA(A) receptor subunits (alpha(1), alpha(2), alpha(3)) in the cat visual cortex and the effects of dark rearing. Mol. Brain Res. 88, 135–143. doi: 10.1016/s0169-328x(01)00042-0
Chen, X., Fischer, S., Rue, M. C. P., Zhang, A., Mukherjee, D., Kanold, P. O., et al. (2024). Whole-cortex in situ sequencing reveals input-dependent area identity. Nature 56, 1–10. doi: 10.1038/s41586-024-07221-6
Cherubini, E., and Conti, F. (2001). Generating diversity at GABAergic synapses. Trends Neurosci. 24, 155–162.
Cho, K. K. A., Khibnik, L., Philpot, B. D., and Bear, M. F. (2009). The ratio of NR2A/B NMDA receptor subunits determines the qualities of ocular dominance plasticity in visual cortex. Proc. Natl. Acad. Sci. U.S.A. 106, 5377–5382. doi: 10.1073/pnas.0808104106
Cingolani, L. A., Thalhammer, A., Yu, L. M. Y., Catalano, M., Ramos, T., Colicos, M. A., et al. (2008). Activity-dependent regulation of synaptic AMPA receptor composition and abundance by β3 integrins. Neuron 58, 749–762. doi: 10.1016/j.neuron.2008.04.011
Clowry, G., Molnár, Z., and Rakic, P. (2010). Renewed focus on the developing human neocortex. J. Anat. 217, 276–288. doi: 10.1111/j.1469-7580.2010.01281.x
Conel, J. L. (1939). The cortex of the newborn. Cambridge, MA: Harvard University Press, 58–60. doi: 10.4159/harvard.9780674187641.c12
Conel, J. L. (1941). The cortex of the one-month infant. Cambridge, MA: Harvard University Press, 145–146. doi: 10.4159/harvard.9780674187658.c39
Conel, J. L. (1947). The cortex of the three-month infant. Cambridge, MA: Harvard University Press, 3–3. doi: 10.4159/harvard.9780674187672.intro
Conel, J. L. (1951). The cortex of the six-month infant. Cambridge, MA: Harvard University Press, 123–126. doi: 10.4159/harvard.9780674187689.c21
Conel, J. L. (1955). The cortex of the fifteen-month infant. Cambridge, MA: Harvard University Press, 121–125. doi: 10.4159/harvard.9780674187696.c16
Conel, J. L. (1959). The cortex of the twenty-four-month infant. Cambridge, MA: Harvard University Press, 165–170. doi: 10.4159/harvard.9780674187702.c13
Conel, J. L. (1963). The cortex of the four-year child. Cambridge, MA: Harvard University Press, 188–195. doi: 10.4159/harvard.9780674187719.c17
Conel, J. L. (1967). The cortex of the six-year child. Cambridge, MA: Harvard University Press, 113–179. doi: 10.4159/harvard.9780674187733.c3
Eickhoff, S. B., Rottschy, C., and Zilles, K. (2007). Laminar distribution and co-distribution of neurotransmitter receptors in early human visual cortex. Brain Struct. Funct. 212, 255–267. doi: 10.1007/s00429-007-0156-y
Eickhoff, S. B., Rottschy, C., Kujovic, M., Palomero-Gallagher, N., and Zilles, K. (2008). Organizational principles of human visual cortex revealed by receptor mapping. Cereb. Cortex 18, 2637–2645. doi: 10.1093/cercor/bhn024
Fagiolini, M., and Hensch, T. K. (2000). Inhibitory threshold for critical-period activation in primary visual cortex. Nature 404, 183–186. doi: 10.1038/35004582
Fagiolini, M., Fritschy, J.-M., Löw, K., Möhler, H., Rudolph, U., and Hensch, T. K. (2004). Specific GABAA circuits for visual cortical plasticity. Science 303, 1681–1683. doi: 10.1126/science.1091032
Fagiolini, M., Katagiri, H., Miyamoto, H., Mori, H., Grant, S. G. N., Mishina, M., et al. (2003). Separable features of visual cortical plasticity revealed by N-methyl-D-aspartate receptor 2A signaling. Proc. Natl. Acad. Sci. U.S.A. 100, 2854–2859. doi: 10.1073/pnas.0536089100
Flower, M. J. (1985). Neuromaturation of the human fetus. J. Med. Philos. 10, 237–251. doi: 10.1093/jmp/10.3.237
Frank, S. M., Becker, M., Qi, A., Geiger, P., Frank, U. I., Rosedahl, L. A., et al. (2022). Efficient learning in children with rapid GABA boosting during and after training. Curr. Biol. 32:5022–5030.e7. doi: 10.1016/j.cub.2022.10.021
Gainey, M. A., Hurvitz-Wolff, J. R., Lambo, M. E., and Turrigiano, G. G. (2009). Synaptic scaling requires the GluR2 subunit of the AMPA receptor. J. Neurosci. 29, 6479–6489. doi: 10.1523/jneurosci.3753-08.2009
Gandal, M. J., Haney, J. R., Wamsley, B., Yap, C. X., Parhami, S., Emani, P. S., et al. (2022). Broad transcriptomic dysregulation occurs across the cerebral cortex in ASD. Nature 611, 532–539. doi: 10.1038/s41586-022-05377-7
Gennatas, E. D., Avants, B. B., Wolf, D. H., Satterthwaite, T. D., Ruparel, K., Ciric, R., et al. (2017). Age-related effects and sex differences in gray matter density, volume, mass, and cortical thickness from childhood to young adulthood. J. Neurosci. 37, 5065–5073. doi: 10.1523/jneurosci.3550-16.2017
Gingrich, K. J., Roberts, W. A., and Kass, R. S. (1995). Dependence of the GABAA receptor gating kinetics on the alpha-subunit isoform: Implications for structure-function relations and synaptic transmission. J. Physiol. 489, 529–543. doi: 10.1113/jphysiol.1995.sp021070
Godovalova, O. S. (2010). Expression of nervous tissue nuclear protein and glial fibrillary acidic protein during morphogenesis of the neocortex. Bull. Exp. Biol. Med. 149, 655–658. doi: 10.1007/s10517-010-1017-x
Gray, N. W., Weimer, R. M., Bureau, I., and Svoboda, K. (2006). Rapid redistribution of synaptic PSD-95 in the neocortex in vivo. PLoS Biol. 4:e370. doi: 10.1371/journal.pbio.0040370
Greer, P. L., Hanayama, R., Bloodgood, B. L., Mardinly, A. R., Lipton, D. M., Flavell, S. W., et al. (2010). The Angelman syndrome protein Ube3A regulates synapse development by ubiquitinating arc. Cell 140, 704–716. doi: 10.1016/j.cell.2010.01.026
Grieco, S. F., Holmes, T. C., and Xu, X. (2019). Neuregulin directed molecular mechanisms of visual cortical plasticity. J. Comp. Neurol. 527, 668–678. doi: 10.1002/cne.24414
Heinen, K., Bosman, L. W. J., Spijker, S., Pelt, J., van, Smit, A. B., et al. (2004). Gabaa receptor maturation in relation to eye opening in the rat visual cortex. Neuroscience 124, 161–171. doi: 10.1016/j.neuroscience.2003.11.004
Hendrickson, A., March, D., Richards, G., Erickson, A., and Shaw, C. (1994). Coincidental appearance of the α1 subunit of the gaba-a receptor and the type ibenzodiazepine receptor near birth in macaque monkey visual cortex. Int. J. Dev. Neurosci. 12, 299–314. doi: 10.1016/0736-5748(94)90078-7
Hendry, S., Huntsman, M., Vinuela, A., Mohler, H., Blas, A., and de, et al. (1994). GABAA receptor subunit immunoreactivity in primate visual cortex: Distribution in macaques and humans and regulation by visual input in adulthood. J. Neurosci. 14, 2383–2401. doi: 10.1523/jneurosci.14-04-02383.1994
Hensch, T. K. (2004). Critical period regulation. Annu. Rev. Neurosci. 27, 549–579. doi: 10.1146/annurev.neuro.27.070203.144327
Hensch, T. K. (2005). Critical period plasticity in local cortical circuits. Nat. Rev. Neurosci. 6, 877–888. doi: 10.1038/nrn1787
Hensch, T. K., and Fagiolini, M. (2005). Excitatory-inhibitory balance and critical period plasticity in developing visual cortex. Prog. Brain Res. 147, 115–124. doi: 10.1016/s0079-6123(04)47009-5
Hensch, T. K., and Quinlan, E. M. (2018). Critical periods in amblyopia. Vis. Neurosci. 35:E014. doi: 10.1017/s0952523817000219
Hensch, T., Stryker, M., and Kash, S. (1998). Local GABA circuit control of experience-dependent plasticity in developing visual cortex. Science 282, 1504–1508. doi: 10.1126/science.282.5393.1504
Heynen, A. J., Yoon, B.-J., Liu, C.-H., Chung, H. J., Huganir, R. L., and Bear, M. F. (2003). Molecular mechanism for loss of visual cortical responsiveness following brief monocular deprivation. Nat. Neurosci. 6, 854–862. doi: 10.1038/nn1100
Honig, L. S., Herrmann, K., and Shatz, C. J. (1996). Developmental changes revealed by immunohistochemical markers in human cerebral cortex. Cereb. Cortex 6, 794–806. doi: 10.1093/cercor/6.6.794
Huang, X., Stodieck, S. K., Goetze, B., Cui, L., Wong, M. H., Wenzel, C., et al. (2015). Progressive maturation of silent synapses governs the duration of a critical period. Proc. Natl. Acad. Sci. U.S.A. 112, E3131–E3140. doi: 10.1073/pnas.1506488112
Huang, Z. J., Kirkwood, A., Pizzorusso, T., Porciatti, V., Morales, B., Bear, M. F., et al. (1999). BDNF regulates the maturation of inhibition and the critical period of plasticity in mouse visual cortex. Cell 98, 739–755. doi: 10.1016/s0092-8674(00)81509-3
Huntley, G. W., Vickers, J. C., Janssen, W., Brose, N., Heinemann, S. F., and Morrison, J. H. (1994). Distribution and synaptic localization of immunocytochemically identified NMDA receptor subunit proteins in sensory-motor and visual cortices of monkey and human. J. Neurosci. 14, 3603–3619. doi: 10.1523/jneurosci.14-06-03603.1994
Huttenlocher, P. R. (1999). Dendritic and synaptic development in human cerebral cortex: Time course and critical periods. Dev. Neuropsychol. 16, 347–349. doi: 10.1207/s15326942dn1603_12
Huttenlocher, P. R., and Dabholkar, A. S. (1997). Regional differences in synaptogenesis in human cerebral cortex. J. Comp. Neurol. 387, 167–178. doi: 10.1002/(sici)1096-9861(19971020)387:2<167::aid-cne1>3.0.co;2-z
Huttenlocher, P. R., Courten, C., de Garey, L. J., and Loos, H. V. (1982). Synaptogenesis in human visual cortex–evidence for synapse elimination during normal development. Neurosci. Lett. 33, 247–252. doi: 10.1016/0304-3940(82)90379-2
Iwai, Y., Fagiolini, M., Obata, K., and Hensch, T. K. (2003). Rapid critical period induction by tonic inhibition in visual cortex. J. Neurosci. 23, 6695–6702. doi: 10.1523/jneurosci.23-17-06695.2003
Jones, E. G. (1993). GABAergic neurons and their role in cortical plasticity in primates. Cereb. Cortex 3, 361–372. doi: 10.1093/cercor/3.5.361-a
Jorstad, N. L., Close, J., Johansen, N., Yanny, A. M., Barkan, E. R., Travaglini, K. J., et al. (2023). Transcriptomic cytoarchitecture reveals principles of human neocortex organization. Science 382:eadf6812. doi: 10.1126/science.adf6812
Judaš, M., Sedmak, G., Pletikos, M., and Jovanov-Milošević, N. (2010). Populations of subplate and interstitial neurons in fetal and adult human telencephalon. J. Anat. 217, 381–399. doi: 10.1111/j.1469-7580.2010.01284.x
Kang, H. J., Kawasawa, Y. I., Cheng, F., Zhu, Y., Xu, X., Li, M., et al. (2011). Spatio-temporal transcriptome of the human brain. Nature 478, 483–489. doi: 10.1038/nature10523
Keith, D., and El-Husseini, A. (2008). Excitation control: Balancing PSD-95 function at the synapse. Front. Mol. Neurosci. 1:4. doi: 10.3389/neuro.02.004.2008
Kirkwood, A., and Bear, M. (1994). Hebbian synapses in visual cortex. J. Neurosci. 14, 1634–1645. doi: 10.1523/jneurosci.14-03-01634.1994
Kleinschmidt, A., Bear, M. F., and Singer, W. (1987). Blockade of “NMDA” receptors disrupts experience-dependent plasticity of kitten striate cortex. Science 238, 355–358.
Kostović, I., and Judaš, M. (2007). Transient patterns of cortical lamination during prenatal life: Do they have implications for treatment? Neurosci. Biobehav. Rev. 31, 1157–1168. doi: 10.1016/j.neubiorev.2007.04.018
Kostovic, I., and Rakic, P. (1980). Cytology and time of origin of interstitial neurons in the white matter in infant and adult human and monkey telencephalon. J. Neurocytol. 9, 219–242. doi: 10.1007/bf01205159
Kostovic, I., and Rakic, P. (1990). Developmental history of the transient subplate zone in the visual and somatosensory cortex of the macaque monkey and human brain. J. Comp. Neurol. 297, 441–470. doi: 10.1002/cne.902970309
Kostović, I., Jovanov-Milošević, N., Radoš, M., Sedmak, G., Benjak, V., Kostović-Srzentić, M., et al. (2014). Perinatal and early postnatal reorganization of the subplate and related cellular compartments in the human cerebral wall as revealed by histological and MRI approaches. Brain Struct. Funct. 219, 231–253. doi: 10.1007/s00429-012-0496-0
Kurcyus, K., Annac, E., Hanning, N. M., Harris, A. D., Oeltzschner, G., Edden, R., et al. (2018). Opposite dynamics of GABA and glutamate levels in the occipital cortex during visual processing. J. Neurosci. 38, 9967–9976. doi: 10.1523/jneurosci.1214-18.2018
Lambo, M. E., and Turrigiano, G. G. (2013). Synaptic and intrinsic homeostatic mechanisms cooperate to increase L2/3 pyramidal neuron excitability during a late phase of critical period plasticity. J. Neurosci. 33, 8810–8819. doi: 10.1523/jneurosci.4502-12.2013
Lardi-Studler, B., Smolinsky, B., Petitjean, C. M., Koenig, F., Sidler, C., Meier, J. C., et al. (2007). Vertebrate-specific sequences in the gephyrin E-domain regulate cytosolic aggregation and postsynaptic clustering. J. Cell Sci. 120, 1371–1382. doi: 10.1242/jcs.003905
Laurie, D., Wisden, W., and Seeburg, P. (1992). The distribution of thirteen GABAA receptor subunit mRNAs in the rat brain. III. Embryonic and postnatal development. J. Neurosci. 12, 4151–4172. doi: 10.1523/jneurosci.12-11-04151.1992
Leonard, J. A., Romeo, R. R., Park, A. T., Takada, M. E., Robinson, S. T., Grotzinger, H., et al. (2019). Associations between cortical thickness and reasoning differ by socioeconomic status in development. Dev. Cogn. Neurosci. 36:100641. doi: 10.1016/j.dcn.2019.100641
Letinic, K., and Kostovic, I. (1998). Postnatal development of calcium-binding proteins calbindin and parvalbumin in human visual cortex. Cereb. Cortex 8, 660–669. doi: 10.1093/cercor/8.7.660
Levelt, C. N., and Hübener, M. (2012). Critical-period plasticity in the visual cortex. Annu. Rev. Neurosci. 35, 309–330. doi: 10.1146/annurev-neuro-061010-113813
Leventhal, A. G., Wang, Y., Pu, M., Zhou, Y., and Ma, Y. (2003). GABA and its agonists improved visual cortical function in senescent monkeys. Science 300, 812–815. doi: 10.1126/science.1082874
Lidow, M. S., Goldman-Rakic, P. S., and Rakic, P. (1991). Synchronized overproduction of neurotransmitter receptors in diverse regions of the primate cerebral cortex. Proc. Natl. Acad. Sci. U.S.A. 88, 10218–10221.
Lin, Y., Stephenson, M. C., Xin, L., Napolitano, A., and Morris, P. G. (2012). Investigating the metabolic changes due to visual stimulation using functional proton magnetic resonance spectroscopy at 7 T. J. Cereb. Blood Flow Metab. 32, 1484–1495. doi: 10.1038/jcbfm.2012.33
Lunghi, C., Emir, U. E., Morrone, M. C., and Bridge, H. (2015). Short-term monocular deprivation alters GABA in the adult human visual cortex. Curr. Biol. 25, 1496–1501. doi: 10.1016/j.cub.2015.04.021
Mackey, A. P., Finn, A. S., Leonard, J. A., Jacoby-Senghor, D. S., West, M. R., Gabrieli, C. F. O., et al. (2015). Neuroanatomical correlates of the income-achievement gap. Psychol. Sci. 26, 925–933. doi: 10.1177/0956797615572233
Maffei, A., and Turrigiano, G. (2008). The age of plasticity: Developmental regulation of synaptic plasticity in neocortical microcircuits. Prog. Brain Res. 169, 211–223. doi: 10.1016/s0079-6123(07)00012-x
Maffei, A., Nelson, S. B., and Turrigiano, G. G. (2004). Selective reconfiguration of layer 4 visual cortical circuitry by visual deprivation. Nat. Neurosci. 7, 1353–1359. doi: 10.1038/nn1351
McGeachie, A. B., Cingolani, L. A., and Goda, Y. (2011). A stabilising influence: Integrins in regulation of synaptic plasticity. Neurosci. Res. 70, 24–29. doi: 10.1016/j.neures.2011.02.006
McGee, A. W., Yang, Y., Fischer, Q. S., Daw, N. W., and Strittmatter, S. M. (2005). Experience-driven plasticity of visual cortex limited by myelin and Nogo receptor. Science 309, 2222–2226. doi: 10.1126/science.1114362
Michel, A. E., and Garey, L. J. (1984). The development of dendritic spines in the human visual cortex. Hum. Neurobiol. 3, 223–227.
Miller, J. A., Ding, S.-L., Sunkin, S. M., Smith, K. A., Ng, L., Szafer, A., et al. (2014). Transcriptional landscape of the prenatal human brain. Nature 508, 199–206. doi: 10.1038/nature13185
Molnár, Z., Clowry, G. J., Šestan, N., Alzu’bi, A., Bakken, T., Hevner, R. F., et al. (2019). New insights into the development of the human cerebral cortex. J. Anat. 235, 432–451. doi: 10.1111/joa.13055
Monier, A., Adle-Biassette, H., Delezoide, A.-L., Evrard, P., Gressens, P., and Verney, C. (2007). Entry and distribution of microglial cells in human embryonic and fetal cerebral cortex. J. Neuropathol. Exp. Neurol. 66, 372–382. doi: 10.1097/nen.0b013e3180517b46
Monier, A., Evrard, P., Gressens, P., and Verney, C. (2006). Distribution and differentiation of microglia in the human encephalon during the first two trimesters of gestation. J. Comp. Neurol. 499, 565–582. doi: 10.1002/cne.21123
Monyer, H., Burnashev, N., Laurie, D. J., Sakmann, B., and Seeburg, P. H. (1994). Developmental and regional expression in the rat brain and functional properties of four NMDA receptors. Neuron 12, 529–540. doi: 10.1016/0896-6273(94)90210-0
Morales, B., Choi, S.-Y., and Kirkwood, A. (2002). Dark rearing alters the development of GABAergic transmission in visual cortex. J. Neurosci. 22, 8084–8090. doi: 10.1523/jneurosci.22-18-08084.2002
Murphy, K. M., Beston, B. R., Boley, P. M., and Jones, D. G. (2005). Development of human visual cortex: A balance between excitatory and inhibitory plasticity mechanisms. Dev. Psychobiol. 46, 209–221. doi: 10.1002/dev.20053
Natu, V. S., Rosenke, M., Wu, H., Querdasi, F. R., Kular, H., Lopez-Alvarez, N., et al. (2021). Infants’ cortex undergoes microstructural growth coupled with myelination during development. Commun. Biol. 4:1191. doi: 10.1038/s42003-021-02706-w
Nowakowski, T. J., Bhaduri, A., Pollen, A. A., Alvarado, B., Mostajo-Radji, M. A., Lullo, E. D., et al. (2017). Spatiotemporal gene expression trajectories reveal developmental hierarchies of the human cortex. Science 358, 1318–1323. doi: 10.1126/science.aap8809
Nowakowski, T. J., Pollen, A. A., Sandoval-Espinosa, C., and Kriegstein, A. R. (2016). Transformation of the radial glia scaffold demarcates two stages of human cerebral cortex development. Neuron 91, 1219–1227. doi: 10.1016/j.neuron.2016.09.005
Petanjek, Z., Banovac, I., Sedmak, D., and Hladnik, A. (2023). Dendritic spines, structure, function, and plasticity. Adv. Neurobiol. 34, 143–221. doi: 10.1007/978-3-031-36159-3_4
Philpot, B. D., Cho, K. K. A., and Bear, M. F. (2007). Obligatory role of NR2A for metaplasticity in visual cortex. Neuron 53, 495–502. doi: 10.1016/j.neuron.2007.01.027
Philpot, B. D., Espinosa, J. S., and Bear, M. F. (2003). Evidence for altered NMDA receptor function as a basis for metaplasticity in visual cortex. J. Neurosci. 23, 5583–5588.
Pinto, J. G. A., Hornby, K. R., Jones, D. G., and Murphy, K. M. (2010). Developmental changes in GABAergic mechanisms in human visual cortex across the lifespan. Front. Cell. Neurosci. 4:16. doi: 10.3389/fncel.2010.00016
Pinto, J. G. A., Jones, D. G., Williams, C. K., and Murphy, K. M. (2015). Characterizing synaptic protein development in human visual cortex enables alignment of synaptic age with rat visual cortex. Front. Neural Circuit 9:3. doi: 10.3389/fncir.2015.00003
Pitchaimuthu, K., Wu, Q., Carter, O., Nguyen, B. N., Ahn, S., Egan, G. F., et al. (2017). Occipital GABA levels in older adults and their relationship to visual perceptual suppression. Sci. Rep. 7:14231. doi: 10.1038/s41598-017-14577-5
Pouille, F., and Scanziani, M. (2001). Enforcement of temporal fidelity in pyramidal cells by somatic feed-forward inhibition. Science 293, 1159–1163. doi: 10.1126/science.1060342
Prange, O., Wong, T. P., Gerrow, K., Wang, Y. T., and El-Husseini, A. (2004). A balance between excitatory and inhibitory synapses is controlled by PSD-95 and neuroligin. Proc. Natl. Acad. Sci. U.S.A. 101, 13915–13920. doi: 10.1073/pnas.0405939101
Pribiag, H., and Stellwagen, D. (2014). Neuroimmune regulation of homeostatic synaptic plasticity. Neuropharmacology 78, 13–22. doi: 10.1016/j.neuropharm.2013.06.008
Priebe, N. J., and McGee, A. W. (2014). Mouse vision as a gateway for understanding how experience shapes neural circuits. Front. Neural Circuits 8:123. doi: 10.3389/fncir.2014.00123
Pritchett, D. B., Sontheimer, H., Shivers, B. D., Ymer, S., Kettenmann, H., Schofield, P. R., et al. (1989). Importance of a novel GABAA receptor subunit for benzodiazepine pharmacology. Nature 338, 582–585. doi: 10.1038/338582a0
Qian, X., Coleman, K., Jiang, S., Kriz, A. J., Marciano, J. H., Luo, C., et al. (2024). Spatial Single-cell Analysis Decodes Cortical Layer and Area Specification. bioRxiv [Preprint]. doi: 10.1101/2024.06.05.597673 bioRxiv: 2024.06.05.597673.
Quinlan, E. M., Philpot, B. D., Huganir, R. L., and Bear, M. F. (1999). Rapid, experience-dependent expression of synaptic NMDA receptors in visual cortex in vivo. Nat. Neurosci. 2, 352–357. doi: 10.1038/7263
Rakic, P. (1972). Mode of cell migration to the superficial layers of fetal monkey neocortex. J. Comp. Neurol. 145, 61–83. doi: 10.1002/cne.901450105
Rakic, P. (1974). Neurons in rhesus monkey visual cortex: Systematic relation between time of origin and eventual disposition. Science 183, 425–427. doi: 10.1126/science.183.4123.425
Ramoa, A. S., Mower, A. F., Liao, D., and Jafri, S. I. (2001). Suppression of cortical NMDA receptor function prevents development of orientation selectivity in the primary visual cortex. J. Neurosci. 21, 4299–4309.
Retz, W., Kornhuber, J., and Riederer, P. (1996). Neurotransmission and the ontogeny of human brain. J. Neural Transm. 103, 403–419. doi: 10.1007/bf01276417
Rivadulla, C. C., Sharma, J. J., and Sur, M. M. (2001). Specific roles of NMDA and AMPA receptors in direction-selective and spatial phase-selective responses in visual cortex. J. Neurosci. 21, 1710–1719.
Rumpel, S., Hatt, H., and Gottmann, K. (1998). Silent synapses in the developing rat visual cortex: Evidence for postsynaptic expression of synaptic plasticity. J. Neurosci. 18, 8863–8874.
Sadahiro, M., Sajo, M., and Morishita, H. (2016). Nicotinic regulation of experience-dependent plasticity in visual cortex. J. Physiol. 110, 29–36. doi: 10.1016/j.jphysparis.2016.11.003
Self, M. W., Kooijmans, R. N., Supèr, H., Lamme, V. A., and Roelfsema, P. R. (2012). Different glutamate receptors convey feedforward and recurrent processing in macaque V1. Proc. Natl. Acad. Sci. U.S.A. 109, 11031–11036. doi: 10.1073/pnas.1119527109/-/dcsupplemental
Sheng, M., Cummings, J., Roldan, L. A., Jan, Y. N., and Jan, L. Y. (1994). Changing subunit composition of heteromeric NMDA receptors during development of rat cortex. Nature 368, 144–147. doi: 10.1038/368144a0
Sieghart, W. (1995). Structure and pharmacology of gamma-aminobutyric acidA receptor subtypes. Pharmacol. Rev. 47, 181–234.
Sigel, E. (2002). Mapping of the benzodiazepine recognition site on GABA-A receptors. Curr. Top. Med. Chem. 2, 833–839. doi: 10.2174/1568026023393444
Siu, C. R., and Murphy, K. M. (2018). The development of human visual cortex and clinical implications. Eye Brain 10, 25–36. doi: 10.2147/eb.s130893
Siu, C. R., Balsor, J. L., Jones, D. G., and Murphy, K. M. (2015). Classic and golli myelin basic protein have distinct developmental trajectories in human visual cortex. Front. Neurosci. 9:138. doi: 10.3389/fnins.2015.00138
Siu, C. R., Beshara, S. P., Jones, D. G., and Murphy, K. M. (2017). Development of glutamatergic proteins in human visual cortex across the lifespan. J. Neurosci. 37, 6031–6042. doi: 10.1523/jneurosci.2304-16.2017
Smith, G. B., and Olsen, R. W. (1995). Functional domains of GABAA receptors. Trends Pharmacol. Sci. 16, 162–168. doi: 10.1016/s0165-6147(00)89009-4
Smith, G. B., Heynen, A. J., and Bear, M. F. (2009). Bidirectional synaptic mechanisms of ocular dominance plasticity in visual cortex. Philos. Trans. R. Soc. B Biol. Sci. 364, 357–367. doi: 10.1098/rstb.2008.0198
Sowell, E. R., Peterson, B. S., Kan, E., Woods, R. P., Yoshii, J., Bansal, R., et al. (2007). Sex differences in cortical thickness mapped in 176 healthy individuals between 7 and 87 years of age. Cereb. Cortex 17, 1550–1560. doi: 10.1093/cercor/bhl066
Stephany, C. -É, Frantz, M. G., and McGee, A. W. (2016). multiple roles for nogo receptor 1 in visual system plasticity. Neuroscience 22, 653–666. doi: 10.1177/1073858415614564
Sur, M., Nagakura, I., Chen, N., and Sugihara, H. (2013). Chapter 9 mechanisms of plasticity in the developing and adult visual cortex. Prog. Brain Res. 207, 243–254. doi: 10.1016/b978-0-444-63327-9.00002-3
Takahashi, H., and Naito, Y. (2017). Drebrin, from structure and function to physiological and pathological roles. Adv. Exp. Med. Biol. 1006, 157–181. doi: 10.1007/978-4-431-56550-5_10
Takashima, S., Chan, F., Becker, L. E., and Armstrong, D. L. (1980). Morphology of the developing visual cortex of the human infant: A quantitative and qualitative Golgi study. J. Neuropathol. Exp. Neurol. 39, 487–501. doi: 10.1097/00005072-198007000-00007
Takesian, A. E., and Hensch, T. K. (2013). Chapter 1 balancing plasticity/stability across brain development. Prog. Brain Res. 207, 3–34. doi: 10.1016/b978-0-444-63327-9.00001-1
Taymourtash, A., Schwartz, E., Nenning, K.-H., Sobotka, D., Licandro, R., Glatter, S., et al. (2022). Fetal development of functional thalamocortical and cortico–cortical connectivity. Cereb. Cortex 33, 5613–5624. doi: 10.1093/cercor/bhac446
Thompson, B., Morrone, M. C., Bex, P., Lozama, A., and Sabel, B. A. (2023). Harnessing brain plasticity to improve binocular vision in amblyopia: An evidence-based update. Eur. J. Ophthalmol. 34, 901–912. doi: 10.1177/11206721231187426
Tooley, U. A., Bassett, D. S., and Mackey, A. P. (2021). Environmental influences on the pace of brain development. Nat. Rev. Neurosci. 22, 372–384. doi: 10.1038/s41583-021-00457-5
Trepel, C., Duffy, K. R., Pegado, V. D., and Murphy, K. M. (1998). Patchy distribution of NMDAR1 subunit immunoreactivity in developing visual cortex. J. Neurosci. 18, 3404–3415.
Tropea, D., Wart, A. V., and Sur, M. (2009). Molecular mechanisms of experience-dependent plasticity in visual cortex. Nat. Neurosci. 364, 341–355. doi: 10.1038/nn1464
Tsumoto, T., and Sato, H. (1985). GABAergic inhibition and orientation selectivity of neurons in the kitten visual cortex at the time of eye opening. Vis. Res. 25, 383–388. doi: 10.1016/0042-6989(85)90063-x
Tyagarajan, S. K., and Fritschy, J.-M. (2014). Gephyrin: A master regulator of neuronal function? Nat. Rev. Neurosci. 15, 141–156. doi: 10.1038/nrn3670
Velmeshev, D., Perez, Y., Yan, Z., Valencia, J. E., Castaneda-Castellanos, D. R., Wang, L., et al. (2023). Single-cell analysis of prenatal and postnatal human cortical development. Science 382:eadf0834. doi: 10.1126/science.adf0834
Wang, L., Wang, C., Moriano, J. A., Chen, S., Zuo, G., Cebrián-Silla, A., et al. (2024). Molecular and cellular dynamics of the developing human neocortex at single-cell resolution. bioRxiv [Preprint]. doi: 10.1101/2024.01.16.575956 bioRxiv: 2024.01.16.575956.
Wijtenburg, S. A., West, J., Korenic, S. A., Kuhney, F., Gaston, F. E., Chen, H., et al. (2017). Glutamatergic metabolites are associated with visual plasticity in humans. Neurosci. Lett. 644, 30–36. doi: 10.1016/j.neulet.2017.02.020
Williams, K., Balsor, J. L., Beshara, S., Beston, B. R., Jones, D. G., and Murphy, K. M. (2015). Experience-dependent central vision deficits: Neurobiology and visual acuity. Vis. Res. 114, 68–78. doi: 10.1016/j.visres.2015.01.021
Williams, K., Irwin, D. A., Jones, D. G., and Murphy, K. M. (2010). Dramatic loss of Ube3A expression during aging of the mammalian cortex. Front. Aging Neurosci. 2:18. doi: 10.3389/fnagi.2010.00018
Wong-Riley, M. T. T., Hevner, R. F., Cutlan, R., Earnest, M., Egan, R., Frost, J., et al. (1993). Cytochrome oxidase in the human visual cortex: Distribution in the developing and the adult brain. Vis. Neurosci. 10, 41–58. doi: 10.1017/s0952523800003217
Yan, X. X., Zheng, D. S., and Garey, L. J. (1992). Prenatal development of GABA-immunoreactive neurons in the human striate cortex. Dev. Brain Res. 65, 191–204. doi: 10.1016/0165-3806(92)90179-z
Yashiro, K., and Philpot, B. D. (2008). Regulation of NMDA receptor subunit expression and its implications for LTD, LTP, and metaplasticity. Neuropharmacology 55, 1081–1094. doi: 10.1016/j.neuropharm.2008.07.046
Yashiro, K., Riday, T. T., Condon, K. H., Roberts, A. C., Bernardo, D. R., Prakash, R., et al. (2009). Ube3a is required for experience-dependent maturation of the neocortex. Nat. Neurosci. 12, 777–783. doi: 10.1038/nn.2327
Zhu, Y., Sousa, A. M. M., Gao, T., Skarica, M., Li, M., Santpere, G., et al. (2018). Spatiotemporal transcriptomic divergence across human and macaque brain development. Science 362:eaat8077. doi: 10.1126/science.aat8077
Keywords: human, visual cortex, V1, development, histology, neuroanatomy, molecular, plasticity
Citation: Murphy KM and Monteiro L (2024) Anatomical and molecular development of the human primary visual cortex. Front. Cell. Neurosci. 18:1427515. doi: 10.3389/fncel.2024.1427515
Received: 03 May 2024; Accepted: 10 September 2024;
Published: 30 September 2024.
Edited by:
Arianna Maffei, Stony Brook University, United StatesReviewed by:
Stephen D. Van Hooser, Brandeis University, United StatesCopyright © 2024 Murphy and Monteiro. This is an open-access article distributed under the terms of the Creative Commons Attribution License (CC BY). The use, distribution or reproduction in other forums is permitted, provided the original author(s) and the copyright owner(s) are credited and that the original publication in this journal is cited, in accordance with accepted academic practice. No use, distribution or reproduction is permitted which does not comply with these terms.
*Correspondence: Kathryn M. Murphy, a211cnBoeUBtY21hc3Rlci5jYQ==
Disclaimer: All claims expressed in this article are solely those of the authors and do not necessarily represent those of their affiliated organizations, or those of the publisher, the editors and the reviewers. Any product that may be evaluated in this article or claim that may be made by its manufacturer is not guaranteed or endorsed by the publisher.
Research integrity at Frontiers
Learn more about the work of our research integrity team to safeguard the quality of each article we publish.