- 1Neurobiological Research Laboratory, Section for Experimental and Clinical Otology, Department of Oto-Rhino-Laryngology, Faculty of Medicine, Medical Center – University of Freiburg, Freiburg, Germany
- 2Faculty of Biology, Bernstein Center Freiburg, University of Freiburg, Freiburg, Germany
- 3Department of Otorhinolaryngology, Faculty of Medicine, Medical Center – University of Freiburg, University of Freiburg, Freiburg, Germany
The mammalian auditory system develops a topographical representation of sound frequencies along its pathways, also called tonotopy. In contrast, sensory deprivation during early development results in no or only rudimentary tonotopic organization. This study addresses two questions: (1) How robust is the central tonotopy when hearing fails in adulthood? (2) What role does age play at time of deafness? To address these questions, we deafened young and old adult rats with previously normal hearing. One month after deafening, both groups were unilaterally supplied with cochlear implants and electrically stimulated for 2 h. The central auditory neurons, which were activated as a result of the local electrical intracochlear stimulation, were visualized using Fos staining. While the auditory system of young rats lost the tonotopic organization throughout the brainstem, the auditory system of the older rats mainly sustained its tonotopy. It can be proposed that plasticity prevails in the central auditory system of young adult rats, while network stability prevails in the brains of aging rats. Consequently, age may be an important factor in protecting a hearing-experienced adult auditory system from a rapid loss of tonotopy when suffering from acute hearing loss. Furthermore, the study provides compelling evidence that acute deafness in young adult patients should be diagnosed as early as possible to prevent maladaptation of the central auditory system and thus achieve the optimal hearing outcome with a hearing prosthesis.
1 Introduction
Around 5% of the world’s population (~430 million people) suffer from disabling hearing loss (WHO, 2024) making it the most common sensory impairment of our age. The majority of these are adults (~396 million). The prevalence of hearing loss increases with age, reaching approximately 25% for the population over 60 years of age, with an upward trend for higher age (Roth et al., 2011; Von Gablenz et al., 2017; WHO, 2024). Age-related hearing loss in humans is mostly attributed to the loss of cochlear outer hair cells and is often associated with changes in the auditory nerve and the central auditory system, among other factors (Willott, 1984; Palombi and Caspary, 1996; McFadden et al., 1997; Ingham et al., 1998; Syka, 2002; Ouda et al., 2015). Currently, this form of hearing loss is not reversible. Nevertheless, following the diagnosis of hearing loss patients can be (re)integrated into an acoustic environment through the use of hearing aids or neuroprostheses such as the cochlear implant (CI). For people with severe to profound sensorineural hearing loss, CIs can be highly beneficial, allowing for near-normal spoken language acquisition. For prelingually deaf patients best performances can be observed when CI implantation takes place during early development, specifically within the first 3 years of life (Kral and Sharma, 2012). Postlingually deaf CI patients who had normal hearing in early development can still achieve good hearing performances in adulthood, even after more than 10 to 30 years of deafness (Moon et al., 2014; Hiel et al., 2016; Medina et al., 2017; Kim et al., 2018) although they show better speech understanding with a duration of deafness less than 10 years and younger age at implantation (Kim et al., 2018). Various studies indicate that age does not influence or limit the outcome with CI in postlingual deafness (Moon et al., 2014; Hiel et al., 2016; Garcia-Iza et al., 2018). To the best of our knowledge, it is still unclear why older CI patients with reduced or altered plasticity (Burke and Barnes, 2006; Apple et al., 2017; Foscarin et al., 2017) can achieve similar or even better hearing performance than younger CI patients with presumably more plastic brains. For example, adult-deafened CI patients demonstrate enhanced performances in sound source localization in comparison to prelingually deafened, early fitted CI patients (Litovsky et al., 2012; Ehlers et al., 2017). Although one possible reason for better directional hearing in older CI patients may be the hearing experience during an early critical period resulting in the development of central binaural circuits, recent studies in an early-deafened, adult CI-fitted animal model provide important evidence that the adult, hearing-inexperienced auditory system can still develop very good directional hearing if it receives informative directional cues via the bilateral CIs from the onset of stimulation (Rosskothen-Kuhl et al., 2021; Buck et al., 2023).
The normal hearing central auditory system, from the cochlear nucleus to the auditory cortex, is organized tonotopically. This means that groups of neurons have the greatest sensitivity to a particular frequency and are arranged in a frequency-specific manner. While the traditional developmental picture assumed a predefined, hard-wired auditory brainstem that forms its tonotopy even without sensory input, more recent studies provide evidence that the precise tonotopy in the auditory system is based on the refinement of neural circuits as a result of activity-dependent synaptic plasticity and/or afferent input after the onset of hearing (Kandler et al., 2009; Mann and Kelley, 2011; Akter et al., 2018). In the case of normal hearing, an organization of the auditory system can be observed already early in life (Kandler et al., 2009). In rats, an adult-like tonotopic arrangement matures before the third week of life (Friauf, 1992). However, previous studies have shown that tonotopy is altered in the absence of auditory input. In various model organisms, early bilateral hearing loss results in the reduction or even complete loss of tonotopic order in subcortical and cortical regions (Raggio and Schreiner, 1999; Leake et al., 2008; Fallon et al., 2009; Rosskothen-Kuhl and Illing, 2012; Jakob et al., 2015; Rauch et al., 2016; Jakob et al., 2019). In rats, we have demonstrated that unilateral or bilateral neonatal deafness results in the absence of tonotopic organization along the deprived auditory pathway (Rosskothen-Kuhl and Illing, 2012; Jakob et al., 2015; Rauch et al., 2016; Rosskothen-Kuhl et al., 2018; Jakob et al., 2019).
Aim of this study was to investigate on the neuronal level why the deafened auditory system of elderly might be still able to benefit from CI supply despite an altered level of plasticity. We focused on the effect of deafness on the mature auditory brainstem and addressed two key questions: firstly, how stable is the tonotopic organization of a hearing-experienced auditory system after 1 month of deafness in adulthood? and secondly, what role does age play at the time of deafness? To address these questions, we investigated the tonotopic organization of the auditory brainstem after 1 month of deafness in adult rats of different ages. The auditory system of deafened rats was re-activated by electrical intracochlear stimulation (EIS), and the stimulation-induced neuronal activity pattern was visualized by staining the plasticity and activity marker Fos in brain sections (Ehret and Fischer, 1991; Friauf, 1992; Guzowski et al., 2001; Jakob and Illing, 2008; Rapanelli et al., 2010; Rosskothen-Kuhl and Illing, 2010, 2012; Rauch et al., 2016; Rosskothen-Kuhl et al., 2018). In many studies on different brain areas and neuronal phenotypes, Fos mRNA and Fos protein have been used as a marker for neuronal activity (Morgan and Curran, 1991; Cohen and Greenberg, 2008; Cruz et al., 2013). Fos belongs to the Immediate-Early-Gene family associated with activity dependent gene expression and its promoter is rapidly switched on in strongly activated neurons (Cohen and Greenberg, 2008; Cruz et al., 2013). In the auditory system, Fos expression requires at least 30–45 min of acoustic or electrical stimulation (Rosskothen-Kuhl and Illing, 2012; Rauch et al., 2016). As shown by Reisch et al. (2007) and Rosskothen-Kuhl et al. (2018), stimulation induced Fos expression in the auditory brainstem occurred only in neurons and not in glial cells. While previous studies have demonstrated that local EIS of hearing-experienced rats results in a tonotopic activation of neurons along the auditory pathway according to the stimulation position, our work in hearing-inexperienced, deaf rats has shown that a comparable intracochlear stimulation leads to a significantly increased number of activated neurons and a broader spread of excitation over almost the entire auditory brainstem (Rosskothen-Kuhl and Illing, 2010, 2012; Jakob et al., 2015; Rauch et al., 2016; Rosskothen-Kuhl et al., 2018; Jakob et al., 2019). Building on our previous work, we were able to show here that the activity pattern of a hearing-experienced, adult-deafened auditory system varies greatly depending on the duration of hearing prior to onset of deafness and thus the age at which deafness occurred. A reduction in tonotopic organization in the auditory system was associated with a younger age at onset of deafness.
2 Materials and methods
2.1 Experimental groups
Forty-seven female Wistar rats were divided into four groups: (1) young (y) or old (o) adult, normal hearing (NH = yNH + oNH) rats (n = 15), (2) neonatally-deafened (ND), young adult rats (n = 9), (3) young adult-deafened (YAD) rats (n = 12), and (4) old adult-deafened (OAD) rats (n = 11). The left cochlea of each rat was electrically stimulated with a CI for 2 h (for details see section 2.5). Additionally, two YAD rats and two OAD rats underwent cochlear implantation on their left side for 2 h without stimulation and served as adult-deafened (AD) “implanted” controls. Further, four adult-deafened rats served as “pure” controls (YAD: n = 2; OAD: n = 2). At the time of perfusion, all rats in the “young” adult group were approximately 16 weeks (=4 months) old, whereas all rats in the “old” adult group were around 13 months old (Figure 1). ND animals and portions of the NH animals have already been utilized in previous studies and served as reference groups (Rosskothen-Kuhl and Illing, 2010, 2012). For an overview of the timeline and experimental treatment of each experimental cohort (see Figure 1). All procedures involving a total 55 rats were approved by the Regierungspräsidium Freiburg (permission number G-10/83) and followed the guidelines of the EU directive 2010/63/EU for animal experiments.
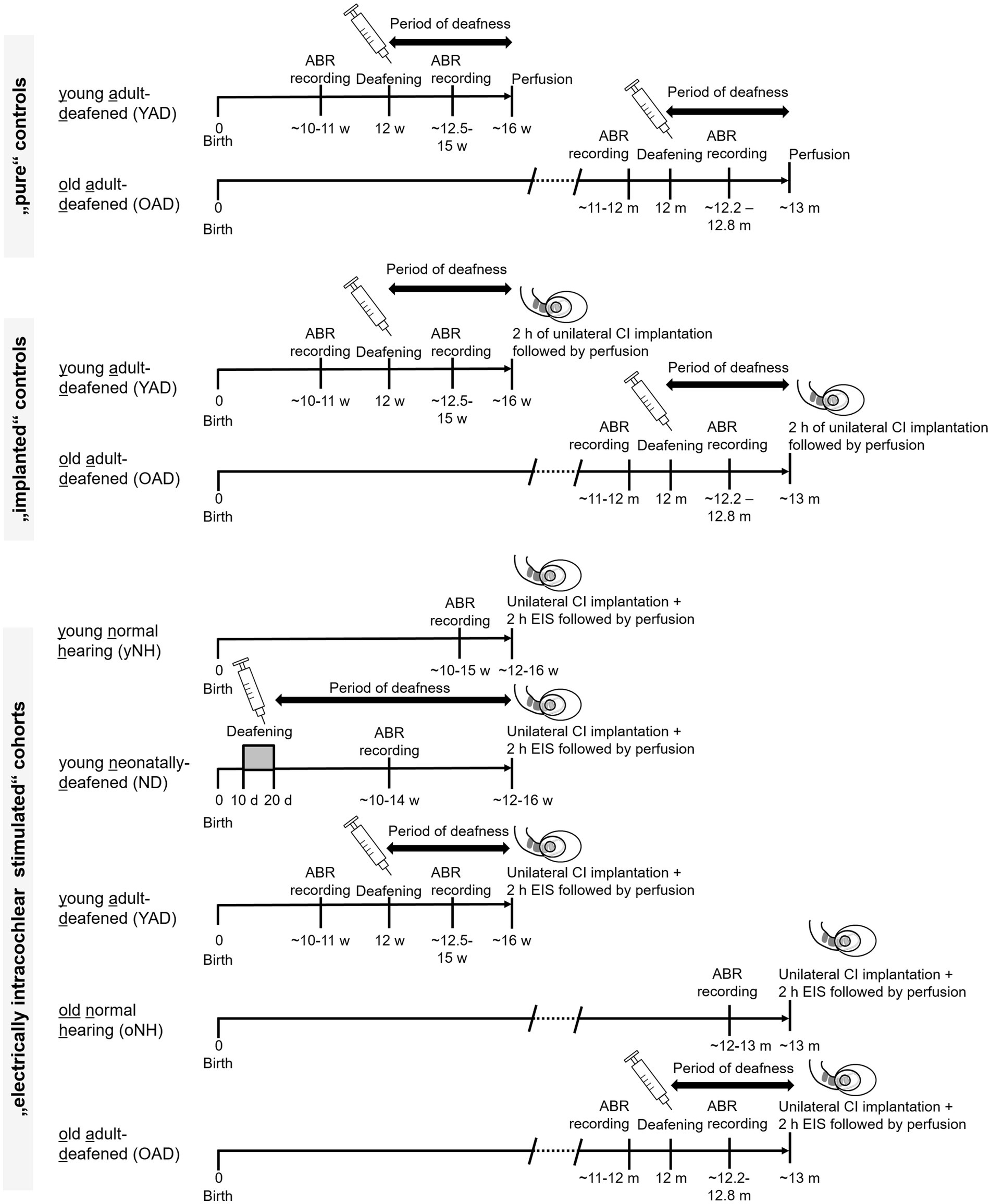
Figure 1. Timeline and experimental treatment of each experimental cohort. Young and old adult-deafened (YAD/OAD) rats were both deafened by a single injection of kanamycin in combination with ethacrynic acid at an age of 12 weeks or 12 months, respectively. Normal hearing and loss of hearing function were verified by ABR measurements before and after the treatment. In contrast, neonatally-deafened (ND) rats were hearing impaired by daily injection of kanamycin from postnatal day 10–20, inclusively, while the young and old normal hearing (yNH/oNH) cohorts remained untreated. All rats of the electrically intracochlear stimulated cohort underwent unilateral cochlear implantation at around 16 weeks of age (young adult cohort) or 13 months of age (old adult cohort), immediately followed by sustained electrical intracochlear stimulation (EIS) for 2 h (h) and subsequent perfusion. m: months, w: weeks; d: days.
2.2 Induction of adult deafness
Corresponding to Liu et al. (2011) three or 12 months old rats were systemically deafened by a single intravenous injection of ethacrynic acid solution (EA, 75 mg/kg body weight, 25 mg/mL solution, e.g., 945 μL EA solution for a 315 g rat, REOMAX, Bioindustrial L.I.M. S.P.A., Italy,) followed by intramuscular injections of kanamycin solution (KM, 500 mg/kg body weight, e.g., 985 μL KM solution for a 315 g rat, K4000, Sigma-Aldrich, Germany). Anesthesia was initiated under 5% isoflurane (Forene 100% [V/V], Abbott GmbH & Co. KG, Germany) in an inhalation chamber and maintained with ~1.5% isoflurane while the rats were kept warm on a heating pad. Before placing a tail vein catheter, the tail of the rats was heated in 38°C warm water for vasodilatation. After rinsing the lateral tail vein with ca. 0.1 mL sterile 0.9% NaCl solution, freshly prepared EA solution was slowly injected into the tail vein. Afterwards, the tail vein was rinsed again with ca. 0.1–0.2 mL 0.9% NaCl solution. In a second step, KM solution was injected intramuscularly. To ensure a sufficient supply of liquid and electrolytes, 1–2 mL Ringer’s solution was injected subcutaneously followed by subcutaneous Carprofen (4–5 mg/kg body weight, 50 mg/mL solution, Carprieve, Norbrook Laboratories Ltd., Northern Ireland) injection for pain relief. The co-administration of EA and KM results in a rapid and permanent hearing loss induced by irreversible lesion of outer and inner hair cells (Figure 5 in Liu et al., 2011).
2.3 Induction of neonatal deafness
Neonatal rats were deafened by daily intraperitoneal injections of kanamycin (400 mg/kg body weight, 50–60 mg/mL in 0.9% NaCl solution, e.g., 320 μL KM solution for a 40 g rat, K0254, Sigma-Aldrich, Germany), from postnatal day 10 to 20 inclusively (Osako et al., 1979; Rosskothen-Kuhl and Illing, 2012). This is known to cause widespread death of inner and outer hair cells (Osako et al., 1979; Matsuda et al., 1999; Argence et al., 2008) while keeping the number of spiral ganglion cells comparable to that in untreated control rats (Osako et al., 1979; Argence et al., 2008).
2.4 Verification of hearing function or hearing loss
Normal hearing or hearing loss due to pharmacological treatment was verified by measuring auditory brainstem responses (ABRs) as described in Jakob et al. (2015), Jakob et al. (2016), Rosskothen-Kuhl et al. (2018), Buck et al. (2021), Buck et al. (2023). In short, under ketamine (80 mg/kg body weight, 10% solution of Ketanest S (25 mg/mL), e.g., 160 μL for a 200 g rat, Medistar Arzneimittelvertrieb GmbH, Germany) and xylazine (12 mg/kg body weight, 50 mg/mL solution, e.g., 120 μL for a 200 g rat, Rompun, Bayer, Germany) anesthesia each ear was stimulated separately through hollow ear bars with 0.5 ms clicks (0.1–3 kHz) with peak amplitudes up to 95 dB SL (SL = individual sensation level per rat). ABRs were recorded by averaging scalp potentials measured with subcutaneous needle electrodes between mastoids and the vertex of the rat’s head over 300 click presentations. While normal hearing rats typically exhibited click ABR thresholds near 0 dB SL, deafened rats showed increased hearing thresholds ≥95 dB SL to broadband click stimuli as well as pure tones (Rosskothen-Kuhl et al., 2021; Buck et al., 2023). Figures 2A,B show ABRs of an adult-deafened rat 1 week before and half a week after deafening by EA and KM injection. Additionally, all kanamycin treated rats consistently failed to show a motor response to a handclap. The absence of this so-called Preyer’s reflex indicates a sustained increase of ABR threshold above 81 dB SPL (Jero et al., 2001).
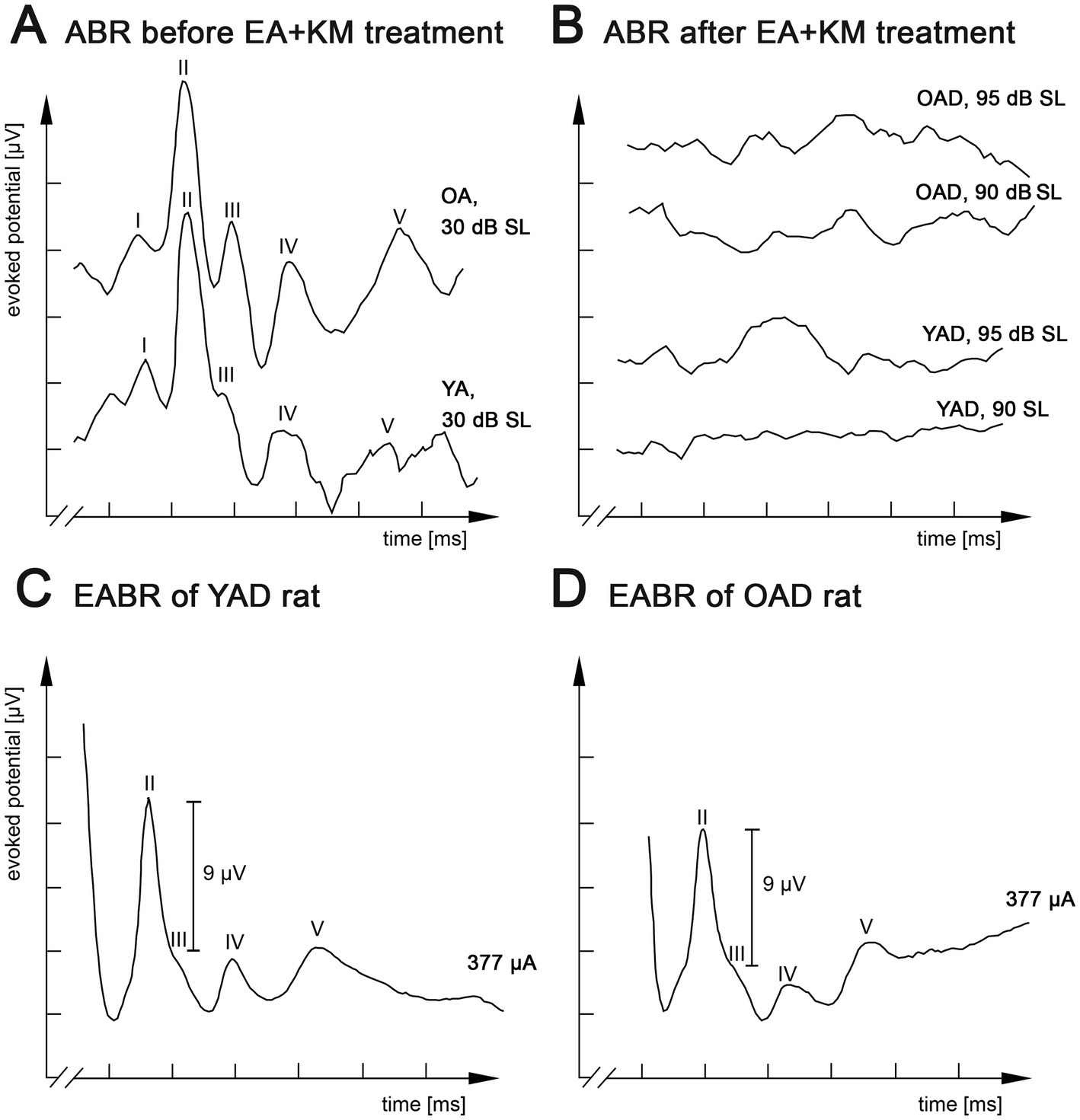
Figure 2. Kanamycin treatment in adult rats results in loss of auditory brainstem responses (ABR) and restored responses by electrical intracochlear stimulation. (A) Acoustic stimulation with broadband click stimuli at 30 dB SL induced comparable ABRs in normally hearing old adult (OA) and young adult (YA) rats. (B) Click-evoked ABR measurements of the same young adult-deafened rat (YAD) and one old adult-deafened (OAD) rat 3 days after treatment with ethacrynic acid (EA) and kanamycin (KM). Electrical intracochlear stimulation at a current level of ~377 μA induced comparable electrically evoked ABRs (EABRs) in YAD (C) and OAD (D) rats. I-V: recorded positive waves of acoustically (A,B) or electrically (C,D) evoked auditory brainstem potentials. x-axis: 2 ms per unit, y-axis: 4 μV per unit.
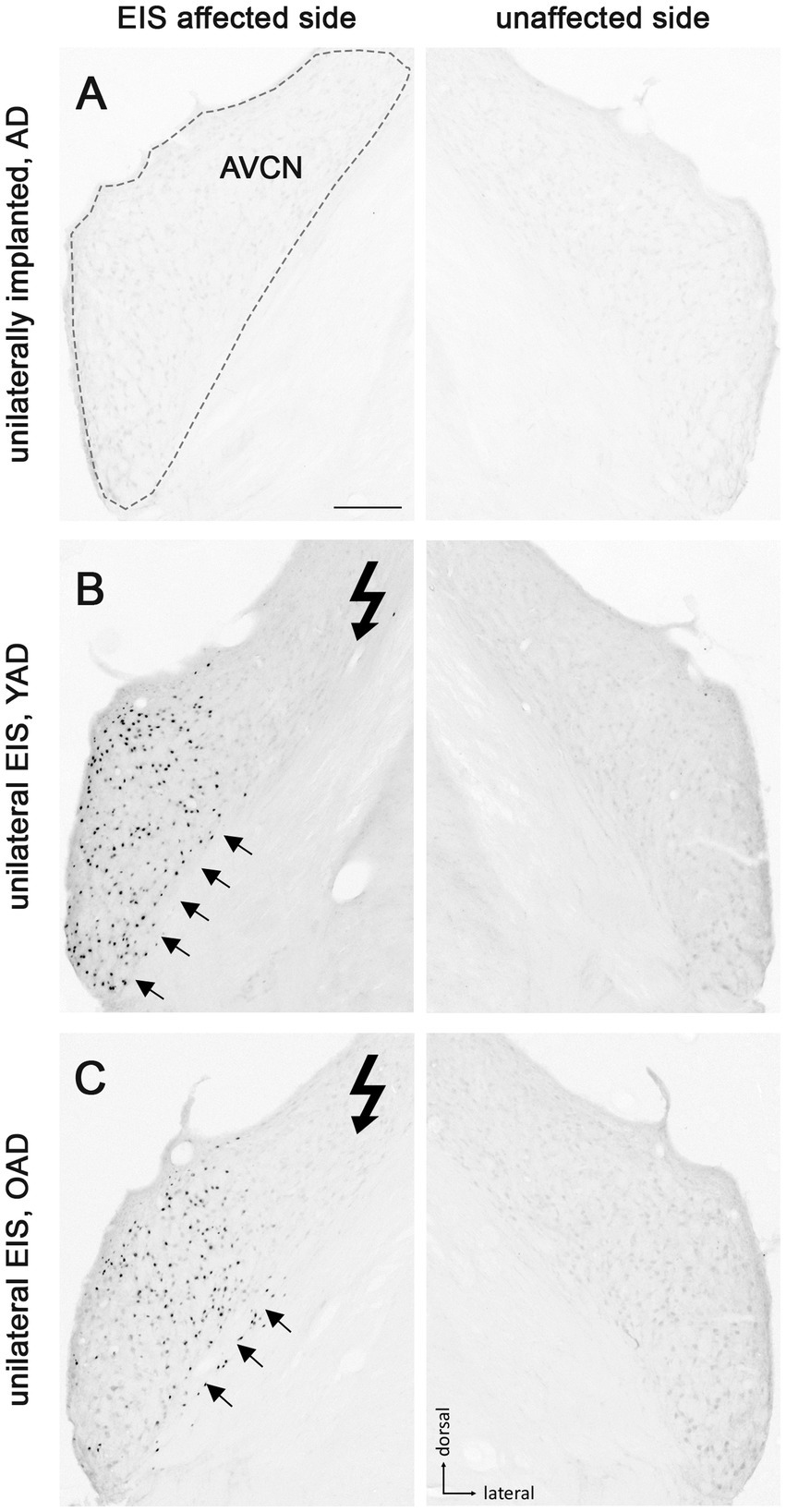
Figure 3. Patterns of Fos expression in the anteroventral cochlear nucleus (AVCN) of adult-deafened rats (AD, representative for the YAD and OAD cohort). (A) No Fos expression was found in unstimulated but unilaterally implanted AD rats in both AVCNs. Dashed line shows the border of AVCN. (B) Unilateral electrical intracochlear stimulation (EIS) of young adult-deafened (YAD) rats resulted in a large number of Fos expressing neurons (black dots) in the middle to ventral region of the ipsilateral AVCN (arrows), affected by EIS. (C) Corresponding to the intracochlear electrode position, old adult-deafened (OAD) rats showed a Fos expression limited to the central region of the ipsilateral AVCN (arrows). Flash symbol: side affected by EIS. All sections are at the level of Bregma −9.8 to −9.68 mm (Paxinos and Watson, 1986). Scale bar for A–C = 200 μm.
2.5 Electrical intracochlear stimulation
Two hours of EIS were applied under urethane anesthesia (intraperitoneal, 1.5 g/kg body weight, 0.25 g/mL urethan in 0.9% NaCl solution, e.g., 1.5 mL urethan solution for a 250 g rat, Fluka AG, Switzerland). In case of adult-deafened rats, unilateral EIS occurred on average 46 days after deafening. The stimulation set-up and cochlear implantation is described in detail in (Rosskothen-Kuhl and Illing, 2010, 2012, 2014; Jakob et al., 2015; Jakob et al., 2019). In short, two rings of an electrode array (CI24RE, Cochlear®, Australia) were inserted through a cochleostomy in a medio-dorsal direction (pointing to apex) into the middle turn of the rat cochlea corresponding to the 8–12 kHz region. The CI was connected to a Nucleus Implant Communicator kindly provided by Cochlear Germany GmbH and Co. KG. Electrically evoked ABRs (EABRs) were recorded to corroborate for the correct placement of stimulation electrodes and to determine an appropriate current level. Current levels for EIS were set to match an EABR amplitude of 9 μV ± 10%. This was achieved by a mean current level of ~340 μA, corresponding to acoustic stimuli of 80–85 dB above hearing threshold of NH rats. Corresponding to Rosskothen-Kuhl and Illing (2012), this stimulation intensity triggers tonotopic activation in the auditory system of NH rats.
2.6 Animal perfusion and preparation of brain sections
Following completion of the post-deafening survival time with or without 2 h of stimulation (see Figure 1 for details), rats were sacrificed by a lethal dose of sodium-thiopental (intraperitoneal, 50 mg/mL per 200 g body weight of Trapanal 2.5 g, Nycomed, Germany) and perfused transcardially for 60 min. For perfusion, 4°C cold fixative containing 4% paraformaldehyde in 0.1 M phosphate buffer at pH 7.4 was used. Brains were removed from the skull and stored overnight in a phosphate buffer containing 20% sucrose. Before preparing brain slices, the brains were frozen in −40°C cold 2-methylbutane (≥99%, Roth, Germany) on dry ice for 2 min. Frontal plane brain sections of 30 μm thickness containing anteroventral cochlear nucleus (AVCN), lateral superior olive (LSO), and central inferior colliculus (CIC) were cut using a cryostat.
2.7 Immunohistochemistry
Here, brain sections were exposed to a primary antibody raised in goat against Fos (SC-52-G, 1:2000, lot. no. L1406/K1808/F1109, Santa Cruz Biotechnology Inc., United States) (Rosskothen-Kuhl and Illing, 2010, 2012; Rosskothen-Kuhl et al., 2018). Visualization of primary antibody binding sites was based on the avidin–biotin technique (Cat. No. PK-6100, Vector Laboratories, United States), followed by staining with 0.05% 3.3-diaminobenzidine tetrahydrochloride (Cat. No. 32750, Sigma, Germany), 0.3% ammonium nickel (II) sulfate hexahydrate (Cat. No. A1827, Sigma, Germany) and 0.0015% H2O2 in 50 mM Tris buffer. A very detailed description of the immunostaining protocol used can be found in our previous studies (Illing et al., 2002; Rosskothen-Kuhl and Illing, 2010, 2012, 2014; Jakob et al., 2015; Jakob et al., 2019).
2.8 Quantification of Fos-positive (+) nuclei
Photographs were taken from ipsilateral AVCN (side of implantation or stimulation), ipsilateral LSO, and contralateral CIC (opposite to implantation or stimulation) of unilaterally stimulated rats using a 10x objective (for AVCN and LSO) or a 5x objective (for CIC) and a digital camera (Axiocam, Zeiss, Germany) at an 8-bit gray tone scale for quantitative evaluation of the staining results. Prior to the automated counting of Fos(+) nuclei, the color information of each image was removed by using Adobe Photoshop CS (Adobe Systems Inc., United States). As shown in Figure 7, two identically sized and equally positioned regions of interest (ROI) were selected in AVCN, LSO, and CIC to determine differences in the spatial Fos expression pattern between the experimental groups (red rectangles). A fixed pair of ROIs (ROI 1, ROI 2) was used per auditory brainstem region of interest (AVCN, LSO, CIC; Figure 7, left).
While ROI 1 was placed in the mid-frequency range of NH rats, corresponding to the intracochlear stimulation position, ROI 2 covered the low frequency region of NH rats corresponding to an unstimulated intracochlear position (Ryan et al., 1988; see Figure 7A, left). Within the defined ROIs, gray value information was spread to the full 8-bit range (from 0 to 256). For automated counting of Fos(+) nuclei, photographs of three to five adjacent sections per rat through the anterior-to-posterior axis of AVCN, LSO, and CIC were chosen in the center of the strongest Fos appearance, after we had qualitatively checked all AVCN, LSO, and CIC sections of each animal for Fos expression. All photographs were imported into the image analysis program iTEM (Olympus, Germany) where detection threshold for gray tone values was set to 145 for AVCN, 165 for LSO, and 200 for CIC. Following the definition of ROIs, detection of the stained nuclei was performed under the settings for ratio (1–4), mean diameter (2–15), elongation (1–5), area (8–100), gray value minimum (200) and roundness (0.1–1). The ratio of ROI 2/ROI 1 was calculated for each brain section to identify differences in the stimulation-induced Fos expression pattern between the four different experimental groups (NH, ND, YAD, and OAD; Figure 7). To avoid a possible zero in the division calculation in the numerator, the number of Fos positive cells in ROI 1 and ROI 2 were each added with plus one. Ratios around 1 indicate a loss of tonotopy, while ratios closer to 0 indicate a more tonotopic organization of the corresponding auditory region.
2.9 Statistical analysis
Statistical analysis was performed using Prism software (GraphPad Software, Inc., United States). We tested our data for normal distributions (using the Kolmogorov–Smirnov test) and equal variances (using the Brown–Forsythe and Bartlett tests). As our data did not consistently fulfill both criteria, we used the non-parametric Kruskal–Wallis and Mann–Whitney tests for statistical analysis. For both, significance level was set to p < 0.05. In case of multiple comparisons, p-values were corrected using Dunn’s multiple comparisons test. All adjusted p-values as well as the number of values per group are reported in Table 2. In addition, we indicate the results of Kruskal–Wallis statistics (H) for each test. Significances were differentiated into (***) for p < 0.001, (**) for p < 0.01, and (*) for p < 0.05 (Table 1). Stereological corrections for counting particles in the sectioned material were not made as particle size was small compared to section thickness and comparisons are based on numerical relationships among objects of similar size rather than on absolute densities. The Fos analysis for AVCN, LSO, and CIC was based on a total of 47 rats.
3 Results
3.1 Effect of kanamycin treatment in adult rats on auditory brainstem responses
Two cohorts of adult-deafened rats were studied: young adult-deafened (YAD) and old adult-deafened (OAD) rats. Before pharmacological treatment with kanamycin both cohorts showed normal hearing thresholds [young rats (n = 12): x̅ = 14.3 dB SL; old rats (n = 11): x̅ = 14.7 dB SL] and ABR responses with five distinguishable peaks (I–V, Figure 2A). Three days after EA + KM treatment, hearing thresholds increased by an average of 92 dB SL for young rats (n = 16 rats) and 93 dB SL for old rats (n = 15 rats; Figure 2B). Despite loss of outer and inner hair cells as previously described by Liu et al. (2011), the functionality of the auditory nerve has been preserved in both groups, which was verified by measuring EABRs with four differentiated peaks (II-V) under identical EIS. For both YAD and OAD rats, a peak II mean amplitude of 9 μV ± 10% was achieved by applying a current of in mean ~ 340 μA (Figures 2C,D).
3.2 Deafening of young but not of old adult rats results in reduction of tonotopic organization
Deafness-induced changes in the tonotopic organization along the auditory brainstem were studied by staining brain sections containing AVCN (Figure 3), LSO (Figure 4), and CIC (Figure 5) for the activity and plasticity marker Fos. Previous studies have demonstrated that Fos is a suitable marker for mapping changes in the activity pattern of the central auditory system (Rosskothen-Kuhl and Illing, 2010, 2012, 2014; Jakob et al., 2015; Jakob et al., 2016; Rosskothen-Kuhl et al., 2018; Jakob et al., 2019). However, its expression in auditory neurons requires at least 30–45 min of acoustic or electrical stimulation (Rosskothen-Kuhl and Illing, 2012).
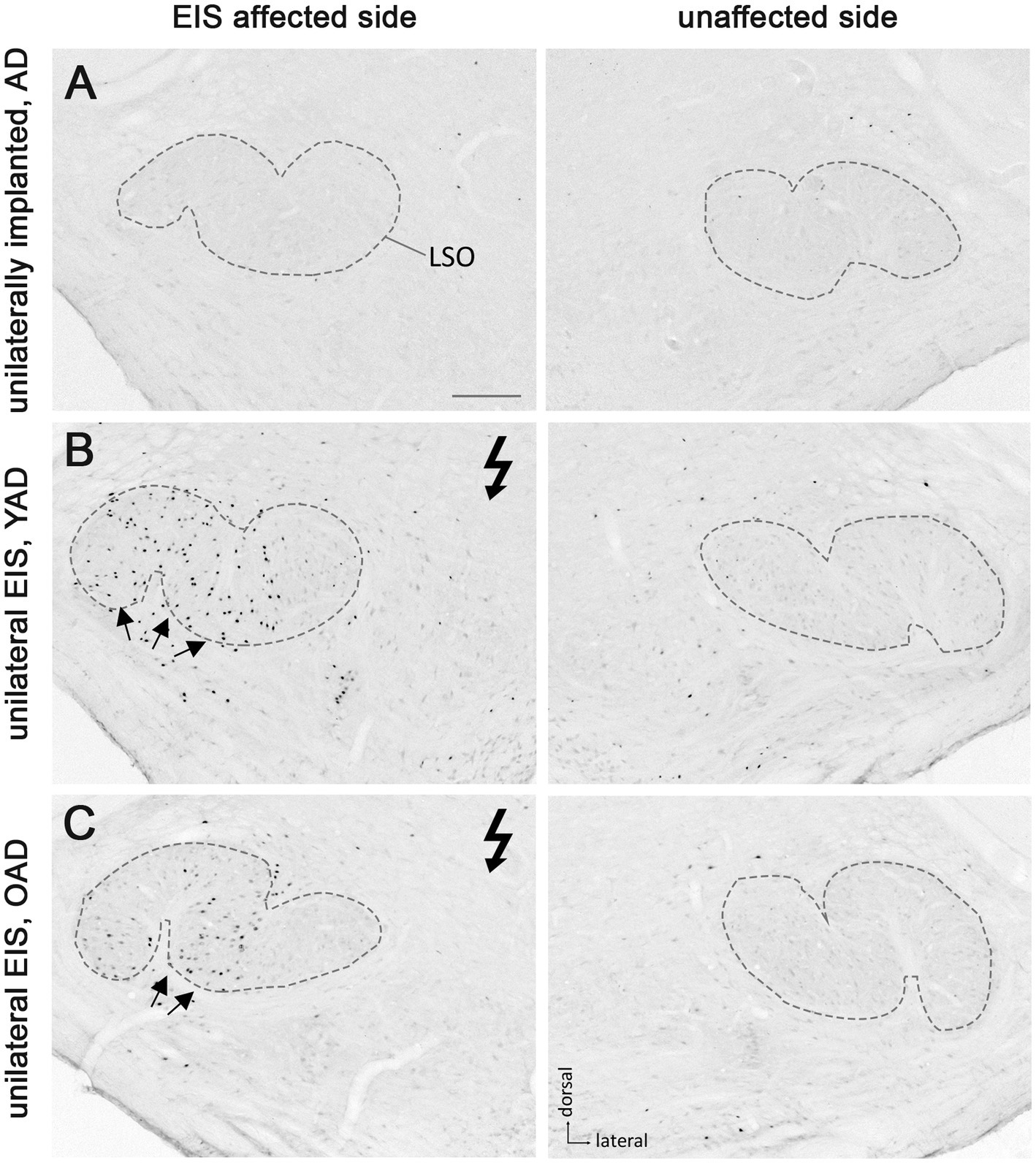
Figure 4. Patterns of Fos expression in the lateral superior olives (LSO) of adult-deafened rats (AD, representative for the YAD and OAD cohort). (A) Marginal Fos expression was found in unstimulated but unilaterally implanted AD rats in both LSOs (dashed lines). (B) Electrical intracochlear stimulation (EIS) of young adult-deafened (YAD) rats resulted in a large number of Fos expressing neurons (black dots) spread over nearly two-thirds of the EIS affected, ipsilateral LSO (arrows). (C) Corresponding to the intracochlear electrode position, old adult-deafened (OAD) rats showed a Fos expression limited to the central region of the ipsilateral LSO (arrows). Flash symbol: side affected by EIS. All sections are at the level of Bregma −9.8 to −9.3 mm (Paxinos and Watson, 1986). Scale bar for A–C = 200 μm.
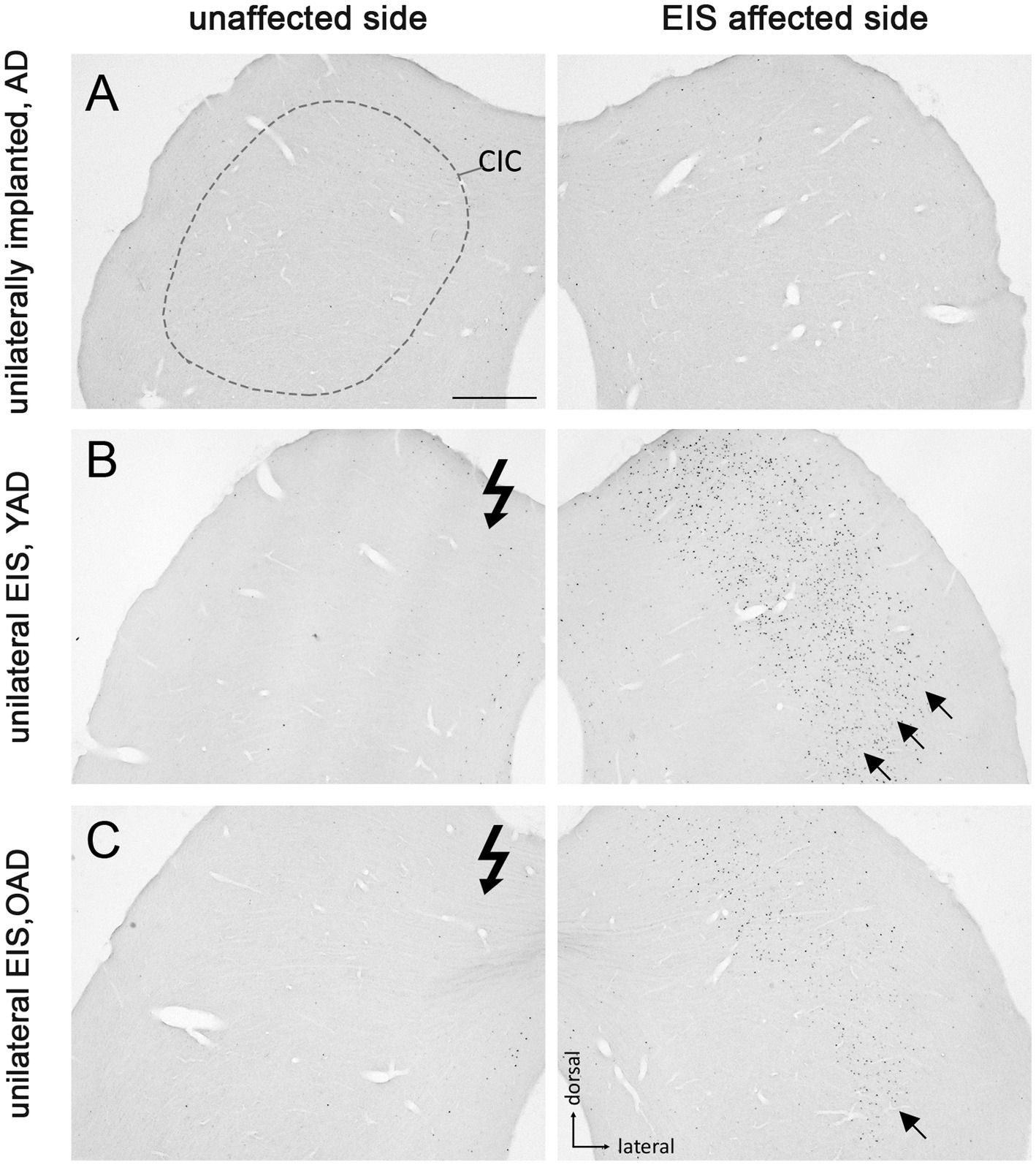
Figure 5. Fos expression pattern in the central inferior colliculus (CIC) of adult-deafened rats (AD, representative for the YAD and OAD cohort). (A) Marginal Fos expression was found in unstimulated but unilaterally implanted AD rats in both CICs. Dashed line shows the border of CIC. (B) Electrical intracochlear stimulation (EIS) of young adult-deafened (YAD) rats resulted in a large number of Fos expressing neurons (black dots) in the middle to lateral area of the contralateral CIC (arrows), side affected by EIS. (C) Corresponding to intracochlear electrode position, old adult-deafened (OAD) rats showed a Fos expression limited to the central region of the contralateral CIC (arrow). EIS affected side: CIC contralateral to EIS. Unaffected side: CIC ipsilateral to EIS. Flash symbol: side affected by EIS. All sections are at the level of Bregma −9.16 to −8.8 mm (Paxinos and Watson, 1986). Scale bar for A–C = 500 μm.
One month after bilateral deafening of YAD and OAD rats, the mid-frequency region of their left cochlea was electrically stimulated for 2 h. As a result, only the neurons of the stimulated auditory pathway (EIS affected side ≙ ipsilateral AVCN, ipsilateral LSO and contralateral CIC) expressed the Fos protein, although differences in the expression pattern were observed between the two cohorts. In YAD rats, a broadly distributed neuronal expression of Fos was observed over more than half of the ipsilateral AVCN (Figure 3B, arrows), the ipsilateral LSO (Figure 4B, arrows), and the contralateral CIC (Figure 5B, arrows) without clear boundaries indicating a tonotopic correspondence with the intracochlear stimulation position. Contralateral to stimulation (unaffected side ≙ contralateral AVCN, contralateral LSO and ipsilateral CIC), no Fos expression was observed in the AVCN, LSO, and CIC of this cohort (Figures 3–5B), which was consistent with the Fos expression pattern of implanted, non-stimulated controls (Figures 3–5A). In contrast, OAD rats showed a lower and more focused tonotopic Fos expression in the ipsilateral AVCN and LSO as well as in the contralateral CIC, corresponding to the position of intracochlear stimulation and indicated by Fos(+) neurons in the middle area of these auditory regions (Figures 3–5C, arrows). Contralateral to the electrical stimulation (unaffected side), OAD rats also showed no Fos expression (Figures 3–5C), corresponding to controls.
3.3 The age at onset of deafness affects the tonotopic organization of the auditory system
Figure 6 shows the neuronal Fos expression in the auditory brainstem (AVCN, LSO, and CIC) of young and old NH (yNH, oNH), OAD, YAD, and ND rats after 2 h of EIS. In previous studies we have demonstrated that the expression pattern of Fos and thus the stimulation-induced activity in the central auditory brainstem massively changes when hearing fails in early development (Rosskothen-Kuhl and Illing, 2010, 2012; Jakob et al., 2015; Rauch et al., 2016; Rosskothen-Kuhl et al., 2018; Jakob et al., 2019). While local intracochlear stimulation of young and old NH (yNH, oNH) rats induces a tonotopic Fos expression in auditory brainstem nuclei (Ryan et al., 1988; Rosskothen et al., 2008; Jakob and Illing, 2008; Rosskothen-Kuhl and Illing, 2010; Rauch et al., 2016) (Figures 6A–F, arrow(s)), local EIS of ND rats results in a spread of neuronal activity beyond the “normal” frequency borders, which is shown by a massive increase and dispersion of Fos-positive nuclei (Rosskothen-Kuhl and Illing, 2012; Jakob et al., 2015; Rauch et al., 2016; Rosskothen-Kuhl et al., 2018; Jakob et al., 2019) (Figures 6G–I, arrows). Neonatally-deafened brains thus show a lack of tonotopic organization along the auditory pathway, which is most likely a result of missing sensory input during early development. When comparing the stimulation-induced Fos expression patterns of NH rats (Figures 6A–F) and ND rats (Figures 6G–I) with the expression patterns observed in young and old adult-deafened animals (Figures 6G–L), it becomes clear that: first, the neuronal activity pattern of YAD rats (Figures 6J–L) is similar if not identical to the pattern of ND rats, and second, OAD rats (Figures 6G–I) show a more tonotopic Fos expression which is similar to the expression pattern of NH animals.
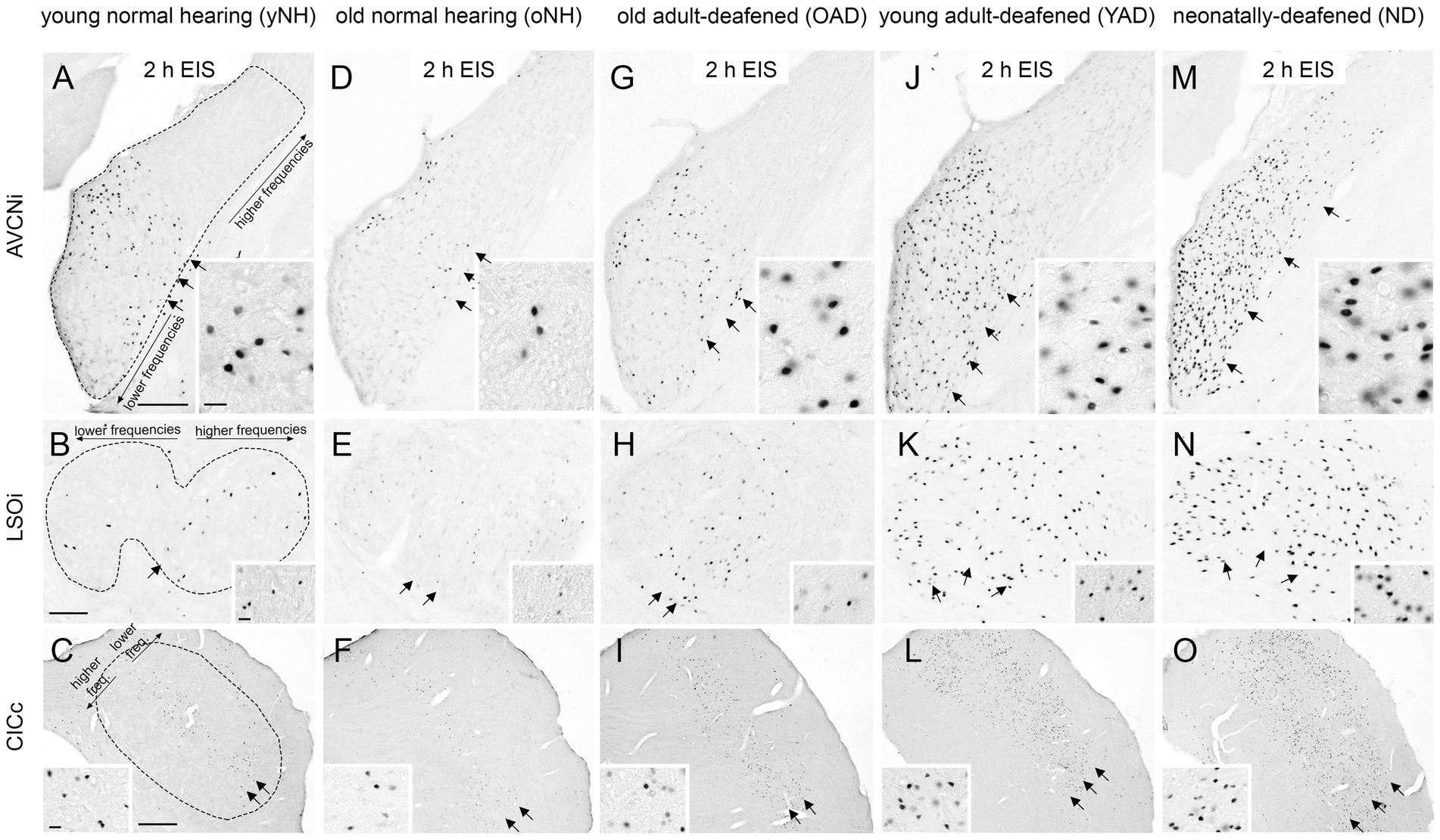
Figure 6. Stimulation-induced Fos expression patterns in neurons of the auditory brainstem of five experimental groups, young and old normal hearing (yNH/oNH), old adult-deafened (OAD), young adult-deafened (YAD), and neonatally-deafened (ND) rats. (A–F) In young (A–C) and old (D–F) NH rats stimulation-induced Fos expression is found in a tonotopic order corresponding to the intracochlear stimulation position of ~8–12 kHz (arrows) in AVCNi (A+D), LSOi (B+E), and CICc (C+F). (G–I) Similar to NH rats, OAD rats showed a Fos expression with a tonotopic distribution (arrows) in the auditory brainstem. (J–L) In contrast, YAD rats showed an increased and spread Fos expression pattern (arrows) indicating a degraded tonotopic organization. (M–O) In the auditory brainstem of ND rats, Fos expression pattern (arrows) was similar if not identical to the pattern of YAD rats, recognizable by an increased number of widely spread Fos-positive neurons in the auditory brainstem regions. Insets show Fos-positive nuclei at 100× magnification. Scale bar = 20 μm. AVCNi: anteroventral cochlear nucleus ipsilateral to electrical stimulation; CICc: central inferior colliculus contralateral to electrical stimulation; LSOi: lateral superior olive ipsilateral to electrical stimulation. Scale bars in A,B = 200 μm, and in C = 500 μm. Scale bars are valid for the entire row. Sub panels (A–C) represent the tontotopic axes for the three different auditory regions.
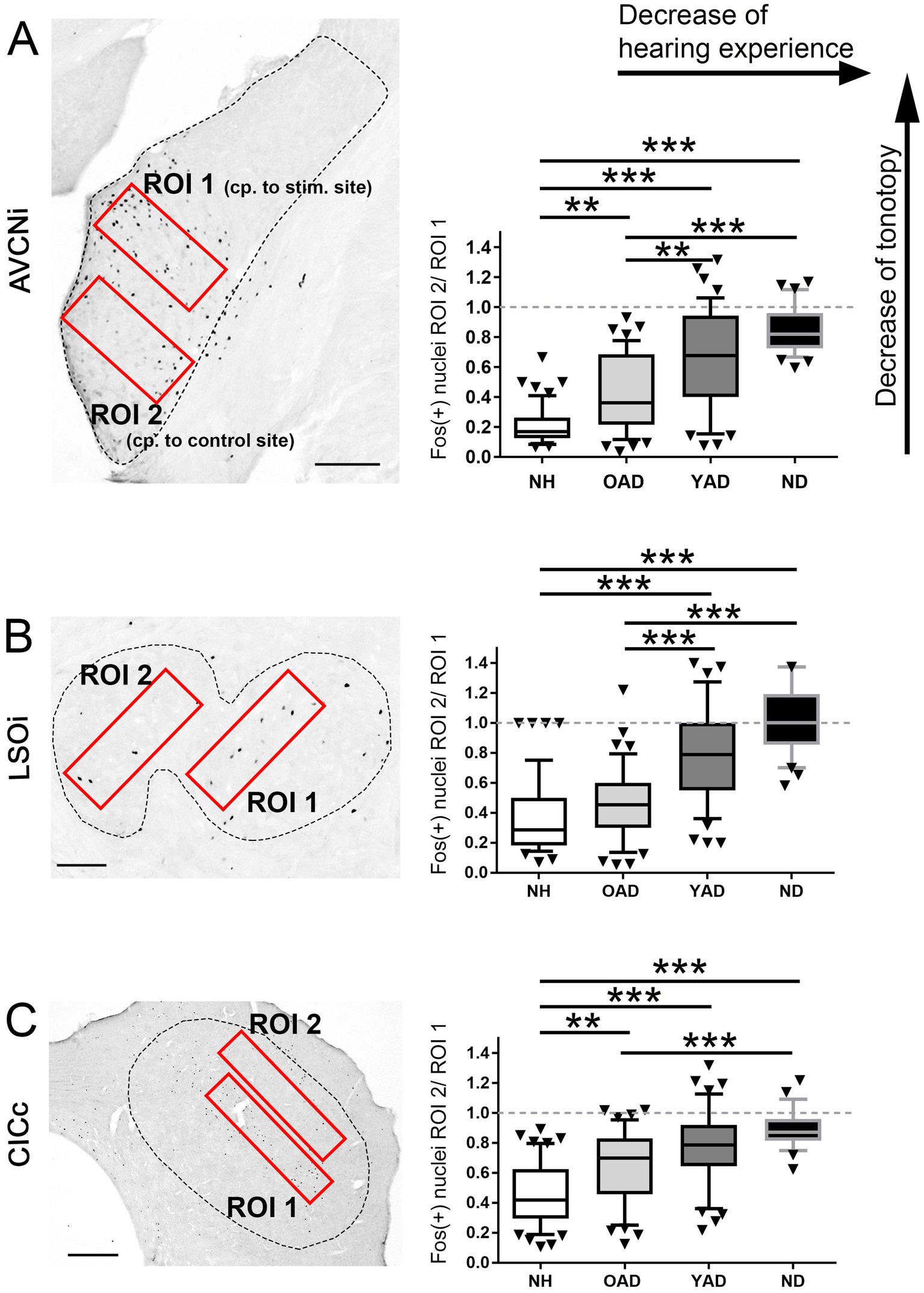
Figure 7. Quantification of the spatial distribution of the stimulation-induced Fos expression in the auditory brainstem of the four experimental groups, normal hearing (NH), old adult-deafened (OAD), young adult-deafened (YAD), and neonatally-deafened (ND) rats. (A–C) Left figures show the two regions of interest (ROIs in red) in AVCNi (A), LSOi (B), and CICc (C) and the figures on the right show the results of the ratio calculation presented as box plots for each of the four cohorts. ROI 1 is placed in the mid-frequency range of a NH rat corresponding (cp.) to the intracochlear stimulation (stim.) position, ROI 2 covers the low frequency region of a NH rat corresponding to an unstimulated intracochlear position. For statistics, the quotient of ROI 2 divided by ROI 1 (ROI 2/ROI 1) was calculated (right figures A–C). A small ratio corresponds to a good tonotopic order; a value of 1 means a Fos expression beyond the mid-frequency range of NH rats and thus a degradation of tonotopic order. Significant differences are shown by asterisks with * for p < 0.05, **for p < 0.01, *** for p < 0.001. Box plots show distribution-based metrics of the 10th and 90th percentile and the median. AVCNi: anteroventral cochlear nucleus ipsilateral to electrical stimulation; CICc: central inferior colliculus contralateral to electrical stimulation; LSOi: lateral superior olive ipsilateral to electrical stimulation. Scale bars in A,B = 200 μm, and in C = 500 μm.
3.4 The shorter the hearing experience before deafness, the less the development or preservation of tonotopic organization in the auditory brainstem
To identify a correlation between tonotopic organization of the auditory system and hearing experience prior to deafness, the topography of stimulation-induced Fos expression in AVCN (Figure 7A, dashed line), LSO (Figure 7B, dashed line), and CIC (Figure 7C, dashed line) was determined for all four experimental groups: (1) young and old NH rats, (2) OAD rats, (3) YAD rats, and (4) ND rats. Defining two regions of interest (ROIs), with ROI 1 lying in the core region of mid-frequency sensitivity of NH rats (corresponding to our intracochlear stimulation position) and ROI 2 positioned in the region of lower frequency sensitivity of NH rats and thus corresponding to an unstimulated intracochlear site (Figure 7, red rectangles), the change of the local distribution of Fos(+) neurons was quantified by calculating the ratio of ROI 2 divided by ROI 1 (ROI 2/ROI 1). As a result, all three brainstem regions showed a significant increase of the ratio (ROI 2/ROI 1) with decrease of the hearing experience (Figure 7; for p-values of statistical analysis, see Table 1). Comparing all four experimental groups (Figure 7), it becomes clear that NH rats (young and old NH rats, which were combined into one cohort due to comparable Fos expression) showed the lowest ratio (median for AVCN = 0.075, LSO = 0.14, and CIC = 0.4), indicating the highest degree of tonotopic order along their auditory brainstem followed by somewhat higher ratios for OAD rats (median for AVCN = 0.34, LSO = 0.4, and CIC = 0.7). For all three auditory regions, the ratios of YAD rats were in median between 0.67 and 0.79 and thus higher than for the NH and OAD cohorts, which points out a massively reduced tonotopic order. The highest ROI 2/ROI 1 ratio with values close to 1 (Figure 7, dashed line in statistical panels) were detected for the ND cohort with the shortest hearing experience (median for AVCN = 0.82, LSO = 1, and CIC = 0.87), meaning that the distribution of Fos(+) neurons in both ROIs was almost equal and thus indicating a loss of tonotopy. In detail, for AVCN, we found significant differences between all groups, except for YAD and ND rats (Figure 6A; Table 1). In the LSO, we detected significant differences between groups, excluding NH vs. OAD rats and YAD vs. ND rats (Figure 6B; Table 2). For CIC, all groups showed significant differences between each other except YAD vs. OAD and YAD vs. ND rats (Figure 6C; Table 1). The detailed statistical results, including p-values, Kruskal–Wallis statistics (H), and number (n) of analyzed sections, are presented in Table 1. Overall, our quantification provides strong evidence that the preservation of tonotopic organization after adult-onset deafness correlates with the duration of hearing experience and thus with the age at deafness. Although they differed significantly from NHs rats in AVCN and CIC, OAD rats (~12 months hearing +1 month deaf) more or less maintained their tonotopy, whereas YAD rats (~3 months hearing +1 month deaf) interestingly lost their established organization already 1 month after deafness.
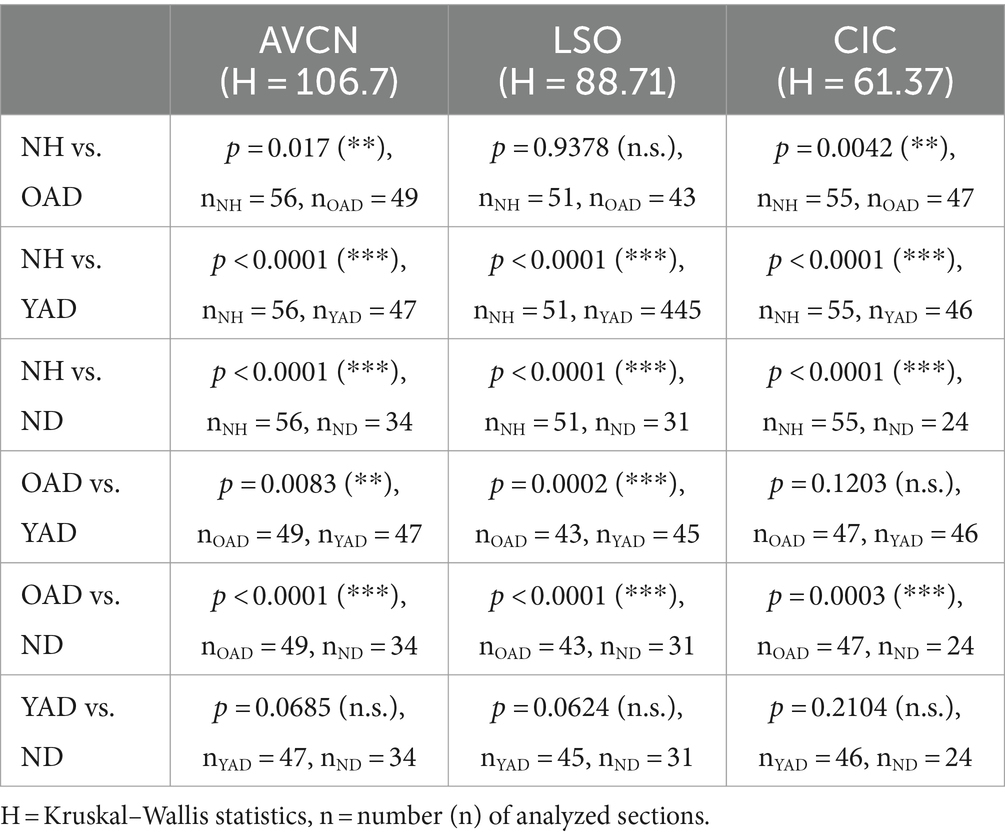
Table 2. Statistical data of Fos quantification in three auditory brainstem regions of all four experimental groups: normally hearing (NH), neonatally-deafened (ND), young adult-deafened (YAD), old adult-deafened (OAD).
4 Discussion
A better understanding of the age-dependent plastic changes that occur in the mature auditory system following acute deafness is an essential step toward improved rehabilitation of hearing-impaired patients and could contribute to improved auditory perception after the fitting with hearing prostheses. IThe present study investigated the impact of deafness on the tonotopic organization of the mature auditory brainstem as a function of age at onset of deafness. One of the most important findings was that the central auditory system of younger and older adult rats differently respond to sudden and severe deafness. Using histological staining of the neuronal activity and plasticity marker Fos, we demonstrated for the first time that just 1 month of bilateral deafness results in a loss of tonotopic order in the auditory brainstem of younger rats, whereas the auditory system of older rats showed not such marked changes in the stimulation-induced Fos expression pattern. This suggests that the tonotopic order, as observed in normal hearing peers, is more or less preserved in older rats despite acute deafness.
4.1 Hearing function and induction of deafness in adulthood
Regardless of age, all of our rats (only female) showed normal hearing thresholds prior to pharmacological deafening in adulthood. This observation coincides with a study on female Wistar rats by Möhrle et al. (2016), in which significant hearing threshold loss could only be detected at the age of 19 months or older. Our 12-month-old animals showed hearing thresholds similar to the thresholds of middle-aged animals (6–10 months) observed by Möhrle et al. (2016). This is different from the observations made for male Wistar rats, where an increase in hearing threshold is already observed at an age of 12 months and even earlier in noise exposed animals (Alvarado et al., 2014; Alvarado et al., 2019). But even if the hearing threshold of our older rats was still unaffected, the hearing function at suprathreshold levels could be different in young and old animals, e.g., due to the loss of spiral ganglion neurons or synaptic contacts of the spiral ganglion cells to the inner hairs cells (Kujawa and Liberman, 2009; Sergeyenko et al., 2013; Möhrle et al., 2016).
In addition to Liu et al. (2011), we were able to demonstrate ototoxic efficacy of the combined compounds EA and KM not only for young adult rats (~3 months old) but also for older rats (~12 months old), as evidenced by the loss of the Preyer’s reflex (see Jero et al., 2001) and a strong increase in hearing thresholds by ~92–93 dB SL, indicating the efficacy of this systemic deafness method regardless of the age of the animals. Despite pharmacologically induced deafness, which according to Liu et al. (2011) is mainly caused by irreversible degeneration of outer and inner hair cells, both groups of EA and KM treated animals showed well differentiated EABRs following cochlear implantation. Under identical stimulation conditions and parameters, these EABRs were comparable to the EABRs of our normal hearing rats (see also Rosskothen-Kuhl et al., 2018; Jakob et al., 2019), indicating good and sufficient preservation of spiral ganglion neurons after 1 month of deafness. However, to our knowledge, it is still unclear whether at all and if so which classes of spiral ganglion fibers are affected by EA and KM treatment in rats and whether they differ depending on age at deafness, which could result in different fiber sensitivity between the two cohorts.
4.2 Loss of tonotopy in younger but not in older auditory systems after acute deafness in adulthood
Fos (also known as c-Fos) belongs to the Immediate Early-Gene family associated with activity-dependent gene expression. The Fos promoter becomes rapidly induced in strongly activated neurons (Morgan and Curran, 1986; Cohen and Greenberg, 2008; Cruz et al., 2013). In the auditory system, Fos mRNA and protein expression can be induced by acoustic (Ehret and Fischer, 1991; Jakob et al., 2019) or electric stimulation (Vischer et al., 1994; Rosskothen et al., 2008; Rauch et al., 2016; Rosskothen-Kuhl et al., 2018).
Thirty to forty-five minutes of sustained electrical stimulation of the cochlea is sufficient to induce neuronal Fos expression along the auditory pathway of both NH as well as ND animals (Rosskothen-Kuhl and Illing, 2010, 2012). While frequency-specific stimulation of the cochlea of NH rats results in a local Fos expression pattern corresponding to the intracochlear stimulation position and thus reveals a tonotopic organization of the auditory pathway (Rosskothen et al., 2008; Rosskothen-Kuhl and Illing, 2010; Jakob, 2011; Jakob et al., 2019), comparable stimulation of ND rats leads to a massively increased number of activated neurons, which are spread over almost the entire frequency range of the different auditory brainstem regions and thus indicates a loss of tonotopy (Rosskothen-Kuhl and Illing, 2012; Jakob et al., 2015; Rauch et al., 2016; Rosskothen-Kuhl et al., 2018; Jakob et al., 2019).
In this study, we could demonstrate that not only neonatal deafness but also acute deafness in young adulthood can result in a degraded tonotopic organization (Figures 3–6). An auditory deprivation of 1 month, which corresponded to a quarter of the animal’s lifetime, was sufficient to degenerate the frequency mapping of the auditory pathway developed in the first 3 months of life as indicated by a spread of Fos(+) neurons beyond the “normal” frequency borders. By directly comparing the stimulation-induced Fos expression levels and patterns of all four cohorts, NH, OAD, YAD, and ND rats, we derived two main conclusions: First, the shorter the duration of deafness, the better the tonotopy of the auditory pathway, and second, with identical duration of deafness in adulthood, as in our YAD vs. OAD cohorts, age at onset of deafness and/or hearing experience appears to influence the preservation of tonotopy. In accordance with our results of the Fos quantification shown in Figure 7, a higher age at onset of deafness and thus a longer period of auditory experience, as in the case of our OAD cohort, seems to have a positive effect on the preservation of the tonotopic organization in the adult auditory system. In contrast to the older animals, the auditory system of younger animals seems to react quickly to the sudden absence of sensory input.
4.2.1 Preservation of tonotopy in the older auditory system
Several studies have already shown that the plasticity level is higher in younger than in older brains (Berardi et al., 2004; Bavelier et al., 2010; Kwok et al., 2011; Sorg et al., 2016; Foscarin et al., 2017). An important role is attributed to the perineuronal nets (PNNs) in the central nervous system. PNNs are part of the extracellular matrix and play an important role in neuronal protection (Suttkus et al., 2012), the stabilization of synaptic contacts (Hockfield et al., 1990) as well as the inhibition of structural and functional plasticity (Frischknecht et al., 2009). In principle, the brain’s extracellular matrix mediates structural stability by enwrapping synaptic contacts fundamental, for example, for long-term memory storage (Happel et al., 2014). In the aging auditory system of rats, Mafi et al. (2020) have shown that the density of PNNs is massively increasing. Especially in the central and dorsal inferior colliculus a strong increase of PNNs on GABAergic and non-GABAergic cells could be identified. In addition, Foscarin et al. (2017) have demonstrated in rats that brain aging changes the sulfation of proteoglycans in PNNs, making the perineuronal nets more inhibitory and thus leading to a decrease in plasticity. Among others, a lower plasticity potential in the auditory system of our OAD cohort could be a reason why the tonotopic organization is still preserved after 1 month of deafness. Presumably, PNN-mediated inhibition of plasticity slows down or even prevents disinhibition of the auditory network in older rats, which can normally be triggered within hours after both juvenile and adult hearing loss (Browne et al., 2012; Llano et al., 2012; Resnik and Polley, 2017; Balaram et al., 2019). This hypothesis is in line with a study by Bavelier et al. (2010) who claim that “… a reduction in plasticity as development proceeds is likely to allow greater adaptability of the organism to variable conditions early in life, while ensuring an efficient neural architecture for known conditions by adulthood.” Whether the tonotopic organization in the OAD rats is still maintained even after a longer period of deafness or is lost with a delay cannot yet be answered and requires a follow-up study on OAD rats with a longer period of deafness.
An altered structural plasticity level discussed here might be one possible explanation for the changes we have identified in the auditory system. Alternatively or in addition, functional plasticity could also play a role here (Irvine, 2018; Kim et al., 2019). Thus, the altered local expression of Fos in the normally tonotopically organized auditory brainstem regions could be a consequence of functional remodeling of the tonotopic representation of the cochlear input to the respective brain region. In contrast to the widespread and accepted hypothesis that aging can be associated with a reduced or even complete loss of plasticity (Kwok et al., 2011; Sorg et al., 2016; Foscarin et al., 2017; Persic et al., 2020), a study by Cisneros-Franco et al. (2018) has shown that passive sound exposure can result in a plastic reorganization of the tonotopic map in the auditory cortex of older (age 22–24 months) but not younger adult rats (age 6–8 months). The increased but dysregulated plasticity was associated with a reduced inhibition of the aging auditory system. However, it should be noted that Cisneros-Franco et al. (2018) refer to cortical and not to subcortical structures and performed the experiments on hearing and not on deafened animals, thus limiting the applicability of these results to our study.
4.2.2 Loss of tonotopy in the young adult auditory system
In addition to the hypothesis that older auditory networks may have a lower plasticity potential, Heusinger et al. (2019) demonstrated a rapid degradation of the extracellular matrix after acute unilateral deafness in young adult rats (age 2–5 months). This was accompanied by an ingrowth of immature synapses into the AVCN of the deaf side. Furthermore, a modulation of the extracellular matrix in the CIC contralateral to deafness was observed already 1 day after deafness. This study thus provides important evidence that the young adult-deafened auditory system has a high plasticity potential and undergoes a remodeling of synaptic connections within only a few days after deafness due to short-term degradation of the extracellular matrix. According to these observations, it can be assumed that the auditory system of our YAD cohort was also plastic and underwent structural and/or functional remodeling as a result of acute hearing loss. A well-described consequence of adult deafness is changes in inhibitory networks. While studies on the auditory brainstem have identified inhibitory changes within days (Bledsoe et al., 1995; Abbott et al., 1999; Vale and Sanes, 2002; Vale et al., 2003), cortical networks undergo disinhibition within a few hours, as indicated by a reduced expression of GABAergic markers (Kotak et al., 2005; Sarro et al., 2008; Takesian et al., 2010; Browne et al., 2012; Llano et al., 2012; Takesian et al., 2012; Mowery et al., 2015; Resnik and Polley, 2017; Balaram et al., 2019). This could be one reason for the dramatic increase in neuronal activity and the accompanying degraded tonotopy in the auditory brainstem of our electrically stimulated YAD rats. In support of this hypothesis, a study by Gallinaro and Rotter (2018) on structural plasticity based on firing rate homeostasis in recurrent neuronal networks provide evidence that connectivity in sensory networks changes depending on stimulation and that a disturbance of the steady state could result in the degradation of connections, for example due to the loss of sensory input.
In agreement with our observations on young-adult rats, studies on other animal models and humans have shown that adult deafness can affect the tonotopic organization of the auditory pathway. Using fMRI on young to middle aged human patients, Wolak et al. (2017) demonstrate that postlingual sensorineural hearing loss affects the tonotopic organization of the auditory cortex. Significant differences in the cortical tonotopic map compared to normal hearing controls were also found in patients with bilateral high-frequency hearing loss (Koops et al., 2020). In line with this, the study by Robertson and Irvine (1989) demonstrates a plasticity of frequency organization in the auditory cortex of adult guinea pigs as early as 35 days after unilateral deafness. In addition, mild noise-induced hearing loss in adulthood leads to a broader frequency tuning in the primary auditory cortex of cats (Seki and Eggermont, 2002). According to Pienkowski and Eggermont (2011), deafness caused by damage to the auditory periphery induces plasticity in the adult auditory cortex, which is reflected in changes in the topographic map, among other things. In addition to the cortex, the auditory thalamus also shows plasticity of the tonotopic organization after unilateral deafness of adult cats (Kamke et al., 2003). In contrast to the auditory cortex and the medial geniculate body in the thalamus, no or only limited and non-permanent reorganization of the tonotopic map has been observed in regions of the auditory brainstem such as the dorsal cochlear nucleus or the CIC after deafness in adulthood (Rajan and Irvine, 1998; Irvine et al., 2003; Pienkowski and Eggermont, 2011). The previous observations for the auditory brainstem thus contrast with the results of our study in young adult-deafened rats, which for the first time demonstrated a deterioration of the tonotopy from the ventral cochlear nucleus via the LSO to the CIC of the auditory brainstem (even) after 4 weeks of auditory deprivation. Although the tonotopy of the auditory system has long been considered resistant to changes, e.g., hearing loss, after completion of a critical developmental phase, the above studies as well as our own results show that plasticity is preserved in the young adult brain and occurs at both cortical and subcortical levels.
4.3 Clinical relevance
As shown by Hiel et al. (2016), age is not a limiting factor for the CI fitting of patients. Even after a long period of deafness of 10–30 years a CI can still be of great benefit (Moon et al., 2014; Hiel et al., 2016; Medina et al., 2017). One possible explanation could be an increased stability of the hearing-experienced, mature auditory network in old age. Despite a possibly reduced plasticity, a better preservation of network organization after deafness could help older patients to analyze sensory input via CI in a meaningful way. Provided that the data from the animal experiments can be transferred to human patients, our study provides evidence that elderly patients might be very suitable for reactivation of their auditory system by CIs due to a higher stability of their mature sensory networks even after a longer period of deafness. This hypothesis is supported, for example, by the better preservation of tonotopic organization after acute deafness in the old auditory system of mammals. This is in contrast to the auditory networks of younger patients, which are probably more plastic and therefore adapt more quickly to changes in sensory input. To date, the performance and benefit of older patients supplied with CI is still discussed controversially. While some studies show a better outcome for the younger adult CI patients (Chatelin et al., 2004; Vermeire et al., 2005; Lundin et al., 2013), others could not identify any differences (Labadie et al., 2000; Haensel et al., 2005; Mosnier et al., 2020; Bourn et al., 2022) or even demonstrate better performances for the elderly (Leung et al., 2005). For example, Leung et al. (2005) show that the age at implantation is only a low predictive value for postoperative performance. Elderly even performed better when the duration of deafness exceeded 25 years compared to their younger counterparts.
Based on our data, we suppose that patients who become deaf at an advanced age might have a larger time window to benefit from CI supply in contrast to young adult patients who should be treated as soon as possible after deafening in order to counteract the degeneration of trained network organizations at an early stage. However, it is very likely that the latter, with appropriate training and rehabilitation, can compensate for a potential loss of network organization in the long term due to their higher level of plasticity.
5 Conclusion
The central auditory system of young and old adult rats adapts differentially to acute deafness in adulthood. Our study, in which the auditory system was (re)activated by electrical stimulation of the cochlea, demonstrated that the auditory pathway of young adult rats after acute deafness exhibits a loss of tonotopy partly resembling that of neonatally-deafened rats. In contrast, after acute deafness in older animals, the auditory system retains its tonotopic order, similar to that of normal hearing animals. We conclude that plasticity predominates in the central auditory system of young adult rats. This is evidenced by the observation that acute deafness results in a degradation of frequency mapping along the auditory pathway after only a short period of deafness. In contrast, network stability seems to prevail in the older auditory system. This could explain the preservation of frequency mapping in the auditory system even after a period of severe deafness and could be an optimal prerequisite for enabling a good hearing outcome after CI supply in older deaf patients.
Data availability statement
The original contributions presented in the study are included in the article/supplementary material, further inquiries can be directed to the corresponding author.
Ethics statement
The animal study was approved by Regierungspräsidium Freiburg, 79083 Freiburg im Breisgau, Permission number G-10/83. The study was conducted in accordance with the local legislation and institutional requirements and followed the guidelines of the EU directive 2010/63/EU for animal experiments.
Author contributions
NR-K: Conceptualization, Data curation, Formal analysis, Funding acquisition, Investigation, Methodology, Project administration, Resources, Software, Supervision, Validation, Visualization, Writing – original draft, Writing – review & editing. SG: Data curation, Formal analysis, Investigation, Methodology, Validation, Writing – review & editing. TJ: Data curation, Formal analysis, Investigation, Visualization, Writing – original draft, Writing – review & editing, Validation.
Funding
The author(s) declare that financial support was received for the research, authorship, and/or publication of this article. This research was supported by a research grant from Cochlear Germany GmbH and Co. KG, Hannover, Germany (Research Agreement IIR-1498).
Acknowledgments
We thank H. Hildebrandt for technical assistance, proofreading, and helpful discussions and R.-B. Illing for continuous support and helpful discussion. Stimulation electrodes, programming software, and hardware components were kindly provided by Cochlear Germany GmbH & Co. KG, Hannover, Germany. We acknowledge support by the Open Access Publication Fund of the University of Freiburg.
Conflict of interest
The authors declare that the research was conducted in the absence of any commercial or financial relationships that could be construed as a potential conflict of interest.
Publisher’s note
All claims expressed in this article are solely those of the authors and do not necessarily represent those of their affiliated organizations, or those of the publisher, the editors and the reviewers. Any product that may be evaluated in this article, or claim that may be made by its manufacturer, is not guaranteed or endorsed by the publisher.
Abbreviations
ABR, Auditory brainstem response; AD, Adult-deafened; AVCN, Anteroventral cochlear nucleus; CI, Cochlear implant; CIC, Central inferior colliculus; cp, Corresponding; dB, Dezibel; EA, Ethacrynic acid; EABR, Electrically evoked ABR; EIS, Electrical intracochlear stimulation; KM, Kanamycin; LSO, Lateral superior olive; ND, Neonatally-deafened; NH, Normal hearing; o, Old; OAD, Old adult-deafened; oNH, Old normal hearing; ROI, Regions of interest; SL, Sensation level; SPL, Sound pressure level; stim, Stimulation; y, Young; YAD, Young adult-deafened; yNH, Young normal hearing.
References
Abbott, S. D., Hughes, L. F., Bauer, C. A., Salvi, R., and Caspary, D. M. (1999). Detection of glutamate decarboxylase isoforms in rat inferior colliculus following acoustic exposure. Neuroscience 93, 1375–1381. doi: 10.1016/s0306-4522(99)00300-0
Akter, N., Adachi, R., Kato, A., Fukaya, R., and Kuba, H. (2018). Auditory input shapes Tonotopic differentiation of Kv1.1 expression in avian Cochlear nucleus during late development. J. Neurosci. 38, 2967–2980. doi: 10.1523/JNEUROSCI.2472-17.2018
Alvarado, J. C., Fuentes-SantamarÃa, V. Ã., Gabaldón-Ull, M. C., Blanco, J. L., and Juiz, J. M. (2014). Wistar rats: a forgotten model of age-related hearing loss. Front. Aging Neurosci. 6:29. doi: 10.3389/fnagi.2014.00029
Alvarado, J. C., Fuentes-Santamaría, V., Gabaldón-Ull, M. C., and Juiz, J. M. (2019). Age-related hearing loss is accelerated by repeated short-duration loud sound stimulation. Front. Neurosci. 13:77. doi: 10.3389/fnins.2019.00077
Apple, D. M., Solano-Fonseca, R., and Kokovay, E. (2017). Neurogenesis in the aging brain. Biochem. Pharmacol. 141, 77–85. doi: 10.1016/j.bcp.2017.06.116
Argence, M., Vassias, I., Kerhuel, L., Vidal, P.-P., and De Waele, C. (2008). Stimulation by cochlear implant in unilaterally deaf rats reverses the decrease of inhibitory transmission in the inferior colliculus. Eur. J. Neurosci. 28, 1589–1602. doi: 10.1111/j.1460-9568.2008.06454.x
Balaram, P., Hackett, T. A., and Polley, D. B. (2019). Synergistic transcriptional changes in AMPA and GABAA receptor genes support compensatory plasticity following unilateral hearing loss. Neuroscience 407, 108–119. doi: 10.1016/j.neuroscience.2018.08.023
Bavelier, D., Levi, D. M., Li, R. W., Dan, Y., and Hensch, T. K. (2010). Removing brakes on adult brain plasticity: from molecular to behavioral interventions. J. Neurosci. 30, 14964–14971. doi: 10.1523/JNEUROSCI.4812-10.2010
Berardi, N., Pizzorusso, T., and Maffei, L. (2004). Extracellular matrix and visual cortical plasticity: freeing the synapse. Neuron 44, 905–908. doi: 10.1016/j.neuron.2004.12.008
Bledsoe, S. C., Nagase, S., Miller, J. M., and Altschuler, R. A. (1995). Deafness-induced plasticity in the mature central auditory system. Neuroreport 7, 225–229. doi: 10.1097/00001756-199512000-00054
Bourn, S. S., Goldstein, M. R., Morris, S. A., and Jacob, A. (2022). Cochlear implant outcomes in the very elderly. Am. J. Otolaryngol. 43:103200. doi: 10.1016/j.amjoto.2021.103200
Browne, C. J., Morley, J. W., and Parsons, C. H. (2012). Tracking the expression of excitatory and inhibitory neurotransmission-related proteins and neuroplasticity markers after noise induced hearing loss. PLoS One 7:e33272. doi: 10.1371/journal.pone.0033272
Buck, A. N., Buchholz, S., Schnupp, J. W., and Rosskothen-Kuhl, N. (2023). Interaural time difference sensitivity under binaural cochlear implant stimulation persists at high pulse rates up to 900 pps. Sci. Rep. 13:3785. doi: 10.1038/s41598-023-30569-0
Buck, A. N., Rosskothen-Kuhl, N., and Schnupp, J. W. (2021). Sensitivity to interaural time differences in the inferior colliculus of cochlear implanted rats with or without hearing experience. Hear. Res. 408:108305. doi: 10.1016/j.heares.2021.108305
Burke, S. N., and Barnes, C. A. (2006). Neural plasticity in the ageing brain. Nat. Rev. Neurosci. 7, 30–40. doi: 10.1038/nrn1809
Chatelin, V., Kim, E. J., Driscoll, C., Larky, J., Polite, C., Price, L., et al. (2004). Cochlear implant outcomes in the elderly. Otol. Neurotol. 25, 298–301. doi: 10.1097/00129492-200405000-00017
Cisneros-Franco, J. M., Ouellet, L., Kamal, B., and de Villers-Sidani, E. (2018). A brain without brakes: reduced inhibition is associated with enhanced but dysregulated plasticity in the aged rat auditory cortex. eNeuro 5:ENEURO.0051-18.2018. doi: 10.1523/ENEURO.0051-18.2018
Cohen, S., and Greenberg, M. E. (2008). Communication between the synapse and the nucleus in neuronal development, plasticity, and disease. Annu. Rev. Cell Dev. Biol. 24, 183–209. doi: 10.1146/annurev.cellbio.24.110707.175235
Cruz, F. C., Koya, E., Guez-Barber, D. H., Bossert, J. M., Lupica, C. R., Shaham, Y., et al. (2013). New technologies for examining neuronal ensembles in drug addiction and fear. Nat. Rev. Neurosci. 14, 743–754. doi: 10.1038/nrn3597
Ehlers, E., Goupell, M. J., Zheng, Y., Godar, S. P., and Litovsky, R. Y. (2017). Binaural sensitivity in children who use bilateral cochlear implants. J. Acoust. Soc. Am. 141, 4264–4277. doi: 10.1121/1.4983824
Ehret, G., and Fischer, R. (1991). Neuronal activity and tonotopy in the auditory system visualized by c-fos gene expression. Brain Res. 567, 350–354. doi: 10.1016/0006-8993(91)90819-h
Fallon, J. B., Irvine, D. R. F., and Shepherd, R. K. (2009). Cochlear implant use following neonatal deafness influences the cochleotopic organization of the primary auditory cortex in cats. J. Comp. Neurol. 512, 101–114. doi: 10.1002/cne.21886
Foscarin, S., Raha-Chowdhury, R., Fawcett, J. W., and Kwok, J. C. F. (2017). Brain ageing changes proteoglycan sulfation, rendering perineuronal nets more inhibitory. Aging (Albany NY) 9, 1607–1622. doi: 10.18632/aging.101256
Friauf, E. (1992). Tonotopic order in the adult and developing auditory system of the rat as shown by c-fos immunocytochemistry. Eur. J. Neurosci. 4, 798–812. doi: 10.1111/j.1460-9568.1992.tb00190.x
Frischknecht, R., Heine, M., Perrais, D., Seidenbecher, C. I., Choquet, D., and Gundelfinger, E. D. (2009). Brain extracellular matrix affects AMPA receptor lateral mobility and short-term synaptic plasticity. Nat. Neurosci. 12, 897–904. doi: 10.1038/nn.2338
Gallinaro, J. V., and Rotter, S. (2018). Associative properties of structural plasticity based on firing rate homeostasis in recurrent neuronal networks. Sci. Rep. 8:3754. doi: 10.1038/s41598-018-22077-3
Garcia-Iza, L., Martinez, Z., Ugarte, A., Fernandez, M., and Altuna, X. (2018). Cochlear implantation in the elderly: outcomes, long-term evolution, and predictive factors. Eur. Arch. Otorrinolaringol. 275, 913–922. doi: 10.1007/s00405-018-4910-y
Guzowski, J. F., Setlow, B., Wagner, E. K., and McGaugh, J. L. (2001). Experience-dependent gene expression in the rat hippocampus after spatial learning: a comparison of the immediate-early genes arc, c-fos, and zif268. J. Neurosci. 21, 5089–5098. doi: 10.1523/JNEUROSCI.21-14-05089.2001
Haensel, J., Ilgner, J., Chen, Y.-S., Thuermer, C., and Westhofen, M. (2005). Speech perception in elderly patients following cochlear implantation. Acta Otolaryngol. 125, 1272–1276. doi: 10.1080/00016480510044214
Happel, M. F. K., Niekisch, H., Castiblanco Rivera, L. L., Ohl, F. W., Deliano, M., and Frischknecht, R. (2014). Enhanced cognitive flexibility in reversal learning induced by removal of the extracellular matrix in auditory cortex. Proc. Natl. Acad. Sci. USA 111, 2800–2805. doi: 10.1073/pnas.1310272111
Heusinger, J., Hildebrandt, H., and Illing, R.-B. (2019). Sensory deafferentation modulates and redistributes neurocan in the rat auditory brainstem. Brain Behav. 9:e01353. doi: 10.1002/brb3.1353
Hiel, A.-L., Gerard, J.-M., Decat, M., and Deggouj, N. (2016). Is age a limiting factor for adaptation to cochlear implant? Eur. Arch. Otorrinolaringol. 273, 2495–2502. doi: 10.1007/s00405-015-3849-5
Hockfield, S., Kalb, R. G., Zaremba, S., and Fryer, H. (1990). Expression of neural proteoglycans correlates with the acquisition of mature neuronal properties in the mammalian brain. Cold Spring Harb. Symp. Quant. Biol. 55, 505–514. doi: 10.1101/sqb.1990.055.01.049
Illing, R.-B., Michler, S. A., Kraus, K. S., and Laszig, R. (2002). Transcription factor modulation and expression in the rat auditory brainstem following electrical intracochlear stimulation. Exp. Neurol. 175, 226–244. doi: 10.1006/exnr.2002.7895
Ingham, N. J., Thornton, S. K., Comis, S. D., and Withington, D. J. (1998). The auditory brainstem response of aged guinea pigs. Acta Otolaryngol. 118, 673–680. doi: 10.1080/00016489850183160
Irvine, D. R. F. (2018). Plasticity in the auditory system. Hear. Res. 362, 61–73. doi: 10.1016/j.heares.2017.10.011
Irvine, D. R. F., Rajan, R., and Smith, S. (2003). Effects of restricted cochlear lesions in adult cats on the frequency organization of the inferior colliculus. J. Comp. Neurol. 467, 354–374. doi: 10.1002/cne.10921
Jakob, T. F. (2011). Stimulationsabhängige Genexpression im zentralen auditorischen System. HNO 59, 230–238. doi: 10.1007/s00106-010-2250-3
Jakob, T. F., Döring, U., and Illing, R.-B. (2015). The pattern of Fos expression in the rat auditory brainstem changes with the temporal structure of binaural electrical intracochlear stimulation. Exp. Neurol. 266, 55–67. doi: 10.1016/j.expneurol.2015.02.015
Jakob, T., and Illing, R.-B. (2008). Laterality, intensity, and frequency of electrical intracochlear stimulation are differentially mapped into specific patterns of gene expression in the rat auditory brainstem. Audiol. Med. 6, 215–227. doi: 10.1080/16513860701875521
Jakob, T. F., Illing, R.-B., and Rosskothen-Kuhl, N. (2019). Monaural neonatal deafness induces inhibition among bilateral auditory networks under binaural activation. Neuroscience 400, 1–16. doi: 10.1016/j.neuroscience.2018.12.033
Jakob, T. F., Rosskothen-Kuhl, N., and Illing, R.-B. (2016). Induction of single-sided deafness in the newborn rat and its consequence for cochlear nucleus volume development. Hear. Res. 333, 210–215. doi: 10.1016/j.heares.2015.09.002
Jero, J., Coling, D. E., and Lalwani, A. K. (2001). The use of Preyer's reflex in evaluation of hearing in mice. Acta Otolaryngol. 121, 585–589. doi: 10.1080/000164801316878863
Kamke, M. R., Brown, M., and Irvine, D. R. F. (2003). Plasticity in the tonotopic organization of the medial geniculate body in adult cats following restricted unilateral cochlear lesions. J. Comp. Neurol. 459, 355–367. doi: 10.1002/cne.10586
Kandler, K., Clause, A., and Noh, J. (2009). Tonotopic reorganization of developing auditory brainstem circuits. Nat. Neurosci. 12, 711–717. doi: 10.1038/nn.2332
Kim, E. J., Feng, C., Santamaria, F., and Kim, J. H. (2019). Impact of auditory experience on the structural plasticity of the AIS in the mouse brainstem throughout the lifespan. Front. Cell. Neurosci. 13:456. doi: 10.3389/fncel.2019.00456
Kim, H., Kang, W. S., Park, H. J., Lee, J. Y., Park, J. W., Kim, Y., et al. (2018). Cochlear implantation in Postlingually deaf adults is time-sensitive towards positive outcome: prediction using advanced machine learning techniques. Sci. Rep. 8:18004. doi: 10.1038/s41598-018-36404-1
Koops, E. A., Renken, R. J., Lanting, C. P., and van Dijk, P. (2020). Cortical Tonotopic map changes in humans are larger in hearing loss than in additional tinnitus. J. Neurosci. 40, 3178–3185. doi: 10.1523/JNEUROSCI.2083-19.2020
Kotak, V. C., Fujisawa, S., Lee, F. A., Karthikeyan, O., Aoki, C., and Sanes, D. H. (2005). Hearing loss raises excitability in the auditory cortex. J. Neurosci. 25, 3908–3918. doi: 10.1523/JNEUROSCI.5169-04.2005
Kral, A., and Sharma, A. (2012). Developmental neuroplasticity after cochlear implantation. Trends Neurosci. 35, 111–122. doi: 10.1016/j.tins.2011.09.004
Kujawa, S. G., and Liberman, M. C. (2009). Adding insult to injury: cochlear nerve degeneration after "temporary" noise-induced hearing loss. J. Neurosci. 29, 14077–14085. doi: 10.1523/JNEUROSCI.2845-09.2009
Kwok, J. C. F., Dick, G., Wang, D., and Fawcett, J. W. (2011). Extracellular matrix and perineuronal nets in CNS repair. Dev. Neurobiol. 71, 1073–1089. doi: 10.1002/dneu.20974
Labadie, R. F., Carrasco, V. N., Gilmer, C. H., and Pillsbury, H. C. (2000). Cochlear implant performance in senior citizens. Otolaryngol. Head Neck Surg. 123, 419–424. doi: 10.1067/mhn.2000.109759
Leake, P. A., Hradek, G. T., Bonham, B. H., and Snyder, R. L. (2008). Topography of auditory nerve projections to the cochlear nucleus in cats after neonatal deafness and electrical stimulation by a cochlear implant. J. Assoc. Res. Otolaryngol. 9, 349–372. doi: 10.1007/s10162-008-0127-x
Leung, J., Wang, N.-Y., Yeagle, J. D., Chinnici, J., Bowditch, S., Francis, H. W., et al. (2005). Predictive models for cochlear implantation in elderly candidates. Arch. Otolaryngol. Head Neck Surg. 131, 1049–1054. doi: 10.1001/archotol.131.12.1049
Litovsky, R. Y., Goupell, M. J., Godar, S., Grieco-Calub, T., Jones, G. L., Garadat, S. N., et al. (2012). Studies on bilateral cochlear implants at the University of Wisconsin's binaural hearing and speech laboratory. J. Am. Acad. Audiol. 23, 476–494. doi: 10.3766/jaaa.23.6.9
Liu, H., Ding, D., Jiang, H., Wu, X., Salvi, R., and Sun, H. (2011). Ototoxic destruction by co-administration of kanamycin and ethacrynic acid in rats. J Zhejiang Univ Sci B 12, 853–861. doi: 10.1631/jzus.B1100040
Llano, D. A., Turner, J., and Caspary, D. M. (2012). Diminished cortical inhibition in an aging mouse model of chronic tinnitus. J. Neurosci. 32, 16141–16148. doi: 10.1523/JNEUROSCI.2499-12.2012
Lundin, K., Näsvall, A., Köbler, S., Linde, G., and Rask-Andersen, H. (2013). Cochlear implantation in the elderly. Cochlear Implants Int. 14, 92–97. doi: 10.1179/1754762812Y.0000000006
Mafi, A. M., Hofer, L. N., Russ, M. G., Young, J. W., and Mellott, J. G. (2020). The density of Perineuronal nets increases with age in the inferior colliculus in the Fischer Brown Norway rat. Front. Aging Neurosci. 12:27. doi: 10.3389/fnagi.2020.00027
Mann, Z. F., and Kelley, M. W. (2011). Development of tonotopy in the auditory periphery. Hear. Res. 276, 2–15. doi: 10.1016/j.heares.2011.01.011
Matsuda, K., Ueda, Y., Doi, T., Tono, T., Haruta, A., Toyama, K., et al. (1999). Increase in glutamate-aspartate transporter (GLAST) mRNA during kanamycin-induced cochlear insult in rats. Hear. Res. 133, 10–16. doi: 10.1016/s0378-5955(99)00050-7
McFadden, S. L., Campo, P., Quaranta, N., and Henderson, D. (1997). Age-related decline of auditory function in the chinchilla (Chinchilla laniger). Hear. Res. 111, 114–126. doi: 10.1016/s0378-5955(97)00099-3
Medina, M. D. M., Polo, R., Gutierrez, A., Muriel, A., Vaca, M., Perez, C., et al. (2017). Cochlear implantation in Postlingual adult patients with long-term auditory deprivation. Otol. Neurotol. 38, e248–e252. doi: 10.1097/MAO.0000000000001257
Möhrle, D., Ni, K., Varakina, K., Bing, D., Lee, S. C., Zimmermann, U., et al. (2016). Loss of auditory sensitivity from inner hair cell synaptopathy can be centrally compensated in the young but not old brain. Neurobiol. Aging 44, 173–184. doi: 10.1016/j.neurobiolaging.2016.05.001
Moon, I. S., Park, S., Kim, H.-N., Lee, W.-S., Kim, S. H., Kim, J.-H., et al. (2014). Is there a deafness duration limit for cochlear implants in post-lingual deaf adults? Acta Otolaryngol. 134, 173–180. doi: 10.3109/00016489.2013.850175
Morgan, J. I., and Curran, T. (1986). Role of ion flux in the control of c-fos expression. Nature 322, 552–555. doi: 10.1038/322552a0
Morgan, J. I., and Curran, T. (1991). Stimulus-transcription coupling in the nervous system: involvement of the inducible proto-oncogenes fos and Jun. Annu. Rev. Neurosci. 14, 421–451. doi: 10.1146/annurev.ne.14.030191.002225
Mosnier, I., Ferrary, E., Aubry, K., Bordure, P., Bozorg-Grayeli, A., Deguine, O., et al. (2020). The French National Cochlear Implant Registry (EPIIC): Cochlear implantation in adults over 65years old. Eur. Ann. Otorhinolaryngol. Head Neck Dis. 137, S19–S25. doi: 10.1016/j.anorl.2020.07.011
Mowery, T. M., Kotak, V. C., and Sanes, D. H. (2015). Transient hearing loss within a critical period causes persistent changes to cellular properties in adult auditory cortex. Cereb. Cortex 25, 2083–2094. doi: 10.1093/cercor/bhu013
Osako, S., Tokimoto, T., and Matsuura, S. (1979). Effects of kanamycin on the auditory evoked responses during postnatal development of the hearing of the rat. Acta Otolaryngol. 88, 359–368. doi: 10.3109/00016487909137180
Ouda, L., Profant, O., and Syka, J. (2015). Age-related changes in the central auditory system. Cell Tissue Res. 361, 337–358. doi: 10.1007/s00441-014-2107-2
Palombi, P. S., and Caspary, D. M. (1996). Physiology of the aged Fischer 344 rat inferior colliculus: responses to contralateral monaural stimuli. J. Neurophysiol. 76, 3114–3125. doi: 10.1152/jn.1996.76.5.3114
Persic, D., Thomas, M. E., Pelekanos, V., Ryugo, D. K., Takesian, A. E., Krumbholz, K., et al. (2020). Regulation of auditory plasticity during critical periods and following hearing loss. Hear. Res. 397:107976. doi: 10.1016/j.heares.2020.107976
Pienkowski, M., and Eggermont, J. J. (2011). Cortical tonotopic map plasticity and behavior. Neurosci. Biobehav. Rev. 35, 2117–2128. doi: 10.1016/j.neubiorev.2011.02.002
Raggio, M. W., and Schreiner, C. E. (1999). Neuronal responses in cat primary auditory cortex to electrical cochlear stimulation. III. Activation patterns in short- and long-term deafness. J. Neurophysiol. 82, 3506–3526. doi: 10.1152/jn.1999.82.6.3506
Rajan, R., and Irvine, D. (1998). Absence of plasticity of the frequency map in dorsal cochlear nucleus of adult cats after unilateral partial cochlear lesions. J. Comp. Neurol. 399, 35–46. doi: 10.1002/(SICI)1096-9861(19980914)399:1<35::AID-CNE3>3.0.CO;2-B
Rapanelli, M., Lew, S. E., Frick, L. R., and Zanutto, B. S. (2010). Plasticity in the rat prefrontal cortex: linking gene expression and an operant learning with a computational theory. PLoS One 5:e8656. doi: 10.1371/journal.pone.0008656
Rauch, A.-K., Rosskothen-Kuhl, N., and Illing, R.-B. (2016). Counter-regulation of the AP-1 monomers pATF2 and Fos: molecular readjustment of brainstem neurons in hearing and deaf adult rats after electrical intracochlear stimulation. Neuroscience 313, 184–198. doi: 10.1016/j.neuroscience.2015.11.025
Reisch, A., Illing, R.-B., and Laszig, R. (2007). Immediate early gene expression invoked by electrical intracochlear stimulation in some but not all types of neurons in the rat auditory brainstem. Exp. Neurol. 208, 193–206. doi: 10.1016/j.expneurol.2007.06.016
Resnik, J., and Polley, D. B. (2017). Fast-spiking GABA circuit dynamics in the auditory cortex predict recovery of sensory processing following peripheral nerve damage. eLife 6:e21452. doi: 10.7554/eLife.21452
Robertson, D., and Irvine, D. R. (1989). Plasticity of frequency organization in auditory cortex of guinea pigs with partial unilateral deafness. J. Comp. Neurol. 282, 456–471. doi: 10.1002/cne.902820311
Rosskothen, N., Hirschmüller-Ohmes, I., and Illing, R.-B. (2008). AP-1 activity rises by stimulation-dependent c-Fos expression in auditory neurons. Neuroreport 19, 1091–1093. doi: 10.1097/WNR.0b013e328303bbeb
Rosskothen-Kuhl, N., Buck, A. N., Li, K., and Schnupp, J. W. (2021). Microsecond interaural time difference discrimination restored by cochlear implants after neonatal deafness. eLife 10:e59300. doi: 10.7554/eLife.59300
Rosskothen-Kuhl, N., Hildebrandt, H., Birkenhäger, R., and Illing, R.-B. (2018). Astrocyte hypertrophy and microglia activation in the rat auditory midbrain is induced by electrical Intracochlear stimulation. Front. Cell. Neurosci. 12:43. doi: 10.3389/fncel.2018.00043
Rosskothen-Kuhl, N., and Illing, R.-B. (2010). Nonlinear development of the populations of neurons expressing c-Fos under sustained electrical intracochlear stimulation in the rat auditory brainstem. Brain Res. 1347, 33–41. doi: 10.1016/j.brainres.2010.05.089
Rosskothen-Kuhl, N., and Illing, R.-B. (2012). The impact of hearing experience on signal integration in the auditory brainstem: a c-Fos study of the rat. Brain Res. 1435, 40–55. doi: 10.1016/j.brainres.2011.11.046
Rosskothen-Kuhl, N., and Illing, R.-B. (2014). Gap43 transcription modulation in the adult brain depends on sensory activity and synaptic cooperation. PLoS One 9:e92624. doi: 10.1371/journal.pone.0092624
Roth, T. N., Hanebuth, D., and Probst, R. (2011). Prevalence of age-related hearing loss in Europe: a review. Eur. Arch. Otorrinolaringol. 268, 1101–1107. doi: 10.1007/s00405-011-1597-8
Ryan, A. F., Furlow, Z., Woolf, N. K., and Keithley, E. M. (1988). The spatial representation of frequency in the rat dorsal cochlear nucleus and inferior colliculus. Hear. Res. 36, 181–189. doi: 10.1016/0378-5955(88)90060-3
Sarro, E. C., Kotak, V. C., Sanes, D. H., and Aoki, C. (2008). Hearing loss alters the subcellular distribution of presynaptic GAD and postsynaptic GABAA receptors in the auditory cortex. Cereb. Cortex 18, 2855–2867. doi: 10.1093/cercor/bhn044
Seki, S., and Eggermont, J. J. (2002). Changes in cat primary auditory cortex after minor-to-moderate pure-tone induced hearing loss. Hear. Res. 173, 172–186. doi: 10.1016/s0378-5955(02)00518-x
Sergeyenko, Y., Lall, K., Liberman, M. C., and Kujawa, S. G. (2013). Age-related cochlear synaptopathy: an early-onset contributor to auditory functional decline. J. Neurosci. 33, 13686–13694. doi: 10.1523/JNEUROSCI.1783-13.2013
Sorg, B. A., Berretta, S., Blacktop, J. M., Fawcett, J. W., Kitagawa, H., Kwok, J. C. F., et al. (2016). Casting a wide net: role of Perineuronal nets in neural plasticity. J. Neurosci. 36, 11459–11468. doi: 10.1523/JNEUROSCI.2351-16.2016
Suttkus, A., Rohn, S., Jäger, C., Arendt, T., and Morawski, M. (2012). Neuroprotection against iron-induced cell death by perineuronal nets - an in vivo analysis of oxidative stress. Am. J. Neurodegener. Dis. 1, 122–129
Syka, J. (2002). Plastic changes in the central auditory system after hearing loss, restoration of function, and during learning. Physiol. Rev. 82, 601–636. doi: 10.1152/physrev.00002.2002
Takesian, A. E., Kotak, V. C., and Sanes, D. H. (2010). Presynaptic GABA(B) receptors regulate experience-dependent development of inhibitory short-term plasticity. J. Neurosci. 30, 2716–2727. doi: 10.1523/JNEUROSCI.3903-09.2010
Takesian, A. E., Kotak, V. C., and Sanes, D. H. (2012). Age-dependent effect of hearing loss on cortical inhibitory synapse function. J. Neurophysiol. 107, 937–947. doi: 10.1152/jn.00515.2011
Vale, C., and Sanes, D. H. (2002). The effect of bilateral deafness on excitatory and inhibitory synaptic strength in the inferior colliculus. Eur. J. Neurosci. 16, 2394–2404. doi: 10.1046/j.1460-9568.2002.02302.x
Vale, C., Schoorlemmer, J., and Sanes, D. H. (2003). Deafness disrupts chloride transporter function and inhibitory synaptic transmission. J. Neurosci. 23, 7516–7524. doi: 10.1523/JNEUROSCI.23-20-07516.2003
Vermeire, K., Brokx, J. P. L., Wuyts, F. L., Cochet, E., Hofkens, A., and van de Heyning, P. H. (2005). Quality-of-life benefit from cochlear implantation in the elderly. Otol. Neurotol. 26, 188–195. doi: 10.1097/00129492-200503000-00010
Vischer, M. W., Häusler, R., and Rouiller, E. M. (1994). Distribution of Fos-like immunoreactivity in the auditory pathway of the Sprague-Dawley rat elicited by cochlear electrical stimulation. Neurosci. Res. 19, 175–185. doi: 10.1016/0168-0102(94)90141-4
Von Gablenz, P., Hoffmann, E., and Holube, I. (2017). Prävalenz von Schwerhörigkeit in Nord- und Süd-Deutschland. HNO 65, 130–135. doi: 10.1007/s00106-016-0318-4
WHO (2024). Deafness and hearing loss. Available at: https://www.who.int/news-room/fact-sheets/detail/deafness-and-hearing-loss.
Willott, J. F. (1984). Changes in frequency representation in the auditory system of mice with age-related hearing impairment. Brain Res. 309, 159–162. doi: 10.1016/0006-8993(84)91022-9
Keywords: aging, adult hearing loss, auditory brainstem, tonotopy, cochlear implant, cochlear nucleus, lateral superior olive, inferior colliculus
Citation: Rosskothen-Kuhl N, Green S and Jakob TF (2024) Does age protect against loss of tonotopy after acute deafness in adulthood? Front. Cell. Neurosci. 18:1424773. doi: 10.3389/fncel.2024.1424773
Edited by:
Christian Keine, University of Oldenburg, GermanyReviewed by:
Kristen Rak, Comprehensive Hearing Center, GermanyKelsey L. Anbuhl, New York University, United States
Copyright © 2024 Rosskothen-Kuhl, Green and Jakob. This is an open-access article distributed under the terms of the Creative Commons Attribution License (CC BY). The use, distribution or reproduction in other forums is permitted, provided the original author(s) and the copyright owner(s) are credited and that the original publication in this journal is cited, in accordance with accepted academic practice. No use, distribution or reproduction is permitted which does not comply with these terms.
*Correspondence: Nicole Rosskothen-Kuhl, bmljb2xlLnJvc3Nrb3RoZW4ta3VobEB1bmlrbGluaWstZnJlaWJ1cmcuZGU=
†Present address: Sarah Green, Max Delbrück Center for Molecular Medicine (MDC), Berlin, Germany