- 1Department of Psychiatry, Yale University School of Medicine, New Haven, CT, United States
- 2Yale Child Study Center, Yale University School of Medicine, New Haven, CT, United States
- 3Department of Psychology, Yale School of Arts and Sciences, New Haven, CT, United States
- 4Center for Brain and Mind Health, Yale University School of Medicine, New Haven, CT, United States
- 5Wu-Tsai Institute, Yale University, New Haven, CT, United States
Introduction: Dysfunction of the cortico-basal circuitry – including its primary input nucleus, the striatum – contributes to neuropsychiatric disorders, such as autism and Tourette Syndrome (TS). These conditions show marked sex differences, occurring more often in males than in females. Regulatory interneurons, such as cholinergic interneurons (CINs) and parvalbumin-expressing GABAergic fast spiking interneurons (FSIs), are implicated in human neuropsychiatric disorders such as TS, and ablation of these interneurons produces relevant behavioral pathology in male mice, but not in females. Here we investigate sex differences in the density and distribution of striatal interneurons.
Methods: We use stereological quantification of CINs, FSIs, and somatostatin-expressing (SOM) GABAergic interneurons in the dorsal striatum (caudate-putamen) and the ventral striatum (nucleus accumbens) in male and female mice.
Results: Males have a higher density of CINs than females, especially in the dorsal striatum; females have equal distribution between dorsal and ventral striatum. FSIs showed similar distributions, with a greater dorsal-ventral density gradient in males than in females. SOM interneurons were denser in the ventral than in the dorsal striatum, with no sex differences.
Discussion: These sex differences in the density and distribution of FSIs and CINs may contribute to sex differences in basal ganglia function, particularly in the context of psychopathology.
Introduction
The cortico-basal circuitry, particularly the striatum (caudate, putamen, and nucleus accumbens), is a locus of pathology in numerous neuropsychiatric disorders, such as autism and Tourette’s Syndrome (TS) (Swerdlow and Koob, 1987; Macpherson and Hikida, 2019; Chen et al., 2022). The striatum is the primary input nucleus and a central hub of the basal ganglia circuitry; it is implicated in numerous neural processes, including motion, effort, habit formation, motivation, and social behavior (Graybiel et al., 1994; Pennartz et al., 2009; Graybiel and Grafton, 2015; Bamford and Bamford, 2019; Suzuki et al., 2021).
The striatum is composed of GABAergic medium spiny neurons (MSNs, comprising ~95% of the neurons in the striatum in rodents) (Gerfen and Wilson, 1996) alongside several populations of regulatory interneurons. These include ChAT-expressing cholinergic interneurons (CINs) and multiple subtypes of GABAergic interneurons, typically identified by their expression of markers such as parvalbumin (PV) and somatostatin (SOM) (Kawaguchi et al., 1995; Kawaguchi, 1997; Marin et al., 2000; Muñoz-Manchado et al., 2018). CINs innervate various GABAergic interneurons, such as PV-expressing fast-spiking interneurons (FSIs) (Chang and Kita, 1992; Kocaturk et al., 2022). They also reciprocally regulate dopamine release (Yorgason et al., 2017; Howe et al., 2019; Chantranupong et al., 2023) and can regulate excitatory glutamatergic input to MSNs (Pakhotin and Bracci, 2007; Ian Antón and Jun, 2011). FSIs are important regulators responsible for fine tuning MSN firing (Bennett and Bolam, 1994; Orduz et al., 2013) and integrate with other interneurons in coordinating movement bouts (Gritton et al., 2019) and regulating behavior (Lee et al., 2017). SOM interneurons play a complex role in regulating MSNs, FSIs, and CINs (Momiyama and Zaborszky, 2006; Cattaneo et al., 2019) and interact with dopaminergic afferents (Hathway et al., 1999; Gazan et al., 2020). Despite being a small percentage of striatal neurons, these interneurons critically regulate striatal function and behavioral output.
Interneuron pathology has been implicated in numerous neuropsychiatric disorders (Kataoka et al., 2010; Rapanelli et al., 2017a; Poppi et al., 2021; Brady et al., 2022). Deficits in cholinergic function are implicated in Parkinson’s, Huntington’s, and Alzheimer’s Diseases, schizophrenia, bipolar disorder, and attention deficit-hyperactivity disorder (Lim et al., 2014). Postmortem studies in human patients with TS have shown significant reductions in striatal CINs and PV-expressing GABAergic interneurons (Kalanithi et al., 2005; Kataoka et al., 2010; Lennington et al., 2016).
Many of these conditions affect males and females differentially; for example, TS is diagnosed approximately twice as often in males as in females (Martino et al., 2013; Scahill et al., 2014). Whether striatal interneuron pathology is similarly sexually dimorphic is unknown, but preclinical evidence is beginning to suggest that it may be. The striatal circuitry can be impacted by the estrous cycle (Cao et al., 2018; Zachry et al., 2021); both GABAergic and cholinergic interneurons express estrogen receptors (ERs) (Almey et al., 2012, 2016). Furthermore, depletion of CINs during development (Cadeddu et al., 2023), or conjoint depletion of both CINs and FSIs in adults (Rapanelli et al., 2017b), produces dysregulated striatal activity and behavioral abnormalities of potential relevance to TS and related conditions – repetitive behavioral pathology, anxiety, and social deficits – in male mice, but not in females. This suggests an underlying sex difference in striatal interneurons, their regulation of local microcircuits, and their role in the modulation of striatum-dependent behaviors.
Sex differences have been described in several aspects of the striatal circuitry. MSNs exhibit significantly higher density and size of dendritic spines in females than in males (Forlano and Woolley, 2010); estrogen modulates spine density (Staffend et al., 2011; Peterson et al., 2015). MSN excitability varies with estrous phase, with higher spontaneous EPSC frequencies during the estrous and proestrus phases (Proaño et al., 2018; Aziz and Mangieri, 2023). Endogenous striatal dopamine levels fluctuate with the estrous cycle (Castner et al., 1993; Xiao and Becker, 1994); estrogen has been shown to potentiate dopamine release (Becker and Beer, 1986; Yoest et al., 2018), and females have higher striatal dopamine release and cycling (Walker et al., 2000; Dluzen and McDermott, 2008; Calipari et al., 2017; Zachry et al., 2021). There is evidence of sexually different estrogen interaction with PV-expressing interneurons in development in other brain regions (Blurton-Jones and Tuszynski, 2002; Sotonyi et al., 2010; Wu et al., 2014). However, whether these sex differences extend to regulatory interneurons of the striatum, such as CINs and FSIs, is not yet clear.
Here, using mice as a model system, we provide the first rigorous comparison between males and females of the density and distribution of three different subtypes of striatal interneurons implicated in striatal function and in neuropsychiatric diseases: PV- and SOM-expressing GABAergic interneurons and CINs.
Materials and methods
Experimental design
All animal use followed protocols approved by Yale’s Institutional Animal Care and Use Committee. Adult male and female wild-type C57BL/6 mice (10–14 weeks old) were group housed in our animal facility in self-ventilating cages, maintained on a 12 h light/dark cycle, and provided with food and water ad libitium.
For histology, mice were anesthetized (ketamine 100 mg/kg and xylazine 10 mg/kg, followed by isoflurane inhalation until no response to noxious stimulus) followed by transcardial perfusion with 4% paraformaldehyde in phosphate buffered saline (PBS) (Thermo Fisher) and brain extraction. Cryostat sections were cut at 30 μm and mounted on Diamond White Glass Slides (Globe). For immunostaining for identification and counting of interneurons, slides were incubated overnight at room temperature with primary antibodies diluted in 0.1 M PBS containing 10% normal goat serum (Vector Labs) and 1% Triton-X 100 (AmericanBio). Primary antibodies were used as follows: Rabbit recombinant monoclonal anti-somatostatin (1:250, Abcam, ab111912), mouse monoclonal anti-ChAT (1:250, Thermo Fisher, MA5-31383), rabbit polyclonal anti-PV (1:250, Abcam, ab11427). Detection was performed with appropriate secondary antibodies: polyclonal goat anti-rabbit coupled to Alexa Fluor 488 (1:1,000, Thermo Fisher, A-11008), polyclonal goat anti-rabbit coupled to Alexa Fluor 568 (1:1,000, Thermo Fisher, A-11011), recombinant polyclonal goat anti-mouse coupled to Alexa Fluor 488 (Thermo Fisher, A-28175), and polyclonal goat anti-mouse coupled to Alexa Fluor 568 (1:1,000, Thermo Fisher, A-11004). Sections were then mounted and coverslipped in ProLong Gold with DAPI (Life Technologies).
Immunostained coronal striatal sections (30 μm, bregma 1.1–0.50 mm) were visualized on a Zeiss Scope. A1 using a Plan-APOCHROMAT 10x objective and an Axiocam 503 Mono at 100× magnification. Microscope fields were systematically tiled over the entire dorsal and ventral striatum and stitched together using the stitching plugin in FIJI (Schindelin et al., 2012) to provide a high-resolution image throughout the extent of the structure. Only undamaged sections with consistent immunostaining through the full extent of the striatum were included in analysis. Sections were analyzed blind to sex. Minor variances in background staining can occur with image stitching, but these did not interfere with quantification as stained neurons remained well-defined and distinct from background.
Whole striatal slice reconstructions were imported into StereoInvestigator 10 (MBF Biosciences) and regions of interest were defined. Sections were selected from the anterior striatum, between bregma 1.10–0.50 mm, where the NAcc is well represented. For whole striatal analyses, the entire anatomical area of the striatum contained in the image, bounded by the internal capsule/corpus callosum white matter and other landmarks following the atlas of Franklin and Paxinos (1997), was selected for quantification. For dorsal/ventral subregion analyses we identified caudate-putamen (CPu) and nucleus accumbens (NAcc) following Franklin and Paxinos (1997) and separately quantified interneuron density in these subregions. To further characterize dorsal-ventral differences in interneurons density, in a secondary analysis the striatum was divided in half by a horizontal line placed halfway between the dorsal and ventral extent of the structure in each slice (see Supplementary Figures 1A–C); quantification of interneurons using this arbitrary division of the striatum is presented in Supplementary material. Neurons were counted using the fractionator tool in StereoInvestigator using a counting box size of 100 × 100 μm, excluding all neurons on the dashed bounding line. Only neurons with clearly defined soma distinct from background were counted. Data collected from left and right striatum were averaged. In total, 22 male and 17 female brains were analyzed, with two randomly selected sections per animal from within the range specified above.
Statistics
All data were imported into JASP Statistical Software (Eric-Jan Wagenmakers, University of Amsterdam) for statistical analysis. Data was tested for normality using the D’Agostino & Pearson test and outliers were excluded. For direct comparisons between sexes for whole striatum and to compare ratios of dorsal to ventral interneurons, a two-tailed unpaired t-test was performed. To compare between sexes for the dorsal and ventral sub-regions of the striatum, a two-way repeated-measures ANOVA was used, followed by Fisher’s LSD post-hoc test. Graphs were generated using GraphPad Prism 10 (Graph Pad Software, LLC). Whiskers on bar and whisker plots indicate the min and max values, with all data points represented on graphs.
Results
We first quantified CIN density in male and female mice (Figures 1A–D). Male mice had a significantly higher density of ChAT+ interneurons in the whole striatum (Figure 1E; two-tailed t-test, t[26] = 2.305, p = 0.031). Males and females showed different patterns of CIN distribution in anatomically defined subregions, the CPu and NAcc. There were significant effects of both sex (Figure 1F; Repeated Measures ANOVA: F(1, 28) = 4.769, p = 0.037) and subregion (F(1, 28) = 5.991 = 5.991, p = 0.021), with no interaction (sex × subregion F(1, 28) = 0.6137, p = 0.44). The density of ChAT interneurons in the female striatum was not different in the CPu and the NAcc (Fisher’s LSD, p = 0.278). In contrast, males showed significantly higher density of ChAT+ neurons in the CPu compared to the NAcc (Fisher’s LSD: p = 0.026). Males had higher overall CIN density in the CPu than females (Fisher’s LSD: p = 0.021), but there were no differences in the NAcc (Fisher’s LSD: p = 0.279) (Figure 1G).
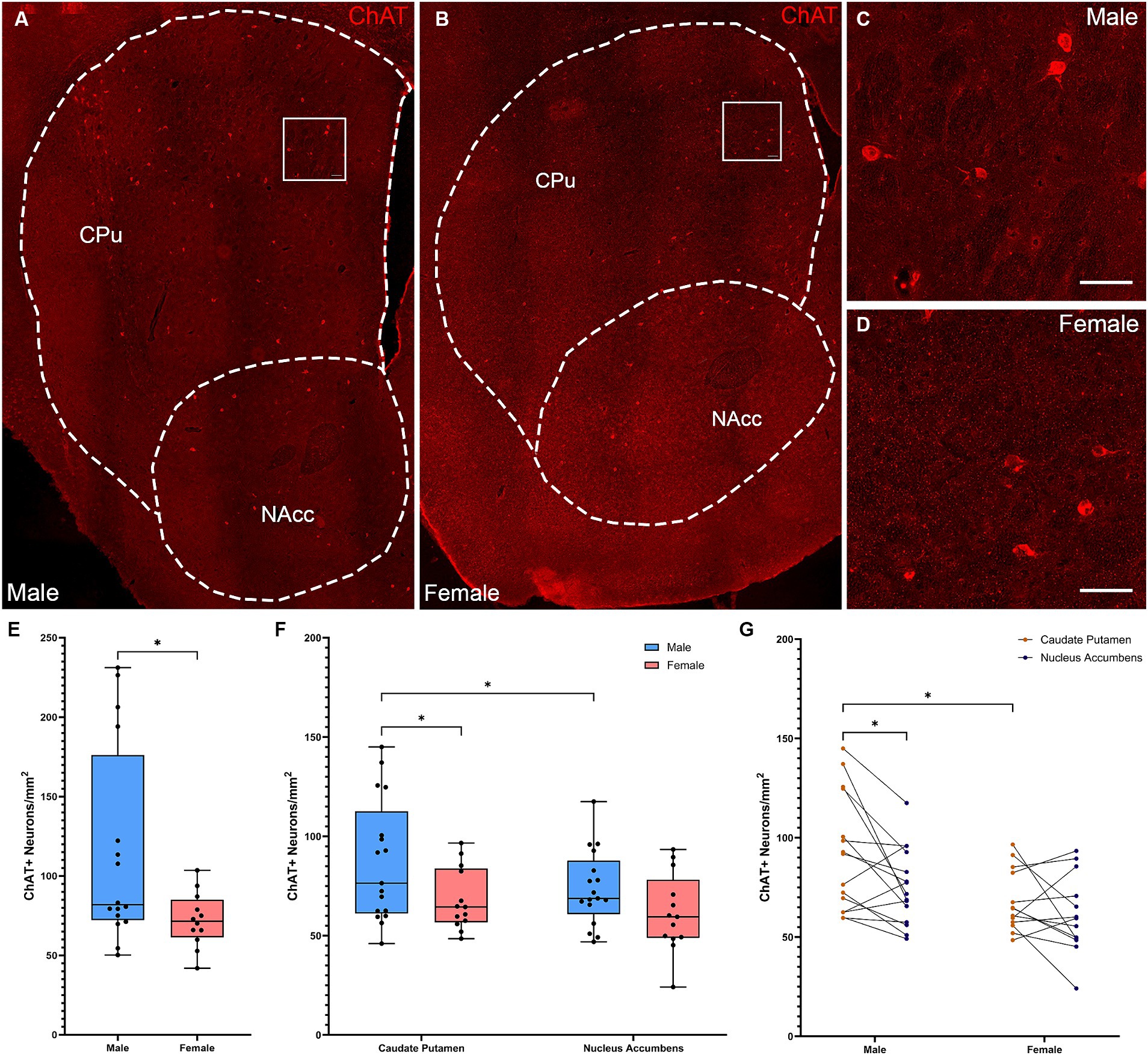
Figure 1. Cholinergic interneurons are denser and show a dorsally skewed distribution in the striatum of male versus female mice. (A) Representative whole striatum reconstruction of high-resolution microscope images of ChAT-expressing interneurons in a male mouse brain, with dashed lines indicating counting regions for CPu and NAcc. (B) Representative whole striatum reconstruction of high-resolution microscope images of ChAT-expressing interneurons in a female mouse brain, with dashed lines indicating counting regions for CPu and NAcc. (C) Higher resolution image of selected region from (A) showing CINs in the male CPu. (D) Higher resolution image of selected region from (B) showing CINs in the female CPu. (E) Stereological quantification of the density of ChAT+ interneurons per mm2 over the whole mouse striatum showing a higher density of ChAT+ neurons in males compared to females (*Two-tailed t-test, t[26] = 2.305, p = 0.029). (F) Quantification of the density of ChAT+ interneurons divided over the CPu and NAcc sub-regions of the striatum reveals a significant effect of subregion (Repeated Measures ANOVA, F[1, 28] = 5.991, p = 0.021) and sex (F[1, 28] = 4.769, p = 0.038); without interaction (F[1, 28] = 0.614, p = 0.44). Males showed significantly higher ChAT+ interneuron density in the CPu compared to the NAcc (*Fisher’s LSD: p = 0.021) while females did not (p = 0.278) and there was higher density of ChAT in the male CPu compared to the female CPu (p = 0.026). (G) Alternate representation of data from (F) showing density gradients from the CPu to the NAcc indicating a significant gradient from CPu to NAcc in males that is not apparent in females. Scalebars equal 20 μm.
To further test for a dorsal-ventral CIN gradient, we also analyzed CIN density in dorsal and ventral striatal subregions, defined by an arbitrary line across the middle of the structure (see Methods). This analysis confirmed the differential distribution of CINs in males and females (Supplementary Figures 1A,D–E; Repeated measures ANOVA: subregion × sex interaction: F(1, 24) = 9.012, p = 0.006; effect of subregion: F(1, 24) = 20.42, p < 0.001, trend of effect of sex: F(1, 26) = 3.996, p = 0.056). CINs exhibited a significant dorsal-ventral gradient in the male striatum (Fisher’s LSD: p < 0.001), but not in females (Fisher’s LSD: p = 0.311). CINs were denser in male dorsal striatum than in females (Fisher’s LSD: p = 0.006); this pattern was not seen ventrally (p = 0.36).
Quantification of overall density of PV+ interneurons in whole striatum (Figures 2A–D) did not reveal significant differences in density between males and females (Figure 2E; t-test, t[36] = 0.595; p = 0.56). However, analyzing across subregions (Figures 2A–C) revealed a different distribution in males and females (Figures 2F–G; Repeated Measures ANOVA: main effect of subregion, F(1, 29) = 57.06, p < 0.001; sex × subregion interaction, F(1, 29) = 7.745, p = 0.009). Both sexes showed significantly higher density of PV+ interneurons in the CPu than in the NAcc (Fisher’s LSD: males p < 0.001, females p = 0.0013); males showed a significantly higher density of PV+ interneurons in the CPu than females (Fisher’s LSD: p = 0.011). Similar effects were seen when cell density was analyzed in arbitrary dorsal and ventral subregions (Supplementary Figures 1B,F,G).
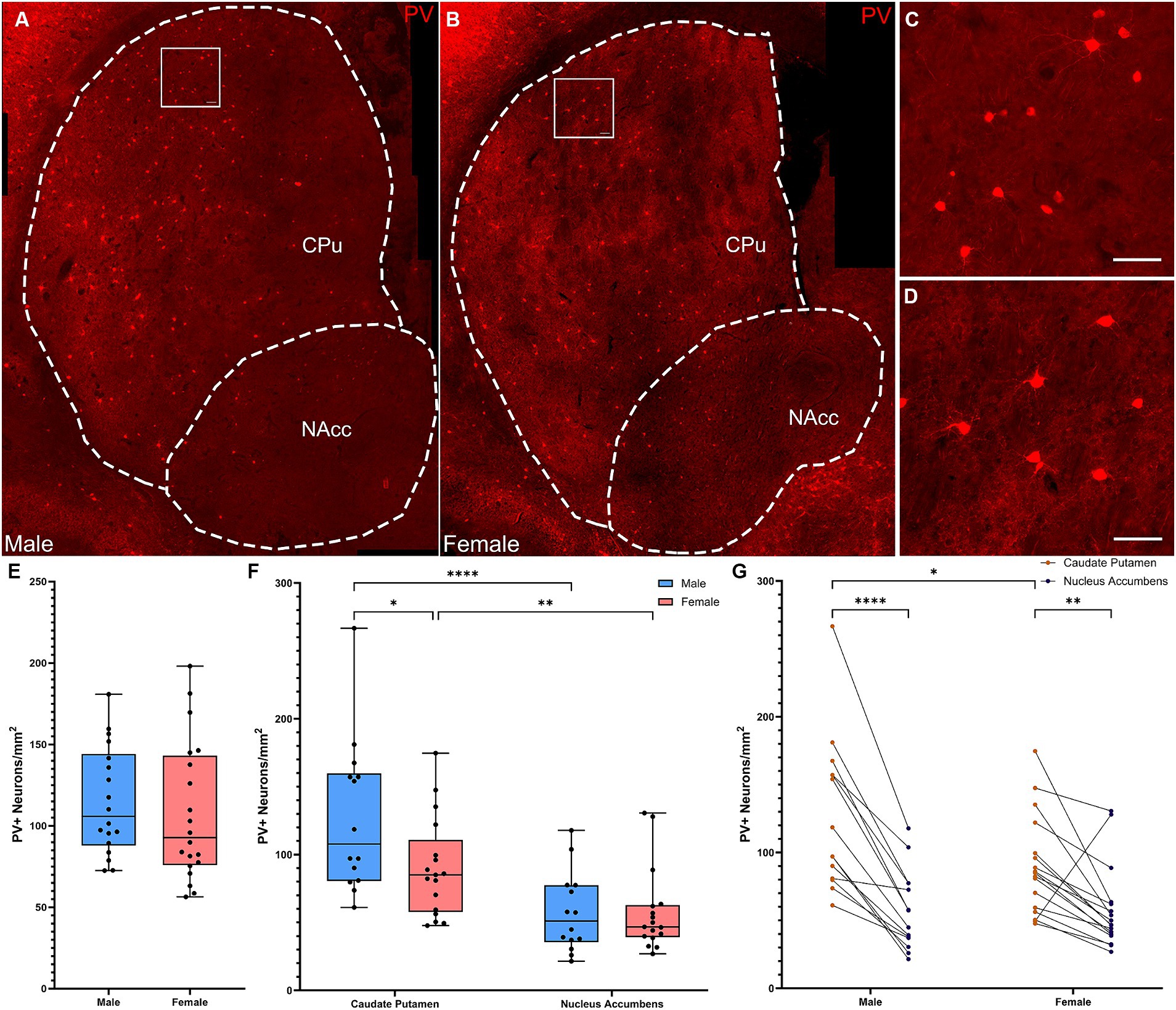
Figure 2. Parvalbumin-expressing interneurons are more concentrated in the dorsal versus the ventral striatum in male, but not female mice. (A) Representative whole striatum reconstruction of high-resolution microscope images of PV-expressing interneurons in a male mouse brain, with dashed lines indicating counting regions for CPu and NAcc. (B) Representative whole striatum reconstruction of high-resolution microscope images of PV-expressing interneurons in a female mouse brain, with dashed lines indicating counting regions for CPu and NAcc. (C) Higher resolution image of selected region from (A) showing PV+ interneurons in the male CPu. (D) Higher resolution image of selected region from (B) showing PV+ interneurons in the female CPu. (E) Quantification of the density of PV+ interneurons per mm2 over the whole mouse striatum using stereological methods shows similar densities of PV+ neurons between sexes (Two-tailed t-test, t[36] = 0.595; p = 0.56). (F) Quantification of the density of PV+ interneurons divided over the CPu and NAcc sub-regions of the striatum reveals a significant effect of subregion (Repeated Measures ANOVA, F(1, 29) = 57.06, p < 0.001) and a significant interaction of sex and subregion [F(1, 29) = 7.745, p = 0.009]. Males showed significantly higher PV+ interneuron density in the CPu compared to the NAcc (****Fisher’s LSD: p < 0.001) while females showed a similar, but less robust gradient (**p = 0.001). PV+ interneurons in the CPu were significantly denser in males than in females (*p = 0.011), but there were no differences in density in the NAcc (p = 0.97). (G) Alternate representation of data from (F) showing density gradients of PV+ interneurons from the CPu to the NAcc. Both males and females showed a dorsal to ventral gradient of PV interneurons, however this was significantly higher in males. Scalebars equal 20 μm.
SOM+ interneuron densities across the full striatum did not differ between males and females (Figures 3A–E, t-test: t[27] = 0.515, p = 0.61). Quantification of SOM+ interneurons by subregion (Figures 3F,G) showed a significant effect of subregion (Figure 3F; Repeated Measures ANOVA: F(1, 23) = 27.45, p < 0.001), but no effect of sex or subregion × sex interaction. Both males (Fisher’s LSD: p = 0.001) and females (Fisher’s LSD: p = 0.001) had significantly more SOM interneurons in the NAcc than in the CPu. A similar pattern was seen when cell density was analyzed in arbitrary dorsal and ventral subregions (Supplementary Figures 1C,H,I).
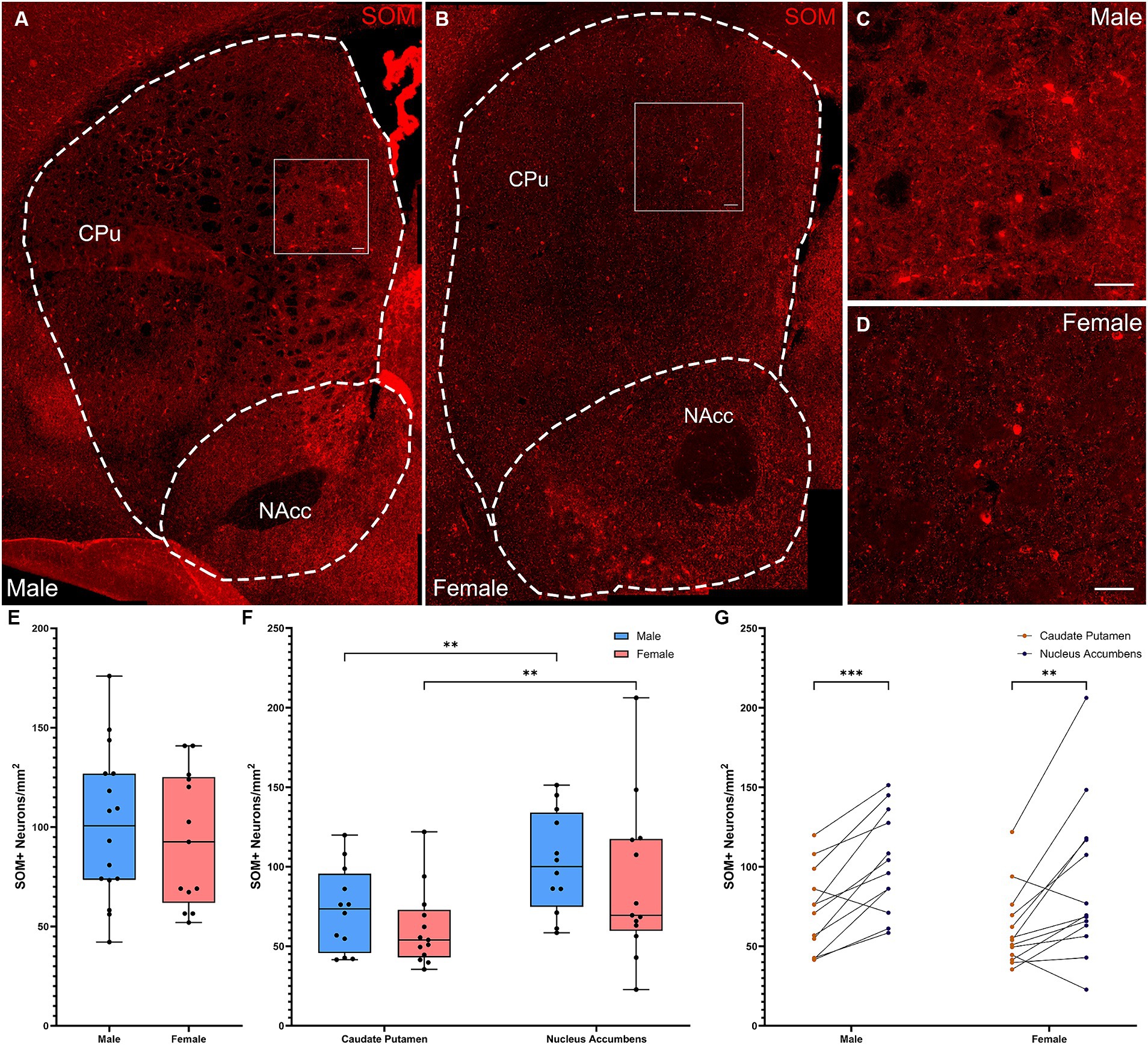
Figure 3. Somatostatin-expressing interneurons are denser in the ventral striatum in both male and female mice. (A) Representative whole striatum reconstruction of high-resolution microscope images of SOM-expressing interneurons in a male mouse brain, with dashed lines indicating counting regions for CPu and NAcc. (B) Representative whole striatum reconstruction of high-resolution microscope images of SOM-expressing interneurons in a female mouse brain, with dashed lines indicating counting regions for CPu and NAcc. (C) Higher resolution image of selected region from (A) showing SOM+ interneurons in the male CPu. (D) Higher resolution image of selected region from (B) showing SOM+ interneurons in the female CPu. (E) Quantification of the density of SOM+ interneurons per mm2 over the whole mouse striatum using stereological methods, showing similar densities of SOM+ neurons between sexes (Two-tailed t-test, t[27] = 0.515, p = 0.61). (F) Quantification of the density of SOM+ interneurons divided over the CPu and NAcc sub-regions of the striatum reveals a significant effect of subregion (Repeated Measures ANOVA, F(1, 23) = 27.45, p < 0.001) and no effect of sex [F(1, 23) = 0.9600, p = 0.337] or interaction [F(1, 23) = 0.01914, p = 0.891]. Both males and females showed significantly higher SOM+ interneuron density in the NAcc compared to the CPu (**Fisher’s LSD: p = 0.001 for both) with no sex differences in density for either the CPu (p = 0.404) or the NAcc (p = 0.346). (G) Alternate representation of the data from (F) showing a significant density gradient from the NAcc to the CPu in both males and females of equal significance (Fisher’s LSD: ***p = 0.0003, **p = 0.009). Scalebars equal 20 μm.
Discussion
Interneurons of the striatum are critical to basal ganglia function (Kreitzer, 2009) and have emerged as a locus of pathology in a number of neuropsychiatric conditions (Rapanelli et al., 2017a; Poppi et al., 2021). Biological sex modulates basal ganglia structure and function (Giedd et al., 1997; Rijpkema et al., 2012), and numerous studies show sex differences in conditions in which basal ganglia dysregulation is implicated, including TS (Martino et al., 2013; Scahill et al., 2014) and autism (Loomes et al., 2017). However, no studies to date have systematically examined sex differences in the distribution, structure, or function of striatal interneurons.
Several populations of striatal interneuron have been found to be reduced in number in post-mortem studies of individuals with persistent adult TS (Kalanithi et al., 2005; Kataoka et al., 2010; Lennington et al., 2016); these studies included both male and female subjects but were not designed to detect sexually dimorphic effects. In mice, we have found experimental depletion of CINs, PV-expressing FSIs, or both to destabilize the striatum, resulting in repetitive behavioral pathology, social deficits, and other behavioral abnormalities (Xu et al., 2015, 2016; Rapanelli et al., 2017b; Cadeddu et al., 2023). Interestingly, these effects are sexually dimorphic, appearing in male but not female mice, despite equivalent levels of interneuron depletion (Rapanelli et al., 2017b; Cadeddu et al., 2023). The underlying differences in striatal anatomy or function that lead to this differential susceptibility remain unclear.
Here, we document intriguing differences between male and female adult mice in striatal interneuron density and distribution. Differential density and distribution of interneurons suggest differences in striatal function, and perhaps in resilience and vulnerability to pathophysiological processes.
CINs play a central role in the striatal microcircuitry. CINs regulate the release of both dopamine (Cachope et al., 2012; Threlfell et al., 2012) and GABA (Nelson et al., 2014) from dopaminergic afferents from the substantia nigra. They also modulate multiple populations of GABAergic interneurons, as well as MSNs (Poppi et al., 2021). We find a significant difference between sexes in both density and distribution of these interneurons: The density of CINs is significantly higher in the CPu in males than in females. The existence of a dorsal to ventral gradient of CINs in males is consistent with previous findings in males (Phelps et al., 1989; Matamales et al., 2016); CIN density in females has not previously been rigorously quantified. Our findings match observations in previous studies, in which a higher CIN density was observed in males than in females (Rapanelli et al., 2017b; Cadeddu et al., 2023); however, these previous observations were incidental, without the careful quantification applied here. While female mice have relatively even distribution of ChAT+ interneurons between the dorsal and ventral striatum, males a greater density in the CPu compared to the NAcc.
The presence of more CINs in the dorsal striatum of males than of females is perhaps surprising, as our previous work shows that males are more sensitive to disruption of these cells (Rapanelli et al., 2017b; Cadeddu et al., 2023). It might have been expected that a higher basal density of CINs would render males more resilient to CIN depletion. The current data suggest a different model, that the dorsal regulatory circuitry in males may be more reliant on CINs than that of females and thus is less able to accommodate pathological CIN disruption.
PV-expressing FSIs are highly active interneurons innervating a wide variety of targets in the striatum through both inhibition and disinhibition circuits (Duhne et al., 2021), and are particularly important in regulating MSNs through feedforward inhibition (Koós and Tepper, 1999; Gittis et al., 2010, 2011; Adler et al., 2013; Lee et al., 2017). There is previous evidence of a dorsoventral gradient of PV distribution (Zahm et al., 2003) in addition to a rostrocaudal gradient (Wu and Parent, 2000); sex effects have not previously been examined. We find effects of both sex and striatal subregion on FSI density. Male mice again exhibit a significantly higher density in the dorsal CPu than in the ventral NAcc; females also show a difference in density in the same direction, but it is less robust. Because FSIs are an important source of inhibition in the striatum, this may suggest that the male and female striatum differ in their excitatory-inhibitory balance. We have previously found depletion of FSIs in the dorsal striatum to produce both repetitive behavioral pathology and elevated anxiety-like behavior (Xu et al., 2016), though it is not yet clear if these effects are specific to males. When both FSIs and CINs are depleted, behavioral effects are seen only in males, suggesting that there may be a sex-specific effect (Rapanelli et al., 2017b).
FSIs and CINs separately regulate MSN activity (Adler et al., 2013; Gritton et al., 2019), and each other (Chang and Kita, 1992; DeBoer and Westerink, 1994; Faust et al., 2016). Sex differences in density and distribution follows the same general pattern for both interneuron types, with higher density in the dorsal CPu region of the striatum and a greater dorsal-ventral gradient toward the NAcc in males than in females in both cases. This may suggest a single developmental process underlying both effects.
We found no sex differences in the density or distribution of SOM-expressing GABAergic interneurons. However, both males and females had more SOM-expressing interneurons in the NAcc compared to the CPu. This pattern, quite distinct from that seen with CINs and FSIs, indicates that the sex differences are not uniform across all components of the striatal microcircuitry but rather differentially affect distinct cells and processes. This reinforces the idea that differential density, distribution, and function of interneurons in males and females leads to distinct regional differences in information processing in the basal ganglia circuitry.
The processes that lead to sex differences in striatal interneurons remain to be unraveled. During development, neuronal loss and dendritic pruning differ between males and females in other brain regions (Markham et al., 2007; Koss et al., 2014; Willing and Juraska, 2015; Drzewiecki et al., 2016); similarly differential cell loss in the striatum may explain our findings. Differences in interneuron number and distribution might derive directly or indirectly from the differential expression of sex hormones in males and females, or from genetic or other non-hormonal factors associated with sex. Sex hormones can influence the remodeling of brain circuits during puberty (Nuñez et al., 2002; Koss et al., 2015). Sex differences in PV-expressing interneurons in the hippocampus are mediated through ERs (Wu et al., 2014), and estrogen can promote PV expression on proopiomelanocortin-expressing neurons of the arcuate nucleus (Sotonyi et al., 2010). In the striatum, ERs are strongly expressed during development, with higher levels in females; expression levels decline in adulthood (Drzewiecki et al., 2021; Krentzel et al., 2021). Estrogen has a clear role in MSNs, where it modulates both spine density and neural activity (Mermelstein et al., 1996; Hu et al., 2006; Schultz et al., 2009), and it modulates striatal dopamine during the estrous cycle (Becker and Cha, 1989; Xiao and Becker, 1994; Calipari et al., 2017; Yoest et al., 2018; Zachry et al., 2021) as well as dopamine-dependent synaptic plasticity (Tozzi et al., 2015). The role of estrogen in striatal interneurons is less clear, although ERs are expressed on CINs (Almey et al., 2012) and in PV interneurons in numerous other brain regions (Blurton-Jones and Tuszynski, 2002; Wu et al., 2014). In summary, there are numerous examples of sex hormones modulating brain development, and specifically regulating interneurons in other brain regions. Whether similar mechanisms operate in the striatum remains to be tested.
This study has several limitations that should be addressed in future work. First, while we used stereological tools to ensure that we did not overcount cells in the examined striatal slices, we did not systematically reconstruct the full anterior–posterior extent of the striatum, and thus we cannot comment on anterior–posterior gradients or calculate the total number of interneurons in the male or female striatum, or its subregions. Second, we rely on the expression of specific markers to identify interneurons; our counts are thus susceptible to error if these markers are differentially expressed in males and females. That said, staining was strong and was qualitatively similar in males and females (see representative micrographs in all figures). Finally, we have examined interneuron number but not more granular aspects of their structure, function, or integration into local microcircuitry. These are important directions for future studies.
In sum, we find sex differences in the density and distribution of striatal interneurons, with a higher density of both CINs and FSIs in the dorsal striatum in males. The mechanisms underlying these differential patterns of interneuron density and distribution remain to be elucidated but are likely to involve sexually dimorphic modulation of developmental processes. Of note, ERs are expressed on both cholinergic (Almey et al., 2012; Kövesdi et al., 2022) and GABAergic interneurons (Almey et al., 2016), providing a potential mechanism for sex hormones to regulate interneuron function. These findings represent a starting point for future work analyzing the impact of these differences on sex dependent outcomes in both normal basal ganglia function and in the pathophysiology of a range of neuropsychiatric conditions, such as TS and autism spectrum disorders.
Data availability statement
The raw data supporting the conclusions of this article will be made available by the authors, without undue reservation.
Ethics statement
The animal study was approved by Yale’s Institutional Animal Care and Use Committee. The study was conducted in accordance with the local legislation and institutional requirements.
Author contributions
MVZ: Conceptualization, Formal analysis, Funding acquisition, Investigation, Project administration, Supervision, Visualization, Writing – original draft. DF: Investigation, Writing – review & editing. CP: Conceptualization, Funding acquisition, Project administration, Supervision, Writing – review & editing, Resources.
Funding
The author(s) declare that financial support was received for the research, authorship, and/or publication of this article. This work was supported by the grants F32MH123088 (MVZ), K24MH121571 (CP), and R01MH127259 (CP). This work was also supported by the State of Connecticut through its support of the Abraham Ribicoff Research Facilities at the Connecticut Mental Health Center; the reported work represents the views of the authors and not of the State of Connecticut.
Acknowledgments
The authors thank Betsy D’Amico and the staff of the Yale Animal Resource Core and Charles River Laboratories for animal husbandry.
Conflict of interest
CP has consulted in the past year for Biohaven Pharmaceuticals, Freedom Biosciences, Ceruvia Life Sciences, Nobilis Therapeutics, Transcend Therapeutics, and F-Prime Capital Partners and has received research support from Biohaven, Freedom, and Transcend; none of these relationships are related to the current work. CP holds equity in Alco Therapeutics and Biohaven Pharmaceuticals and has pending patents related to the treatment of OCD, the actions of psychedelic drugs, and the role of specific antibodies in neuroimmune pathophysiology; again, none of these relationships are related to the current work.
The remaining authors declare that the research was conducted in the absence of any commercial or financial relationships that could be construed as a potential conflict of interest.
Publisher’s note
All claims expressed in this article are solely those of the authors and do not necessarily represent those of their affiliated organizations, or those of the publisher, the editors and the reviewers. Any product that may be evaluated in this article, or claim that may be made by its manufacturer, is not guaranteed or endorsed by the publisher.
Supplementary material
The Supplementary material for this article can be found online at: https://www.frontiersin.org/articles/10.3389/fncel.2024.1415015/full#supplementary-material
References
Adler, A., Katabi, S., Finkes, I., Prut, Y., and Bergman, H. (2013). Different correlation patterns of cholinergic and GABAergic interneurons with striatal projection neurons. Front. Syst. Neurosci. 7:47. doi: 10.3389/fnsys.2013.00047
Almey, A., Filardo, E. J., Milner, T. A., and Brake, W. G. (2012). Estrogen receptors are found in glia and at extranuclear neuronal sites in the dorsal striatum of female rats: evidence for cholinergic but not dopaminergic colocalization. Endocrinology 153, 5373–5383. doi: 10.1210/en.2012-1458
Almey, A., Milner, T. A., and Brake, W. G. (2016). Estrogen receptor α and G-protein coupled estrogen receptor 1 are localized to GABAergic neurons in the dorsal striatum. Neurosci. Lett. 622, 118–123. doi: 10.1016/j.neulet.2016.04.023
Aziz, H. C., and Mangieri, R. A. (2023). Sexually dimorphic characteristics of dopamine D1 receptor-expressing neurons within the shell of the nucleus accumbens of adolescent mice. Res. Sq. :rs.3.rs-3717874. doi: 10.21203/rs.3.rs-3717874/v1
Bamford, I. J., and Bamford, N. S. (2019). The Striatum’s role in executing rational and irrational economic behaviors. Neuroscientist 25, 475–490. doi: 10.1177/1073858418824256
Becker, J. B., and Beer, M. E. (1986). The influence of estrogen on nigrostriatal dopamine activity: behavioral and neurochemical evidence for both pre- and postsynaptic components. Behav. Brain Res. 19, 27–33. doi: 10.1016/0166-4328(86)90044-6
Becker, J. B., and Cha, J. H. (1989). Estrous cycle-dependent variation in amphetamine-induced behaviors and striatal dopamine release assessed with microdialysis. Behav. Brain Res. 35, 117–125. doi: 10.1016/S0166-4328(89)80112-3
Bennett, B. D., and Bolam, J. P. (1994). Synaptic input and output of parvalbumin-immunoreactive neurons in the neostriatum of the rat. Neuroscience 62, 707–719. doi: 10.1016/0306-4522(94)90471-5
Blurton-Jones, M., and Tuszynski, M. H. (2002). Estrogen receptor-beta colocalizes extensively with parvalbumin-labeled inhibitory neurons in the cortex, amygdala, basal forebrain, and hippocampal formation of intact and ovariectomized adult rats. J. Comp. Neurol. 452, 276–287. doi: 10.1002/cne.10393
Brady, M. V., Mariani, J., Koca, Y., Szekely, A., King, R. A., Bloch, M. H., et al. (2022). Mispatterning and interneuron deficit in Tourette Syndrome basal ganglia organoids. Mol. Psychiatry 27, 5007–5019. doi: 10.1038/s41380-022-01880-5
Cachope, R., Mateo, Y., Mathur, B. N., Irving, J., Wang, H. L., Morales, M., et al. (2012). Selective activation of cholinergic interneurons enhances accumbal phasic dopamine release: setting the tone for reward processing. Cell Rep. 2, 33–41. doi: 10.1016/j.celrep.2012.05.011
Cadeddu, R., Van Zandt, M., Santovito, L. S., Odeh, K., Anderson, C. J., Flanagan, D., et al. (2023). Prefrontal allopregnanolone mediates the adverse effects of acute stress in a mouse model of tic pathophysiology. Neuropsychoph. 48, 288–1299. doi: 10.1038/s41386-023-01603-6
Calipari, E. S., Juarez, B., Morel, C., Walker, D. M., Cahill, M. E., Ribeiro, E., et al. (2017). Dopaminergic dynamics underlying sex-specific cocaine reward. Nat. Commun. 8:13877. doi: 10.1038/ncomms13877
Cao, J., Willett, J. A., Dorris, D. M., and Meitzen, J. (2018). Sex differences in medium spiny neuron excitability and glutamatergic synaptic input: heterogeneity across striatal regions and evidence for estradiol-dependent sexual differentiation. Front. Endocrinol. 9:173. doi: 10.3389/fendo.2018.00173
Castner, S. A., Xiao, L., and Becker, J. B. (1993). Sex differences in striatal dopamine: in vivo microdialysis and behavioral studies. Brain Res. 610, 127–134. doi: 10.1016/0006-8993(93)91225-H
Cattaneo, S., Zaghi, M., Maddalena, R., Bedogni, F., Sessa, A., and Taverna, S. (2019). Somatostatin-expressing interneurons co-release GABA and glutamate onto different postsynaptic targets in the striatum. bioRxiv :566984. doi: 10.1101/566984
Chang, H. T., and Kita, H. (1992). Interneurons in the rat striatum: relationships between parvalbumin neurons and cholinergic neurons. Brain Res. 574, 307–311. doi: 10.1016/0006-8993(92)90830-3
Chantranupong, L., Beron, C. C., Zimmer, J. A., Wen, M. J., Wang, W., and Sabatini, B. L. (2023). Dopamine and glutamate regulate striatal acetylcholine in decision-making. Nature 621, 577–585. doi: 10.1038/s41586-023-06492-9
Chen, J., Tian, C., Zhang, Q., Xiang, H., Wang, R., Hu, X., et al. (2022). Changes in volume of subregions within basal ganglia in obsessive–compulsive disorder: a study with atlas-based and VBM methods. Front. Neurosci. 16:890616. doi: 10.3389/fnins.2022.890616
DeBoer, P., and Westerink, B. H. C. (1994). GABAergic modulation of striatal cholinergic interneurons: an in vivo microdialysis study. J. Neurochem. 62, 70–75. doi: 10.1046/j.1471-4159.1994.62010070.x
Dluzen, D. E., and McDermott, J. L. (2008). Sex differences in dopamine- and vesicular monoamine-transporter functions. Ann. N. Y. Acad. Sci. 1139, 140–150. doi: 10.1196/annals.1432.010
Drzewiecki, C. M., Sellinger, E. P., and Juraska, J. M. (2021). Impact of pubertal onset on region-specific Esr2 expression. J. Neuroendocrinol. 33:e13029. doi: 10.1111/jne.13029
Drzewiecki, C. M., Willing, J., and Juraska, J. M. (2016). Synaptic number changes in the medial prefrontal cortex across adolescence in male and female rats: a role for pubertal onset. Synapse 70, 361–368. doi: 10.1002/syn.21909
Duhne, M., Lara-González, E., Laville, A., Padilla-Orozco, M., Ávila-Cascajares, F., Arias-García, M., et al. (2021). Activation of parvalbumin-expressing neurons reconfigures neuronal ensembles in murine striatal microcircuits. Eur. J. Neurosci. 53, 2149–2164. doi: 10.1111/ejn.14670
Faust, T. W., Assous, M., Tepper, J. M., and Koós, T. (2016). Neostriatal GABAergic interneurons mediate cholinergic inhibition of spiny projection neurons. J. Neurosci. 36, 9505–9511. doi: 10.1523/JNEUROSCI.0466-16.2016
Forlano, P. M., and Woolley, C. S. (2010). Quantitative analysis of pre- and postsynaptic sex differences in the nucleus accumbens. J. Comp. Neurol. 518, 1330–1348. doi: 10.1002/cne.22279
Franklin, K. B. J., and Paxinos, G. (1997). The mouse brain in stereotaxic coordinates. Translated from Eng by. San Diego: Academic Press.
Gazan, A., Rial, D., and Schiffmann, S. N. (2020). Ablation of striatal somatostatin interneurons affects MSN morphology and electrophysiological properties, and increases cocaine-induced hyperlocomotion in mice. Eur. J. Neurosci. 51, 1388–1402. doi: 10.1111/ejn.14581
Gerfen, C. R., and Wilson, C. J. (1996). “Chapter II the basal ganglia” in Handbook of chemical neuroanatomy eds. L. W. Swanson, A. BjÖrklund, and T. HÖkfelt (Elsevier), 12, 371–468.
Giedd, J. N., Castellanos, F. X., Rajapakse, J. C., Vaituzis, A. C., and Rapoport, J. L. (1997). Sexual dimorphism of the developing human brain. Prog. Neuro-Psychopharmacol. Biol. Psychiatry 21, 1185–1201. doi: 10.1016/S0278-5846(97)00158-9
Gittis, A. H., Leventhal, D. K., Fensterheim, B. A., Pettibone, J. R., Berke, J. D., and Kreitzer, A. C. (2011). Selective inhibition of striatal fast-spiking interneurons causes dyskinesias. J. Neurosci. 31, 15727–15731. doi: 10.1523/JNEUROSCI.3875-11.2011
Gittis, A. H., Nelson, A. B., Thwin, M. T., Palop, J. J., and Kreitzer, A. C. (2010). Distinct roles of GABAergic interneurons in the regulation of striatal output pathways. J. Neurosci. 30, 2223–2234. doi: 10.1523/JNEUROSCI.4870-09.2010
Graybiel, A. M., Aosaki, T., Flaherty, A. W., and Kimura, M. (1994). The basal ganglia and adaptive motor control. Science 265, 1826–1831. doi: 10.1126/science.8091209
Graybiel, A. M., and Grafton, S. T. (2015). The striatum: where skills and habits meet. Cold Spring Harb. Perspect. Biol. 7:a021691. doi: 10.1101/cshperspect.a021691
Gritton, H. J., Howe, W. M., Romano, M. F., DiFeliceantonio, A. G., Kramer, M. A., Saligrama, V., et al. (2019). Unique contributions of parvalbumin and cholinergic interneurons in organizing striatal networks during movement. Nat. Neurosci. 22, 586–597. doi: 10.1038/s41593-019-0341-3
Hathway, G. J., Humphrey, P. P., and Kendrick, K. M. (1999). Evidence that somatostatin sst2 receptors mediate striatal dopamine release. Br. J. Pharmacol. 128, 1346–1352. doi: 10.1038/sj.bjp.0702934
Howe, M., Ridouh, I., Allegra Mascaro, A. L., Larios, A., Azcorra, M., and Dombeck, D. A. (2019). Coordination of rapid cholinergic and dopaminergic signaling in striatum during spontaneous movement. eLife 8:e44903. doi: 10.7554/eLife.44903
Hu, M., Watson, C. J., Kennedy, R. T., and Becker, J. B. (2006). Estradiol attenuates the K+-induced increase in extracellular GABA in rat striatum. Synapse 59, 122–124. doi: 10.1002/syn.20221
Ian Antón, O., and Jun, B. D. (2011). Cholinergic modulation of synaptic integration and dendritic excitability in the striatum. Curr. Opin. Neurobiol. 21, 425–432. doi: 10.1016/j.conb.2011.04.004
Kalanithi, P. S., Zheng, W., Kataoka, Y., DiFiglia, M., Grantz, H., Saper, C. B., et al. (2005). Altered parvalbumin-positive neuron distribution in basal ganglia of individuals with Tourette syndrome. Proc. Natl. Acad. Sci. U. S. A. 102, 13307–13312. doi: 10.1073/pnas.0502624102
Kataoka, Y., Kalanithi, P. S., Grantz, H., Schwartz, M. L., Saper, C., Leckman, J. F., et al. (2010). Decreased number of parvalbumin and cholinergic interneurons in the striatum of individuals with Tourette syndrome. J. Comp. Neurol. 518, 277–291. doi: 10.1002/cne.22206
Kawaguchi, Y. (1997). Neostriatal cell subtypes and their functional roles. Neurosci. Res. 27, 1–8. doi: 10.1016/S0168-0102(96)01134-0
Kawaguchi, Y., Wilson, C. J., Augood, S. J., and Emson, P. C. (1995). Striatal interneurones: chemical, physiological and morphological characterization. Trends Neurosci. 18, 527–535. doi: 10.1016/0166-2236(95)98374-8
Kocaturk, S., Guven, E. B., Shah, F., Tepper, J. M., and Assous, M. (2022). Cholinergic control of striatal GABAergic microcircuits. Cell Rep. 41:111531. doi: 10.1016/j.celrep.2022.111531
Koós, T., and Tepper, J. M. (1999). Inhibitory control of neostriatal projection neurons by GABAergic interneurons. Nat. Neurosci. 2, 467–472. doi: 10.1038/8138
Koss, W. A., Belden, C. E., Hristov, A. D., and Juraska, J. M. (2014). Dendritic remodeling in the adolescent medial prefrontal cortex and the basolateral amygdala of male and female rats. Synapse 68, 61–72. doi: 10.1002/syn.21716
Koss, W. A., Lloyd, M. M., Sadowski, R. N., Wise, L. M., and Juraska, J. M. (2015). Gonadectomy before puberty increases the number of neurons and glia in the medial prefrontal cortex of female, but not male, rats. Dev. Psychobiol. 57, 305–312. doi: 10.1002/dev.21290
Kövesdi, E., Udvarácz, I., Kecskés, A., Szőcs, S., Farkas, S., Faludi, P., et al. (2022). 17β-estradiol does not have a direct effect on the function of striatal cholinergic interneurons in adult mice in vitro. Front. Endocrinol. 13:993552. doi: 10.3389/fendo.2022.993552
Kreitzer, A. C. (2009). Physiology and pharmacology of striatal neurons. Annu. Rev. Neurosci. 32, 127–147. doi: 10.1146/annurev.neuro.051508.135422
Krentzel, A. A., Willett, J. A., Johnson, A. G., and Meitzen, J. (2021). Estrogen receptor alpha, G-protein coupled estrogen receptor 1, and aromatase: developmental, sex, and region-specific differences across the rat caudate-putamen, nucleus accumbens core and shell. J. Comp. Neurol. 529, 786–801. doi: 10.1002/cne.24978
Lee, K., Holley, S. M., Shobe, J. L., Chong, N. C., Cepeda, C., Levine, M. S., et al. (2017). Parvalbumin interneurons modulate striatal output and enhance performance during associative learning. Neuron 93, 1451–1463.e4. doi: 10.1016/j.neuron.2017.02.033
Lennington, J. B., Coppola, G., Kataoka-Sasaki, Y., Fernandez, T. V., Palejev, D., Li, Y., et al. (2016). Transcriptome analysis of the human striatum in Tourette syndrome. Biol. Psychiatry 79, 372–382. doi: 10.1016/j.biopsych.2014.07.018
Lim, S. A. O., Kang, U. J., and McGehee, D. S. (2014). Striatal cholinergic interneuron regulation and circuit effects. Front. Synaptic Neurosci. 6:22. doi: 10.3389/fnsyn.2014.00022
Loomes, R., Hull, L., and Mandy, W. P. L. (2017). What is the male-to-female ratio in autism spectrum disorder? A systematic review and meta-analysis. J. Am. Acad. Child Adolesc. Psychiatry 56, 466–474. doi: 10.1016/j.jaac.2017.03.013
Macpherson, T., and Hikida, T. (2019). Role of basal ganglia neurocircuitry in the pathology of psychiatric disorders. Psychiatry Clin. Neurosci. 73, 289–301. doi: 10.1111/pcn.12830
Marin, O., Anderson, S. A., and Rubenstein, J. L. (2000). Origin and molecular specification of striatal interneurons. J. Neurosci. 20, 6063–6076. doi: 10.1523/JNEUROSCI.20-16-06063.2000
Markham, J. A., Morris, J. R., and Juraska, J. M. (2007). Neuron number decreases in the rat ventral, but not dorsal, medial prefrontal cortex between adolescence and adulthood. Neuroscience 144, 961–968. doi: 10.1016/j.neuroscience.2006.10.015
Martino, D., Macerollo, A., and Leckman, J. F. (2013). Neuroendocrine aspects of Tourette syndrome. Int. Rev. Neurobiol. 112, 239–279. doi: 10.1016/B978-0-12-411546-0.00009-3
Matamales, M., Götz, J., and Bertran-Gonzalez, J. (2016). Quantitative imaging of cholinergic interneurons reveals a distinctive spatial organization and a functional gradient across the mouse striatum. PLoS One 11:e0157682. doi: 10.1371/journal.pone.0157682
Mermelstein, P. G., Becker, J. B., and Surmeier, D. J. (1996). Estradiol reduces calcium currents in rat neostriatal neurons via a membrane receptor. J. Neurosci. 16, 595–604. doi: 10.1523/JNEUROSCI.16-02-00595.1996
Momiyama, T., and Zaborszky, L. (2006). Somatostatin presynaptically inhibits both GABA and glutamate release onto rat basal forebrain cholinergic neurons. J. Neurophysiol. 96, 686–694. doi: 10.1152/jn.00507.2005
Muñoz-Manchado, A. B., Gonzales, C. B., Zeisel, A., Munguba, H., Bekkouche, B., Skene, N. G., et al. (2018). Diversity of interneurons in the dorsal striatum revealed by single-cell RNA sequencing and PatchSeq. Cell Rep. 24, 2179–2190.e7. doi: 10.1016/j.celrep.2018.07.053
Nelson, A. B., Hammack, N., Yang, C. F., Shah, N. M., Seal, R. P., and Kreitzer, A. C. (2014). Striatal cholinergic interneurons drive GABA release from dopamine terminals. Neuron 82, 63–70. doi: 10.1016/j.neuron.2014.01.023
Nuñez, J. L., Sodhi, J., and Juraska, J. M. (2002). Ovarian hormones after postnatal day 20 reduce neuron number in the rat primary visual cortex. J. Neurobiol. 52, 312–321. doi: 10.1002/neu.10092
Orduz, D., Bischop, D. P., Schwaller, B., Schiffmann, S. N., and Gall, D. (2013). Parvalbumin tunes spike-timing and efferent short-term plasticity in striatal fast spiking interneurons. J. Physiol. 591, 3215–3232. doi: 10.1113/jphysiol.2012.250795
Pakhotin, P., and Bracci, E. (2007). Cholinergic interneurons control the excitatory input to the striatum. J. Neurosci. 27, 391–400. doi: 10.1523/JNEUROSCI.3709-06.2007
Pennartz, C. M. A., Berke, J. D., Graybiel, A. M., Ito, R., Lansink, C. S., van der Meer, M., et al. (2009). Corticostriatal interactions during learning, memory processing, and decision making. J. Neurosci. 29, 12831–12838. doi: 10.1523/JNEUROSCI.3177-09.2009
Peterson, B. M., Mermelstein, P. G., and Meisel, R. L. (2015). Estradiol mediates dendritic spine plasticity in the nucleus accumbens core through activation of mGluR5. Brain Struct. Funct. 220, 2415–2422. doi: 10.1007/s00429-014-0794-9
Phelps, P. E., Brady, D. R., and Vaughn, J. E. (1989). The generation and differentiation of cholinergic neurons in rat caudate—putamen. Dev. Brain Res. 46, 47–60. doi: 10.1016/0165-3806(89)90142-9
Poppi, L. A., Ho-Nguyen, K. T., Shi, A., Daut, C. T., and Tischfield, M. A. (2021). Recurrent implication of striatal cholinergic interneurons in a range of neurodevelopmental, neurodegenerative, and neuropsychiatric disorders. Cells 10:907. doi: 10.3390/cells10040907
Proaño, S. B., Morris, H. J., Kunz, L. M., Dorris, D. M., and Meitzen, J. (2018). Estrous cycle-induced sex differences in medium spiny neuron excitatory synaptic transmission and intrinsic excitability in adult rat nucleus accumbens core. J. Neurophysiol. 120, 1356–1373. doi: 10.1152/jn.00263.2018
Rapanelli, M., Frick, L. R., and Pittenger, C. (2017a). The role of interneurons in autism and Tourette syndrome. Trends Neurosci. 40, 397–407. doi: 10.1016/j.tins.2017.05.004
Rapanelli, M., Frick, L. R., Xu, M., Groman, S. M., Jindachomthong, K., Tamamaki, N., et al. (2017b). Targeted interneuron depletion in the dorsal striatum produces autism-like behavioral abnormalities in male but not female mice. Biol. Psychiatry 82, 194–203. doi: 10.1016/j.biopsych.2017.01.020
Rijpkema, M., Everaerd, D., van der Pol, C., Franke, B., Tendolkar, I., and Fernández, G. (2012). Normal sexual dimorphism in the human basal ganglia. Hum. Brain Mapp. 33, 1246–1252. doi: 10.1002/hbm.21283
Scahill, L., Specht, M., and Page, C. (2014). The prevalence of tic disorders and clinical characteristics in children. J. Obsessive Compuls. Relat. Disord. 3, 394–400. doi: 10.1016/j.jocrd.2014.06.002
Schindelin, J., Arganda-Carreras, I., Frise, E., Kaynig, V., Longair, M., Pietzsch, T., et al. (2012). Fiji: an open-source platform for biological-image analysis. Nat. Methods 9, 676–682. doi: 10.1038/nmeth.2019
Schultz, K. N., von Esenwein, S. A., Hu, M., Bennett, A. L., Kennedy, R. T., Musatov, S., et al. (2009). Viral vector-mediated overexpression of estrogen receptor-alpha in striatum enhances the estradiol-induced motor activity in female rats and estradiol-modulated GABA release. J. Neurosci. 29, 1897–1903. doi: 10.1523/JNEUROSCI.4647-08.2009
Sotonyi, P., Gao, Q., Bechmann, I., and Horvath, T. L. (2010). Estrogen promotes parvalbumin expression in arcuate nucleus POMC neurons. Reprod. Sci. 17, 1077–1080. doi: 10.1177/1933719110379651
Staffend, N. A., Loftus, C. M., and Meisel, R. L. (2011). Estradiol reduces dendritic spine density in the ventral striatum of female Syrian hamsters. Brain Struct. Funct. 215, 187–194. doi: 10.1007/s00429-010-0284-7
Suzuki, S., Lawlor, V. M., Cooper, J. A., Arulpragasam, A. R., and Treadway, M. T. (2021). Distinct regions of the striatum underlying effort, movement initiation and effort discounting. Nat. Hum. Behav. 5, 378–388. doi: 10.1038/s41562-020-00972-y
Swerdlow, N. R., and Koob, G. F. (1987). Dopamine, schizophrenia, mania, and depression: toward a unified hypothesis of cortico-striatopallido-thalamic function. Behav. Brain Sci. 10, 197–208. doi: 10.1017/S0140525X00047488
Threlfell, S., Lalic, T., Platt, N. J., Jennings, K. A., Deisseroth, K., and Cragg, S. J. (2012). Striatal dopamine release is triggered by synchronized activity in cholinergic interneurons. Neuron 75, 58–64. doi: 10.1016/j.neuron.2012.04.038
Tozzi, A., de Iure, A., Tantucci, M., Durante, V., Quiroga-Varela, A., Giampà, C., et al. (2015). Endogenous 17β-estradiol is required for activity-dependent long-term potentiation in the striatum: interaction with the dopaminergic system. Front. Cell. Neurosci. 9:192. doi: 10.3389/fncel.2015.00192
Walker, Q. D., Rooney, M. B., Wightman, R. M., and Kuhn, C. M. (2000). Dopamine release and uptake are greater in female than male rat striatum as measured by fast cyclic voltammetry. Neuroscience 95, 1061–1070. doi: 10.1016/s0306-4522(99)00500-x
Willing, J., and Juraska, J. M. (2015). The timing of neuronal loss across adolescence in the medial prefrontal cortex of male and female rats. Neuroscience 301, 268–275. doi: 10.1016/j.neuroscience.2015.05.073
Wu, Y. C., Du, X., van den Buuse, M., and Hill, R. A. (2014). Sex differences in the adolescent developmental trajectory of parvalbumin interneurons in the hippocampus: a role for estradiol. Psychoneuroendocrinology 45, 167–178. doi: 10.1016/j.psyneuen.2014.03.016
Wu, Y., and Parent, A. (2000). Striatal interneurons expressing calretinin, parvalbumin or NADPH-diaphorase: a comparative study in the rat, monkey and human. Brain Res. 863, 182–191. doi: 10.1016/S0006-8993(00)02135-1
Xiao, L., and Becker, J. B. (1994). Quantitative microdialysis determination of extracellular striatal dopamine concentration in male and female rats: effects of estrous cycle and gonadectomy. Neurosci. Lett. 180, 155–158. doi: 10.1016/0304-3940(94)90510-X
Xu, M., Kobets, A., Du, J. C., Lennington, J., Li, L., Banasr, M., et al. (2015). Targeted ablation of cholinergic interneurons in the dorsolateral striatum produces behavioral manifestations of Tourette syndrome. Proc. Natl. Acad. Sci. U S A. 112, 893–8. doi: 10.1073/pnas.1419533112
Xu, M., Li, L., and Pittenger, C. (2016). Ablation of fast-spiking interneurons in the dorsal striatum, recapitulating abnormalities seen post-mortem in Tourette syndrome, produces anxiety and elevated grooming. Neuroscience. 324, 321–9. doi: 10.1016/j.neuroscience.2016.02.074
Yoest, K. E., Quigley, J. A., and Becker, J. B. (2018). Rapid effects of ovarian hormones in dorsal striatum and nucleus accumbens. Horm. Behav. 104, 119–129. doi: 10.1016/j.yhbeh.2018.04.002
Yorgason, J. T., Zeppenfeld, D. M., and Williams, J. T. (2017). Cholinergic interneurons underlie spontaneous dopamine release in nucleus accumbens. J. Neurosci. 37, 2086–2096. doi: 10.1523/JNEUROSCI.3064-16.2017
Zachry, J. E., Nolan, S. O., Brady, L. J., Kelly, S. J., Siciliano, C. A., and Calipari, E. S. (2021). Sex differences in dopamine release regulation in the striatum. Neuropsychopharmacology 46, 491–499. doi: 10.1038/s41386-020-00915-1
Keywords: sex differences, interneurons, striatum, parvalbumin, GABAergic, cholinergic, basal ganglia
Citation: Van Zandt M, Flanagan D and Pittenger C (2024) Sex differences in the distribution and density of regulatory interneurons in the striatum. Front. Cell. Neurosci. 18:1415015. doi: 10.3389/fncel.2024.1415015
Edited by:
Maurizio Giustetto, University of Turin, ItalyReviewed by:
Veronica Ghiglieri, Università telematica San Raffaele, ItalyGiuseppe Sciamanna, Saint Camillus International University of Health and Medical Sciences, Italy
Copyright © 2024 Van Zandt, Flanagan and Pittenger. This is an open-access article distributed under the terms of the Creative Commons Attribution License (CC BY). The use, distribution or reproduction in other forums is permitted, provided the original author(s) and the copyright owner(s) are credited and that the original publication in this journal is cited, in accordance with accepted academic practice. No use, distribution or reproduction is permitted which does not comply with these terms.
*Correspondence: Meghan Van Zandt, bWVnaGFuLnZhbnphbmR0QHlhbGUuZWR1; Christopher Pittenger, Y2hyaXN0b3BoZXIucGl0dGVuZ2VyQHlhbGUuZWR1