- 1Department of Philosophy, Social Sciences and Education, University of Perugia, Perugia, Italy
- 2Laboratory of Experimental and Behavioral Neurophysiology, IRCCS Santa Lucia Foundation, Rome, Italy
- 3Department of Psychology, University Sapienza of Rome, Rome, Italy
Schizophrenia is a complex and severe mental disorder that affects approximately 1% of the global population. It is characterized by a wide range of symptoms, including delusions, hallucinations, disorganized speech and behavior, and cognitive impairment. Recent research has suggested that the immune system dysregulation may play a significant role in the pathogenesis of schizophrenia, and glial cells, such as astroglia and microglia known to be involved in neuroinflammation and immune regulation, have emerged as potential players in this process. The aim of this systematic review is to summarize the glial hallmarks of schizophrenia, choosing as cellular candidate the astroglia and microglia, and focusing also on disease-associated psychological (cognitive and emotional) changes. We conducted a systematic review following the Preferred Reporting Items for Systematic Reviews and Meta-Analyses guidelines. We searched PubMed, Scopus, and Web of Science for articles that investigated the differences in astroglia and microglia in patients with schizophrenia, published in the last 5 years. The present systematic review indicates that changes in the density, morphology, and functioning of astroglia and microglia may be involved in the development of schizophrenia. The glial alterations may contribute to the pathogenesis of schizophrenia by dysregulating neurotransmission and immune responses, worsening cognitive capabilities. The complex interplay of astroglial and microglial activation, genetic/epigenetic variations, and cognitive assessments underscores the intricate relationship between biological mechanisms, symptomatology, and cognitive functioning in schizophrenia.
1 Introduction
Schizophrenia is a complex, multi-dimensional, and enduring psychiatric disorder characterized by a broad spectrum of clinical manifestations that include positive (hallucinations, delusions, disorganized speech, and thought disturbances), negative (reduction or absence of typical emotional, motivational, or interest-related behaviors), and cognitive (impairment in working memory, verbal memory, attention, and executive functions) symptoms (Owen et al., 2016; Correll and Schooler, 2020). Typically emerging during late adolescence to early adulthood, schizophrenia exhibits gender-specific onset patterns, with males often experiencing an earlier onset (late adolescence to early twenties) compared to females (early twenties to early thirties) (Hollis et al., 2008; McGrath et al., 2008). Despite subtle changes in cognition and social relationships preceding formal diagnosis by several years, the diagnosis is often delayed, and patients already display significant brain changes in terms of structure, neurochemistry, and connectivity (Millan et al., 2016; Rodrigues-Neves et al., 2022). The clinical presentation, trajectory, and prognosis of schizophrenia are highly heterogeneous, marked by recurrent and challenging-to-predict relapses, often leading to a chronic course of illness (Owen et al., 2016). The heterogeneity of symptoms and their late recognition make outlining a pathophysiological profile challenging. Despite extensive research, specific structural and functional dysregulations, prodromal and unique to schizophrenia, have not been conclusively identified. Patients with schizophrenia exhibit reductions in the volume of the caudate nucleus, thalamus, and prefrontal cortex (PFC) (Lewis, 2012; Haijma et al., 2013; Meyer-Lindenberg and Tost, 2014). Dysregulation of dopaminergic neurotransmission is a key feature, with dopamine excess in subcortical limbic area linked to positive symptoms (Howes and Kapur, 2009; Walter et al., 2009), and dopamine deficiency in the PFC associated with negative and cognitive symptoms (Fusar-Poli et al., 2011). Cognitive deficits also correlate with impaired glutamatergic neurotransmission (Kantrowitz and Javitt, 2010; Barch and Ceaser, 2012).
Interestingly, a comprehensive genome-wide association study in schizophrenia has emphasized common variations in genes encoding for the glutamate receptors, voltage-dependent calcium channel proteins, and dopamine receptors D2 (DRD2), supporting the role of glutamatergic and dopaminergic signaling disruption in schizophrenia pathogenesis (Schizophrenia Working Group of the Psychiatric Genomics Consortium, 2014). Moreover, the same working group found associations between schizophrenia and genes with important roles in immunity, in line with observational and clinical studies highlighting the connections between immune and inflammatory processes in psychiatric disorders (Benros et al., 2012; Smyth and Lawrie, 2013; Kroken et al., 2018; Hughes and Ashwood, 2020). More recently, research on psychiatric and neurological disorders has increasingly pointed to the neural-immune interface as potential source of disease markers, thereby enabling the identification of new neurobiological targets (Afridi et al., 2021; Chen et al., 2023).
The neuroimmune system is implied in a wide variety of brain functions, such as regulation of the neuroinflammatory status, modulation of neurotransmission and neuroplasticity, central nervous system (CNS) development, and response to injuries (Dantzer, 2018; O’Reilly and Tom, 2020). The neuroimmune system comprises glial cells like astrocytes, microglia, oligodendrocytes, and NG2 positive cells (Takahashi and Sakurai, 2013). Specifically, astrocytes encompass diverse cell populations, primarily categorized based on morphological characteristics and/or marker expressions (Zhang and Barres, 2010). Astrocytes provide metabolic support to neurons, regulate extracellular water and electrolyte balance, and synthesize an extensive array of extracellular matrix proteins and growth factors (De Keyser et al., 2008). Moreover, astrocytes have a pivotal role in synaptic formation, efficacy, and plasticity (Barker and Ullian, 2010; Kucukdereli et al., 2011; Suzuki et al., 2011), and release neurotransmitters, including D-serine, ATP, and glutamate (Hamilton and Attwell, 2010), exerting a direct influence on brain homeostasis. In brain pathologies, activated astrocytes have the capacity to modulate the immune responses producing proinflammatory cytokines [such as interleukin (IL)-6, IL-1β, tumor necrosis factor (TNF)-α, and interferon γ], reactive oxygen species (ROS), and matrix metalloproteases, contributing thus to the neuroinflammatory response (De Keyser et al., 2008).
Another essential type of neuroimmune heterogeneous cells is the microglia, categorized in subpopulations according to the expression of particular gene clusters. It presents distinct transcriptomic profiles with brain region specificity that change over time to functionally respond to particular demands of different developmental phases (Matcovitch-Natan et al., 2016; Thion et al., 2018). Acting as phagocytes, microglial cells also produce proinflammatory cytokines along with ROS (Woodburn et al., 2021). Microglia plays a key role in the synaptic pruning, contributes to the proper establishment and maturation of neural circuits, and influences growth and survival of dopaminergic neurons in the context of inflammation (Saijo and Glass, 2011; Coomey et al., 2020).
The regulation of astrocyte and microglia activity is crucial for physiological brain functioning and homeostasis, and any disruption in these processes may have detrimental impact on mental health. Astrocytes and microglia are able to eliminate or strengthen cells and synapses, functions of high relevance in the onset of schizophrenia and its clinical progression. This systematic review focused on recent findings (from the past 5 years) exploring the involvement of astrocytes and microglia evaluated by using neurobiological, morphological, and/or genetic and epigenetic analyses in patients with schizophrenia, aiming to better clarify (i) astroglial and microglial cellular targets in the disease, and (ii) their relationship with psychological (cognitive and emotional) correlates.
2 Materials and methods
2.1 Protocol
This systematic review was created using the Preferred Reporting Items for Systematic Reviews and Meta-Analyses (PRISMA) guidelines (Page et al., 2021). In Figure 1, the PRISMA flowchart of the study selection was reported.
2.2 Search strategy and study selection
A systematic literature search was performed on three databases: PubMed, Scopus, and Web of Science to identify articles published in the 5 years prior to the research date (28 April 2023), focused on the following areas of interest: “schizophrenia” and “astrocytes and microglia alterations.” After keywords selection, the search was performed within “Title and abstract” in PubMed and Scopus, and within “Topic” in Web of Science. In the following, advanced searches for each database are reported:
– PubMed advanced search: (((”schizophrenia”) AND ((”microglia” OR “Iba1” OR “microglial cell*”) OR (”astroglial cell*” OR “astroglia” OR “GFAP” OR “astrocytes”))) NOT “review”).
– Scopus advanced search: (((”schizophrenia”) AND ((”microglia” OR “Iba1” OR “microglial cell*”) OR (”astroglial cell*” OR “astroglia” OR “GFAP” OR “astrocytes”))) AND NOT “review”).
– Web of Science advanced search: (((”schizophrenia”) AND ((”microglia” OR “Iba1” OR “microglial cell*”) OR (”astroglial cell*” OR “astroglia” OR “GFAP” OR “astrocytes”))) NOT “review”).
The screening was independently performed by three different authors.
2.3 Inclusion and exclusion criteria
The PICOS model was used to determine the inclusion criteria:
– P (population): “individuals diagnosed with schizophrenia”
– I (intervention): not applicable
– C (comparators): “individuals without a psychiatric diagnosis (i.e., control and placebo groups)”
– O (outcome): “astrocytes and microglia evaluations”
– S (study design): “observational studies, cohort studies, clinical trials, cross-sectional studies, and case-control studies.”
All human studies were included despite the study design. On the contrary, all studies including animal models or in vitro and in silico studies were excluded. In order to properly adhere to the research question, we excluded articles that did not involve patients with schizophrenia whose diagnosis was reported and involved a validated method. Additionally, we excluded articles that analyzed astrocyte and microglia alterations in patients with schizophrenia, but not considering at least one of the following methodologies:
– Neurobiological analyses
– Morphological analyses
– Genetic analyses
– Epigenetic analyses.
2.4 Data extraction
After defining inclusion and exclusion criteria and having completed the selection of studies, data were extracted and summarized in the Tables, reporting the following information:
– Description of all selected studies including in vivo/ex vivo, sample details, diagnosis, medical treatments, and exclusion criteria (Table 1)
– Methodologies (Table 2) and results (Table 3) of investigations on astrocytes including neurobiological and morphological analyses, genetic and epigenetic analyses, and psychological assessment
– Methodologies (Table 4) and results (Table 5) of investigations on microglia including neurobiological and morphological analyses, genetic and epigenetic analyses, and psychological assessment
– Methodologies (Table 6) and results (Table 7) of investigations on both astrocytes and microglia including neurobiological and morphological analyses, genetic and epigenetic analyses, and psychological assessment.
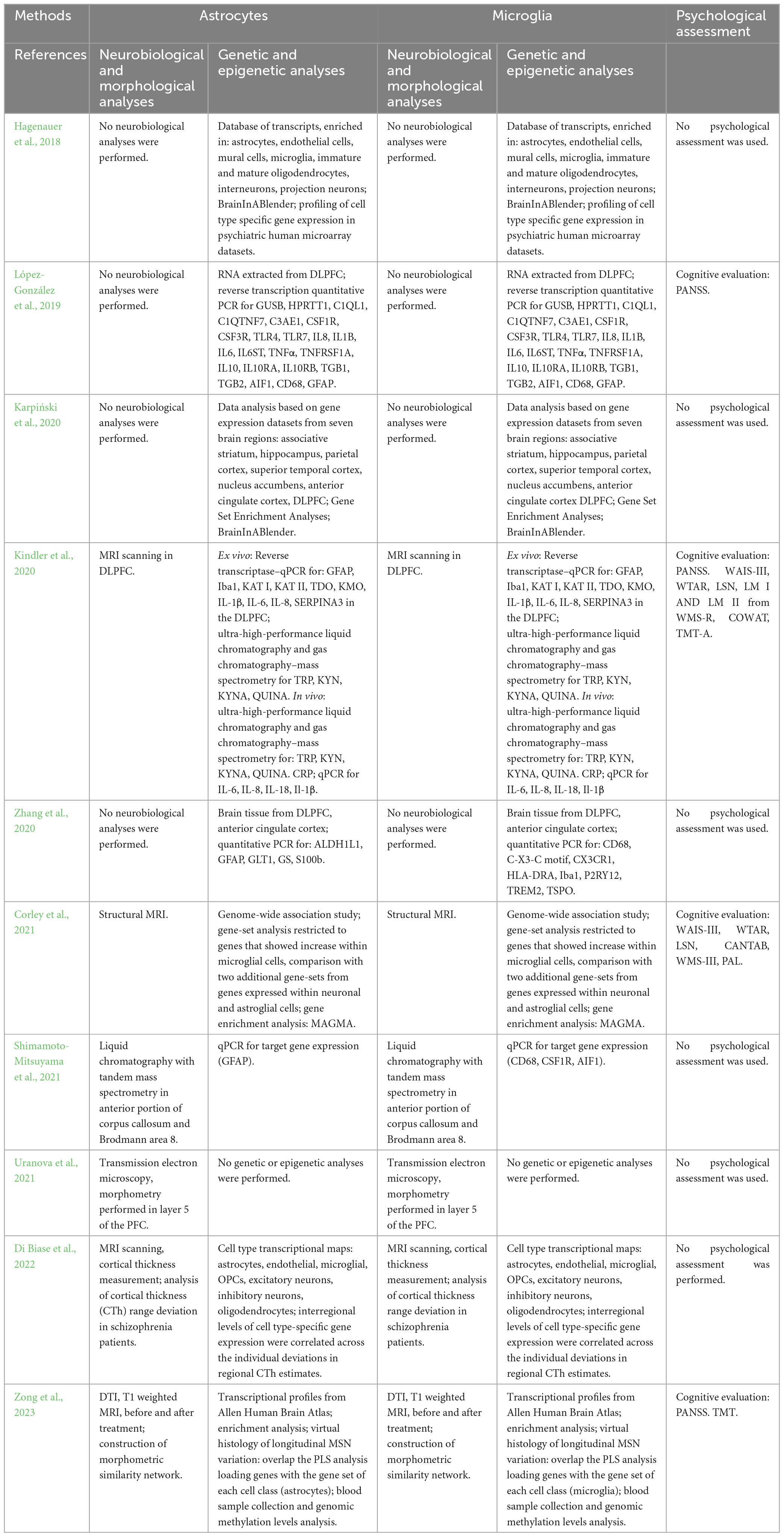
Table 6. Summary of the methodological approach of studies performing analyses on both astrocytes and microglia.
3 Results
3.1 Selected studies investigating astroglia and microglia in patients with a diagnosis of schizophrenia
The research in the three databases produced a total of 1,358 articles, divided, respectively, in 338 out of PubMed search, 561 out of Scopus, and 459 out of Web of Science. After excluding 595 duplicates, 763 papers were screened by reading title and abstract and out of these 678 publications were discarded and 85 were selected for the full-text screening. After the screening, 57 articles were discarded as they did not match the inclusion criteria. The reasons for exclusion, along with their corresponding numbers, are reported in Figure 1. A total of 28 articles were used to write the systematic review. Of these articles, 20 focused on astrocyte analysis (Hagenauer et al., 2018; O’Donovan et al., 2018; López-González et al., 2019; Amoah et al., 2020; Chan et al., 2020; Karpiński et al., 2020; Kindler et al., 2020; Rodrigues-Amorim et al., 2020; Zhang et al., 2020; Corley et al., 2021; Jeon et al., 2021; Ranganathan et al., 2021; Shimamoto-Mitsuyama et al., 2021; Uranova et al., 2021; Di Biase et al., 2022; Endres et al., 2022; Huang et al., 2022; Pinjari et al., 2022; Schmitt et al., 2022; Zong et al., 2023), and 18 focused on microglia analysis (Hagenauer et al., 2018; López-González et al., 2019; Adorjan et al., 2020; Karpiński et al., 2020; Kindler et al., 2020; Petrasch-Parwez et al., 2020; Uranova et al., 2020, 2021; Zhang et al., 2020; Corley et al., 2021; Hill et al., 2021; Jiang et al., 2021; Shimamoto-Mitsuyama et al., 2021; Di Biase et al., 2022; Vikhreva and Uranova, 2022; Jenkins et al., 2023; Yu et al., 2023; Zong et al., 2023). Importantly, on the total of the researches concerning astroglia and microglia measures, 10 evaluated both aspects. Finally, 7 studies also included psychological (cognitive or emotional) assessments. Figure 2 depicts the percentages of the divisions relative to the total number of articles.
3.2 Neurobiological and morphological analyses on astroglia
The methodologies and results concerning investigations on astroglia in patients with schizophrenia are summarized in Tables 2, 3.
3.2.1 Ex vivo
Based on the literature screening, only 1 article performed ex vivo neurobiological and morphological analyses on astrocytes (Schmitt et al., 2022). In this post-mortem investigation, a stereological approach was applied to analyze number and density of astrocytes, oligodendrocytes, and neurons in the hippocampal Cornu Ammonis (CA) 4, as well as of granular neurons in the dentate gyrus (DG) of left and right hemispheres in patients with schizophrenia and healthy controls. The results indicated a decrease in number and density of oligodendrocytes in the bilateral CA4 of patients. However, the number and density of astrocytes and neurons, as well as mean volume of CA4 and the DG, did not differ between patients and controls, even after adjustments for variables, such as post-mortem interval and age. The results suggest a potential deficit in oligodendrocyte maturation or a loss of mature oligodendrocytes, implicating an impaired myelination in schizophrenia.
3.2.2 In vivo
Regarding in vivo neurobiological and morphological analyses of astrocytes, the literature screening produced 6 studies (Rodrigues-Amorim et al., 2020; Jeon et al., 2021; Ranganathan et al., 2021; Endres et al., 2022; Huang et al., 2022; Pinjari et al., 2022), 3 of whom evaluated the astrocytes by performing analyses of the glial fibrillary acidic protein (GFAP) marker, a structural component of astrocytes cytoskeleton (Rodrigues-Amorim et al., 2020; Ranganathan et al., 2021; Huang et al., 2022). GFAP is the protein of intermediate filament in astrocytes and it is commonly used as a hallmark of reactive astrocytes, contributing to the neuroplasticity by dynamic processes, such as the morphology of dendrites, synapsis formation, and neuronal migration and differentiation (Moehle et al., 2012; Kapitein and Hoogenraad, 2015). Furthermore, in the case of reactive astrogliosis, the reactive astrocytes show altered expression of many genes and the GFAP gene upregulation that coincides with changes in the immune response and neural communication (Hol and Pekny, 2015). Rodrigues-Amorim et al. (2020) focused on three structural proteins, such as GFAP, β-III tubulin (protein of microtubule of dendrites and axons), and neurofilament light chain (Nf-L) (structural protein exclusive of neurons) that are associated with neurological impairment and clinical progression of neurodegenerative disorders, with the aim of establishing a pattern of neurodegeneration in patients with schizophrenia. Estimating plasma protein levels through quantitative immunoblotting and ELISA kit, the authors showed higher GFAP, β-III tubulin, and Nf-L levels in patients with schizophrenia compared to controls, especially in the subgroup of chronic clozapine-treated patients, suggesting that the three structural proteins may be potential biomarkers to indirectly measure the neuronal damage in schizophrenia. Similarly, Ranganathan et al. (2021) carried out nanoparticle tracking analysis in plasma of patients with schizophrenia and healthy controls, to determine the size and concentration of circulating exosomes. Exosomes are small secretory microvesicles that influence neuronal and glial function via their microRNA (miRNA) cargo, positioning them as a novel and effective method of cell-to-cell communication with important implications for brain disease. Through Western Blot analyses, the authors also examined CD9 exosomal membrane marker and GFAP level, showing a higher concentration of exosomal GFAP in the plasma from patients compared to healthy controls. Furthermore, Huang et al. (2022) measured the levels of GFAP and S100β—another astrocytic marker—as well as IL-6, nerve growth factor (NGF), brain derived neurotrophic factor (BDNF), and TNF-α in the peripheral blood of patients with schizophrenia and healthy controls. Contrary to previous studies, their results showed no significant difference in GFAP concentration between groups, whereas a higher concentration of S100β, IL-6, NGF, and TNF-α was detected in patients’ group compared to control group. The levels of astrocytic marker S100β, P-selectin (cell adhesion molecule involved in periphery-to-brain communication pathway), and IL-6 were also measured in the plasma samples of patients with schizophrenia and controls (Pinjari et al., 2022). In the patients, P-selectin levels positively correlated with S100β and IL-6 levels, supporting the hypothesis that in schizophrenia peripheral immune activation may be associated to neuroinflammation, resulting thus in astrocytic activation. In another study (Endres et al., 2022), serum and cerebrospinal fluid samples from patients with schizophreniform and affective syndromes were examined for immunoglobulin G anti-CNS autoantibodies using tissue-based assays with indirect immunofluorescence, as part of an expanded routine clinical practice. Anti-CNS immunoglobulin G autoantibodies were identified in 18% of patients (serum 9%, CSF 18%), and among the five principal patterns that emerged one pattern was related to antibodies against astrocytes (serum 1%, CSF 1%). Finally, Jeon et al. (2021) focused on an indirect measurement of astrocytic function, by analyzing myo-inositol through 7-Tesla magnetic resonance spectroscopy at the dorsal part of anterior cingulate cortex (ACC). Specifically, myo-inositol is a molecule highly expressed in astrocytes (Brand et al., 1993), and reactive astrogliosis can cause its increased value, just as GFAP marker. For this reason, patients were scanned at baseline and after 6 months of antipsychotic treatment. At baseline, subjects affected by first-episode schizophrenia had lower levels of myo-inositol than healthy controls, while no difference was detected at follow-up, after antipsychotic treatment. Lower than normal levels of myo-inositol may indicate impaired astroglial activity, vulnerability for redox imbalance, excitotoxicity, and inappropriate astroglia-mediated inflammatory defense in the face of adversity. This supports the possibility of a putative astroglial deficit predating the first presentation of psychosis, likely of early developmental or of inflammatory origin (Dietz et al., 2020; d’Almeida et al., 2020), but showing a trend of reversal with early intervention.
3.3 Neurobiological and morphological analyses on microglia
The methodologies and results concerning investigations on microglia in patients with schizophrenia are summarized in Tables 4, 5.
3.3.1 Ex vivo
Based on literature screening, 5 articles performed ex vivo neurobiological and morphological analyses on microglia (Adorjan et al., 2020; Petrasch-Parwez et al., 2020; Uranova et al., 2020; Hill et al., 2021; Vikhreva and Uranova, 2022). Transmission electron microscopy and morphometry were conducted on microglia and adjacent oligodendrocytes in layer 5 of the PFC extracted from post-mortem brains of two groups of subjects with schizophrenia, exhibiting predominantly positive or negative symptoms, and healthy controls (Uranova et al., 2020). The qualitative analysis revealed microglial activation and dystrophic alterations in both microglia and oligodendrocytes, situated in close proximity to each other in both clinical subgroups when compared to controls. Furthermore, both clinical subgroups exhibited a reduction in volume density and number of mitochondria, alongside an increase in the number of lipofuscin granules in oligodendrocytes and microglia. In microglia, volume density of lipofuscin granules as well as volume density and number of vacuoles of endoplasmic reticulum were significantly increased in the clinical subgroup exhibiting predominantly positive symptoms, compared to controls. In this clinical subgroup, the area of the nucleus of microglial cells showed negative correlations with age and age at illness onset. The findings suggest that microglial dystrophy may contribute to oligodendrocyte dystrophy, particularly in subjects with positive symptoms during relapse, and that mitochondria in both microglia and oligodendrocytes emerge as potential targets for treatment strategies in schizophrenia. Very similarly, post-mortem electron microscopy morphometric assessment was conducted on the layer 5 of PFC of patients with chronic attack-like progressive or continuous schizophrenia, in comparison to healthy controls (Vikhreva and Uranova, 2022). Various parameters, such as the numerical density of microglia, microglial soma and nucleus areas, nucleus-cytoplasm ratio, volume fraction, area and number of mitochondria, vacuoles of endoplasmic reticulum, and lipofuscin granules were estimated. The results indicated decreases in the volume fraction and number of mitochondria in both groups of patients, along with increases in these parameters for lipofuscin granules compared to the control group. Furthermore, patients with attack-like progressive schizophrenia exhibited increased microglial density and vacuole area, compared to the controls. Specifically, microglial density was higher in the subgroup of young patients (≤50 years) compared to the subgroup of older controls (>50 years). Furthermore, young patients displayed increases in microglial soma and nuclear areas compared to elderly controls, elderly patients with attack-like progressive schizophrenia, and young patients with continuous schizophrenia. Notably, in the patients with attack-like progressive schizophrenia, contrary to patients with continuous schizophrenia, microglial soma and nuclear areas, as well as the number of mitochondria, correlated negatively with age, while the area of lipofuscin granules correlated positively with age and disease duration. Thus, chronic attack-like progressive schizophrenia is characterized by increased microglial reactivity at a young age, accompanied by dystrophic changes in microglia that progress with age and disease duration. In contrast, continuous schizophrenia is associated with decreased microglial reactivity and non-progressive dystrophic changes.
One mechanism by which neuron-microglial communication occurs is via fractalkine signaling, and in this framework Hill et al. (2021) quantified microglia and analyzed the association between the chemokine fractalkine (CX3CL1) and its microglial receptor (CX3CR1), in post-mortem brain tissue derived from dorsolateral prefrontal cortex (DLPFC) of patients with schizophrenia, or bipolar disorder, and matched controls. While CX3CR1 levels did not differ between both clinical groups and control groups, fractalkine levels were lower in patients with schizophrenia, compared to controls, and unchanged in patients with bipolar disorder. A negative association was observed between fractalkine protein levels and lifetime antipsychotic dose in patients with schizophrenia. Presence of undifferentiated or paranoid schizophrenia subtypes, prescribed antidepressants or mood stabilizers, and cause of death (suicide or other cause) did not impact levels of fractalkine and CX3CR1.
In contrast to these data, other studies have not found any difference (Adorjan et al., 2020; Petrasch-Parwez et al., 2020). By quantifying ionized calcium-binding adapter molecule 1 (Iba1) and transmembrane protein 119 (TMEM119)-immunopositive cells in striatal and cortical neurons, Adorjan et al. (2020) assessed the state of microglial activation in schizophrenia. They showed no difference between patients and controls, reporting that amoeboid forms with large cell bodies characteristic of activated microglia were occasionally seen in both groups. Accordingly, Petrasch-Parwez et al. (2020) revealed no differences in microglial density in the anterior midcingulate cortex (aMCC) of patients with schizophrenia and healthy controls. Nevertheless, the authors found a significant lateralization of microglial density to the right aMCC in patients with schizophrenia.
3.3.2 In vivo
Regarding in vivo neurobiological and morphological analyses of microglia, the literature screening did not produce any result consistent with inclusion criteria.
3.4 Neurobiological and morphological analyses on both astroglia and microglia
The methodologies and results concerning investigations on astroglia and microglia in patients with schizophrenia are summarized in Tables 6, 7.
3.4.1 Ex vivo
One study investigated the activation of both astroglia and microglia as potential contributors to the development of schizophrenia, performing ex vivo neurobiological and morphological analyses (Uranova et al., 2021). Transmission electron microscopy and morphometry techniques were employed to assess microglial density and ultrastructural parameters in layer 5 of the PFC from post-mortem brain samples of individuals with chronic schizophrenia and healthy controls. Only in patients, the researchers found the presence of dystrophic (containing damaged mitochondria) and hyperreactive (containing many vacuoles) astrocytes adjacent to microglia, characterized by focal lysis and membranous debris in the cytoplasm. The same result was found for oligodendrocytes and neurons. Microglia morphometric analysis demonstrated a 20% increase in microglial density in patients, particularly in the younger ones (≤50 years old), those with a shorter duration of illness (≤26 years), and those characterized by early age at the disease onset (≤21 years), compared to controls. Furthermore, a decrease in area, volume fraction, and number of mitochondria, as well as an increase in area, volume fraction, and number of vacuoles and lipofuscin granules were found in patients compared to the healthy subjects, especially in both young and elderly patients compared to their respective controls. The volume fraction and the number of lipofuscin granules were positively correlated with age and duration of the disease in patients. Apoptotic microglia engulfed by dystrophic astrocytes supports the evidence that microgliosis (associated with age, duration of illness, and age at the onset of the disease) indicates signs of toxicity in the PFC gray matter of patients with schizophrenia.
3.4.2 In vivo
Regarding in vivo neurobiological and morphological analyses of both astroglia and microglia, the literature screening did not produce any result consistent with inclusion criteria.
3.5 Genetic and epigenetic analyses on astroglia
The methodologies and results concerning investigations on astroglia in patients with schizophrenia are summarized in Tables 2, 3.
3.5.1 Ex vivo
Regarding ex vivo genetic and epigenetic analyses of astroglia, the literature screening produced 3 studies (O’Donovan et al., 2018; Amoah et al., 2020; Chan et al., 2020). O’Donovan et al. (2018) meticulously isolated enriched populations of pyramidal neurons and astrocytes from the DLPFC of patients with schizophrenia and control subjects, employing laser capture microdissection, and then examined the expression of components within the adenosine system, by using quantitative polymerase chain reaction (qPCR). Intriguingly, the investigation revealed notable alterations in the enriched populations of both astrocytes and neurons, encompassing various metabolic and catabolic pathways. Specifically, a reduction in mRNA levels of ectonucleoside triphosphate diphosphohydrolase-1 (ENTPD1) and ENTPD2 in the enriched populations of astrocytes was found, suggesting a modulation in the expression of genes that play a pivotal role in the regulation of ATP metabolism within astrocytes. These changes may contribute to a diminished availability of substrates necessary for the generation of adenosine.
Starting from the assumption that in the brain miRNAs can modulate multiple molecular functions from neurogenesis to neuronal differentiation, from circuitry establishment to plasticity (McNeill and Van Vactor, 2012), a recent investigation unveiled noteworthy findings through the application of mature miRNA profiling and quantitative real-time PCR (qRT-PCR) in the orbitofrontal cortex (OFC) of patients with schizophrenia, or bipolar disorder, and control subjects (Amoah et al., 2020). Specifically, an elevation in the mature miRNA levels of miR-223, an exosome-secreted miRNA known for its targeting of glutamate receptors, was found in the OFC of patients with schizophrenia or bipolar disorder who had a positive history of psychosis at the time of death. This upregulation was correlated positively with astrocyte-enriched inflammation-related mRNA SERPINA3 and negatively with its target genes coding for glutamate ionotropic receptor NMDA-type subunit 2B and glutamate ionotropic receptor AMPA-type subunit 2. Additionally, miR-223 was found to be enriched within astrocytes and highly expressed in both glial and neuronal exosomes. Its expression was also differentially regulated by antipsychotic treatment in neuronal and astrocytic cultures. Furthermore, intriguing correlations between changes in miR-223 levels and expression of inflammatory (positive association) and GABAergic genes (negative association) were demonstrated. Notably, the introduction of astrocytic exosomes to cortical neurons led to an increase in neuronal miR-223 expression and reversed by inhibiting miR-223 in astrocytes, suggesting that miRNA altered by psychosis and enriched in glial cells, potentially under the influence of antipsychotic drugs, was released via exosomes to suppress the expression of neuronal NMDA receptor genes. Finally, Chan et al. (2020) utilized methylome-wide association (MWAS) data in blood of patients with schizophrenia. By comparing the MWAS results with findings of three existing large-scale array-based methylation studies on schizophrenia, researchers found two most significant sites: the first one was located in the MFN2 gene, which encodes mitofusin-2 that regulates Ca2+ transfer from the endoplasmic reticulum to mitochondria, and the second one in ALDH1A2 gene, which encodes a key enzyme in the production of astrocyte-derived retinoic acid in the brain. Notably, retinoids are potent morphogens critical for neurodevelopment and broadly implicated in schizophrenia (Goodman, 1998).
3.5.2 In vivo
Regarding in vivo genetic and epigenetic analyses of astroglia, the literature screening did not produce any result consistent with inclusion criteria.
3.6 Genetic and epigenetic analyses on microglia
The methodologies and results concerning investigations on microglia in patients with schizophrenia are summarized in Tables 4, 5.
3.6.1 Ex vivo
Regarding ex vivo genetic and epigenetic analyses of microglia, the literature screening produced 4 studies (Adorjan et al., 2020; Hill et al., 2021; Jenkins et al., 2023; Yu et al., 2023). Employing qPCR technology, Jenkins et al. (2023) quantified transcript levels for complement components and microglia-specific phagocytic markers from post-mortem PFC of patients with schizophrenia and control subjects. The authors found markedly higher mRNA levels for C4, which marks synapses for phagocytosis by microglia, and for C1q, which initiates the classical complement pathway that includes C4, in the patients when compared to control subjects. Furthermore, in the same patients gene expression was upregulated for multiple microglia specific markers that enable phagocytic activity, including TAM receptor tyrosine kinases (Axl, MerTK) and lysosome-associated membrane glycoprotein CD68. Transcript levels for these markers of microglial phagocytosis were positively correlated with each other and with C4 mRNA levels in patients. Furthermore, higher mRNA levels for THIK1 (selectively expressed by microglia and regulating cytokine release in activated microglia) and lower mRNA levels for purinergic receptor P2Y12 (selectively expressed by microglia and scarcely expressed in activated microglia) were found in patients when compared to controls. In addition, mRNA levels for C3 or CR3 (which are additional components of the complement pathway), or for the microglia-specific markers triggering receptor expressed on myeloid cells-2 (TREM2) and its transmembrane binding partner (involved in microglial phagocytosis of spines), were not different between patients and non-psychiatric subjects. No effects of antipsychotic treatment on microglia-related gene expression were found. Analyzing the role of striatal interneurons and cortical neurons and their associations with inflammation in schizophrenia, Adorjan et al. (2020) reported no differences in the transcript levels of Iba1 and TMEM119 between patients and healthy controls. In parallel, carrying out droplet digital PCR to quantify mRNA levels for fractalkine, CX3CR1 and metalloproteinase 10 (ADAM10), Hill et al. (2021) did not show differences in the mRNA expressions in the post-mortem DLPFC of patients affected from schizophrenia, bipolar disorder, and healthy controls. Yu et al. (2023) also examined transcriptional profiles in the DLPFC of patients with schizophrenia and healthy controls through post-mortem RNA-sequencing to analyze sex-specific differences in gene expression. Using Gene Ontology Analysis and Principal Component Analysis, the researchers revealed sex-specific genes associated with schizophrenia: in the female group, schizophrenia-relevant genes were overexpressed in midbrain dopaminergic and GABAergic neurons and in PFC C3-microglia.
3.6.2 In vivo
Regarding in vivo genetic and epigenetic analyses of microglia, the literature screening produced only 1 result consistent with inclusion criteria (Jiang et al., 2021). The authors utilized several datasets of patients with schizophrenia and healthy controls to analyze the association between gene component and structural brain component, employing also an independent in vivo cohort for a second validation. Their results showed a negative correlation between the PFC/insula structural component and microglia-related genes. In the validation cohort, one of the most contributing single nucleotide polymorphisms was located in microglial-related TMEM119 gene.
3.7 Genetic and epigenetic analyses on both astroglia and microglia
The methodologies and results concerning investigations on astroglia and microglia in patients with schizophrenia are summarized in Tables 6, 7.
3.7.1 Ex vivo
Based on the literature screening, 7 articles performed ex vivo genetic and epigenetic analyses on astrocytes and microglia (Hagenauer et al., 2018; López-González et al., 2019; Karpiński et al., 2020; Kindler et al., 2020; Zhang et al., 2020; Shimamoto-Mitsuyama et al., 2021; Di Biase et al., 2022). Two studies employed a method that utilizes the collective expression of cell type transcripts in brain tissue samples to predict cell type expression (Hagenauer et al., 2018; Di Biase et al., 2022). Hagenauer et al. (2018) compiled a database of transcripts that were specifically enriched in one of the primary brain cell types based on previous single-cell or purified cell-type transcriptomic experiments: astrocytes, microglia, endothelial cells, mural cells, immature and mature oligodendrocytes, interneurons, and projection neurons. Specific cell-type transcripts were identified using forebrain or cortical samples of subjects with schizophrenia, major depressive disorder, or bipolar disorder. No difference emerged in astrocyte and microglia indices in the PFC of patients with schizophrenia. In Di Biase et al. (2022), the authors employed gene expression data from post-mortem brains for transcriptional analysis of specific cortical regions and cell types: astrocytes, microglia, endothelial cells, oligodendrocytes, oligodendrocyte progenitors, and excitatory and inhibitory neurons. In individuals with schizophrenia classified in cell-based subtypes, the associations between cortical thickness deviation profile and interregional gene expression maps of specific cell types were investigated, proving that cortical thickness deviations covaried with interregional expression levels of genes marking astrocytes (but not microglia), as well as endothelial cells, oligodendrocyte progenitors, and excitatory and inhibitory neurons.
Transcriptome and gene-set enrichment analyses were also conducted based on seven datasets collecting gene expression data from associative striatum, hippocampus, anterior PFC, superior temporal cortex, middle frontal area, DLPFC, and parietal cortex (Karpiński et al., 2020). Applying a method to deconvolute RNA expression data and estimate indexes of brain cell types, the authors observed decreased indexes of astrocytes and neurons in patients with schizophrenia in comparison to controls, both in DLPF and parietal cortex. Furthermore, in the patients (compared to controls) the index of microglia was higher in DLPFC, while indexes of microglia and endothelial cells were lower in parietal cortex. The deregulation of twenty-one key genes, including astroglial and microglial markers, was also analyzed in post-mortem DLPFC of elderly subjects with chronic schizophrenia (López-González et al., 2019). No altered expression levels for astroglial marker GFAP and lower expression levels for microglia marker CD68 were found in patients with schizophrenia compared to controls. Very similarly, mRNA expression of GFAP, Iba1, IL-1β, IL-6, IL-8, SERPINA3, as well as of kynurenine aminotransferases (KAT I, KAT II, belonging to kynurenine pathway of tryptophan catabolism linking immune system activation with neurotransmitter signaling) was measured in the post-mortem DLPFC of subjects with schizophrenia and healthy controls (Kindler et al., 2020). Results showed a positive correlation between GFAP and KAT I mRNA expression in both patients and controls, and a weaker correlation between GFAP and KAT II mRNA expression only in patients. Finally, investigating whether suicidal and non-suicidal patients with schizophrenia differ from each other in terms of glial gene expression in the PFC, qPCR was performed on isolated gray matter of the DLPFC and ACC, using a panel of common markers for astrocytes, microglia and oligodendrocytes in patients with schizophrenia who died from either suicide or from natural causes and who were compared to healthy controls (Zhang et al., 2020). Namely, mRNA expression of 16 glia-related markers including those for astroglia (aldehyde dehydrogenase 1 family member L1–ALDH1L1, and GFAP) and microglia (CX3CR1, Iba1, P2Y12 and TREM2) was quantified. In DLPFC and ACC, the expression of the astrocytic ALDH1L1 marker was higher in patients (especially who committed suicide) compared to controls. In parallel, in ACC the mRNA expression of microglial CX3CR1 and P2RY12 markers was higher in suicidal patients compared to no-suicidal patients, and TREM2 expression was lower in no-suicidal patients. The findings emphasize the heterogeneity in glia gene alterations in patients with schizophrenia in relation to suicidal conduct.
Finally, by using liquid chromatography coupled to tandem mass spectrometry and qPCR analyses, Shimamoto-Mitsuyama et al. (2021) carried out post-mortem investigations of the lipid contents of the corpus callosum from patients with schizophrenia and healthy controls, revealing low gene expression levels of microglial markers and colony-stimulating factor 1 receptor (known to regulate the density of microglia) in patients, but the same levels of astroglial (GFAP) markers between patients and controls. Genes associated to Iba1 (in the cited paper reported as allograft inflammatory factor 1–AIF1) and CD68 were downregulated in patients with schizophrenia compared to controls. The impaired schizophrenia-related expression of microglial Iba1 marker, found in the corpus callosum, was confirmed in the frontal cortex of the patients with schizophrenia. Gene expression analyses of inflammatory cytokines (such as IL-1β, IL-6, and TNF-α) revealed that the expression levels of IL-1β were significantly lower in patients than controls. Although inflammation is a major topic in schizophrenia research, these results show signs of anti-inflammatory reaction in patients with schizophrenia.
3.7.2 In vivo
Regarding in vivo genetic and epigenetic analyses of astroglia and microglia, 2 articles deal with omic-investigations (Corley et al., 2021; Zong et al., 2023). Namely, Zong et al. (2023) performed an imaging-transcriptomic-epigenetic analysis to test whether omics data and cortical connectivity organization, measured by using morphometric similarity network (MSN), could be biomarkers to predict treatment outcomes and cognitive deficits in the early phase of schizophrenia. Patients, before and after 8-week risperidone monotherapy, and healthy controls underwent magnetic resonance imaging (MRI) and diffusion tensor imaging (DTI) protocol for MSN data investigations. In parallel, peripheral blood samples were collected for genomic DNA methylation status examinations. To identify MSN variations-related genes, it was performed a virtual histology with the gene set of specific cell classes: astrocytes, microglia, oligodendrocytes, endothelial cells, oligodendrocyte progenitors, and excitatory and inhibitory neurons. From enrichment analysis emerged that longitudinal alterations in MSN after the treatment were primarily enriched in neurobiological, immunologic, metabolic, and cognitive processes. Furthermore, 174 genes were highlighted in astrocytes after the treatment with the antipsychotic drug. Finally, in patients with a diagnosis of schizophrenia or other psychotic disorders compared with healthy participants, Corley et al. (2021) performed a gene-set analysis restricted to genes associated to astroglia cells, comparing them to gene-sets from genes associated to microglia and neuronal cells. Using a recent tool for gene-set analysis, it was demonstrated that the astroglia and microglia gene-sets were not enriched for genes associated with schizophrenia risk.
3.8 Psychological correlates
Based on the literature screening, 7 articles reported psychological (cognitive and emotional) assessment (López-González et al., 2019; Kindler et al., 2020; Rodrigues-Amorim et al., 2020; Corley et al., 2021; Jeon et al., 2021; Ranganathan et al., 2021; Zong et al., 2023), 6 of whom utilized the Positive and Negative Syndrome Scale (PANSS) (López-González et al., 2019; Kindler et al., 2020; Rodrigues-Amorim et al., 2020; Jeon et al., 2021; Ranganathan et al., 2021; Zong et al., 2023), a widely used scale, validated for measuring severity and prevalence of positive or negative symptoms in schizophrenia (Opler et al., 2017). Investigating the schizophrenia-relevant protein biomarkers in plasma exosomes derived from subjects affected by schizophrenia and healthy controls, Ranganathan et al. (2021) administered PANSS to patients and concluded that the psychopathological symptomatology did not correlate with concentration of astroglial marker GFAP in circulating exosomes. Similarly, Rodrigues-Amorim et al. (2020) administered PANSS to patients with first-episode schizophrenic psychosis or chronic schizophrenia, and showed that the positive and negative subscale scores of two groups of patients were not significantly different, even if in the two groups a significantly different plasma GFAP level was found. Zong et al. (2023) administered PANSS subscales and Trail Making Test (TMT)–which examines visual-motor coordination and attention (TMT-A) and task switching (TMT-B) to drug-naïve first-episode schizophrenia patients, before and after risperidone treatment, who in parallel were submitted to neuroimaging and omic evaluations. The findings showed an association between longitudinal changes in cortical connectivity organization after treatment and the longitudinal alterations on the PANSS general psychopathology subscale. In a post-mortem study evaluating the possible deregulation of genes involved in immune system and its association with ante-mortem PANSS evaluations, López-González et al. (2019) found no significant association between severity of negative symptoms and any altered gene. Furthermore, in a longitudinal investigation of microglial integrity status, quantified by ACC myo-inositol, Jeon et al. (2021) scored patients affected from first-episode schizophrenia and healthy controls on PANSS, Social and Occupational Functioning Assessment Scale (SOFAS), and Calgary Depression Scale for Schizophrenia (CDSS). Findings highlighted that myo-inositol concentration correlated negatively with PANSS scores, weakly with SOFAS scores, and not at all with CDSS score. More extensively, Kindler et al. (2020) evaluated cognitive domains of verbal memory, language, working memory, processing speed, and perceptual organization in chronic schizophrenia or schizoaffective patients and healthy controls, administering in addition to PANSS and TMT-A, also the Wechsler Adult Intelligence Scale-Third Edition (WAIS-III), Wechsler Test of Adult Reading (WTAR), WAIS-III Letter-Number Sequencing (LNS) test, Logical Memory I and II, Verbal Memory tests of the Wechsler Memory Scale-Revised (WMS-R), the Controlled Oral Word Association Test (COWAT), and F-A-S verbal fluency test. Blood plasma samples were collected from each participant submitted to cognitive assessment and, after, to MRI scanning. Results indicated a significant reduction of cognitive domain scores in patients with schizophrenia compared to controls, as well as a decrease in DLPFC volumes. Moreover, dividing patients according to their high and low levels of cytokines, the authors showed that the patients characterized by high cytokines levels had worse scores in premorbid intellectual and attentive evaluations compared to patients characterized by low cytokines levels.
Finally, Corley et al. (2021) administered WAIS-III, WTAR, LSN test, Spatial Working Memory task and Paired Associations Learning (PAL) task from the Cambridge Automated Neuropsychological Test Battery to patients with schizophrenia, to investigate the association between cognitive domain alterations and schizophrenia risk-associated glia gene expression. Results indicated no association between schizophrenia-related polygenic astroglia and microglia score and severity of symptoms. However, schizophrenia-related microglial polygenic score was inversely associated to scores in IQ performance, IQ full-scale, and episodic memory. In a replication sample, total gray matter volume mediated the association between microglial polygenic score and episodic memory performances.
4 Discussion
Astroglial and microglial cells have been implicated in schizophrenia, yet the precise glia-associated mechanisms in the pathophysiology of disease remain poorly understood. This is primarily due to conflicting and scattered results in studies that have measured these glial populations by employing various methods, ranging from morphological analyses to epigenetic investigations. This complexity is further exacerbated from the inherently intricate clinical picture of the schizophrenia. In general, astrocytes play vital roles in maintaining the redox balance in the brain (Bélanger et al., 2011), clearing extracellular glutamate from synaptic space (Schousboe et al., 2014), maintaining synaptic integrity, and supporting myelination (Volterra and Meldolesi, 2005; Bélanger and Magistretti, 2009), all critical processes in the mechanistic pathways suspected in schizophrenia. In the present systematic review, many post-mortem and in vivo neurobiological and morphological studies conducted on individuals with schizophrenia suggest an astrocyte overactivation (Ranganathan et al., 2021; Endres et al., 2022; Huang et al., 2022; Pinjari et al., 2022). Namely, hyperreactive and dystrophic astrocytes adjacent to microglia, oligodendrocytes, and neurons, were characterized by focal lysis and membranous debris in the cytoplasm, indicating signs of toxicity (Uranova et al., 2021). Furthermore, structural proteins such as GFAP, component of astrocytes cytoskeleton and indicative of reactive astrocytes, appear to significantly influence schizophrenia (Rodrigues-Amorim et al., 2020), by altering neuroplasticity through dynamic processes that involve dendrite morphology, synapsis formation, neuronal migration and differentiation. Moreover, the structural proteins, detectable in the peripheral samples, may serve as potential biomarkers for indirectly measuring neuronal damage, especially in the prodromal stages of schizophrenia, as suggested by the higher concentration of exosomal GFAP in the plasma of patients in comparison to healthy controls (Ranganathan et al., 2021).
Throughout brain development phases, microglial cells closely communicate with the newborn neurons, being sensitive and responsive to neurochemical signs, exerting a crucial modulatory role in the formation/maturation of neurons and neuronal circuits, contributing to brain homeostasis. Microglial cells are also able to mount an inflammatory response to insults, and perform synaptic pruning, which involves the phagocytosis of synapses (Paolicelli et al., 2011; Schafer et al., 2012). Notably, an aberrant synaptic pruning occurs in schizophrenia (Germann et al., 2021; Parellada and Gassó, 2021). Patients, especially those exhibiting predominantly positive symptoms, displayed microglial activation and dystrophic alterations in the PFC (Uranova et al., 2020, 2021). Namely, it is reported that younger patients exhibited increased microglial density, with heightened reactivity at a young age (in the early phase of disease), and dystrophic changes progressing with age and disease duration (in the chronic phases). Furthermore, chronic attack-like progressive schizophrenia was characterized by increased microglial reactivity at young age, accompanied by dystrophic changes in microglia that progress with age and disease duration, while continuous schizophrenia was associated with decreased microglial reactivity and non-progressive dystrophic changes (Vikhreva and Uranova, 2022). Thus, the presence of hyperactivated and apoptotic microglia engulfed by hyperactivated and dystrophic astrocytes supports the evidence that microgliosis and astrogliosis in patients with schizophrenia, associated with age, duration of illness, and age at disease onset, are signs of toxicity that may be precipitating factors for synaptic pruning.
However, conflicting findings have been reported in other post-mortem and in vivo neurobiological and morphological studies, in which the overactivation of astroglia (Jeon et al., 2021; Schmitt et al., 2022) and microglia (Adorjan et al., 2020; Petrasch-Parwez et al., 2020) was not observed in various brain regions of patients with schizophrenia compared to controls. To clarify the discrepancies in the role of astroglial and microglial activation, along with morphological changes, in the context of schizophrenia the omic-studies conducted to explore glia-associated genetic and epigenetic aspects are crucial. Each omic-study provided insight into different mechanisms potentially linked to the pathophysiology of schizophrenia. Data revealed schizophrenia-related alterations in astrocytes associated with variations in metabolic and catabolic pathways in DLPFC (O’Donovan et al., 2018), upregulation of inflammation pathways and downregulation of genes related to glutamate receptors in OFC (Amoah et al., 2020), and involvement of the ALDH1A2 gene that encodes an enzyme in the production of astrocyte-derived retinoic acid, potent morphogen critical for neurodevelopment (Chan et al., 2020). Furthermore, in individuals with schizophrenia, cortical thickness deviations covaried with interregional expression levels of genes marking astrocytes (Di Biase et al., 2022). These studies collectively shed light on the complex genetic and epigenetic landscape associated with astroglial involvement in schizophrenia, emphasizing potential links to ATP metabolism, miRNA regulation, and retinoid-related pathways.
Vague insight into microglia-related molecular mechanisms associated with schizophrenia have been gained by many ex vivo studies employing genetic and epigenetic analyses of microglia. On one hand, multiple microglia-specific markers related to phagocytic activity and regulating cytokine release in activated microglia were upregulated in the PFC of patients with schizophrenia when compared to healthy subjects (Jenkins et al., 2023). On the other hand, contrasting results demonstrated low gene expression levels of microglial markers measured in the corpus callosum (Shimamoto-Mitsuyama et al., 2021), parietal cortex (Karpiński et al., 2020), and DLPFC (López-González et al., 2019) of patients with schizophrenia. These results along with those related to no enrichment for genes associated with schizophrenia risk in microglia gene-sets (Hagenauer et al., 2018; Adorjan et al., 2020; Corley et al., 2021; Hill et al., 2021; Di Biase et al., 2022) suggest potential anti-inflammatory or no-inflammatory reactions in the context of schizophrenia. A part of these studies reported also a lack of relationship (Hagenauer et al., 2018; López-González et al., 2019), or even an inverse relationship (Karpiński et al., 2020) between astroglia-related genes and schizophrenia risk.
Sex-specific and suicide-related differences were also found. Examining transcriptional profiles in the DLPFC of patients with schizophrenia and healthy controls through post-mortem RNA-sequencing, it has been demonstrated that schizophrenia-relevant genes were overexpressed in midbrain dopaminergic and GABAergic neurons, as well as in PFC C3-microglia of females (Yu et al., 2023). Further, higher expression of the astrocytic marker ALDH1L1 was observed in the DLPFC and ACC of patients, especially those who committed suicide, compared to controls (Zhang et al., 2020). In parallel, in ACC the microglial markers CX3CR1 and P2RY12 were higher and the microglial marker TREM2 was lower in suicidal patients compared to non-suicidal patients. Again, such findings emphasize the heterogeneity in glia gene alterations in patients with schizophrenia, also in relation to sex or suicidal conduct.
Importantly, several studies have conducted psychological (cognitive and emotional) assessments in individuals with schizophrenia, utilizing various scales and tests. A breakdown of the key findings of the mentioned studies indicates the complex interplay among cognitive functioning, symptomatology, and underlying glial mechanisms in schizophrenia. Scattering results range from no association between schizophrenia-related polygenic astroglia and microglia score and symptom severity (Corley et al., 2021) to microglial polygenic score inversely associated with scores in IQ performance, IQ full-scale, and episodic memory performances (Corley et al., 2021). Even if no significant association between severity of negative symptoms and any altered gene was found (López-González et al., 2019), and even if psychopathological symptomatology did not correlate with the concentration of the astroglial marker GFAP in circulating exosomes (Ranganathan et al., 2021), the myo-inositol concentration (index of microglial integrity status) correlated negatively with PANSS scores (Jeon et al., 2021), and patients with high cytokine levels had worse scores in premorbid intellectual and attentive evaluations (Kindler et al., 2020).
Overall, the alterations in neuron-glia communication are critical for understanding the pathological mechanisms of psychiatric disorders due to commitment of neurotransmitter systems, excitatory-inhibitory balance, neurotrophic state, and inflammatory response (Reis de Assis et al., 2021; Wartchow et al., 2023). Namely, in schizophrenia as well as in other neuropsychiatric disorders, as bipolar, mood and autism spectrum disorders, delirium, psychosis, and dementia, the activated microglia can induce the triad astrocyte reactivity, neuroinflammation, and oxidative stress (Schmitz et al., 2023). These changes in cellular responses and mechanisms causing neuronal death result in cognitive dysfunction, an often-aggravating factor of psychiatric disorders. Conversely, glioprotective factors attenuate glial damage by generating specific responses that can protect glial cells themselves and/or neurons, resulting in improved brain functioning and homeostasis (Quincozes-Santos et al., 2021). In this regard, cognitive functions impaired in schizophrenia primarily rely on excitatory synapses and dendritic spine density, crucial for neuroplasticity in cortical areas (Cahill et al., 2009). In turn, the density of synaptic connections and dendritic spines is partially regulated by astroglia and microglia which, beside their roles in neuroimmune and neuroinflammation regulation, are involved in the phagocytosis of synapses and dendritic spines (Tremblay et al., 2010; Paolicelli et al., 2011; Sekar et al., 2016; Sellgren et al., 2017; Filipello et al., 2018; Mallya et al., 2019). In schizophrenia, the overactivated astroglia and microglia may signify an immune system response to continuous dysregulation of processes related to neuroplasticity or an early reaction to early insults, possibly followed by astrogliosis and microgliosis. In both scenarios, astroglia and microglia play a crucial role in finely regulating synapse formation and elimination, contingent upon the accurate detection of neuronal/synaptic molecular signs. Astroglia and microglia undergo morphological adaptions based on their brain location and stimuli, an issue extensively studied in the context of mental diseases (Caetano et al., 2017; Simões-Henriques et al., 2020; Gaspar et al., 2021). Consequently, any alteration in astroglia and microglia has the potential to disrupt the normal course of development, influencing the number, maturation degree, and function of synapses/neurons, ultimately impacting behavior, cognitive functions, and health in the context of schizophrenia.
5 Limitation
Clinical findings are influenced by a myriad of confounding factors that must be carefully considered in future studies. These factors encompass individual variability, sex and age of onset, prevalence of positive or negative symptoms, and type and duration of pharmacological treatment for schizophrenia. Markers for astroglia and microglia used in post-mortem studies exhibit certain limitations, particularly in terms of selectivity. An overestimation of astroglia and microglia alterations may arise from the contribution of other cells stained by the same markers. Moreover, the impact of the cause of death, as well as the influence of the post-mortem interval, are crucial aspects to be considered in the design of future studies on schizophrenia-related changes in astroglia and microglia. Additionally, there are obvious technical limitations in evaluating astroglia and microglia, or their correlates, in living patients. It is imperative to reconsider and implement a variety of different markers, along with a clear definition of the outcomes to be analyzed. The interpretation of normal, increased, or decreased marker levels needs to be reexamined through a consensus evaluation, considering pathophysiological implications and their correlation with the clinical presentation of schizophrenia. Recently, in vivo microglial function in the brain has been quantified by using positron emission tomography (PET) and radiotracers specific for TSPO, a protein that is overexpressed by the activated microglia. Unfortunately, high TSPO levels compared to their relatively low physiological expression are not limited to neuroinflammation but also associated with other types of inflammation attributed to impaired cell metabolism, tumor proliferation, and autoimmune diseases. Consequently, the detection of neuroinflammation via TSPO detection may not be cell type-specific and may also involve non-inflammatory neuronal activity. This reduced specificity together with inability to differentiate between the detrimental and beneficial effects of inflammation, and inability to differentiate microglia and astroglia roles, might represent a limitation of TSPO as biomarker in brain imaging. An additional complicating factor for second-generation radioligands is their susceptibility to genetic polymorphisms responsible for differences in binding affinity that renders necessary prior genotyping of the patients. In spite of such restraints, PET with radioligands targeting the TSPO has been used to detect patterns of neuroinflammation in vivo, assess disease severity and progression, and therapeutic efficacy of anti-inflammatory drugs (Ottoy et al., 2018; Selvaraj et al., 2018; Veronese et al., 2018; De Picker et al., 2019; Schifani et al., 2019; Laurikainen et al., 2020; Conen et al., 2021; Da Silva et al., 2021; Marques et al., 2021).
Finally, the search for peripheral biomarkers reflecting neuropathological changes in schizophrenia is ongoing, even if obtaining relevant tissues in living patients proves challenging, and results have remained elusive. Exploring circulating extracellular vesicles represents a promising approach to identify potential peripheral biomarkers for schizophrenia. However, further studies are essential to elucidate the underlying mechanisms and explore potential therapeutic targets in astroglia and microglia for the treatment of schizophrenia.
Data availability statement
The original contributions presented in the study are included in the article/supplementary material, further inquiries can be directed to the corresponding author/s.
Author contributions
DL: Conceptualization, Project administration, Supervision, Visualization, Writing – original draft, Writing – review and editing. MPa: Data curation, Formal Analysis, Investigation, Methodology, Software, Validation, Visualization, Writing – original draft, Writing – review and editing. DD: Data curation, Formal Analysis, Investigation, Methodology, Resources, Software, Validation, Visualization, Writing – original draft, Writing – review and editing. AP: Data curation, Formal Analysis, Investigation, Methodology, Validation, Visualization, Writing – review and editing. DC: Conceptualization, Data curation, Supervision, Validation, Visualization, Writing – review and editing. MPe: Conceptualization, Supervision, Validation, Visualization, Writing – review and editing. CM: Funding acquisition, Supervision, Validation, Writing – review and editing. LP: Funding acquisition, Resources, Supervision, Validation, Writing – review and editing.
Funding
The author(s) declare financial support was received for the research, authorship, and/or publication of this article. This work was partially supported by University of Perugia (to DL and CM) and Ricerca Corrente Fondazione Santa Lucia (to LP).
Conflict of interest
The authors declare that the research was conducted in the absence of any commercial or financial relationships that could be construed as a potential conflict of interest.
The author(s) declared that they were an editorial board member of Frontiers, at the time of submission. This had no impact on the peer review process and the final decision.
Publisher’s note
All claims expressed in this article are solely those of the authors and do not necessarily represent those of their affiliated organizations, or those of the publisher, the editors and the reviewers. Any product that may be evaluated in this article, or claim that may be made by its manufacturer, is not guaranteed or endorsed by the publisher.
References
Adorjan, I., Sun, B., Feher, V., Tyler, T., Veres, D., Chance, S. A., et al. (2020). Evidence for decreased density of calretinin-immunopositive neurons in the caudate nucleus in patients with Schizophrenia. Front. Neuroanat. 14:581685. doi: 10.3389/fnana.2020.581685
Afridi, R., Seol, S., Kang, H. J., and Suk, K. (2021). Brain-immune interactions in neuropsychiatric disorders: lessons from transcriptome studies for molecular targeting. Biochem. Pharmacol. 188:114532. doi: 10.1016/j.bcp.2021.114532
Amoah, S. K., Rodriguez, B. A., Logothetis, C. N., Chander, P., Sellgren, C. M., Weick, J. P., et al. (2020). Exosomal secretion of a psychosis-altered miRNA that regulates glutamate receptor expression is affected by antipsychotics. Neuropsychopharmacology 45, 656–665. doi: 10.1038/s41386-019-0579-1
Barch, D. M., and Ceaser, A. (2012). Cognition in schizophrenia: core psychological and neural mechanisms. Trends Cogn. Sci. 16, 27–34.
Barker, A. J., and Ullian, E. M. (2010). Astrocytes and synaptic plasticity. Neuroscientist 16, 40–50.
Bélanger, M., Allaman, I., and Magistretti, P. J. (2011). Brain energy metabolism: focus on astrocyte-neuron metabolic cooperation. Cell Metab. 14, 724–738.
Bélanger, M., and Magistretti, P. J. (2009). The role of astroglia in neuroprotection. Dialogues Clin. Neurosci. 11, 281–295.
Benros, M. E., Mortensen, P. B., and Eaton, W. W. (2012). Autoimmune diseases and infections as risk factors for schizophrenia. Ann. N. Y. Acad. Sci. 1262, 56–66.
Brand, A., Richter-Landsberg, C., and Leibfritz, D. (1993). Multinuclear NMR studies on the energy metabolism of glial and neuronal cells. Dev. Neurosci. 15, 289–298.
Caetano, L., Pinheiro, H., Patrício, P., Mateus-Pinheiro, A., Alves, N. D., Coimbra, B., et al. (2017). Adenosine A2A receptor regulation of microglia morphological remodeling-gender bias in physiology and in a model of chronic anxiety. Mol. Psychiatry 22, 1035–1043. doi: 10.1038/mp.2016.173
Cahill, M. E., Xie, Z., Day, M., Photowala, H., Barbolina, M. V., Miller, C. A., et al. (2009). Kalirin regulates cortical spine morphogenesis and disease-related behavioral phenotypes. Proc. Natl. Acad. Sci. U S A. 106, 13058–13063. doi: 10.1073/pnas.0904636106
Chan, R. F., Shabalin, A. A., Montano, C., Hannon, E., Hultman, C. M., Fallin, M. D., et al. (2020). Independent Methylome-wide association studies of Schizophrenia detect consistent case-control differences. Schizophr. Bull. 46, 319–327. doi: 10.1093/schbul/sbz056
Chen, Y., Dai, J., Tang, L., Mikhailova, T., Liang, Q., Li, M., et al. (2023). Neuroimmune transcriptome changes in patient brains of psychiatric and neurological disorders. Mol. Psychiatry 28, 710–721.
Conen, S., Gregory, C. J., Hinz, R., Smallman, R., Corsi-Zuelli, F., Deakin, B., et al. (2021). Neuroinflammation as measured by positron emission tomography in patients with recent onset and established schizophrenia: implications for immune pathogenesis. Mol. Psychiatry 26, 5398–5406. doi: 10.1038/s41380-020-0829-y
Coomey, R., Stowell, R., Majewska, A., and Tropea, D. (2020). The role of microglia in neurodevelopmental disorders and their therapeutics. Curr. Top. Med. Chem. 20, 272–276.
Corley, E., Holleran, L., Fahey, L., Corvin, A., Morris, D. W., and Donohoe, G. (2021). Microglial-expressed genetic risk variants, cognitive function and brain volume in patients with schizophrenia and healthy controls. Transl. Psychiatry 11:490. doi: 10.1038/s41398-021-01616-z
Correll, C. U., and Schooler, N. R. (2020). Negative symptoms in Schizophrenia: a review and clinical guide for recognition, assessment, and treatment. Neuropsychiatr. Dis. Treat 16, 519–534.
Da Silva, T., Guma, E., Hafizi, S., Koppel, A., Rusjan, P., Kennedy, J. L., et al. (2021). Genetically predicted brain C4A expression is associated with TSPO and hippocampal morphology. Biol. Psychiatry 90, 652–660. doi: 10.1016/j.biopsych.2021.06.021
d’Almeida, O. C., Violante, I. R., Quendera, B., Moreno, C., Gomes, L., and Castelo-Branco, M. (2020). The neurometabolic profiles of GABA and glutamate as revealed by proton magnetic resonance spectroscopy in type 1 and type 2 diabetes. PLoS One 15:e0240907. doi: 10.1371/journal.pone.0240907
Dantzer, R. (2018). Neuroimmune interactions: from the brain to the immune system and vice versa. Physiol. Rev. 98, 477–504. doi: 10.1152/physrev.00039.2016
De Keyser, J., Mostert, J. P., and Koch, M. W. (2008). Dysfunctional astrocytes as key players in the pathogenesis of central nervous system disorders. J. Neurol. Sci. 267, 3–16.
De Picker, L., Ottoy, J., Verhaeghe, J., Deleye, S., Wyffels, L., Fransen, E., et al. (2019). State-associated changes in longitudinal [18F]-PBR111 TSPO PET imaging of psychosis patients: evidence for the accelerated ageing hypothesis? Brain Behav. Immun. 77, 46–54. doi: 10.1016/j.bbi.2018.11.318
Di Biase, M. A., Geaghan, M. P., Reay, W. R., Seidlitz, J., Weickert, C. S., Pébay, A., et al. (2022). Cell type-specific manifestations of cortical thickness heterogeneity in schizophrenia. Mol. Psychiatry 27, 2052–2060. doi: 10.1038/s41380-022-01460-7
Dietz, A. G., Goldman, S. A., and Nedergaard, M. (2020). Glial cells in schizophrenia: a unified hypothesis. Lancet Psychiatry 7, 272–281.
Endres, D., von Zedtwitz, K., Matteit, I., Bünger, I., Foverskov-Rasmussen, H., Runge, K., et al. (2022). Spectrum of novel anti-central nervous system autoantibodies in the cerebrospinal fluid of 119 patients with Schizophreniform and affective disorders. Biol. Psychiatry 92, 261–274. doi: 10.1016/j.biopsych.2022.02.010
Filipello, F., Morini, R., Corradini, I., Zerbi, V., Canzi, A., Michalski, B., et al. (2018). The microglial innate immune receptor TREM2 is required for synapse elimination and normal brain connectivity. Immunity 48, 979–991.e8. doi: 10.1016/j.immuni.2018.04.016
Fusar-Poli, P., Howes, O. D., Allen, P., Broome, M., Valli, I., Asselin, M.-C., et al. (2011). Abnormal prefrontal activation directly related to pre-synaptic striatal dopamine dysfunction in people at clinical high risk for psychosis. Mol. Psychiatry 16, 67–75. doi: 10.1038/mp.2009.108
Gaspar, R., Soares-Cunha, C., Domingues, A. V., Coimbra, B., Baptista, F. I., Pinto, L., et al. (2021). Resilience to stress and sex-specific remodeling of microglia and neuronal morphology in a rat model of anxiety and anhedonia. Neurobiol. Stress 14:100302. doi: 10.1016/j.ynstr.2021.100302
Germann, M., Brederoo, S. G., and Sommer, I. E. C. (2021). Abnormal synaptic pruning during adolescence underlying the development of psychotic disorders. Curr. Opin. Psychiatry 34, 222–227.
Goodman, A. B. (1998). Three independent lines of evidence suggest retinoids as causal to schizophrenia. Proc. Natl. Acad. Sci. U S A. 95, 7240–7244. doi: 10.1073/pnas.95.13.7240
Hagenauer, M. H., Schulmann, A., Li, J. Z., Vawter, M. P., Walsh, D. M., Thompson, R. C., et al. (2018). Inference of cell type content from human brain transcriptomic datasets illuminates the effects of age, manner of death, dissection, and psychiatric diagnosis. PLoS One 13:e0200003. doi: 10.1371/journal.pone.0200003
Haijma, S. V., Van Haren, N., Cahn, W., Koolschijn, P. C. M. P., Hulshoff Pol, H. E., and Kahn, R. S. (2013). Brain volumes in schizophrenia: a meta-analysis in over 18 000 subjects. Schizophr. Bull. 39, 1129–1138. doi: 10.1093/schbul/sbs118
Hamilton, N. B., and Attwell, D. (2010). Do astrocytes really exocytose neurotransmitters? Nat. Rev. Neurosci. 11, 227–238.
Hill, S. L., Shao, L., and Beasley, C. L. (2021). Diminished levels of the chemokine fractalkine in post-mortem prefrontal cortex in schizophrenia but not bipolar disorder. World J. Biol. Psychiatry 22, 94–103. doi: 10.1080/15622975.2020.1755451
Hol, E. M., and Pekny, M. (2015). Glial fibrillary acidic protein (GFAP) and the astrocyte intermediate filament system in diseases of the central nervous system. Curr. Opin. Cell Biol. 32, 121–130.
Hollis, C., Groom, M. J., Das, D., Calton, T., Bates, A. T., Andrews, H. K., et al. (2008). Different psychological effects of cannabis use in adolescents at genetic high risk for schizophrenia and with attention deficit/hyperactivity disorder (ADHD). Schizophr. Res. 105, 216–223. doi: 10.1016/j.schres.2008.07.010
Howes, O. D., and Kapur, S. (2009). The dopamine hypothesis of schizophrenia: version III–the final common pathway. Schizophr. Bull. 35, 549–562. doi: 10.1093/schbul/sbp006
Huang, Z., Kang, M., Li, G., Xiong, P., Chen, H., Kang, L., et al. (2022). Predictive effect of Bayes discrimination in the level of serum protein factors and cognitive dysfunction in schizophrenia. J. Psychiatr. Res. 151, 539–545. doi: 10.1016/j.jpsychires.2022.05.004
Hughes, H. K., and Ashwood, P. (2020). Overlapping evidence of innate immune dysfunction in psychotic and affective disorders. Brain Behav. Immun. Health 2:100038.
Jenkins, A. K., Lewis, D. A., and Volk, D. W. (2023). Altered expression of microglial markers of phagocytosis in schizophrenia. Schizophr. Res. 251, 22–29.
Jeon, P., Mackinley, M., Théberge, J., and Palaniyappan, L. (2021). The trajectory of putative astroglial dysfunction in first episode schizophrenia: a longitudinal 7-Tesla MRS study. Sci. Rep. 11:22333. doi: 10.1038/s41598-021-01773-7
Jiang, W., Rootes-Murdy, K., Chen, J., Bizzozero, N. I. P., Calhoun, V. D., van Erp, T. G. M., et al. (2021). Multivariate alterations in insula - medial prefrontal cortex linked to genetics in 12q24 in schizophrenia. Psychiatry Res. 306:114237. doi: 10.1016/j.psychres.2021.114237
Kantrowitz, J. T., and Javitt, D. C. (2010). N-methyl-d-aspartate (n.d.) receptor dysfunction or dysregulation: the final common pathway on the road to schizophrenia? Brain Res. Bull. 83, 108–121. doi: 10.1016/j.brainresbull.2010.04.006
Kapitein, L. C., and Hoogenraad, C. C. (2015). Building the neuronal microtubule cytoskeleton. Neuron 87, 492–506.
Karpiński, P., Samochowiec, J., Sąsiadek, M. M., and Łaczmański, Ł, and Misiak, B. (2020). Analysis of global gene expression at seven brain regions of patients with schizophrenia. Schizophr. Res. 223, 119–127. doi: 10.1016/j.schres.2020.06.032
Kindler, J., Lim, C. K., Weickert, C. S., Boerrigter, D., Galletly, C., Liu, D., et al. (2020). Dysregulation of kynurenine metabolism is related to proinflammatory cytokines, attention, and prefrontal cortex volume in schizophrenia. Mol. Psychiatry 25, 2860–2872. doi: 10.1038/s41380-019-0401-9
Kroken, R. A., Sommer, I. E., Steen, V. M., Dieset, I., and Johnsen, E. (2018). Constructing the immune signature of schizophrenia for clinical use and research; an integrative review translating descriptives into diagnostics. Front. Psychiatry 9:753. doi: 10.3389/fpsyt.2018.00753
Kucukdereli, H., Allen, N. J., Lee, A. T., Feng, A., Ozlu, M. I., Conatser, L. M., et al. (2011). Control of excitatory CNS synaptogenesis by astrocyte-secreted proteins Hevin and SPARC. Proc. Natl. Acad. Sci. U S A. 108, E440–E449. doi: 10.1073/pnas.1104977108
Laurikainen, H., Vuorela, A., Toivonen, A., Reinert-Hartwall, L., Trontti, K., Lindgren, M., et al. (2020). Elevated serum chemokine CCL22 levels in first-episode psychosis: associations with symptoms, peripheral immune state and in vivo brain glial cell function. Transl. Psychiatry 10:94. doi: 10.1038/s41398-020-0776-z
Lewis, D. A. (2012). Cortical circuit dysfunction and cognitive deficits in schizophrenia–implications for preemptive interventions. Eur. J. Neurosci. 35, 1871–1878. doi: 10.1111/j.1460-9568.2012.08156.x
López-González, I., Pinacho, R., Vila, È, Escanilla, A., Ferrer, I., and Ramos, B. (2019). Neuroinflammation in the dorsolateral prefrontal cortex in elderly chronic schizophrenia. Eur. Neuropsychopharmacol. 29, 384–396. doi: 10.1016/j.euroneuro.2018.12.011
Loughland, C., Draganic, D., McCabe, K., Richards, J., Nasir, A., Allen, J., et al. (2010). Australian Schizophrenia research bank: A database of comprehensive clinical, endophenotypic and genetic data for aetiological studies of schizophrenia. Aust. N. Z. J. Psychiatry 44, 1029–1035. doi: 10.3109/00048674.2010.501758
Mallya, A. P., Wang, H.-D., Lee, H. N. R., and Deutch, A. Y. (2019). Microglial pruning of synapses in the prefrontal cortex during adolescence. Cereb. Cortex 29, 1634–1643.
Marques, T. R., Veronese, M., Owen, D. R., Rabiner, E. A., Searle, G. E., and Howes, O. D. (2021). Specific and non-specific binding of a tracer for the translocator-specific protein in schizophrenia: an [11C]-PBR28 blocking study. Eur. J. Nucl. Med. Mol. Imaging 48, 3530–3539. doi: 10.1007/s00259-021-05327-x
Matcovitch-Natan, O., Winter, D. R., Giladi, A., Vargas Aguilar, S., Spinrad, A., Sarrazin, S., et al. (2016). Microglia development follows a stepwise program to regulate brain homeostasis. Science 353:aad8670. doi: 10.1126/science.aad8670
McGrath, J., Saha, S., Chant, D., and Welham, J. (2008). Schizophrenia: a concise overview of incidence, prevalence, and mortality. Epidemiol. Rev. 30, 67–76. doi: 10.1093/epirev/mxn001
Meyer-Lindenberg, A., and Tost, H. (2014). Neuroimaging and plasticity in schizophrenia. Restor. Neurol. Neurosci. 32, 119–127.
Millan, M. J., Andrieux, A., Bartzokis, G., Cadenhead, K., Dazzan, P., Fusar-Poli, P., et al. (2016). Altering the course of schizophrenia: progress and perspectives. Nat. Rev. Drug Discov. 15, 485–515.
Moehle, M. S., Luduena, R. F., Haroutunian, V., Meador-Woodruff, J. H., and McCullumsmith, R. E. (2012). Regional differences in expression of β-tubulin isoforms in schizophrenia. Schizophr. Res. 135, 181–186.
O’Donovan, S. M., Sullivan, C., Koene, R., Devine, E., Hasselfeld, K., Moody, C. L., et al. (2018). Cell-subtype-specific changes in adenosine pathways in schizophrenia. Neuropsychopharmacology 43, 1667–1674. doi: 10.1038/s41386-018-0028-6
Opler, M. G. A., Yavorsky, C., and Daniel, D. G. (2017). Positive and Negative Syndrome Scale (PANSS) training: challenges, solutions, and future directions. Innov. Clin. Neurosci. 14, 77–81.
O’Reilly, M. L., and Tom, V. J. (2020). Neuroimmune system as a driving force for plasticity following CNS injury. Front. Cell Neurosci. 14:187. doi: 10.3389/fncel.2020.00187
Ottoy, J., De Picker, L., Verhaeghe, J., Deleye, S., Wyffels, L., Kosten, L., et al. (2018). 18F-PBR111 PET imaging in healthy controls and Schizophrenia: test-retest reproducibility and quantification of neuroinflammation. J. Nucl. Med. 59, 1267–1274. doi: 10.2967/jnumed.117.203315
Page, M. J., McKenzie, J. E., Bossuyt, P. M., Boutron, I., Hoffmann, T. C., Mulrow, C. D., et al. (2021). The PRISMA 2020 statement: an updated guideline for reporting systematic reviews. BMJ 372:n71. doi: 10.1136/bmj.n71
Paolicelli, R. C., Bolasco, G., Pagani, F., Maggi, L., Scianni, M., Panzanelli, P., et al. (2011). Synaptic pruning by microglia is necessary for normal brain development. Science 333, 1456–1458.
Parellada, E., and Gassó, P. (2021). Glutamate and microglia activation as a driver of dendritic apoptosis: a core pathophysiological mechanism to understand schizophrenia. Transl. Psychiatry 11:271. doi: 10.1038/s41398-021-01385-9
Petrasch-Parwez, E., Schöbel, A., Benali, A., Moinfar, Z., Förster, E., Brüne, M., et al. (2020). Lateralization of increased density of Iba1-immunopositive microglial cells in the anterior midcingulate cortex of schizophrenia and bipolar disorder. Eur. Arch. Psychiatry Clin. Neurosci. 270, 819–828.
Pinjari, O. F., Dasgupta, S. K., and Okusaga, O. O. (2022). Plasma soluble P-selectin, Interleukin-6 and S100B protein in patients with schizophrenia: a pilot study. Psychiatr. Q. 93, 335–345. doi: 10.1007/s11126-021-09954-3
Quincozes-Santos, A., Santos, C. L., de Souza Almeida, R. R., da Silva, A., Thomaz, N. K., Costa, N. L. F., et al. (2021). Gliotoxicity and Glioprotection: the dual role of glial cells. Mol. Neurobiol. 58, 6577–6592.
Ranganathan, M., Rahman, M., Ganesh, S., D’Souza, D. C., Skosnik, P. D., Radhakrishnan, R., et al. (2021). Analysis of circulating exosomes reveals a peripheral signature of astrocytic pathology in schizophrenia. World J. Biol. Psychiatry 23, 33–45. doi: 10.1080/15622975.2021.1907720
Reis de Assis, D., Szabo, A., Requena Osete, J., Puppo, F., O’Connell, K. S., Akkouh, I., et al. (2021). Using iPSC models to understand the role of estrogen in neuron-glia interactions in Schizophrenia and bipolar disorder. Cells 10:209. doi: 10.3390/cells10020209
Rodrigues-Amorim, D., Rivera-Baltanás, T., Del Carmen Vallejo-Curto, M., Rodriguez-Jamardo, C., and de Las Heras, E. (2020). Plasma β-III tubulin, neurofilament light chain and glial fibrillary acidic protein are associated with neurodegeneration and progression in schizophrenia. Sci. Rep. 10:14271.
Rodrigues-Neves, A. C., Ambrósio, A. F., and Gomes, C. A. (2022). Microglia sequelae: brain signature of innate immunity in schizophrenia. Transl. Psychiatry 12:493. doi: 10.1038/s41398-022-02197-1
Saijo, K., and Glass, C. K. (2011). Microglial cell origin and phenotypes in health and disease. Nat. Rev. Immunol. 11, 775–787.
Schafer, D. P., Lehrman, E. K., Kautzman, A. G., Koyama, R., Mardinly, A. R., Yamasaki, R., et al. (2012). Microglia sculpt postnatal neural circuits in an activity and complement-dependent manner. Neuron 74, 691–705. doi: 10.1016/j.neuron.2012.03.026
Schifani, C., Hafizi, S., Tseng, H.-H., Gerritsen, C., Kenk, M., Wilson, A. A., et al. (2019). Preliminary data indicating a connection between stress-induced prefrontal dopamine release and hippocampal TSPO expression in the psychosis spectrum. Schizophr. Res. 213, 80–86. doi: 10.1016/j.schres.2018.10.008
Schizophrenia Working Group of the Psychiatric Genomics Consortium (2014). Biological insights from 108 schizophrenia-associated genetic loci. Nature 511, 421–427.
Schmitt, A., Tatsch, L., Vollhardt, A., Schneider-Axmann, T., Raabe, F. J., Roell, L., et al. (2022). Decreased oligodendrocyte number in hippocampal subfield CA4 in Schizophrenia: a replication study. Cells 11:3242.
Schmitz, I., da Silva, A., Bobermin, L. D., Gonçalves, C.-A., Steiner, J., and Quincozes-Santos, A. (2023). The Janus face of antipsychotics in glial cells: focus on glioprotection. Exp. Biol. Med. 248, 2120–2130. doi: 10.1177/15353702231222027
Schousboe, A., Scafidi, S., Bak, L. K., Waagepetersen, H. S., and McKenna, M. C. (2014). Glutamate metabolism in the brain focusing on astrocytes. Adv. Neurobiol. 11, 13–30.
Sekar, A., Bialas, A. R., de Rivera, H., Davis, A., Hammond, T. R., Kamitaki, N., et al. (2016). Schizophrenia risk from complex variation of complement component 4. Nature 530, 177–183.
Sellgren, C. M., Sheridan, S. D., Gracias, J., Xuan, D., Fu, T., and Perlis, R. H. (2017). Patient-specific models of microglia-mediated engulfment of synapses and neural progenitors. Mol. Psychiatry 22, 170–177. doi: 10.1038/mp.2016.220
Selvaraj, S., Bloomfield, P. S., Cao, B., Veronese, M., Turkheimer, F., and Howes, O. D. (2018). Brain TSPO imaging and gray matter volume in schizophrenia patients and in people at ultra high risk of psychosis: an [11C]PBR28 study. Schizophr. Res. 195, 206–214. doi: 10.1016/j.schres.2017.08.063
Shimamoto-Mitsuyama, C., Nakaya, A., Esaki, K., Balan, S., Iwayama, Y., Ohnishi, T., et al. (2021). Lipid pathology of the corpus callosum in schizophrenia and the potential role of abnormal gene regulatory networks with reduced microglial marker expression. Cereb. Cortex 31, 448–462. doi: 10.1093/cercor/bhaa236
Simões-Henriques, C., Mateus-Pinheiro, M., Gaspar, R., Pinheiro, H., Mendes Duarte, J., Baptista, F. I., et al. (2020). Microglia cytoarchitecture in the brain of adenosine A2A receptor knockout mice: brain region and sex specificities. Eur. J. Neurosci. 51, 1377–1387. doi: 10.1111/ejn.14561
Smyth, A. M., and Lawrie, S. M. (2013). The neuroimmunology of schizophrenia. Clin. Psychopharmacol. Neurosci. 11, 107–117.
Suzuki, A., Stern, S. A., Bozdagi, O., Huntley, G. W., Walker, R. H., Magistretti, P. J., et al. (2011). Astrocyte-neuron lactate transport is required for long-term memory formation. Cell 144, 810–823.
Takahashi, N., and Sakurai, T. (2013). Roles of glial cells in schizophrenia: possible targets for therapeutic approaches. Neurobiol. Dis. 53, 49–60.
Thion, M. S., Ginhoux, F., and Garel, S. (2018). Microglia and early brain development: an intimate journey. Science 362, 185–189. doi: 10.1126/science.aat0474
Tremblay, M. -È, Lowery, R. L., and Majewska, A. K. (2010). Microglial interactions with synapses are modulated by visual experience. PLoS Biol. 8:e1000527. doi: 10.1371/journal.pbio.1000527
Uranova, N. A., Vikhreva, O. V., and Rakhmanova, V. I. (2021). Abnormal microglial reactivity in gray matter of the prefrontal cortex in schizophrenia. Asian J. Psychiatr 63:102752. doi: 10.1016/j.ajp.2021.102752
Uranova, N. A., Vikhreva, O. V., Rakhmanova, V. I., and Orlovskaya, D. D. (2020). Dystrophy of oligodendrocytes and adjacent microglia in prefrontal gray matter in Schizophrenia. Front. Psychiatry 11:204. doi: 10.3389/fpsyt.2020.00204
Van Essen, D. C., Smith, S. M., Barch, D. M., Behrens, T. E. J., Yacoub, E., Ugurbil, K., et al. (2013). The WU-Minn human connectome project: An overview. Neuroimage 80, 62–79. doi: 10.1016/j.neuroimage.2013.05.041
Veronese, M., Reis Marques, T., Bloomfield, P. S., Rizzo, G., Singh, N., Jones, D., et al. (2018). Kinetic modelling of [11C]PBR28 for 18 kDa translocator protein PET data: a validation study of vascular modelling in the brain using XBD173 and tissue analysis. J. Cereb. Blood Flow Metab. 38, 1227–1242.
Vikhreva, O. V., and Uranova, N. A. (2022). Microglial reactivity in the prefrontal cortex in Schizophrenia with different types of course. Neurosci. Behav. Phys. 52, 639–644.
Volterra, A., and Meldolesi, J. (2005). Astrocytes, from brain glue to communication elements: the revolution continues. Nat. Rev. Neurosci. 6, 626–640. doi: 10.1038/nrn1722
Walter, H., Kammerer, H., Frasch, K., Spitzer, M., and Abler, B. (2009). Altered reward functions in patients on atypical antipsychotic medication in line with the revised dopamine hypothesis of schizophrenia. Psychopharmacology 206, 121–132. doi: 10.1007/s00213-009-1586-4
Wartchow, K. M., Scaini, G., and Quevedo, J. (2023). Glial-neuronal interaction in synapses: a possible mechanism of the pathophysiology of bipolar disorder. Adv. Exp. Med. Biol. 1411, 191–208.
Woodburn, S. C., Bollinger, J. L., and Wohleb, E. S. (2021). The semantics of microglia activation: neuroinflammation, homeostasis, and stress. J. Neuroinflammation 18:258. doi: 10.1186/s12974-021-02309-6
Yu, Z., Ueno, K., Funayama, R., Sakai, M., Nariai, N., Kojima, K., et al. (2023). Sex-specific differences in the transcriptome of the human dorsolateral prefrontal cortex in Schizophrenia. Mol. Neurobiol. 60, 1083–1098. doi: 10.1007/s12035-022-03109-6
Zhang, X., Kim, J., and Tonegawa, S. (2020). Amygdala reward neurons form and store fear extinction memory. Neuron 105, 1077–1093.e7. doi: 10.1016/j.neuron.2019.12.025
Zhang, Y., and Barres, B. A. (2010). Astrocyte heterogeneity: an underappreciated topic in neurobiology. Curr. Opin. Neurobiol. 20, 588–594. doi: 10.1016/j.conb.2010.06.005
Zong, X., Zhang, J., Li, L., Yao, T., Ma, S., Kang, L., et al. (2023). Virtual histology of morphometric similarity network after risperidone monotherapy and imaging-epigenetic biomarkers for treatment response in first-episode schizophrenia. Asian J. Psychiatr 80:103406. doi: 10.1016/j.ajp.2022.103406
Keywords: GFAP, Iba1, cognitive symptoms, inflammatory pathways, immune system
Citation: Laricchiuta D, Papi M, Decandia D, Panuccio A, Cutuli D, Peciccia M, Mazzeschi C and Petrosini L (2024) The role of glial cells in mental illness: a systematic review on astroglia and microglia as potential players in schizophrenia and its cognitive and emotional aspects. Front. Cell. Neurosci. 18:1358450. doi: 10.3389/fncel.2024.1358450
Received: 19 December 2023; Accepted: 29 January 2024;
Published: 14 February 2024.
Edited by:
Veronica Ghiglieri, Università Telematica San Raffaele, ItalyReviewed by:
Kazuhiko Yamamuro, Nara Medical University, JapanAlessandro Usiello, Second University of Naples, Italy
Copyright © 2024 Laricchiuta, Papi, Decandia, Panuccio, Cutuli, Peciccia, Mazzeschi and Petrosini. This is an open-access article distributed under the terms of the Creative Commons Attribution License (CC BY). The use, distribution or reproduction in other forums is permitted, provided the original author(s) and the copyright owner(s) are credited and that the original publication in this journal is cited, in accordance with accepted academic practice. No use, distribution or reproduction is permitted which does not comply with these terms.
*Correspondence: Daniela Laricchiuta, ZGFuaWVsYS5sYXJpY2NoaXV0YUB1bmlwZy5pdA==
†Present address: Davide Decandia and Anna Panuccio PhD Program in Behavioral Neuroscience, University Sapienza of Rome, Rome, Italy
‡These authors share first authorship