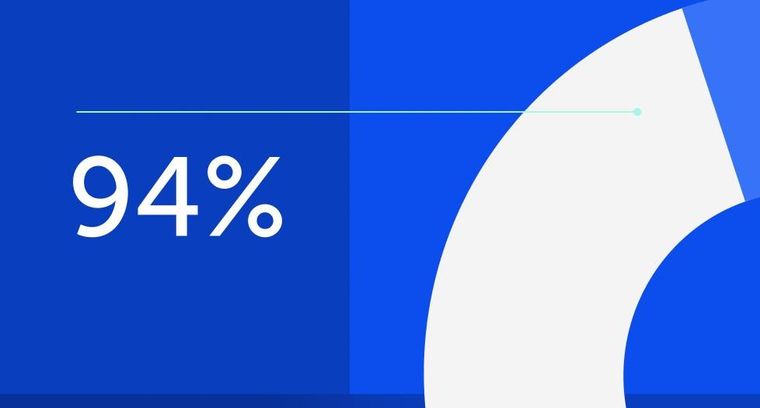
94% of researchers rate our articles as excellent or good
Learn more about the work of our research integrity team to safeguard the quality of each article we publish.
Find out more
REVIEW article
Front. Cell. Neurosci., 20 March 2024
Sec. Cellular Neuropathology
Volume 18 - 2024 | https://doi.org/10.3389/fncel.2024.1351734
This article is part of the Research TopicDevelopmental Brain Diseases Through the Lens of iPS Cells and OrganoidsView all 8 articles
Stem cell-derived organoid technology is a powerful tool that revolutionizes the field of biomedical research and extends the scope of our understanding of human biology and diseases. Brain organoids especially open an opportunity for human brain research and modeling many human neurological diseases, which have lagged due to the inaccessibility of human brain samples and lack of similarity with other animal models. Brain organoids can be generated through various protocols and mimic whole brain or region-specific. To provide an overview of brain organoid technology, we summarize currently available protocols and list several factors to consider before choosing protocols. We also outline the limitations of current protocols and challenges that need to be solved in future investigation of brain development and pathobiology.
Organoid refers to a mini cluster of cells growing in a three-dimensional (3D) environment in vitro and recapitulating a structural and functional organ in vivo (Corro et al., 2020). Human organoids can be formed from tissue fragments or stem cells because cells and tissues can self-organize and dissociate-aggregate. Organoids derived from human tissue samples, such as biopsies, surgical specimens, or fetal material, are also called primary organoids (Cala et al., 2023). Most primary organoids use extracellular matrix (ECM) as scaffolds to support the growth of primary cells under specific culture conditions. For example, breast epithelial cells can fully form 3D ducts and ductules that exhibit the function of milk protein secretion by using matrigel as scaffolds (Li et al., 1987; Shannon et al., 1987; Dekkers et al., 2021). With the rapid development of stem cell technologies, 3D organoids derived from stem cells have been widely adopted; many studies have been using stem cells derived organoids to recapitulate the key structure and function of organs such as the kidney, lung, intestine, brain, and retina under physical or pathological conditions (Lancaster et al., 2013; Morizane et al., 2015; Crespo et al., 2017; Miller et al., 2019; Norrie et al., 2021).
Due to the inaccessibility of human brain tissue and lack of appropriate in vitro models, our understanding of human brain development and function still needs to catch up. Recent advances in 3D organoid technology provide us with a powerful tool to investigate the complexity of brain development and functions. The first study of 3D cerebral organoids was published by Lancaster et al. (2013). Since then, many brain organoid protocols have been developed (Qian et al., 2018; Sloan et al., 2018; Tanaka et al., 2020; Kim et al., 2021; Valiulahi et al., 2021; Lee et al., 2022). In the current review, 223 articles were obtained from Pubmed using the search terms “protocol, brain organoids, stem cell” from 2013 to 2023; we removed reviews and brain organoid-unrelated articles and added a few articles through cross-referencing. A total of 114 articles were included and reviewed in Supplementary Table 1.
Organoid protocol: Generation of brain organoids starts with 3D embryoid body (EB) formation, neural induction, differentiation, and maturation. Brain organoids can be generated through either unguided (36 out of 114 articles) or guided (78 out of 114 articles) protocols. Through the unguided protocol, stem cells undergo spontaneous differentiation without any extrinsic factors, and these organoids contain multiple cell types and brain regions (Lancaster et al., 2013; Lancaster and Knoblich, 2014). In contrast, stem cells undergo guided differentiation (Eiraku et al., 2008; Kadoshima et al., 2013; Pasca et al., 2015) by the addition of extrinsic factors to mimic morphogen gradient during embryonic brain development and to pattern these organoids with region-specific identity. Patterning of region-specific organoids is achieved by manipulations of Suppressor of Mothers Against Decapentaplegic (SMAD), Wingless/integrated (WNT), Sonic hedgehog (SHH), retinoic acid (RA), and other signaling pathways, such as FGF and Notch, during EB formation and neural induction. First, SMAD inhibition includes inhibiting bone morphogenetic protein (BMP) and tumor growth factor ß (TGFß) signaling pathways that promote neuroectodermal fate. Then, the dorsal and ventral patterning are obtained through BMP/WNT inhibition and SHH activation, respectively, while rostralization and caudalization are obtained by inhibition or activation of RA, WNT, and FGF signaling pathways (Tanaka and Park, 2021; Zhang et al., 2022). FGFs and Notch signals affect neuroepithelial patterning either directly or through modulations of SHH signaling pathway (Gutin et al., 2006; Kong et al., 2015; Farreny et al., 2018). Furthermore, the strength and exposure time of diffusible morphogens and crosstalk among different signaling pathways are also critical for precise pattern formation (Fattah et al., 2023). Various combinations of morphogens from each signaling pathway were applied to generate region-specific organoids, such as dorsal (Pasca et al., 2015; Sebastian et al., 2023), ventral (Sloan et al., 2018; Eigenhuis et al., 2023; Mulder et al., 2023), hippocampal (Jacob et al., 2020), cerebellum (Silva et al., 2020; Atamian et al., 2024), hindbrain (Valiulahi et al., 2021), and spinal cord (Lee et al., 2022) brain regions. As shown in Supplementary Table 1, cortical and dorsal forebrain organoids often use BMP and TGF inhibitors; in contrast, caudal parts of the brain, such as hindbrain and spinal cord organoids, frequently use WNT, RA, and FGF activators instead. Within region-specific organoid protocols, cortical organoids are the most pursued. Therefore, we use cortical organoids as an example to discuss several factors that need to be considered before deciding on protocol, such as the use of extracellular matrix (ECM), assembloids, cellular stress, and multi-rosette vs. single-rosette organoids (Figure 1).
Figure 1. 3D Brain organoids can be generated through guided and unguided protocols to mimic either the whole brain or specific brain regions. Several factors such as the use of extracellular matrix (e.g., matrigel), the number of rosettes within each organoid, and the choice of assembloids should be considered before deciding on the protocol. 3D organoids can be used as a model system to investigate neurodevelopmental, and neurodegenerative diseases, as well as drug discovery. The figure was created with Biorender.com.
Extracellular matrix: ECM is known to play an essential role in directing cell fate and differentiation, especially in promoting the stem cell niche for self-organization, differentiation, and organoid expansion (Hughes et al., 2010; Tang et al., 2023). Indeed, early exposure to exogenous ECM such as matrigel can trigger quick neuroepithelial morphogenesis (Martins-Costa et al., 2023); organoids without ECM form compact unpolarized tissues with a lack of large ventricles and loss of certain types of radial glial cells during neuronal differentiations as compared to organoids with ECM (Chiaradia et al., 2023). However, Martins-Costa et al. (2023) demonstrated that cell types and tissue morphology over long-term organoid development are comparable and independent of using exogenous ECM. Furthermore, they speculated that permanently supplementing exogenous ECM may contribute to mispatterning in the organoid culture.
ECM embedding was widely used in organoid protocols by many investigators (67 out of 114 articles). The current ECMs used in the cortical organoid culture (Heo et al., 2022) include natural scaffolds such as matrigel (Lancaster et al., 2013), decellularized tissue-derived scaffolds (Cho et al., 2021; Simsa et al., 2021) and synthetic polymer-based scaffolds (Lancaster et al., 2017; Oksdath et al., 2018; Hofer and Lutolf, 2021). Matrigel is the most used ECM preparation in the organoid protocols (Mulder et al., 2023). Matrigel is extracted from murine Engelbreth-Holm-Swarm sarcomas cells containing more than 1800 unique proteins (Hughes et al., 2010). Undefined features, manual embedding, and potential matrigel batch-to-batch variability resulted in a higher variability and lower reproducibility in organoids generated with matrigel. Although this variability can be minimized by more defined matrigel alternatives such as synthetic hydrogel scaffolds (Table 1) (Barry et al., 2017; Heo et al., 2022), the development of engineered scaffolds is still in the early stage, and the effect of these biomaterials for the organoid cultures need further investigation.
Assembloids: The development of organoid technology has enabled us to aim at assembling various regions of the brain to model human diseases in vitro. Like other organs, the human brain contains multiple regions and cell types. Organoids generated through the unguided protocol contain diverse cells and brain regions but can exhibit significant variations among organoids or batches (Camp et al., 2015). In contrast, region-specific organoids generated through the guided protocol contain a limited type of neurons and increased reproducibility (Chiaradia et al., 2023). Assembloids provide a partial solution to overcome such limitations (Table 1). Assembloids are obtained by incorporating two or more organoids with different regional identities into multi-region assembloids, or organoids with varying types of cell into multi-lineage assembloids, or inter-individual assembloids as well as inter-species assembloids (Pasca et al., 2022).
The human brain has three primary regions: the cerebrum, cerebellum, and brain stem. The cerebrum is further divided into two hemispheres, each with four lobes: frontal, temporal, parietal, and occipital. Each lobe can then be separated into multiple areas, and each serving distinct functions. The wiring among these areas connects the brain into a network that allows the brain to perform more sophisticated functions. The availability of region-specific brain organoid protocol will enable researchers to build a variety of assembloids to model the interconnection among these regions and mimic as much as possible developmental processes and diseases that span multiple areas of the brain (Kelley and Pasca, 2022). For instance, fusion between dorsal and ventral forebrain organoids is used to model interneuron migration (Bagley et al., 2017; Birey et al., 2017; Samarasinghe et al., 2021). Fusions of cortical organoids with other brain regions such as (1) thalamus to model thalamus dysfunction-related psychiatric disorders (Xiang et al., 2019, 2020; Angulo Salavarria et al., 2023); (2) thalamus and retina to model the projections of visual system (Fligor et al., 2021); (3) striatum to model the dysfunction in neural circuits of cortico-striatal pathway as observed in autism spectrum disorder and schizophrenia (Miura et al., 2020, 2022); and (4) spinal cord and muscle to model cortical control of motor contractions (Knecht et al., 2019; Andersen et al., 2020). Other brain regions, such as the hypothalamus and pituitary, can be fused as assembloids to model the hypothalamic-pituitary axis (Kasai et al., 2020).
The central nervous system contains neurons and non-neuronal cells such as astrocytes, oligodendrocytes, microglia, epithelial cells, pericytes, and endothelial cells. Co-culturing microglia or endothelial cells with brain organoids forms multi-lineage assembloids to study the interactions between neurons and other non-neuronal cell types that impact neuronal functions. Microglia are primary immune cells in the brain that not only protect the brain from pathological insults (Xu et al., 2016) but also play a critical role in neural development, synaptic formation, and neural network maturation (Tong and Vidyadaran, 2016; Coomey et al., 2020). Since microglia are derived from mesoderm lineage and neurons are derived from ectoderm lineage, co-cultured microglia with brain organoids can be achieved by using the unguided protocol for spontaneous formation of microglia, or introducing primary microglia and stem cell-derived microglia or microglial progenitor cells to brain organoids (Ormel et al., 2018; Zhang W. et al., 2023). Stem cell-derived microglia function and morphologically resemble in vivo microglia; they become ameboid and phagocytic upon activation (Haenseler et al., 2017). A controllable proportion and distribution of microglia in organoids are essential to study the microglial function under physiological conditions or to model microglial dysfunction-related neurological disorders from neuropsychiatric disorders to neurodegenerative diseases (Xu et al., 2021; Schafer et al., 2023). Co-culture of endothelial cells with brain organoids forms a vascular-like network and vascularized organoids (Pham et al., 2018; Cakir et al., 2019; Shi et al., 2020; Sun et al., 2022, 2023) which makes it possible to recapitulate the critical processes of vasculogenesis, angiogenesis, and vasculopathy related human diseases. Additionally, co-culturing peripheral neurons, such as enteric neurons, with human intestinal organoids expands our scope to study the connection between the gut and brain (Ryan et al., 1985; Hampton, 2017; Schlieve et al., 2017; Horvath et al., 2023).
Cellular stress: Because of a lack of functional blood vessels and other supporting non-neuronal cells, organoids cannot fully mimic the intrinsic complexity of brain tissue. Indeed, Bhaduri et al. (2020) have demonstrated that organoids across all protocols have increased activation of glycolysis and ER stress pathways and impaired cell-subtype specifications compared to fetal tissue. Increasing cellular stress and forming a necrotic core have been reported in organoids that are larger than 500 μm in diameter due to insufficient perfusion of oxygen, nutrients, and catabolites (Hirschhaeuser et al., 2010; Langan et al., 2016; Magliaro et al., 2019).
Several strategies have been attempted to address these questions (Table 1). For example, spinning bioreactor or orbital shaking instead of static culture is commonly used to improve the delivery of oxygen and nutrients in organoids (Lancaster and Knoblich, 2014). Higher levels of oxygen (40% instead of 20% O2) culture and continuous laminar flow supplied with the Millifludic culture system are also applied for long-term organoid culture (Kadoshima et al., 2013; Berger et al., 2018). Neocortical organoid slices cultured on an air-liquid interface allow sliced cortical plates to continue expansion, neurogenesis, and maturation (Qian et al., 2020; Giandomenico et al., 2021). Furthermore, using a small molecule cocktail named CEPT (chroman 1, emricasan, polyamines, trans-ISRIB), a polypharmacological approach can enhance cytoprotection and improve organoid survival (Ryu et al., 2023). In addition, organoid transplantation was confirmed to alleviate the cellular stress in organoids (Bhaduri et al., 2020) and improve organoid maturity, cellular complexity, and brain function (Cao et al., 2023; Jgamadze et al., 2023; Wang et al., 2023). Lastly, Vertesy et al. (2022) developed a computational algorithm method, Gruffi, to remove the stressed cells from the organoid single-cell RNAseq dataset, and therefore, improves the bioinformatic data analysis after the organoids are collected.
Multi- or single-rosette organoids: Although the diverse cell types and regions contribute to the complexity of the human brain, brain regions arise from one neural tube during embryogenesis in vivo. The current brain organoid protocols (Lancaster et al., 2013; Pasca et al., 2015) have multiple rosettes within each organoid, and each rosette acts as an independent organizing center. The unpredictable number and organization of rosettes within each organoid results in a lack of reproducibility and fidelity (Table 1). Therefore, the self-organizing single rosette (SOSR) organoid was developed and adopted by several laboratories in the past few years (Knight et al., 2018; Wang et al., 2022; Takla et al., 2023). These well-defined SOSR organoids with reproducible size and cytoarchitecture offer improved reproducibility and fidelity (Knight et al., 2018; Tidball et al., 2023) than the existing organoid protocols (Lancaster et al., 2013; Pasca et al., 2015) and can be used as a reliable model to recapitulate the neural development and related disorders in the human brain.
In vitro organoids vs. in vivo fetal brain: The 3D organoids recapitulate many unique features of the human brain and have been widely used as a model system to study brain development and neuropathological disorders. However, how well do in vitro organoids match up with the in vivo fetal brain condition? The equivalent age still needs to be determined.
Transcriptomic analysis is the most used method to address this question. Amiri et al. (2018) compared the transcriptomic data between fetal cortex samples and 11 to 41-day-old dorsal forebrain organoids generated through Mariani's guided protocol. They revealed that the organoids' transcriptomes map to the human fetal cortex between 8 and 16 post-conception weeks (PCW) of development (Mariani et al., 2015). To minimize the effect of organoid protocols on data analysis, Magni et al. (2022) generated the cortical organoids with three different protocols: one unguided approach with ECM embedding, two guided approaches with or without ECM embedding and WNT activation. They concluded that 3 months of cortical organoids closely resembled 9 PCW or 20 PCW human embryonic cortex samples more than 25-day early organoids (Magni et al., 2022) based on the neuronal maturation gene expression profile. Gordon et al. (2021) compared gene expression profiles of cortical organoid culture with the BrainSpan dataset at multiple time points over a more extended period (up to 652 days). BrainSpan is a reference database that contains developmental and postnatal transcriptome information from in vivo human brains. They reported that cortical organoids before 250 days map to the prenatal stage (10–38 PCW), and 250 to 300-day-old organoids represent the transition between prenatal and postnatal stages in vivo (Kang et al., 2011; Gordon et al., 2021). Recently, Cheroni et al. (2022) compared the RNAseq dataset from in-house generated cortical brain organoids (Pasca et al., 2015) and three other organoids, including forebrain organoids (Qian et al., 2016), telencephalic organoids (Mariani et al., 2015), and minimally-guided organoids (Luo et al., 2016) at comparable time-point (from 1 to 200 days) with fetal cortex at 8–37 PCW. They demonstrated that forebrain and minimally-guided organoids showed a more rapid transcriptional maturation than cortical brain organoids because 60-day forebrain and minimally-guided organoids showed a similarity with late PCE fetal cortex that cortical brain organoids reached by 100 days (Cheroni et al., 2022). Those studies further confirmed the presence of heterochronicity across different protocols when dissecting the equivalent age of brain organoids relative to the fetal brain.
Using DNA methylation sequencing, Luo et al. (2016) demonstrated that 40 to 60-day-old organoids recapitulate many epigenetic signatures of the mid-fetal (12–16 PCW) brain. Trevino et al. (2020) used the ATAC-seq to show that 40 to 80-day-old organoids resemble the human fetal brain at 8–10 PCW, 80–250-day-old organoids resemble mid- to the late fetal stage (10 PCW to birth), and postnatal stage are more similar to organoids after 350 days (Lewis et al., 2021).
To our knowledge, only one electrophysiological functional study compared the age of organoids with in vivo fetal brains. Trujillo et al. (2019) used multi-electrode array to record organoids for up to 10 months; they compared the local field potential of organoids with the previously published preterm EEG recordings from preterm infants ranging from 24 to 38 weeks post-menstrual age. They report that complex oscillatory waves of cortical organoids after 28 weeks of maturation resemble the electrophysiological signature of preterm human infant EEG (Trujillo et al., 2019).
In summary, 2~3-month organoid culture can mimic in vivo early to mid-fetal brain development, and ~10-month-old organoids are more likely to reach the transition stage between prenatal and postnatal brain development. The exact age equivalent is still challenging because different organoid protocols were used in each study. For studies that mimic drug treatment or hormone surge at a specific time or period during fetal brain development (Madhavan et al., 2018; Kelava et al., 2022), carefully choosing organoid protocol and adopting equivalent age is needed.
Stem cell-derived 3D organoids, especially brain organoids, have been a powerful tool to open an opportunity for human brain research and model many neurological diseases such as neurodevelopmental disorders and neurodegenerative diseases (Silbereis et al., 2016) (Figure 1).
Modeling neurodevelopmental disorders: Organoid culture resembles early fetal brain development and can model a variety of neurodevelopmental disorders such as autism spectrum disorders, schizophrenia, attention-deficit/hyperactivity disorder, Down syndrome, and fragile X syndrome (Chan et al., 2020; Kang et al., 2021; Notaras et al., 2022; Rabeling and Goolam, 2022; Zhao and Haddad, 2022; Santos et al., 2023; Zhang D. et al., 2023). Neurodevelopmental disorders are a group of conditions that affect the development and maturation of the human brain and impact patients' ability to learn, speech, behavior, memory, and emotions. Autism spectrum disorder (ASD) is one of the most studied neurodevelopmental disorders using stem cells and organoids (Chan et al., 2020; Santos et al., 2023). ASD has a broad spectrum of phenotypes with highly heterogeneous features. More than 1,000 genes have been reported to be associated with the risk of ASD (Antaki et al., 2022; Qiu et al., 2022). Brain organoids allow us to investigate the role of individual ASD risk genes such as FOXG1, SHNAK3, and CNTNAP2 in the neuropathology of ASD and study the potential interaction between ASD risk genes and environments or drugs in vitro (Mariani et al., 2015; De Jong et al., 2021; Schmidt, 2021; Meng et al., 2022; Wang et al., 2022). Furthermore, network analysis reveals that those ASD risk genes have tissue-specific transcriptional convergence implicating fetal brain development, neurogenesis, and synaptic processes (De La Torre-Ubieta et al., 2016; Wen et al., 2016; Sullivan et al., 2019; Paulsen et al., 2022). Consistently, Paulsen et al. (2022) have reported that ASD cortical organoids indeed showed cell-type-specific developmental abnormality. As compared to control organoids, ASD organoids with three individual ASD risk genes, including SUV420H1, ARID1B, and CHD8, converge on a phenotype of asynchronous neuronal development and abnormal circuit activity due to premature expansion of GABAergic neuron linage, but each gene works with different molecular mechanisms (Paulsen et al., 2022). Therefore, organoids act as a valuable tool that allows us to investigate brain development and disease pathology at the cellular, molecular and network levels and also bridge our knowledge gap between genetic analysis and neuropathological observations.
Modeling environmental effects on neurological disorders: Prenatal environmental adversities, including infectious agents, medication, and substance use, are risk factors for neurodevelopmental disorders. Adverse prenatal exposure is often associated with abnormal brain development and has cognitive consequences (Debost et al., 2017; Bolte et al., 2019; Smith, 2021). Organoids have been used to investigate the effect of infectious agents such as Zika virus (ZIKV), Severe acute respiratory syndrome coronavirus 2 (SARS-CoV-2), and Herpes simplex virus type I on brain cytotoxicity (Ramani et al., 2020; Xu and Wen, 2021; Qiao et al., 2022). A ZIKV outbreak, which increased the number of infants born with microcephaly in Brazil, led scientists to investigate the potential linkage between the virus and brain malformation. Studies have shown that ZIKV infection induces cell death of neural progenitor cells in brain organoids and reduces proliferation zones and disruption of cortical layers (Cugola et al., 2016; Garcez et al., 2016). Later, another study used organoids to demonstrate that duramycin and ivermectin have therapeutic potential for anti-ZIKV infection due to a significant reduction in the adverse effect of ZIKV infection on cortical development (Watanabe et al., 2017).
Prenatal substance exposure is increasingly becoming a significant public health concern due to its impact on women's health and child development (Hirai et al., 2021). For instance, maternal opioid use during pregnancy affects fetal brain development and causes cognitive dysfunction. These problems persist into adolescence or later, even if the mother was treated with methadone or buprenorphine. Kaltentach et al. used clinical assessment to evaluate brain development in infants at 3–36 months of age born to mothers who were opioid-dependent and treated with either buprenorphine or methadone during pregnancy. They reported that buprenorphine or methadone exposure had no deleterious effect on the usual physical and mental development of children (Oh et al., 2011). However, our laboratory has used brain organoids to investigate the impact of both buprenorphine and methadone on brain development. Dwivedi et al. (2023) have shown that methadone exposure altered transcriptional program, especially in synaptogenesis during early cortical development. Furthermore, Yao et al. (2020) found that methadone dose-dependently inhibits the growth of brain organoids and suppresses neural network activities; buprenorphine does not affect neural growth in brain organoids and had a mild suppression of network activities as compared with methadone (Yao et al., 2023). Therefore, organoid technology can help in our understanding of drug exposure as well as potential therapeutic modalities.
Modeling neurodegenerative diseases: Besides neurodevelopmental diseases, organoids can be a valuable tool for modeling neurodegenerative diseases such as Alzheimer's disease (AD), Parkinson's disease, and Huntington's disease (Bose et al., 2022; Bubnys and Tsai, 2022; Metzger et al., 2022). AD is characterized by two major pathological features: amyloid plaque (Amyloid ß accumulation) and neurofibrillary tangles (tau hyperphosphorylation). iPSC-derived organoids from both early-onset familial AD (FAD) and late-onset sporadic AD (SAD) patients accurately capture these neuropathological features and have an increased amyloid β peptide (Aβ), Aβ42/40 ratio and tau hyperphosphorylation (Chen et al., 2018; Gonzalez et al., 2018; Kuehner et al., 2021; Park et al., 2021; Bubnys and Tsai, 2022; Yanakiev et al., 2022). Progressively increased Aβ accumulation was observed in a time-dependent manner. For example, Raja et al. (2016) reported an increased Aβ accumulation from 60 days to 90 days in organoids with APP duplication. Similarly, Zhao and Haddad (2022) used Down syndrome (DS) organoids as a model to study AD pathology and reported an increased Aβ accumulation from 8 weeks organoids to 12 weeks organoids.
Recently, Arber et al. (2021) reported that FAD organoids with presenilin mutation not only resemble AD pathology but also exhibit abnormal neurogenesis, such as premature terminal differentiation of neural progenitor cells and a trend of reduced abundance of newborn neurons. Abnormal neurogenesis observed in AD organoids suggests that the pathology of AD brain may start as early as fetal brain development. In clinics, AD biomarkers such as cerebrospinal fluid Aß42 and Tau can be detected decades before the onset of AD dementia (Sperling et al., 2011; Bateman et al., 2012; Buchhave et al., 2012). Pre-tangle alterations with positive immunostaining of phosphor-tau AT8 are detected in about 12.5% of non-selected autopsy cases under 20 years of age (Braak et al., 2011). Therefore, Arendt et al. (2017) have proposed an argument for the developmental origin of AD. AD pathology may begin as early as the fetal stage or childhood; the developmental defect may not lead to disease but increase the susceptibility to disease onset with the second hit from either genetic or environmental stimulus later in life (Arendt et al., 2017). A similar assumption is also proposed for Parkinson's disease due to altered dopaminergic neurogenesis (Barlow et al., 2007; Schwamborn, 2018), which may partially explain why we observe the pathology of neurodegenerative disease in stem cell-derived brain organoids, a neurodevelopmental model.
Organoid technology is a powerful tool that revolutionized the field of biomedical research and extended the scope of our understanding of human biology and diseases in both breadth and depth. Brain organoids can be generated in large quantities and the application of brain organoids for high-throughput drug screening, transplantation, and toxicology therefore becomes time- and cost-efficient as compared to the use of traditional animal models (Lee et al., 2018; Renner et al., 2020; Wang et al., 2020; Dong et al., 2021; Groveman et al., 2021). However, limitations and challenges still exist (Table 1). For example, organoids do not fully resemble brain cytoarchitecture and function due to missing cell types and often brain parts and structures. Although incorporation of additional cell types, such as microglia, astrocytes, vascular tissue, and other brain regions, will improve the complexity of brain organoids, organoid protocols must also be improved to reduce the variation among individual organoids or batches and prevent organoids from cellular stress. Furthermore, extending the duration of organoid culture may better recapitulate the later stages of human brain development and the aging brain manifested in neurodegenerative disease.
HZ: Conceptualization, Writing – original draft, Writing – review & editing. GH: Conceptualization, Funding acquisition, Supervision, Writing – review & editing.
The author(s) declare financial support was received for the research, authorship, and/or publication of this article. This work was supported by National Institutes of Health (NIH) grant (1R01DA053372).
The authors declare that the research was conducted in the absence of any commercial or financial relationships that could be construed as a potential conflict of interest.
All claims expressed in this article are solely those of the authors and do not necessarily represent those of their affiliated organizations, or those of the publisher, the editors and the reviewers. Any product that may be evaluated in this article, or claim that may be made by its manufacturer, is not guaranteed or endorsed by the publisher.
The Supplementary Material for this article can be found online at: https://www.frontiersin.org/articles/10.3389/fncel.2024.1351734/full#supplementary-material
Amiri, A., Coppola, G., Scuderi, S., Wu, F., Roychowdhury, T., Liu, F., et al. (2018). Transcriptome and epigenome landscape of human cortical development modeled in organoids. Science 362:6720. doi: 10.1126/science.aat6720
Andersen, J., Revah, O., Miura, Y., Thom, N., Amin, N. D., Kelley, K. W., et al. (2020). Generation of functional human 3D cortico-motor assembloids. Cell 183, 1913–1929. e1926. doi: 10.1016/j.cell.2020.11.017
Angulo Salavarria, M. M., Dell'amico, C., D'agostino, A., Conti, L., and Onorati, M. (2023). Cortico-thalamic development and disease: from cells, to circuits, to schizophrenia. Front. Neuroanat. 17:1130797. doi: 10.3389/fnana.2023.1130797
Antaki, D., Guevara, J., Maihofer, A. X., Klein, M., Gujral, M., Grove, J., et al. (2022). A phenotypic spectrum of autism is attributable to the combined effects of rare variants, polygenic risk and sex. Nat. Genet. 54, 1284–1292. doi: 10.1038/s41588-022-01064-5
Arber, C., Lovejoy, C., Harris, L., Willumsen, N., Alatza, A., Casey, J. M., et al. (2021). Familial Alzheimer's disease mutations in PSEN1 lead to premature human stem cell neurogenesis. Cell Rep. 34:108615. doi: 10.1016/j.celrep.2020.108615
Arendt, T., Stieler, J., and Ueberham, U. (2017). Is sporadic Alzheimer's disease a developmental disorder? J. Neurochem. 143, 396–408. doi: 10.1111/jnc.14036
Atamian, A., Birtele, M., Hosseini, N., Nguyen, T., Seth, A., Del Dosso, A., et al. (2024). Human cerebellar organoids with functional Purkinje cells. Cell Stem Cell 31, 39–51. doi: 10.1016/j.stem.2023.11.013
Bagley, J. A., Reumann, D., Bian, S., Levi-Strauss, J., and Knoblich, J. A. (2017). Fused cerebral organoids model interactions between brain regions. Nat. Methods 14, 743–751. doi: 10.1038/nmeth.4304
Barlow, B. K., Cory-Slechta, D. A., Richfield, E. K., and Thiruchelvam, M. (2007). The gestational environment and Parkinson's disease: evidence for neurodevelopmental origins of a neurodegenerative disorder. Reprod. Toxicol. 23, 457–470. doi: 10.1016/j.reprotox.2007.01.007
Barry, C., Schmitz, M. T., Propson, N. E., Hou, Z., Zhang, J., Nguyen, B. K., et al. (2017). Uniform neural tissue models produced on synthetic hydrogels using standard culture techniques. Exp. Biol. Med. 242, 1679–1689. doi: 10.1177/1535370217715028
Bateman, R. J., Xiong, C., Benzinger, T. L., Fagan, A. M., Goate, A., Fox, N. C., et al. (2012). Clinical and biomarker changes in dominantly inherited Alzheimer's disease. N. Engl. J. Med. 367, 795–804. doi: 10.1056/NEJMoa1202753
Berger, E., Magliaro, C., Paczia, N., Monzel, A. S., Antony, P., Linster, C. L., et al. (2018). Millifluidic culture improves human midbrain organoid vitality and differentiation. Lab Chip 18, 3172–3183. doi: 10.1039/C8LC00206A
Bhaduri, A., Andrews, M. G., Mancia Leon, W., Jung, D., Shin, D., Allen, D., et al. (2020). Cell stress in cortical organoids impairs molecular subtype specification. Nature 578, 142–148. doi: 10.1038/s41586-020-1962-0
Birey, F., Andersen, J., Makinson, C. D., Islam, S., Wei, W., Huber, N., et al. (2017). Assembly of functionally integrated human forebrain spheroids. Nature 545, 54–59. doi: 10.1038/nature22330
Bolte, S., Girdler, S., and Marschik, P. B. (2019). The contribution of environmental exposure to the etiology of autism spectrum disorder. Cell. Mol. Life Sci. 76, 1275–1297. doi: 10.1007/s00018-018-2988-4
Bose, A., Petsko, G. A., and Studer, L. (2022). Induced pluripotent stem cells: a tool for modeling Parkinson's disease. Trends Neurosci. 45, 608–620. doi: 10.1016/j.tins.2022.05.001
Braak, H., Thal, D. R., Ghebremedhin, E., and Del Tredici, K. (2011). Stages of the pathologic process in Alzheimer disease: age categories from 1 to 100 years. J. Neuropathol. Exp. Neurol. 70, 960–969. doi: 10.1097/NEN.0b013e318232a379
Bubnys, A., and Tsai, L. H. (2022). Harnessing cerebral organoids for Alzheimer's disease research. Curr. Opin. Neurobiol. 72, 120–130. doi: 10.1016/j.conb.2021.10.003
Buchhave, P., Minthon, L., Zetterberg, H., Wallin, A. K., Blennow, K., Hansson, O., et al. (2012). Cerebrospinal fluid levels of beta-amyloid 1-42, but not of tau, are fully changed already 5 to 10 years before the onset of Alzheimer dementia. Arch. Gen. Psychiatry 69, 98–106. doi: 10.1001/archgenpsychiatry.2011.155
Cakir, B., Xiang, Y., Tanaka, Y., Kural, M. H., Parent, M., Kang, Y. J., et al. (2019). Engineering of human brain organoids with a functional vascular-like system. Nat. Methods 16, 1169–1175. doi: 10.1038/s41592-019-0586-5
Cala, G., Sina, B., Coppi, D., Giobbe, P. G. G., and Gerli, M. F. M. (2023). Primary human organoids models: current progress and key milestones. Front. Bioeng. Biotechnol. 11:1058970. doi: 10.3389/fbioe.2023.1058970
Camp, J. G., Badsha, F., Florio, M., Kanton, S., Gerber, T., Wilsch-Brauninger, M., et al. (2015). Human cerebral organoids recapitulate gene expression programs of fetal neocortex development. Proc. Natl. Acad. Sci. USA. 112, 15672–15677. doi: 10.1073/pnas.1520760112
Cao, S. Y., Yang, D., Huang, Z. Q., Lin, Y. H., Wu, H. Y., Chang, L., et al. (2023). Cerebral organoids transplantation repairs infarcted cortex and restores impaired function after stroke. NPJ Regen. Med. 8:27. doi: 10.1038/s41536-023-00301-7
Chan, W. K., Griffiths, R., Price, D. J., and Mason, J. O. (2020). Cerebral organoids as tools to identify the developmental roots of autism. Mol. Autism 11:58. doi: 10.1186/s13229-020-00360-3
Chen, M., Lee, H. K., Moo, L., Hanlon, E., Stein, T., Xia, W., et al. (2018). Common proteomic profiles of induced pluripotent stem cell-derived three-dimensional neurons and brain tissue from Alzheimer patients. J. Proteomics 182, 21–33. doi: 10.1016/j.jprot.2018.04.032
Cheroni, C., Trattaro, S., Caporale, N., Lopez-Tobon, A., Tenderini, E., Sebastiani, S., et al. (2022). Benchmarking brain organoid recapitulation of fetal corticogenesis. Transl. Psychiatry 12, 520. doi: 10.1038/s41398-022-02279-0
Chiaradia, I., Imaz-Rosshandler, I., Nilges, B. S., Boulanger, J., Pellegrini, L., Das, R., et al. (2023). Tissue morphology influences the temporal program of human brain organoid development. Cell Stem Cell 30, 1351–1367. doi: 10.1016/j.stem.2023.09.003
Cho, A. N., Jin, Y., An, Y., Kim, J., Choi, Y. S., Lee, J. S., et al. (2021). Microfluidic device with brain extracellular matrix promotes structural and functional maturation of human brain organoids. Nat. Commun. 12:4730. doi: 10.1038/s41467-021-24775-5
Coomey, R., Stowell, R., Majewska, A., and Tropea, D. (2020). The role of microglia in neurodevelopmental disorders and their therapeutics. Curr. Top. Med. Chem. 20, 272–276. doi: 10.2174/1568026620666200221172619
Corro, C., Novellasdemunt, L., and Li, V. S. W. (2020). A brief history of organoids. Am. J. Physiol. Cell Physiol. 319, C151–C165. doi: 10.1152/ajpcell.00120.2020
Crespo, M., Vilar, E., Tsai, S. Y., Chang, K., Amin, S., Srinivasan, T., et al. (2017). Colonic organoids derived from human induced pluripotent stem cells for modeling colorectal cancer and drug testing. Nat. Med. 23, 878–884. doi: 10.1038/nm.4355
Cugola, F. R., Fernandes, I. R., Russo, F. B., Freitas, B. C., Dias, J. L., Guimaraes, K. P., et al. (2016). The Brazilian Zika virus strain causes birth defects in experimental models. Nature 534, 267–271. doi: 10.1038/nature18296
De Jong, D., Llapashtica, J. O., Genestine, C., Strauss, M., Provenzano, K., Sun, F., et al. (2021). Cortical overgrowth in a preclinical forebrain organoid model of CNTNAP2-associated autism spectrum disorder. Nat. Commun. 12:4087. doi: 10.1038/s41467-021-24358-4
De La Torre-Ubieta, D., Won, L., Stein, H. J. L., and Geschwind, D. H. (2016). Advancing the understanding of autism disease mechanisms through genetics. Nat. Med. 22, 345–361. doi: 10.1038/nm.4071
Debost, J. P., Larsen, J. T., Munk-Olsen, T., Mortensen, P. B., Meyer, U., Petersen, L., et al. (2017). Joint effects of exposure to prenatal infection and peripubertal psychological trauma in schizophrenia. Schizophr. Bull. 43, 171–179. doi: 10.1093/schbul/sbw083
Dekkers, J. F., Van Vliet, E. J., Sachs, N., Rosenbluth, J. M., Kopper, O., Rebel, H. G., et al. (2021). Long-term culture, genetic manipulation and xenotransplantation of human normal and breast cancer organoids. Nat. Protoc. 16, 1936–1965. doi: 10.1038/s41596-020-00474-1
Dong, X., Xu, S. B., Chen, X., Tao, M., Tang, X. Y., Fang, K. H., et al. (2021). Human cerebral organoids establish subcortical projections in the mouse brain after transplantation. Mol. Psychiatry 26, 2964–2976. doi: 10.1038/s41380-020-00910-4
Dwivedi, I., Caldwell, A. B., Zhou, D., Wu, W., Subramaniam, S., Haddad, G. G., et al. (2023). Methadone alters transcriptional programs associated with synapse formation in human cortical organoids. Transl. Psychiatry 13:151. doi: 10.1038/s41398-023-02397-3
Eigenhuis, K. N., Somsen, H. B., Van Der Kroeg, M., Smeenk, H., Korporaal, A. L., Kushner, S. A., et al. (2023). A simplified protocol for the generation of cortical brain organoids. Front. Cell. Neurosci. 17:1114420. doi: 10.3389/fncel.2023.1114420
Eiraku, M., Watanabe, K., Matsuo-Takasaki, M., Kawada, M., Yonemura, S., Matsumura, M., et al. (2008). Self-organized formation of polarized cortical tissues from ESCs and its active manipulation by extrinsic signals. Cell Stem Cell 3, 519–532. doi: 10.1016/j.stem.2008.09.002
Farreny, M. A., Agius, E., Bel-Vialar, S., Escalas, N., Khouri-Farah, N., Soukkarieh, C., et al. (2018). FGF signaling controls Shh-dependent oligodendroglial fate specification in the ventral spinal cord. Neural Dev. 13:3. doi: 10.1186/s13064-018-0100-2
Fattah, A. R. A., Grebenyuk, S., De Rooij, D., Salmon, L. P. M. H. I., and Ranga, A. (2023). Neuroepithelial organoid patterning is mediated by a neighborhood watch mechanism. Cell Rep. 42:113498. doi: 10.1016/j.celrep.2023.113498
Fligor, C. M., Lavekar, S. S., Harkin, J., Shields, P. K., Vanderwall, K. B., Huang, K. C., et al. (2021). Extension of retinofugal projections in an assembled model of human pluripotent stem cell-derived organoids. Stem Cell Rep. 16, 2228–2241. doi: 10.1016/j.stemcr.2021.05.009
Garcez, P. P., Loiola, E. C., Madeiro Da Costa, R., Higa, L. M., Trindade, P., Delvecchio, R., et al. (2016). Zika virus impairs growth in human neurospheres and brain organoids. Science 352, 816–818. doi: 10.1126/science.aaf6116
Giandomenico, S. L., Sutcliffe, M., and Lancaster, M. A. (2021). Generation and long-term culture of advanced cerebral organoids for studying later stages of neural development. Nat. Protoc. 16, 579–602. doi: 10.1038/s41596-020-00433-w
Gonzalez, C., Armijo, E., Bravo-Alegria, J., Becerra-Calixto, A., Mays, C. E., Soto, C., et al. (2018). Modeling amyloid beta and tau pathology in human cerebral organoids. Mol. Psychiatry 23, 2363–2374. doi: 10.1038/s41380-018-0229-8
Gordon, A., Yoon, S. J., Tran, S. S., Makinson, C. D., Park, J. Y., Andersen, J., et al. (2021). Long-term maturation of human cortical organoids matches key early postnatal transitions. Nat. Neurosci. 24, 331–342. doi: 10.1038/s41593-021-00802-y
Groveman, B. R., Ferreira, N. C., Foliaki, S. T., Walters, R. O., Winkler, C. W., Race, B., et al. (2021). Human cerebral organoids as a therapeutic drug screening model for Creutzfeldt-Jakob disease. Sci. Rep. 11:5165. doi: 10.1038/s41598-021-84689-6
Gutin, G., Fernandes, M., Palazzolo, L., Paek, H., Yu, K., Ornitz, D. M., et al. (2006). FGF signalling generates ventral telencephalic cells independently of SHH. Development 133, 2937–2946. doi: 10.1242/dev.02465
Haenseler, W., Sansom, S. N., Buchrieser, J., Newey, S. E., Moore, C. S., Nicholls, F. J., et al. (2017). A highly efficient human pluripotent stem cell microglia model displays a neuronal-co-culture-specific expression profile and inflammatory response. Stem Cell Rep. 8, 1727–1742. doi: 10.1016/j.stemcr.2017.05.017
Hampton, T. (2017). Organoids reveal clues to gut-brain communication. JAMA 318, 787–788. doi: 10.1001/jama.2017.11545
Heo, J. H., Kang, D., Seo, S. J., and Jin, Y. (2022). Engineering the extracellular matrix for organoid culture. Int. J. Stem Cells 15, 60–69. doi: 10.15283/ijsc21190
Hirai, A. H., Ko, J. Y., Owens, P. L., Stocks, C., and Patrick, S. W. (2021). Neonatal abstinence syndrome and maternal opioid-related diagnoses in the US, 2010-2017. JAMA 325, 146–155. doi: 10.1001/jama.2020.24991
Hirschhaeuser, F., Menne, H., Dittfeld, C., West, J., Mueller-Klieser, W., Kunz-Schughart, L. A., et al. (2010). Multicellular tumor spheroids: an underestimated tool is catching up again. J. Biotechnol. 148, 3–15. doi: 10.1016/j.jbiotec.2010.01.012
Hofer, M., and Lutolf, M. P. (2021). Engineering organoids. Nat. Rev. Mater 6, 402–420. doi: 10.1038/s41578-021-00279-y
Horvath, T. D., Haidacher, S. J., Engevik, M. A., Luck, B., Ruan, W., Ihekweazu, F., et al. (2023). Interrogation of the mammalian gut-brain axis using LC-MS/MS-based targeted metabolomics with in vitro bacterial and organoid cultures and in vivo gnotobiotic mouse models. Nat. Protoc. 18, 490–529. doi: 10.1038/s41596-022-00767-7
Hughes, C. S., Postovit, L. M., and Lajoie, G. A. (2010). Matrigel: a complex protein mixture required for optimal growth of cell culture. Proteomics 10, 1886–1890. doi: 10.1002/pmic.200900758
Jacob, F., Pather, S. R., Huang, W. K., Zhang, F., Wong, S. Z. H., Zhou, H., et al. (2020). Human pluripotent stem cell-derived neural cells and brain organoids reveal SARS-CoV-2 neurotropism predominates in choroid plexus epithelium. Cell Stem Cell 27, 937–950. doi: 10.1016/j.stem.2020.09.016
Jgamadze, D., Lim, J. T., Zhang, Z., Harary, P. M., Germi, J., Mensah-Brown, K., et al. (2023). Structural and functional integration of human forebrain organoids with the injured adult rat visual system. Cell Stem Cell 30, 137–152. doi: 10.1016/j.stem.2023.01.004
Kadoshima, T., Sakaguchi, H., Nakano, T., Soen, M., Ando, S., Eiraku, M., et al. (2013). Self-organization of axial polarity, inside-out layer pattern, and species-specific progenitor dynamics in human ES cell-derived neocortex. Proc. Natl. Acad. Sci. USA. 110, 20284–20289. doi: 10.1073/pnas.1315710110
Kang, H. J., Kawasawa, Y. I., Cheng, F., Zhu, Y., Xu, X., Li, M., et al. (2011). Spatio-temporal transcriptome of the human brain. Nature 478, 483–489. doi: 10.1038/nature10523
Kang, Y., Zhou, Y., Li, Y., Han, Y., Xu, J., Niu, W., et al. (2021). A human forebrain organoid model of fragile X syndrome exhibits altered neurogenesis and highlights new treatment strategies. Nat. Neurosci. 24, 1377–1391. doi: 10.1038/s41593-021-00913-6
Kasai, T., Suga, H., Sakakibara, M., Ozone, C., Matsumoto, R., Kano, M., et al. (2020). Hypothalamic contribution to pituitary functions is recapitulated in vitro using 3D-cultured human ips cells. Cell Rep 30, 18–24. doi: 10.1016/j.celrep.2019.12.009
Kelava, I., Chiaradia, I., Pellegrini, L., Kalinka, A. T., and Lancaster, M. A. (2022). Androgens increase excitatory neurogenic potential in human brain organoids. Nature 602, 112–116. doi: 10.1038/s41586-021-04330-4
Kelley, K. W., and Pasca, S. P. (2022). Human brain organogenesis: Toward a cellular understanding of development and disease. Cell 185, 42–61. doi: 10.1016/j.cell.2021.10.003
Kim, J., Sullivan, G. J., and Park, I. H. (2021). How well do brain organoids capture your brain? iScience 24:102063. doi: 10.1016/j.isci.2021.102063
Knecht, S., Jensen, H. J. A., and Saue, T. (2019). Relativistic quantum chemical calculations show that the uranium molecule U(2) has a quadruple bond. Nat. Chem. 11, 40–44. doi: 10.1038/s41557-018-0158-9
Knight, G. T., Lundin, B. F., Iyer, N., Ashton, L. M., Sethares, W. A., Willett, R. M., et al. (2018). Engineering induction of singular neural rosette emergence within hPSC-derived tissues. Elife 7:30. doi: 10.7554/eLife.37549.030
Kong, J. H., Yang, L., Dessaud, E., Chuang, K., Moore, D. M., Rohatgi, R., et al. (2015). Notch activity modulates the responsiveness of neural progenitors to sonic hedgehog signaling. Dev. Cell 33, 373–387. doi: 10.1016/j.devcel.2015.03.005
Kuehner, J. N., Chen, J., Bruggeman, E. C., Wang, F., Li, Y., Xu, C., et al. (2021). 5-hydroxymethylcytosine is dynamically regulated during forebrain organoid development and aberrantly altered in Alzheimer's disease. Cell Rep. 35:109042. doi: 10.1016/j.celrep.2021.109042
Lancaster, M. A., Corsini, N. S., Wolfinger, S., Gustafson, E. H., Phillips, A. W., Burkard, T. R., et al. (2017). Guided self-organization and cortical plate formation in human brain organoids. Nat. Biotechnol. 35, 659–666. doi: 10.1038/nbt.3906
Lancaster, M. A., and Knoblich, J. A. (2014). Generation of cerebral organoids from human pluripotent stem cells. Nat. Protoc. 9, 2329–2340. doi: 10.1038/nprot.2014.158
Lancaster, M. A., Renner, M., Martin, C. A., Wenzel, D., Bicknell, L. S., Hurles, M. E., et al. (2013). Cerebral organoids model human brain development and microcephaly. Nature 501, 373–379. doi: 10.1038/nature12517
Langan, L. M., Dodd, N. J., Owen, S. F., Purcell, W. M., Jackson, S. K., Jha, A. N., et al. (2016). Direct measurements of oxygen gradients in spheroid culture system using electron parametric resonance oximetry. PLoS ONE 11:e0149492. doi: 10.1371/journal.pone.0149492
Lee, J. H., Shin, H., Shaker, M. R., Kim, H. J., Park, S. H., Kim, J. H., et al. (2022). Production of human spinal-cord organoids recapitulating neural-tube morphogenesis. Nat. Biomed. Eng. 6, 435–448. doi: 10.1038/s41551-022-00868-4
Lee, S. H., Hu, W., Matulay, J. T., Silva, M. V., Owczarek, T. B., Kim, K., et al. (2018). Tumor evolution and drug response in patient-derived organoid models of bladder cancer. Cell 173, 515–528. doi: 10.1016/j.cell.2018.03.017
Lewis, E. M. A., Kaushik, K., Sandoval, L. A., Antony, I., Dietmann, S., Kroll, K. L., et al. (2021). Epigenetic regulation during human cortical development: Seq-ing answers from the brain to the organoid. Neurochem. Int. 147, 105039. doi: 10.1016/j.neuint.2021.105039
Li, M. L., Aggeler, J., Farson, D. A., Hatier, C., Hassell, J., Bissell, M. J., et al. (1987). Influence of a reconstituted basement membrane and its components on casein gene expression and secretion in mouse mammary epithelial cells. Proc. Natl. Acad. Sci. U. S. A. 84, 136–140. doi: 10.1073/pnas.84.1.136
Luo, C., Lancaster, M. A., Castanon, R., Nery, J. R., Knoblich, J. A., Ecker, J. R., et al. (2016). Cerebral organoids recapitulate epigenomic signatures of the human fetal brain. Cell Rep. 17, 3369–3384. doi: 10.1016/j.celrep.2016.12.001
Madhavan, M., Nevin, Z. S., Shick, H. E., Garrison, E., Clarkson-Paredes, C., Karl, M., et al. (2018). Induction of myelinating oligodendrocytes in human cortical spheroids. Nat. Methods 15, 700–706. doi: 10.1038/s41592-018-0081-4
Magliaro, C., Rinaldo, A., and Ahluwalia, A. (2019). Allometric scaling of physiologically-relevant organoids. Sci. Rep. 9, 11890. doi: 10.1038/s41598-019-48347-2
Magni, M., Bossi, B., Conforti, P., Galimberti, M., Dezi, F., Lischetti, T., et al. (2022). Brain regional identity and cell type specificity landscape of human cortical organoid models. Int. J. Mol. Sci. 23:159. doi: 10.3390/ijms232113159
Mariani, J., Coppola, G., Zhang, P., Abyzov, A., Provini, L., Tomasini, L., et al. (2015). FOXG1-dependent dysregulation of GABA/glutamate neuron differentiation in autism spectrum disorders. Cell 162, 375–390. doi: 10.1016/j.cell.2015.06.034
Martins-Costa, C., Pham, V. A., Sidhaye, J., Novatchkova, M., Wiegers, A., Peer, A., et al. (2023). Morphogenesis and development of human telencephalic organoids in the absence and presence of exogenous extracellular matrix. EMBO J. 42:e113213. doi: 10.15252/embj.2022113213
Meng, Q., Zhang, W., Wang, X., Jiao, C., Xu, S., Liu, C., et al. (2022). Human forebrain organoids reveal connections between valproic acid exposure and autism risk. Transl. Psychiatry 12:130. doi: 10.1038/s41398-022-01898-x
Metzger, J. J., Pereda, C., Adhikari, A., Haremaki, T., Galgoczi, S., Siggia, E. D., et al. (2022). Deep-learning analysis of micropattern-based organoids enables high-throughput drug screening of Huntington's disease models. Cell Rep. Methods 2:100297. doi: 10.1016/j.crmeth.2022.100297
Miller, A. J., Dye, B. R., Ferrer-Torres, D., Hill, D. R., Overeem, A. W., Shea, L. D., et al. (2019). Generation of lung organoids from human pluripotent stem cells in vitro. Nat. Protoc. 14, 518–540. doi: 10.1038/s41596-018-0104-8
Miura, Y., Li, M. Y., Birey, F., Ikeda, K., Revah, O., Thete, M. V., et al. (2020). Generation of human striatal organoids and cortico-striatal assembloids from human pluripotent stem cells. Nat. Biotechnol. 38, 1421–1430. doi: 10.1038/s41587-020-00763-w
Miura, Y., Li, M. Y., Revah, O., Yoon, S. J., Narazaki, G., Pasca, S. P., et al. (2022). Engineering brain assembloids to interrogate human neural circuits. Nat. Protoc. 17, 15–35. doi: 10.1038/s41596-021-00632-z
Morizane, R., Lam, A. Q., Freedman, B. S., Kishi, S., Valerius, M. T., Bonventre, J. V., et al. (2015). Nephron organoids derived from human pluripotent stem cells model kidney development and injury. Nat. Biotechnol. 33, 1193–1200. doi: 10.1038/nbt.3392
Mulder, L. A., Depla, J. A., Sridhar, A., Wolthers, K., Pajkrt, D., Vieira De Sa, R., et al. (2023). A beginner's guide on the use of brain organoids for neuroscientists: a systematic review. Stem Cell Res. Ther. 14:87. doi: 10.1186/s13287-023-03302-x
Norrie, J. L., Nityanandam, A., Lai, K., Chen, X., Wilson, M., Stewart, E., et al. (2021). Retinoblastoma from human stem cell-derived retinal organoids. Nat. Commun. 12:4535. doi: 10.1038/s41467-021-24781-7
Notaras, M., Lodhi, A., Dundar, F., Collier, P., Sayles, N. M., Tilgner, H., et al. (2022). Schizophrenia is defined by cell-specific neuropathology and multiple neurodevelopmental mechanisms in patient-derived cerebral organoids. Mol. Psychiatry 27, 1416–1434. doi: 10.1038/s41380-021-01316-6
Oh, D., Zidovska, A., Xu, Y., and Needleman, D. J. (2011). Development of time-integrated multipoint moment analysis for spatially resolved fluctuation spectroscopy with high time resolution. Biophys. J. 101, 1546–1554. doi: 10.1016/j.bpj.2011.08.013
Oksdath, M., Perrin, S. L., Bardy, C., Hilder, E. F., Deforest, C. A., Arrua, R. D., et al. (2018). Review: synthetic scaffolds to control the biochemical, mechanical, and geometrical environment of stem cell-derived brain organoids. APL Bioeng. 2:041501. doi: 10.1063/1.5045124
Ormel, P. R., De Sá, D., Van Bodegraven, R. V., Karst, E. J., Harschnitz, H., Sneeboer, O., et al. (2018). Microglia innately develop within cerebral organoids. Nat. Commun. 9:684. doi: 10.1038/s41467-018-06684-2
Park, J. C., Jang, S. Y., Lee, D., Lee, J., Kang, U., Chang, H., et al. (2021). A logical network-based drug-screening platform for Alzheimer's disease representing pathological features of human brain organoids. Nat. Commun. 12, 280. doi: 10.1038/s41467-020-20440-5
Pasca, A. M., Sloan, S. A., Clarke, L. E., Tian, Y., Makinson, C. D., Huber, N., et al. (2015). Functional cortical neurons and astrocytes from human pluripotent stem cells in 3D culture. Nat. Methods 12, 671–678. doi: 10.1038/nmeth.3415
Pasca, S. P., Arlotta, P., Bateup, H. S., Camp, J. G., Cappello, S., Gage, F. H., et al. (2022). A nomenclature consensus for nervous system organoids and assembloids. Nature 609, 907–910. doi: 10.1038/s41586-022-05219-6
Paulsen, B., Velasco, S., Kedaigle, A. J., Pigoni, M., Quadrato, G., Deo, A. J., et al. (2022). Autism genes converge on asynchronous development of shared neuron classes. Nature 602, 268–273. doi: 10.1038/s41586-021-04358-6
Pham, M. T., Pollock, K. M., Rose, M. D., Cary, W. A., Stewart, H. R., Zhou, P., et al. (2018). Generation of human vascularized brain organoids. Neuroreport 29, 588–593. doi: 10.1097/WNR.0000000000001014
Qian, X., Jacob, F., Song, M. M., Nguyen, H. N., Song, H., Ming, G. L., et al. (2018). Generation of human brain region-specific organoids using a miniaturized spinning bioreactor. Nat. Protoc. 13, 565–580. doi: 10.1038/nprot.2017.152
Qian, X., Nguyen, H. N., Song, M. M., Hadiono, C., Ogden, S. C., Hammack, C., et al. (2016). Brain-region-specific organoids using mini-bioreactors for modeling ZIKV exposure. Cell 165, 1238–1254. doi: 10.1016/j.cell.2016.04.032
Qian, X., Su, Y., Adam, C. D., Deutschmann, A. U., Pather, S. R., Goldberg, E. M., et al. (2020). Sliced human cortical organoids for modeling distinct cortical layer formation. Cell Stem Cell 26, 766–781. doi: 10.1016/j.stem.2020.02.002
Qiao, H., Zhao, W., Guo, M., Zhu, L., Chen, T., Wang, J., et al. (2022). Cerebral organoids for modeling of HSV-1-induced-amyloid beta associated neuropathology and phenotypic rescue. Int. J. Mol. Sci. 23:178. doi: 10.1101/2022.02.11.480178
Qiu, S., Qiu, Y., Li, Y., and Cong, X. (2022). Genetics of autism spectrum disorder: an umbrella review of systematic reviews and meta-analyses. Transl. Psychiatry 12:249. doi: 10.1038/s41398-022-02009-6
Rabeling, A., and Goolam, M. (2022). Cerebral organoids as an in vitro model to study autism spectrum disorders. Gene Ther.12, 1–16. doi: 10.1038/s41434-022-00356-z
Raja, W. K., Mungenast, A. E., Lin, Y. T., Ko, T., Abdurrob, F., Seo, J., et al. (2016). Self-Organizing 3D human neural tissue derived from induced pluripotent stem cells recapitulate Alzheimer's disease phenotypes. PLoS ONE 11:e0161969. doi: 10.1371/journal.pone.0161969
Ramani, A., Muller, L., Ostermann, P. N., Gabriel, E., Abida-Islam, P., Muller-Schiffmann, A., et al. (2020). SARS-CoV-2 targets neurons of 3D human brain organoids. EMBO J. 39:e106230. doi: 10.15252/embj.2020106230
Renner, H., Grabos, M., Becker, K. J., Kagermeier, T. E., Wu, J., Otto, M., et al. (2020). A fully automated high-throughput workflow for 3D-based chemical screening in human midbrain organoids. Elife 9:904. doi: 10.7554/eLife.52904.sa2
Ryan, L. J., Tepper, J. M., Sawyer, S. F., Young, S. J., and Groves, P. M. (1985). Autoreceptor activation in central monoamine neurons: modulation of neurotransmitter release is not mediated by intermittent axonal conduction. Neuroscience 15, 925–931. doi: 10.1016/0306-4522(85)90243-X
Ryu, S., Weber, C., Chu, P. H., Ernest, B., Jovanovic, V. M., Deng, T., et al. (2023). Stress-free cell aggregation by using the CEPT cocktail enhances embryoid body and organoid fitness. Biofabrication 16:13. doi: 10.1088/1758-5090/ad0d13
Samarasinghe, R. A., Miranda, O. A., Buth, J. E., Mitchell, S., Ferando, I., Watanabe, M., et al. (2021). Identification of neural oscillations and epileptiform changes in human brain organoids. Nat. Neurosci. 24, 1488–1500. doi: 10.1038/s41593-021-00906-5
Santos, J. L. S., Araujo, C. A., Rocha, C. G., Costa-Ferro, Z. S. M., and Souza, B. S. F. (2023). Modeling autism spectrum disorders with induced pluripotent stem cell-derived brain organoids. Biomolecules 13:260. doi: 10.3390/biom13020260
Schafer, S. T., Mansour, A. A., Schlachetzki, J. C. M., Pena, M., Ghassemzadeh, S., Mitchell, L., et al. (2023). An in vivo neuroimmune organoid model to study human microglia phenotypes. Cell 186, 2111–2126. e2120. doi: 10.1016/j.cell.2023.04.022
Schlieve, C. R., Fowler, K. L., Thornton, M., Huang, S., Hajjali, I., Hou, X., et al. (2017). Neural crest cell implantation restores enteric nervous system function and alters the gastrointestinal transcriptome in human tissue-engineered small intestine. Stem Cell Rep. 9, 883–896. doi: 10.1016/j.stemcr.2017.07.017
Schmidt, S. (2021). Autism in three dimensions: using brain organoids to study potential gene-environment interactions. Environ. Health Perspect. 129:104003. doi: 10.1289/EHP10301
Schwamborn, J. C. (2018). Is Parkinson's disease a neurodevelopmental disorder and will brain organoids help us to understand it? Stem Cells Dev. 27, 968–975. doi: 10.1089/scd.2017.0289
Sebastian, R., Pavon, N. S., Song, Y., Diep, K. T., and Pak, C. (2023). Method to generate dorsal forebrain brain organoids from human pluripotent stem cells. Methods Mol. Biol. 2683, 169–183. doi: 10.1007/978-1-0716-3287-1_13
Shannon, J. M., Mason, R. J., and Jennings, S. D. (1987). Functional differentiation of alveolar type II epithelial cells in vitro: effects of cell shape, cell-matrix interactions and cell-cell interactions. Biochim. Biophys. Acta 931, 143–156. doi: 10.1016/0167-4889(87)90200-X
Shi, Y., Sun, L., Wang, M., Liu, J., Zhong, S., Li, R., et al. (2020). Vascularized human cortical organoids (vOrganoids) model cortical development in vivo. PLoS Biol. 18:e3000705. doi: 10.1371/journal.pbio.3000705
Silbereis, J. C., Pochareddy, S., Zhu, Y., Li, M., and Sestan, N. (2016). The cellular and molecular landscapes of the developing human central nervous system. Neuron 89, 248–268. doi: 10.1016/j.neuron.2015.12.008
Silva, T. P., Fernandes, T. G., Nogueira, D. E. S., Rodrigues, C. V., Bekman, E. P., Hashimura, Y., et al. (2020). Scalable generation of mature cerebellar organoids from human pluripotent stem cells and characterization by immunostaining. J. Vis. Exp. 160:e61143. doi: 10.3791/61143
Simsa, R., Rothenbucher, T., Gurbuz, H., Ghosheh, N., Emneus, J., Jenndahl, L., et al. (2021). Brain organoid formation on decellularized porcine brain ECM hydrogels. PLoS ONE 16:e0245685. doi: 10.1371/journal.pone.0245685
Sloan, S. A., Andersen, J., Pasca, A. M., Birey, F., and Pasca, S. P. (2018). Generation and assembly of human brain region-specific three-dimensional cultures. Nat. Protoc. 13, 2062–2085. doi: 10.1038/s41596-018-0032-7
Smith, B. L. (2021). Improving translational relevance: the need for combined exposure models for studying prenatal adversity. Brain Behav. Immun. Health 16, 100294. doi: 10.1016/j.bbih.2021.100294
Sperling, R. A., Aisen, P. S., Beckett, L. A., Bennett, D. A., Craft, S., Fagan, A. M., et al. (2011). Toward defining the preclinical stages of Alzheimer's disease: recommendations from the National Institute on Aging-Alzheimer's Association workgroups on diagnostic guidelines for Alzheimer's disease. Alzheimers. Dement. 7, 280–292. doi: 10.1016/j.jalz.2011.03.003
Sullivan, J. M., Rubeis, D. e, and Schaefer, S. (2019). Convergence of spectrums: neuronal gene network states in autism spectrum disorder. Curr. Opin. Neurobiol. 59, 102–111. doi: 10.1016/j.conb.2019.04.011
Sun, X., Kofman, S., Ogbolu, V. C., Karch, C. M., Ibric, L., Qiang, L., et al. (2023). Vascularized brain assembloids with enhanced cellular complexity provide insights into the cellular deficits of tauopathy. Stem Cells. 42, 107–115. doi: 10.1101/2023.06.30.547293
Sun, X. Y., Ju, X. C., Li, Y., Zeng, P. M., Wu, J., Zhou, Y. Y., et al. (2022). Generation of vascularized brain organoids to study neurovascular interactions. Elife 11:76707. doi: 10.7554/eLife.76707
Takla, T. N., Luo, J., Sudyk, R., Huang, J., Walker, J. C., Vora, N. L., et al. (2023). A shared pathogenic mechanism for valproic acid and SHROOM3 knockout in a brain organoid model of neural tube defects. Cells 12:697. doi: 10.3390/cells12131697
Tanaka, Y., Cakir, B., Xiang, Y., Sullivan, G. J., and Park, I. H. (2020). Synthetic analyses of single-cell transcriptomes from multiple brain organoids and fetal brain. Cell Rep. 30, 1682–1689. e1683. doi: 10.1016/j.celrep.2020.01.038
Tanaka, Y., and Park, I. H. (2021). Regional specification and complementation with non-neuroectodermal cells in human brain organoids. J. Mol. Med. 99, 489–500. doi: 10.1007/s00109-021-02051-9
Tang, X., Wang, Z., Khutsishvili, D., Cheng, Y., Wang, J., Tang, J., et al. (2023). Volumetric compression by heterogeneous scaffold embedding promotes cerebral organoid maturation and does not impede growth. Cell Syst. 14, 872–882. e873. doi: 10.1016/j.cels.2023.09.004
Tidball, A. M., Niu, W., Ma, Q., Takla, T. N., Walker, J. C., Margolis, J. L., et al. (2023). Deriving early single-rosette brain organoids from human pluripotent stem cells. Stem Cell Rep. 18, 2498–2514. doi: 10.1016/j.stemcr.2023.10.020
Tong, C. K., and Vidyadaran, S. (2016). Role of microglia in embryonic neurogenesis. Exp. Biol. Med. 241, 1669–1675. doi: 10.1177/1535370216664430
Trevino, A. E., Sinnott-Armstrong, N., Andersen, J., Yoon, S. J., Huber, N., Pritchard, J. K., et al. (2020). Chromatin accessibility dynamics in a model of human forebrain development. Science 367:1645. doi: 10.1126/science.aay1645
Trujillo, C. A., Gao, R., Negraes, P. D., Gu, J., Buchanan, J., Preissl, S., et al. (2019). Complex oscillatory waves emerging from cortical organoids model early human brain network development. Cell Stem Cell 25, 558–569. doi: 10.1016/j.stem.2019.08.002
Valiulahi, P., Vidyawan, V., Puspita, L., Oh, Y., Juwono, V. B., Sittipo, P., et al. (2021). Generation of caudal-type serotonin neurons and hindbrain-fate organoids from hPSCs. Stem Cell Rep. 16, 1938–1952. doi: 10.1016/j.stemcr.2021.06.006
Vertesy, A., Eichmuller, O. L., Naas, J., Novatchkova, M., Esk, C., Balmana, M., et al. (2022). Gruffi: an algorithm for computational removal of stressed cells from brain organoid transcriptomic datasets. EMBO J. 41:e111118. doi: 10.15252/embj.2022111118
Wang, M., Gage, F. H., and Schafer, S. T. (2023). Transplantation strategies to enhance maturity and cellular complexity in brain organoids. Biol. Psychiatry 93, 616–621. doi: 10.1016/j.biopsych.2023.01.004
Wang, Y., Chiola, S., Yang, G., Russell, C., Armstrong, C. J., Wu, Y., et al. (2022). Modeling human telencephalic development and autism-associated SHANK3 deficiency using organoids generated from single neural rosettes. Nat. Commun. 13, 5688. doi: 10.1038/s41467-022-33364-z
Wang, Z., Wang, S. N., Xu, T. Y., Hong, C., Cheng, M. H., Zhu, P. X., et al. (2020). Cerebral organoids transplantation improves neurological motor function in rat brain injury. CNS Neurosci. Ther. 26, 682–697. doi: 10.1111/cns.13286
Watanabe, M., Buth, J. E., Vishlaghi, N., De La Torre-Ubieta, L., Taxidis, J., Khakh, B. S., et al. (2017). Self-organized cerebral organoids with human-specific features predict effective drugs to combat zika virus infection. Cell Rep. 21, 517–532. doi: 10.1016/j.celrep.2017.09.047
Wen, Y., Alshikho, M. J., and Herbert, M. R. (2016). Pathway network analyses for autism reveal multisystem involvement, major overlaps with other diseases and convergence upon MAPK and calcium signaling. PLoS ONE 11:e0153329. doi: 10.1371/journal.pone.0153329
Xiang, Y., Cakir, B., and Park, I. H. (2020). Generation of regionally specified human brain organoids resembling thalamus development. STAR Protoc. 1:100001. doi: 10.1016/j.xpro.2019.100001
Xiang, Y., Tanaka, Y., Cakir, B., Patterson, B., Kim, K. Y., Sun, P., et al. (2019). hESC-derived thalamic organoids form reciprocal projections when fused with cortical organoids. Cell Stem Cell 24, 487–497. doi: 10.1016/j.stem.2018.12.015
Xu, J., and Wen, Z. (2021). Brain organoids: studying human brain development and diseases in a dish. Stem Cells Int. 2021:5902824. doi: 10.1155/2021/5902824
Xu, L., He, D., and Bai, Y. (2016). Microglia-mediated inflammation and neurodegenerative disease. Mol. Neurobiol. 53, 6709–6715. doi: 10.1007/s12035-015-9593-4
Xu, R., Boreland, A. J., Li, X., Erickson, C., Jin, M., Atkins, C., et al. (2021). Developing human pluripotent stem cell-based cerebral organoids with a controllable microglia ratio for modeling brain development and pathology. Stem Cell Rep. 16, 1923–1937. doi: 10.1016/j.stemcr.2021.06.011
Yanakiev, M., Soper, O., Berg, D. A., and Kang, E. (2022). Modelling Alzheimer's disease using human brain organoids: current progress and challenges. Expert Rev. Mol. Med. 25:e3. doi: 10.1017/erm.2022.40
Yao, H., Hu, D., Wang, J., Wu, W., Zhao, H. H., Wang, L., et al. (2023). Buprenorphine and methadone differentially alter early brain development in human cortical organoids. Neuropharmacology 239:109683. doi: 10.1016/j.neuropharm.2023.109683
Yao, H., Wu, W., Cerf, I., Zhao, H. W., Wang, J., Negraes, P. D., et al. (2020). Methadone interrupts neural growth and function in human cortical organoids. Stem Cell Res. 49, 102065. doi: 10.1016/j.scr.2020.102065
Zhang, D., Eguchi, N., Okazaki, S., Sora, I., and Hishimoto, A. (2023). Telencephalon organoids derived from an individual with ADHD show altered neurodevelopment of early cortical layer structure. Stem Cell Rev. Rep. 19, 1482–1491. doi: 10.1007/s12015-023-10519-z
Zhang, W., Jiang, J., Xu, Z., Yan, H., Tang, B., Liu, C., et al. (2023). Microglia-containing human brain organoids for the study of brain development and pathology. Mol. Psychiatry 28, 96–107. doi: 10.1038/s41380-022-01892-1
Zhang, Z., O'laughlin, R., Song, H., and Ming, G. L. (2022). Patterning of brain organoids derived from human pluripotent stem cells. Curr. Opin. Neurobiol. 74:102536. doi: 10.1016/j.conb.2022.102536
Keywords: cerebral organoid, protocol, limitations, stem cell, human diseases
Citation: Zhao HH and Haddad G (2024) Brain organoid protocols and limitations. Front. Cell. Neurosci. 18:1351734. doi: 10.3389/fncel.2024.1351734
Received: 06 December 2023; Accepted: 19 February 2024;
Published: 20 March 2024.
Edited by:
Dirk M. Hermann, University of Duisburg-Essen, GermanyReviewed by:
Eunchai Kang, University of Aberdeen, United KingdomCopyright © 2024 Zhao and Haddad. This is an open-access article distributed under the terms of the Creative Commons Attribution License (CC BY). The use, distribution or reproduction in other forums is permitted, provided the original author(s) and the copyright owner(s) are credited and that the original publication in this journal is cited, in accordance with accepted academic practice. No use, distribution or reproduction is permitted which does not comply with these terms.
*Correspondence: Gabriel Haddad, Z2hhZGRhZEBoZWFsdGgudWNzZC5lZHU=
Disclaimer: All claims expressed in this article are solely those of the authors and do not necessarily represent those of their affiliated organizations, or those of the publisher, the editors and the reviewers. Any product that may be evaluated in this article or claim that may be made by its manufacturer is not guaranteed or endorsed by the publisher.
Research integrity at Frontiers
Learn more about the work of our research integrity team to safeguard the quality of each article we publish.