Corrigendum: What can the common fruit fly teach us about stroke?: lessons learned from the hypoxic tolerant Drosophila melanogaster
- 1Department of Psychology, Westfield State University, Westfield, MA, United States
- 2Department of Biology, Eastern Connecticut State University, Willimantic, CT, United States
- 3Department of Biology, Westfield State University, Westfield, MA, United States
Stroke, resulting in hypoxia and glucose deprivation, is a leading cause of death and disability worldwide. Presently, there are no treatments that reduce neuronal damage and preserve function aside from tissue plasminogen activator administration and rehabilitation therapy. Interestingly, Drosophila melanogaster, the common fruit fly, demonstrates robust hypoxic tolerance, characterized by minimal effects on survival and motor function following systemic hypoxia. Due to its organized brain, conserved neurotransmitter systems, and genetic similarity to humans and other mammals, uncovering the mechanisms of Drosophila’s tolerance could be a promising approach for the development of new therapeutics. Interestingly, a key facet of hypoxic tolerance in Drosophila is organism-wide metabolic suppression, a response involving multiple genes and pathways. Specifically, studies have demonstrated that pathways associated with oxidative stress, insulin, hypoxia-inducible factors, NFκB, Wnt, Hippo, and Notch, all potentially contribute to Drosophila hypoxic tolerance. While manipulating the oxidative stress response and insulin signaling pathway has similar outcomes in Drosophila hypoxia and the mammalian middle cerebral artery occlusion (MCAO) model of ischemia, effects of Notch pathway manipulation differ between Drosophila and mammals. Additional research is warranted to further explore how other pathways implicated in hypoxic tolerance in Drosophila, such as NFκB, and Hippo, may be utilized to benefit mammalian response to ischemia. Together, these studies demonstrate that exploration of the hypoxic response in Drosophila may lead to new avenues of research for stroke treatment in humans.
1 Introduction
Stroke, or cerebral ischemia, is a leading cause of death and disability worldwide. In the United States, over 795,000 strokes occur each year and the death rate is 41.1 per 100,000 people. Most strokes are ischemic strokes, characterized by blood clots that restrict blood flow to the brain (Center for Disease Control and Prevention, 2023). This results in loss of glucose and diminished oxygen, or hypoxia, to the brain. With ischemic strokes, the first line of defense is tissue plasminogen activator (TPA) administration, which breaks up blood clots and restores blood flow, a process known as reperfusion that re-introduces tissue to oxygen and glucose. While TPA treatment has a positive effect on stroke outcome (Prabhakaran et al., 2015), reperfusion increases reactive oxygen species, leading to a cascade of cellular responses that causes additional injury (Zhang et al., 2022). While thousands of clinical trials for stroke have been completed, none have led to significant advances in treatment (Ward and Carmichael, 2020). Furthermore, most preclinical research on ischemia uses the rodent middle cerebral artery occlusion (MCAO) model. MCAO closely mimics the physical attributes of human ischemic stroke, but results in high variability in infarct size and mortality (Ström et al., 2013). These limitations offer opportunities for alternative models to identify new therapeutic strategies.
Exploring hypoxic tolerant species like Drosophila melanogaster may be a suitable alternative (Del Río and Montaner, 2021). Drosophila are a commonly used model in neuroscience due to their centralized brain and shared neurotransmitter systems with mammals. Drosophila behavior can be easily quantified to assess disease induction and potential treatments. In addition, flies have a short lifespan and high numbers of progeny, making it feasible to examine the effects of aging on neurological disease with large sample sizes. Most importantly, flies exhibit substantial genetic similarity to humans with many conserved genes contributing to hypoxic responses (Zhou and Haddad, 2013, Table 1). While many transgenic mouse models exist, flies can be genetically manipulated more efficiently using the GAL4/UAS system to induce or suppress any gene under a specific promoter via defined temperatures or stages of development (Yamaguchi and Yoshida, 2018). This is an advantage over transgenic mice, which may initiate compensatory mechanisms during development, clouding interpretation of the results (Kreiner, 2015). Thus, Drosophila represent an ideal model to study genetic mechanisms that are difficult, if not impossible, to dissect in mammals.
In this review, we discuss recent findings exploring the mechanisms behind hypoxic tolerance in Drosophila and how those mechanisms may help with the development of human therapeutics. We first introduce how stroke is modeled in flies and potential mechanisms that may contribute to hypoxic tolerance. We then discuss research exploring hypoxic-responsive pathways in flies (Figure 1) and how those findings compare to outcomes using the MCAO model of stroke.
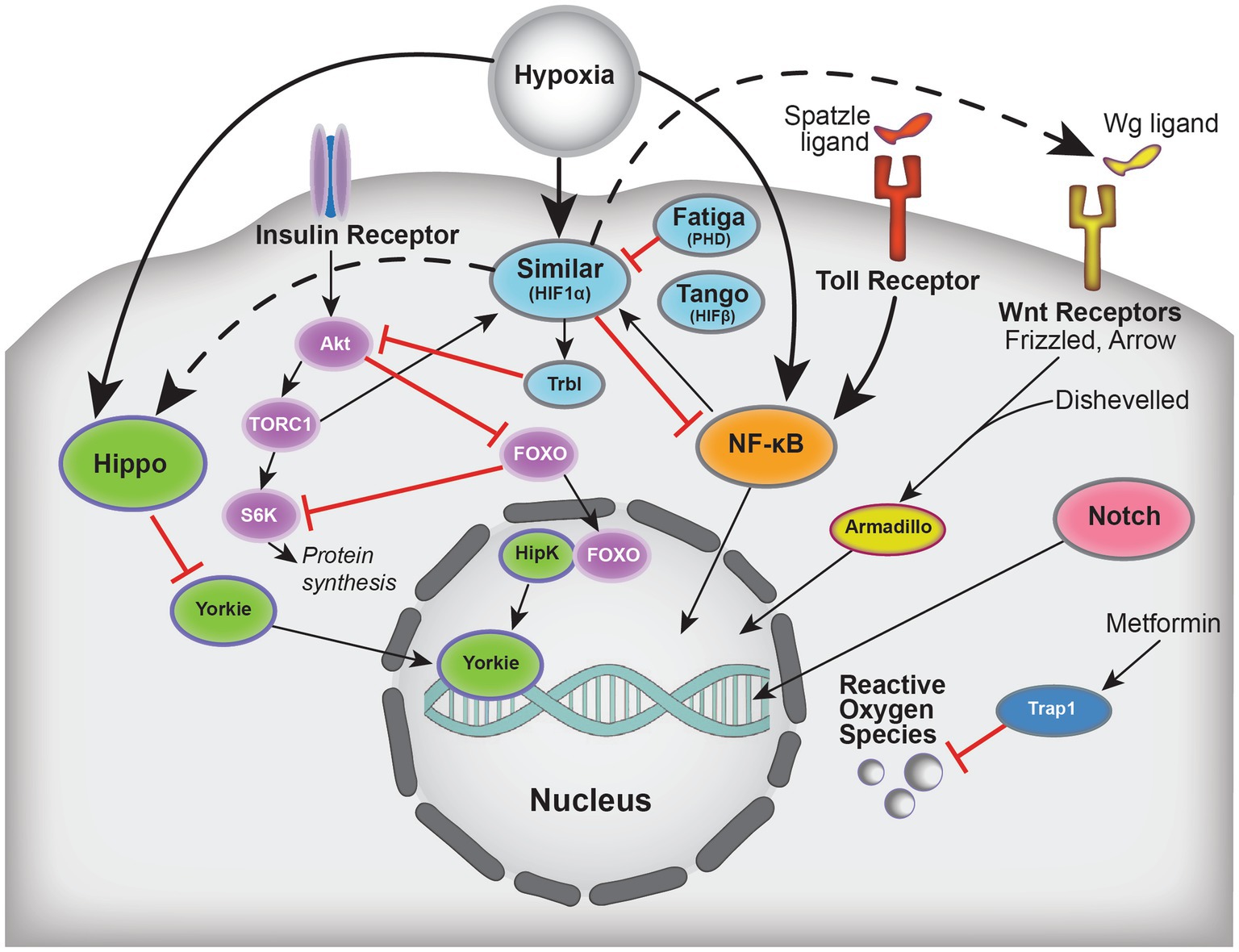
Figure 1. Schematic summarizing select players implicated in hypoxic tolerance and the crosstalk between these pathways, which include Hippo, Insulin, HIF (Drosophila Similar, Tango, Fatiga), NFκB, Wnt, Notch, and oxidative stress. Solid arrows indicate activation, dashed arrows indicate regulation, and blunted red lines represent inhibition.
2 Modeling stroke-induced hypoxia in Drosophila melanogaster
Drosophila have an open circulatory system where a combined blood and interstitial fluid, hemolymph, fills the body cavity and is pushed by the heart (Romero et al., 2007). Because Drosophila lack blood vessels, ischemic stroke cannot be achieved by blood vessel occlusion. Thus, stroke-induced hypoxia is often modeled by exposing the organism to low-(hypoxia) or no-(anoxia) oxygen conditions (Jung et al., 2022).
Following hypoxic or anoxic conditions, flies are monitored for survival for several hours to days, and demonstrate temporary immobility and lethargy, known as stupor. Both time to recover from stupor (Ma and Haddad, 1999) and climbing ability in the negative geotaxis assay (Kokott-Vuong et al., 2021) are commonly used to assess hypoxic response. Larval hypoxic tolerance is measured by rate of eclosion, or emergence of the adult from the pupa (Gersten et al., 2014).
While larvae can survive hypoxia for their entire larval stage with no impact on eclosion rate, hypoxia leads to decreased body size and shortened lifespan (Polan et al., 2020). Adult flies can also survive long periods of hypoxia and anoxia [reviewed in Haddad et al. (1997a,b); Habib et al. (2021)]. Because Drosophila can persist in hypoxic environments, they likely possess numerous adaptations that facilitate this behavior as discussed in detail below.
3 Adaptations by Drosophila in response to hypoxia
A major contributor to anoxia tolerance is whole-body metabolic suppression (Deliu et al., 2017; Habib et al., 2021). Sensors within the fat body of Drosophila, an organ that stores energy and regulates metabolic function (Arrese and Soulages, 2010), detect hypoxic conditions and transmit that information throughout the body to alter metabolic rate (Texada et al., 2019; Noguchi et al., 2022). Recent work demonstrated that survival in anoxic conditions was correlated with decreased protein, ATP, and anaerobic end products in adult versus larval flies, suggesting enhanced metabolic suppression in adults (Campbell et al., 2018, 2019a). Furthermore, reducing metabolic function by exposure to lower temperatures significantly improves survival and decreases reactive oxygen species (ROS) production following hypoxia (Habib et al., 2021).
Genome-wide analyses have identified genes that may play a role in hypoxic tolerance, including metabolic suppression (Gersten et al., 2014). Furthermore, the severity of hypoxia (mild vs. strong or acute vs. chronic) dictates which genes are expressed (Liu et al., 2006). Campbell et al. (2019b) identified several genes that may facilitate adaptation to hypoxia as RNAi knockdown of these genes reduced survival following hypoxia. Although flies exhibit some unique responses to hypoxia, contributing to their tolerance, they also employ numerous mechanisms that are shared with mammals.
4 Studies in flies confirming shared mechanisms with mammals
4.1 Oxidative stress responses
The role of oxidative stress in Drosophila hypoxia tolerance has been explored and reviewed in Zhou et al. (2011), Zhou and Haddad (2013). Unlike mammals, flies do not exhibit a significant increase in metabolic activity or ROS production following re-exposure to oxygen (reperfusion). However, flies expressing a mutant version of TRAP1 (Table 1), a mitochondrial chaperone belonging to the heat shock protein (HSP) 90 family that inhibits ROS accumulation, demonstrate increased metabolic activity and ROS production after hypoxia which results in decreased survival and motor recovery (Kokott-Vuong et al., 2021). TRAP1 mutant phenotypes are rescued with the antidiabetic and antioxidant drug metformin (Nasri and Rafieian-Kopaei, 2014; Kokott-Vuong et al., 2021). Similarly, decreased infarct size with metformin treatment was observed in the rodent MCAO model (Arbeláez-Quintero and Palacios, 2017) and humans taking metformin for type-2 diabetes have a more favorable outcome following ischemia (Jia et al., 2015).
In addition to TRAP1, other HSPs influence oxidative stress. Specifically, Hsp70 mRNA levels increase during reperfusion following hypoxia in both Drosophila and mammals (Habib et al., 2021; Kokott-Vuong et al., 2021) and may contribute to oxidative stress resistance. Flies overexpressing Hsp70 and 23 experience greater survival in hypoxic conditions (Zhou and Haddad, 2013). Similarly, pharmacological and genetic induction of Hsp70 after MCAO is protective in rodents (Kim et al., 2018). Given the similarities between oxidative stress functions in ischemia and hypoxic tolerance in Drosophila, further exploration into these pathways may reveal promising therapies for ischemia and stroke.
4.2 Insulin
Several members of the insulin signaling pathway have been implicated in hypoxic tolerance in Drosophila, likely acting through the fat body (Table 1). As mentioned above, the Drosophila fat body plays a major role in hypoxic detection and subsequent metabolic suppression (Texada et al., 2019; Noguchi et al., 2022). As a result of this metabolic suppression following hypoxia, larvae exhibit a significant decrease in growth and body size that contributes to their ability to survive hypoxic conditions. Dilp2, a ligand for the Drosophila insulin receptor, accumulates in insulin-producing cells after hypoxia, resulting in a decrease in Dilp2 release and subsequent insulin receptor activation. Overexpression of the insulin receptor reverses this phenotype, suggesting that decreased insulin signaling following hypoxia plays a role in larval growth inhibition (Wong et al., 2014).
Insulin receptor ligand binding results in activation of the TORC1 complex that in turn activates the ribosomal protein S6K. Contrary to mammalian cells where induction of mTOR and its effectors seem to reduce protein synthesis as an adaptation to hypoxic conditions, in Drosophila, downregulation of this pathway appears to be important in the Drosophila hypoxia response (Zhou and Haddad, 2013). For example, suppression of TORC1 in adipose tissue contributes to hypoxia adaptation in larvae by controlling body growth (Lee et al., 2019) while TORC1 induction decreases eclosion rates during hypoxic conditions. Overexpression of S6K significantly reduces survival in hypoxic conditions (Lee et al., 2019). Similarly, inhibiting S6K in mouse MCAO decreases ischemic infarct size (Chi et al., 2019).
FOXO, a transcription factor inhibited by insulin pathway activation, mediates tolerance to 5% hypoxia in both adult and larval flies reared in those conditions (Barretto et al., 2020). Adult foxo mutants experience significantly decreased survival when exposed to severe hypoxia. In mammals, increasing FOXO3 using adenovirus in rats (Zhou et al., 2019) and inhibition of miRNA targets of FOXO in mice (Yan et al., 2020) are protective against MCAO. Given the similarities in the insulin system between mammals and Drosophila and that insulin inhibition appears to contribute to hypoxic tolerance, additional research into insulin signaling may reveal potential targets for ischemic therapies.
5 Discoveries in flies warranting further exploration in mammals
5.1 Hypoxia-inducible factors
Hypoxia inducible factors (HIFs) mediate Drosophila’s response to hypoxia (Table 1; Dekanty et al., 2005). There are several HIF human homologs in Drosophila including Sima (similar, HIF1α homolog); Tgo (tango, HIFβ homolog) and Fatiga (fatiga, PHD homolog). Tgo is constitutively expressed regardless of oxygen conditions, while sima expression only occurs following hypoxic conditions (Romero et al., 2007). The HIF pathway in Drosophila has been previously reviewed (Gorr et al., 2010; Zhou and Haddad, 2013) and different Drosophila HIF homologs appear to play distinct roles in hypoxia (Misra et al., 2017; Baccino-Calace et al., 2020).
Sima regulation by insulin and TOR pathways is conserved (Romero et al., 2007), and NF-κB (Bandarra et al., 2014) regulates sima induction. Sima activation seems necessary for hypoxia adaptation as overexpression of sima triggers the inhibition of fat body growth, a critical event in hypoxia tolerance, via activation of Trbl and subsequent inhibition of Akt signaling (Noguchi et al., 2022). In Drosophila, null mutants of sima result in larvae that cannot adapt to hypoxia (Centanin et al., 2005) and cardiac problems in adult flies following acute hypoxia (Zarndt et al., 2015). Interestingly, in adults, the loss-of-function sima mutant does not show differences in survival after anoxia compared to controls (Vigne et al., 2009), suggesting a more pivotal role of sima in larvae than adults. In mammals, HIF function is not consistent, as contradictory results using the MCAO model were observed (reviewed in He et al., 2021).
Loss-of-function fatiga mutants have high lethality in normoxic conditions, which is reversed if sima function is also lost (Centanin et al., 2005). In the MCAO model, inhibiting PHDs, which degrade HIF in normoxic conditions, decreases damage (reviewed in Davis et al., 2019) but this neuroprotection may be independent of HIF1α (Li et al., 2019). Lastly, tgo (Tango) seems important in tracheal development (Emmons et al., 1999) but loss-of-function tgo mutants have not been examined in response to hypoxia. Similarly, no publications directly manipulating HIF1β in mammalian models of ischemia exist. While HIF1α function in mammalian stroke has been investigated, studies investigating the role of HIFβ subunits are necessary as they might be valuable targets for future stroke treatments.
5.2 NF-κB
In Drosophila, pathogens trigger the immune response, which is mediated by the NF-κB system (Minakhina and Steward, 2006). NF-κB is released from sequestration by IKK (inhibitor of kB protein kinase), allowing it to bind to promoters of effector genes (Barretto et al., 2020). The cascade involving the release of NF-κB is triggered by the activation of the Toll receptor bound with the Spatzle ligand (Valanne et al., 2011). Although the Toll receptor system in hypoxia has not been examined in Drosophila, toll-receptor 4 expression in endothelial cells decreases following hypoxia, a response mediated by the presence of ROS (Ishida et al., 2002). In support of this finding, inhibiting Toll-like receptor 4 attenuated several hypoxic–ischemic injuries within the brains of neonatal rats (Zhu et al., 2021). Specific subunits of NF-κB, dorsal (p65 homolog), relish (p50/p52 homolog) and dif (p65 homolog), are activated in both adults and larvae following 24 h of hypoxia (Table 1; Bandarra et al., 2014).
Non-specific pharmacological inhibition of NF-κB protects against MCAO damage in mammals (reviewed in Harari and Liao, 2010). Interestingly, studies of MCAO induction in mice lacking p50 demonstrate contradicting data showing both increased and decreased infarct size (Harari and Liao, 2010), suggesting a complicated interplay between factors of the NF-κB signaling pathway in mammals. Currently, few studies exist in Drosophila investigating components of the NF-κB pathway during hypoxia. However, one study using a Drosophila relish mutant observed decreased survival following hypoxia compared to wild type controls (Barretto et al., 2020), supporting MCAO findings in p50 knockout mice (Li et al., 2008). More studies manipulating components of this pathway or tissue-specific expression of Toll-like receptors need to be conducted to elucidate their role in hypoxic tolerance.
5.3 Wnt
The canonical Wnt pathway is highly conserved, playing a role in disease and several developmental events. The pathway is activated following binding of the glycoprotein Wingless (Wg) to the transmembrane receptors Frizzled (Fz) and Arrow (Arr). Once activated by Wg, Fz and Arr recruit the intracellular protein, Dishevelled (Dsh) which, together with other proteins, suppress the activation of a destruction complex, ultimately resulting in the translocation of the transcription factor, Armadillo (arm) to the nucleus and initiation of gene expression (Komiya and Habas, 2008; Bejsovec, 2013).
In Drosophila bred to tolerate hypoxic conditions, polymorphisms of Wnt pathway members may contribute to this generational tolerance (Gersten et al., 2014). Furthermore, a p-element screen revealed several Wnt-related genes as pivotal in hypoxic tolerance (Azad et al., 2012). Indeed, neuron-specific overexpression of Wnt pathway signaling increased rates of eclosion and knocking them down decreased rates of eclosion (Gersten et al., 2014). Likewise, activation of Wnt signaling in mammalian models of stroke seems promising (reviewed in Mo et al., 2022).
Sima (HIF1α homolog) triggers the production of Wg (the Wnt pathway ligand, Table 1) to facilitate Wnt signaling in neurons, suggesting Wnt signaling underlies hypoxic tolerance (Chen et al., 2019). However, this research was conducted with trachealess mutants and not with hypoxic conditions. Supporting the involvement of Wnt in hypoxic tolerance, a down-stream factor of Wnt activation, the Swim protein, is upregulated following adult and larval hypoxia and induces stem cell proliferation in brain injury (Simões et al., 2022). Further exploration of Wnt signaling, especially in adult Drosophila is warranted to understand the contribution of this pathway in hypoxic tolerance as current research is primarily in larva.
5.4 Hippo
The Hippo pathway has Drosophila and mammalian homologs and has been reviewed by Fu et al. (2022) (Table 1). This pathway is activated by stress signals, including hypoxia. There is also opportunity for crosstalk between this pathway and others that are activated during hypoxia, like HIFα and insulin (Fu et al., 2022). A member of the Hippo pathway, homeodomain interacting protein kinase (Hipk), phosphorylates Yorkie, a downstream effector within the pathway, which then translocates to the nucleus to regulate transcription of effector genes (Snigdha et al., 2019; Steinmetz et al., 2021). In the context of hypoxia, Hipk binds to FOXO and regulates low oxygen survival. Indeed, 1% oxygen conditions increased expression of Hipk and other Hippo members, and fewer Hipk knock-down flies survived these conditions (Ding et al., 2022). Moreover, degradation of Hipk using F-box protein 3 in rat MCAO significantly increases infarct size (Gao et al., 2022). This suggests that activation of the Hippo pathway may be a promising target for stroke treatment and additional studies into this pathway are needed.
5.5 Notch
Involvement of the Notch signaling pathway in hypoxic tolerance has been reviewed extensively for Drosophila (Table 1; Zhou and Haddad, 2013). Notch is a transmembrane protein which, following proteolytic cleavage releases its intracellular domain which regulates transcription (Bray, 2006). Flies with loss-of-function mutations or RNAi-mediated knockdown of Notch are extremely sensitive to low levels of oxygen. Conversely, those with gain-of-function mutations are highly resistant to hypoxia (Zhou et al., 2011). Absence of Notch in excitatory amino acid transporter-positive glial cells decreases eclosion rates under hypoxic conditions, suggesting a possible mechanism for the tolerance (Zhou et al., 2021). Furthermore, Notch signaling elements interact with HIF pathways to regulate hypoxic adaptation, including high altitude adaptation in human populations (O'Brien et al., 2022).
While the Notch signaling pathway appears to be protective in Drosophila hypoxia, this is one instance where the findings in Drosophila are not corroborated by mammalian research. The role of Notch in ischemia has been reviewed (Arumugam et al., 2018) and it appears to be a pro-apoptotic factor, contributing to neuronal death following stroke. Lipoxin A4, an anti-inflammatory agent (Li et al., 2021), has positive effects on stroke and seems to work by suppressing the actions of Notch. Conversely, administration of osthole reduced cerebral infarct following MCAO in rats through activation of the Notch pathway (Guan et al., 2017). Whatever difference exist in these pathways, it appears that the actions of Notch in mammalian models of stroke are not as clear as those observed in Drosophila hypoxia, so further study of Notch in hypoxic tolerance may not be beneficial.
6 Discussion
Drosophila melanogaster is a promising model to study hypoxia toward the goal of identifying stroke-related treatments. Drosophila demonstrate robust hypoxic tolerance and highly conserved genetic pathways with mammals. Notably, pathways involved in oxidative stress and insulin signaling have similar effects in Drosophila and mammals, suggesting further exploration of these pathways in models of stroke are warranted. Of the pathways we explored in this review, NF-κB and Hippo appear to be the most understudied in mammals, and in the case of Hippo, in Drosophila as well. Alterations in these pathways appear to influence/confer tolerance to hypoxia in Drosophila. Therefore, revealing the contributions of these pathways may help identify factors for the treatment of stroke. Due to the relative simplicity of genetic manipulation, short lifespan, and myriad behavioral assays, Drosophila are an untapped resource for screening potential targets/treatments for stroke in a cost-effective and efficient manner.
Author contributions
PQ-M: Conceptualization, Investigation, Writing – original draft, Writing – review & editing. KL: Writing – review & editing, Visualization. RW: Visualization, Writing – review & editing, Conceptualization, Investigation, Writing – original draft.
Funding
The author(s) declare that financial support was received for the research, authorship, and/or publication of this article. Publication fees were funded in part by the Connecticut State University American Association of University Professors (CSU-AAUP) Research Grant to KL.
Conflict of interest
The authors declare that the research was conducted in the absence of any commercial or financial relationships that could be construed as a potential conflict of interest.
The author(s) declared that they were an editorial board member of Frontiers, at the time of submission. This had no impact on the peer review process and the final decision.
Publisher’s note
All claims expressed in this article are solely those of the authors and do not necessarily represent those of their affiliated organizations, or those of the publisher, the editors and the reviewers. Any product that may be evaluated in this article, or claim that may be made by its manufacturer, is not guaranteed or endorsed by the publisher.
References
Arbeláez-Quintero, I., and Palacios, M. (2017). To use or not to use metformin in cerebral ischemia: a review of the application of metformin in stroke rodents. Stroke Res. Treat. 2017:9756429. doi: 10.1155/2017/9756429
Arrese, E. L., and Soulages, J. L. (2010). Insect fat body: energy, metabolism, and regulation. Annu. Rev. Entomol. 55, 207–225. doi: 10.1146/annurev-ento-112408-085356
Arumugam, T. V., Baik, S. H., Balaganapathy, P., Sobey, C. G., Mattson, M. P., and Jo, D. G. (2018). Notch signaling and neuronal death in stroke. Prog. Neurobiol. 165-167, 103–116. doi: 10.1016/j.pneurobio.2018.03.002
Azad, P., Zhou, D., Zarndt, R., and Haddad, G. G. (2012). Identification of genes underlying hypoxia tolerance in Drosophila by a P-element screen. G3 (Bethesda, Md.) 2, 1169–1178. doi: 10.1534/g3.112.003681
Baccino-Calace, M., Prieto, D., Cantera, R., and Egger, B. (2020). Compartment and cell-type specific hypoxia responses in the developing Drosophila brain. Biol. Open. 9:bio053629. doi: 10.1242/bio.053629
Bandarra, D., Biddlestone, J., Mudie, S., Muller, H. A., and Rocha, S. (2014). Hypoxia activates IKK-NF-κB and the immune response in Drosophila melanogaster. Biosci. Rep. 34:e00127. doi: 10.1042/BSR20140095
Barretto, E. C., Polan, D. M., Beevor-Potts, A. N., Lee, B., and Grewal, S. S. (2020). Tolerance to hypoxia is promoted by FOXO regulation of the innate immunity transcription factor NF-κB/relish in Drosophila. Genetics 215, 1013–1025. doi: 10.1534/genetics.120.303219
Bejsovec, A. (2013). Wingless/Wnt signaling in Drosophila: the pattern and the pathway. Mol. Reprod. Dev. 80, 882–894. doi: 10.1002/mrd.22228
Bray, S. J. (2006). Notch signaling: a simple pathway becomes complex. Nat. Rev. Mol. Cell Biol. 7, 678–689. doi: 10.1038/nrm2009
Campbell, J. B., Andersen, M. K., Overgaard, J., and Harrison, J. F. (2018). Paralytic hypo-energetic state facilitates anoxia tolerance despite ionic imbalance in adult Drosophila melanogaster. J. Exp. Biol. 221:jeb177147. doi: 10.1242/jeb.177147
Campbell, J. B., Overby, P. F., Gray, A. E., Smith, H. C., and Harrison, J. F. (2019b). Genome-wide association analysis of anoxia tolerance in drosophila melanogaster. G3 9, 2989–2999. doi: 10.1534/g3.119.400421
Campbell, J. B., Werkhoven, S., and Harrison, J. F. (2019a). Metabolomics of anoxia tolerance in Drosophila melanogaster: evidence against substrate limitation and for roles of protective metabolites and paralytic hypometabolism. Am. J. Physiol. Regul. Integr. Comp. Physiol. 317, R442–R450. doi: 10.1152/ajpregu.00389.2018
Centanin, L., Ratcliffe, P. J., and Wappner, P. (2005). Reversion of lethality and growth defects in Fatiga oxygen-sensor mutant flies by loss of hypoxia-inducible factor-alpha/Sima. EMBO Rep. 6, 1070–1075. doi: 10.1038/sj.embor.7400528
Chen, P. Y., Tsai, Y. W., Cheng, Y. J., Giangrande, A., and Chien, C. T. (2019). Glial response to hypoxia in mutants of NPAS1/3 homolog Trachealess through Wg signaling to modulate synaptic Bouton organization. PLoS Genet. 15:e1007980. doi: 10.1371/journal.pgen.1007980
Chi, O. Z., Kiss, G. K., Mellender, S. J., Liu, X., Liu, S., Jacinto, E., et al. (2019). Inhibition of p70 ribosomal S6 kinase 1 (S6K1) by PF-4708671 decreased infarct size in early cerebral ischemia-reperfusion with decreased BBB permeability. Eur. J. Pharm. 855, 202–207. doi: 10.1016/j.ejphar.2019.05.010
Davis, C. K., Jain, S. A., Bae, O. N., Majid, A., and Rajanikant, G. K. (2019). Hypoxia mimetic agents for ischemic stroke. Front. Cell Dev. Biol. 6:175. doi: 10.3389/fcell.2018.00175
Dekanty, A., Lavista-Llanos, S., Irisarri, M., Oldham, S., and Wappner, P. (2005). The insulin-PI3K/TOR pathway induces a HIF-dependent transcriptional response in Drosophila by promoting nuclear localization of HIF-alpha/Sima. J. Cell Sci. 118, 5431–5441. doi: 10.1242/jcs.02648
Del Río, C., and Montaner, J. (2021). Hypoxia tolerant species: the wisdom of nature translated into targets for stroke therapy. Int. J. Mol. Sci. 22:11131. doi: 10.3390/ijms222011131
Deliu, L. P., Ghosh, A., and Grewal, S. S. (2017). Investigation of protein synthesis in Drosophila larvae using puromycin labelling. Biol. Open. 6, 1229–1234. doi: 10.1242/bio.026294
Ding, K., Barretto, E. C., Johnston, M., Lee, B., Gallo, M., Grewal, S. S., et al. (2022). Transcriptome analysis of FOXO-dependent hypoxia gene expression identifies Hipk as a regulator of low oxygen tolerance in Drosophila. G3 (Bethesda, Md.). 12:jkac263. doi: 10.1093/g3journal/jkac263
Emmons, R. B., Duncan, D., Estes, P. A., Kiefel, P., Mosher, J. T., Sonnenfeld, M., et al. (1999). The spineless-aristapedia and tango bHLH-PAS proteins interact to control antennal and tarsal development in Drosophila. Development 126, 3937–3945. doi: 10.1242/dev.126.17.3937
Fu, M., Hu, Y., Lan, T., Guan, K. L., Luo, T., Luo, M., et al. (2022). The Hippo signalling pathway and its implications in human health and diseases. Signal Transduct. Targeted Ther. 7:376. doi: 10.1038/s41392-022-01191-9
Gao, Y., Xiao, X., Luo, J., Wang, J., Peng, Q., Zhao, J., et al. (2022). E3 ubiquitin ligase FBXO3 drives neuroinflammation to aggravate cerebral ischemia/reperfusion injury. Int. J. Mol. Sci. 23:13648. doi: 10.3390/ijms232113648
Gersten, M., Zhou, D., Azad, P., Haddad, G. G., and Subramaniam, S. (2014). Wnt pathway activation increases hypoxia tolerance during development. PLoS One 9:e103292. doi: 10.1371/journal.pone.0103292
Gorr, T. A., Wichmann, D., Hu, J., Hermes-Lima, M., Welker, A. F., Terwilliger, N., et al. (2010). Hypoxia tolerance in animals: biology and application. Physiol. Biochem. Zool. 83, 733–752. doi: 10.1086/648581
Guan, J., Wei, X., Qu, S., Lv, T., Fu, Q., and Yuan, Y. (2017). Osthole prevents cerebral ischemia-reperfusion injury via the notch signaling pathway. Biochem. Cell Biol. 95, 459–467. doi: 10.1139/bcb-2016-0233
Habib, P., Jung, J., Wilms, G. M., Kokott-Vuong, A., Habib, S., Schulz, J. B., et al. (2021). Posthypoxic behavioral impairment and mortality of Drosophila melanogaster are associated with high temperatures, enhanced predeath activity and oxidative stress. Exp. Mol. Med. 53, 264–280. doi: 10.1038/s12276-021-00565-3
Haddad, G. G., Sun, Y. A., Wyman, R. J., and Xu, T. (1997a). Genetic basis of tolerance to O2 deprivation in Drosophila melanogaster. Proc. Natl. Acad. Sci. USA 94, 10809–10812. doi: 10.1073/pnas.94.20.10809
Haddad, G. G., Wyman, R. J., Mohsenin, A., Sun, Y., and Krishnan, S. N. (1997b). Behavioral and Electrophysiologic responses of Drosophila melanogaster to prolonged periods of anoxia. J. Insect Physiol. 43, 203–210. doi: 10.1016/S0022-1910(96)00084-4
Harari, O. A., and Liao, J. K. (2010). NF-κB and innate immunity in ischemic stroke. Ann. N. Y. Acad. Sci. 1207, 32–40. doi: 10.1111/j.1749-6632.2010.05735.x
He, Q., Ma, Y., Liu, J., Zhang, D., Ren, J., Zhao, R., et al. (2021). Biological functions and regulatory mechanisms of hypoxia-inducible factor-1α in ischemic stroke. Front. Immunol. 12:801985. doi: 10.3389/fimmu.2021.801985
Ishida, I., Kubo, H., Suzuki, S., Suzuki, T., Akashi, S., Inoue, K., et al. (2002). Hypoxia diminishes toll-like receptor 4 expression through reactive oxygen species generated by mitochondria in endothelial cells. J. Immunol. 169, 2069–2075. doi: 10.4049/jimmunol.169.4.2069
Jia, J., Cheng, J., Ni, J., and Zhen, X. (2015). Neuropharmacological actions of metformin in stroke. Curr. Neuropharmacol. 13, 389–394. doi: 10.2174/1570159X13666150205143555
Jung, J., Fehr, A. T., Voigt, A., and Habib, P. (2022). Standardized hypoxia-reoxygenation protocol to assess posthypoxic neurobehavioral impairments and molecular mechanisms in Drosophila melanogaster. STAR Protoc. 3:101634. doi: 10.1016/j.xpro.2022.101634
Kim, J. Y., Han, Y., Lee, J. E., and Yenari, M. A. (2018). The 70-kDa heat shock protein (Hsp70) as a therapeutic target for stroke. Expert Opin. Ther. Targets 22, 191–199. doi: 10.1080/14728222.2018.1439477
Kokott-Vuong, A., Jung, J., Fehr, A. T., Kirschfink, N., Noristani, R., Voigt, A., et al. (2021). Increased post-hypoxic oxidative stress and activation of the PERK branch of the UPR in Trap1-deficient Drosophila melanogaster is abrogated by metformin. Int. J. Mol. Sci. 22:11586. doi: 10.3390/ijms222111586
Komiya, Y., and Habas, R. (2008). Wnt signal transduction pathways. Organogenesis 4, 68–75. doi: 10.4161/org.4.2.5851
Kreiner, G. (2015). Compensatory mechanisms in genetic models of neurodegeneration: are the mice better than humans? Front. Cell. Neurosci. 9:56. doi: 10.3389/fncel.2015.00056
Lee, B., Barretto, E. C., and Grewal, S. S. (2019). TORC1 modulation in adipose tissue is required for organismal adaptation to hypoxia in Drosophila. Nat. Commun. 10:1878. doi: 10.1038/s41467-019-09643-7
Li, Q. Q., Ding, D. H., Wang, X. Y., Sun, Y. Y., and Wu, J. (2021). Lipoxin A4 regulates microglial M1/M2 polarization after cerebral ischemia-reperfusion injury via the notch signaling pathway. Exp. Neurol. 339:113645. doi: 10.1016/j.expneurol.2021.113645
Li, K., Li, T., Wang, Y., Xu, Y., Zhang, S., Culmsee, C., et al. (2019). Sex differences in neonatal mouse brain injury after hypoxia-ischemia and adaptaquin treatment. J. Neurochem. 150, 759–775. doi: 10.1111/jnc.14790
Li, J., Lu, Z., Li, W. L., Yu, S. P., and Wei, L. (2008). Cell death and proliferation in NF-kappaB p50 knockout mouse after cerebral ischemia. Brain Res. 1230, 281–289. doi: 10.1016/j.brainres.2008.06.130
Liu, G., Roy, J., and Johnson, E. A. (2006). Identification and function of hypoxia-response genes in Drosophila melanogaster. Physiol. Genomics 25, 134–141. doi: 10.1152/physiolgenomics.00262.2005
Ma, E., and Haddad, G. G. (1999). Isolation and characterization of the hypoxia-inducible factor 1beta in Drosophila melanogaster. Brain Res. Mol. Brain Res. 73, 11–16. doi: 10.1016/S0169-328X(99)00224-7
Minakhina, S., and Steward, R. (2006). Nuclear factor-kappa B pathways in Drosophila. Oncogene 25, 6749–6757. doi: 10.1038/sj.onc.1209940
Misra, T., Baccino-Calace, M., Meyenhofer, F., Rodriguez-Crespo, D., Akarsu, H., Armenta-Calderón, R., et al. (2017). A genetically encoded biosensor for visualising hypoxia responses in vivo. Biology Open. 6, 296–304.
Mo, Z., Zeng, Z., Liu, Y., Zeng, L., Fang, J., and Ma, Y. (2022). Activation of Wnt/beta-catenin signaling pathway as a promising therapeutic candidate for cerebral ischemia/reperfusion injury. Front. Pharmacol. 13:914537. doi: 10.3389/fphar.2022.914537
Nasri, H., and Rafieian-Kopaei, M. (2014). Metformin: current knowledge. J. Res. Med. Sci. 19, 658–664.
Noguchi, K., Yokozeki, K., Tanaka, Y., Suzuki, Y., Nakajima, K., Nishimura, T., et al. (2022). Sima, a Drosophila homolog of HIF-1α, in fat body tissue inhibits larval body growth by inducing tribbles gene expression. Genes Cells 27, 145–151. doi: 10.1111/gtc.12913
O'Brien, K. A., Murray, A. J., and Simonson, T. S. (2022). Notch signaling and cross-talk in hypoxia: a candidate pathway for high-altitude adaptation. Life (Basel). 12:437. doi: 10.3390/life12030437
Polan, D. M., Alansari, M., Lee, B., and Grewal, S. S. (2020). Early-life hypoxia alters adult physiology and reduces stress resistance and lifespan in Drosophila. J. Exp. Biol. 223:jeb226027. doi: 10.1242/jeb.226027
Prabhakaran, S., Ruff, I., and Bernstein, R. A. (2015). Acute stroke intervention: a systematic review. JAMA 313, 1451–1462. doi: 10.1001/jama.2015.3058
Romero, N. M., Dekanty, A., and Wappner, P. (2007). Cellular and developmental adaptations to hypoxia: a Drosophila perspective. Method Enzymol. 435, 123–144. doi: 10.1016/S0076-6879(07)35007-6
Simões, A. R., Neto, M., Alves, C. S., Santos, M. B., Fernández-Hernández, I., Veiga-Fernandes, H., et al. (2022). Damage-responsive neuro-glial clusters coordinate the recruitment of dormant neural stem cells in Drosophila. Dev. Cell 57, 1661–1675.e7. doi: 10.1016/j.devcel.2022.05.015
Snigdha, K., Gangwani, K. S., Lapalikar, G. V., Singh, A., and Kango-Singh, M. (2019). Hippo signaling in cancer: lessons from Drosophila models. Front. Cell Dev. Biol. 7:85. doi: 10.3389/fcell.2019.00085
Steinmetz, E. L., Dewald, D. N., and Walldorf, U. (2021). Drosophila homeodomain-interacting protein kinase (Hipk) phosphorylates hippo/warts signalling effector Yorkie. Int. J. Mol. Sci. 22:1862. doi: 10.3390/ijms22041862
Ström, J. O., Ingberg, E., Theodorsson, A., and Theodorsson, E. (2013). Method parameters' impact on mortality and variability in rat stroke experiments: a meta-analysis. BMC Neurosci. 14:41. doi: 10.1186/1471-2202-14-41
Texada, M. J., Jørgensen, A. F., Christensen, C. F., Koyama, T., Malita, A., Smith, D. K., et al. (2019). A fat-tissue sensor couples growth to oxygen availability by remotely controlling insulin secretion. Nat. Commun. 10:1955. doi: 10.1038/s41467-019-09943-y
Valanne, S., Wang, J. H., and Rämet, M. (2011). The Drosophila toll signaling pathway. J. Immunol. 186, 649–656. doi: 10.4049/jimmunol.1002302
Vigne, P., Tauc, M., and Frelin, C. (2009). Strong dietary restrictions protect Drosophila against anoxia/reoxygenation injuries. PLoS One 4:e5422. doi: 10.1371/journal.pone.0005422
Ward, N. S., and Carmichael, S. T. (2020). Blowing up neural repair for stroke recovery: preclinical and clinical trial considerations. Stroke 51, 3169–3173. doi: 10.1161/STROKEAHA.120.030486
Wong, D. M., Shen, Z., Owyang, K. E., and Martinez-Agosto, J. A. (2014). Insulin- and warts-dependent regulation of tracheal plasticity modulates systemic larval growth during hypoxia in Drosophila melanogaster. PLoS One 9:e115297. doi: 10.1371/journal.pone.0115297
Yamaguchi, M., and Yoshida, H. (2018). Drosophila as a model organism. Adv. Exp. Med. Biol. 1076, 1–10. doi: 10.1007/978-981-13-0529-0_1
Yan, Q., Sun, S. Y., Yuan, S., Wang, X. Q., and Zhang, Z. C. (2020). Inhibition of microRNA-9-5p and microRNA-128-3p can inhibit ischemic stroke-related cell death in vitro and in vivo. IUBMB Life 72, 2382–2390. doi: 10.1002/iub.2357
Zarndt, R., Piloto, S., Powell, F. L., Haddad, G. G., Bodmer, R., and Ocorr, K. (2015). Cardiac responses to hypoxia and reoxygenation in Drosophila. Am. J. Physiol. Regul. Integr. Comp. Physiol. 309, R1347–R1357. doi: 10.1152/ajpregu.00164.2015
Zhang, Q., Jia, M., Wang, Y., Wang, Q., and Wu, J. (2022). Cell death mechanisms in cerebral ischemia-reperfusion injury. Neurochem. Res. 47, 3525–3542. doi: 10.1007/s11064-022-03697-8
Zhou, D., and Haddad, G. G. (2013). Genetic analysis of hypoxia tolerance and susceptibility in Drosophila and humans. Annu. Rev. Genomics Hum. Genet. 14, 25–43. doi: 10.1146/annurev-genom-091212-153439
Zhou, D., Stobdan, T., Visk, D., Xue, J., and Haddad, G. G. (2021). Genetic interactions regulate hypoxia tolerance conferred by activating notch in excitatory amino acid transporter 1-positive glial cells in Drosophila melanogaster. G3 (Bethesda) 11:jkab038. doi: 10.1093/g3journal/jkab038
Zhou, D., Udpa, N., Gersten, M., Visk, D. W., Bashir, A., Xue, J., et al. (2011). Experimental selection of hypoxia-tolerant Drosophila melanogaster. Proc. Natl. Acad. Sci. U.S.A. 108, 2349–2354. doi: 10.1073/pnas.1010643108
Zhou, H., Wang, X., Ma, L., Deng, A., Wang, S., and Chen, X. (2019). FoxO3 transcription factor promotes autophagy after transient cerebral ischemia/reperfusion. Int. J. Neurosci. 129, 738–745. doi: 10.1080/00207454.2018.1564290
Keywords: hypoxia resistance, Drosophila melanogaster, oxidative stress, insulin, notch, hypoxia inducible factors, NF-κB, stroke
Citation: Quadros-Mennella PS, Lucin KM and White RE (2024) What can the common fruit fly teach us about stroke?: lessons learned from the hypoxic tolerant Drosophila melanogaster. Front. Cell. Neurosci. 18:1347980. doi: 10.3389/fncel.2024.1347980
Edited by:
Creed Stary, Stanford University, United StatesReviewed by:
Neha Nanda, Harvard Medical School, United StatesJacopo Di Gregorio, University of L'Aquila, Italy
Copyright © 2024 Quadros-Mennella, Lucin and White. This is an open-access article distributed under the terms of the Creative Commons Attribution License (CC BY). The use, distribution or reproduction in other forums is permitted, provided the original author(s) and the copyright owner(s) are credited and that the original publication in this journal is cited, in accordance with accepted academic practice. No use, distribution or reproduction is permitted which does not comply with these terms.
*Correspondence: Robin E. White, cndoaXRlQHdlc3RmaWVsZC5tYS5lZHU=