- 1Division of Medical Sciences, University of Victoria, Victoria, BC, Canada
- 2Centre for Advanced Materials and Related Technology, Victoria, BC, Canada
- 3Department of Medicine, University of British Columbia, Vancouver, BC, Canada
- 4Department of Surgery, University of British Columbia, Vancouver, BC, Canada
- 5British Columbia Children’s Hospital Research Institute, Vancouver, BC, Canada
- 6Département de Psychiatrie et de Neurosciences, Faculté de Médecine, Université Laval, Québec City, QC, Canada
- 7Axe neurosciences, Centre de Recherche du CHU de Québec, Université Laval, Québec City, QC, Canada
- 8Department of Molecular Medicine, Université Laval, Québec City, QC, Canada
- 9Department of Biochemistry and Molecular Biology, University of British Columbia, Vancouver, BC, Canada
- 10Department of Neurology and Neurosurgery, McGill University, Montréal, QC, Canada
The central nervous system (CNS) is an essential hub for neuronal communication. As a major component of the CNS, glial cells are vital in the maintenance and regulation of neuronal network dynamics. Research on microglia, the resident innate immune cells of the CNS, has advanced considerably in recent years, and our understanding of their diverse functions continues to grow. Microglia play critical roles in the formation and regulation of neuronal synapses, myelination, responses to injury, neurogenesis, inflammation, and many other physiological processes. In parallel with advances in microglial biology, cutting-edge techniques for the characterization of microglial properties have emerged with increasing depth and precision. Labeling tools and reporter models are important for the study of microglial morphology, ultrastructure, and dynamics, but also for microglial isolation, which is required to glean key phenotypic information through single-cell transcriptomics and other emerging approaches. Strategies for selective microglial depletion and modulation can provide novel insights into microglia-targeted treatment strategies in models of neuropsychiatric and neurodegenerative conditions, cancer, and autoimmunity. Finally, fate mapping has emerged as an important tool to answer fundamental questions about microglial biology, including their origin, migration, and proliferation throughout the lifetime of an organism. This review aims to provide a comprehensive discussion of these established and emerging techniques, with applications to the study of microglia in development, homeostasis, and CNS pathologies.
Introduction
Microglia are the resident innate immune cells of the central nervous system (CNS). Originating in the yolk sac and colonizing the CNS during early embryonic development (Ginhoux et al., 2010; Gomez Perdiguero et al., 2015), microglia perform various physiological roles in the development and maintenance of CNS homeostasis, continuously monitoring their microenvironment and responding to cues (Šišková and Tremblay, 2013; Ransohoff and El Khoury, 2015; Augusto-Oliveira et al., 2019). During homeostasis, these cells notably assist in maintaining neuronal and glial populations, clear extracellular debris, and regulate axonal myelination, neuronal activity and neurotransmitter levels as pivotal players of CNS function, plasticity and integrity (Gomez-Nicola and Perry, 2015; Cornell et al., 2022). Dysregulation of microglial activities is linked to several neurodevelopmental, neurological and psychiatric disorders, leading to neuronal damage and cognitive impairment (Figure 1; Tremblay and Majewska, 2011; Kwon and Koh, 2020).
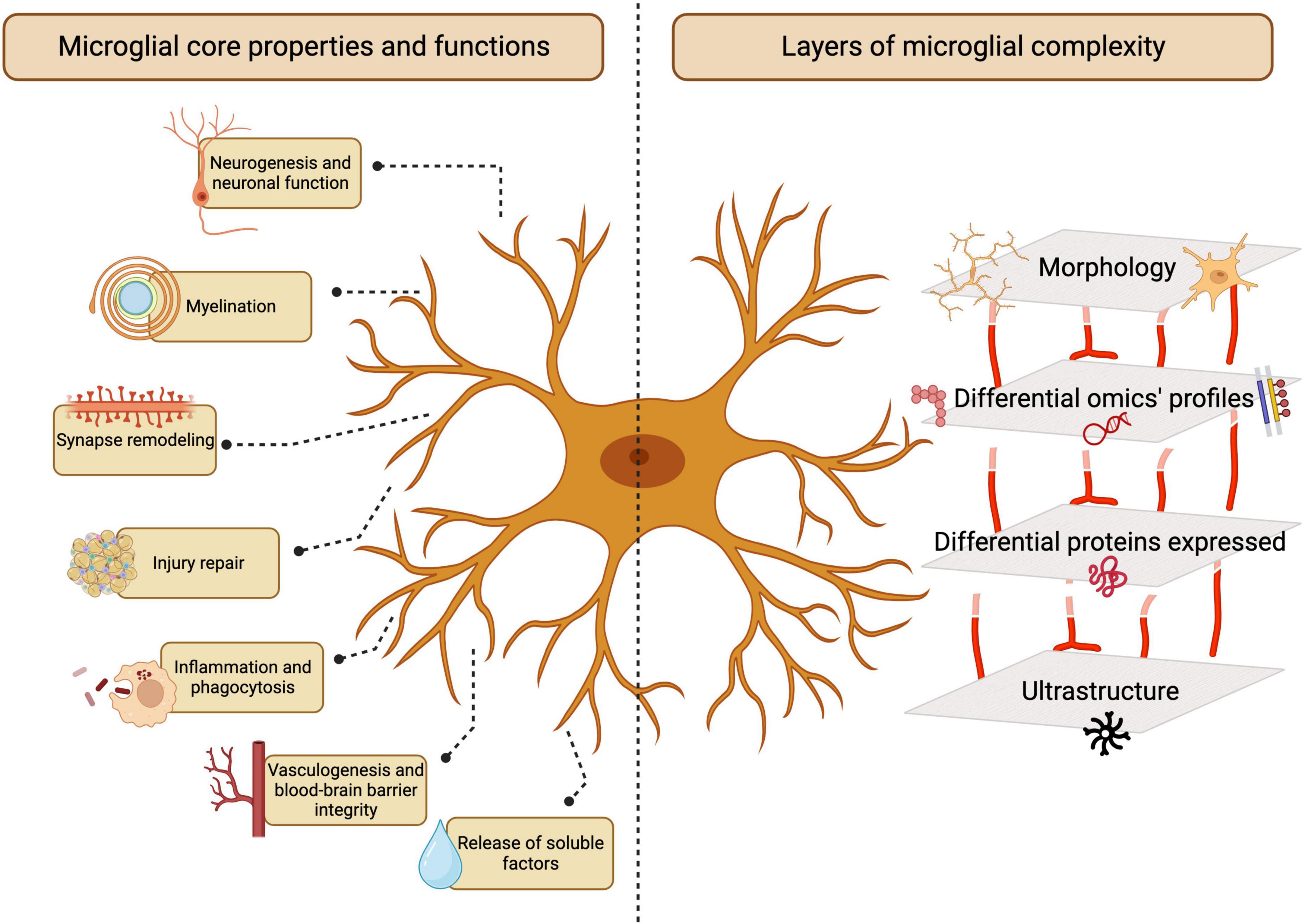
Figure 1. Microglia functions in the central nervous system (CNS). Microglia play essential and multifaceted roles in the CNS throughout homeostasis and disease. The study of microglia involves several layers of complexity, ranging from morphological analysis to emerging multi-omics-based characterization. Created with BioRender.com.
The wide range of microglial functions in the CNS is attributable to the established concept of microglia as a heterogeneous and dynamic cell population. Microglia coexist in a plethora of structurally and functionally distinct states, including homeostatic and disease-associated phenotypes (Keren-Shaul et al., 2017; Butovsky and Weiner, 2018; Deczkowska et al., 2018; Hammond et al., 2019; Li et al., 2019a; Stratoulias et al., 2019). These states are not fixed; rather, microglia undergo phenotypic shifts, often rapidly, in response to diverse stimuli (Nimmerjahn et al., 2005; Bennett et al., 2016; Krasemann et al., 2017). The microglial sensome, composed of various receptors and signaling molecules, enables these cells to detect changes in their environment and respond accordingly, actively regulating CNS homeostasis (Nimmerjahn et al., 2005; Hanisch and Kettenmann, 2007; Hickman et al., 2013; Rubino et al., 2018; Carrier et al., 2022). Understanding microglia is a challenging endeavor due to the complex nature of these cells, which express diverse receptors and exhibit different states/phenotypes that are context-dependent. These states are influenced by various factors such as age, environmental exposures, disease, and sex (Carrier et al., 2022; Bobotis B. C. et al., 2023; González Ibáñez et al., 2023; Picard et al., 2023). Moreover, multimodal approaches are often employed in order to distinguish these cells from non-parenchymal cells in the CNS and infiltrating myeloid cells. Through the combination of genetic labeling, imaging, and knockout (KO) studies with proteomic, metabolomic and transcriptomic analyses, our understanding of microglial behavior, phenotype, and function in the CNS has greatly advanced in recent years.
The field of immunology has advanced substantially through the use of imaging tools. From Élie Metchnikoff’s pioneering visualization of phagocytosis in 1882 (Tan and Dee, 2009) to modern techniques such as 3-dimensional electron microscopy (EM) and two-photon in vivo microscopy (Maco et al., 2014), the range of tools available for studying the CNS immune system has significantly expanded. Notably, this has enabled a more comprehensive understanding of the microglial sensome and the multi-dimensional mechanisms underlying microglial physiological and immunological functions (Hickman et al., 2013; Sierra et al., 2019; Carrier et al., 2021). As we strive to understand the role of microglia in both pathology and homeostasis, the ability to visualize, selectively modulate or deplete these cells across different contexts has become increasingly important (Hickman et al., 2013; Konishi and Kiyama, 2018). Characterizing the microglial sensome and identifying specific microglial markers are crucial endeavors to furthering understanding of the mechanisms underlying microglia-mediated CNS inflammation and microglial involvement in models of various disorders of the CNS (Hickman et al., 2013; Carrier et al., 2022). This review will examine emerging techniques for microglial imaging and visualization, through antibody-mediated labeling, EM, reporter models, and fate mapping, as well as strategies for the specific depletion or genetic modification of microglia to gain insights into their function. Given that microglia reside in the CNS, much of our knowledge of the roles of microglia in living organisms derives from work in rodents. Thus, we primarily focus on the study of microglia in mouse models, corroborating results with studies of human microglia where possible.
Antibody-mediated identification of microglial markers
Antibodies are versatile tools that can be used to identify, isolate, quantify, localize and visualize specific targets in a biological sample (Goldman, 2000). Researchers have adopted the use of antibodies for a wide range of specialized techniques, such as immunohistochemistry (IHC), enzyme-linked immunosorbent assays (ELISA), Western blotting, flow cytometry and fluorescence-activated cell sorting (FACS). Several commonly targeted microglial surface proteins, discussed in detail below, enable the antibody-mediated visualization of microglia in the CNS, since these cells express a great variety of receptors to mediate extensive interactions with other cell types and their microenvironment (Casali and Reed-Geaghan, 2021). The subcellular location of microglial proteins is an important consideration, as intracellular and extracellular protein targets require distinct labeling approaches and provide different insights into microglial populations and their dynamics (Figure 2). For instance, while membrane and extracellular proteins can shed light on microglial reactivity, inflammatory processes and interactions with other cell types (Szepesi et al., 2018; Jurga et al., 2020); intracellular proteins can elucidate cytoskeletal reorganization processes, with implications for cell migration and morphology (Sasaki et al., 2001; Ohsawa et al., 2004; Rosito et al., 2023). Microglia produce a number of cytokines and extracellularly-active enzymes (Kingham and Pocock, 2001; Lee et al., 2010; Smith et al., 2012). The cytokines and secreted proteins they release serve as distinctive markers for identifying microglia and offer valuable insights into their functionality, state, and phenotype. Specific microglial states are characterized by distinct profiles of released cytokines and differentially expressed genes; for instance, the disease-associated microglia (DAM; further discussed in the following section) downregulate homeostatic genes and reactive states of microglia are known to produce elevated levels of pro-inflammatory cytokines, such as IL-1, IL-6, and TNF-α (Smith et al., 2012; Šišková and Tremblay, 2013; Jurga et al., 2020; Paolicelli et al., 2022; Rosito et al., 2023). As certain microglial proteins are differentially expressed under inflammatory conditions, and can furthermore be induced on other cell types, validation of individual markers within the specific model of interest is advisable (Honarpisheh et al., 2020). Key microglial surface, intracellular and secreted proteins will also be summarized in Table 1.
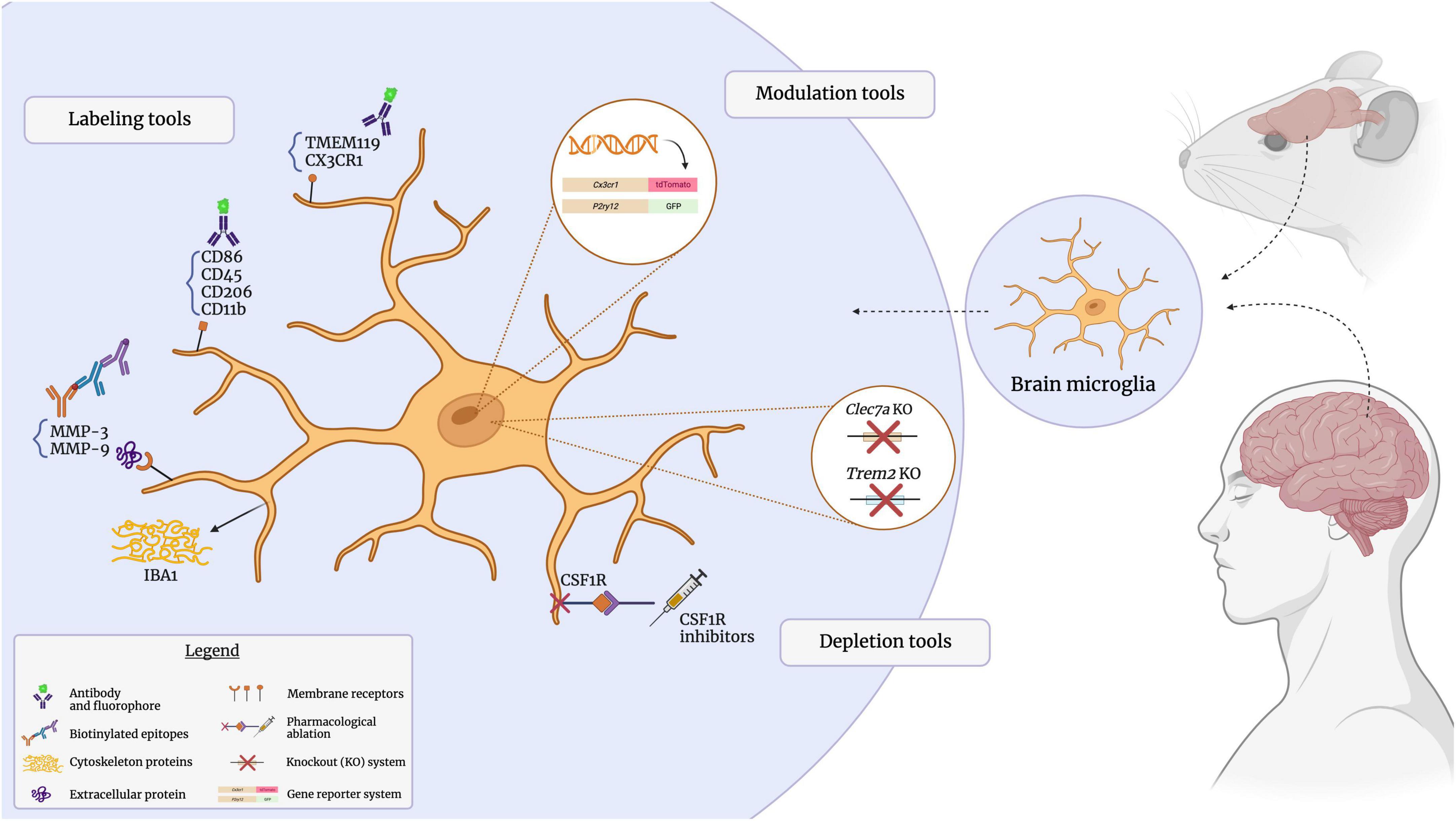
Figure 2. Visualizing the microglial sensome. The microglial sensome comprises a plethora of extracellular, intracellular, and secreted proteins. Antibody-mediated detection, as well as genetic and pharmacological approaches to selectively visualize and modulate these targets through immunostaining assays, reporter-based strategies, and knockout models, have greatly contributed to our understanding of microglial function. Created with BioRender.com.
Membrane-associated surface markers
Microglia present unique transcriptional signatures related to their activity and the environmental context. Under homeostatic conditions, certain surface proteins are highly expressed, such as the CX3-motif chemokine receptor (CX3CR1), the P2Y12 purinergic receptor (P2RY12), and transmembrane protein 119 (TMEM119), among others (Song and Colonna, 2018; Paolicelli et al., 2022). However, microglial states induced by pathological insults, such as the DAM and microglial neurodegenerative phenotype (MGnD), adopt a distinct gene signature characterized by downregulation of homeostatic Cx3cr1, P2ry12, and Tmem119, together with upregulation of Trem2, Apoe, Clec7a, and Cd11c (Keren-Shaul et al., 2017; Krasemann et al., 2017; Butovsky and Weiner, 2018; Deczkowska et al., 2018; Paolicelli et al., 2022). While their functions have not been fully elucidated, DAM are involved in phagocytosis, clearance of apoptotic cell bodies and inflammatory responses (Deczkowska et al., 2018; Dubbelaar et al., 2018; Casali and Reed-Geaghan, 2021). The case of DAM illustrates that certain markers can differentially identify key microglial states during homeostasis and pathology, and these will be the subject of the following discussion. These markers are often used in immunostaining but also are frequently employed in Cre/lox systems, knockout models, using FACS and other methods (Ruan et al., 2020; Perrone et al., 2023). As an illustration of the diversity of techniques used to study microglial markers, Li et al. (2019b) combined FACS, MACS, flow cytometry and q-PCR on whole adult mouse brain tissue to investigate differences between microglial and macrophage responses to intracerebral hemorrhage. Their findings revealed elevated expressions of Tmem119 and Sall1 in the sorted microglial cell population (Ly6g–CD11b+CD45intPI–), while Ccr2 and CD69 were highly expressed in the monocyte/macrophage sorted cell population (Ly6g–CD11b+CD45highPI–), highlighting the utility of multifaceted approaches using multiple markers to reliably distinguish microglia. Notably, microglia expressing both Tmem119 and Sall1 exhibited increased expression following intracerebral hemorrhage, whereas monocytes/macrophages maintained consistent levels of both Ccr2+/CD69+ in the sham and hemorrhage groups (Li et al., 2019b). As discussed in the following sections, distinct sets of markers are commonly used to identify microglia depending on which technique is employed.
CD11b, one of the first identified β-glucan receptors, is an abundant surface protein primarily expressed on cells of the myeloid lineage that has been reliably used for microglial visualization. CD11b assists in endothelial adhesion and regulates the uptake of complement-coated particles by phagocytosis (Xia et al., 1999; Nikodemova and Watters, 2012; Schmid et al., 2018). As CD11b is also expressed by peripherally-derived myeloid cells in the CNS (Fischer and Reichmann, 2001; Greter et al., 2015), it must be paired together with other markers to identify microglia more specifically. It is often co-utilized with universally expressed leukocyte surface proteins such as CD45, the combination of which has utility for identifying microglia by flow cytometry (Bruzelius et al., 2021).
CD45, present on all leukocytes in varying levels, and CD68, a lysosomal marker present in endosomes and lysosomes of the mononuclear phagocytic lineages [including microglia, border-associated macrophages (BAMs) and macrophages], can be used to identify microglia in the CNS and other immune cells (Hendrickx et al., 2017; Rangaraju et al., 2018; Waller et al., 2019; Bruzelius et al., 2021). CD45, a protein tyrosine phosphatase, plays a role in the proliferation and differentiation of immune cells (Penninger et al., 2001), but tends to be expressed at lower levels on microglia than on CNS-infiltrating myeloid cells during non-inflammatory conditions, while CD68 is associated with inflammatory responses, phagolysosomal activity, and the regulation of antigen presentation (Song et al., 2011; Chistiakov et al., 2017). Both CD45 and CD68 contribute to the microglial sensome under homeostatic conditions, although CD45+ and CD68+ signatures are associated with microglial reactivity in response to inflammation, trauma or pathology (Perego et al., 2011; Hoogland et al., 2015; Hendrickx et al., 2017; Rice et al., 2017; Swanson et al., 2023). In combination with other markers, such as ionized calcium-binding adaptor molecule 1 (IBA1) and CD11b, CD45 can aid in distinguishing microglia from other CNS infiltrated myeloid cells and non-parenchymal cell populations, using, for instance, flow cytometry (Waller et al., 2019; Bruzelius et al., 2021). Microglia are generally identifiable as CD11b+CD45int, in contrast to CD11b+CD45hi, being usually attributed to CNS-infiltrating myeloid cells or BAMs at a steady state (Greter et al., 2015; Martin et al., 2017; Rubino et al., 2018; Honarpisheh et al., 2020). Nevertheless, under specific conditions, microglia may exhibit CD45high expression. Studies conducted in models of stroke, cerebral amyloid angiopathy, and aging indicate that Tmem119 and P2RY12 expression is present in both CD45int and CD45high FACS-sorted cells myeloid cell populations (Honarpisheh et al., 2020). Moreover, a small population of CD11b+CD45high mononuclear phagocytes has been identified by flow cytometry in the surroundings of Aβ plaques in the 5XFAD mouse model of Alzheimer’s disease (AD) pathology, and these cells exhibit transcriptional similarities to DAM, such as upregulation of Trem2 and Cd11c (Rangaraju et al., 2018). Additionally, CD44 is also often employed to emphasize CNS-infiltrated myeloid cells, given that macrophages, for example, typically exhibit CD44high expression. In pathology, microglia can exhibit CD44 expression, especially when exposed to lipopolysaccharide (LPS) and in degenerative states like amyotrophic lateral sclerosis. The findings also indicate that primary cultured microglial cells express CD44 upon exposure to LPS and interferon-γ. Moreover, the results align with increased CD44 expression observed following treatment with interferon-γ and TNF-α (Matsumoto et al., 2012; Liu S. et al., 2023).
Thus, the identification of microglia by flow cytometry in pathological conditions remains complicated, requiring fate mapping approaches to distinguish these lineages robustly. Additionally, distinguishing microglia from CNS-infiltrated macrophages is crucial for the precise characterization and understanding of the functionally of each cell type. Notably, BAMs form a distinct, specialized population naturally located in the choroid plexus (CP), meninges and perivascular spaces (Dermitzakis et al., 2023). Given the immune-privileged nature of the CNS, it is naturally constituted and protected by microglia, parenchymal tissue-resident macrophage, along with non-parenchymal cells, the BAMs. The resemblance to microglia is significant, as these cells are also derived from the yolk sac and share certain commonly expressed genes with microglia. For instance, they exhibit low expression levels of P2y12 and Tmem119, while microglia express these genes at higher levels (Rajan et al., 2020). Single-cell approaches, using mouse enriched subdural meninges (SDM) CPepi-BAMs, have also demonstrated that BAM subsets express common DAM signature genes, including an upregulation of genes involved in lipid metabolism or phagocytosis, such as Cst7, Apoe, Clec7a, and Lpl (Van Hove et al., 2019). BAMs often express lymphatic-like gene signatures, such as Pdpn and Lyve1, frequently associated with cell migration. Expression levels naturally differ among distinct subpopulations of BAMs located in various non-parenchymal regions, such as the or meninges (Møllgård et al., 2023). Interestingly, microglia and BAMs share the same erythromyeloid progenitors (EMPs) in the yolk sac, however, fate mapping approaches revealed that these EMPs seem to depend on CD206 expression for their differentiation into microglia or BAMs (Masuda et al., 2022). While only a few microglia start to express CD206 at the embryonic day (E)12.5, BAMs start to highly express it at E10.5 (Masuda et al., 2022). BAMs will be further discussed in the subsequent fate mapping sections. To ensure the characterization and distinction of microglia, BAMs and peripherally derived myeloid cells, complementary techniques are often employed, such as flow cytometry, fate mapping and single-cell transcriptome analysis. IHC can also aid in discerning microglia from peripherally-derived myeloid cells, for example based on higher levels of IBA1 and TMEM119 expression in microglia (Kishimoto et al., 2019).
Both CD86 and CD206 characterize reactive microglia but are associated with distinct functional properties and microglial phenotypes, which are extensively examined in immunohistochemistry and flow cytometry. The C-type lectin CD206 plays a role in recognizing pathogens and clearing glycoproteins from the circulation (Suzuki et al., 2018; Tanaka et al., 2021). Not specific to microglia, CD86 and CD206 are also expressed by other cells in the CNS, such as astrocytes and BAMs, and throughout the body, being found in dendritic cells, macrophages/monocytes and B/T-lymphocytes (Xing et al., 2018; Sun et al., 2019; Trzupek et al., 2020). Upregulation of CD206 is observed in pathological conditions, such as glioma, where increased microglial pinocytosis and phagocytosis mediate the clearance of debris and resolution of inflammation (Tanaka et al., 2021). CD86, also a transmembrane protein, provides a costimulatory signal essential for the activation and proliferation of T cells. Hence it is abundantly expressed on antigen-presenting cells, including microglia, macrophages, dendritic cells, and B cells, and is upregulated in response to injury (Louveau et al., 2015; Hellström Erkenstam et al., 2016). Remarkably, it is seen an increase in both CD86+ and CD206+ subsets among brain CD11b+ cells, in models of traumatic brain injury, neonatal brain hypoxia-ischemia and cerebral ischemia-reperfusion (Jin et al., 2012; Hellström Erkenstam et al., 2016; Bai et al., 2017; Yusuying et al., 2022).
As microglia respond to many cues in the microenvironment, they also naturally express receptors associated with neuronal communication (Butler et al., 2021). CX3CR1 is widely expressed in the CNS, for instance, in macrophages and microglia, and its ligand, fractalkine (CX3CL1), is an important chemokine produced by neurons (Wolf et al., 2013; Szepesi et al., 2018). The CX3CL1-CX3CR1 axis mediates the development and plasticity of neuronal circuits, with functional consequences for brain connectivity, as notably shown by comparing the CX3CR1KO/KO mice with CX3CR1KO/+ mice (Drissen et al., 2019; Lauro et al., 2019). Under physiological conditions, CX3CR1-expressing microglia in the brain are extensively involved in dynamic surveillance and maintenance of brain homeostasis (Lauro et al., 2019). CX3CR1 signaling is also important for microglial motility and the regulation of the microglial response to injury and inflammation. Studies employing immunostaining and q-PCR in mouse models of AD pathology and ischemic stroke have shown that CX3CR1high microglia have increased migration to sites of pathology, whereas CX3CR1low microglia tend to remain in a surveillance state (Tang et al., 2014; Hickman et al., 2019). Similarly, CX3CR1-deficient microglia exhibit delayed migration toward sites of laser-induced injury and impaired repopulation following depletion (Liang et al., 2009; Zhang et al., 2018).
In recent years, TMEM119 had emerged as a microglial marker with high specificity (Bennett et al., 2016; Satoh et al., 2016). Initially identified as a regulator of osteoblast differentiation, TMEM119 is thought to regulate the Wnt/β-catenin pathway, important for proliferation, migration, and genetic stability, but further research is required to fully elucidate its functions in microglia (Satoh et al., 2016; Yang et al., 2021). Selectively expressed by yolk sac-derived cells in the CNS (e.g., microglia and BAMs in the CNS), TMEM119 broadly distinguishes resident microglia from infiltrating macrophages (Haynes et al., 2006; Segal and Giger, 2016; Bohnert et al., 2020; Vankriekelsvenne et al., 2022). TMEM119 immunostaining can be combined with IBA1 to permit distinct visualization of microglia and brain-infiltrating macrophages by immunofluorescence (van Wageningen et al., 2019). However, caution should be exercised when using TMEM119 as a selective marker for microglia. TMEM119 protein expression has been shown to be state-dependent, and can be strongly downregulated by reactive microglia in pathological conditions (Haynes et al., 2006; Segal and Giger, 2016; Bohnert et al., 2020; Vankriekelsvenne et al., 2022).
P2Y receptors are metabotropic purinergic receptors that signal in response to adenosine triphosphate (ATP), notably released by injured cells (van Wageningen et al., 2019; von Kügelgen, 2019; Gómez Morillas et al., 2021). These receptors are extensively present in microglia, but also detected in astrocytes within the CNS, in both humans and rodents (Franke et al., 2004; Milior et al., 2020; Walker et al., 2020). P2RY12 is particularly important for microglia-neuron communication, particularly through regulating microglial chemotaxis toward neuronal-derived ATP in response to injury (Haynes et al., 2006). P2RY12 serves as an effective marker for observing microglial motility, frequently revealing surveillance movements, membrane ruffling, and live imaging of process extension or retraction. In fact, in vitro and in vivo studies have shown that microglia lacking P2RY12 receptors are unable to migrate or extend processes toward nucleotides (Haynes et al., 2006; Lin et al., 2020). Microglial P2RY12 is also a key mediator of synaptic plasticity and behavior (Sipe et al., 2016; Lowery et al., 2021) and contributes to synaptic loss in models of chronic stress (Bollinger et al., 2023). Similarly to TMEM119, P2RY12 is often downregulated under inflammatory or pathological conditions (Haynes et al., 2006; van Wageningen et al., 2019; Lin et al., 2020). In post-mortem tissue samples from multiple sclerosis patients, immunoreactivity for both TMEM119 and P2RY12 was decreased in the centers of white matter lesions, which correlated with the presence of lymphocyte infiltrates and proinflammatory cytokines (van Wageningen et al., 2019). However, in a mouse model of status epilepticus, microglial P2RY12 expression and purinergic signaling were increased in the hippocampus (Avignone et al., 2008), highlighting the complex dynamics of P2RY12 expression across pathologies. Likewise, the pattern of purine-induced motility in human microglia within epileptic tissue appears to have some similarities to that observed in rodent microglia. As reported by Milior and colleagues, low doses of purine in human tissue promoted microglial branch extension, whereas in high concentrations, process retraction and membrane ruffling were facilitated (Milior et al., 2020). Notably, in the context of microglial branch extension, activation of P2Y12 was essential, mirroring that observed in rodents. Yet, the mechanism for microglial processes retraction was mediated by the activation of P2Y1 and P2Y13 receptors, rather than A2A—as classically seen in rodents (Orr et al., 2009; Milior et al., 2020). P2RY12 is extensively used in KO models, fate mapping, and reporter models driven by the purinergic signaling promoter, common within heterozygous GFP reporter mice under the fractalkine receptor promoter or TMEM119. This combination also enables in vivo imaging using two-photon microscopy (Bisht et al., 2021).
Intracellular proteins and transcriptional factors
Intracellular proteins are important for vital microglial functions, such as cell organization, metabolism and adhesion, and can consistently aid in microglial visualization (Zhao et al., 2022). IBA1 is the most common cytoplasmic protein used for microglial immunostaining (Ohsawa et al., 2004; Tremblay et al., 2010). It was first isolated from rats in 1996, and labels both microglia, macrophages/monocytes and BAMs in the CNS (Imai et al., 1996; Sasaki et al., 2001; Jurga et al., 2020). IBA1 is primarily responsible for cytoskeletal reorganization, which enables processes such as membrane ruffling, migration and phagocytosis (Sasaki et al., 2001; Ohsawa et al., 2004; Hopperton et al., 2018; Sobue et al., 2021). IBA1 is commonly used in IHC, mostly in conjugation with other antibodies of interest such as TMEM119 and P2RY12, to aid the distinction of infiltrating myeloid cells, which stain dimly with these antibodies. IBA1-IHC can also be coupled to other techniques, such as CX3CR1-GFP reporter mice or single-cell approaches to describe diverse microglial subpopulations across various physiological and pathological conditions in the CNS (Ibanez et al., 2019; Lituma et al., 2021; González Ibáñez et al., 2023). In both white and gray matter, IBA1 has high sensitivity for microglia and appears to be expressed across a majority of microglial states (Shapiro et al., 2009). Moreover, IBA1 expression is found across many vertebrate and invertebrate species, highlighting its expression in humans and rodents (Swanson et al., 2020; Sharma et al., 2021). Interestingly, studies show that IBA1 expression in human microglia may be altered in pathological states, including AD (Hopperton et al., 2018). Under these conditions, microglial morphology and ultrastructure can aid in identifying microglial phenotypes. For instance, dark microglia, a phenotype identified by EM and found in mouse models and human post-mortem samples of AD pathology, express very low levels of IBA1 but can be distinguished by their unique ultrastructural characteristics, notably an electron-dense (dark) cytoplasm and nucleoplasm (Bisht et al., 2016). In contrast to mouse models, dark microglia observed to date in human patients with AD stain positive for IBA1 (St-Pierre et al., 2022a). Dystrophic microglia represent another morphologically-defined phenotype, staining positive for IBA1 but exhibiting thin, fragmented processes (Streit et al., 2004, 2009), and are found in brains of human AD patients in proportionately greater quantities than during normal aging (Shahidehpour et al., 2021). These microglia are thought to be senescent and dysfunctional, and their presence correlates with neuropathology in AD (Streit et al., 2009, 2020).
The advent of novel transcriptomic techniques, such as microarrays and RNA sequencing, has facilitated the identification of key microglial regulators of transcription (Olah et al., 2020). Researchers recently demonstrated that the Spalt-like 1 gene (Sall1) and the hexosaminidase β-subunit (Hexb) are selectively expressed in murine and human brain microglia and play important roles in normal microglial maturation and function (Butovsky and Weiner, 2018). In mice, SALL1 is expressed by microglia and by other mononuclear or resident glial cells of the CNS, such as astrocytes (Buttgereit et al., 2016; Chi et al., 2019). SALL1 is highly expressed in young murine CNS microglia associated with critical functions, such as neural maturation and synaptic pruning (Buttgereit et al., 2016; Holtman et al., 2017; Sobue et al., 2021; Scott et al., 2022). Microglia-specific Sall1 deletion in vivo results in altered morphology and converts surveillant microglia to a reactive, proinflammatory phenotype (Buttgereit et al., 2016). Additionally, SALL1 is expressed during development and persists throughout the life of the cell, making it a useful marker for tracking microglia across the lifespan (Buttgereit et al., 2016).
Similarly, Hexb is a lysosomal enzyme that plays critical role in lysis processes, breaking down fatty compounds such as sphingolipids and gangliosides (Kuil et al., 2019). Recent studies using RNAscope and single-cell RNA sequencing have shown that Hexb is expressed in brain microglia and its expression is highly restricted to these cells within the CNS (Masuda et al., 2020; Faust et al., 2023). Notably, it was not detected in CNS-associated macrophages according to Masuda et al. (2020); however, it has been identified in non-CNS cells, such as Ly6C+ monocytes obtained from the bone marrow (Hickman et al., 2013; Monaghan et al., 2019; Masuda et al., 2020; Shah et al., 2022). HEXB may also play a role in pathology, with elevated gene expression of HEXB associated with poor prognosis in human glioblastoma (Jia et al., 2021). Although HEXB immunostaining has been utilized in combination with TMEM119 to directly identify microglia (Jia et al., 2021), Hexb-based gene reporter and fate mapping tools, have also recently provided important insights into the behavior and functions of microglia in vivo (Masuda et al., 2020). Additionally, the promoter region of Hexb has recently been characterized, enabling the study of Hexb as a potential target for microglia-targeted gene therapies in humans (Shah et al., 2022).
Nuclear-associated markers
Classically, transcription factors modulate transcription within the nucleus, providing effective targets for identifying microglial cells. For instance, PU.1 is exclusively found in microglia in the brain, however, it is also expressed throughout the body by other cells, such as macrophages, granulocytes, and B cells (Pimenova et al., 2021; Cakir et al., 2022). PU.1 is a crucial regulator of microglial development and homeostatic function. In fact, KO of PU.1 in BV2, an immortalized mouse microglial cell line, abates most of the homeostatic microglial signatures. Conversely, it upregulates genes associated with DAMs, including Lpl and Clec7a, as assessed through q-PCR and Western Blotting in BV2-lineage cells (Pimenova et al., 2021). Moreover, elevated PU.1 expression is associated with an elevated risk of AD, whereas reduced expression is believed to offer some protective effect and contribute to improving inflammation balance by microglia, result observed in primary human mixed glial cultures following siRNA-mediated KO of PU.1 (Rustenhoven et al., 2018; Pimenova et al., 2021; Ralvenius et al., 2023). Sall1 can also be referred to as a nuclear marker for microglia. In mice, the initiation of Sall1 expression occurs at E11 to 12 in hematopoietic progenitor cells derived from the yolk sac. These cells infiltrate the developing brain and ultimately differentiate into resident microglia. Notably, the expression of Sall1 is dependent on TGFβ1 signaling, an instrumental pathway in microglial differentiation and survival (Fixsen et al., 2023). The expression of Sall1 by microglia is context-dependent, it can decline when microglia are transplanted from the brain to an in vitro environment, underscoring the importance of the brain micro-environment to sustain an authentic in vivo microglial phenotype (Gosselin et al., 2017; Fixsen et al., 2023).
Similarly, single-cell technology has identified ZEB1 and MAFB as reliable nuclear markers for microglia. MAFB exhibits diffuse expression throughout the brain and body in both mice and humans, including neurons, microglia/macrophages, other glial cells, Kupffer cells in the liver, and Hofbauer cells, fetal origin macrophages in placenta (Anderson et al., 2023; Liu S. et al., 2023; Liu W. et al., 2023; Vondra et al., 2023). On the other hand, ZEB1 is expressed in radial glial cells and intermediate progenitor cells, as well as mature astrocytes, but seems to be downregulated in neuronal lineages (Gupta et al., 2021). Using multiomics and a methodology to delineate peak-gene-transcription factor trios in nuclei isolated from human post-mortem DLPFC tissues, Anderson et al. (2023) showed that the regulation of MAFB in microglia has been linked to high DAM-like signatures, including TRL3 and CD84. Interestingly, ZEB1 in neurons was identified in half of all AD-specific trios with target genes involved in regulating ion channel signaling (ITPR1, CAMK2A, CACNB3). In contrast, ZEB1 was not identified in control (non-AD) specific trios, suggesting that it may experience upregulation under pathological conditions (Maimaiti et al., 2019; Poonaki et al., 2022; Anderson et al., 2023).
Extracellular and secreted proteins
The extracellular matrix (ECM) is a non-cellular component present in all tissues that provides a physical scaffold, biochemical and biomechanical cues, and helps organize cellular morphology and structure. Each tissue has unique ECM characteristics and structure, with specific growth factors and enzymes determining its composition and function (Theocharis et al., 2016; Lam et al., 2019). As an extracellular component, the ECM is constituted of a variety of matrix proteins that are produced by many different cell types. Components of the ECM can actively influence the adhesion, migration, and morphology of microglial cells. For instance, various components of the ECM, such as fibronectin, vitronectin and laminin, interact with microglial cell surface receptors. For instance, microglia can adhere to these specific ECM molecules through integrin receptors, reshaping microglial activity and motility according to the ECM environment (Milner and Campbell, 2002). Notably, neuronal IL 33 contributes significantly to synapse plasticity in the hippocampus of adult mice. While IL-33 guides microglial engulfment of the ECM, its deficiency results in compromised ECM engulfment, leading to the accumulation of ECM proteins in proximity to synapses (Nguyen et al., 2020). Notably, all synapses are surrounded by interstitial extracellular matrix (iECM), mostly regulating the synapse volume, however, certain synapses are enclosed by a condensed version of the ECM, known as perineuronal nets (PNNs) (Fawcett et al., 2022). Specifically, PNNs form a specialized ECM type in the CNS, providing structural support for cellular elements and maintaining synapse homeostasis. These nets are mostly formed by chondroitin sulfate proteoglycans (CSPGs) attached to a hyaluronan structure (Fawcett et al., 2022). PNNs form a net-like structure that surrounds the cell body and dendrites, enveloping synapses. It is now evident that PNNs significantly contribute to plasticity control. Interestingly, approximately 80% of parvalbumin+ neurons in the primary somatosensory cortex are coated with PNNs. Venturino et al. (2021) showed that microglia closely interact with parvalbumin neurons in the cortex using immunostaining against IBA1 and parvalbumin itself in adult mice. Moreover, parvalbumin neurons and IBA1+ microglia significantly increased matrix metalloproteinase (MMP)-9 levels upon ketamine exposure in cortical layers 3–5 (Venturino et al., 2021).
Specifically, microglia, as well as monocytes, macrophages, astrocytes and lymphocytes, produce MMPs such as MMP-3 and MMP-9, which mediate the degradation of multiple ECM components (Lindberg et al., 2001; Patnaik et al., 2021). MMPs play important physiological roles in synaptic plasticity, learning and memory (Meighan et al., 2006, 2007; Szepesi et al., 2014; Bijata et al., 2017). In fact, MMP-9 is especially vital for the formation of sensory circuits in the early postnatal period, demonstrating increased activity that aligns with synaptic reorganization during critical period plasticity and developmental windows across the CNS (Hanania et al., 2012; Reinhard et al., 2015; Shih et al., 2021; Akol et al., 2022). MMPs have been implicated in various disease states, with hippocampal induction of MMP-9 activity recently shown to mediate the development of depressive-like behavior in a mouse model of chronic stress (Bijata et al., 2022). Microglial upregulation of MMPs is also associated with inflammation or injury (Rosell et al., 2006; Colognato and Tzvetanova, 2011; Könnecke and Bechmann, 2013). Microglial-secreted MMP-3 and MMP-9 in turn influence microglia themselves, promoting production of reactive oxygen species and proinflammatory cytokines such as TNF-α and IL-1β (Kim et al., 2005; Woo et al., 2008; Könnecke and Bechmann, 2013). Recently, Kim J. et al. (2021) employed MMP-9 staining by IHC to identify microglia in the aqueous humor of patients with age-related macular degeneration and in related mouse models of choroidal neovascularization. MMP-9 expression co-localized with IBA1+ reactive microglia defined by an amoeboid morphology, and choroidal lesions. Notably, MMP-9 expression was suppressed by minocycline, a modulator of microglia (Kim J. et al., 2021). Thus, while the ECM itself is not specific to microglia, the use of specific ECM proteins as markers in combination with other techniques can provide a means of identifying and tracking reactive microglial states throughout physiological and pathological contexts.
Microglia in nanoscale: electron microscopy ultrastructure analysis
The visualization of microglia using fluorescent targets has proven highly informative (Davalos et al., 2005; Nimmerjahn et al., 2005). However, the spatial resolution of fluorescence-based techniques are limited by the wavelength of the photon (Carrier et al., 2020). EM overcomes this limitation, permitting detailed examination of intracellular structures at nanometer resolution (Tremblay et al., 2010; Tremblay and Majewska, 2019; Carrier et al., 2020; Bordeleau et al., 2021). At a glance, microglia are visibly smaller than other glial cells and neurons (Nahirney and Tremblay, 2021), with the soma often less than 10 μm in diameter, and display a more triangular or elongated shape than other glial cells, but this varies depending on the tissue context (St-Pierre et al., 2022b). Microglia are also distinguished by their association with pockets of extracellular space and long stretches of endoplasmic reticulum (Tremblay et al., 2010; Nahirney and Tremblay, 2021). Microglial cell bodies are distinctive from their neuronal counterparts by a patchy “leopard” distribution of heterochromatin on the pale euchromatin background, as seen in Figure 3 (St-Pierre et al., 2022b). Additionally, the boundary of the nucleus is commonly surrounded by heterochromatin (St-Pierre et al., 2022b). Microglia in EM can sometimes be identified by the presence of intracellular inclusions such as lysosomes, lipofuscin granules, and lipid bodies (St-Pierre et al., 2022b). These cytoplasmic inclusions also provide information on the current activity of the microglia analyzed, providing insight into cellular health and function (El Hajj et al., 2019; Lecours et al., 2020; Savage et al., 2020). Interactions between microglia and neurons, particularly synapses, can be identified by directly comparing appositions of microglial cell bodies and processes with synaptic elements (Savage et al., 2020; González Ibáñez et al., 2023), while insights into phagocytosis of different cargos such as synapses can further be obtained through examining the contents of microglial intracellular inclusions (Nahirney and Tremblay, 2021). EM can be further combined with immunostaining to glean essential information about the relationship between microglial structure and function. For instance, IBA1 immunostaining is commonly employed with EM to identify microglia in the CNS (Carrier et al., 2020). Notably, dark microglia, described above, were identified based on distinctive ultrastructural characteristics in EM (Bisht et al., 2016), but their reactive state and phagocytic activity have been supported by immunostaining, which demonstrates low expression of homeostatic CX3CR1 and P2RY12 and strong immunopositivity for TREM2 (Bisht et al., 2016; St-Pierre et al., 2020). These cells are a unique state associated with multiple pathological conditions including AD mouse models and human post-mortem AD brain samples (St-Pierre et al., 2020, 2022a). Microglial structural analysis has also been key in defining their involvement in AD, Huntington and Parkinson’s disease pathology, as well as in chronic stress, schizophrenia and neurodevelopmental disorders (El Hajj et al., 2019; Hui et al., 2020; Lecours et al., 2020; Savage et al., 2020; Bordeleau et al., 2021; González Ibáñez et al., 2023).
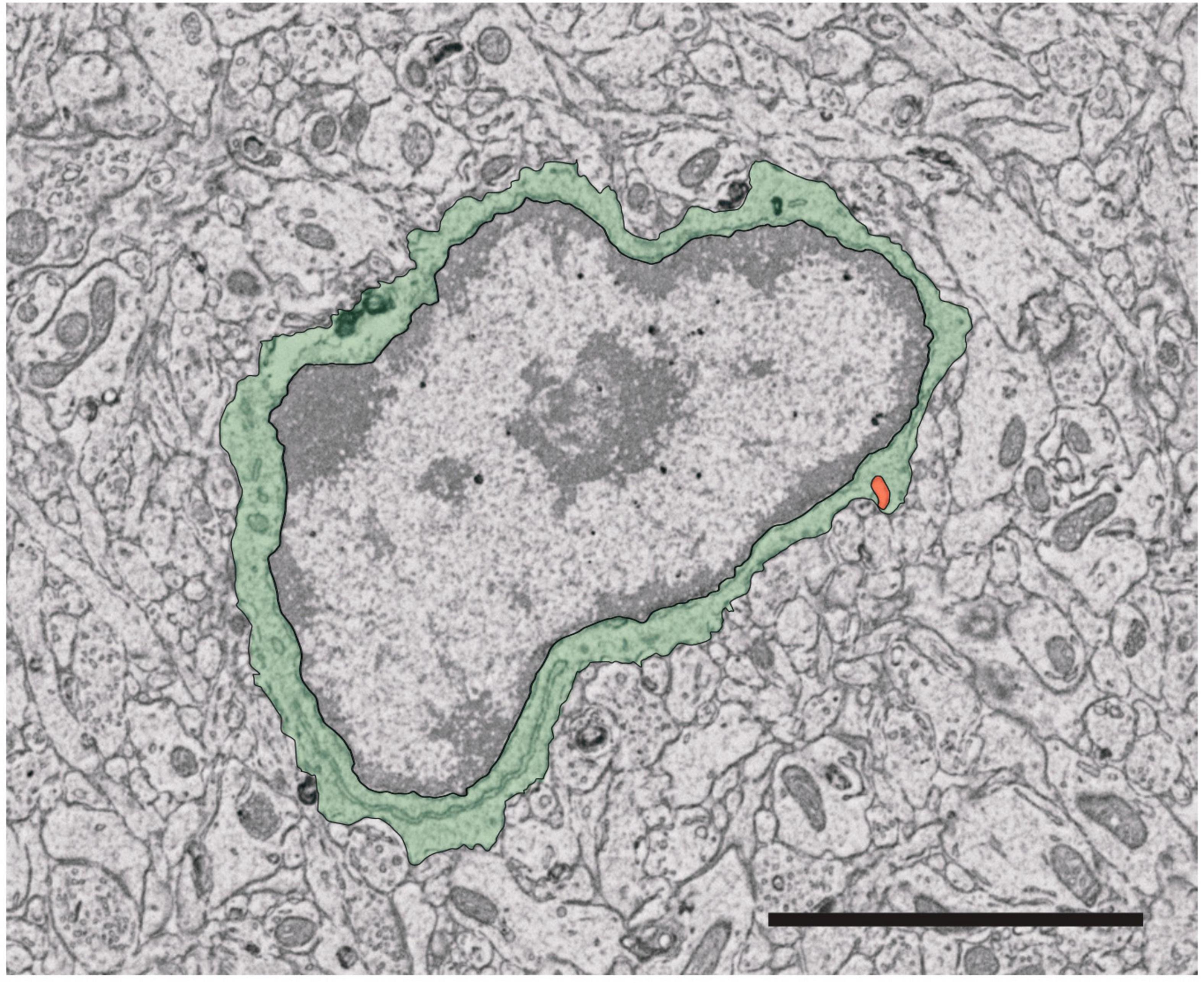
Figure 3. Identification of microglia for ultrastructural analysis. Microglia cell bodies in the mouse brain can be recognized based on key visual characteristics. The cell body shape varies widely between microglia but exhibits a more elongated and/or triangular shape compared to other brain cells (pseudocolored here in green). Furthermore, the cell nucleus (surrounded by the green pseudocolored cytoplasm) is characterized by two regions, heterochromatin (darker portion of the nucleus) and euchromatin (pale region of the nucleus). Certain intracellular organelles can also aid in identifying microglia, notably the presence of long stretches of endoplasmic reticulum cisternae and a phagosome (pseudocolored in orange). Scale bar: 3 μm.
Genetically modulating microglia with reporter models
First developed in the early 1980s, gene reporter models are versatile tools to visualize, characterize and isolate specific cell populations in vivo (Jefferson et al., 1986, 1987; Serganova and Blasberg, 2019). Through the expression of an easily detectable fluorescent reporter protein under the control of a microglia-specific promoter, reporter genes have provided key insights into the dynamics and activities of these cells (Jiang et al., 2008). Additionally, reporter models can be used to investigate the roles of microglia in various physiological and pathological processes, and to characterize the effects of genetic mutations or disease states on microglial functions (Nonnenmacher and Weber, 2012; Serganova and Blasberg, 2019).
Constitutive reporter models for microglial study
The advent of homologous recombination-based techniques has allowed researchers to place a reporter gene of interest into a microglia-specific locus, replacing the endogenously expressed allele. This has been used effectively to create mouse models expressing fluorescent reporters in place of myeloid or microglia-specific proteins such as CX3CR1 (Jung et al., 2000). Constitutive reporter models have proven immeasurably useful for the visualization, identification, and study of microglia through the targeting of countless microglial genes such as Cx3cr1 (Garcia et al., 2013), Tmem119 (McKinsey et al., 2020; Ruan et al., 2020), Sall1 (Buttgereit et al., 2016; Baba et al., 2019) and Hexb (Masuda et al., 2020). The intrinsic fluorescence of reporter molecules such as eGFP and tdTomato (tdT) eliminates the need for IHC and other techniques requiring cell fixation, allowing researchers to gain insights into microglial interactions with their surroundings in an in vivo context. However, a disadvantage to this approach is the potential presence of mutations incurred by the random insertion of the construct into the genome (Wieghofer et al., 2015).
Modern approaches extensively employ the Cre/loxP system, which allows cell type-specific gene inactivation or activation (Orban et al., 1992). In this paradigm, the bacteriophage-derived Cre recombinase is expressed under cell-specific control, and precisely and irreversibly excises a distal sequence of DNA flanked by upstream and downstream loxP sequences, known as the “floxed” sequence (Orban et al., 1992; Wieghofer et al., 2015). Cre can be expressed in a microglial locus, for instance, under the Cx3cr1 promoter (Parkhurst et al., 2013; Yona et al., 2013), in mice engineered to harbor a construct consisting of a strong constitutive promoter, often Rosa26, followed by a floxed STOP codon preceding a reporter gene such as YFP (abbreviated R26YFP) (Friedrich and Soriano, 1991; Wieghofer et al., 2015). In this example, Cre expression is governed by the endogenous regulation of Cx3cr1 and is therefore constitutively expressed in microglia and other myeloid cells that normally would highly express Cx3cr1 (Parkhurst et al., 2013; Yona et al., 2013). In CX3CR1+ cells, Cre excises the floxed STOP codon, leading to constitutive expression of the reporter under the control of the strong promoter. Hence, CX3CR1+ cells are labeled with the reporter gene of interest, enabling, for example, their identification via fluorescence microscopy or flow cytometry, or FACS-mediated isolation. Despite their utility, constitutive reporter models are limited by their property of labeling all the cells that express the gene of interest at any point during development, as Cre-mediated excision of the floxed STOP codon is irreversible. This leads to off-target cell labeling, potentially confounding results. For example, neurons transiently express Cx3cr1 during development and are labeled in Cx3cr1Cre-based constitutive models (Haimon et al., 2018; Zhao et al., 2019), highlighting the need to interpret the results of such models cautiously. This issue is resolved with inducible reporter systems, discussed below. Reporter models to study microglia are summarized in Table 2.
Inducible reporter models for microglial study
More recently, the advent of inducible Cre-based systems has revolutionized the field, enabling precise temporal labeling of specific cell populations and their progeny. This approach makes use of a modified estrogen receptor (ER, MER, ERT, or ERT2), which can bind the estrogen analog 4-hydroxytamoxifen but not endogenous estrogens (Littlewood et al., 1995; Metzger et al., 1995; Feil et al., 1997; Indra et al., 1999). Development of CreER or CreERT2 fusion proteins has enabled researchers to control when Cre expression is induced, thus allowing temporal-specific induction of reporter gene expression (Feil et al., 1997; Hayashi and McMahon, 2002). Since Cre-mediated excision of the floxed sequence is irreversible, reporter gene expression is maintained in all subsequent daughter cells following mitosis, enabling the tracking of specific cell populations and their progeny (Hayashi and McMahon, 2002; Wieghofer et al., 2015). Drawing on our earlier example of Cx3cr1, placing CreER under the control of Cx3cr1 (Cx3cr1CreER) and administering tamoxifen at a specific time in the mouse lifespan would induce constitutive reporter gene expression in all cells expressing Cx3cr1 at that timepoint, as well as in all progenies of those cells. This critical property of inducible Cre systems has allowed researchers to selectively deplete microglia, to precisely map microglial populations in a spatiotemporally controlled manner, and to identify key activities of microglia during development, homeostasis, and pathology (Zhao et al., 2019; Dai et al., 2022; Sahasrabuddhe and Ghosh, 2022), as discussed in the following sections.
However, it is important to note that inducible microglia-targeted CreER lines vary considerably in their stability, with a recent analysis showing that Cx3cr1CreER tools demonstrate particularly high rates of spontaneous recombination in the absence of tamoxifen, compared to Tmem119CreER- or HexbCreER-based lines (Faust et al., 2023). The authors also demonstrated, in some cases, reduced expression of the endogenous gene under the promoter driving CreER expression (Faust et al., 2023); as the genes used often encode essential microglial proteins, this could impair the function of Cre-targeted microglia and confound results. Although microglial gene expression is largely unaffected by tamoxifen, it was recently demonstrated that tamoxifen administration can itself lead to lower protein expression of P2RY12 (Faust et al., 2023). These issues represent important considerations when interpreting data from Cre-based microglial reporter, KO, and fate-mapping models.
Cre-based reporter systems are inherently limited by the specificity of the target gene for the cell type of interest. For example, inducible Cx3cr1-based systems, commonly used to target microglia, also affect CNS-associated and perivascular macrophages (Haimon et al., 2018; Masuda et al., 2020; Faust et al., 2023). To overcome this limitation, binary “split Cre” transgenic systems have been developed, requiring the cell of interest to simultaneously express two separate fragments (NCre and CCre) of the Cre recombinase enzyme placed under the control of different cell-specific promoters, which dimerize into a functional protein only when co-expressed (Jullien et al., 2003; Hirrlinger et al., 2009). In the context of microglia, this approach was leveraged using the clustered regularly interspaced short palindromic repeats (CRISPR)/Cas9 system to co-express NCre and CCre under the control of Cx3cr1 and Sall1, selectively targeting Cx3cr1+/Sall1+ microglia, but not Cx3cr1+/Sall1– infiltrating or vasculature-associated myeloid cells (Kim J.-S. et al., 2021). This enabled differential analysis of microglial and BAMs translatomes through a Cre-mediated RiboTag strategy (Kim J.-S. et al., 2021), highlighting strong potential of binary Cre systems for future use in high-specificity studies of microglial biology.
Microglial ablation: genetic and pharmacological approaches
As discussed, several methods can be used to achieve microglial visualization and provide a more complete understanding of these cells in the CNS (Tang et al., 2015). However, by depleting microglia, researchers can directly assess their role in a particular physiological or pathophysiological process by observing the consequences of their removal through targeted genetic and pharmacological ablation methods (Saxena et al., 2021).
The most target-specific depletion methods are gene KO models achieved by homologous recombination or, more recently, CRISPR-based genome editing, to excise a specific microglial gene (Albadri et al., 2017; Wang and Sun, 2019; Ayanoğlu et al., 2020; Damasceno et al., 2020; Krais and Johnson, 2020; Zuccaro et al., 2020). Although genome editing techniques offer many advantages, inherent limitations include the potential for instability at the altered site, as well as the possibility of unintended insertions and deletions (Doudna and Charpentier, 2014; Wang and Sun, 2019). On the other hand, pharmacological ablation allows the use of chemicals to eliminate or reduce the function of microglia. These drugs can be designed to block the activity of specific proteins or signaling pathways that are important for microglial survival or function (Stojiljkovic et al., 2022). It should be noted that these techniques are not without flaws, and their specificity and efficacy may vary depending on the context and the model used (Han et al., 2017). One important caveat to depletion methods that kill living microglia is their potential to exert non-specific effects on other CNS-cell types, such as astrocytes or BAMs, in response to dead or dying microglial cells (Elmore et al., 2014; Unger et al., 2018; Marino Lee et al., 2021).
Diverse proteins have been targeted for the depletion of microglia, including markers previously discussed in the context of visualization. The following section will focus on highlighting additional proteins that may be useful for this purpose.
Genetic depletion of microglial subsets
As a result of advances in gene editing techniques, methods of microglial depletion—based on KO animal models of essential genes in microglial survival and function—have provided a more comprehensive understanding into the roles of microglia in development, homeostasis, and pathology. Meanwhile, selective KO models of microglial genes across various contexts have helped us better understand the roles of specific genes and microglial states dependent on those genes.
Microglia, as well as monocytes and other macrophage populations, highly express the receptor for colony-stimulating factor-1 (CSF1R). CSF1R signaling through its ligands, CSF-1, and IL-34 (Wang et al., 2012), is essential for microglial development, survival, proliferation and release of proinflammatory factors (Mitrasinovic et al., 2005; Ginhoux et al., 2010; Erblich et al., 2011; Elmore et al., 2014; Green et al., 2020). As a result, CSF1R is a well-established target for microglial depletion. The loss of CSF1R in Csf1r–/– mice leads to nearly a complete lack of microglia, with > 99% depletion at E16 and postnatal day 1 (Ginhoux et al., 2010; Erblich et al., 2011). However, Csf1r–/– mice also show deficits in brain size and function, as well as some deficiencies of the olfactory bulb development, myelination and bone structure, leading to a premature death (Erblich et al., 2011; Green et al., 2020). It thus requires the use of inducible Cre/lox systems or pharmacological inhibitors of CSF1R (discussed below) to selectively deplete microglia in adult mice. Pons et al. (2021) used the Cre/loxP system to delete CSF1R in 3-month old mice, achieving a KO efficiency of 89%, and showed that loss of Csf1r in adulthood did not impact microglial survival. Intriguingly, deletion of Csf1r in adult APP-PS1 mice significantly ameliorated AD amyloid-beta pathology (Pons et al., 2021), highlighting the potential of this tool to elucidate the complex roles of microglia in neurodegenerative disease. Deletion of Csf1r regulatory elements, such as the fms-intronic regulatory element (FIRE), can also deplete microglia by selectively abolishing Csf1r expression in a cell- and tissue-specific manner (Rojo et al., 2019). Mice with CRISPR-mediated homozygous FIRE deletions completely lack microglia by IBA1 and P2RY12 immunostaining, as well as several other tissue-resident macrophage populations, but otherwise develop into healthy fertile adults without the systemic defects observed in Csf1r–/– mice. Notably, flow cytometry identified retention of CD11b+/CD45hi brain macrophages, which also co-expressed the perivascular macrophage marker CD169 by immunostaining (Rojo et al., 2019), suggesting a high degree of depletion specificity to microglia. Researchers have since crossed FIRE KO mice with the 5XFAD mouse model to study the effects of selectively lacking microglia in AD pathology, showing that the absence of microglia ameliorated amyloid-beta pathology but worsened cerebral amyloid angiopathy, which could be prevented by intra-hippocampal transplantation of wild-type microglial cells (Kiani Shabestari et al., 2022). The use of highly selective microglial depletion models holds great promise to elucidate microglial roles, and associated therapeutic targets, across diverse pathologies.
As previously discussed, the CX3CR1 receptor is a powerful tool to investigate neuron-microglia communication (Wolf et al., 2013), and Cx3cr1 KO models have been critical for our understanding of the fractalkine signaling pathway. Pagani et al. (2015) demonstrated that microglia-specific Cx3cr1 KO leads to acute deficits in the developing hippocampus, including impairments in the response to extrinsic ATP and altered electrophysiological properties. In mouse models of chronic stress, Cx3cr1 deficiency attenuated stress-induced alterations to microglial morphology and reduced phagocytosis (Milior et al., 2016), highlighting a key role for microglia-neuron communication in the response to stress. An additional method microglial depletion is achieved by placing the diphtheria toxin receptor (DTR) under the control of a microglial promoter using inducible Cre-loxP models, allowing for an efficient depletion when diphtheria toxin is administered. Using Cx3cr1CreER:R26iDTR mice, Parkhurst et al. (2013) selectively depleted Cx3cr1-expressing microglia to show a critical role for these cells in brain-derived neurotrophic factor-dependent learning and synaptic plasticity. A recent study used similar tools to assess the effects of depleting Cx3cr1+ microglia on kainic acid-induced status epilepticus (Wu et al., 2020). Depletion of microglia through inducible expression of diphtheria toxin, DTR or KO of the essential Csf1r in Cx3cr1+ cells, led to exacerbated disease pathology, increased mortality and enhanced neurodegeneration (Wu et al., 2020). However, as Cx3cr1 is also expressed on infiltrating and border-associated myeloid cells, future studies would benefit from employing more selective depletion tools such as binary Cre-based systems targeting multiple co-expressed microglial genes (Kim J.-S. et al., 2021).
Triggering receptor expressed on myeloid cells-2 (TREM2) is essential for microglial synaptic pruning and formation of normal neural circuitry during brain development by mediating phosphatidylserine-dependent phagocytosis of apoptotic neurons (Filipello et al., 2018; Scott-Hewitt et al., 2020). It is also involved in the microglial response to injury and in the recognition of soluble factors (Quan et al., 2009; McQuade et al., 2020). Microglial TREM2 is heavily implicated in disease and is required for the transformation into DAM, MgND and other pathology-associated states (Keren-Shaul et al., 2017; Krasemann et al., 2017; Deczkowska et al., 2018). TREM2 is selectively expressed on microglia within the CNS, but is found on other myeloid-derived cells such as macrophages/monocytes, dendritic cells and granulocytes in the periphery (Yaghmoor et al., 2014; Gratuze et al., 2018). Soluble forms of TREM2 increase microglial survival and proliferation and induce morphological changes during inflammatory responses (Zhong et al., 2017). Furthermore, TREM2 variants in humans have been strongly implicated in AD risk (Guerreiro et al., 2013; Jonsson et al., 2013; Sims et al., 2017). Genetic Trem2 deletion models have greatly contributed to our knowledge of its normal functions and implications in microglial biology, generally implicating TREM2 as a critical mediator of phagocytosis in development and pathological states. Trem2–/– mice show decreased microglial numbers in the CA1 region of the hippocampus with reductions in reactive microglial morphologies, increased synaptic density and overactive excitatory neurotransmission, highlighting the importance of Trem2 in the removal of extraneous synapses during neural development (Filipello et al., 2018). These deficits are recapitulated in human iPSC-derived microglia in vitro, which following CRISPR-mediated deletion of TREM2, are unable to phagocytose apolipoprotein E or fibrillar amyloid-beta, and present impaired survival in response to stress (McQuade et al., 2020). In line with this, 5XFAD mice deficient in Trem2, or its downstream mediator Syk, display an impaired amyloid-beta plaque clearance by microglia (Wang et al., 2015, 2022), and do not develop DAM (Keren-Shaul et al., 2017; Wang et al., 2022). Similarly, in a humanized mouse model of Tau pathology, crossed mice lacking Trem2 presented augmented Tau aggregation and phosphorylation with altered morphology in IBA1+ microglia by IHC (Bemiller et al., 2017). TREM2 is also expressed on plaque-associated dark microglia by EM in the APP-PS1 model of AD pathology (Bisht et al., 2016), suggesting a role for dark microglia in amyloid-beta phagocytosis. However, selective deletion of Trem2 in microglia leads to an altered transcriptional profile and phenotype, temporarily improving disease in mouse models of traumatic spinal cord injury and experimental autoimmune encephalomyelitis (Gao et al., 2023). Overall, Trem2 KO models have enabled the selective depletion of pathologically relevant microglial populations, shedding light on the diverse roles of microglia in disease.
In a similar fashion, C-type lectin domain family member 7a (CLEC7a), also known as DECTIN-1, is expressed on cells of the monocyte lineage and functions in the recognition and phagocytosis of fungal and bacterial pathogens (Brown et al., 2002). CLEC7a was also shown to play a role in cancer progression through its influences on tumor-associated macrophages (Daley et al., 2017). CLEC7a is expressed in microglia, where it promotes phagocytosis upon ligation (Shah et al., 2008). CLEC7a signaling directly activates SYK-mediated pathways downstream of TREM2, enhancing phagocytosis and representing a potential therapeutic target for individuals with deficits in TREM2 function (Wang et al., 2022). In mouse models of AD pathology, brain Clec7a is significantly upregulated in DAM and MgND states (Keren-Shaul et al., 2017; Krasemann et al., 2017; Deczkowska et al., 2018). CLEC7a is also upregulated by microglia in mouse models of experimental autoimmune encephalomyelitis (Krasemann et al., 2017; Deerhake et al., 2021), with Clec7a–/– mice displaying more severe disease, although these effects were more likely mediated by CNS-infiltrating myeloid cells than microglia (Deerhake et al., 2021). These examples highlight the potential for CLEC7a KO models to continue to enhance our understanding of microglial roles in disease conditions.
Pharmacological depletion of microglia
The pharmacological depletion of microglia in the CNS has been a valuable and accessible tool in order to define their role across contexts of health and disease, with the advantage of being less challenging than generating and utilizing a KO model (Barnett et al., 2021). This has been made possible due to a vast array of compounds that demonstrate the capacity to modulate microglial numbers and control their activities, notably in mediating CNS inflammation (Barnett et al., 2021).
As discussed, the use of CSF1R inhibitors is an emerging technique with demonstrated efficacy in the ablation of microglia in the CNS (Elmore et al., 2014; Fujiwara et al., 2021). The CSF1R inhibitors, such as PLX3397 and PLX5622, are CNS-permeable and selectively inhibit tyrosine kinase receptors on macrophages and microglia, although the specificity of these inhibitors varies depending on the specific compound used (Green et al., 2020; Hupp and Iliev, 2020), with PLX5622 recently shown to exert effects on peripheral bone marrow and tissue-resident macrophages (Lei et al., 2020). CSF1R inhibitors are orally bioavailable, but their routes of administration can also include intravenous or subcutaneous injection (Hupp and Iliev, 2020). Moreover, CSF1R inhibitors have been shown to reduce microglial proliferation, depleting the majority of the brain population (Elmore et al., 2014; Acharya et al., 2016; Fu et al., 2020). It has been reported that most of IBA1+ microglia are eliminated within the first week of administration—as assessed with staining against caspase-3, a marker for cell apoptosis. Approximately 90% of microglia can be eliminated following 7 days of PLX3397 treatment, shown using flow cytometry in whole brains of CX3CR1-GFP+/– adult mice (Elmore et al., 2014). Effects remain with sustained administration of CSF1R inhibitors—for instance, after 21 days of PLX3397 treatment (Elmore et al., 2014). However, microglial repopulation is notable between drug withdrawal and the 3-day recovery time point. This highlights the critical period of 48–72 h for microglial repopulation after CSF1R inhibition (Elmore et al., 2014; Henry et al., 2020; Shi et al., 2022). PLX3397 has undergone exploration in humans; however, its efficacy has not yet achieved that observed in mice. In a phase II clinical trial for recurrent glioblastoma, a daily oral dose of 1,000 mg of PLX3397 was well-tolerated and successfully crossed the blood-brain barrier of 37 patients (mean age of 58.5; 25 males and 12 females). Nevertheless, it demonstrated no effectiveness in reducing tumor size or microglial density within the tumor tissue. IHC for IBA1 was conducted and used to quantify cell numbers and staining intensity. No statistically significant differences were observed in IBA1+ microglia between pre-study (archival) and on-study (surgical) samples, whether treated with PLX3397, following the standard of care with radiation therapy complemented with temozolomide chemotherapy, or when compared to historical control samples (Butowski et al., 2016).
Interestingly, the elimination of microglia using CSF1R inhibitors was reported to prevent amyloid-beta plaque formation and disease progression in the 5XFAD and APP-PS1 mouse models of AD pathology (Olmos-Alonso et al., 2016; Spangenberg et al., 2019), and to reduce accumulation of pathogenic Tau in the Tg2541 tauopathy model, extending survival of female but not male mice (Johnson et al., 2023). Likely microglia, BAMs have the fully capacity to repopulate via local self-renewal after depletion via PLX3397 (Van Hove et al., 2019). These studies highlight CSF1R as a potential target to deplete microglia in therapeutic approaches for neurodegenerative diseases.
Minocycline, a drug initially developed to treat bacterial infections, has been shown to control and deplete inflammatory cells in the CNS (Garrido-Mesa et al., 2013; Scholz et al., 2015). Recently, it was reported that tetracyclines not only display anti-microbial activity, but also act as anti-inflammatory and anti-apoptotic agents while impairing proteolysis and angiogenesis (Bernier and Dréno, 2001; Scholz et al., 2015). Moreover, minocycline has emerged as a potential neuroprotective agent in experimental models for several CNS disorders, such as brain ischemia (Yrjänheikki et al., 1998), brain injury (Sanchez Mejia et al., 2001), Parkinson’s disease (Du et al., 2001; Thomas and Le, 2004), Huntington disease (Chen et al., 2000) and AD (Choi et al., 2007). Notably, minocycline appears to exert an anti-inflammatory influence on microglia (Bassett et al., 2021; Long et al., 2023). To exemplify, in a mouse model of subarachnoid hemorrhage (SAH), minocycline treatment was administered daily until day 7 post-SAH and continued every second day until day 14. IHC for IBA1 and a functional phagocytosis assay, employing glucose-coated beads cross-linked with a lipid bilayer and co-localized with IBA1+ cells in brain slices, indicated a significant reduction in the phagocytic activity of microglia/macrophages. This reduction was associated with decreased spatial interaction with neurons (NeuN+) and a decline in neuronal apoptosis (TUNEL+/NeuN+) (Blecharz-Lang et al., 2022).
The efficiency of minocycline in depleting microglia can vary depending on the specific conditions of the study, such as the dosage, route of administration and duration of treatment, however, overall it has been shown to be a relatively effective method to modulate microglial populations, typically achieving 50–90% depletion (Blecharz-Lang et al., 2022). However, minocycline also exhibits broad off-target effects in the CNS, and thus results from minocycline-based depletion studies should be considered with caution (Strahan et al., 2017).
Likewise, clodronate-containing liposomes (Clod-Lips) constitute an effective approach to deplete macrophage-like cells across tissues (Rooijen and Sanders, 1994; Moreno, 2018). Initially discovered as an osteoclast inhibitor, clodronate, following phagocytosis and entry into the macrophage cytosol, perturbs iron metabolism and inhibits mitochondrial respiration through its actions as an ATP analog, leading to apoptosis (Lehenkari et al., 2002; Moreno, 2018; Opperman et al., 2019). The efficiency of clodronate depends on its route of administration; intrarectal and intraperitoneal administration deplete around 50% of the macrophage population, while 90% can be achieved through the intravenous route (Weisser et al., 2012). A major drawback to Clod-Lips is their requirement for direct infusion into the CNS, as well as their demonstrated off-target effects on blood vessels (Han et al., 2019). Similarly, Takagi et al. (2020) demonstrated that Clod-Lips markedly attenuated the activation of mouse brain circuits for TLR2-mediated inflammation in a hypothermia mouse model.
Mac-1-saporin (Mac-1-sap), a chemical conjugate of a CD11b monoclonal antibody and the ribosome-inactivating protein saporin, is another pharmacological option for microglial ablation that has been shown to deplete CD11b+ cells in mouse models (Acharjee et al., 2018). Although not specific to microglia, targeting all CD11b+ cells, intrathecal injection of Mac-1-sap depletes around 50% microglia in the CNS (Yao et al., 2016). Following depletion, repopulating microglia were shown to respond to injury, re-establishing normal function after ablation (Yao et al., 2016). In vitro work further investigated the depletion of microglia from mouse hippocampal cultures in a model of ischemia-like oxygen-glucose deprivation. In this context, seven-day treatment with Mac-1-sap drove neural apoptosis and astrogliosis one day after oxygen-glucose deprivation (Montero et al., 2009), supporting the importance of microglia in the response to ischemic injury.
Fate mapping: tracking microglia through development, health, and disease
In general, fate mapping approaches aim to mark specific subsets of cells at a given point in time, allowing researchers to track the spatial and temporal distribution, roles, and dynamics of these cells and their progeny. Early work by Lawson et al. (1992) employed radioactive [H-3]-thymidine pulses, observing that F4/80+ microglia incorporated labeled nucleotides to synthesize new DNA and proliferated to produce new microglia in situ. Modern experiments have used similar pulse-chase approaches to characterize microglial proliferation and turnover under steady-state conditions, showing that microglia are a dynamic, actively self-renewing population (Askew et al., 2017). However, most fate mapping studies approaches have focused on mouse models that genetically target microglia, with molecular markers increasing in both sensitivity and specificity over the years in tune with our rapidly advancing understanding of microglial gene expression profiles (Tay et al., 2017). Recent advances in microglia-specific genetic fate mapping technologies have greatly enhanced our understanding of microglial origin, dynamics in development and homeostasis, and functions in pathology. Importantly, modern fate mapping studies have enabled researchers to precisely disentangle the roles of microglia from other myeloid populations in the CNS and have revealed great heterogeneity within the microglia themselves.
Microglial fate mapping during development
Several important microglial fate mapping tools were developed specifically aiming to decipher the origin of microglia. In these approaches, early embryonic progenitor cells are labeled based on specific gene expression patterns during development, and their progeny is followed to identify the progenitor cells giving rise to microglia and the key transcription factors regulating these processes. In a landmark study, Ginhoux et al. (2010) used fate-mapping techniques to determine that microglia develop from yolk sac-derived myeloid progenitors that arise prior to E8 in mice. In this study, Cre was co-expressed with Runx1, a transcription factor expressed in early hematopoietic progenitors, in mice harboring a Rosa26-fl-STOP-fl-YFP construct (Runx1MerCreMer:R26YFP). Tamoxifen administration at E7.5 labeled all subsequent microglia as YFP+, demonstrating that microglia develop from yolk sac-derived Runx1-dependent progenitors (Ginhoux et al., 2010). This finding was later confirmed using a similar approach (Hoeffel et al., 2015), and Runx1-based fate mapping was again employed to show that retinal microglia share a common yolk-sac origin with brain microglia (O’Koren et al., 2019). Additional research further described microglial development by co-expressing CreER with CSF1R, a constitutively expressed receptor in microglia, monocytes, and macrophages, in R26YFP mice. Using tamoxifen administration to induce YFP labeling at different time points in development further revealed that microglia develop independently from bone-marrow hematopoietic stem cells (Schulz et al., 2012; Gomez Perdiguero et al., 2015; Hoeffel et al., 2015). Another study took advantage of the transcription factor Kit, expressed in early yolk sac progenitors, using KitMerCreMer:R26YFP mice to show that only microglia, along with a subset of Langerhans cells, develop from early (E7.5) Kit+ erythromyeloid progenitors in the yolk sac (Sheng et al., 2015). Thus, microglial fate mapping tools have been crucial to building our current knowledge of microglia as an ontogenetically distinct macrophage lineage.
Microglial fate mapping during adult homeostasis
Several key questions in modern microglial biology relate to dissecting the roles of microglia from other CNS myeloid cells and distinguishing among heterogeneous states within the microglia. Fate mapping studies have been critical in addressing these fundamental concepts. O’Koren et al. employed a combination of fate mapping and 12-color flow cytometry to definitively distinguish between retinal microglia and monocyte-derived macrophages, which both express CX3CR1, in CX3CR1YFP–CreER:R26RFP mice. Following tamoxifen administration, both lineages expressed RFP, however, short-lived monocyte-derived macrophages were replaced by RFP– cells over a subsequent “washout” period, while long-lived microglia retained expression of RFP (O’Koren et al., 2016). Using a similar mouse model, the time course of microglial and macrophage infiltration into the various compartments of the eye was recently mapped at high spatiotemporal resolution (Wieghofer et al., 2021). More recently, researchers identified prominin 1 as a marker of committed myeloid progenitors in the adult CNS under homeostatic conditions, and employed a novel fate mapping approach using Prom1CreERT2:R26tdT mice to demonstrate that Prominin-1 (Prom1)+ progenitors also contribute to microglial proliferation during homeostasis (Prater et al., 2021).
Heterogeneity within the microglial population is an emerging concept that, until recently, could not be explored with available fate-mapping tools. To address this issue, Tay et al. (2017) created the “Microfetti” mouse strain, a cross between the existing CX3CR1CreER line and a R26Confetti strain, which contains a four-color reporter system. In Microfetti mice, tamoxifen administration causes random expression of one of four fluorescent reporters in each cell thus creating up to ten distinct color combinations in the homozygous state (Tay et al., 2017). Using mathematical models to evaluate the likelihood of spatial association of same-color cells due to chance versus cell division, the authors achieved detailed tracing of microglial cells and their progeny at single-cell resolution. This revolutionary approach revealed that microglial proliferation is a stochastic process governed by regional environmental cues during homeostasis (Tay et al., 2017).
Microglial fate mapping during pathology
Microglia, as the innate immune cells of the CNS, play diverse and complex roles in the response to tissue injury, infection and in chronic pathological states. Fate mapping studies have allowed researchers to discern the distinct roles of microglia, BAM subsets, and infiltrating peripheral myeloid cells. For example, the source of repopulating microglia following experimental CSF1R-mediated depletion has been extensively debated. Huang et al. (2018) employed a fate mapping approach to address this issue. Using CX3CR1CreERT2:R26tdT mice given tamoxifen early in postnatal life, they showed that all repopulating cells were tdT+ and were thus exclusively derived from surviving microglia, rather than peripheral monocytes (Huang et al., 2018). Subsequent research using a similar fate mapping model has traced these repopulating microglia to a MAC2+ progenitor population with an immature gene expression signature that displayed resistance to CSF1R inhibition (Zhan et al., 2020). Other groups have employed comparable techniques to replicate these results in the retina (Zhang et al., 2018; O’Koren et al., 2019).
Single-cell-resolution fate mapping, using the CX3CR1CreER:R26Confetti model described above, showed that microglia proliferate in waves of clonal expansion under pathological conditions, and that homeostasis is restored through both migration away from the site of pathology and apoptosis (Tay et al., 2017). A recent study mapped the dynamics of microglial populations in an inducible mouse model of AD pathology (Wu et al., 2021). Using a KitMerCreMer:R26YFP system with tamoxifen-mediated YFP induction periodically over 8 months, microglia, BAMs and parenchymal macrophages were shown to remain relatively stable throughout disease progression, while other myeloid cells were rapidly and continuously replaced by bone marrow-derived monocytes (Wu et al., 2021). In cuprizone-induced demyelination, fate mapping using CX3CR1CreER–iresGFP:R26dsRed mice also revealed that microglia, not bone marrow-derived macrophages, are the primary cells involved in the remyelination response (Marzan et al., 2021).
Fate mapping in mouse models of retinal pathology have also provided intriguing findings. In a CX3CR1CreERT2:R26tdT mouse model of retinal ischemia-reperfusion, proliferation of resident microglia and recruitment of peripheral monocytes were found to play similarly important roles in the inflammatory response (Ahmed et al., 2017), while a study in a similar model of optic nerve crush injury found that microglia, not peripheral monocytes, were the primary cells recruited to the site of injury (Heuss et al., 2018).
A comparison of common microglial fate mapping systems
As diverse microglial fate mapping systems have proven useful for the analysis of microglial dynamics in development, homeostasis and disease, data has become available on the efficacies of various mouse strains in targeting microglia. Following the advent of Cx3cr1Cre-based models, their specificity for microglia was assessed and directly compared to earlier Lyz2Cre and Cd11cCre mice, through direct crosses to R26YFP strains (Goldmann et al., 2013). While Cx3cr1Cre-targeted microglia with higher sensitivity and specificity than Lyz2Cre or Cd11cCre, recombination was also observed in CX3CR1+ infiltrating peripheral myeloid cells. However, long-lived microglia, which retained a stable expression of YFP, could still be distinguished after a washout period of several weeks, while short-lived peripheral CX3CR1+ monocytes turned over rapidly and were replaced by YFP– cells (Goldmann et al., 2013). Similar studies validated the utility of this approach as a means of distinguishing CX3CR1+ infiltrating monocytes and dendritic cells from resident parenchymal microglia (O’Koren et al., 2016; Mrdjen et al., 2018; Jordão et al., 2019), although it has been noted that some BAM subsets, such as those residing in the perivascular spaces meninges and CP, are replaced more slowly than other subsets and thus cannot be readily distinguished through this approach alone (Mrdjen et al., 2018; Jordão et al., 2019). Later work also established that many BAM subsets, in fact, express CX3CR1 (Goldmann et al., 2016). Accordingly, off-target recombination in non-parenchymal CNS macrophages is observed in CX3CRCreER systems (Chappell-Maor et al., 2020; Faust et al., 2023).
Recent advances in single-cell transcriptomics have identified a battery of novel microglia-enriched genes, several of which have been leveraged to generate Cre-based tools with applications to fate mapping. Examples include Sall1 (Buttgereit et al., 2016), Tmem119 (Kaiser and Feng, 2019), P2ry12 (McKinsey et al., 2020), and Hexb (Masuda et al., 2020). Sall1CreER targets microglia with high sensitivity and specificity, notably showing no recombination in CNS-infiltrating peripheral myeloid cells and nearly all non-parenchymal BAMs with the exception of a small subset of CP BAMs (Buttgereit et al., 2016; Van Hove et al., 2019; Chappell-Maor et al., 2020). However, Sall1 is a critical regulator of microglial homeostasis (Buttgereit et al., 2016), likely rendering this approach less suitable for microglial fate mapping in pathological states. Furthermore, off-target recombination was recently reported in neuroectoderm-derived CNS cells in Sall1CreER mice (Chappell-Maor et al., 2020). Likewise, the Tmem119CreERT2 line (Kaiser and Feng, 2019) labeled adult microglia with high specificity, although some recombination was noted in leptomeningeal macrophages and other non-IBA1+ cells thought to be perivascular or meningeal fibroblasts (Kaiser and Feng, 2019; McKinsey et al., 2020). The P2ry12CreER mouse line was developed with the goal of preserving endogenous expression of the functional P2RY12 receptor, which plays important roles in nucleotide-sensing during the microglial response to injury, seen as a benefit in contrast to the reduced expression of Sall1 in Sall1CreER knock-in strains (McKinsey et al., 2020). The P2ry12CreER specifically and efficiently labeled embryonic as well as adult microglia, offering an advantage over the Tmem119CreERT2 construct, which mediated off-target recombination in CD31+ endothelial cells during development (Kaiser and Feng, 2019; McKinsey et al., 2020). No off-target recombination was observed in perivascular macrophages or in circulating blood cells, although a subset of CP macrophages were labeled, possibly corresponding to the microglia-like Kolmer’s epiplexus BAMs described by Van Hove et al. (2019) and McKinsey et al. (2020). However, like Sall1, Tmem119, and P2RY12 are strongly associated with homeostatic microglial signatures (Kaiser and Feng, 2019; McKinsey et al., 2020). In fact, it was proposed that Tmem119-based systems could potentially be studied as sensors of disease states, as the expression of this gene may be perturbed under pathological conditions (Ruan et al., 2020). Hexb-based genetic tools have also recently been developed (Masuda et al., 2020). Massively parallel single-cell RNA-sequencing identified Hexb as a highly enriched gene in microglia that, unlike Tmem119, Sall1, or P2ry12, is consistently expressed across conditions of homeostasis and disease. HexbCreERT2:R26YFP mice showed highly specific recombination in microglia, with distinct advantages over Cx3cr1- and Sall1-based systems (Masuda et al., 2020). However, although fate mapping validation experiments showed that HexbCreERT2 did not label other CNS macrophage subsets or infiltrating peripheral monocytes, a measurable subset of microglia were not labeled (Masuda et al., 2020). Additionally, a recent study demonstrated that Tmem119CreER and HexbCreER lines show low recombination efficiency relative to the established Cx3cr1CreER based tools (Faust et al., 2023). Recombination efficiency was shown to depend on the genetic distance separating the two loxP sites, with shorter inter-loxP distances promoting efficient recombination (Faust et al., 2023). Thus, further optimization of Tmem119-, Sall1-, Hexb-, P2ry12-, and Cx3cr1-based tools using evidence-driven methods will likely be required to exploit their full potential.
The previously described CX3CR1CreER:R26Confetti model offers a unique advantage in that fate mapping can be achieved with single-cell resolution (Tay et al., 2017). This has allowed detailed analyses of microglial dynamics under homeostatic and pathological conditions, greatly enhancing our ability to examine heterogeneity within the microglial population and track the migration and proliferation of individual cells (Tay et al., 2017). In the context of a recent explosion of microglial single-cell transcriptomics studies, approaches of this nature hold great promise as key techniques in the study of microglia at an unprecedented resolution.
Translational applications
In contrast to animal models, visualization of microglia in the living human brain remains a significant challenge. There are, however, several emerging techniques (Janssen et al., 2018), which can enable the study of microglial dynamics in living humans (Figure 4). A powerful technique used in clinical and fundamental research to study brain immunity is positron emission tomography (PET) (Gerhard et al., 2003; Di Biase et al., 2017; Hu et al., 2020). PET utilizes the binding of radioactive isotopes to a target molecule to visualize and quantify that molecule (Berger, 2003). PET has already highlighted the activity of microglia in contexts of neurodegeneration, inflammation and neurodevelopmental disorders, as measured using the translocator protein (TSPO) as a target (Yankam Njiwa et al., 2017; Hu et al., 2020; Lavisse et al., 2021; Seelaar and Van Swieten, 2021; Simpson et al., 2022; Vainio et al., 2022). Furthermore, microglia were shown to be highly active in people with schizophrenia using TSPO, as the upregulation of TSPO correlates with higher mitochondrial activity (Conen et al., 2021). However, TSPO is not specific to microglia, having been identified on astrocytes, endothelial cells and neurons and has been shown to vary considerably among brain regions (Daugherty et al., 2013; Betlazar et al., 2018; Gong et al., 2019). TSPO also demonstrates greater selectivity for microglia and distinct expression kinetics during inflammation in rodents compared to humans (Nutma et al., 2021a,b), limiting the translational potential of TSPO-based findings to the clinic. To address these issues, new ligands have been developed to target microglial proteins already common in animal research such as P2RY12, TREM1 and CSF1R, increasing the correlative research potential of the technique (Berdyyeva et al., 2019; Chaney et al., 2019, 2020; Zhou et al., 2021; Coughlin et al., 2022).
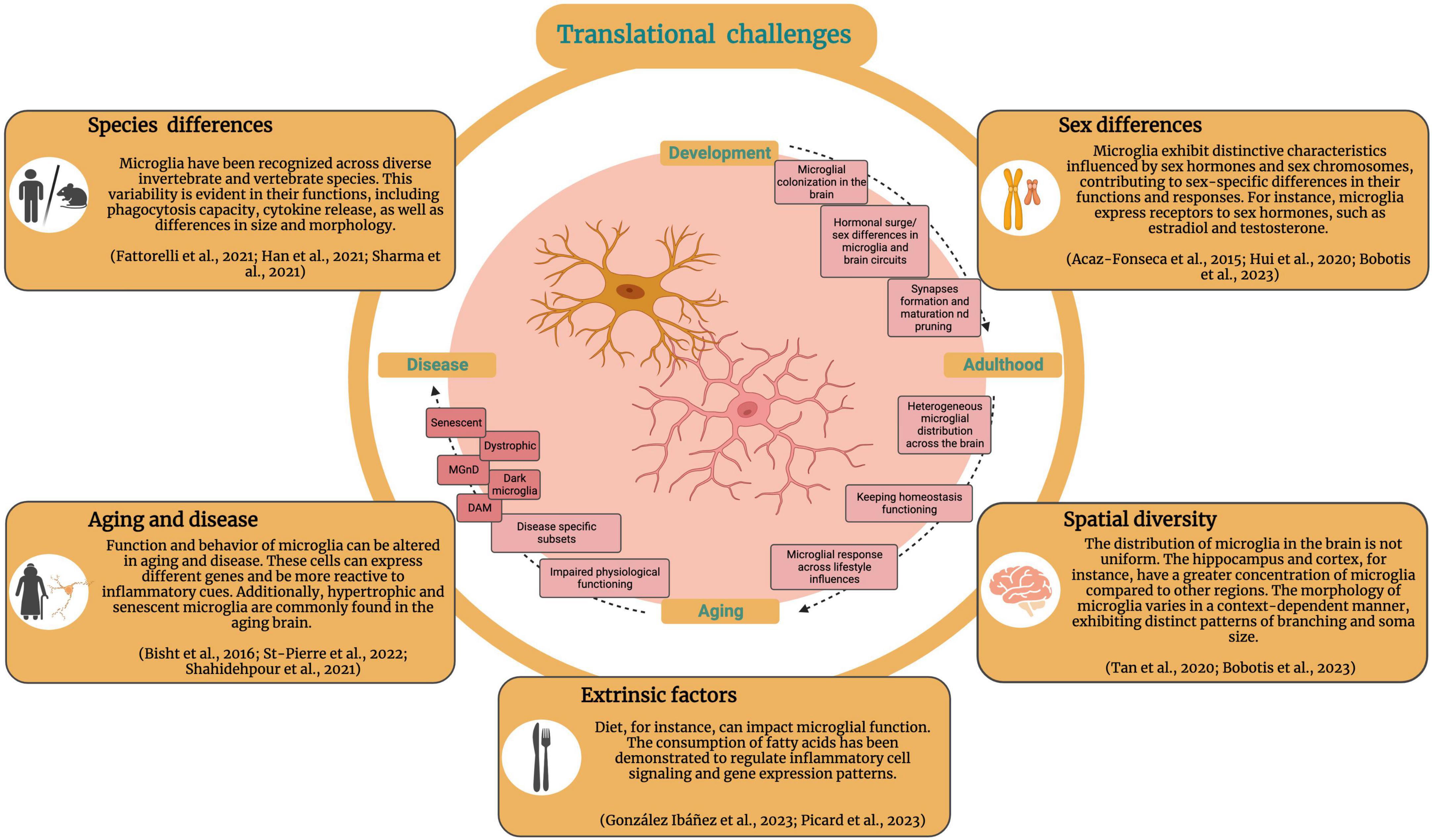
Figure 4. Translational applications for the study and targeting of human microglia. Current methods and associated challenges for the study of human microglia and translation of microglia-targeted therapies to the clinic. Created with BioRender.com.
Diffusion-weighted magnetic resonance imaging (DW-MRI), which leverages the random motion of water molecules to obtain high-resolution microimaging, is emerging as a promising alternative strategy to PET for microglial study in vivo. DW-MRI has already been shown to detect changes in microglial proliferation, density morphology in living mice and rats (Yi et al., 2019; Garcia-Hernandez et al., 2022). Thus, DW-MRI could feasibly be applied as a non-invasive approach for the clinical study of microglia in living humans and remains an exciting field of research.
Methods to study human microglia ex vivo have also gained traction in recent years, with brain tissue samples obtained perioperatively from living donors yielding novel insights into human microglial phenotypes (Milior et al., 2019). In a sample of nine patients undergoing neurosurgery for refractory epilepsy, Morin-Brureau et al. (2018) provided a detailed characterization of the brain region-specific diversity of microglial morphologies and phenotypic marker expression. Ex vivo two-photon live imaging studies can also yield insights into human microglial dynamics, and have aided in elucidating P2RY12/13-dependent responses to ADP stimuli or tissue damage (Morin-Brureau et al., 2018; Milior et al., 2020). This cohort also provided a unique opportunity to study the correlation between seizure timing and frequency with microglial phenotype, with inferential data suggesting transient proinflammatory cytokine release in the microglial response to seizure (Morin-Brureau et al., 2018). Although access to brain tissue samples from living humans remains a challenge, the rapidly advancing study of ex vivo microglial dynamics promises to clarify roles for microglia in human pathologies, elucidating therapeutically relevant differences between microglial function in humans and animal models.
Finally novel in vitro models can assist in the study of human microglia, such as the differentiation of microglia from human induced pluripotent stem cells (Cuní-López et al., 2022). This additional in vitro model can be useful for recapitulating the features of authentic human microglia and enabling the cells to mature under CNS-derived conditions (Cuní-López et al., 2022). Plenty of protocols are emerging (Rustenhoven et al., 2016; Speicher et al., 2019), from primary to pluripotent stem cell-derived culture, and early results are beginning to elucidate a diversity of new mechanisms of human microglial function (Haenseler et al., 2017; Bassil et al., 2021; Popova et al., 2021; Dräger et al., 2022; Banerjee et al., 2023; Dolan et al., 2023).
Discussion
Overall, research has progressed greatly since the initial description of microglia using silver staining in brightfield microscopy (Sierra et al., 2019). Significant advances in the methods used to study microglia have made it possible to characterize in detail the microglial sensome, define microglial subsets and their distinct phenotypic markers and elucidate the functional roles of diverse microglial genes and proteins in health and disease.
Antibody-mediated identification of microglia has evolved substantially as the diversity and complexity of these cells has been increasingly recognized. A key challenge to overcome has been to define the specificity of protein markers for microglia relative to other CNS-associated macrophages and non-immune cells, as well as their context-dependence and regulation during inflammation and pathology. The combination of multiple techniques, such as high-dimensional flow cytometry, FACS, and IHC has enabled increasingly detailed phenotyping of microglia, and our understanding of the sensitivity and specificity of microglial markers continues to grow. The advent of single-cell transcriptomics has enabled increasingly granular analysis of microglial phenotypes, with a particularly high volume of data in the contexts of development and neurodegeneration (Keren-Shaul et al., 2017; Krasemann et al., 2017; Hammond et al., 2019; Li et al., 2019a; Olah et al., 2020), and is already yielding valuable tools for the antibody-mediated identification of specific functional subpopulations as well as the broad visualization of microglia across diverse spatial and temporal settings.
In parallel, sophisticated modern imaging techniques have granted researchers the capacity to image microglial dynamics at a single-cell resolution through two-photon in vivo imaging and gain unprecedented insights into microglial ultrastructure and their interactions with neighboring cells and the extracellular environment through EM (Maco et al., 2014; Nahirney and Tremblay, 2021). These techniques will continue to enhance our understanding of the relationship between microglial structure and function, complementing the large body of work leveraging microglial markers to study functional properties.
Selective microglial depletion is becoming increasingly accessible as our understanding of the roles and specificity of microglial genes and proteins continues to grow. These studies have shed light on the critical functions of microglia as a whole, as well as their individual components, across an expanding list of disease settings. Studies investigating the highly specific genetic or pharmacological depletion of increasingly relevant microglial phenotypes promises to advance microglia-targeted therapies for currently untreatable diseases, as exemplified by recent elucidations of the roles of Trem2, Apoe, Clec7a and others in neuropathological conditions (Wang et al., 2015, 2022; Krasemann et al., 2017; Deerhake et al., 2021).
Gene reporter and fate-mapping systems have gradually improved as models to investigate microglial development, homeostatic function and roles in pathology. Cre-based systems have often been limited by sparse knowledge of microglial and myeloid gene expression patterns and inherent flaws such as spontaneous recombination or low recombination efficiency (Chappell-Maor et al., 2020; Van Hove et al., 2020; Faust et al., 2023). However, as our understanding of microglial markers has improved, so too has our ability to exploit these markers to selectively label, visualize and track microglia. As recently demonstrated, the application of single-cell RNA-sequencing could be a promising approach to identify future genetic targets with high specificity to microglia (Masuda et al., 2020). As more options for microglial reporter systems continue to emerge, systematic comparisons of the efficiency, selectivity and generalizability of these models have been and will be critical in aiding researchers to confidently select the most appropriate reporter model for their application (Faust et al., 2023). With recent advances enabling the visualization of individual microglial cells (Tay et al., 2017) and selectively studying microglia with high specificity (Kim J.-S. et al., 2021), this area of research holds high potential to answer some of the most essential questions in microglial biology.
The translation of microglia-targeted therapies remains to the clinic remains limited to date, and characterizing microglial phenotypes and functions in humans will be critical to achieve this. Species-specific differences may greatly impact the translation of microglia-targeted therapies (Fattorelli et al., 2021), and more work is needed to understand human microglial gene expression and function in order to modulate these for therapeutic purposes. Consideration must also be given to factors such as sex in the context of clinical translation, as microglial density, size, phagocytic activity, morphology and target marker expression can vary between sexes (Bobotis B. C. et al., 2023), and this is also species-dependent (Han et al., 2021; Sharma et al., 2021). For instance, sexual dimorphism is observed in diseases such as AD, when assessing post-mortem brain tissue samples, female patient microglia exhibited diverse morphologies in contrast to male samples, predominantly amoeboid with increased CD68 immunoreactivity (Guillot-Sestier et al., 2021). Moreover, microglia are also influenced by disease-specific context, aging, brain location and extrinsic factors such as medications and drugs (Savchenko et al., 1997; Bollinger et al., 2017; Wang et al., 2021; VanderZwaag et al., 2023), presenting a significant obstacle to the translation of microglia-targeted therapies.
With the core proteins and genes discussed in this review, as well as established and emerging techniques for investigating microglia in homeostasis and disease, our knowledge of microglia and their roles in pathology is continuing to grow exponentially. Given the spatial complexity and heterogeneity of microglia in terms of spatial and temporal characteristics (Carrier et al., 2020), it is critical to employ a broad variety of complementary techniques in integration in order to fully characterize all aspects of this unique subset of immune cells. As microglia are increasingly implicated in a growing list of CNS diseases, optimizing these tools to improve the study of microglia promises to enhance our understanding of fundamental concepts in microglial biology and elucidate novel therapeutic targets with important clinical implications.
Author contributions
BB: Conceptualization, Project administration, Writing – original draft, Writing – review & editing. TH: Conceptualization, Writing – original draft, Writing – review & editing. MC: Writing – original draft, Writing – review & editing. M-ÈT: Funding acquisition, Resources, Supervision, Writing – original draft, Writing – review & editing.
Funding
The authors declare financial support was received for the research, authorship, and/or publication of this article. This work was carried out with the aid of a grant from the International Development Research Centre (IDRC; project ID 109925). BB was supported by a master award from the Division of Medical Sciences at University of Victoria. TH was supported by a British Columbia Children’s Hospital Research Institute Doctoral Award and the University of British Columbia MD/Ph.D. program. MC was supported by a doctoral training award from Fonds de Recherche du Québec–Santé. This work was supported by research grants from CIHR awarded to M-ÈT., who was a College Member of the Royal Society of Canada and a Canada Research Chair (Tier II) in Neurobiology of Aging and Cognition.
Acknowledgments
We are grateful to our colleagues in the Tremblay lab for sharing their insights and providing helpful assistance. We respectfully acknowledge the ləḱwəŋən (Lkwungen), xwməθkwəýəm (Musqueam), Skwxwú7mesh (Squamish), and səlilwətaɬ (Tsleil-Waututh) Nations peoples on whose traditional, ancestral and unceded territory the University of Victoria and University of British Columbia stand, and the Songhees, Esquimalt and WSÁNEĆ peoples whose historical relationships with the land continue to this day. We would like to express our gratitude to Preprint.org for hosting a prior version of this manuscript. The preprint, titled “Emerging Techniques for the Study of Microglia: Visualization, Depletion, and Fate Mapping” authored by BB, TH, MC, and M-ÈT, was instrumental in disseminating our research findings (Bobotis B. et al., 2023).
Conflict of interest
The authors declare that the research was conducted in the absence of any commercial or financial relationships that could be construed as a potential conflict of interest.
The authors declared that they were an editorial board member of Frontiers, at the time of submission. This had no impact on the peer review process and the final decision.
Publisher’s note
All claims expressed in this article are solely those of the authors and do not necessarily represent those of their affiliated organizations, or those of the publisher, the editors and the reviewers. Any product that may be evaluated in this article, or claim that may be made by its manufacturer, is not guaranteed or endorsed by the publisher.
Abbreviations
A2A, adenosine A2A receptor; AD, Alzheimer’s disease; ATP, adenosine triphosphate; BAM, border-associated macrophage; CLEC7a, DECTIN-1, C-type lectin 7a; Clod-Lips, clodronate liposomes; CNS, central nervous system; CSF1R, colony-stimulating factor-1 receptor; CX3CL1, fractalkine; CX3CR1, CX3-motif chemokine receptor 1; fractalkine receptor; DAM, disease-associated microglia; DLPFC, dorsolateral prefrontal cortex; DW-MRI, diffusion-weighted magnetic resonance imaging; DTR, diphtheria toxin receptor; ECM, extracellular matrix; ELISA, enzyme-linked immunosorbent assay; EM, electron microscopy; ER, estrogen receptor; ERT, ERT2, tamoxifen-inducible estrogen receptor; FACS, fluorescence-activated cell sorting; FIRE, fms-intronic regulatory element; GFP, green fluorescent protein; HEXB, hexosaminidase β-subunit; IHC, immunohistochemistry; IL, interleukin; KO, knockout; Mac-1-sap, Mac-1-saporin; MACS, magnetic-activated cell sorting; MAFB, MAF BZIP transcription factor B; MER, mutated estrogen receptor; MGnD, microglia neurodegenerative phenotype; MMP, matrix metalloprotease; P2RY12, purinergic receptor P2Y12; PET, positron emission tomography; PNNs, perineuronal nets; q-PCR, quantitative polymerase chain reaction; SALL1, spalt-like transcription factor 1; TGF-β1, transforming growth factor beta 1; TLR2, Toll-like receptor 2; TMEM119, transmembrane protein 119; TREM2, triggering receptor expressed on myeloid cells 2; TSPO, translocator protein; ZEB1, Zinc-finger E-box binding homeobox 1.
References
Acharjee, S., Verbeek, M., Gomez, C. D., Bisht, K., Lee, B., Benoit, L., et al. (2018). Reduced microglial activity and enhanced glutamate transmission in the basolateral amygdala in early CNS autoimmunity. J. Neurosci. 38, 9019–9033.
Acharya, M. M., Green, K. N., Allen, B. D., Najafi, A. R., Syage, A., Minasyan, H., et al. (2016). Elimination of microglia improves cognitive function following cranial irradiation. Sci. Rep. 6:31545. doi: 10.1038/srep31545
Agalave, N. M., Lane, B. T., Mody, P. H., Szabo-Pardi, T. A., and Burton, M. D. (2020). Isolation, culture, and downstream characterization of primary microglia and astrocytes from adult rodent brain and spinal cord. J. Neurosci. Methods 340:108742.
Ahmed, A., Wang, L.-L., Abdelmaksoud, S., Aboelgheit, A., Saeed, S., and Zhang, C.-L. (2017). Minocycline modulates microglia polarization in ischemia-reperfusion model of retinal degeneration and induces neuroprotection. Sci. Rep. 7:14065. doi: 10.1038/s41598-017-14450-5
Akol, I., Kalogeraki, E., Pielecka-Fortuna, J., Fricke, M., and Löwel, S. (2022). MMP2 and MMP9 activity is crucial for adult visual cortex plasticity in healthy and stroke-affected mice. J. Neurosci. 42, 16–32. doi: 10.1523/JNEUROSCI.0902-21.2021
Albadri, S., Del Bene, F., and Revenu, C. (2017). Genome editing using CRISPR/Cas9-based knock-in approaches in zebrafish. Methods 121–122, 77–85.
Anderson, A. G., Rogers, B. B., Loupe, J. M., Rodriguez-Nunez, I., Roberts, S. C., White, L. M., et al. (2023). Single nucleus multiomics identifies ZEB1 and MAFB as candidate regulators of Alzheimer’s disease-specific cis-regulatory elements. Cell Genomics 3:100263. doi: 10.1016/j.xgen.2023.100263
Askew, K., Li, K., Olmos-Alonso, A., Garcia-Moreno, F., Liang, Y., Richardson, P., et al. (2017). Coupled proliferation and apoptosis maintain the rapid turnover of microglia in the adult brain. Cell Rep. 18, 391–405. doi: 10.1016/j.celrep.2016.12.041
Augusto-Oliveira, M., Arrifano, G. P., Lopes-Araújo, A., Santos-Sacramento, L., Takeda, P. Y., Anthony, D. C., et al. (2019). What do microglia really do in healthy adult brain? Cells 8:1293.
Avignone, E., Ulmann, L., Levavasseur, F., Rassendren, F., and Audinat, E. (2008). Status Epilepticus induces a particular microglial activation state characterized by enhanced purinergic signaling. J. Neurosci. 28, 9133–9144. doi: 10.1523/JNEUROSCI.1820-08.2008
Ayanoğlu, F. B., Elçin, A. E., and Elçin, Y. M. (2020). Bioethical issues in genome editing by CRISPR-Cas9 technology. Turk. J. Biol. 44, 110–120.
Baba, Y., Watabe, Y., Sagara, H., and Watanabe, S. (2019). Sall1 plays pivotal roles for lens fiber cell differentiation in mouse. Biochem. Biophys. Res. Commun. 512, 927–933. doi: 10.1016/j.bbrc.2019.03.098
Bai, R., Gao, H., Han, Z., Huang, S., Ge, X., Chen, F., et al. (2017). Flow Cytometric characterization of T cell subsets and microglia after repetitive mild traumatic brain injury in rats. Neurochem. Res. 42, 2892–2901. doi: 10.1007/s11064-017-2310-0
Banerjee, P., Mehta, A. R., Nirujogi, R. S., Cooper, J., James, O. G., Nanda, J., et al. (2023). Cell-autonomous immune dysfunction driven by disrupted autophagy in C9orf72-ALS iPSC-derived microglia contributes to neurodegeneration. Sci. Adv. 9:eabq0651. doi: 10.1126/sciadv.abq0651
Barnett, A. M., Crews, F. T., and Coleman, L. G. (2021). Microglial depletion and repopulation: a new era of regenerative medicine? Neural Regen. Res. 16, 1204–1205. doi: 10.4103/1673-5374.300439
Bassett, B., Subramaniyam, S., Fan, Y., Varney, S., Pan, H., Carneiro, A. M. D., et al. (2021). Minocycline alleviates depression-like symptoms by rescuing decrease in neurogenesis in dorsal hippocampus via blocking microglia activation/phagocytosis. Brain Behav. Immun. 91, 519–530. doi: 10.1016/j.bbi.2020.11.009
Bassil, R., Shields, K., Granger, K., Zein, I., Ng, S., and Chih, B. (2021). Improved modeling of human AD with an automated culturing platform for iPSC neurons, astrocytes and microglia. Nat. Commun. 12:5220. doi: 10.1038/s41467-021-25344-6
Bemiller, S. M., McCray, T. J., Allan, K., Formica, S. V., Xu, G., Wilson, G., et al. (2017). TREM2 deficiency exacerbates tau pathology through dysregulated kinase signaling in a mouse model of tauopathy. Mol. Neurodegener. 12:74. doi: 10.1186/s13024-017-0216-6
Bennett, M. L., Bennett, F. C., Liddelow, S. A., Ajami, B., Zamanian, J. L., Fernhoff, N. B., et al. (2016). New tools for studying microglia in the mouse and human CNS. Proc. Natl. Acad. Sci. 113, E1738–E1746.
Berdyyeva, T., Xia, C., Taylor, N., He, Y., Chen, G., Huang, C., et al. (2019). PET imaging of the P2X7 ion channel with a novel tracer [18F]JNJ-64413739 in a rat model of neuroinflammation. Mol. Imaging Biol. 21, 871–878.
Betlazar, C., Harrison-Brown, M., Middleton, R. J., Banati, R., and Liu, G.-J. (2018). Cellular sources and regional variations in the expression of the neuroinflammatory marker Translocator Protein (TSPO) in the normal brain. Int. J. Mol. Sci. 19:2707. doi: 10.3390/ijms19092707
Bijata, M., Bączyńska, E., Müller, F. E., Bijata, K., Masternak, J., Krzystyniak, A., et al. (2022). Activation of the 5-HT7 receptor and MMP-9 signaling module in the hippocampal CA1 region is necessary for the development of depressive-like behavior. Cell Rep. 38:110532. doi: 10.1016/j.celrep.2022.110532
Bijata, M., Labus, J., Guseva, D., Stawarski, M., Butzlaff, M., Dzwonek, J., et al. (2017). Synaptic remodeling depends on signaling between serotonin receptors and the extracellular matrix. Cell Rep. 19, 1767–1782. doi: 10.1016/j.celrep.2017.05.023
Bisht, K., Okojie, K. A., Sharma, K., Lentferink, D. H., Sun, Y.-Y., Chen, H.-R., et al. (2021). Capillary-associated microglia regulate vascular structure and function through PANX1-P2RY12 coupling in mice. Nat. Commun. 12:5289. doi: 10.1038/s41467-021-25590-8
Bisht, K., Sharma, K. P., Lecours, C., Gabriela Sánchez, M., El Hajj, H., Milior, G., et al. (2016). Dark microglia: a new phenotype predominantly associated with pathological states. Glia 64, 826–839. doi: 10.1002/glia.22966
Blecharz-Lang, K. G., Patsouris, V., Nieminen-Kelhä, M., Seiffert, S., Schneider, U. C., and Vajkoczy, P. (2022). Minocycline attenuates microglia/macrophage phagocytic activity and inhibits SAH-induced neuronal cell death and inflammation. Neurocrit. Care 37, 410–423. doi: 10.1007/s12028-022-01511-5
Bobotis, B., Halvorson, T., Carrier, M., and Tremblay, M. -È (2023). Emerging techniques for the study of microglia: visualization, depletion and fate mapping. Biol. Life Sci. doi: 10.20944/preprints202308.2190.v1
Bobotis, B. C., Braniff, O., Gargus, M., Akinluyi, E. T., Awogbindin, I. O., and Tremblay, M. -È (2023). Sex differences of microglia in the healthy brain from embryonic development to adulthood and across lifestyle influences. Brain Res. Bull. 202:110752. doi: 10.1016/j.brainresbull.2023.110752
Bohnert, S., Seiffert, A., Trella, S., Bohnert, M., Distel, L., Ondruschka, B., et al. (2020). TMEM119 as a specific marker of microglia reaction in traumatic brain injury in postmortem examination. Int. J. Legal Med. 134, 2167–2176. doi: 10.1007/s00414-020-02384-z
Bollinger, J. L., Collins, K. E., Patel, R., and Wellman, C. L. (2017). Behavioral stress alters corticolimbic microglia in a sex- and brain region-specific manner. PLoS One 12:e0187631. doi: 10.1371/journal.pone.0187631
Bollinger, J. L., Dadosky, D. T., Flurer, J. K., Rainer, I. L., Woodburn, S. C., and Wohleb, E. S. (2023). Microglial P2Y12 mediates chronic stress-induced synapse loss in the prefrontal cortex and associated behavioral consequences. Neuropsychopharmacology 48, 1347–1357. doi: 10.1038/s41386-022-01519-7
Bordeleau, M., Fernández, de Cossío, L., Lacabanne, C., Savage, J. C., Vernoux, N., et al. (2021). Maternal high-fat diet modifies myelin organization, microglial interactions, and results in social memory and sensorimotor gating deficits in adolescent mouse offspring. Brain Behav. Immun. Health 15:100281. doi: 10.1016/j.bbih.2021.100281
Brown, G. D., Taylor, P. R., Reid, D. M., Willment, J. A., Williams, D. L., Martinez-Pomares, L., et al. (2002). Dectin-1 is a major β-Glucan receptor on macrophages. J. Exp. Med. 196, 407–412.
Bruzelius, A., Hidalgo, I., Boza-Serrano, A., Hjelmér, A.-G., Tison, A., Deierborg, T., et al. (2021). The human bone marrow harbors a CD45- CD11B+ cell progenitor permitting rapid microglia-like cell derivative approaches. Stem Cells Transl. Med. 10, 582–597. doi: 10.1002/sctm.20-0127
Butler, C. A., Popescu, A. S., Kitchener, E. J. A., Allendorf, D. H., Puigdellívol, M., and Brown, G. C. (2021). Microglial phagocytosis of neurons in neurodegeneration, and its regulation. J. Neurochem. 158, 621–639.
Butovsky, O., and Weiner, H. L. (2018). Microglial signatures and their role in health and disease. Nat. Rev. Neurosci. 19, 622–635.
Butowski, N., Colman, H., De Groot, J. F., Omuro, A. M., Nayak, L., Wen, P. Y., et al. (2016). Orally administered colony stimulating factor 1 receptor inhibitor PLX3397 in recurrent glioblastoma: an Ivy foundation early phase clinical trials consortium phase II study. Neuro Oncol. 18, 557–564. doi: 10.1093/neuonc/nov245
Buttgereit, A., Lelios, I., Yu, X., Vrohlings, M., Krakoski, N. R., Gautier, E. L., et al. (2016). Sall1 is a transcriptional regulator defining microglia identity and function. Nat. Immunol. 17, 1397–1406.
Cakir, B., Tanaka, Y., Kiral, F. R., Xiang, Y., Dagliyan, O., Wang, J., et al. (2022). Expression of the transcription factor PU.1 induces the generation of microglia-like cells in human cortical organoids. Nat. Commun. 13:430. doi: 10.1038/s41467-022-28043-y
Carrier, M., Dolhan, K., Bobotis, B. C., Desjardins, M., and M., -È. (2022). The implication of a diversity of non-neuronal cells in disorders affecting brain networks. Front. Cell Neurosci. 16:1015556. doi: 10.3389/fncel.2022.1015556
Carrier, M., Robert, M. -È, González Ibáñez, F., Desjardins, M., and Tremblay, M. -È (2020). Imaging the neuroimmune dynamics across space and time. Front. Neurosci. 14:903. doi: 10.3389/fnins.2020.00903
Carrier, M., Šimončičová, E., St-Pierre, M.-K., McKee, C., and Tremblay, M. -È (2021). Psychological stress as a risk factor for accelerated cellular aging and cognitive decline: the involvement of microglia-neuron crosstalk. Front. Mol. Neurosci. 14:749737. doi: 10.3389/fnmol.2021.749737
Casali, B. T., and Reed-Geaghan, E. G. (2021). Microglial function and regulation during development, homeostasis and Alzheimer’s disease. Cells 10:957.
Chaney, A., Cropper, H., Johnson, E., Stevens, M., and James, M. (2019). Imaging the invaders: TREM1 as a novel PET imaging biomarker of peripheral infiltrating myeloid cells and potential therapeutic target in multiple sclerosis. J. Nuclear Med. 60:1506b.
Chaney, A., Wilson, E., Jain, P., Cropper, H., Swarovski, M., Lucot, K., et al. (2020). TREM1-PET imaging of pro-inflammatory myeloid cells distinguishes active disease from remission in multiple sclerosis. J. Nuclear Med. 61, 199–199.
Chappell-Maor, L., Kolesnikov, M., Kim, J.-S., Shemer, A., Haimon, Z., Grozovski, J., et al. (2020). Comparative analysis of CreER transgenic mice for the study of brain macrophages: a case study. Eur. J. Immunol. 50, 353–362. doi: 10.1002/eji.201948342
Chen, M., Ona, V. O., Li, M., Ferrante, R. J., Fink, K. B., Zhu, S., et al. (2000). Minocycline inhibits caspase-1 and caspase-3 expression and delays mortality in a transgenic mouse model of Huntington disease. Nat. Med. 6, 797–801. doi: 10.1038/77528
Chi, D., Zhang, W., Jia, Y., Cong, D., and Hu, S. (2019). Spalt-Like Transcription Factor 1 (SALL1) gene expression inhibits cell proliferation and cell migration of human glioma cells through the Wnt/β-catenin signaling pathway. Med. Sci. Monit. Basic Res. 25, 128–138.
Chistiakov, D. A., Killingsworth, M. C., Myasoedova, V. A., Orekhov, A. N., and Bobryshev, Y. V. (2017). CD68/macrosialin: not just a histochemical marker. Lab. Invest. 97, 4–13.
Choi, Y., Kim, H.-S., Shin, K. Y., Kim, E.-M., Kim, M., Kim, H.-S., et al. (2007). Minocycline attenuates neuronal cell death and improves cognitive impairment in Alzheimer’s disease models. Neuropsychopharmacology 32, 2393–2404.
Colognato, H., and Tzvetanova, I. D. (2011). Glia unglued: how signals from the extracellular matrix regulate the development of myelinating glia. Dev. Neurobiol. 71, 924–955. doi: 10.1002/dneu.20966
Conen, S., Gregory, C. J., Hinz, R., Smallman, R., Corsi-Zuelli, F., Deakin, B., et al. (2021). Neuroinflammation as measured by positron emission tomography in patients with recent onset and established schizophrenia: implications for immune pathogenesis. Mol. Psychiatry 26, 5398–5406. doi: 10.1038/s41380-020-0829-y
Cornell, J., Salinas, S., Huang, H.-Y., and Zhou, M. (2022). Microglia regulation of synaptic plasticity and learning and memory. Neural Regen. Res. 17, 705–716.
Coughlin, J. M., Du, Y., Lesniak, W. G., Harrington, C. K., Brosnan, M. K., O’Toole, R., et al. (2022). First-in-human use of 11C-CPPC with positron emission tomography for imaging the macrophage colony-stimulating factor 1 receptor. EJNMMI Res. 12:64. doi: 10.1186/s13550-022-00929-4
Cuní-López, C., Stewart, R., Quek, H., and White, A. R. (2022). Recent advances in microglia modelling to address translational outcomes in neurodegenerative diseases. Cells 11:1662. doi: 10.3390/cells11101662
Dai, S., Wei, J., Zhang, H., Luo, P., Yang, Y., Jiang, X., et al. (2022). Intermittent fasting reduces neuroinflammation in intracerebral hemorrhage through the Sirt3/Nrf2/HO-1 pathway. J. Neuroinflammation 19:122. doi: 10.1186/s12974-022-02474-2
Daley, D., Mani, V. R., Mohan, N., Akkad, N., Ochi, A., Heindel, D. W., et al. (2017). Dectin 1 activation on macrophages by galectin 9 promotes pancreatic carcinoma and peritumoral immune tolerance. Nat. Med. 23, 556–567.
Damasceno, J. D., Reis-Cunha, J., Crouch, K., Beraldi, D., Lapsley, C., Tosi, L. R. O., et al. (2020). Conditional knockout of RAD51-related genes in Leishmania major reveals a critical role for homologous recombination during genome replication. PLoS Genet. 16:e1008828. doi: 10.1371/journal.pgen.1008828
Daugherty, D. J., Selvaraj, V., Chechneva, O. V., Liu, X.-B., Pleasure, D. E., and Deng, W. (2013). A TSPO ligand is protective in a mouse model of multiple sclerosis. EMBO Mol. Med. 5, 891–903. doi: 10.1002/emmm.201202124
Davalos, D., Grutzendler, J., Yang, G., Kim, J. V., Zuo, Y., Jung, S., et al. (2005). ATP mediates rapid microglial response to local brain injury in vivo. Nat. Neurosci. 8, 752–758. doi: 10.1038/nn1472
Deczkowska, A., Keren-Shaul, H., Weiner, A., Colonna, M., Schwartz, M., and Amit, I. (2018). Disease-associated microglia: a universal immune sensor of neurodegeneration. Cell 173, 1073–1081. doi: 10.1016/j.cell.2018.05.003
Deerhake, M. E., Danzaki, K., Inoue, M., Cardakli, E. D., Nonaka, T., Aggarwal, N., et al. (2021). Dectin-1 limits autoimmune neuroinflammation and promotes myeloid cell-astrocyte crosstalk via Card9-independent expression of Oncostatin M. Immunity 54, 484–498.e8. doi: 10.1016/j.immuni.2021.01.004
Dermitzakis, I., Theotokis, P., Evangelidis, P., Delilampou, E., Evangelidis, N., Chatzisavvidou, A., et al. (2023). CNS border-associated macrophages: ontogeny and potential implication in disease. Curr. Issues Mol. Biol. 45, 4285–4300. doi: 10.3390/cimb45050272
Di Biase, M. A., Zalesky, A., O’keefe, G., Laskaris, L., Baune, B. T., Weickert, C. S., et al. (2017). PET imaging of putative microglial activation in individuals at ultra-high risk for psychosis, recently diagnosed and chronically ill with schizophrenia. Transl. Psychiatry 7:e1225. doi: 10.1038/tp.2017.193
Dolan, M.-J., Therrien, M., Jereb, S., Kamath, T., Gazestani, V., Atkeson, T., et al. (2023). Exposure of iPSC-derived human microglia to brain substrates enables the generation and manipulation of diverse transcriptional states in vitro. Nat. Immunol. 24, 1382–1390. doi: 10.1038/s41590-023-01558-2
Doudna, J. A., and Charpentier, E. (2014). Genome editing. the new frontier of genome engineering with CRISPR-Cas9. Science 346:1258096.
Dräger, N. M., Sattler, S. M., Huang, C. T.-L., Teter, O. M., Leng, K., Hashemi, S. H., et al. (2022). A CRISPRi/a platform in human iPSC-derived microglia uncovers regulators of disease states. Nat. Neurosci. 25, 1149–1162. doi: 10.1038/s41593-022-01131-4
Drissen, R., Thongjuea, S., Theilgaard-Mönch, K., and Nerlov, C. (2019). Identification of two distinct pathways of human myelopoiesis. Sci. Immunol. 4:eaau7148. doi: 10.1126/sciimmunol.aau7148
Du, Y., Ma, Z., Lin, S., Dodel, R. C., Gao, F., Bales, K. R., et al. (2001). Minocycline prevents nigrostriatal dopaminergic neurodegeneration in the MPTP model of Parkinson’s disease. Proc. Natl. Acad. Sci. U S A. 98, 14669–14674.
Dubbelaar, M. L., Kracht, L., Eggen, B. J. L., and Boddeke, E. W. G. M. (2018). The kaleidoscope of microglial phenotypes. Front. Immunol. 9:1753. doi: 10.3389/fimmu.2018.01753
El Hajj, H., Savage, J. C., Bisht, K., Parent, M., Vallières, L., Rivest, S., et al. (2019). Ultrastructural evidence of microglial heterogeneity in Alzheimer’s disease amyloid pathology. J. Neuroinflammation 16:87.
Elmore, M. R. P., Najafi, A. R., Koike, M. A., Dagher, N. N., Spangenberg, E. E., Rice, R. A., et al. (2014). Colony-stimulating factor 1 receptor signaling is necessary for microglia viability, unmasking a microglia progenitor cell in the adult brain. Neuron 82, 380–397. doi: 10.1016/j.neuron.2014.02.040
Erblich, B., Zhu, L., Etgen, A. M., Dobrenis, K., and Pollard, J. W. (2011). Absence of colony stimulation factor-1 receptor results in loss of microglia, disrupted brain development and olfactory deficits. PLoS One 6:e26317. doi: 10.1371/journal.pone.0026317
Fattorelli, N., Martinez-Muriana, A., Wolfs, L., Geric, I., De Strooper, B., and Mancuso, R. (2021). Stem-cell-derived human microglia transplanted into mouse brain to study human disease. Nat. Protoc. 16, 1013–1033.
Faust, T. E., Feinberg, P. A., O’Connor, C., Kawaguchi, R., Chan, A., Strasburger, H., et al. (2023). A comparative analysis of microglial inducible Cre lines. Cell Rep. 42:113031.
Fawcett, J. W., Fyhn, M., Jendelova, P., Kwok, J. C. F., Ruzicka, J., and Sorg, B. A. (2022). The extracellular matrix and perineuronal nets in memory. Mol. Psychiatry 27, 3192–3203.
Feil, R., Wagner, J., Metzger, D., and Chambon, P. (1997). Regulation of Cre recombinase activity by mutated estrogen receptor ligand-binding domains. Biochem. Biophys. Res. Commun. 237, 752–757.
Filipello, F., Morini, R., Corradini, I., Zerbi, V., Canzi, A., Michalski, B., et al. (2018). The microglial innate immune receptor TREM2 is required for synapse elimination and normal brain connectivity. Immunity 48, 979–991.e8. doi: 10.1016/j.immuni.2018.04.016
Fischer, H.-G., and Reichmann, G. (2001). Brain dendritic cells and macrophages/microglia in central nervous system Inflammation1. J. Immunol. 166, 2717–2726. doi: 10.4049/jimmunol.166.4.2717
Fixsen, B. R., Han, C. Z., Zhou, Y., Spann, N. J., Saisan, P., Shen, Z., et al. (2023). SALL1 enforces microglia-specific DNA binding and function of SMADs to establish microglia identity. Nat. Immunol. 24, 1188–1199. doi: 10.1038/s41590-023-01528-8
Franke, H., Krügel, U., Grosche, J., Heine, C., Härtig, W., Allgaier, C., et al. (2004). P2Y receptor expression on astrocytes in the nucleus accumbens of rats. Neuroscience 127, 431–441.
Friedrich, G., and Soriano, P. (1991). Promoter traps in embryonic stem cells: a genetic screen to identify and mutate developmental genes in mice. Genes Dev. 5, 1513–1523. doi: 10.1101/gad.5.9.1513
Fu, H., Zhao, Y., Hu, D., Wang, S., Yu, T., and Zhang, L. (2020). Depletion of microglia exacerbates injury and impairs function recovery after spinal cord injury in mice. Cell Death Dis. 11:528. doi: 10.1038/s41419-020-2733-4
Fujiwara, T., Yakoub, M. A., Chandler, A., Christ, A. B., Yang, G., Ouerfelli, O., et al. (2021). CSF1/CSF1R signaling inhibitor pexidartinib (PLX3397) reprograms tumor-associated macrophages and stimulates T-cell infiltration in the sarcoma microenvironment. Mol. Cancer Ther. 20, 1388–1399. doi: 10.1158/1535-7163.MCT-20-0591
Gao, H., Di, J., Clausen, B. H., Wang, N., Zhu, X., Zhao, T., et al. (2023). Distinct myeloid population phenotypes dependent on TREM2 expression levels shape the pathology of traumatic versus demyelinating CNS disorders. Cell Rep. 42:112629.
Garcia, J. A., Cardona, S. M., and Cardona, A. E. (2013). Analyses of microglia effector function using CX3CR1-GFP knock-in mice. Methods Mol. Biol. 1041, 307–317. doi: 10.1007/978-1-62703-520-0_27
Garcia-Hernandez, R., Cerdán Cerdá, A., Trouve Carpena, A., Drakesmith, M., Koller, K., Jones, D. K., et al. (2022). Mapping microglia and astrocyte activation in vivo using diffusion MRI. Sci. Adv. 8:eabq2923. doi: 10.1126/sciadv.abq2923
Garrido-Mesa, N., Zarzuelo, A., and Gálvez, J. (2013). Minocycline: far beyond an antibiotic. Br. J. Pharmacol. 169, 337–352.
Gerhard, A., Banati, R. B., Goerres, G. B., Cagnin, A., Myers, R., Gunn, R. N., et al. (2003). [11C](R)-PK11195 PET imaging of microglial activation in multiple system atrophy. Neurology 61, 686–689.
Ginhoux, F., Greter, M., Leboeuf, M., Nandi, S., See, P., Gokhan, S., et al. (2010). Fate mapping analysis reveals that adult microglia derive from primitive macrophages. Science 330, 841–845. doi: 10.1126/science.1194637
Goldman, R. D. (2000). Antibodies: indispensable tools for biomedical research. Trends Biochem. Sci. 25, 593–595.
Goldmann, T., Wieghofer, P., Jordão, M. J. C., Prutek, F., Hagemeyer, N., Frenzel, K., et al. (2016). Origin, fate and dynamics of macrophages at central nervous system interfaces. Nat. Immunol. 17, 797–805. doi: 10.1038/ni.3423
Goldmann, T., Wieghofer, P., Müller, P. F., Wolf, Y., Varol, D., Yona, S., et al. (2013). A new type of microglia gene targeting shows TAK1 to be pivotal in CNS autoimmune inflammation. Nat. Neurosci. 16, 1618–1626. doi: 10.1038/nn.3531
Gómez Morillas, A., Besson, V. C., and Lerouet, D. (2021). Microglia and neuroinflammation: what place for P2RY12? Int. J. Mol. Sci. 22:1636. doi: 10.3390/ijms22041636
Gomez Perdiguero, E., Klapproth, K., Schulz, C., Busch, K., Azzoni, E., Crozet, L., et al. (2015). Tissue-resident macrophages originate from yolk-sac-derived erythro-myeloid progenitors. Nature 518, 547–551.
Gomez-Nicola, D., and Perry, V. H. (2015). Microglial dynamics and role in the healthy and diseased brain: a paradigm of functional plasticity. Neuroscientist 21, 169–184. doi: 10.1177/1073858414530512
Gong, J., Szego, ÉM., Leonov, A., Benito, E., Becker, S., Fischer, A., et al. (2019). Translocator protein ligand protects against neurodegeneration in the MPTP mouse model of Parkinsonism. J. Neurosci. 39, 3752–3769.
González Ibáñez, F., Halvorson, T., Sharma, K., McKee, C. G., Carrier, M., Picard, K., et al. (2023). Ketogenic diet changes microglial morphology and the hippocampal lipidomic profile differently in stress susceptible versus resistant male mice upon repeated social defeat. Brain Behav. Immun. 114, 383–406.
González-Prieto, M., Gutiérrez, I. L., García-Bueno, B., Caso, J. R., Leza, J. C., Ortega-Hernández, A., et al. (2021). Microglial CX3CR1 production increases in Alzheimer’s disease and is regulated by noradrenaline. Glia 69, 73–90. doi: 10.1002/glia.23885
Gosselin, D., Skola, D., Coufal, N. G., Holtman, I. R., Schlachetzki, J. C. M., Sajti, E., et al. (2017). An environment-dependent transcriptional network specifies human microglia identity. Science 356:eaal3222.
Gratuze, M., Leyns, C. E. G., and Holtzman, D. M. (2018). New insights into the role of TREM2 in Alzheimer’s disease. Mol. Neurodegeneration 13:66.
Green, K. N., Crapser, J. D., and Hohsfield, L. A. (2020). To kill a microglia: a case for CSF1R inhibitors. Trends Immunol. 41, 771–784. doi: 10.1016/j.it.2020.07.001
Greter, M., Lelios, I., and Croxford, A. L. (2015). Microglia versus myeloid cell nomenclature during brain inflammation. Front. Immunol. 6:249. doi: 10.3389/fimmu.2015.00249
Guerreiro, R., Wojtas, A., Bras, J., Carrasquillo, M., Rogaeva, E., Majounie, E., et al. (2013). TREM2 variants in Alzheimer’s disease. N. Engl. J. Med. 368, 117–127.
Guillot-Sestier, M.-V., Araiz, A. R., Mela, V., Gaban, A. S., O’Neill, E., Joshi, L., et al. (2021). Microglial metabolism is a pivotal factor in sexual dimorphism in Alzheimer’s disease. Commun. Biol. 4:711. doi: 10.1038/s42003-021-02259-y
Gupta, B., Errington, A. C., Jimenez-Pascual, A., Eftychidis, V., Brabletz, S., Stemmler, M. P., et al. (2021). The transcription factor ZEB1 regulates stem cell self-renewal and cell fate in the adult hippocampus. Cell Rep. 36:109588. doi: 10.1016/j.celrep.2021.109588
Haenseler, W., Sansom, S. N., Buchrieser, J., Newey, S. E., Moore, C. S., Nicholls, F. J., et al. (2017). A highly efficient human pluripotent stem cell microglia model displays a neuronal-co-culture-specific expression profile and inflammatory response. Stem Cell Rep. 8, 1727–1742. doi: 10.1016/j.stemcr.2017.05.017
Haimon, Z., Volaski, A., Orthgiess, J., Boura-Halfon, S., Varol, D., Shemer, A., et al. (2018). Re-evaluating microglia expression profiles using RiboTag and cell isolation strategies. Nat. Immunol. 19, 636–644. doi: 10.1038/s41590-018-0110-6
Hammond, T. R., Dufort, C., Dissing-Olesen, L., Giera, S., Young, A., Wysoker, A., et al. (2019). Single-cell RNA sequencing of microglia throughout the mouse lifespan and in the injured brain reveals complex cell-state changes. Immunity 50, 253–271.e6. doi: 10.1016/j.immuni.2018.11.004
Han, J., Fan, Y., Zhou, K., Blomgren, K., and Harris, R. A. (2021). Uncovering sex differences of rodent microglia. J. Neuroinflammation 18:74.
Han, J., Harris, R. A., and Zhang, X.-M. (2017). An updated assessment of microglia depletion: current concepts and future directions. Mol. Brain 10:25. doi: 10.1186/s13041-017-0307-x
Han, X., Li, Q., Lan, X., El-Mufti, L., Ren, H., and Wang, J. (2019). Microglial depletion with clodronate liposomes increases proinflammatory cytokine levels, induces astrocyte activation, and damages blood vessel integrity. Mol. Neurobiol. 56, 6184–6196. doi: 10.1007/s12035-019-1502-9
Hanania, R., Sun, H. S., Xu, K., Pustylnik, S., Jeganathan, S., and Harrison, R. E. (2012). Classically activated macrophages use stable microtubules for matrix metalloproteinase-9 (MMP-9) secretion. J. Biol. Chem. 287, 8468–8483. doi: 10.1074/jbc.M111.290676
Hanisch, U.-K., and Kettenmann, H. (2007). Microglia: active sensor and versatile effector cells in the normal and pathologic brain. Nat. Neurosci. 10, 1387–1394. doi: 10.1038/nn1997
Hayashi, S., and McMahon, A. P. (2002). Efficient recombination in diverse tissues by a tamoxifen-inducible form of Cre: a tool for temporally regulated gene activation/inactivation in the mouse. Dev. Biol. 244, 305–318. doi: 10.1006/dbio.2002.0597
Haynes, S. E., Hollopeter, G., Yang, G., Kurpius, D., Dailey, M. E., Gan, W.-B., et al. (2006). The P2Y12 receptor regulates microglial activation by extracellular nucleotides. Nat. Neurosci. 9, 1512–1519.
Hellström Erkenstam, N., Smith, P. L. P., Fleiss, B., Nair, S., Svedin, P., Wang, W., et al. (2016). Temporal characterization of microglia/macrophage phenotypes in a mouse model of neonatal hypoxic-ischemic brain injury. Front. Cell Neurosci. 10:286. doi: 10.3389/fncel.2016.00286
Hendrickx, D. A. E., van Eden, C. G., Schuurman, K. G., Hamann, J., and Huitinga, I. (2017). Staining of HLA-DR, Iba1 and CD68 in human microglia reveals partially overlapping expression depending on cellular morphology and pathology. J. Neuroimmunol. 309, 12–22. doi: 10.1016/j.jneuroim.2017.04.007
Henry, R. J., Ritzel, R. M., Barrett, J. P., Doran, S. J., Jiao, Y., Leach, J. B., et al. (2020). Microglial depletion with CSF1R inhibitor during chronic phase of experimental traumatic brain injury reduces neurodegeneration and neurological deficits. J. Neurosci. 40, 2960–2974. doi: 10.1523/JNEUROSCI.2402-19.2020
Heuss, N. D., Pierson, M. J., Roehrich, H., McPherson, S. W., Gram, A. L., Li, L., et al. (2018). Optic nerve as a source of activated retinal microglia post-injury. Acta Neuropathol. Commun. 6:66. doi: 10.1186/s40478-018-0571-8
Hickman, S. E., Allison, E. K., Coleman, U., Kingery-Gallagher, N. D., and El Khoury, J. (2019). Heterozygous CX3CR1 deficiency in microglia restores neuronal β-amyloid clearance pathways and slows progression of Alzheimer’s like-disease in PS1-APP mice. Front. Immunol. 10:2780. doi: 10.3389/fimmu.2019.02780
Hickman, S. E., Kingery, N. D., Ohsumi, T. K., Borowsky, M. L., Wang, L., Means, T. K., et al. (2013). The microglial sensome revealed by direct RNA sequencing. Nat. Neurosci. 16, 1896–1905. doi: 10.1038/nn.3554
Hirrlinger, J., Scheller, A., Hirrlinger, P. G., Kellert, B., Tang, W., Wehr, M. C., et al. (2009). Split-cre complementation indicates coincident activity of different genes in vivo. PLoS One 4:e4286. doi: 10.1371/journal.pone.0004286
Hoeffel, G., Chen, J., Lavin, Y., Low, D., Almeida, F. F., See, P., et al. (2015). C-Myb(+) erythro-myeloid progenitor-derived fetal monocytes give rise to adult tissue-resident macrophages. Immunity 42, 665–678. doi: 10.1016/j.immuni.2015.03.011
Holtman, I. R., Skola, D., and Glass, C. K. (2017). Transcriptional control of microglia phenotypes in health and disease. J. Clin. Invest. 127, 3220–3229. doi: 10.1172/JCI90604
Honarpisheh, P., Lee, J., Banerjee, A., Blasco-Conesa, M. P., Honarpisheh, P., d’Aigle, J., et al. (2020). Potential caveats of putative microglia-specific markers for assessment of age-related cerebrovascular neuroinflammation. J. Neuroinflammation 17:366. doi: 10.1186/s12974-020-02019-5
Hoogland, I. C. M., Houbolt, C., van Westerloo, D. J., van Gool, W. A., and van de Beek, D. (2015). Systemic inflammation and microglial activation: systematic review of animal experiments. J. Neuroinflammation 12:114. doi: 10.1186/s12974-015-0332-6
Hopperton, K. E., Mohammad, D., Trépanier, M. O., Giuliano, V., and Bazinet, R. P. (2018). Markers of microglia in post-mortem brain samples from patients with Alzheimer’s disease: a systematic review. Mol. Psychiatry 23, 177–198. doi: 10.1038/mp.2017.246
Hu, W., Pan, D., Wang, Y., Bao, W., Zuo, C., Guan, Y., et al. (2020). PET imaging for dynamically monitoring neuroinflammation in APP/PS1 mouse model using [18F]DPA714. Front. Neurosci. 14:810. doi: 10.3389/fnins.2020.00810
Huang, Y., Xu, Z., Xiong, S., Sun, F., Qin, G., Hu, G., et al. (2018). Repopulated microglia are solely derived from the proliferation of residual microglia after acute depletion. Nat. Neurosci. 21, 530–540.
Hui, C. W., Vecchiarelli, H. A., Gervais, É, Luo, X., Michaud, F., Scheefhals, L., et al. (2020). Sex differences of microglia and synapses in the hippocampal dentate gyrus of adult mouse offspring exposed to maternal immune activation. Front. Cell Neurosci. 14:558181. doi: 10.3389/fncel.2020.558181
Hupp, S., and Iliev, A. I. (2020). CSF-1 receptor inhibition as a highly effective tool for depletion of microglia in mixed glial cultures. J. Neurosci. Methods 332:108537. doi: 10.1016/j.jneumeth.2019.108537
Ibanez, F. G., Picard, K., Bordelau, M., Sharma, K., Bisht, K., and Tremblay, M.-E. (2019). Immunofluorescence staining using IBA1 and TMEM119 for microglial density, morphology and peripheral myeloid cell infiltration analysis in mouse brain. J. Vis. Exp. 152. doi: 10.3791/60510
Imai, Y., Ibata, I., Ito, D., Ohsawa, K., and Kohsaka, S. (1996). A novel gene iba1 in the major histocompatibility complex class III region encoding an EF hand protein expressed in a monocytic lineage. Biochem. Biophys. Res. Commun 224, 855–862. doi: 10.1006/bbrc.1996.1112
Indra, A. K., Warot, X., Brocard, J., Bornert, J. M., Xiao, J. H., Chambon, P., et al. (1999). Temporally-controlled site-specific mutagenesis in the basal layer of the epidermis: comparison of the recombinase activity of the tamoxifen-inducible Cre-ER(T) and Cre-ER(T2) recombinases. Nucleic Acids Res. 27, 4324–4327. doi: 10.1093/nar/27.22.4324
Janssen, B., Vugts, D. J., Windhorst, A. D., and Mach, R. H. (2018). PET imaging of microglial activation-beyond targeting TSPO. Molecules 23:607. doi: 10.3390/molecules23030607
Jefferson, R. A., Burgess, S. M., and Hirsh, D. (1986). beta-Glucuronidase from Escherichia coli as a gene-fusion marker. Proc. Natl. Acad. Sci. U S A. 83, 8447–8451. doi: 10.1073/pnas.83.22.8447
Jefferson, R. A., Kavanagh, T. A., and Bevan, M. W. (1987). GUS fusions: beta-glucuronidase as a sensitive and versatile gene fusion marker in higher plants. EMBO J. 6, 3901–3907. doi: 10.1002/j.1460-2075.1987.tb02730.x
Jia, M., Zhang, W., Zhu, J., Huang, C., Zhou, J., Lian, J., et al. (2021). Microglia-specific expression of HEXA and HEXB leads to poor prognosis in glioblastoma patients. Front. Oncol. 11:685893. doi: 10.3389/fonc.2021.685893
Jiang, T., Xing, B., and Rao, J. (2008). Recent developments of biological reporter technology for detecting gene expression. Biotechnol. Genet. Eng. Rev. 25, 41–75.
Jin, X., Ishii, H., Bai, Z., Itokazu, T., and Yamashita, T. (2012). Temporal changes in cell marker expression and cellular infiltration in a controlled cortical impact model in adult male C57BL/6 mice. PLoS One 7:e41892. doi: 10.1371/journal.pone.0041892
Johnson, N. R., Yuan, P., Castillo, E., Lopez, T. P., Yue, W., Bond, A., et al. (2023). CSF1R inhibitors induce a sex-specific resilient microglial phenotype and functional rescue in a tauopathy mouse model. Nat. Commun. 14:118. doi: 10.1038/s41467-022-35753-w
Jonsson, T., Stefansson, H., Steinberg, S., Jonsdottir, I., Jonsson, P. V., Snaedal, J., et al. (2013). Variant of TREM2 associated with the risk of Alzheimer’s disease. N. Engl. J. Med. 368, 107–116.
Jordão, M. J. C., Sankowski, R., Brendecke, S. M., Sagar, Locatelli, G., Tai, Y.-H., et al. (2019). Single-cell profiling identifies myeloid cell subsets with distinct fates during neuroinflammation. Science 363:eaat7554. doi: 10.1126/science.aat7554
Jullien, N., Sampieri, F., Enjalbert, A., and Herman, J. (2003). Regulation of Cre recombinase by ligand-induced complementation of inactive fragments. Nucleic Acids Res. 31:e131. doi: 10.1093/nar/gng131
Jung, S., Aliberti, J., Graemmel, P., Sunshine, M. J., Kreutzberg, G. W., Sher, A., et al. (2000). Analysis of fractalkine receptor CX(3)CR1 function by targeted deletion and green fluorescent protein reporter gene insertion. Mol. Cell Biol. 20, 4106–4114. doi: 10.1128/MCB.20.11.4106-4114.2000
Jurga, A. M., Paleczna, M., and Kuter, K. Z. (2020). Overview of general and discriminating markers of differential microglia phenotypes. Front. Cell Neurosci. 14:198. doi: 10.3389/fncel.2020.00198
Kaiser, T., and Feng, G. (2019). Tmem119-EGFP and Tmem119-CreERT2 transgenic mice for labeling and manipulating microglia. eNeuro 6:ENEURO.0448-18.2019. doi: 10.1523/ENEURO.0448-18.2019
Keren-Shaul, H., Spinrad, A., Weiner, A., Matcovitch-Natan, O., Dvir-Szternfeld, R., Ulland, T. K., et al. (2017). A unique microglia type associated with restricting development of Alzheimer’s disease. Cell 169, 1276–1290.e17. doi: 10.1016/j.cell.2017.05.018
Kiani Shabestari, S., Morabito, S., Danhash, E. P., McQuade, A., Sanchez, J. R., Miyoshi, E., et al. (2022). Absence of microglia promotes diverse pathologies and early lethality in Alzheimer’s disease mice. Cell Rep. 39:110961. doi: 10.1016/j.celrep.2022.110961
Kim, J., Kim, J.-H., Do, J. Y., Lee, J. Y., Yanai, R., Lee, I., et al. (2021). Key role of microglial matrix metalloproteinases in choroidal neovascularization. Front. Cell. Neurosci. 15:638098. doi: 10.3389/fncel.2021.638098
Kim, J.-S., Kolesnikov, M., Peled-Hajaj, S., Scheyltjens, I., Xia, Y., Trzebanski, S., et al. (2021). A binary Cre transgenic approach dissects microglia and CNS border-associated macrophages. Immunity 54, 176–190.e7. doi: 10.1016/j.immuni.2020.11.007
Kim, Y. S., Kim, S. S., Cho, J. J., Choi, D. H., Hwang, O., Shin, D. H., et al. (2005). Matrix metalloproteinase-3: a novel signaling proteinase from apoptotic neuronal cells that activates microglia. J. Neurosci. 25, 3701–3711. doi: 10.1523/JNEUROSCI.4346-04.2005
Kingham, P. J., and Pocock, J. M. (2001). Microglial secreted cathepsin B induces neuronal apoptosis. J. Neurochem. 76, 1475–1484.
Kishimoto, I., Okano, T., Nishimura, K., Motohashi, T., and Omori, K. (2019). Early development of resident macrophages in the mouse cochlea depends on yolk sac hematopoiesis. Front. Neurol. 10:1115. doi: 10.3389/fneur.2019.01115
Konishi, H., and Kiyama, H. (2018). Microglial TREM2/DAP12 signaling: a double-edged sword in neural diseases. Front. Cell Neurosci. 12:206. doi: 10.3389/fncel.2018.00206
Könnecke, H., and Bechmann, I. (2013). The role of microglia and matrix metalloproteinases involvement in neuroinflammation and gliomas. Clin. Dev. Immunol. 2013:914104. doi: 10.1155/2013/914104
Krais, J. J., and Johnson, N. (2020). BRCA1 mutations in cancer: coordinating deficiencies in homologous recombination with tumorigenesis. Cancer Res. 80, 4601–4609.
Krasemann, S., Madore, C., Cialic, R., Baufeld, C., Calcagno, N., Fatimy, R. E., et al. (2017). The TREM2-APOE pathway drives the transcriptional phenotype of dysfunctional microglia in neurodegenerative diseases. Immunity 47, 566–581.e9. doi: 10.1016/j.immuni.2017.08.008
Kuil, L. E., López Martí, A., Carreras Mascaro, A., van den Bosch, J. C., van den Berg, P., van der Linde, H. C., et al. (2019). Hexb enzyme deficiency leads to lysosomal abnormalities in radial glia and microglia in zebrafish brain development. Glia 67, 1705–1718. doi: 10.1002/glia.23641
Kwon, H. S., and Koh, S.-H. (2020). Neuroinflammation in neurodegenerative disorders: the roles of microglia and astrocytes. Transl. Neurodegener. 9:42.
Lam, D., Enright, H. A., Cadena, J., Peters, S. K. G., Sales, A. P., Osburn, J. J., et al. (2019). Tissue-specific extracellular matrix accelerates the formation of neural networks and communities in a neuron-glia co-culture on a multi-electrode array. Sci. Rep. 9:4159. doi: 10.1038/s41598-019-40128-1
Lauro, C., Chece, G., Monaco, L., Antonangeli, F., Peruzzi, G., Rinaldo, S., et al. (2019). Fractalkine modulates microglia metabolism in brain Ischemia. Front. Cell. Neurosci. 13:414. doi: 10.3389/fncel.2019.00414
Lavisse, S., Goutal, S., Wimberley, C., Tonietto, M., Bottlaender, M., Gervais, P., et al. (2021). Increased microglial activation in patients with Parkinson disease using [18F]-DPA714 TSPO PET imaging. Parkinsonism Relat. Disord. 82, 29–36. doi: 10.1016/j.parkreldis.2020.11.011
Lawson, L. J., Perry, V. H., and Gordon, S. (1992). Turnover of resident microglia in the normal adult mouse brain. Neuroscience 48, 405–415.
Lecours, C., St-Pierre, M.-K., Picard, K., Bordeleau, M., Bourque, M., Awogbindin, I. O., et al. (2020). Levodopa partially rescues microglial numerical, morphological, and phagolysosomal alterations in a monkey model of Parkinson’s disease. Brain Behav. Immun. 90, 81–96. doi: 10.1016/j.bbi.2020.07.044
Lee, E.-J., Moon, P.-G., Baek, M.-C., and Kim, H.-S. (2014). Comparison of the effects of matrix metalloproteinase inhibitors on TNF-α release from activated microglia and TNF-α converting enzyme activity. Biomol. Ther. 22, 414–419. doi: 10.4062/biomolther.2014.099
Lee, E.-J., Woo, M.-S., Moon, P.-G., Baek, M.-C., Choi, I.-Y., Kim, W.-K., et al. (2010). α-Synuclein activates microglia by inducing the expressions of matrix metalloproteinases and the subsequent activation of protease-activated receptor-1. J. Immunol. 185, 615–623. doi: 10.4049/jimmunol.0903480
Lehenkari, P. P., Kellinsalmi, M., Näpänkangas, J. P., Ylitalo, K. V., Mönkkönen, J., Rogers, M. J., et al. (2002). Further insight into mechanism of action of clodronate: inhibition of mitochondrial ADP/ATP translocase by a nonhydrolyzable, adenine-containing metabolite. Mol. Pharmacol. 61, 1255–1262. doi: 10.1124/mol.61.5.1255
Lei, F., Cui, N., Zhou, C., Chodosh, J., Vavvas, D. G., and Paschalis, E. I. (2020). CSF1R inhibition by a small-molecule inhibitor is not microglia specific; affecting hematopoiesis and the function of macrophages. Proc. Natl. Acad. Sci. 117, 23336–23338.
Li, Q., Cheng, Z., Zhou, L., Darmanis, S., Neff, N. F., Okamoto, J., et al. (2019a). Developmental heterogeneity of microglia and brain myeloid cells revealed by deep single-cell RNA sequencing. Neuron 101, 207–223.e10. doi: 10.1016/j.neuron.2018.12.006
Li, Q., Lan, X., Han, X., and Wang, J. (2019b). Expression of Tmem119/Sall1 and Ccr2/CD69 in FACS-sorted microglia- and monocyte/macrophage-enriched cell populations after intracerebral hemorrhage. Front. Cell. Neurosci. 12:520. doi: 10.3389/fncel.2018.00520
Liang, K. J., Lee, J. E., Wang, Y. D., Ma, W., Fontainhas, A. M., Fariss, R. N., et al. (2009). Regulation of dynamic behavior of retinal microglia by CX3CR1 signaling. Invest. Ophthalmol. Visual Sci. 50, 4444–4451. doi: 10.1167/iovs.08-3357
Lin, S.-S., Tang, Y., Illes, P., and Verkhratsky, A. (2020). The safeguarding microglia: central role for P2Y12 receptors. Front. Pharmacol. 11:627760. doi: 10.3389/fphar.2020.627760
Lindberg, R. L., De Groot, C. J., Montagne, L., Freitag, P., van der Valk, P., Kappos, L., et al. (2001). The expression profile of matrix metalloproteinases (MMPs) and their inhibitors (TIMPs) in lesions and normal appearing white matter of multiple sclerosis. Brain 124, 1743–1753. doi: 10.1093/brain/124.9.1743
Littlewood, T. D., Hancock, D. C., Danielian, P. S., Parker, M. G., and Evan, G. I. (1995). A modified oestrogen receptor ligand-binding domain as an improved switch for the regulation of heterologous proteins. Nucleic Acids Res. 23, 1686–1690. doi: 10.1093/nar/23.10.1686
Lituma, P. J., Woo, E., O’Hara, B. F., Castillo, P. E., Sibinga, N. E. S., and Nandi, S. (2021). Altered synaptic connectivity and brain function in mice lacking microglial adapter protein Iba1. Proc. Natl. Acad. Sci. U.S.A. 118:e2115539118. doi: 10.1073/pnas.2115539118
Liu, S., Liu, Z., Shang, A., Xun, J., Lv, Z., Zhou, S., et al. (2023). CD44 is a potential immunotherapeutic target and affects macrophage infiltration leading to poor prognosis. Sci. Rep. 13:9657. doi: 10.1038/s41598-023-33915-4
Liu, W., Zhou, X., Yao, Q., Chen, C., Zhang, Q., Ding, K., et al. (2023). In situ expansion and reprogramming of Kupffer cells elicit potent tumoricidal immunity against liver metastasis. J. Clin. Invest. 133:e157937. doi: 10.1172/JCI157937
Long, Y., Wang, Y., Shen, Y., Huang, J., Li, Y., Wu, R., et al. (2023). Minocycline and antipsychotics inhibit inflammatory responses in BV-2 microglia activated by LPS via regulating the MAPKs/ JAK-STAT signaling pathway. BMC Psychiatry 23:514. doi: 10.1186/s12888-023-05014-1
Louveau, A., Nerrière-Daguin, V., Vanhove, B., Naveilhan, P., Neunlist, M., Nicot, A., et al. (2015). Targeting the CD80/CD86 costimulatory pathway with CTLA4-Ig directs microglia toward a repair phenotype and promotes axonal outgrowth: CTLA4-Ig regulates microglia and axon growth. Glia 63, 2298–2312. doi: 10.1002/glia.22894
Lowery, R. L., Mendes, M. S., Sanders, B. T., Murphy, A. J., Whitelaw, B. S., Lamantia, C. E., et al. (2021). Loss of P2Y12 has behavioral effects in the adult mouse. Int. J. Mol. Sci. 22:1868. doi: 10.3390/ijms22041868
Maco, B., Holtmaat, A., Jorstad, A., Fua, P., and Knott, G. W. (2014). Correlative in vivo 2-photon imaging and focused ion beam scanning electron microscopy: 3D analysis of neuronal ultrastructure. Methods Cell Biol. 124, 339–361. doi: 10.1016/B978-0-12-801075-4.00016-1
Maimaiti, S., Koshida, R., Ojima, M., Kulathunga, K., Oishi, H., and Takahashi, S. (2019). Neuron-specific Mafb knockout causes growth retardation accompanied by an impaired growth hormone/insulin-like growth factor I axis. Exp. Anim. 68, 435–442. doi: 10.1538/expanim.18-0182
Marino Lee, S., Hudobenko, J., McCullough, L. D., and Chauhan, A. (2021). Microglia depletion increase brain injury after acute ischemic stroke in aged mice. Exp. Neurol. 336:113530.
Martin, E., El-Behi, M., Fontaine, B., and Delarasse, C. (2017). Analysis of microglia and monocyte-derived macrophages from the central nervous system by flow cytometry. J. Vis. Exp. 55781. doi: 10.3791/55781
Marzan, D. E., Brügger-Verdon, V., West, B. L., Liddelow, S., Samanta, J., and Salzer, J. L. (2021). Activated microglia drive demyelination via CSF1R signaling. Glia 69, 1583–1604. doi: 10.1002/glia.23980
Masuda, T., Amann, L., Monaco, G., Sankowski, R., Staszewski, O., Krueger, M., et al. (2022). Specification of CNS macrophage subsets occurs postnatally in defined niches. Nature 604, 740–748.
Masuda, T., Amann, L., Sankowski, R., Staszewski, O., Lenz, M., d’ Errico, P., et al. (2020). Novel Hexb-based tools for studying microglia in the CNS. Nat. Immunol. 21, 802–815.
Matsumoto, T., Imagama, S., Hirano, K., Ohgomori, T., Natori, T., Kobayashi, K., et al. (2012). CD44 expression in astrocytes and microglia is associated with ALS progression in a mouse model. Neurosci. Lett. 520, 115–120. doi: 10.1016/j.neulet.2012.05.048
McKinsey, G. L., Lizama, C. O., Keown-Lang, A. E., Niu, A., Santander, N., Larpthaveesarp, A., et al. (2020). A new genetic strategy for targeting microglia in development and disease. Elife 9:e54590.
McQuade, A., Kang, Y. J., Hasselmann, J., Jairaman, A., Sotelo, A., Coburn, M., et al. (2020). Gene expression and functional deficits underlie TREM2-knockout microglia responses in human models of Alzheimer’s disease. Nat. Commun. 11:5370.
Meighan, P. C., Meighan, S. E., Davis, C. J., Wright, J. W., and Harding, J. W. (2007). Effects of matrix metalloproteinase inhibition on short- and long-term plasticity of schaffer collateral/CA1 synapses. J. Neurochem. 102, 2085–2096. doi: 10.1111/j.1471-4159.2007.04682.x
Meighan, S. E., Meighan, P. C., Choudhury, P., Davis, C. J., Olson, M. L., Zornes, P. A., et al. (2006). Effects of extracellular matrix-degrading proteases matrix metalloproteinases 3 and 9 on spatial learning and synaptic plasticity. J. Neurochem. 96, 1227–1241. doi: 10.1111/j.1471-4159.2005.03565.x
Metzger, D., Clifford, J., Chiba, H., and Chambon, P. (1995). Conditional site-specific recombination in mammalian cells using a ligand-dependent chimeric Cre recombinase. Proc. Natl. Acad. Sci. U S A. 92, 6991–6995. doi: 10.1073/pnas.92.15.6991
Milior, G., Chali, F., Dos Santos, T., Royer, J., Miles, R., and Morin-Brureau, M. (2019). “Transcriptomics and live imaging to define functional phenotypes of microglia in pathological human tissue,” in Microglia: Methods and Protocols Methods in Molecular Biology, eds O. Garaschuk and A. Verkhratsky (New York, NY: Springer), 325–336. doi: 10.1007/978-1-4939-9658-2_24
Milior, G., Lecours, C., Samson, L., Bisht, K., Poggini, S., Pagani, F., et al. (2016). Fractalkine receptor deficiency impairs microglial and neuronal responsiveness to chronic stress. Brain Behav. Immun. 55, 114–125.
Milior, G., Morin-Brureau, M., Chali, F., Le Duigou, C., Savary, E., Huberfeld, G., et al. (2020). Distinct P2Y receptors mediate extension and retraction of microglial processes in epileptic and peritumoral human tissue. J. Neurosci. 40, 1373–1388. doi: 10.1523/JNEUROSCI.0218-19.2019
Milner, R., and Campbell, I. L. (2002). Cytokines regulate microglial adhesion to laminin and astrocyte extracellular matrix via protein kinase c-dependent activation of the α6β1 integrin. J. Neurosci. 22, 1562–1572.
Mitrasinovic, O. M., Grattan, A., Robinson, C. C., Lapustea, N. B., Poon, C., Ryan, H., et al. (2005). Microglia overexpressing the macrophage colony-stimulating factor receptor are neuroprotective in a microglial-hippocampal organotypic coculture system. J. Neurosci. 25, 4442–4451. doi: 10.1523/JNEUROSCI.0514-05.2005
Møllgård, K., Beinlich, F. R. M., Kusk, P., Miyakoshi, L. M., Delle, C., Plá, V., et al. (2023). A mesothelium divides the subarachnoid space into functional compartments. Science 379, 84–88. doi: 10.1126/science.adc8810
Monaghan, K. L., Zheng, W., Hu, G., and Wan, E. C. K. (2019). Monocytes and monocyte-derived antigen-presenting cells have distinct gene signatures in experimental model of multiple sclerosis. Front. Immunol. 10:2779. doi: 10.3389/fimmu.2019.02779
Montero, M., González, B., and Zimmer, J. (2009). Immunotoxic depletion of microglia in mouse hippocampal slice cultures enhances ischemia-like neurodegeneration. Brain Res. 1291, 140–152. doi: 10.1016/j.brainres.2009.06.097
Moreno, S. G. (2018). Depleting macrophages in vivo with clodronate-liposomes. Methods Mol. Biol. 1784, 259–262.
Morin-Brureau, M., Milior, G., Royer, J., Chali, F., LeDuigou, C., Savary, E., et al. (2018). Microglial phenotypes in the human epileptic temporal lobe. Brain J. Neurol. 141:3343.
Mrdjen, D., Pavlovic, A., Hartmann, F. J., Schreiner, B., Utz, S. G., Leung, B. P., et al. (2018). High-dimensional single-cell mapping of central nervous system immune cells reveals distinct myeloid subsets in health, aging, and disease. Immunity 48, 380–395.e6.
Nahirney, P. C., and Tremblay, M.-E. (2021). Brain ultrastructure: putting the pieces together. Front. Cell Dev. Biol. 9:629503. doi: 10.3389/fcell.2021.629503
Nguyen, P. T., Dorman, L. C., Pan, S., Vainchtein, I. D., Han, R. T., Nakao-Inoue, H., et al. (2020). Microglial remodeling of the extracellular matrix promotes synapse plasticity. Cell 182, 388–403.e15. doi: 10.1016/j.cell.2020.05.050
Nikodemova, M., and Watters, J. J. (2012). Efficient isolation of live microglia with preserved phenotypes from adult mouse brain. J. Neuroinflammation 9:147. doi: 10.1186/1742-2094-9-147
Nimmerjahn, A., Kirchhoff, F., and Helmchen, F. (2005). Resting microglial cells are highly dynamic surveillants of brain parenchyma in vivo. Science 308, 1314–1318.
Nonnenmacher, M., and Weber, T. (2012). Intracellular transport of recombinant adeno-associated virus vectors. Gene Ther. 19, 649–658.
Nutma, E., Ceyzériat, K., Amor, S., Tsartsalis, S., Millet, P., Owen, D. R., et al. (2021a). Cellular sources of TSPO expression in healthy and diseased brain. Eur. J. Nucl. Med. Mol. Imaging 49, 146–163.
Nutma, E., Gebro, E., Marzin, M. C., van der Valk, P., Matthews, P. M., Owen, D. R., et al. (2021b). Activated microglia do not increase 18 kDa translocator protein (TSPO) expression in the multiple sclerosis brain. Glia 69, 2447–2458.
Ohsawa, K., Imai, Y., Sasaki, Y., and Kohsaka, S. (2004). Microglia/macrophage-specific protein Iba1 binds to fimbrin and enhances its actin-bundling activity. J. Neurochem. 88, 844–856. doi: 10.1046/j.1471-4159.2003.02213.x
O’Koren, E. G., Mathew, R., and Saban, D. R. (2016). Fate mapping reveals that microglia and recruited monocyte-derived macrophages are definitively distinguishable by phenotype in the retina. Sci. Rep. 6:20636. doi: 10.1038/srep20636
O’Koren, E. G., Yu, C., Klingeborn, M., Wong, A. Y. W., Prigge, C. L., Mathew, R., et al. (2019). Microglial function is distinct in different anatomical locations during retinal homeostasis and degeneration. Immunity 50, 723–737.e7. doi: 10.1016/j.immuni.2019.02.007
Olah, M., Menon, V., Habib, N., Taga, M. F., Ma, Y., Yung, C. J., et al. (2020). Single cell RNA sequencing of human microglia uncovers a subset associated with Alzheimer’s disease. Nat. Commun. 11:6129. doi: 10.1038/s41467-020-19737-2
Olmos-Alonso, A., Schetters, S. T. T., Sri, S., Askew, K., Mancuso, R., Vargas-Caballero, M., et al. (2016). Pharmacological targeting of CSF1R inhibits microglial proliferation and prevents the progression of Alzheimer’s-like pathology. Brain 139, 891–907. doi: 10.1093/brain/awv379
Opperman, K. S., Vandyke, K., Clark, K. C., Coulter, E. A., Hewett, D. R., Mrozik, K. M., et al. (2019). Clodronate-liposome mediated macrophage depletion abrogates multiple myeloma tumor establishment in vivo. Neoplasia 21, 777–787. doi: 10.1016/j.neo.2019.05.006
Orban, P. C., Chui, D., and Marth, J. D. (1992). Tissue- and site-specific DNA recombination in transgenic mice. Proc. Natl. Acad. Sci. U S A. 89, 6861–6865.
Orr, A. G., Orr, A. L., Li, X.-J., Gross, R. E., and Traynelis, S. F. (2009). Adenosine A(2A) receptor mediates microglial process retraction. Nat. Neurosci. 12, 872–878.
Pagani, F., Paolicelli, R. C., Murana, E., Cortese, B., Di Angelantonio, S., Zurolo, E., et al. (2015). Defective microglial development in the hippocampus of Cx3cr1 deficient mice. Front. Cell Neurosci. 9:111. doi: 10.3389/fncel.2015.00111
Paolicelli, R. C., Sierra, A., Stevens, B., Tremblay, M.-E., Aguzzi, A., Ajami, B., et al. (2022). Microglia states and nomenclature: a field at its crossroads. Neuron 110, 3458–3483.
Parkhurst, C. N., Yang, G., Ninan, I., Savas, J. N., Yates, J. R., Lafaille, J. J., et al. (2013). Microglia promote learning-dependent synapse formation through brain-derived neurotrophic factor. Cell 155, 1596–1609. doi: 10.1016/j.cell.2013.11.030
Patnaik, S., Rai, M., Jalali, S., Agarwal, K., Badakere, A., Puppala, L., et al. (2021). An interplay of microglia and matrix metalloproteinase MMP9 under hypoxic stress regulates the opticin expression in retina. Sci. Rep. 11:7444. doi: 10.1038/s41598-021-86302-2
Peng, J., Wang, K., Xiang, W., Li, Y., Hao, Y., and Guan, Y. (2019). Rosiglitazone polarizes microglia and protects against pilocarpine-induced status epilepticus. CNS Neurosci. Ther. 25, 1363–1372. doi: 10.1111/cns.13265
Penninger, J. M., Irie-Sasaki, J., Sasaki, T., and Oliveira-dos-Santos, A. J. (2001). CD45: new jobs for an old acquaintance. Nat. Immunol. 2, 389–396. doi: 10.1038/87687
Perego, C., Fumagalli, S., and De Simoni, M.-G. (2011). Temporal pattern of expression and colocalization of microglia/macrophage phenotype markers following brain ischemic injury in mice. J. Neuroinflammation 8:174.
Perrone, M., Pagano, M., Belardo, C., Ricciardi, F., Ricciardi, F., Fusco, A., et al. (2023). Potential role of the hydroxyl carboxylic acid receptor type 2 (HCAR2) in microglia pathophysiology: a possible cross-talk with C-X-C chemokine receptor 1 (CXCR1). Neuropharmacology 228:109456. doi: 10.1016/j.neuropharm.2023.109456
Picard, K., Corsi, G., Decoeur, F., Di Castro, M. A., Bordeleau, M., Persillet, M., et al. (2023). Microglial homeostasis disruption modulates non-rapid eye movement sleep duration and neuronal activity in adult female mice. Brain Behav. Immun. 107, 153–164. doi: 10.1016/j.bbi.2022.09.016
Pimenova, A. A., Herbinet, M., Gupta, I., Machlovi, S. I., Bowles, K. R., Marcora, E., et al. (2021). Alzheimer’s-associated PU.1 expression levels regulate microglial inflammatory response. Neurobiol. Dis. 148:105217. doi: 10.1016/j.nbd.2020.105217
Pons, V., Lévesque, P., Plante, M.-M., and Rivest, S. (2021). Conditional genetic deletion of CSF1 receptor in microglia ameliorates the physiopathology of Alzheimer’s disease. Alzheimers Res. Ther. 13:8. doi: 10.1186/s13195-020-00747-7
Poonaki, E., Kahlert, U. D., Meuth, S. G., and Gorji, A. (2022). The role of the ZEB1-neuroinflammation axis in CNS disorders. J. Neuroinflammation 19:275. doi: 10.1186/s12974-022-02636-2
Popova, G., Soliman, S. S., Kim, C. N., Keefe, M. G., Hennick, K. M., Jain, S., et al. (2021). Human microglia states are conserved across experimental models and regulate neural stem cell responses in chimeric organoids. Cell Stem Cell 28, 2153–2166.e6. doi: 10.1016/j.stem.2021.08.015
Prater, K. E., Aloi, M. S., Pathan, J. L., Winston, C. N., Chernoff, R. A., Davidson, S., et al. (2021). A subpopulation of microglia generated in the adult mouse brain originates from Prominin-1-expressing progenitors. J. Neurosci. 41, 7942–7953. doi: 10.1523/JNEUROSCI.1893-20.2021
Quan, Y., Möller, T., and Weinstein, J. R. (2009). Regulation of Fcgamma receptors and immunoglobulin G-mediated phagocytosis in mouse microglia. Neurosci. Lett. 464, 29–33. doi: 10.1016/j.neulet.2009.08.013
Rajan, W. D., Wojtas, B., Gielniewski, B., Miró-Mur, F., Pedragosa, J., Zawadzka, M., et al. (2020). Defining molecular identity and fates of CNS-border associated macrophages after ischemic stroke in rodents and humans. Neurobiol Dis. 137:104722. doi: 10.1016/j.nbd.2019.104722
Ralvenius, W. T., Mungenast, A. E., Woolf, H., Huston, M. M., Gillingham, T. Z., Godin, S. K., et al. (2023). A novel molecular class that recruits HDAC/MECP2 complexes to PU.1 motifs reduces neuroinflammation. J. Exp. Med. 220, e20222105. doi: 10.1084/jem.20222105
Rangaraju, S., Raza, S. A., Li, N. X., Betarbet, R., Dammer, E. B., Duong, D., et al. (2018). Differential phagocytic properties of CD45low microglia and CD45high brain mononuclear phagocytes—activation and age-related effects. Front. Immunol. 9:405. doi: 10.3389/fimmu.2018.00405
Ransohoff, R. M., and El Khoury, J. (2015). Microglia in health and disease. Cold Spring Harb. Perspect. Biol. 8:a020560.
Reifschneider, A., Robinson, S., van Lengerich, B., Gnörich, J., Logan, T., Heindl, S., et al. (2022). Loss of TREM2 rescues hyperactivation of microglia, but not lysosomal deficits and neurotoxicity in models of progranulin deficiency. EMBO J. 41:e109108. doi: 10.15252/embj.2021109108
Reinhard, S. M., Razak, K., and Ethell, I. M. (2015). A delicate balance: role of MMP-9 in brain development and pathophysiology of neurodevelopmental disorders. Front. Cell Neurosci. 9:280. doi: 10.3389/fncel.2015.00280
Rice, R. A., Pham, J., Lee, R. J., Najafi, A. R., West, B. L., and Green, K. N. (2017). Microglial repopulation resolves inflammation and promotes brain recovery after injury. Glia 65, 931–944. doi: 10.1002/glia.23135
Rojo, R., Raper, A., Ozdemir, D. D., Lefevre, L., Grabert, K., Wollscheid-Lengeling, E., et al. (2019). Deletion of a Csf1r enhancer selectively impacts CSF1R expression and development of tissue macrophage populations. Nat. Commun. 10:3215. doi: 10.1038/s41467-019-11053-8
Rooijen, N. V., and Sanders, A. (1994). Liposome mediated depletion of macrophages: mechanism of action, preparation of liposomes and applications. J. Immunol. Methods 174, 83–93. doi: 10.1016/0022-1759(94)90012-4
Rosell, A., Ortega-Aznar, A., Alvarez-Sabín, J., Fernández-Cadenas, I., Ribó, M., Molina, C. A., et al. (2006). Increased brain expression of matrix Metalloproteinase-9 after ischemic and hemorrhagic human stroke. Stroke 37, 1399–1406.
Rosito, M., Sanchini, C., Gosti, G., Moreno, M., De Panfilis, S., Giubettini, M., et al. (2023). Microglia reactivity entails microtubule remodeling from acentrosomal to centrosomal arrays. Cell Rep. 42:112104. doi: 10.1016/j.celrep.2023.112104
Ruan, C., Sun, L., Kroshilina, A., Beckers, L., De Jager, P., Bradshaw, E. M., et al. (2020). A novel Tmem119-tdTomato reporter mouse model for studying microglia in the central nervous system. Brain Behav. Immun. 83, 180–191. doi: 10.1016/j.bbi.2019.10.009
Rubino, S. J., Mayo, L., Wimmer, I., Siedler, V., Brunner, F., Hametner, S., et al. (2018). Acute microglia ablation induces neurodegeneration in the somatosensory system. Nat. Commun. 9:4578. doi: 10.1038/s41467-018-05929-4
Rustenhoven, J., Park, T. I.-H., Schweder, P., Scotter, J., Correia, J., Smith, A. M., et al. (2016). Isolation of highly enriched primary human microglia for functional studies. Sci. Rep. 6:19371.
Rustenhoven, J., Smith, A. M., Smyth, L. C., Jansson, D., Scotter, E. L., Swanson, M. E. V., et al. (2018). PU.1 regulates Alzheimer’s disease-associated genes in primary human microglia. Mol. Neurodegeneration 13:44.
Sahasrabuddhe, V., and Ghosh, H. S. (2022). Cx3Cr1-Cre induction leads to microglial activation and IFN-1 signaling caused by DNA damage in early postnatal brain. Cell Rep. 38:110252. doi: 10.1016/j.celrep.2021.110252
Salman, H., Shuai, X., Nguyen-Lefebvre, A. T., Giri, B., Ren, M., Rauchman, M., et al. (2018). SALL1 expression in acute myeloid leukemia. Oncotarget 9, 7442–7452. doi: 10.18632/oncotarget.23448
Sanchez Mejia, R. O., Ona, V. O., Li, M., and Friedlander, R. M. (2001). Minocycline reduces traumatic brain injury-mediated caspase-1 activation, tissue damage, and neurological dysfunction. Neurosurgery 48, 1393–1399. doi: 10.1097/00006123-200106000-00051
Sasaki, Y., Ohsawa, K., Kanazawa, H., Kohsaka, S., and Imai, Y. (2001). Iba1 is an actin-cross-linking protein in macrophages/microglia. Biochem. Biophys. Res. Commun. 286, 292–297. doi: 10.1006/bbrc.2001.5388
Satoh, J., Kino, Y., Asahina, N., Takitani, M., Miyoshi, J., Ishida, T., et al. (2016). TMEM119 marks a subset of microglia in the human brain. Neuropathology 36, 39–49. doi: 10.1111/neup.12235
Savage, J. C., St-Pierre, M.-K., Carrier, M., El Hajj, H., Novak, S. W., Sanchez, M. G., et al. (2020). Microglial physiological properties and interactions with synapses are altered at presymptomatic stages in a mouse model of Huntington’s disease pathology. J. Neuroinflammation 17:98. doi: 10.1186/s12974-020-01782-9
Savchenko, V. L., Nikonenko, I. R., Skibo, G. G., and McKanna, J. A. (1997). Distribution of microglia and astrocytes in different regions of the normal adult rat brain. Neurophysiology 29, 343–351.
Saxena, S., Kruys, V., Vamecq, J., and Maze, M. (2021). The role of microglia in perioperative neuroinflammation and neurocognitive disorders. Front. Aging Neurosci. 13:671499. doi: 10.3389/fnagi.2021.671499
Schmid, M. C., Khan, S. Q., Kaneda, M. M., Pathria, P., Shepard, R., Louis, T. L., et al. (2018). Integrin CD11b activation drives anti-tumor innate immunity. Nat. Commun. 9:5379.
Scholz, R., Sobotka, M., Caramoy, A., Stempfl, T., Moehle, C., and Langmann, T. (2015). Minocycline counter-regulates pro-inflammatory microglia responses in the retina and protects from degeneration. J. Neuroinflammation 12:209. doi: 10.1186/s12974-015-0431-4
Schulz, C., Gomez Perdiguero, E., Chorro, L., Szabo-Rogers, H., Cagnard, N., Kierdorf, K., et al. (2012). A lineage of myeloid cells independent of Myb and hematopoietic stem cells. Science 336, 86–90.
Scott, E. P., Breyak, E., Nishinakamura, R., and Nakagawa, Y. (2022). The zinc finger transcription factor Sall1 is required for the early developmental transition of microglia in mouse embryos. Glia 70, 1720–1733. doi: 10.1002/glia.24192
Scott-Hewitt, N., Perrucci, F., Morini, R., Erreni, M., Mahoney, M., Witkowska, A., et al. (2020). Local externalization of phosphatidylserine mediates developmental synaptic pruning by microglia. EMBO J. 39:e105380. doi: 10.15252/embj.2020105380
Seelaar, H., and Van Swieten, J. C. (2021). In vivo PET imaging of neuroinflammation in familial frontotemporal dementia. J. Neurol. Neurosurg. Psychiatry 92:231.
Segal, B. M., and Giger, R. J. (2016). Stable biomarker for plastic microglia. Proc. Natl. Acad. Sci. U S A. 113, 3130–3132. doi: 10.1073/pnas.1601669113
Serganova, I., and Blasberg, R. G. (2019). Molecular imaging with reporter genes: has its promise been delivered? J. Nucl. Med. 60, 1665–1681.
Shah, S., Wong, L. M., Ellis, K., Bodnar, B., Saribas, S., Ting, J., et al. (2022). Microglia-specific promoter activities of HEXB gene. Front. Cell Neurosci. 16:808598. doi: 10.3389/fncel.2022.808598
Shah, V. B., Huang, Y., Keshwara, R., Ozment-Skelton, T., Williams, D. L., and Keshvara, L. (2008). β-Glucan activates microglia without inducing cytokine production in Dectin-1-dependent manner. J. Immunol. 180, 2777–2785.
Shahidehpour, R. K., Higdon, R. E., Crawford, N. G., Neltner, J. H., Ighodaro, E. T., Patel, E., et al. (2021). Dystrophic microglia are associated with neurodegenerative disease and not healthy aging in the human brain. Neurobiol. Aging 99, 19–27.
Shapiro, L. A., Perez, Z. D., Foresti, M. L., Arisi, G. M., and Ribak, C. E. (2009). Morphological and ultrastructural features of Iba1-immunolabeled microglial cells in the hippocampal dentate gyrus. Brain Res. 1266, 29–36. doi: 10.1016/j.brainres.2009.02.031
Sharma, K., Bisht, K., and Eyo, U. B. (2021). A comparative biology of microglia across species. Front. Cell Dev. Biol. 9, 652748.
Sheng, J., Ruedl, C., and Karjalainen, K. (2015). Most tissue-resident macrophages except microglia are derived from fetal hematopoietic stem cells. Immunity 43, 382–393.
Shi, F.-J., Xie, H., Zhang, C.-Y., Qin, H.-F., Zeng, X.-W., Lou, H., et al. (2021). Is Iba-1 protein expression a sensitive marker for microglia activation in experimental diabetic retinopathy? Int. J. Ophthalmol. 14, 200–208. doi: 10.18240/ijo.2021.02.04
Shi, L., Rocha, M., Zhang, W., Jiang, M., Li, S., Ye, Q., et al. (2020). Genome-wide transcriptomic analysis of microglia reveals impaired responses in aged mice after cerebral ischemia. J. Cereb. Blood Flow Metab. 40, S49–S66. doi: 10.1177/0271678X20925655
Shi, W., Zhang, J., Shang, Z., Zhang, Y., Xia, Y., Fu, H., et al. (2022). Restorative therapy using microglial depletion and repopulation for central nervous system injuries and diseases. Front. Immunol. 13:969127. doi: 10.3389/fimmu.2022.969127
Shih, Y.-C., Chen, P.-Y., Ko, T.-M., Huang, P.-H., Ma, H., and Tarng, D.-C. (2021). MMP-9 deletion attenuates arteriovenous fistula neointima through reduced perioperative vascular inflammation. Int. J. Mol. Sci. 22:5448. doi: 10.3390/ijms22115448
Sierksma, A., Lu, A., Mancuso, R., Fattorelli, N., Thrupp, N., Salta, E., et al. (2020). Novel Alzheimer risk genes determine the microglia response to amyloid-β but not to TAU pathology. EMBO Mol. Med. 12:e10606.
Sierra, A., Paolicelli, R. C., and Kettenmann, H. (2019). Cien Años de Microglía: milestones in a century of microglial research. Trends Neurosci. 42, 778–792.
Simpson, D., Gharehgazlou, A., Da Silva, T., Labrie-Cleary, C., Wilson, A. A., Meyer, J. H., et al. (2022). In vivo imaging translocator protein (TSPO) in autism spectrum disorder. Neuropsychopharmacology 47, 1421–1427.
Sims, R., van der Lee, S. J., Naj, A. C., Bellenguez, C., Badarinarayan, N., Jakobsdottir, J., et al. (2017). Rare coding variants in PLCG2, ABI3, and TREM2 implicate microglial-mediated innate immunity in Alzheimer’s disease. Nat. Genet. 49, 1373–1384. doi: 10.1038/ng.3916
Sipe, G. O., Lowery, R. L., Tremblay, M. -È, Kelly, E. A., Lamantia, C. E., and Majewska, A. K. (2016). Microglial P2Y12 is necessary for synaptic plasticity in mouse visual cortex. Nat. Commun. 7:10905.
Šišková, Z., and Tremblay, M. -È (2013). Microglia and synapse: interactions in health and neurodegeneration. Neural Plast. 2013:425845.
Smith, J. A., Das, A., Ray, S. K., and Banik, N. L. (2012). Role of pro-inflammatory cytokines released from microglia in neurodegenerative diseases. Brain Res. Bull. 87, 10–20.
Sobue, A., Komine, O., Hara, Y., Endo, F., Mizoguchi, H., Watanabe, S., et al. (2021). Microglial gene signature reveals loss of homeostatic microglia associated with neurodegeneration of Alzheimer’s disease. Acta Neuropathol. Commun. 9:1.
Song, L., Lee, C., and Schindler, C. (2011). Deletion of the murine scavenger receptor CD68[S]. J. Lipid Res. 52, 1542–1550.
Song, W. M., and Colonna, M. (2018). The identity and function of microglia in neurodegeneration. Nat. Immunol. 19, 1048–1058.
Sousa, C., Golebiewska, A., Poovathingal, S. K., Kaoma, T., Pires-Afonso, Y., Martina, S., et al. (2018). Single-cell transcriptomics reveals distinct inflammation-induced microglia signatures. EMBO Rep. 19:e46171. doi: 10.15252/embr.201846171
Spangenberg, E., Severson, P. L., Hohsfield, L. A., Crapser, J., Zhang, J., Burton, E. A., et al. (2019). Sustained microglial depletion with CSF1R inhibitor impairs parenchymal plaque development in an Alzheimer’s disease model. Nat. Commun. 10:3758. doi: 10.1038/s41467-019-11674-z
Speicher, A. M., Wiendl, H., Meuth, S. G., and Pawlowski, M. (2019). Generating microglia from human pluripotent stem cells: novel in vitro models for the study of neurodegeneration. Mol. Neurodegener. 14:46. doi: 10.1186/s13024-019-0347-z
Stojiljkovic, M. R., Schmeer, C., and Witte, O. W. (2022). Pharmacological depletion of microglia leads to a dose-dependent reduction in inflammation and senescence in the aged murine brain. Neuroscience 488, 1–9. doi: 10.1016/j.neuroscience.2022.02.018
St-Pierre, M.-K., Carrier, M., González Ibáñez, F., Šimončičová, E., Wallman, M.-J., Valličres, L., et al. (2022a). Ultrastructural characterization of dark microglia during aging in a mouse model of Alzheimer’s disease pathology and in human post-mortem brain samples. J. Neuroinflammation 19:235. doi: 10.1186/s12974-022-02595-8
St-Pierre, M.-K., Carrier, M., Lau, V., and Tremblay, M. -È (2022b). Investigating microglial ultrastructural alterations and intimate relationships with neuronal stress, dystrophy, and degeneration in mouse models of Alzheimer’s disease. Methods Mol. Biol. 2515, 29–58. doi: 10.1007/978-1-0716-2409-8_3
St-Pierre, M.-K., Šimončičová, E., Bögi, E., and Tremblay, M. -È (2020). Shedding light on the dark side of the microglia. ASN Neuro 12:1759091420925335. doi: 10.1177/1759091420925335
Strahan, J. A., Walker, W. H., Montgomery, T. R., and Forger, N. G. (2017). Minocycline causes widespread cell death and increases microglial labeling in the neonatal mouse brain. Dev. Neurobiol. 77, 753–766. doi: 10.1002/dneu.22457
Stratoulias, V., Venero, J. L., and Tremblay, M. -È, and Joseph, B. (2019). Microglial subtypes: diversity within the microglial community. EMBO J. 38:e101997.
Streit, W. J., Braak, H., Xue, Q.-S., and Bechmann, I. (2009). Dystrophic (senescent) rather than activated microglial cells are associated with tau pathology and likely precede neurodegeneration in Alzheimer’s disease. Acta Neuropathol. 118, 475–485. doi: 10.1007/s00401-009-0556-6
Streit, W. J., Khoshbouei, H., and Bechmann, I. (2020). Dystrophic microglia in late-onset Alzheimer’s disease. Glia 68, 845–854.
Streit, W. J., Sammons, N. W., Kuhns, A. J., and Sparks, D. L. (2004). Dystrophic microglia in the aging human brain. Glia 45, 208–212.
Sun, R., Yang, Y., Gu, Z., Tang, X., Zhang, C., Kou, W., et al. (2019). Silencing of CD86 in dendritic cells by small interfering RNA regulates cytokine production in T cells from patients with allergic rhinitis in vitro. Mol. Med. Rep. 20, 3893–3900. doi: 10.3892/mmr.2019.10638
Suzuki, Y., Shirai, M., Asada, K., Yasui, H., Karayama, M., Hozumi, H., et al. (2018). Macrophage mannose receptor, CD206, predict prognosis in patients with pulmonary tuberculosis. Sci. Rep. 8:13129. doi: 10.1038/s41598-018-31565-5
Swanson, M. E. V., Mrkela, M., Murray, H. C., Cao, M. C., Turner, C., Curtis, M. A., et al. (2023). Microglial CD68 and L-ferritin upregulation in response to phosphorylated-TDP-43 pathology in the amyotrophic lateral sclerosis brain. Acta Neuropathol. Commun. 11:69. doi: 10.1186/s40478-023-01561-6
Swanson, M. E. V., Scotter, E. L., Smyth, L. C. D., Murray, H. C., Ryan, B., Turner, C., et al. (2020). Identification of a dysfunctional microglial population in human Alzheimer’s disease cortex using novel single-cell histology image analysis. Acta Neuropathol. Commun. 8:170. doi: 10.1186/s40478-020-01047-9
Szepesi, Z., Hosy, E., Ruszczycki, B., Bijata, M., Pyskaty, M., Bikbaev, A., et al. (2014). Synaptically released matrix metalloproteinase activity in control of structural plasticity and the cell surface distribution of GluA1-AMPA receptors. PLoS One 9:e98274. doi: 10.1371/journal.pone.0098274
Szepesi, Z., Manouchehrian, O., Bachiller, S., and Deierborg, T. (2018). Bidirectional microglia–neuron communication in health and disease. Front. Cell. Neurosci. 12:323. doi: 10.3389/fncel.2018.00323
Takagi, S., Murayama, S., Torii, K., Takemura-Morita, S., Kurganov, E., Nagaoka, S., et al. (2020). Depletion of microglia and macrophages with clodronate liposomes attenuates zymosan-induced Fos expression and hypothermia in the adult mouse. J. Neuroimmunol. 344:577244. doi: 10.1016/j.jneuroim.2020.577244
Tan, S. Y., and Dee, M. K. (2009). Elie Metchnikoff (1845-1916): discoverer of phagocytosis. Singapore Med. J. 50, 456–457.
Tanaka, S., Ohgidani, M., Hata, N., Inamine, S., Sagata, N., Shirouzu, N., et al. (2021). CD206 expression in induced microglia-like cells from peripheral blood as a surrogate biomarker for the specific immune microenvironment of neurosurgical diseases including Glioma. Front. Immunol. 12:670131. doi: 10.3389/fimmu.2021.670131
Tang, P. W., Chua, P. S., Chong, S. K., Mohamad, M. S., Choon, Y. W., Deris, S., et al. (2015). A review of gene knockout strategies for microbial cells. Recent Pat. Biotechnol. 9, 176–197.
Tang, Z., Gan, Y., Liu, Q., Yin, J.-X., Liu, Q., Shi, J., et al. (2014). CX3CR1 deficiency suppresses activation and neurotoxicity of microglia/macrophage in experimental ischemic stroke. J. Neuroinflammation 11:26. doi: 10.1186/1742-2094-11-26
Tay, T. L., Mai, D., Dautzenberg, J., Fernández-Klett, F., Lin, G., and Sagar, et al. (2017). A new fate mapping system reveals context-dependent random or clonal expansion of microglia. Nat. Neurosci. 20, 793–803.
Theocharis, A. D., Skandalis, S. S., Gialeli, C., and Karamanos, N. K. (2016). Extracellular matrix structure. Adv. Drug Deliv. Rev. 97, 4–27.
Thomas, M., and Le, W. D. (2004). Minocycline: neuroprotective mechanisms in Parkinson’s disease. Curr. Pharm. Des. 10, 679–686.
Tremblay, M. -È, Lowery, R. L., and Majewska, A. K. (2010). Microglial interactions with synapses are modulated by visual experience. PLoS Biol. 8:e1000527. doi: 10.1371/journal.pbio.1000527
Tremblay, M. -È, and Majewska, A. K. (2011). A role for microglia in synaptic plasticity? Commun. Integr. Biol. 4, 220–222.
Tremblay, M. -È, and Majewska, A. K. (2019). Ultrastructural analyses of microglial interactions with synapses. Methods Mol. Biol. 2034, 83–95.
Trzupek, D., Dunstan, M., Cutler, A. J., Lee, M., Godfrey, L., Jarvis, L., et al. (2020). Discovery of CD80 and CD86 as recent activation markers on regulatory T cells by protein-RNA single-cell analysis. Genome Med. 12:55. doi: 10.1186/s13073-020-00756-z
Unger, M. S., Schernthaner, P., Marschallinger, J., Mrowetz, H., and Aigner, L. (2018). Microglia prevent peripheral immune cell invasion and promote an anti-inflammatory environment in the brain of APP-PS1 transgenic mice. J. Neuroinflammation 15:274. doi: 10.1186/s12974-018-1304-4
Vainio, S. K., Dickens, A. M., Matilainen, M., López-Picón, F. R., Aarnio, R., Eskola, O., et al. (2022). Dimethyl fumarate decreases short-term but not long-term inflammation in a focal EAE model of neuroinflammation. EJNMMI Res. 12:6.
Van Hove, H., Antunes, A. R. P., De Vlaminck, K., Scheyltjens, I., Van Ginderachter, J. A., and Movahedi, K. (2020). Identifying the variables that drive tamoxifen-independent CreERT2 recombination: implications for microglial fate mapping and gene deletions. Eur. J. Immunol. 50, 459–463. doi: 10.1002/eji.201948162
Van Hove, H., Martens, L., Scheyltjens, I., De Vlaminck, K., Pombo Antunes, A. R., De Prijck, S., et al. (2019). A single-cell atlas of mouse brain macrophages reveals unique transcriptional identities shaped by ontogeny and tissue environment. Nat. Neurosci. 22, 1021–1035. doi: 10.1038/s41593-019-0393-4
van Wageningen, T. A., Vlaar, E., Kooij, G., Jongenelen, C. A. M., Geurts, J. J. G., and van Dam, A.-M. (2019). Regulation of microglial TMEM119 and P2RY12 immunoreactivity in multiple sclerosis white and grey matter lesions is dependent on their inflammatory environment. Acta Neuropathol. Commun. 7:206. doi: 10.1186/s40478-019-0850-z
VanderZwaag, J., Halvorson, T., Dolhan, K., Šimončičová, E., Ben-Azu, B., and Tremblay, M. -È (2023). The missing piece? a case for Microglia’s prominent role in the therapeutic action of anesthetics, ketamine, and psychedelics. Neurochem. Res. 48, 1129–1166. doi: 10.1007/s11064-022-03772-0
Vankriekelsvenne, E., Chrzanowski, U., Manzhula, K., Greiner, T., Wree, A., Hawlitschka, A., et al. (2022). Transmembrane protein 119 is neither a specific nor a reliable marker for microglia. Glia 70, 1170–1190.
Venturino, A., Schulz, R., De Jesús-Cortés, H., Maes, M. E., Nagy, B., Reilly-Andújar, F., et al. (2021). Microglia enable mature perineuronal nets disassembly upon anesthetic ketamine exposure or 60-Hz light entrainment in the healthy brain. Cell Rep. 36:109313. doi: 10.1016/j.celrep.2021.109313
Vondra, S., Höbler, A.-L., Lackner, A. I., Raffetseder, J., Mihalic, Z. N., Vogel, A., et al. (2023). The human placenta shapes the phenotype of decidual macrophages. Cell Rep. 42:111977.
Walker, D. G., Tang, T. M., Mendsaikhan, A., Tooyama, I., Serrano, G. E., Sue, L. I., et al. (2020). Patterns of expression of purinergic receptor P2RY12, a putative marker for non-activated microglia, in aged and Alzheimer’s disease brains. Int. J. Mol. Sci. 21:678. doi: 10.3390/ijms21020678
Waller, R., Baxter, L., Fillingham, D. J., Coelho, S., Pozo, J. M., Mozumder, M., et al. (2019). Iba-1-/CD68+ microglia are a prominent feature of age-associated deep subcortical white matter lesions. PLoS One 14:e0210888. doi: 10.1371/journal.pone.0210888
Wang, C., and Sun, H. (2019). [Progress in gene knockout mice]. Sheng Wu Gong Cheng Xue Bao 35, 784–794.
Wang, Q., Davis, P. B., Qi, X., Chen, S. G., Gurney, M. E., Perry, G., et al. (2021). Gut–microbiota–microglia–brain interactions in Alzheimer’s disease: knowledge-based, multi-dimensional characterization. Alzheimer’s Res. Ther. 13:177. doi: 10.1186/s13195-021-00917-1
Wang, S., Sudan, R., Peng, V., Zhou, Y., Du, S., Yuede, C. M., et al. (2022). TREM2 drives microglia response to amyloid-β via SYK-dependent and -independent pathways. Cell 185, 4153–4169.e19.
Wang, Y., Cella, M., Mallinson, K., Ulrich, J. D., Young, K. L., Robinette, M. L., et al. (2015). TREM2 lipid sensing sustains the microglial response in an Alzheimer’s disease model. Cell 160, 1061–1071. doi: 10.1016/j.cell.2015.01.049
Wang, Y., Szretter, K. J., Vermi, W., Gilfillan, S., Rossini, C., Cella, M., et al. (2012). IL-34 is a tissue-restricted ligand of CSF1R required for the development of Langerhans cells and microglia. Nat. Immunol. 13, 753–760. doi: 10.1038/ni.2360
Weisser, S. B., van Rooijen, N., and Sly, L. M. (2012). Depletion and reconstitution of macrophages in mice. J. Vis. Exp. 4105. doi: 10.3791/4105
Wieghofer, P., Hagemeyer, N., Sankowski, R., Schlecht, A., Staszewski, O., Amann, L., et al. (2021). Mapping the origin and fate of myeloid cells in distinct compartments of the eye by single-cell profiling. EMBO J. 40:e105123. doi: 10.15252/embj.2020105123
Wieghofer, P., Knobeloch, K.-P., and Prinz, M. (2015). Genetic targeting of microglia. Glia 63, 1–22.
Wolf, Y., Yona, S., Kim, K.-W., and Jung, S. (2013). Microglia, seen from the CX3CR1 angle. Front. Cell Neurosci. 7:26. doi: 10.3389/fncel.2013.00026
Woo, M.-S., Park, J.-S., Choi, I.-Y., Kim, W.-K., and Kim, H.-S. (2008). Inhibition of MMP-3 or -9 suppresses lipopolysaccharide-induced expression of proinflammatory cytokines and iNOS in microglia. J. Neurochem. 106, 770–780.
Wu, W., Li, Y., Wei, Y., Bosco, D. B., Xie, M., Zhao, M.-G., et al. (2020). Microglial depletion aggravates the severity of acute and chronic seizures in mice. Brain Behav. Immunity 89, 245–255. doi: 10.1016/j.bbi.2020.06.028
Wu, X., Saito, T., Saido, T. C., Barron, A. M., and Ruedl, C. (2021). Microglia and CD206+ border-associated mouse macrophages maintain their embryonic origin during Alzheimer’s disease. Elife 10:e71879. doi: 10.7554/eLife.71879
Xia, Y., Vetvicka, V., Yan, J., Hanikýrová, M., Mayadas, T., and Ross, G. D. (1999). The beta-glucan-binding lectin site of mouse CR3 (CD11b/CD18) and its function in generating a primed state of the receptor that mediates cytotoxic activation in response to iC3b-opsonized target cells. J. Immunol. 162, 2281–2290.
Xing, C., Li, W., Deng, W., Ning, M., and Lo, E. H. (2018). A potential gliovascular mechanism for microglial activation: differential phenotypic switching of microglia by endothelium versus astrocytes. J. Neuroinflammation 15:143. doi: 10.1186/s12974-018-1189-2
Yaghmoor, F., Noorsaeed, A., Alsaggaf, S., Aljohani, W., Scholtzova, H., Boutajangout, A., et al. (2014). The Role of TREM2 in Alzheimer’s disease and other neurological disorders. J. Alzheimers Dis. Parkinsonism 4:160.
Yang, B., Wang, F., and Zheng, G. (2021). Transmembrane protein TMEM119 facilitates the stemness of breast cancer cells by activating Wnt/β-catenin pathway. Bioengineered 12, 4856–4867.
Yankam Njiwa, J., Costes, N., Bouillot, C., Bouvard, S., Fieux, S., Becker, G., et al. (2017). Quantitative longitudinal imaging of activated microglia as a marker of inflammation in the pilocarpine rat model of epilepsy using [11C]-(R)-PK11195 PET and MRI. J. Cereb. Blood Flow Metab. 37, 1251–1263.
Yao, Y., Echeverry, S., Shi, X. Q., Yang, M., Yang, Q. Z., Wang, G. Y. F., et al. (2016). Dynamics of spinal microglia repopulation following an acute depletion. Sci. Rep. 6:22839. doi: 10.1038/srep22839
Yeo, H.-G., Hong, J. J., Lee, Y., Yi, K. S., Jeon, C.-Y., Park, J., et al. (2019). Increased CD68/TGFβ co-expressing microglia/ macrophages after transient middle cerebral artery occlusion in rhesus monkeys. Exp. Neurobiol. 28, 458–473.
Yi, S. Y., Barnett, B. R., Torres-Velázquez, M., Zhang, Y., Hurley, S. A., Rowley, P. A., et al. (2019). Detecting microglial density with quantitative multi-compartment diffusion MRI. Front. Neurosci. 13:81. doi: 10.3389/fnins.2019.00081
Yona, S., Kim, K.-W., Wolf, Y., Mildner, A., Varol, D., Breker, M., et al. (2013). Fate mapping reveals origins and dynamics of monocytes and tissue macrophages under homeostasis. Immunity 38, 79–91. doi: 10.1016/j.immuni.2012.12.001
Yrjänheikki, J., Keinänen, R., Pellikka, M., Hökfelt, T., and Koistinaho, J. (1998). Tetracyclines inhibit microglial activation and are neuroprotective in global brain ischemia. Proc. Natl. Acad. Sci. U S A. 95, 15769–15774.
Yusuying, S., Yusuyin, S., and Cheng, X. (2022). Translocator protein regulate polarization phenotype transformation of microglia after cerebral ischemia–reperfusion injury. Neuroscience 480, 203–216.
Zhan, L., Fan, L., Kodama, L., Sohn, P. D., Wong, M. Y., Mousa, G. A., et al. (2020). A MAC2-positive progenitor-like microglial population is resistant to CSF1R inhibition in adult mouse brain. Elife 9:e51796. doi: 10.7554/eLife.51796
Zhang, Y., Zhao, L., Wang, X., Ma, W., Lazere, A., Qian, H.-H., et al. (2018). Repopulating retinal microglia restore endogenous organization and function under CX3CL1-CX3CR1 regulation. Sci. Adv. 4:eaa8492. doi: 10.1126/sciadv.aap8492
Zhao, J.-F., Ren, T., Li, X.-Y., Guo, T.-L., Liu, C.-H., and Wang, X. (2022). Research progress on the role of microglia membrane proteins or receptors in neuroinflammation and degeneration. Front. Cell Neurosci. 16:831977. doi: 10.3389/fncel.2022.831977
Zhao, X.-F., Alam, M. M., Liao, Y., Huang, T., Mathur, R., Zhu, X., et al. (2019). Targeting microglia using Cx3cr1-Cre lines: revisiting the specificity. eNeuro 6:ENEURO.0114-19.2019. doi: 10.1523/ENEURO.0114-19.2019
Zhong, L., Chen, X.-F., Wang, T., Wang, Z., Liao, C., Wang, Z., et al. (2017). Soluble TREM2 induces inflammatory responses and enhances microglial survival. J. Exp. Med. 214, 597–607. doi: 10.1084/jem.20160844
Zhou, X., Ji, B., Seki, C., Nagai, Y., Minamimoto, T., Fujinaga, M., et al. (2021). PET imaging of colony-stimulating factor 1 receptor: a head-to-head comparison of a novel radioligand, 11C-GW2580, and 11C-CPPC, in mouse models of acute and chronic neuroinflammation and a rhesus monkey. J. Cereb. Blood Flow Metab. 41, 2410–2422. doi: 10.1177/0271678X211004146
Keywords: microglia, microglial markers, fate mapping, electron microscopy, positron emission tomography, reporter genes, microglial depletion, Cre/lox systems
Citation: Bobotis BC, Halvorson T, Carrier M and Tremblay M-È (2024) Established and emerging techniques for the study of microglia: visualization, depletion, and fate mapping. Front. Cell. Neurosci. 18:1317125. doi: 10.3389/fncel.2024.1317125
Received: 10 October 2023; Accepted: 15 January 2024;
Published: 15 February 2024.
Edited by:
Maria Grazia Mola, University of Bari Aldo Moro, ItalyReviewed by:
Daniel Erny, University of Freiburg Medical Center, GermanyGiampaolo Milior, Collège de France, France
Copyright © 2024 Bobotis, Halvorson, Carrier and Tremblay. This is an open-access article distributed under the terms of the Creative Commons Attribution License (CC BY). The use, distribution or reproduction in other forums is permitted, provided the original author(s) and the copyright owner(s) are credited and that the original publication in this journal is cited, in accordance with accepted academic practice. No use, distribution or reproduction is permitted which does not comply with these terms.
*Correspondence: Marie-Ève Tremblay, ZXZldHJlbWJsYXlAdXZpYy5jYQ==
†These authors have contributed equally to this work and share first authorship