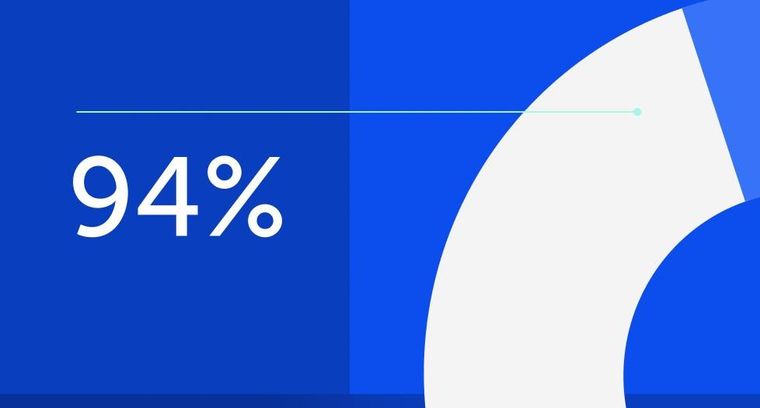
94% of researchers rate our articles as excellent or good
Learn more about the work of our research integrity team to safeguard the quality of each article we publish.
Find out more
MINI REVIEW article
Front. Cell. Neurosci., 21 December 2023
Sec. Cellular Neurophysiology
Volume 17 - 2023 | https://doi.org/10.3389/fncel.2023.1327621
This article is part of the Research TopicNew Insights into Intracellular Pathways and Therapeutic Targets in CNS DiseasesView all 10 articles
Glioblastoma (GB) is a highly malignant primary brain tumor with limited treatment options and poor prognosis. Despite current treatment approaches, including surgical resection, radiation therapy, and chemotherapy with temozolomide (TMZ), GB remains mostly incurable due to its invasive growth pattern, limited drug penetration beyond the blood-brain barrier (BBB), and resistance to conventional therapies. One of the main challenges in GB treatment is effectively eliminating infiltrating cancer cells that remain in the brain parenchyma after primary tumor resection. We’ve reviewed the most recent challenges and surveyed the potential strategies aimed at enhancing local treatment outcomes.
Glioblastoma is the most prevalent malignant primary brain tumor and recent scientific and technical advances have allowed for a deeper understanding of the etiologic relevance of the heterogeneity of GB. Based on the current classification of the tumors of the central nervous system (CNS), among adult-type diffuse gliomas, astrocytic tumors without mutations in the Isocitrate dehydrogenase (IDH) genes are termed glioblastomas IDH-wildtype (Torp et al., 2022). The presence of microvascular proliferation and/or necrosis and at least one molecular alteration identified as predictive of tumor aggressiveness, such as Epithelial Growth Factor Receptor (EGFR) amplification, Telomerase promoter (TERTp) mutations (Brás et al., 2023) up-regulation of chromosome 7 and loss of chromosome 10 (Zhao et al., 2023) allows for a diagnosis of glioblastoma IDH-wildtype CNS WHO grade 4 (since now, GB).
Despite all the innovation that generates the “holistic representation of the evolving disease” (Asleh et al., 2023), GB remains mostly incurable, with a median survival of 14 months and a 5-year survival rate of 5.7%, primarily due to its challenging characteristics: (i) invasive growth pattern, making complete surgical resection nearly impossible; (ii) localization beyond the blood-brain barrier (BBB), which severely limits drug penetration; (iii) resistance to conventional radiotherapy and chemotherapy (Grochans et al., 2022).
Indeed, GB is strongly refractory to most anti-tumor treatments, one of the main reasons lying in the difficulties of tackling its cellular heterogeneity. In the tumor, in fact, the cell population is represented by differentiated GB cells, stem-like cells (GSCs) as well as by some elements of the microenvironment milieu among which endothelial cells, vascular pericytes, macrophages, and other types of immune cells (Zhao et al., 2023). Sequencing technology advances and transcriptional stratification has further corroborated the complexity of this picture contributing to further subdivide GB in proneural, classical and mesenchymal subtypes. In this scenario, the developmental dynamicity of GSCs add another layer of complexity to the heterogeneous nature of the tumors. GSCs are indeed considered at the top of hierarchic lineage of cells holding stem cell-like regeneration ability (Lathia et al., 2015) but share several common molecular markers with normal adult neural stem cells and progenitor cells, originating some ambiguity regarding their definition and identification (Zhang G. et al., 2023). Recently, single-cell RNA sequencing (scRNA-seq) on patient derived samples suggest that GBM cells do not exist as separate populations but rather evolve from stemness to differentiation (Yan et al., 2023). These findings are hardly good news, as GSCs have been found to be deeply implicated in tumor progression, drug resistance and tumor recurrence (Guo et al., 2023). The attention of researchers therefore turns to this class of cells and their plastic nature in search for new regulatory protein to be pharmacologically targeted as Achilles’ heel of tumor cells survival. In this scenario, new homeostatic regulatory proteins were identified: for example, the ZIP14 (SLC39A14) protein, which mediates the cellular uptake of manganese, iron and zinc, has been indicated as a possible mediator of cellular ferroptosis-related cell death (Zhao et al., 2022; Zhang Y. et al., 2023). Similarly, the chloride intracellular channel-1 (CLIC1) is known to be instrumental for tumor proliferation in several solid tumor including GB and his overexpression on GSCs is inversely associated with patient survival (Setti et al., 2013), suggesting CLIC1 to be a potential target and prognostic biomarker (Randhawa and Jahani-Asl, 2023). Accordingly, a novel class of biguanide-based derivatives used as CLIC1-inhibitors has been recently developed and holds promises for the treatment of CLIC1-expressing glioblastomas (Barbieri et al., 2022).
Interestingly, the presence of stem cells in the bulk of glioblastomas has been also strongly associated to cancer multidrug resistance (MDR) (Mattei et al., 2021), which remains a serious challenge in GB therapy as it seriously limits the effects of different chemotherapeutic drugs (Tian et al., 2023). Indeed, as MDR has been often associated with the expression of p-glycoprotein (p-gp), an ATP-binding cassette (ABC) transporter that promotes efflux of chemotherapeutics from tumor cells, this protein has been recently suggested as a druggable therapeutic target (Hasan et al., 2023). Indeed, the p-gp inhibitor reversan, in association with magnetic nanoparticle-mediated hyperthermia, was able to increase the cytotoxic effect of Doxorubicine treatment to eliminate bulk tumors along with the GSC population (Hasan et al., 2023).
Mounting evidence has also been gathered to clarify the role of proteins of the tumor micro-environment (TME) mediating the regulation of cell adhesion and migration (Caverzán et al., 2023; Khan et al., 2023). In this view, TME becomes the scenario of the dynamic dialogue between tumor cells and tumor infiltrating immune system elements, mostly tumor-associated macrophages (TAMs), monocytes, T cells and resident microglia (Eisenbarth and Wang, 2023). TAMs indeed provide a major contribution to cancer growth and immunosuppression, causing resistance even to the most effective immunotherapies, ultimately paving the way to tumor recurrences (Zhang L. et al., 2023). Moreover, in aggressive brain tumors, TME host tumor associated hyper-vascularization. Indeed, the malfunctioning of the tight junctions in the endothelial cells of the tumor associated vascularization may favor the buildup of fluid in the tumor district leading to edema and increased intracranial pressure, partially restored with steroid anti-inflammatory therapy (Cenciarini et al., 2019).
Blood-Brain Barrier’s pericyte’s disruption and hypoxia sustain small vessel proliferation and, in the small vessels district, the dialogue between cancer cells proteins such Nestin and CD133 and endothelial biomarkers such as CD34 proteins facilitates brain metastasis, sustaining tumor cells circulation and subsequent tissue infiltration (Schiffer et al., 2018). More recently, the development of vascularization in GB was also described using genomic analytical tools, to create a risk prediction model for GB, where prognostic differentially expressed angiogenesis-related genes (PDEARGs) become potentially druggable prognostic biomarkers of regulatory networks and provide valuable insight to tackle the role of neovascularization in these tumors (Wan et al., 2023).
Recurrent tumors also exhibit changes in the microenvironment composition, characterized by higher levels of tumor-infiltrating lymphocytes (TILs), macrophages, and increased expression of Programmed Death-Ligand1 (PD-L1) and Programmed Cell Death Protein1 (PD-1) compared to primary tumors (Karschnia et al., 2023; White et al., 2023). This suggests a strong relationship between tumor heterogeneity, immune system involvement in recurrences (Hoogstrate et al., 2023; White et al., 2023) and supports the potential use of peritumoral microenvironmental markers for patient stratification (Riahi Samani et al., 2023). Furthermore, these findings may drive the development of novel precision immunotherapeutic tools (Pornnoppadol et al., 2023; White et al., 2023).
Importantly, a key challenge in GB treatment is effectively targeting and eliminating infiltrating cancer cells that persist in the brain parenchyma after primary tumor resection. Current treatment approaches involve a complex combination of surgical resection, radiation therapy, and concurrent chemotherapy using temozolomide (TMZ) (Fisher and Adamson, 2021). However, the anti-tumor efficacy of TMZ is significantly hindered by its limited ability to cross the BBB (reaching only 20% of blood concentration) (Grochans et al., 2022) and various cellular mechanisms conferring therapeutic resistance. Following the surgical removal of the primary tumor, a lower residual tumor volume is positively correlated with a more favorable prognosis. In this context, it is crucial to identify the microscopic tumor margins hidden within the brain parenchyma and prevent loco-regional recurrence (Bütof et al., 2022). Thus, the quest is to develop effective treatments capable of intercepting residual cells, possibly tumor stem cells, beyond the BBB while potentially countering the activity of favorable microenvironmental elements sustaining tumor invasiveness (Garcia-Diaz et al., 2023).
Here, we aimed at highlighting various strategies employed to overcome the challenges posed by the BBB. Nanomaterial-based delivery systems will be discussed, emphasizing their ability to enhance drug penetration into the brain and improve treatment efficacy and the use of nanomaterials loaded with single drugs or combination therapies. The focus will be centered on how these approaches can enhance the therapeutic effect by targeting multiple pathways or mechanisms involved in tumor growth and progression. Moreover, the review will delve into targeting druggable microenvironmental factors that contribute to the development of recurrent tumors. Finally, we will explore the potential of local drug delivery strategies for drug repurposing will be examined. Understanding and targeting these factors may help preventing the growth of residual cancer cells and reduce the risk of recurrence.
The BBB poses a significant challenge for the systemic delivery of chemotherapeutic agents to treat intracranial diseases. It is composed of endothelial cells, pericytes, astrocytes, microglia, and neurons, forming a structural barrier that restricts the passage of molecules into the brain (Sprowls et al., 2019). Endothelial cells lining the brain’s capillaries express tight junctions that seal the gaps between adjacent cells, preventing the free movement of molecules between the blood and the brain. Additionally, these cells lack fenestrations and pinocytic activity, further impeding the passage of substances. Pericytes, closely associated with endothelial cells, regulate capillary diameter and cerebral blood flow while supporting microvascular stability and the BBB’s structural integrity. Astrocytes play a crucial role in maintaining the barrier function through their foot processes, which establish a direct interface between vascular and neuroglial compartments.
Under normal physiological conditions, the BBB’s specific ion channels and transporters maintain an optimal microenvironment for brain function, regulating the levels of neurotransmitters, controlling ionic homeostasis, and protecting against neurotoxins. Water-soluble nutrients, metabolites and small lipid-soluble molecules can passively diffuse through the BBB and enter the brain (Kadry et al., 2020).
Blood-Brain Barrier’s tight junctions and membrane transporters stringently control the exchange of molecules and ions between the blood and the brain. Limited penetration is observed for small lipophilic molecules or those agents that can actively enter by binding to carrier proteins such as transferrin receptor (TfR), epidermal growth factor receptor (EGFR), glucose, or immunoglobulins (Triguero et al., 1989; Arora et al., 2020; Cui et al., 2023; Pornnoppadol et al., 2023).
Traditionally, the possibility of overcoming the BBB to concentrate systemically administered treatments (Thombre et al., 2023) and diagnostic tracers into the brain (Bae et al., 2023; Figure 1A) is achieved through temporary opening and partial disruption of tight junctions by physical interaction of focused high/low-frequency ultrasound (FUS) (Johansen et al., 2023; Mehta et al., 2023) as well as by using osmotic gradients (Cosolo et al., 1989; Figure 1B). Less conventional methods also include the use of isoflurane (Noorani et al., 2023) to increase the permeability of the brain to small hydrophilic molecules.
Figure 1. Overcoming BBB with systemically delivered therapies. (A) Superselective Intra-Arterial Cerebral Infusion (SIACI) and intravenous injection (I.V) are often administered in conjunction with by temporary opening of the BBB to facilitate delivery to the brain. (B) Focused ultrasound (FUS) and Osmotic forces are little invasive methods to temporary disrupt the tightly ordered pavement of the blood vessels constituting the BBB allowing blood-brain exchanges.
Extracranial magnetic resonance imaging (MRI) guided application of focused ultrasounds (FUS) in combination with circulating microbubbles (MB) has shown promise for the treatment of CNS diseases, including GB. High-intensity focused ultrasound (HIFU) can be used for cell and tissue-specific thermo-ablation, directly targeting GB cells (Johansen et al., 2023).
Low-intensity FUS has been FDA-approved for the treatment of untreatable tremors in Parkinson’s disease (NCT03608553). This technique can ameliorate the symptoms in patients. On the other hand, the use of low-intensity FUS combined with circulating MB can temporarily open the BBB (FUS-BBBO) enabling the delivery of anticancer molecules into the brain that may have been ineffective when administered systemically. For example, the intracranial concentration of Panobinostat, administered intra-peritoneally in combination with ultrasound/Magnetic Resonance Imaging (FUS/MRI), was significantly increased compared to sole intraperitoneal injection (Martinez et al., 2023). This led to a significant reduction in tumor volume and extended survival in a patient-derived xenograft (PDX) orthotopic model.
FUS has also been beneficial in the delivery of newly designed conjugates, such as hyaluronic acid (HA) with camptothecin (CPT) and doxorubicin (DOX) (Sun et al., 2022). The flexibility of the polymer in these conjugates facilitates BBB crossing. Similarly, FUS application increased the tumor penetration of a new sensitizer system constituted using a polymeric block, the pH-sensitive polyglutamic acid (PGA) and the chemotherapeutic agent and sonosensitizer DOX, camouflaged with human U87-MG cell membranes administered systemically. This nano-system effectively delivered doxorubicin into U87-MG cells, resulting in tumor growth reduction and overcoming of drug resistance (Chen et al., 2023).
In recent developments, MB-FUS systems are being used actively in drug delivery for cancer immunotherapy. FUS-induced bursting of MB generates an acoustic emission-dependent expression of the proinflammatory marker ICAM-1. Simultaneously, MB-FUS enhances local delivery of anti-PD1 agents and promotes infiltration of T lymphocytes in the tumor microenvironment (Lee et al., 2022).
Other authors combined charged MB with the negatively charged Gambogic Acid (GA) (an active component of a traditional Chinese medicine effective as antiproliferative and tumor infiltration agent) loaded polymeric nanoparticle (GA/PLGA) to form a GA/PLGA-charged MB complex (Dong et al., 2022). Similarly, FUS application benefits the distribution and long-term accumulation of 89Zr labeled/cetuximab, an anti EGFR antibody in a preclinical mouse model (Sacks et al., 2018).
In an innovative strategy, intracranial drug depots for small molecules were implanted in mice using bio-orthogonal click chemistry technology, which exploits extracellular matrix molecules to anchor tissue-reactive anchoring of click groups (TRAC). FUS and MB played a crucial role in enabling the non-invasive loading of these drug depots, allowing prolonged and spatially controlled treatment (Moody et al., 2023).
A phase 2 clinical trial (NCT03744026) has been designed for the use of a low intensity pulsed ultrasound (LIPU) device implanted into the skull of patients at the time of primary tumor surgical removal. A different trial (NCT04528680) combined LIPU with injected MB to efficiently increase the delivery of systemically infused albumin-linked paclitaxel in patients with recurrent glioblastoma. LIPU-MB is also being tested in combination with other therapies, such as albumin-bound paclitaxel and carboplatin (Sonabend et al., 2023).
Overall, the application of FUS in combination with MB holds great potential for the treatment of CNS diseases, including glioblastoma. It allows for targeted treatment, enhanced drug delivery, and potential synergies with immunotherapy approaches.
Maximizing the safe resection of tumor mass upon diagnosis is crucial for treatment, as the extent of residual volume directly correlates with patient survival. However, even after resection, there are often residual cells hidden within the uneven margins of the resected area, which can lead to tumor recurrence over time. In situ post peri-surgery applications aim to concentrate treatments to maximize the chances of killing these remaining cells. However, the presence of surrounding neurons necessitates minimizing functional deficits (Figure 2).
Figure 2. In situ and intranasal delivery. (A) Convection enhanced delivery (CED) and (B) injection of therapy solutions/gel directly into the parenchyma normally after primary tumor resection. (C) Deposition of matrices (solid polymers and gels) and mini-devices (micro-needles on patches) in direct contact with the after-resection cavity borders. (D) The intranasal route is a portal to the Central Nervous System as therapies inhaled or instilled on the nasal mucosa can bypass the BBB travelling through the olfactory neurons and trigeminal nerves, effectively reaching the Olfactory Bulb and from here different areas of the brain.
Currently, in situ delivery is obtained by convection-enhanced delivery (CED) (Sperring et al., 2023; Thompson et al., 2023) (Clinical Trial: NCT03043391) achieved by gently pushing drugs into the tumor (Figure 2A). Similarly, the intra-arterial delivery of chemicals, with or without the assistance of osmotic gradient (Chu et al., 2022; Wang et al., 2023) can result in effective intracranial treatment. However, the deposition of biocompatible drug-enriched injectable matrices (Figure 2B) that locally release active treatments seems to be the most promising strategy for intracranial controlled release. When resection is possible, patients are surgically implanted with a polymeric matrix for the semi-controlled release of carmustine, the FDA-approved Gliadel®. However, this implant has a degree of rigidity that limits its use. With the development of innovative biomaterials such as smart materials, polymeric matrices and hydrogels it will be possible to design more adaptable materials able to adhere to the surgical resection margins of the cavity (Gu et al., 2022; Figure 2C).
Many of these formulations can incorporate nanomaterials that are sensitive to environmental stimuli, including temperature (such as thermogels) (Gu et al., 2022), chemical stimuli such as acidity/CO2 (Younis et al., 2023), reactive oxygen species (ROS) (Habra et al., 2022), magnetic force, or X-ray irradiation (Yun et al., 2022). Additionally, the customization of different nanoparticles (Idlas et al., 2023) and the loading of various molecules (Lin et al., 2023) allow for unlimited potential therapeutic combinations. In some cases, nanoparticles loaded with chemotherapeutics are dispersed into gel matrices (Gu et al., 2022), which function as a shelter to reduce molecule degradation and unwanted cytotoxicity (Erthal et al., 2023). New formulations for in situ delivery aim at optimizing the effects of first-generation anti-glioblastoma agents such as carmustine and temozolomide, either alone or in combination, to maximize their curative effects, for example overcoming the resistance to TMZ using the O6 alkylguanine DNA alkyltransferase inhibitor, dialdehyde O6 benzylguanine (DABG), tested in xenograft (Chu et al., 2023) or intracranially delivered, minimizing peripheral toxicity (Chen et al., 2022; Iturrioz-Rodríguez et al., 2023).
In situ delivery also presents an opportunity for targeting the tumor microenvironment and stimulating the tumor-suppressive immune system. Recently, hyaluronic acid (HA) has been utilized as an injectable delivery platform for combinatory treatments. HA-Doxorubicin and HA-CpG, an agonist of Toll-like receptor (Catania et al., 2023), were used to stimulate tumor-associated macrophages (TAM). Similarly, DOX-loaded mesoporous polydopamine (MPDA) nanoparticles were encapsulated in macrophage-derived nanovesicles (M1NVs) and used as immunostimulant effectors, while fibrin hydrogels serving as in situ delivery vehicles (Zhang R. et al., 2023).
GB recurrence has been recently modeled, in preclinical settings, by partially resecting the primary tumor. Bianco and coworkers have successfully demonstrated this approach (Bianco et al., 2017; Kubelt et al., 2023), and more recently, a minimally modified technique has been developed (Sun et al., 2023). Live imaging is frequently used to monitor the inevitable reappearance of the intracranial tumor due to residual cells and the presence of glioma stem cells (GSCs). Following resection, the cavity created in the brain tissue provides an opportunity for delivering curative treatments. One promising approach involves injecting a biodegradable thermosensitive triblock copolymer, poly (D,L-lactic acid-co-glycolic acid)-b-poly(ethylene glycol)-b-poly(D, L-lactic acid-co-glycolic acid) (PLGA-PEG-PLGA), that contain the anticancer therapy for sustained local release. These formulations mostly include TMZ (Zhang R. et al., 2023); however combinatorial treatment can be achieve also using less conventional anti-GB agents such as curcumin (Liang et al., 2023). Intra-tumor injection can also be performed with allosteric tissue elements (Figure 2B). In a phase I trial, a suicide gene therapy was attempted by injecting adipose tissue-derived mesenchymal stem cells (ADSCs) carrying the herpes simplex virus-thymidine kinase (HSV-TK) gene into patients with non-surgically removable recurrent GB (Oraee-Yazdani et al., 2023). A notable innovative therapeutic tool carrier has recently been developed (Sun et al., 2022). This carrier consists of allomelanin nanoparticles (AMNPs) loaded with a checkpoint inhibitor, CLP002, and camouflaged with cancer cell membrane coatings (CCM). The delivery system, named AMNP@CLP@CCM, can penetrate the BBB, interfering with immune activity, and sustaining photo-thermal ablation with AMNPs. Ultimately, these combined effects will synergistically kill tumor cells.
The murine model of intracranial resection of primary tumor mass is frequently used for the preclinical investigation of treatment against recurrences, filling the resection cavity with the local therapy. “Smart” hydrogels, such as chitosan-based thermogels enriched with SiO2-TMZ loaded nanoparticles, have proven effective in reducing recurrences in a U87-MG recurrence model. The use of silica particles as carriers represents an unconventional but equally effective choice compared to the more commonly used PCL-TMZ loaded nanoparticles (Gherardini et al., 2023). Similarly, photosensitive polymeric matrices have been previously employed to treat recurrences with paclitaxel (PTX) (Zhao et al., 2018). A novel approach to target residual cells in GB involved the use of polymeric microneedle patches designed to anchor within the irregular margin. These patches were loaded with polymer-coated nanoparticles containing either cannabidiol (CBD) or olaparib (OLA). The uniqueness of the study lies in both the choice of the carriers and the therapeutic agents (Muresan et al., 2023). OLA, a PARP inhibitor, has been utilized in combination with TMZ in both preclinical studies (Zampieri et al., 2021) and clinical trials (Lesueur et al., 2019). However, there are only a few encouraging reports on the use of CBD, the non-psychotropic component of cannabis, showing tumor growth reduction (Lah et al., 2022) and increased survival rates in glioblastoma patients (Likar et al., 2021).
The intranasal route offers a direct pathway for active molecules to reach the CNS, making it an attractive option for drug delivery to the brain. This route serves two purposes: delivering drugs directly to the brain (nose-to-brain) and increasing peripheral vascular concentrations (nose-to-blood), similarly to pulmonary delivery methods. The olfactory nerve pathways serve as the gateway to the Olfactory Bulb, where drugs deposited on the nasal mucosa can travel through the olfactory neurons and trigeminal nerves, effectively reaching different areas of the brain (Figure 2D).
Through this way, active formulations can avoid first pass (enzymatic degradation and clearance) metabolism, increasing their bioavailability in the CNS, minimizing the occurrence of peripheral side effects. However, it is important to note that local damage of the nasal mucosa and brain tissue can limit the amount of active drugs that can be delivered.
In general, highly lipophilic drugs or nanoparticles with a low molecular weight are well-suited for intranasal delivery strategies. These characteristics enable better penetration and absorption through the nasal mucosa, facilitating efficient transport to the brain.
Recently, the utilization of intranasal routes for treating GB has been reviewed, focusing on the advantages and disadvantages for patients (Goel et al., 2022). Furthermore, in the past decade, the availability of tunable nanoparticles has stimulated multidisciplinary research to design and test various promising formulations (Montegiove et al., 2022). A recently completed clinical trial (NCT04091503) examined the safety and effectiveness of intranasal administration of TMZ in patients with GB and, recently, nanocarriers have been specifically designed for intranasal delivery. For instance, a monoclonal antibody anti-EphA3 was utilized to target TMZ-loaded gold nanoparticles (anti-EphA3-TMZ@GNPsTMZ) for photothermal therapy in nose-to-brain delivery (Yu et al., 2022). Chitosan-coated PLGA nanoparticles were optimized for nose-to-brain delivery of carmustine into the healthy Albino rat brain, resulting in significantly increased chemotherapeutic concentration compared to plasma levels. However, no indication of efficacy against tumor growth in vivo was reported (Ahmad et al., 2022).
Decorating nanoparticles with transferrin to achieve targeting of TfR appears to be an efficient approach for intranasal delivery, as already demonstrated (Sandbhor et al., 2023). Lipid nanoparticles (LNPs) were used for the co-delivery of paclitaxel (PTX) and miltefosine (HePc), a proapoptotic agent, resulting in significant tumor reduction and increased survival in mice after intranasal treatment compared to systemic Taxol® and nasal free drug administration. The role of meningeal pathways and of the lymphatic system in intranasal delivery has recently been studied revealing reduced liposomal transport in GB (Semyachkina-Glushkovskaya et al., 2022). The application of biocompatible infrared photo stimulation of meningeal lymphatic vessels in the cribriform plate could potentially enhance flux and delivery. Finally, we highlight few rare examples of nanoparticle tracing after intranasal delivery (Han et al., 2023) mapping their localization in the tumor site, brain parenchyma and more significantly intercepting accumulation in internal organs and overall body districts (NIR and SPECT/CT imaging), which is crucial for targeting and assessing off-target toxicity. Gold nanorods (AuNRs) tested as a platform for delivery in CNS were functionalized by adding the fluorescent dye Cyanine5 (Cy5) for optical imaging or a metal chelator, diethylenetriaminepentaacetic dianhydride (DTPA) hinged by PEG to 111Indium for nuclear detection, allowing detection of the brain area distribution and the peripheral organ distribution of the NP after entering the CNS (Han et al., 2023).
In conclusion, the nose-to-brain route holds great potential for repurposing conventional drugs for novel therapeutic applications.
Drug repurposing is a cost-effective strategy for cancer treatment, serving as an alternative to de novo drug discovery. In the case of GB, candidate molecules for repurposing are often identified through biomedical and biogenetic profiling of patients and in silico docking simulations (McGowan et al., 2023; Roy et al., 2023).
Among these candidates, FDA-approved drugs that are currently used for treating other conditions have shown promise (Jones et al., 2023; Moretti et al., 2023; Murali and Karuppasamy, 2023; Vítovcová et al., 2023). Recent preclinical examples include Flubendazole, an inhibitor of microtubule growth that activates autophagy and STAT3-dependent apoptosis, and auranofin (AF), an inhibitor of TrxR1, used alone or in combination with the prooxidant menadione (Szeliga and Rola, 2022). Hydroquinidine (HQ) is another repurposing candidate (Yavuz and Demircan, 2023) to induce GBM cell death overcoming TMZ resistance that could be a good candidate for in vivo study.
Repurposing or repositioning anticancer agents that have demonstrated efficacy in vitro but failed to produce therapeutic effects in vivo, or those causing unacceptable off-target effects when administered systemically, could find a second life through local delivery. For example, intranasal administration of Temozolomide (TMZ) (Clinical Trial: NCT04091503) or systemic administration of Placlitaxel, in combination with albumin to induce osmotic opening of BBB (NCT04528680) is under investigation, as well as the intracranial deposition of Paclitaxel in OncoGel® NCT00479765 (Morales and Mousa, 2022) to elicit a stronger antitumor effect. A single dose of intra-arterial mannitol should facilitate the temporary opening of the BBB to allow superselective intra-arterial cerebral infusion (SIACI) (Figure 1A) of a high single dose of TMZ (up to 250mg/m2) to achieve a more efficient tumor site targeting (NCT01180816). Similarly, SIACI protocol with mannose osmotic pretreatment is attempted with Cetuximab (CTX), targeting the Epidermal Growth Factor Receptor (EGFR) is under study in a Phase I clinical trial to treat recurrent GB (Clinical Trial: NCT02861898).
Recent advances in loco-regional treatment in GB focused on overcoming the BBB and targeting microenvironmental proteins to enhance therapeutic efficacy. “The Holy Grail,” “the Magic Bullet,” “the Trojan Horse”: these are all metaphors that can be used to define the goal to find a mythic nano-devise or a molecular tool that could be able to overcome the BBB and deliver its anticancer therapy for an effective personalized treatments to benefit GB patients. On the other hand, strategies such as focused ultrasounds with microbubbles, intracranial drug depots, and in situ delivery using nanomaterials and biomaterials show promise in improving drug delivery combating GB recurrence and improving patient’s overall survival and quality of life.
MC: Conceptualization, Data curation, Funding acquisition, Project administration, Writing−original draft. GI: Conceptualization, Data curation, Methodology, Software, Writing−original draft. VB: Conceptualization, Data curation, Writing−original draft. LG: Conceptualization, Data curation, Methodology, Writing−original draft, Writing−review and editing.
The author(s) declare financial support was received for the research, authorship, and/or publication of the article. This work was supported by the specific funding from Regione Toscana/Istituto per lo Studio, la Prevenzione e la Rete Oncologica (ISPRO) to MC.
The authors declare that the research was conducted in the absence of any commercial or financial relationships that could be construed as a potential conflict of interest.
All claims expressed in this article are solely those of the authors and do not necessarily represent those of their affiliated organizations, or those of the publisher, the editors and the reviewers. Any product that may be evaluated in this article, or claim that may be made by its manufacturer, is not guaranteed or endorsed by the publisher.
PLGA-PEG-PLGA, (D,L-lactic acid-co-glycolic acid)-b-poly(ethylene glycol)-b-poly(D, L-lactic acid-co-glycolic acid); ADSC, Adipose tissue-Derived mesenchymal Stem Cells; AMNPs, Allomelanin Nanoparticles; ABC, ATP-binding cassette; AF, Auranofin; BBB, Blood-Brain Barrier; CPT, Camptothecin; CCM, Cancer Cell Membrane; CBD, Cannabidiol; CNS, Centra Nervous System; CTX, Cetuximab; CLIC1, Chloride intracellular channel-1; CED, Convection Enhanced Delivery; Cy5, Cyanine5; DABG, Dialdehyde O6 Benzylguanine; DTPA, Diethylenetriaminepentaacetic Dianhydride; DOX, Doxorubicin; EGFR, Epidermal Growth Factor Receptor; FDA, Food and Drugs Administration; FUS, Frequency Ultrasound; FUS-BBBO, FUS combined with circulating microbubbles can temporarily open the BBB; GA, Gambogic Acid; GB, Glioblastoma; GSCs, Glioma Stem Cells; AuNRs, Gold nanorods; HSV-TK, Herpes Simplex Virus-Thymidine Kinase; HIFU, High Intensity Focused Ultrasound; HA, Hyaluronic Acid; HQ, Hydroquinidine; ICAM-1, Intracellular Adesion Molecule 1; LNPs, Lipid Nanoparticles; LIPU, Low-Intensity Pulsed Ultrasound; M1NVs, Macrophage-Derived Nanovesicles; MRI, Magnetic Resonance Imaging; MPDA, Mesoporous Polydopamine; MB, Microbubbles; HePc, Miltefosine; anti-EphA3-TMZ@GNPsTMZ), Monoclonal antibody anti-EphA3 – TMZ-loaded gold nanoparticles; MDR, Multidrug resistance; OLA, Olaparib; p-gp , p-glycoprotein ; PTX, Paclitaxel; PDX, Patient-Derived Xenograft; PARP, Poly (ADP-ribose) polymerase; PLGA, poly lactic-co-glycolic acid; PCL, Polycaprolactone; PGA, Polyglutamic Acid; PDEARGs, Prognostic differentially expressed angiogenesis-related genes; PD-1, Programmed Cell Death Protein 1; PD-L1, Programmed Death-Ligand 1; ROS, Reactive Oxygen Species; SiO2, Silicate; SIACI, Superselective Intra-Arterial Cerebral Infusion; TMZ, Temozolomide; TrxR1, Thioredoxin Reductase 1; TRAC, Tissue-Reactive Anchoring of Click groups; TfR, Transferrin Receptor; TAM, Tumor-Associated Macrophages; TILs, Tumor-Infiltrating Lymphocytes; ZIP14, Zrt- and Irt-like (ZIP) proteins transporters.
Ahmad, S., Khan, I., Pandit, J., Emad, N. A., Bano, S., Dar, K. I., et al. (2022). Brain targeted delivery of carmustine using chitosan coated nanoparticles via nasal route for glioblastoma treatment. Int. J. Biol. Macromol. 221, 435–445. doi: 10.1016/j.ijbiomac.2022.08.210
Arora, S., Sharma, D., and Singh, J. (2020). GLUT-1: An effective target to deliver brain-derived neurotrophic factor gene across the blood brain barrier. ACS Chem. Neurosci. 11, 1620–1633. doi: 10.1021/acschemneuro.0c00076
Asleh, K., Dery, V., Taylor, C., Davey, M., Djeungoue-Petga, M. A., and Ouellette, R. J. (2023). Extracellular vesicle-based liquid biopsy biomarkers and their application in precision immuno-oncology. Biomark. Res. 11:99. doi: 10.1186/s40364-023-00540-2
Bae, S., Liu, K., Pouliopoulos, A. N., Ji, R., and Konofagou, E. E. (2023). Real-time passive acoustic mapping with enhanced spatial resolution in neuronavigation-guided focused ultrasound for blood-brain barrier opening. IEEE Trans. Biomed. Eng. 70, 2874–2885. doi: 10.1109/TBME.2023.3266952
Barbieri, F., Bosio, A. G., Pattarozzi, A., Tonelli, M., Bajetto, A., Verduci, I., et al. (2022). Chloride intracellular channel 1 activity is not required for glioblastoma development but its inhibition dictates glioma stem cell responsivity to novel biguanide derivatives. J. Exp. Clin Cancer Res. 41:53. doi: 10.1186/s13046-021-02213-0
Bianco, J., Bastiancich, C., Joudiou, N., Gallez, B., des Rieux, A., and Danhier, F. (2017). Novel model of orthotopic U-87 MG glioblastoma resection in athymic nude mice. J. Neurosci. Methods 284, 96–102. doi: 10.1016/j.jneumeth.2017.04.019
Brás, J. P., Jesus, T. T., Prazeres, H., Lima, J., Soares, P., and Vinagre, J. (2023). TERTmonitor-qPCR detection of TERTp mutations in glioma. Genes 14:1693.
Bütof, R., Hönscheid, P., Aktar, R., Sperling, C., Tillner, F., Rassamegevanon, T., et al. (2022). Orthotopic glioblastoma models for evaluation of the clinical target volume concept. Cancers 14:4559. doi: 10.3390/cancers14194559
Catania, G., Rodella, G., Vanvarenberg, K., Préat, V., and Malfanti, A. (2023). Combination of hyaluronic acid conjugates with immunogenic cell death inducer and CpG for glioblastoma local chemo-immunotherapy elicits an immune response and induces long-term survival. Biomaterials 294:122006. doi: 10.1016/j.biomaterials.2023.122006
Caverzán, M. D., Beaugé, L., Oliveda, P. M., Cesca González, B., Bühler, E. M., and Ibarra, L. E. (2023). Exploring monocytes-macrophages in immune microenvironment of glioblastoma for the design of novel therapeutic strategies. Brain Sci. 13:542. doi: 10.3390/brainsci13040542
Cenciarini, M., Valentino, M., Belia, S., Sforna, L., Rosa, P., Ronchetti, S., et al. (2019). Dexamethasone in glioblastoma multiforme therapy: Mechanisms and controversies. Front. Mol. Neurosci. 12:65. doi: 10.3389/fnmol.2019.00065
Chen, H., Zhang, S., Fang, Q., He, H., Ren, J., Sun, D., et al. (2023). Biomimetic nanosonosensitizers combined with noninvasive ultrasound actuation to reverse drug resistance and sonodynamic-enhanced chemotherapy against orthotopic glioblastoma. ACS Nano 17, 421–436. doi: 10.1021/acsnano.2c08861
Chen, S., Qiu, Q., Wang, D., She, D., Yin, B., Gu, G., et al. (2022). Dual-sensitive drug-loaded hydrogel system for local inhibition of post-surgical glioma recurrence. J. Control Release 349, 565–579. doi: 10.1016/j.jconrel.2022.07.011
Chu, C., Jablonska, A., Gao, Y., Lan, X., Lesniak, W. G., Liang, Y., et al. (2022). Hyperosmolar blood-brain barrier opening using intra-arterial injection of hyperosmotic mannitol in mice under real-time MRI guidance. Nat. Protoc. 17, 76–94. doi: 10.1038/s41596-021-00634-x
Chu, W., Houston, Z. H., Fletcher, N. L., Huda, P., Ahamed, M., Lim, T. X., et al. (2023). Development and validation of a targeted treatment for brain tumors using a multi-drug loaded, relapse-resistant polymeric theranostic. Biomacromolecules 24, 2674–2690. doi: 10.1021/acs.biomac.3c00138
Cosolo, W. C., Martinello, P., Louis, W. J., and Christophidis, N. (1989). Blood-brain barrier disruption using mannitol: Time course and electron microscopy studies. Am. J. Physiol. 256(2 Pt. 2), R443–R447. doi: 10.1152/ajpregu.1989.256.2.R443
Cui, J., Wang, X., Li, J., Zhu, A., Du, Y., Zeng, W., et al. (2023). Immune exosomes loading self-assembled nanomicelles traverse the blood-brain barrier for chemo-immunotherapy against glioblastoma. ACS Nano doi: 10.1021/acsnano.2c10219 [Epub ahead of print].
Dong, L., Li, N., Wei, X., Wang, Y., Chang, L., Wu, H., et al. (2022). A gambogic acid-loaded delivery system mediated by ultrasound-targeted microbubble destruction: A promising therapy method for malignant cerebral glioma. Int. J. Nanomed. 17, 2001–2017. doi: 10.2147/IJN.S344940
Eisenbarth, D., and Wang, Y. A. (2023). Glioblastoma heterogeneity at single cell resolution. Oncogene 42, 2155–2165. doi: 10.1038/s41388-023-02738-y
Erthal, L., Shi, Y. C., Sweeney, K. C., Gobbo, O. L., and Ruiz-Hernandez, E. (2023). Nanocomposite formulation for a sustained release of free drug and drug-loaded responsive nanoparticles: An approach for a local therapy of glioblastoma multiforme. Sci. Rep. 13:5094. doi: 10.1038/s41598-023-32257-5
Fisher, J. P., and Adamson, D. C. (2021). Current FDA-approved therapies for high-grade malignant gliomas. Biomedicines 9:324. doi: 10.3390/biomedicines9030324
Garcia-Diaz, C., Pöysti, A., Mereu, E., Clements, M. P., Brooks, L. J., Galvez-Cancino, F., et al. (2023). Glioblastoma cell fate is differentially regulated by the microenvironments of the tumor bulk and infiltrative margin. Cell Rep. 42:112472. doi: 10.1016/j.celrep.2023.112472
Gherardini, L., Vetri Buratti, V., Maturi, M., Inzalaco, G., Locatelli, E., Sambri, L., et al. (2023). Loco-regional treatment with temozolomide-loaded thermogels prevents glioblastoma recurrences in orthotopic human xenograft models. Sci. Rep. 13:4630. doi: 10.1038/s41598-023-31811-5
Goel, H., Kalra, V., Verma, S. K., Dubey, S. K., and Tiwary, A. K. (2022). Convolutions in the rendition of nose to brain therapeutics from bench to bedside: Feats & fallacies. J. Control Release 341, 782–811. doi: 10.1016/j.jconrel.2021.12.009
Grochans, S., Cybulska, A. M., Simińska, D., Korbecki, J., Kojder, K., Chlubek, D., et al. (2022). Epidemiology of glioblastoma multiforme-literature review. Cancers 14:2412. doi: 10.3390/cancers14102412
Gu, W., Fan, R., Quan, J., Cheng, Y., Wang, S., Zhang, H., et al. (2022). Intracranial in situ thermosensitive hydrogel delivery of temozolomide accomplished by PLGA-PEG-PLGA triblock copolymer blending for GBM treatment. Polymers 14:3368. doi: 10.3390/polym14163368
Guo, X., Qiu, W., Wang, C., Qi, Y., Li, B., Wang, S., et al. (2023). Neuronal activity promotes glioma progression by inducing proneural-to-mesenchymal transition in glioma stem cells. Cancer Res. doi: 10.1158/0008-5472.CAN-23-0609 [Epub ahead of print].
Habra, K., Morris, R. H., McArdle, S. E. B., and Cave, G. W. V. (2022). Controlled release of carnosine from poly(lactic- co-glycolic acid) beads using nanomechanical magnetic trigger towards the treatment of glioblastoma. Nanoscale Adv. 4, 2242–2249. doi: 10.1039/d2na00032f
Han, S., Wang, J. T., Yavuz, E., Zam, A., Rouatbi, N., Utami, R. N., et al. (2023). Spatiotemporal tracking of gold nanorods after intranasal administration for brain targeting. J. Control Release 357, 606–619. doi: 10.1016/j.jconrel.2023.04.022
Hasan, U., Rajakumara, E., and Giri, J. (2023). Reversal of multidrug resistance by the synergistic effect of reversan and hyperthermia to potentiate the chemotherapeutic response of doxorubicin in glioblastoma and glioblastoma stem cells. ACS Appl. Biomater. doi: 10.1021/acsabm.3c00644 [Epub ahead of print].
Hoogstrate, Y., Draaisma, K., Ghisai, S. A., van Hijfte, L., Barin, N., de Heer, I., et al. (2023). Transcriptome analysis reveals tumor microenvironment changes in glioblastoma. Cancer Cell 41, 678–692.e7. doi: 10.1016/j.ccell.2023.02.019
Idlas, P., Lepeltier, E., Bastiat, G., Pigeon, P., McGlinchey, M. J., Lautram, N., et al. (2023). Physicochemical characterization of ferrocifen lipid nanocapsules: Customized drug delivery systems guided by the molecular structure. Langmuir. 39, 1885–1896. doi: 10.1021/acs.langmuir.2c02910
Iturrioz-Rodríguez, N., Sampron, N., and Matheu, A. (2023). Current advances in temozolomide encapsulation for the enhancement of glioblastoma treatment. Theranostics 13, 2734–2756. doi: 10.7150/thno.82005
Johansen, P. M., Hansen, P. Y., Mohamed, A. A., Girshfeld, S. J., Feldmann, M., and Lucke-Wold, B. (2023). Focused ultrasound for treatment of peripheral brain tumors. Explor. Drug Sci. 1, 107–125. doi: 10.37349/eds.2023.00009
Jones, D., Whitehead, C. A., Dinevska, M., Widodo, S. S., Furst, L. M., Morokoff, A. P., et al. (2023). Repurposing FDA-approved drugs as inhibitors of therapy-induced invadopodia activity in glioblastoma cells. Mol. Cell Biochem. 478, 1251–1267. doi: 10.1007/s11010-022-04584-0
Kadry, H., Noorani, B., and Cucullo, L. (2020). A blood-brain barrier overview on structure, function, impairment, and biomarkers of integrity. Fluids Barriers CNS 17:69. doi: 10.1186/s12987-020-00230-3
Karschnia, P., Young, J. S., Dono, A., Häni, L., Sciortino, T., Bruno, F., et al. (2023). Prognostic validation of a new classification system for extent of resection in glioblastoma: A report of the RANO resect group. Neuro Oncol. 25, 940–954. doi: 10.1093/neuonc/noac193
Khan, F., Pang, L., Dunterman, M., Lesniak, M. S., Heimberger, A. B., and Chen, P. (2023). Macrophages and microglia in glioblastoma: Heterogeneity, plasticity, and therapy. J Clin Invest. 133:e163446. doi: 10.1172/JCI163446
Kubelt, C., Hellmold, D., Peschke, E., Hauck, M., Will, O., Schütt, F., et al. (2023). Establishment of a rodent glioblastoma partial resection model for chemotherapy by local drug carriers-sharing experience. Biomedicines 11:1518. doi: 10.3390/biomedicines11061518
Lah, T. T., Majc, B., Novak, M., Sušnik, A., Breznik, B., Porčnik, A., et al. (2022). The cytotoxic effects of cannabidiol and cannabigerol on glioblastoma stem cells may mostly involve GPR55 and TRPV1 signalling. Cancers 14:5918. doi: 10.3390/cancers14235918
Lathia, J. D., Mack, S. C., Mulkearns-Hubert, E. E., Valentim, C. L., and Rich, J. N. (2015). Cancer stem cells in glioblastoma. Genes Dev. 29, 1203–1217. doi: 10.1101/gad.261982.115
Lee, H., Guo, Y., Ross, J. L., Schoen, S., Degertekin, F. L., and Arvanitis, C. (2022). Spatially targeted brain cancer immunotherapy with closed-loop controlled focused ultrasound and immune checkpoint blockade. Sci. Adv. 8:eadd2288. doi: 10.1126/sciadv.add2288
Lesueur, P., Lequesne, J., Grellard, J. M., Dugué, A., Coquan, E., Brachet, P. E., et al. (2019). Phase I/IIa study of concomitant radiotherapy with olaparib and temozolomide in unresectable or partially resectable glioblastoma: OLA-TMZ-RTE-01 trial protocol. BMC Cancer 19:198. doi: 10.1186/s12885-019-5413-y
Liang, Q., Zhuo, Y., Wu, X., Zheng, S., Zhuang, J., Wang, K., et al. (2023). Curcumin combining temozolomide formed localized nanogel for inhibition of postsurgical chemoresistant glioblastoma. Nanomedicine 18, 907–921. doi: 10.2217/nnm-2023-0058
Likar, R., Koestenberger, M., Stutschnig, M., and Nahler, G. (2021). Cannabidiol μay prolong survival in patients with glioblastoma multiforme. Cancer Diagn. Progn. 1, 77–82. doi: 10.21873/cdp.10011
Lin, F. H., Hsu, Y., Chang, K., and Shyong, Y. (2023). Porous hydroxyapatite carrier enables localized and sustained delivery of honokiol for glioma treatment. Eur. J. Pharm. Biopharm. 189, 224–232. doi: 10.1016/j.ejpb.2023.06.016
Martinez, P., Nault, G., Steiner, J., Wempe, M. F., Pierce, A., Brunt, B., et al. (2023). MRI-guided focused ultrasound blood-brain barrier opening increases drug delivery and efficacy in a diffuse midline glioma mouse model. Neurooncol. Adv. 5:vdad111. doi: 10.1093/noajnl/vdad111
Mattei, V., Santilli, F., Martellucci, S., Delle Monache, S., Fabrizi, J., Colapietro, A., et al. (2021). The importance of tumor stem cells in glioblastoma resistance to therapy. Int. J. Mol. Sci. 22:3863. doi: 10.3390/ijms22083863
McGowan, E., Sanjak, J., Mathé, E. A., and Zhu, Q. (2023). Integrative rare disease biomedical profile based network supporting drug repurposing or repositioning, a case study of glioblastoma. Orphanet J. Rare Dis. 18:301. doi: 10.1186/s13023-023-02876-2
Mehta, N. H., Shah, H. A., Ben-Shalom, N., and D’Amico, R. S. (2023). Sonolucent cranioplasty: Is therapeutic FUS the next frontier? J. Clin. Neurosci. 114, 129–130. doi: 10.1016/j.jocn.2023.06.016
Montegiove, N., Calzoni, E., Emiliani, C., and Cesaretti, A. (2022). Biopolymer nanoparticles for nose-to-brain drug delivery: A new promising approach for the treatment of neurological diseases. J. Funct. Biomater. 13:125. doi: 10.3390/jfb13030125
Moody, C. T., Durham, P. G., Dayton, P. A., and Brudno, Y. (2023). Loading intracranial drug-eluting reservoirs across the blood-brain barrier with focused ultrasound. Ultrasound Med. Biol. 49, 1679–1685. doi: 10.1016/j.ultrasmedbio.2023.03.012
Morales, D. E., and Mousa, S. (2022). Intranasal delivery in glioblastoma treatment: Prospective molecular treatment modalities. Heliyon 8:e09517. doi: 10.1016/j.heliyon.2022.e09517
Moretti, I. F., Lerario, A. M., Sola, P. R., Macedo-da-Silva, J., Baptista, M. D. S., Palmisano, G., et al. (2023). GBM cells exhibit susceptibility to metformin treatment according to TLR4 pathway activation and metabolic and antioxidant status. Cancers 15:587. doi: 10.3390/cancers15030587
Murali, P., and Karuppasamy, R. (2023). Imidazole and biphenyl derivatives as anti-cancer agents for glioma therapeutics: Computational drug repurposing strategy. Anticancer Agents Med. Chem. 23, 1085–1101. doi: 10.2174/1871520623666230125090815
Muresan, P., McCrorie, P., Smith, F., Vasey, C., Taresco, V., Scurr, D. J., et al. (2023). Development of nanoparticle loaded microneedles for drug delivery to a brain tumour resection site. Eur. J. Pharm. Biopharm. 182, 53–61. doi: 10.1016/j.ejpb.2022.11.016
Noorani, B., Chowdhury, E. A., Alqahtani, F., Ahn, Y., Nozohouri, E., Zoubi, S., et al. (2023). Effects of Volatile Anesthetics versus Ketamine on Blood-Brain Barrier Permeability via Lipid-Mediated Alterations of Endothelial Cell Membranes. J Pharmacol Exp Ther. 385, 135–145. doi: 10.1124/jpet.122.001281
Oraee-Yazdani, S., Tavanaei, R., Rostami, F., Hajarizadeh, A., Mehrabadi, M., Akhlaghpasand, M., et al. (2023). Suicide gene therapy using allogeneic adipose tissue-derived mesenchymal stem cell gene delivery vehicles in recurrent glioblastoma multiforme: A first-in-human, dose-escalation, phase I clinical trial. J. Transl. Med. 21:350. doi: 10.1186/s12967-023-04213-4
Pornnoppadol, G., Bond, L. G., Lucas, M. J., Zupancic, J. M., Kuo, Y. H., Zhang, B., et al. (2023). Bispecific antibody shuttles targeting CD98hc mediate efficient and long-lived brain delivery of IgGs. Cell Chem Biol. doi: 10.1016/j.chembiol.2023.09.008 [Epub ahead of print].
Randhawa, K., and Jahani-Asl, A. (2023). CLIC1 regulation of cancer stem cells in glioblastoma. Curr. Top. Membr. 92, 99–123. doi: 10.1016/bs.ctm.2023.09.004
Riahi Samani, Z., Parker, D., Akbari, H., Wolf, R. L., Brem, S., Bakas, S., et al. (2023). Artificial intelligence-based locoregional markers of brain peritumoral microenvironment. Sci. Rep. 13:963. doi: 10.1038/s41598-022-26448-9
Roy, P. K., Majumder, R., and Mandal, M. (2023). In-silico identification of novel DDI2 inhibitor in glioblastoma via repurposing FDA approved drugs using molecular docking and MD simulation study. J. Biomol. Struct. Dyn. doi: 10.1080/07391102.2023.2204371 [Epub ahead of print].
Sacks, D., Baxter, B., Campbell, B. C. V., Carpenter, J. S., Cognard, C., Dippel, D., et al. (2018). Multisociety consensus quality improvement revised consensus statement for endovascular therapy of acute ischemic stroke: From the American Association of Neurological Surgeons (AANS), American Society of Neuroradiology (ASNR), Cardiovascular and Interventional Radiology Society of Europe (CIRSE), Canadian Interventional Radiology Association (CIRA), Congress of Neurological Surgeons (CNS), European Society of Minimally Invasive Neurological Therapy (ESMINT), European Society of Neuroradiology (ESNR), European Stroke Organization (ESO), Society for Cardiovascular Angiography and Interventions (SCAI), Society of Interventional Radiology (SIR), Society of NeuroInterventional Surgery (SNIS), and World Stroke Organization (WSO). J. Vasc. Interv. Radiol. 29, 441–453. doi: 10.1016/j.jvir.2017.11.026
Sandbhor, P., Goda, J., Mohanty, B., Gera, P., Yadav, S., Chekuri, G., et al. (2023). Targeted nano-delivery of chemotherapy via intranasal route suppresses in vivo glioblastoma growth and prolongs survival in the intracranial mouse model. Drug Deliv. Transl. Res. 13, 608–626. doi: 10.1007/s13346-022-01220-8
Schiffer, D., Mellai, M., Bovio, E., Bisogno, I., Casalone, C., and Annovazzi, L. (2018). Glioblastoma niches: From the concept to the phenotypical reality. Neurol. Sci. 39, 1161–1168. doi: 10.1007/s10072-018-3408-0
Semyachkina-Glushkovskaya, O., Shirokov, A., Blokhina, I., Telnova, V., Vodovozova, E., Alekseeva, A., et al. (2022). Intranasal delivery of liposomes to glioblastoma by photostimulation of the lymphatic system. Pharmaceutics 15:36. doi: 10.3390/pharmaceutics15010036
Setti, M., Savalli, N., Osti, D., Richichi, C., Angelini, M., Brescia, P., et al. (2013). Functional role of CLIC1 ion channel in glioblastoma-derived stem/progenitor cells. J. Natl. Cancer Inst. 105, 1644–1655. doi: 10.1093/jnci/djt278
Sonabend, A. M., Gould, A., Amidei, C., Ward, R., Schmidt, K. A., Zhang, D. Y., et al. (2023). Repeated blood-brain barrier opening with an implantable ultrasound device for delivery of albumin-bound paclitaxel in patients with recurrent glioblastoma: A phase 1 trial. Lancet Oncol. 24, 509–522. doi: 10.1016/S1470-2045(23)00112-2
Sperring, C. P., Argenziano, M. G., Savage, W. M., Teasley, D. E., Upadhyayula, P. S., Winans, N. J., et al. (2023). Convection-enhanced delivery of immunomodulatory therapy for high-grade glioma. Neurooncol. Adv. 5:vdad044. doi: 10.1093/noajnl/vdad044
Sprowls, S. A., Arsiwala, T. A., Bumgarner, J. R., Shah, N., Lateef, S. S., Kielkowski, B. N., et al. (2019). Improving CNS delivery to brain metastases by blood-tumor barrier disruption. Trends Cancer 5, 495–505. doi: 10.1016/j.trecan.2019.06.003
Sun, S., Shi, D., Liu, J., Lu, J., Dou, P., Zhou, Z., et al. (2023). Glioblastoma relapse post-resection model for therapeutic hydrogel investigations. J. Vis. Exp. doi: 10.3791/65026
Sun, T., Krishnan, V., Pan, D. C., Filippov, S. K., Ravid, S., Sarode, A., et al. (2022). Ultrasound-mediated delivery of flexibility-tunable polymer drug conjugates for treating glioblastoma. Bioeng. Transl. Med. 8:e10408. doi: 10.1002/btm2.10408
Szeliga, M., and Rola, R. (2022). Menadione potentiates auranofin-induced glioblastoma cell death. Int. J. Mol. Sci. 23:15712. doi: 10.3390/ijms232415712
Thombre, R., Mess, G., Kempski Leadingham, K. M., Kapoor, S., Hersh, A., Acord, M., et al. (2023). Towards standardization of the parameters for opening the blood-brain barrier with focused ultrasound to treat glioblastoma multiforme: A systematic review of the devices, animal models, and therapeutic compounds used in rodent tumor models. Front. Oncol. 12:1072780. doi: 10.3389/fonc.2022.1072780
Thompson, E. M., Landi, D., Brown, M. C., Friedman, H. S., McLendon, R., Herndon, J. E., et al. (2023). Recombinant polio-rhinovirus immunotherapy for recurrent paediatric high-grade glioma: A phase 1b trial. Lancet Child Adolesc. Health 7, 471–478. doi: 10.1016/S2352-4642(23)00031-7
Tian, Y., Lei, Y., Wang, Y., Lai, J., Wang, J., and Xia, F. (2023). Mechanism of multidrug resistance to chemotherapy mediated by P-glycoprotein (Review). Int. J. Oncol. 63:119. doi: 10.3892/ijo.2023.5567
Torp, S. H., Solheim, O., and Skjulsvik, A. J. (2022). The WHO 2021 classification of central nervous system tumours: A practical update on what neurosurgeons need to know-a minireview. Acta Neurochir. 164, 2453–2464. doi: 10.1007/s00701-022-05301-y
Triguero, D., Buciak, J. B., Yang, J., and Pardridge, W. M. (1989). Blood-brain barrier transport of cationized immunoglobulin G: Enhanced delivery compared to native protein. Proc. Natl. Acad. Sci. U.S.A. 86, 4761–4765. doi: 10.1073/pnas.86.12.4761
Vítovcová, B., Skarková, V., Havelek, R., Soukup, J., Pande, A., Caltová, K., et al. (2023). Flubendazole exhibits anti-glioblastoma effect by inhibiting STAT3 and promoting cell cycle arrest. Sci. Rep. 13:5993. doi: 10.1038/s41598-023-33047-9
Wan, Z., Zuo, X., Wang, S., Zhou, L., Wen, X., Yao, Y., et al. (2023). Identification of angiogenesis-related genes signature for predicting survival and its regulatory network in glioblastoma. Cancer Med. 12, 17445–17467. doi: 10.1002/cam4.6316
Wang, W., He, H., Zeng, S., Cho, H. Y., Minea, R. O., Swenson, S. D., et al. (2023). Enhanced brain entry of checkpoint-inhibitory therapeutic antibodies facilitated by intraarterial NEO100 in mouse models of brain-localized malignancies. J. Neurosurg. 139, 822–830. doi: 10.3171/2022.12.JNS221285
White, K., Connor, K., Meylan, M., Bougoüin, A., Salvucci, M., Bielle, F., et al. (2023). Identification, validation and biological characterisation of novel glioblastoma tumour microenvironment subtypes: Implications for precision immunotherapy. Ann. Oncol. 34, 300–314. doi: 10.1016/j.annonc.2022.11.008
Yan, H., Zhu, J., Ping, Y., Yan, M., Liao, G., Yuan, H., et al. (2023). The heterogeneous cellular states of glioblastoma stem cells revealed by single cell analysis. Stem Cells 41, 111–125. doi: 10.1093/stmcls/sxac088
Yavuz, M., and Demircan, T. (2023). The effect of hydroquinidine on proliferation and apoptosis of TMZ-sensitive and -resistant GBM Cells. Anticancer Agents Med. Chem. 23, 938–952. doi: 10.2174/1871520623666221125115542
Younis, M. R., He, Y., Yao, X., He, G., Liu, H., Huang, P., et al. (2023). Acidity/carbon dioxide-sensitive triblock polymer-grafted photoactivated vesicles for programmed release of chemotherapeutic drugs against glioblastoma. Acta Biomater. 157, 442–450. doi: 10.1016/j.actbio.2022.11.053
Yu, Y., Wang, A., Wang, S., Sun, Y., Chu, L., Zhou, L., et al. (2022). Efficacy of temozolomide-conjugated gold nanoparticle photothermal therapy of drug-resistant glioblastoma and its mechanism study. Mol. Pharm. 19, 1219–1229. doi: 10.1021/acs.molpharmaceut.2c00083
Yun, B., Gu, Z., Liu, Z., Han, Y., Sun, Q., and Li, Z. (2022). Reducing chemo-/radioresistance to boost the therapeutic efficacy against temozolomide-resistant glioblastoma. ACS Appl. Mater. Interfaces 14, 38617–38630. doi: 10.1021/acsami.2c12348
Zampieri, L. X., Sboarina, M., Cacace, A., Grasso, D., Thabault, L., Hamelin, L., et al. (2021). Olaparib is a mitochondrial complex i inhibitor that kills temozolomide-resistant human glioblastoma cells. Int. J. Mol. Sci. 22:11938. doi: 10.3390/ijms222111938
Zhang, G., Xu, X., Zhu, L., Li, S., Chen, R., Lv, N., et al. (2023). A novel molecular classification method for glioblastoma based on tumor cell differentiation trajectories. Stem Cells Int. 2023:2826815. doi: 10.1155/2023/2826815
Zhang, L., Jiang, Y., Zhang, G., and Wei, S. (2023). The diversity and dynamics of tumor-associated macrophages in recurrent glioblastoma. Front. Immunol. 14:1238233. doi: 10.3389/fimmu.2023.1238233
Zhang, R., Ye, Y., Wu, J., Gao, J., Huang, W., Qin, H., et al. (2023). Immunostimulant in situ fibrin gel for post-operative glioblastoma treatment by macrophage reprogramming and photo-chemo-immunotherapy. ACS Appl. Mater. Interfaces 15, 17627–17640. doi: 10.1021/acsami.3c00468
Zhang, Y., Wu, X., Zhu, J., Lu, R., and Ouyang, Y. (2023). Knockdown of SLC39A14 inhibits glioma progression by promoting erastin-induced ferroptosis SLC39A14 knockdown inhibits glioma progression. BMC Cancer 23:1120. doi: 10.1186/s12885-023-11637-0
Zhao, J., Wang, Y., Tao, L., and Chen, L. (2022). Iron transporters and ferroptosis in malignant brain tumors. Front. Oncol. 12:861834. doi: 10.3389/fonc.2022.861834
Zhao, M., Danhier, F., Bastiancich, C., Joudiou, N., Ganipineni, L. P., Tsakiris, N., et al. (2018). Post-resection treatment of glioblastoma with an injectable nanomedicine-loaded photopolymerizable hydrogel induces long-term survival. Int. J. Pharm. 548, 522–529. doi: 10.1016/j.ijpharm.2018.07.033
Keywords: glioblastoma, local delivery, microenvironment, blood brain barrier, drug repurposing
Citation: Chiariello M, Inzalaco G, Barone V and Gherardini L (2023) Overcoming challenges in glioblastoma treatment: targeting infiltrating cancer cells and harnessing the tumor microenvironment. Front. Cell. Neurosci. 17:1327621. doi: 10.3389/fncel.2023.1327621
Received: 25 October 2023; Accepted: 05 December 2023;
Published: 21 December 2023.
Edited by:
Maria Cristina D’Adamo, LUM Giuseppe Degennaro University, ItalyReviewed by:
Mario Valentino, University of Malta, MaltaCopyright © 2023 Chiariello, Inzalaco, Barone and Gherardini. This is an open-access article distributed under the terms of the Creative Commons Attribution License (CC BY). The use, distribution or reproduction in other forums is permitted, provided the original author(s) and the copyright owner(s) are credited and that the original publication in this journal is cited, in accordance with accepted academic practice. No use, distribution or reproduction is permitted which does not comply with these terms.
*Correspondence: Lisa Gherardini, bGlzYS5naGVyYXJkaW5pQGNuci5pdA==
Disclaimer: All claims expressed in this article are solely those of the authors and do not necessarily represent those of their affiliated organizations, or those of the publisher, the editors and the reviewers. Any product that may be evaluated in this article or claim that may be made by its manufacturer is not guaranteed or endorsed by the publisher.
Research integrity at Frontiers
Learn more about the work of our research integrity team to safeguard the quality of each article we publish.