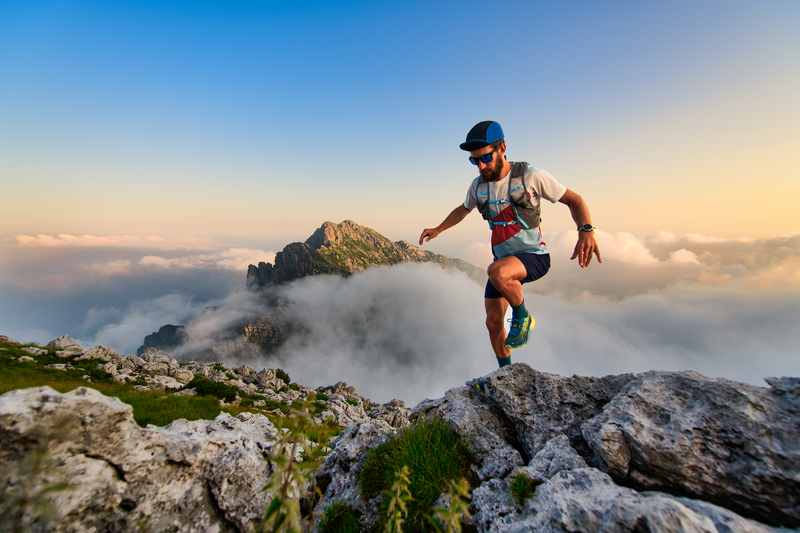
94% of researchers rate our articles as excellent or good
Learn more about the work of our research integrity team to safeguard the quality of each article we publish.
Find out more
REVIEW article
Front. Cell. Neurosci. , 30 November 2023
Sec. Cellular Neuropathology
Volume 17 - 2023 | https://doi.org/10.3389/fncel.2023.1291255
This article is part of the Research Topic Cellular and Molecular Mechanisms of Neurotropic Viral Infection View all 6 articles
Intracranial (i.c.) inoculation of susceptible mice with a glial-tropic strain of mouse hepatitis virus (JHMV), a murine coronavirus, results in an acute encephalomyelitis followed by viral persistence in white matter tracts accompanied by chronic neuroinflammation and demyelination. Microglia serve numerous functions including maintenance of the healthy central nervous system (CNS) and are among the first responders to injury or infection. More recently, studies have demonstrated that microglia aid in tailoring innate and adaptive immune responses following infection by neurotropic viruses including flaviviruses, herpesviruses, and picornaviruses. These findings have emphasized an important role for microglia in host defense against these viral pathogens. In addition, microglia are also critical in optimizing immune-mediated control of JHMV replication within the CNS while restricting the severity of demyelination and enhancing remyelination. This review will highlight our current understanding of the molecular and cellular mechanisms by which microglia aid in host defense, limit neurologic disease, and promote repair following CNS infection by a neurotropic murine coronavirus.
The Coronaviridae family is composed of large (26–32 kb), enveloped, single-stranded RNA viruses in the order Nidovirales (Bergmann et al., 2006; Gorbalenya et al., 2006) that are classified into groups based on shared sequencing homologies and serologic cross-reactivity (Bergmann et al., 2006; Gorbalenya et al., 2006). Their naming dates to the 1960s when McIntosh et al. (1967) isolated infectious bronchitis virus (IBV)-like virions from the upper respiratory tracts of patients with common colds. When electron micrographs of avian IBV and the newly isolated human strains were compared, the “club- or pear-shaped” projections on their viral coats showed strikingly similar characteristics, which ultimately led to grouping these viruses together with the coronavirus family name, based on the Latin root, “corona,” for their crown-like appearance (Tyrrell and Myint, 1996).
The neuroattenuated John Howard Mueller (JHM) strain of mouse hepatitis virus (JHMV) is a well-characterized laboratory strain that can cause severe encephalomyelitis and demyelination in adult mice (Cheever et al., 1949; Fleming et al., 1986). Infection of susceptible mice with JHMV has proven to be an effective mouse model for studying various immunologic and pathologic features associated with viral-induced neurologic disease. Following a sublethal intracranial (i.c.) infection with JHMV, virus rapidly infects and replicates within ependymal cells lining the lateral ventricles (Wang et al., 1992; Lane et al., 1998). Within 24-h, JHMV rapidly spreads and penetrates further into the parenchyma where astrocytes, oligodendrocytes, and microglia are targets of infection, while neurons are relatively spared (Fleming et al., 1986; Wang et al., 1992). Viral titers within the brain peak between days 5 and 7 post-infection (p.i.) and decline below levels of detection by plaque assay (∼100 PFU/g tissue) between 10 and 14 days p.i. Sterilizing immunity is incomplete, as viral antigen and RNA are capable of persisting within the CNS (Marten et al., 2000), and this is associated with chronic neuroinflammation leading to an immune-mediated demyelinating disease, which is mediated by inflammatory T cells and myeloid cells (Perlman et al., 1999; Wu and Perlman, 1999; Wu et al., 2000; Glass et al., 2001, 2004; Liu et al., 2001c; Dufour et al., 2002; Pewe and Perlman, 2002; Pewe et al., 2002; Kim and Perlman, 2005; Bergmann et al., 2006; Sariol et al., 2020). More recently, roles for microglia in both demyelination and remyelination in JHMV-infected mice have been implicated (Wheeler et al., 2018; Mangale et al., 2020; Sariol et al., 2020). Due to the clinical and histologic similarities to the human demyelinating disease, multiple sclerosis (MS), the JHMV model of viral-induced neurologic disease is considered a relevant pre-clinical model of MS (Lane and Buchmeier, 1997; Bergmann et al., 2006).
Microglia are the brain’s resident immune cells, comprising approximately 5–12% of all cells found in the brain, and, together with perivascular, choroid plexus, and meningeal macrophages, comprise the macrophage compartment of the CNS. Originally presumed to be “resting,” microglia in the healthy adult brain are highly dynamic, surveying the entire brain parenchyma every 24 h. In this “surveying” state, microglia exhibit a ramified morphology and serve to support neuronal function and health via physical interactions and a vast repertoire of released signaling molecules and enzymes (Nimmerjahn et al., 2005; Kierdorf and Prinz, 2017; Hume et al., 2019). Recent research indicates that these cells have a long lifespan, sustain their population through self-renewal, and display molecular and transcriptional diversity based on location within the CNS (Reu et al., 2017; Masuda et al., 2019; Zhan et al., 2019). When faced with infections, aging, or injuries, microglia adapt in terms of transcription, morphology, and function; changes that are typically beneficial or vital for recovery (Hammond et al., 2019; Mathys et al., 2019). In acute inflammatory events, the pro-inflammatory response resolves and microglia continue their surveillance of the brain parenchyma, returning to brain homeostasis. However, in response to injury or infection, the equilibrium between microglial surveillance and activation can be disturbed, creating a chronic neuroinflammatory state that can lead to tissue damage. Beyond their immune roles, it’s now understood that microglia play other roles in the homeostatic and developing brain (Salter Michael and Beggs, 2014; Li and Barres, 2018; Prinz et al., 2019). These findings have occurred across a backdrop of increasingly elegant methodological advances including single cell analyses (Hammond et al., 2019; Li et al., 2019; Masuda et al., 2019), microglial ablation paradigms (Elmore et al., 2014; Bruttger et al., 2015; Han et al., 2017, 2019; Green et al., 2020), and in vivo imaging techniques (Nimmerjahn et al., 2005; Miyamoto et al., 2016; Liu et al., 2019; Stowell et al., 2019), that together have characterized the dynamic influence microglia have on virtually all major CNS cell-types over the lifespan of an organism.
We recently employed scRNAseq on flow-sorted CD45+ cells enriched from the CNS of JHMV-infected mice at defined times to better understand these processes. We chose to examine brains during peak innate (day 3 p.i.) and adaptive (day 7 p.i.) responses, and spinal cords to evaluate immune cell responses during chronic demyelination (day 21 p.i.) (Syage et al., 2020). Downstream analysis of processed data on cells isolated from brains and spinal cords of control and infected mice at days 3, 7, and 21 p.i., revealed 22 unique clusters (Figure 1A). With regard to the four microglia subsets (MG1, MG2, MG3, and Cycling MG), each had unique transcriptional signatures enabling grouping into specific subsets, further enforcing the notion that microglia exhibit dynamic responses following JHMV infection of the CNS (Figure 1B).
Figure 1. scRNAseq analysis of CD45+ cells within the CNS of JHMV-infected mice. C57BL/6 mice were infected i.c. with JHMV and brains collected at days 3 and 7 p.i. and spinal cords collected at day 21 p.i. (A) UMAP plot showing aggregate data of the immune landscape in brains and spinal cords of uninfected (control) and infected mice at 3, 7, and 21 days p.i. with JHMV revealing 22 distinct cell clusters (5–6 mice pooled per group). (B) Heatmap showing top 5 differentially expressed transcripts between heterogeneous subpopulations of microglia. Each heatmap is generated with subset data from microglia populations and isolated from all other clusters outside of what is shown in each individual map. Data are aggregated from uninfected (control) and infected at days 3, 7, and 21 p.i. Columns represent the different clusters, and rows represent expression of transcripts. Figures derived from Syage et al. (2020).
Examination of innate anti-viral immune responses by microglia following JHMV infection revealed increased interferon alpha (IFN-α) responses. This observation was accompanied by increased expression of transcripts encoding anti-viral response factors including Myd88, Rsad2 (Viperin), and Tmem173 (STING) that were enriched in distinct subpopulations of microglia (Figures 2A, B). Expression of anti-viral effector responses at early stages of JHMV infection appear regulated within different subpopulations of microglia and this may reflect response to viral infection within discrete anatomic locations within the brain and/or exposure to type I interferons that can affect expression of anti-viral transcripts.
Figure 2. Microglial innate immune response following JHMV infection of the CNS. (A) Gene set enrichment analysis (GSEA) for IFN-α responses in microglia at day 3 p.i. from brains of JHMV-infected mice. Area under the curve represents enrichment of response genes. Normalized enrichment scores and p-values shown. (B) Data showing expression of Myd88, Rsad2, and Tmem173 transcripts in microglia subpopulations as well as overall temporal expression at defined times p.i. with JHMV. Box plots show interquartile range, median value (bold horizontal bar), and average expression value per sample (red dots). ns, not significant, ***p < 0.001, ****p < 0.0001. Images derived from Syage et al. (2020).
Viral replication peaks between 5 and 7 days p.i., and this is accompanied by a robust inflammatory response within the CNS. At the same time, there is a decline in viral replication that is associated with infiltration of virus-specific CD4+ and CD8+ T cells expressing IFN-γ and perforin (Bergmann et al., 2004). Microglia are responsive to IFN-γ signaling (Figure 3A), which enhances expression of MHC class I-associated transcripts (Figure 3B) and MHC class II-associated transcripts (Figure 3C). Temporal analysis of these transcripts in microglia show very low expression in uninfected mice that gradually increases by day 3 p.i. and is dramatically elevated by days 7 and 21 p.i (Figures 3B, C). JHMV infection invokes expression of T cell chemoattractant chemokines, CXCL9 and CXCL10, that attract activated T and B lymphocytes into the CNS via binding to the receptor CXCR3; this is central in promoting an effective host defense by controlling viral replication (Liu et al., 2001a,b, c; Phares et al., 2013). While astrocytes are important sources of both CXCL19 and CXCL10 in response to CNS infection by JHMV (Lane et al., 1998; Liu et al., 2001a; Phares et al., 2013), microglia subsets also express these transcripts within the brain at days 3 and 7 p.i. but to a lesser extent within the spinal cord at day 21 p.i (Figure 3D; Syage et al., 2020). We interpret these findings to indicate that microglia exert an important role in host defense following JHMV infection during acute disease by aiding in attracting virus-specific T cells into the CNS and subsequently presenting viral antigen. During chronic immune-mediated demyelinating disease, microglia may play a more subordinate role to astrocytes and monocyte/macrophages with regards to expressing T cell chemoattractants.
Figure 3. IFN-γ signaling activates microglia and corresponds with increased expression of MHC class I and II transcripts. (A) Gene set enrichment analysis (GSEA) for IFN-γ responses in microglia at day 7 p.i. from brains of JHMV-infected mice. Area under the curve represents enrichment of response genes. Normalized enrichment scores and p-values shown. Box plots showing expression of (B) MHC class I-associated genes, H2-K1 and H2-Q7, and (C) expression of MHC class II-associated genes, H2-Aa and H2-Eb1, in subpopulations of microglia in control mice and at defined times p.i. (D) Expression of Cxcl9 and Cxcl10 transcripts in microglia (MG) subsets in control mice and at defined times p.i. Box plots show interquartile range, median value (bold horizontal bar), and average expression value per sample (red dots). ns, not significant, **p < 0.01, ****p < 0.0001. Images derived from Syage et al. (2020).
We have also determined that commensals aid in host defense following JHMV infection of the CNS through enhancing microglia function (Brown et al., 2019). Germfree mice or animals that receive antibiotics are unable to control JHMV replication within the brain during acute disease. The impaired ability of virus-specific T cells in germfree mice to control viral replication was not due to T cell-intrinsic defects but was the result of deficient MHC class II expression on microglia. Moreover, oral administration of toll-like receptor (TLR) to JHMV-infected mice limited the severity of clinical disease, and this correlated with increased MHC class II expression on microglia and efficient control of viral replication within the brains. Further work revealed that signaling through TLR4 is important in the homeostatic activation of microglia, as targeted genetic disruption of Tlr4 within microglia leads to increased viral-induced clinical disease (Brown et al., 2019). These findings demonstrate that gut immune-stimulatory products can influence microglia function to prevent CNS damage following viral infection.
By day 21 p.i., JHMV persists within the spinal cords of surviving mice, and this is associated with ongoing neuroinflammation and demyelination in which inflammatory T cells and monocytes/macrophages contribute to white matter damage (Wu and Perlman, 1999; Wu et al., 2000; Glass et al., 2004). Microglia express transcripts encoding genes associated with demyelination including Apolipoprotein E (Apoe), Transmembrane glycoprotein NMB (Gpnmb), Osteopontin (Spp1), and Triggering receptor expressed on myeloid cells 2 (Trem2) (Chabas et al., 2001; Ulrich and Holtzman, 2016; Hendrickx et al., 2017; Krasemann et al., 2017; Figure 4A). Under non-disease conditions, microglia function to maintain tissue homeostasis and have also been shown to be important in maintaining overall myelin health through regulation of myelin growth as well as preservation of myelin integrity (Yin et al., 2017; Amor et al., 2022; McNamara et al., 2023). Following JHMV-induced demyelination, microglia subsets express transcripts encoding markers associated with remyelination, including cystatin F (Cst7) (Ma et al., 2011; Shimizu et al., 2017), insulin-like growth factor 1 (Igf1) (Ye et al., 2002; Wlodarczyk et al., 2017), and lipoprotein lipase (Lpl) (Bruce et al., 2018; Figure 4B). The highest levels of expression for Cst7, Igf1, and Lpl occurred within the spinal cord at day 21 p.i. Additionally, microglia express transcripts associated with phagocytosis of myelin debris at both days 14 and 21 p.i. and electron micrographs show microglia containing phagocytosed myelin and axonal debris at day 21 p.i. (Sariol et al., 2020), a function that is essential for remyelination to occur. These findings support the notion that microglia may either directly or indirectly influence remyelination within the spinal cord by contributing to expression of genes encoding proteins that regulate oligodendrocyte precursor cell (OPC) survival and maturation (Lloyd and Miron, 2019; Lloyd et al., 2019).
Figure 4. Microglia contribute to JHMV-induced demyelination and promote remyelination. (A) Expression of transcripts, Apoe, Gpnmb, Spp1, and Trem2, that are associated with demyelination are elevated in spinal cords at day 21 p.i. in specific subpopulations of microglia in control mice and at defined times p.i. (B) Expression of remyelination markers, Cst7, Igf1, and Lpl, are also increased in specific subpopulations of microglia in spinal cords at day 21 p.i. with JHMV. Box plots show interquartile range, median value (bold horizontal bar), and average expression value per sample (red dots). ns, not significant, *p < 0.05, **p < 0.01, ****p < 0.0001. Images derived from Syage et al. (2020).
The functional roles of microglia in contributing to host defense in response to CNS infection with neurotropic viruses have been greatly elucidated by findings demonstrating that mice lacking colony stimulating factor 1 receptor (Csfr1-/-) lack microglia, emphasizing the importance of this signaling pathway in microglia development (Ginhoux et al., 2010). Subsequent studies by Elmore et al. (2014) showed that blocking CSF1R signaling in adult mice through administration of CSF1R antagonists leads to > 95% reduction in microglia, demonstrating that CSF1R is necessary for microglia survival. More recently, treatment of mice with PLX5622, a brain penetrant and selective antagonist of the CSF1R that results in a dramatic reduction in microglia, has helped elucidate the functional roles of these cells in pre-clinical models of neurodegenerative disease (Elmore et al., 2014; Dagher et al., 2015; Acharya et al., 2016; Spangenberg et al., 2019). Wheeler et al. (2018) were the first to demonstrate that administration of PLX5622 prior to JHMV-infected mice resulted in increased mortality, associated with impaired control of viral replication within the brain during early stages of disease. Increased viral titers in PLX5622-treated mice were associated with a reduction in MHC class II expression on monocytes/macrophages similar to findings by Wheeler et al. (2018). Subsequent studies showed that PLX5622-mediated ablation of microglia results in increased susceptibility to West Nile virus (Seitz et al., 2018; Funk and Klein, 2019), Japanese encephalitis virus (JEV) (Seitz et al., 2018), and Theiler’s murine encephalomyelitis virus (TMEV) (Waltl et al., 2018; Sanchez et al., 2019), further supporting a protective role for microglia against acute viral-induced encephalitis.
Our laboratory depleted microglia via administration of PLX5622 to mice prior to JHMV-infection to further evaluate how microglia tailor the immune environment in response to CNS infection (Mangale et al., 2020). We employed scRNAseq to better understand how depletion of microglia within the CNS influenced transcriptional responses by myeloid cells and infiltrating lymphocytes. Similar to Wheeler et al. (2018), our findings indicated that depletion of microglia prior to JHMV infection resulted in increased mortality, associated with higher viral titers within brains and spinal cords. Expression of type I interferons (IFN) is a critical first line of defense that limits viral dissemination, and microglia are considered important sentinel cells capable of type I IFN expression (Ireland et al., 2008). We found that ablation of microglia led to increased expression of IFN-α in both macrophages and dendritic cells, suggesting that these cells were responding to the overall increased levels of virus within the CNS. Although we did not examine expression of type I IFNs in other cells types, it is also reasonable to argue that resident CNS cells, including neurons and astrocytes, were also able to respond to elevated levels of virus through production of type I IFNs (Hwang and Bergmann, 2018, 2019, 2020). There was not a dramatic change in immune cell infiltration into the CNS of JHMV-infected, PLX5622-treated mice compared to control mice, arguing that microglia may not be critical in terms of impacting neuroinflammation in response to infection. However, CD4+ T cells from PLX5622-treated mice did exhibit a reduced activation state, as determined by a reduction in expression of transcripts encoding Th1-associated transcription factor, Tbet, and activation markers, Cd69 and Cd44. This overall muted CD4+ T cell response was associated with a reduction in expression of MHC class II transcripts and protein by macrophages within the brains of PLX5622-treated mice. In contrast, there were no differences in expression of MHC class I expression on macrophages in PLX5622-treated mice, and expression of both MHC class I and II transcripts in dendritic cells was not affected. Collectively, these findings argue that during acute disease, microglia tailor the CNS microenvironment in response to JHMV infection by influencing effective host defense mechanisms that enable efficient T cell-mediated control of viral replication. Presumably, this is done through secretion of cytokines/chemokines that allow for efficient expression of MHC class II, promoting effective anti-viral CD4+ T cell responses, which subsequently enhance anti-viral CD8+ T cell responses.
As part of our studies evaluating how PLX5622 treatment impacted host defense in response to CNS infection by JHMV, we also determined how microglia ablation affected the severity of demyelination. PLX5622 treatment led to increased spinal cord demyelination compared to control mice (Figures 5A, B). scRNAseq analysis indicated that the increase in white matter damage in PLX5622 was associated with increased expression of transcripts encoding molecules associated with demyelination including Apoe (Krasemann et al., 2017), Spp1 (Chabas et al., 2001), and Trem2 (Ulrich and Holtzman, 2016; Krasemann et al., 2017) in macrophage clusters. In addition, electron microscopy (EM) revealed impaired remyelination in PLX5622-treated mice as indicated by an increase in the g-ratio (the ratio of the inner axonal diameter to the total outer fiber diameter) (Figures 5C, D). There was a reduction in expression of transcripts associated with remyelination including Cst7, Igf1, and Lpl in spinal cord macrophages of PLX5622-treated mice (Figure 5E; Ye et al., 2002; Ma et al., 2011; Hlavica et al., 2017; Shimizu et al., 2017; Wlodarczyk et al., 2017; Bruce et al., 2018; Durose et al., 2019). This suggests that microglia impacted the expression of molecules associated with both demyelination and remyelination in macrophages.
Figure 5. The severity of spinal cord demyelination is increased in PLX5622-treated mice. (A) Representative images of H&E/LFB-stained spinal cord sections showing an increase in severity of demyelination (dashed black lines) in JHMV-infected mice treated with PLX5622 compared to control treated mice at day 14 (B) Quantification of spinal cord demyelination reveals a significant increase after PLX5622 treatment compared to control-treated animals at days 14 and 21 p.i. (C) Representative EM images (1200×) from spinal cords from control and PLX5622-treated mice showing normal myelinated axons (white arrowheads), demyelinated axons (black arrows), and remyelinated axons (blue arrows) at day 21 p.i. (D) Calculation of g-ratio of control and PLX5622; scatter plot depicting individual g-ratios from lateral white matter columns of control (gray) and PLX5622 (green)-treated mice as a function of axon diameter. (E) t-SNE plots showing decreased expression of transcripts encoding remyelination-associated markers, Cst7, Igf1, and Lpl in spinal cords of PLX5622-treated mice compared to controls at day 14 p.i. *p < 0.05, **p < 0.01, ****p < 0.0001. Images derived from Mangale et al. (2020).
Determining how targeted ablation of microglia influences the microenvironment once JHMV-induced demyelination is already established is another important and clinically relevant question for potentially treating a number of neurodegenerative diseases. Elegant studies by Sariol et al. (2020) demonstrated that treatment with PLX5622 at various times following JHMV resulted in varied outcomes in host defense, demyelination, and remyelination. For example, depletion of microglia beginning at day 7 p.i. with JHMV resulted in worsened clinical disease, associated with increased demyelination and reduced remyelination, yet CNS viral titers were not affected when compared to control mice. Consistently, ablation of microglia during acute disease (day 7 p.i.) led to increased accumulation of extracellular vesiculated myelin and cellular debris in the spinal cords of PLX5622-treated mice, combined with demonstrated differential expression of genes involved in myelin debris clearance. In contrast, PLX5622-treatment beginning at day 15 p.i. did not affect the severity of clinical disease nor increase demyelination. Subsequently, we published findings indicating that PLX5622-mediated depletion of microglia beginning at day 14 p.i led to an increase in the severity of demyelination and impaired remyelination compared to control mice. As well, gene expression analysis revealed that ablating microglia resulted in altered expression of genes associated with immune cell activation and phagocytosis of myelin debris (Cheng et al., 2023). Collectively, these findings argue that PLX5622 treatment following JHMV infection does affect the severity of both demyelination and remyelination. This indicates that microglia continue to exert an important role in influencing neuropathology during acute/sub-acute stages of disease, and phagocytosis of myelin debris specifically by microglia is important in regulating tissue damage and repair.
Coronaviruses that have been identified that infect humans (HCoV’s) include HCoV-229E, HCoV-OC43, HCoV-NL63, HCoV-HKU1, severe acute respiratory syndrome (SARS)-CoV, Middle East respiratory syndrome (MERS)-CoV, and SARS-CoV-2. Notably, infection with all of these viruses has been associated with effects on neurologic function (Morgello, 2020). The four commonly circulating HCoV’s, 229E, OC43, NL63, and HKU1, are often referred to as the common cold coronaviruses and account for approximately 15–20% of seasonal colds and are associated with mild-to-moderate infections of the upper respiratory tract (Holmes and Lai, 1996; Perlman et al., 1999; Wang et al., 2020). Comparatively, SARS-CoV-1, MERS-CoV, and SARS-CoV-2 have been associated with severe respiratory disease and have prompted public health emergencies. In addition to severe respiratory disease following infection with these viruses, CNS invasion and neuropathology have been reported (Iadecola et al., 2020). In comparison with SARS-CoV-1 and MERS-CoV, neurologic symptoms which range in severity are more common following SARS-CoV-2 infection. Neuropathological findings associated with SARS-CoV-2 infection include lymphocyte inflammation, acute hypoxic-ischemic changes, astrogliosis, and spontaneous hemorrhage (Pleasure et al., 2020; Lou et al., 2021; Maury et al., 2021; Schwabenland et al., 2021; Stein et al., 2023). In addition, microglial activation is also commonly detected in COVID-19 patients (Pleasure et al., 2020; Lou et al., 2021; Maury et al., 2021; Schwabenland et al., 2021; Stein et al., 2023). SARS-CoV-2 viral RNA and antigen have been detected within the CNS and cerebral spinal fluid of COVID-19 patients upon post-mortem analysis. This finding, along with the observation that the virus is able to infect and replicate within resident cells of the CNS, supports the view that the virus is neurotropic (Matschke et al., 2020; Bellon et al., 2021; Olivarria et al., 2022; Proust et al., 2023). Neurologic symptoms associated with COVID-19 are thought to occur via a variety of different mechanisms, including endothelial damage of the blood-brain-barrier (BBB), which is associated with increased capillary damage (Helms et al., 2020; Magro et al., 2020). While cytokine storm is considered to be a major cause of acute respiratory distress syndrome (ARDS) and multiple organ failure (Chousterman et al., 2017), it also is considered to be a contributing factor of neurological complications of COVID-19 (Almutairi et al., 2021; Wang and Perlman, 2022). It has been observed that patients with more severe COVID-19 have a more drastic inflammatory immune response (Chen et al., 2020; Huang et al., 2020), leading to the release of proinflammatory cytokines (Huang et al., 2020; Ragab et al., 2020; Ye et al., 2020). Some of these inflammatory markers including IL-6 and IL-1β are also elevated in animal models (Klein et al., 2021) and are associated with impaired neurogenesis and hippocampal dependent memory (Garber et al., 2018; Kong et al., 2019). IL-6 is associated with increased viral loads and disease severity (Chen et al., 2020, 2021) and has been demonstrated to be involved in neurodegenerative diseases mediated by neuroinflammation (Strafella et al., 2020). Increased cytokine levels have also been seen in patients experiencing pneumonia and hypoxia (Arnaldez et al., 2020), and hypoxic changes in vitro and in patients have been associated with neuronal death and loss (Song et al., 2021; Solomon and Liang, 2022). In addition, evidence of neuronal degeneration and changes in glial cell morphology are also present in some hippocampal tissues derived from COVID-19 patients (Bayat et al., 2022). As indicated above, microglia have been shown to have distinct roles in host defense and disease in response to infection of the murine CNS with JHMV; similarly, microglia are suspected to have some role in neurologic manifestations associated with COVID-19 as well as long COVID (also called Post-Acute Sequelae of COVID, PASC) (Schwabenland et al., 2021; Albornoz et al., 2022; Jeong et al., 2022; Monje and Iwasaki, 2022; Strong, 2023; Thaweethai et al., 2023; Wei et al., 2023). Moreover, animal models of SARS-CoV-2 infection have demonstrated microglial activation and a role in the expression of cytokines/chemokines associated with neuroinflammation (Munoz-Fontela et al., 2020; Amruta et al., 2022; Olivarria et al., 2022). Ongoing studies involving COVID-19 patients as well as pre-clinical animal studies will ultimately reveal how microglia and other CNS resident cells affect neurologic disease in SARS-CoV-2-infected individuals.
It is now well-recognized that, in addition to serving as the immune sentinel cell of the brain, microglia exert key roles in CNS development, tissue homeostasis, and response to both injury and infection. These diverse roles of microglia have been clearly revealed within the context of viral infection of the CNS. The JHMV model of viral-induced encephalomyelitis and immune-mediated demyelination has provided important insight with regards to how microglia influence host defense during acute disease, as well as how these cells participate in restricting demyelination and enhancing remyelination. An overview of roles of microglia in distinct stages of defense and disease in response to JHMV infection of the CNS is provided in Figure 6. Ongoing studies, using increasingly sophisticated approaches, are required to gain additional insight into the molecular mechanisms by which microglia impact the microenvironment and influence resident CNS cells and inflammatory cells to effectively respond to viral infection and aid in tissue recovery, with the ultimate goal of identifying unique therapeutic targets that may aid in dampening disease progression in human demyelinating diseases as well as individuals infected with human coronaviruses.
Figure 6. Roles of microglia in defense, disease, and repair in response to JHMV infection of the CNS. Overview of influence of microglia at defined stages following CNS infection of the CNS. Microglia aid in defense on at innate and adaptive stages of infection through release of type I interferons along with other antiviral pathways as well as antigen presentation to virus-specific T cells (Wheeler et al., 2018; Mangale et al., 2020). While these anti-viral responses help control viral replication, JHMV persists in white matter tracts resulting in chronic inflammation and demyelination. Microglia can secrete pro-inflammatory cytokines/chemokines that attract inflammatory T cells and myeloid cells that amplify white matter damage yet microglia may also restrict the severity of demyelination potentially by regulating expression of disease-associated genes in other myeloid cells (Mangale et al., 2020; Sariol et al., 2020; Syage et al., 2020). In addition, recent evidence supports a role for microglia in restricting the severity of demyelination as well as promoting remyelination through phagocytosis of myelin debris and secretion of factors that promote OPC recruitment/survival and maturation into mature myelin-producing oligodendrocytes (Lloyd et al., 2019; Sariol et al., 2020; Syage et al., 2020; Cheng et al., 2023).
AS: Conceptualization, Writing – original draft, Writing – review & editing. CP: Conceptualization, Writing – original draft, Writing – review & editing. YC: Writing – review & editing. VM: Writing – review & editing. KG: Writing – review & editing. TL: Funding acquisition, Project administration, Writing – original draft, Writing – review & editing.
The authors declare financial support was received for the research, authorship, and/or publication of this article. This work was supported by funding from the National Institutes of Health (NIH) grants R35NS116835 (TL) and R01NS083801 (KG).
We gratefully acknowledge the technical assistance of Cynthia Manlapaz and Kellie Fernandez with regards to assistance in experiments associated with studies related to this review.
The authors declare that the research was conducted in the absence of any commercial or financial relationships that could be construed as a potential conflict of interest.
All claims expressed in this article are solely those of the authors and do not necessarily represent those of their affiliated organizations, or those of the publisher, the editors and the reviewers. Any product that may be evaluated in this article, or claim that may be made by its manufacturer, is not guaranteed or endorsed by the publisher.
Acharya, M., Green, K., Allen, B., Najafi, A., Syage, A., Minasyan, H., et al. (2016). Elimination of microglia improves cognitive function following cranial irradiation. Sci. Rep. 6:31545. doi: 10.1038/srep31545
Albornoz, E., Amarilla, A., Modhiran, N., Parker, S., Li, X., Wijesundara, D., et al. (2022). SARS-CoV-2 drives NLRP3 inflammasome activation in human microglia through spike protein. Mol. Psychiatry 28, 2878–2893. doi: 10.1038/s41380-022-01831-0
Almutairi, M., Sivandzade, F., Albekairi, T., Alqahtani, F., and Cucullo, L. (2021). Neuroinflammation and Its Impact on the Pathogenesis of COVID-19. Front. Med. 8:745789. doi: 10.3389/fmed.2021.745789
Amor, S., McNamara, N., Gerrits, E., Marzin, M., Kooistra, S., Miron, V., et al. (2022). White matter microglia heterogeneity in the CNS. Acta Neuropathol. 143, 125–141.
Amruta, N., Ismael, S., Leist, S., Gressett, T., Srivastava, A., and Dinnon, K. III, et al. (2022). Mouse adapted SARS-CoV-2 (MA10) viral infection induces neuroinflammation in standard laboratory mice. Viruses 15:114. doi: 10.3390/v15010114
Arnaldez, F., O’Day, S., Drake, C., Fox, B., Fu, B., Urba, W., et al. (2020). The society for immunotherapy of cancer perspective on regulation of interleukin-6 signaling in COVID-19-related systemic inflammatory response. J. Immunother. Cancer 8:e000930. doi: 10.1136/jitc-2020-000930
Bayat, A., Azimi, H., Hassani Moghaddam, M., Ebrahimi, V., Fathi, M., Vakili, K., et al. (2022). COVID-19 causes neuronal degeneration and reduces neurogenesis in human hippocampus. Apoptosis 27, 852–868.
Bellon, M., Schweblin, C., Lambeng, N., Cherpillod, P., Vazquez, J., Lalive, P., et al. (2021). Cerebrospinal fluid features in severe acute respiratory syndrome coronavirus 2 (SARS-CoV-2) reverse transcription polymerase chain reaction (RT-PCR) positive patients. Clin. Infect. Dis. 73, e3102–e3105.
Bergmann, C., Lane, T., and Stohlman, S. (2006). Coronavirus infection of the central nervous system: Host-virus stand-off. Nat. Rev. Microbiol. 4, 121–132. doi: 10.1038/nrmicro1343
Bergmann, C., Parra, B., Hinton, D., Ramakrishna, C., Dowdell, K., and Stohlman, S. (2004). Perforin and gamma interferon-mediated control of coronavirus central nervous system infection by CD8 T cells in the absence of CD4 T cells. J. Virol. 78, 1739–1750. doi: 10.1128/jvi.78.4.1739-1750.2004
Brown, D., Soto, R., Yandamuri, S., Stone, C., Dickey, L., Gomes-Neto, J., et al. (2019). The microbiota protects from viral-induced neurologic damage through microglia-intrinsic TLR signaling. Elife 8:e47117. doi: 10.7554/eLife.47117
Bruce, K., Gorkhali, S., Given, K., Coates, A., Boyle, K., Macklin, W., et al. (2018). Lipoprotein lipase is a feature of alternatively-activated microglia and may facilitate lipid uptake in the CNS during demyelination. Front. Mol. Neurosci. 11:57. doi: 10.3389/fnmol.2018.00057
Bruttger, J., Karram, K., Wortge, S., Regen, T., Marini, F., Hoppmann, N., et al. (2015). Genetic cell ablation reveals clusters of local self-renewing microglia in the mammalian central nervous system. Immunity 43, 92–106. doi: 10.1016/j.immuni.2015.06.012
Chabas, D., Baranzini, S., Mitchell, D., Bernard, C., Rittling, S., Denhardt, D., et al. (2001). The influence of the proinflammatory cytokine, osteopontin, on autoimmune demyelinating disease. Science 294, 1731–1735.
Cheever, F., Daniels, J., Pappenheimer, A., and Bailey, O. (1949). A Murine Virus (JHM) Causing disseminated encephalomyelitis with extensive destruction of myelin: I. Isolation and biological properties of the virus. J. Exp. Med. 90, 181–194. doi: 10.1084/jem.90.3.181
Chen, L., Hoiland, R., Stukas, S., Wellington, C., and Sekhon, M. (2021). Assessing the importance of interleukin-6 in COVID-19. Lancet Respir. Med. 9:e13.
Chen, X., Zhao, B., Qu, Y., Chen, Y., Xiong, J., Feng, Y., et al. (2020). Detectable serum severe acute respiratory syndrome coronavirus 2 viral load (RNAemia) is closely correlated with drastically elevated interleukin 6 level in critically ill patients with coronavirus disease 2019. Clin. Infect. Dis. 71, 1937–1942. doi: 10.1093/cid/ciaa449
Cheng, Y., Javonillo, D., Pachow, C., Scarfone, V., Fernandez, K., Walsh, C., et al. (2023). Ablation of microglia following infection of the central nervous system with a neurotropic murine coronavirus infection leads to increased demyelination and impaired remyelination. J. Neuroimmunol. 381:578133. doi: 10.1016/j.jneuroim.2023.578133
Chousterman, B., Swirski, F., and Weber, G. (2017). Cytokine storm and sepsis disease pathogenesis. Semin. Immunopathol. 39, 517–528.
Dagher, N., Najafi, A., Kayala, K., Elmore, M., White, T., Medeiros, R., et al. (2015). Colony-stimulating factor 1 receptor inhibition prevents microglial plaque association and improves cognition in 3xTg-AD mice. J. Neuroinflamm. 12:139. doi: 10.1186/s12974-015-0366-9
Dufour, J., Dziejman, M., Liu, M., Leung, J., Lane, T., and Luster, A. (2002). IFN-γ-inducible protein 10 (IP-10; CXCL10)-deficient mice reveal a role for IP-10 in effector T cell generation and trafficking. J. Immunol. 168, 3195–3204. doi: 10.4049/jimmunol.168.7.3195
Durose, W., Shimizu, T., Li, J., Abe, M., Sakimura, K., Chetsawang, B., et al. (2019). Cathepsin C modulates myelin oligodendrocyte glycoprotein-induced experimental autoimmune encephalomyelitis. J. Neurochem. 148, 413–425. doi: 10.1111/jnc.14581
Elmore, M., Najafi, A., Koike, M., Dagher, N., Spangenberg, E., Rice, R., et al. (2014). Colony-stimulating factor 1 receptor signaling is necessary for microglia viability, unmasking a microglia progenitor cell in the adult brain. Neuron 82, 380–397. doi: 10.1016/j.neuron.2014.02.040
Fleming, J., Trousdale, M., el-Zaatari, F., Stohlman, S., and Weiner, L. (1986). Pathogenicity of antigenic variants of murine coronavirus JHM selected with monoclonal antibodies. J. Virol. 58, 869–875.
Funk, K., and Klein, R. (2019). CSF1R antagonism limits local restimulation of antiviral CD8(+) T cells during viral encephalitis. J. Neuroinflamm. 16:22. doi: 10.1186/s12974-019-1397-4
Garber, C., Vasek, M., Vollmer, L., Sun, T., Jiang, X., and Klein, R. (2018). Astrocytes decrease adult neurogenesis during virus-induced memory dysfunction via IL-1. Nat. Immunol. 19, 151–161. doi: 10.1038/s41590-017-0021-y
Ginhoux, F., Greter, M., Leboeuf, M., Nandi, S., See, P., Gokhan, S., et al. (2010). Fate mapping analysis reveals that adult microglia derive from primitive macrophages. Science 330, 841–845. doi: 10.1126/science.1194637
Glass, W., Hickey, M., Hardison, J., Liu, M., Manning, J., and Lane, T. (2004). Antibody targeting of the CC chemokine ligand 5 results in diminished leukocyte infiltration into the central nervous system and reduced neurologic disease in a viral model of multiple sclerosis. J. Immunol. 172, 4018–4025. doi: 10.4049/jimmunol.172.7.4018
Glass, W., Liu, M., Kuziel, W., and Lane, T. (2001). Reduced macrophage infiltration and demyelination in mice lacking the chemokine receptor CCR5 following infection with a neurotropic coronavirus. Virology 288, 8–17. doi: 10.1006/viro.2001.1050
Gorbalenya, A., Enjuanes, L., Ziebuhr, J., and Snijder, E. (2006). Nidovirales: Evolving the largest RNA virus genome. Virus Res. 117, 17–37.
Green, K., Crapser, J., and Hohsfield, L. (2020). To kill a microglia: A case for CSF1R inhibitors. Trends Immunol. 41, 771–784. doi: 10.1016/j.it.2020.07.001
Hammond, T., Dufort, C., Dissing-Olesen, L., Giera, S., Young, A., Wysoker, A., et al. (2019). Single-cell RNA sequencing of microglia throughout the mouse lifespan and in the injured brain reveals complex cell-state changes. Immunity 50, 253–271.e6. doi: 10.1016/j.immuni.2018.11.004
Han, J., Harris, R., and Zhang, X. (2017). An updated assessment of microglia depletion: Current concepts and future directions. Mol. Brain 10:25. doi: 10.1186/s13041-017-0307-x
Han, J., Zhu, K., Zhang, X., and Harris, R. (2019). Enforced microglial depletion and repopulation as a promising strategy for the treatment of neurological disorders. Glia 67, 217–231. doi: 10.1002/glia.23529
Helms, J., Kremer, S., Merdji, H., Clere-Jehl, R., Schenck, M., Kummerlen, C., et al. (2020). Neurologic features in severe SARS-CoV-2 infection. N. Engl. J. Med. 382, 2268–2270.
Hendrickx, D., van Scheppingen, J., van der Poel, M., Bossers, K., Schuurman, K., van Eden, C., et al. (2017). Gene expression profiling of multiple sclerosis pathology identifies early patterns of demyelination surrounding chronic active lesions. Front. Immunol. 8:1810. doi: 10.3389/fimmu.2017.01810
Hlavica, M., Delparente, A., Good, A., Good, N., Plattner, P., Seyedsadr, M., et al. (2017). Intrathecal insulin-like growth factor 1 but not insulin enhances myelin repair in young and aged rats. Neurosci. Lett. 648, 41–46.
Holmes, K., and Lai, M. (1996). “Coronaviridae: The viruses and their replication,” in Fields virology, 3rd Edn, eds B. Fields, D. Knipe, and P. Howley (Philadelphia: Lippincott-Raven Publishers), 1075–1094.
Huang, C., Wang, Y., Li, X., Ren, L., Zhao, J., Hu, Y., et al. (2020). Clinical features of patients infected with 2019 novel coronavirus in Wuhan, China. Lancet 395, 497–506.
Hume, D., Irvine, K., and Pridans, C. (2019). The mononuclear phagocyte system: The relationship between monocytes and macrophages. Trends Immunol. 40, 98–112.
Hwang, M., and Bergmann, C. (2018). Alpha/beta interferon (IFN-alpha/beta) signaling in astrocytes mediates protection against viral encephalomyelitis and regulates IFN-gamma-dependent responses. J. Virol. 92, e01901–17. doi: 10.1128/JVI.01901-17
Hwang, M., and Bergmann, C. (2019). Intercellular communication is key for protective IFNalpha/beta signaling during viral central nervous system infection. Viral Immunol. 32, 1–6. doi: 10.1089/vim.2018.0101
Hwang, M., and Bergmann, C. (2020). Neuronal ablation of alpha/beta interferon (IFN-alpha/beta) signaling exacerbates central nervous system viral dissemination and impairs IFN-gamma responsiveness in microglia/macrophages. J. Virol. 94, e422–e420. doi: 10.1128/JVI.00422-20
Iadecola, C., Anrather, J., and Kamel, H. (2020). Effects of COVID-19 on the nervous system. Cell 183, 16–27.e1.
Ireland, D., Stohlman, S., Hinton, D., Atkinson, R., and Bergmann, C. (2008). Type I interferons are essential in controlling neurotropic coronavirus infection irrespective of functional CD8 T cells. J. Virol. 82, 300–310. doi: 10.1128/JVI.01794-07
Jeong, G., Lyu, J., Kim, K., Chung, Y., Yoon, G., Lee, S., et al. (2022). SARS-CoV-2 infection of microglia elicits proinflammatory activation and apoptotic cell death. Microbiol. Spectr. 10:e0109122. doi: 10.1128/spectrum.01091-22
Kim, T., and Perlman, S. (2005). Viral expression of CCL2 is sufficient to induce demyelination in RAG1-/- mice infected with a neurotropic coronavirus. J. Virol. 79, 7113–7120. doi: 10.1128/JVI.79.11.7113-7120.2005
Klein, R., Soung, A., Sissoko, C., Nordvig, A., Canoll, P., Mariani, M., et al. (2021). COVID-19 induces neuroinflammation and loss of hippocampal neurogenesis. Res. Sq. 145, 4193–4201. doi: 10.21203/rs.3.rs-1031824/v1
Kong, X., Gong, Z., Zhang, L., Sun, X., Ou, Z., Xu, B., et al. (2019). JAK2/STAT3 signaling mediates IL-6-inhibited neurogenesis of neural stem cells through DNA demethylation/methylation. Brain Behav. Immun. 79, 159–173. doi: 10.1016/j.bbi.2019.01.027
Krasemann, S., Madore, C., Cialic, R., Baufeld, C., Calcagno, N., El Fatimy, R., et al. (2017). The TREM2-APOE pathway drives the transcriptional phenotype of dysfunctional microglia in neurodegenerative diseases. Immunity 47, 566–581.e9. doi: 10.1016/j.immuni.2017.08.008
Lane, T., and Buchmeier, M. (1997). Murine coronavirus fection: A paradigm for virus-induced demyelinating disease. Trends Microbiol. 5, 9–14.
Lane, T., Asensio, V., Yu, N., Paoletti, A., Campbell, I., and Buchmeier, M. (1998). Dynamic regulation of alpha- and beta-chemokine expression in the central nervous system during mouse hepatitis virus-induced demyelinating disease. J. Immunol. 160, 970–978.
Li, Q., and Barres, B. (2018). Microglia and macrophages in brain homeostasis and disease. Nat. Rev. Immunol. 18, 225–242.
Li, Q., Cheng, Z., Zhou, L., Darmanis, S., Neff, N., Okamoto, J., et al. (2019). Developmental heterogeneity of microglia and brain myeloid cells revealed by deep single-cell RNA sequencing. Neuron 101, 207–223.e10. doi: 10.1016/j.neuron.2018.12.006
Liu, M., Armstrong, D., Hamilton, T., and Lane, T. (2001a). Expression of Mig (Monokine Induced by Interferon-) is important in T lymphocyte recruitment and host defense following viral infection of the central nervous system. J. Immunol. 166, 1790–1795. doi: 10.4049/jimmunol.166.3.1790
Liu, M., Chen, B., Oertel, P., Buchmeier, M., Hamilton, T., Armstrong, D., et al. (2001b). The CXC chemokines IP-10 and Mig are essential in host defense following infection with a neurotropic coronavirus. Adv. Exp. Med. Biol. 494, 323–327. doi: 10.1007/978-1-4615-1325-4_48
Liu, M., Keirstead, H., and Lane, T. (2001c). Neutralization of the chemokine CXCL10 reduces inflammatory cell invasion and demyelination and improves neurological function in a viral model of multiple sclerosis. J. Immunol. 167, 4091–4097. doi: 10.4049/jimmunol.167.7.4091
Liu, Y., Ying, Y., Li, Y., Eyo, U., Chen, T., Zheng, J., et al. (2019). Neuronal network activity controls microglial process surveillance in awake mice via norepinephrine signaling. Nat. Neurosci. 22, 1771–1781. doi: 10.1038/s41593-019-0511-3
Lloyd, A., and Miron, V. (2019). The pro-remyelination properties of microglia in the central nervous system. Nat. Rev. Neurol. 15, 447–458. doi: 10.1038/s41582-019-0184-2
Lloyd, A., Davies, C., Holloway, R., Labrak, Y., Ireland, G., Carradori, D., et al. (2019). Central nervous system regeneration is driven by microglia necroptosis and repopulation. Nat. Neurosci. 22, 1046–1052. doi: 10.1038/s41593-019-0418-z
Lou, J., Movassaghi, M., Gordy, D., Olson, M., Zhang, T., Khurana, M., et al. (2021). Neuropathology of COVID-19 (neuro-COVID): Clinicopathological update. Free Neuropathol. 2, 2–2. doi: 10.17879/freeneuropathology-2021-2993
Ma, J., Tanaka, K., Shimizu, T., Bernard, C., Kakita, A., Takahashi, H., et al. (2011). Microglial cystatin F expression is a sensitive indicator for ongoing demyelination with concurrent remyelination. J. Neurosci. Res. 89, 639–649. doi: 10.1002/jnr.22567
Magro, C., Mulvey, J., Berlin, D., Nuovo, G., Salvatore, S., Harp, J., et al. (2020). Complement associated microvascular injury and thrombosis in the pathogenesis of severe COVID-19 infection: A report of five cases. Transl. Res. 220, 1–13. doi: 10.1016/j.trsl.2020.04.007
Mangale, V., Syage, A., Ekiz, H., Skinner, D., Cheng, Y., Stone, C., et al. (2020). Microglia influence host defense, disease, and repair following murine coronavirus infection of the central nervous system. Glia 68, 2345–2360. doi: 10.1002/glia.23844
Marten, N., Stohlman, S., and Bergmann, C. (2000). Role of viral persistence in retaining CD8(+) T cells within the central nervous system. J. Virol. 74, 7903–7910.
Masuda, T., Sankowski, R., Staszewski, O., Bottcher, C., Amann, L., Sagar, et al. (2019). Spatial and temporal heterogeneity of mouse and human microglia at single-cell resolution. Nature 566, 388–392.
Mathys, H., Davila-Velderrain, J., Peng, Z., Gao, F., Mohammadi, S., Young, J., et al. (2019). Single-cell transcriptomic analysis of Alzheimer’s disease. Nature 570, 332–337.
Matschke, J., Lutgehetmann, M., Hagel, C., Sperhake, J., Schroder, A., Edler, C., et al. (2020). Neuropathology of patients with COVID-19 in Germany: A post-mortem case series. Lancet Neurol. 19, 919–929. doi: 10.1016/S1474-4422(20)30308-2
Maury, A., Lyoubi, A., Peiffer-Smadja, N., de Broucker, T., and Meppiel, E. (2021). Neurological manifestations associated with SARS-CoV-2 and other coronaviruses: A narrative review for clinicians. Rev. Neurol. 177, 51–64.
McIntosh, K., Dees, J., Becker, W., Kapikian, A., and Chanock, R. (1967). Recovery in tracheal organ cultures of novel viruses from patients with respiratory disease. Proc. Natl. Acad. Sci. U.S.A. 57, 933–940. doi: 10.1073/pnas.57.4.933
McNamara, N., Munro, D., Bestard-Cuche, N., Uyeda, A., Bogie, J., Hoffmann, A., et al. (2023). Microglia regulate central nervous system myelin growth and integrity. Nature 613, 120–129.
Miyamoto, A., Wake, H., Ishikawa, A., Eto, K., Shibata, K., Murakoshi, H., et al. (2016). Microglia contact induces synapse formation in developing somatosensory cortex. Nat. Commun. 7:12540. doi: 10.1038/ncomms12540
Munoz-Fontela, C., Dowling, W., Funnell, S., Gsell, P., Riveros-Balta, A., Albrecht, R., et al. (2020). Animal models for COVID-19. Nature 586, 509–515.
Nimmerjahn, A., Kirchhoff, F., and Helmchen, F. (2005). Resting microglial cells are highly dynamic surveillants of brain parenchyma in vivo. Science 308:1314.
Olivarria, G., Cheng, Y., Furman, S., Pachow, C., Hohsfield, L., Smith-Geater, C., et al. (2022). Microglia do not restrict SARS-CoV-2 replication following infection of the central nervous system of K18-human ACE2 transgenic mice. J. Virol. 96:e0196921.
Perlman, S., Lane, T., and Buchmeier, M. (1999). “Coronaviruses: Hepatitis, peritonitis, and central nervous system disease,” in Effects of microbes on the immune system, Vol. 1, eds M. Cunningham and R. Fujinami (Philadelphia: Lippincott Williams & Wilkins), 331–348.
Pewe, L., and Perlman, S. (2002). Cutting edge: CD8 T cell-mediated demyelination is IFN-gamma dependent in mice infected with a neurotropic coronavirus. J. Immunol. 168, 1547–1551. doi: 10.4049/jimmunol.168.4.1547
Pewe, L., Haring, J., and Perlman, S. (2002). CD4 T-cell-mediated demyelination is increased in the absence of gamma interferon in mice infected with mouse hepatitis virus. J. Virol. 76, 7329–7333. doi: 10.1128/jvi.76.14.7329-7333.2002
Phares, T., Stohlman, S., Hinton, D., and Bergmann, C. (2013). Astrocyte-derived CXCL10 drives accumulation of antibody-secreting cells in the central nervous system during viral encephalomyelitis. J. Virol. 87, 3382–3392. doi: 10.1128/JVI.03307-12
Pleasure, S., Green, A., and Josephson, S. (2020). The spectrum of neurologic disease in the severe acute respiratory syndrome coronavirus 2 pandemic infection: Neurologists move to the frontlines. JAMA Neurol. 77, 679–680. doi: 10.1001/jamaneurol.2020.1065
Prinz, M., Jung, S., and Priller, J. (2019). Microglia biology: One century of evolving concepts. Cell 179, 292–311.
Proust, A., Queval, C., Harvey, R., Adams, L., Bennett, M., and Wilkinson, R. (2023). Differential effects of SARS-CoV-2 variants on central nervous system cells and blood-brain barrier functions. J. Neuroinflamm. 20:184. doi: 10.1186/s12974-023-02861-3
Ragab, D., Salah Eldin, H., Taeimah, M., Khattab, R., and Salem, R. (2020). The COVID-19 cytokine storm; What We Know So Far. Front. Immunol. 11:1446. doi: 10.3389/fimmu.2020.01446
Reu, P., Khosravi, A., Bernard, S., Mold, J., Salehpour, M., Alkass, K., et al. (2017). The lifespan and turnover of microglia in the human brain. Cell Rep. 20, 779–784.
Salter Michael, W., and Beggs, S. (2014). Sublime microglia: Expanding roles for the guardians of the CNS. Cell 158, 15–24. doi: 10.1016/j.cell.2014.06.008
Sanchez, J., DePaula-Silva, A., Doty, D., Truong, A., Libbey, J., and Fujinami, R. (2019). Microglial cell depletion is fatal with low level picornavirus infection of the central nervous system. J. Neurovirol. 25, 415–421. doi: 10.1007/s13365-019-00740-3
Sariol, A., Mackin, S., Allred, M., Ma, C., Zhou, Y., Zhang, Q., et al. (2020). Microglia depletion exacerbates demyelination and impairs remyelination in a neurotropic coronavirus infection. Proc. Natl. Acad. Sci. U.S.A. 117, 24464–24474. doi: 10.1073/pnas.2007814117
Schwabenland, M., Salie, H., Tanevski, J., Killmer, S., Lago, M., Schlaak, A., et al. (2021). Deep spatial profiling of human COVID-19 brains reveals neuroinflammation with distinct microanatomical microglia-T-cell interactions. Immunity 54, 1594–1610.e11. doi: 10.1016/j.immuni.2021.06.002
Seitz, S., Clarke, P., and Tyler, K. (2018). Pharmacologic depletion of microglia increases viral load in the brain and enhances mortality in murine models of flavivirus-induced encephalitis. J. Virol. 92, e00525–18. doi: 10.1128/JVI.00525-18
Shimizu, T., Wisessmith, W., Li, J., Abe, M., Sakimura, K., Chetsawang, B., et al. (2017). The balance between cathepsin C and cystatin F controls remyelination in the brain of Plp1-overexpressing mouse, a chronic demyelinating disease model. Glia 65, 917–930. doi: 10.1002/glia.23134
Solomon, M., and Liang, C. (2022). Human coronaviruses: The emergence of SARS-CoV-2 and management of COVID-19. Virus Res. 319:198882.
Song, E., Zhang, C., Israelow, B., Lu-Culligan, A., Prado, A., Skriabine, S., et al. (2021). Neuroinvasion of SARS-CoV-2 in human and mouse brain. J. Exp. Med. 218:e20202135.
Spangenberg, E., Severson, P., Hohsfield, L., Crapser, J., Zhang, J., Burton, E., et al. (2019). Sustained microglial depletion with CSF1R inhibitor impairs parenchymal plaque development in an Alzheimer’s disease model. Nat. Commun. 10:3758. doi: 10.1038/s41467-019-11674-z
Stein, J., Kaes, M., Smola, S., and Schulz-Schaeffer, W. (2023). Neuropathology in COVID-19 autopsies is defined by microglial activation and lesions of the white matter with emphasis in cerebellar and brain stem areas. Front. Neurol. 14:1229641. doi: 10.3389/fneur.2023.1229641
Stowell, R., Sipe, G., Dawes, R., Batchelor, H., Lordy, K., Whitelaw, B., et al. (2019). Noradrenergic signaling in the wakeful state inhibits microglial surveillance and synaptic plasticity in the mouse visual cortex. Nat. Neurosci. 22, 1782–1792.
Strafella, C., Caputo, V., Termine, A., Barati, S., Caltagirone, C., Giardina, E., et al. (2020). Investigation of genetic variations of IL6 and IL6R as potential prognostic and pharmacogenetics biomarkers: Implications for COVID-19 and neuroinflammatory disorders. Life 10:351. doi: 10.3390/life10120351
Strong, M. (2023). SARS-CoV-2, aging, and Post-COVID-19 neurodegeneration. J. Neurochem. 165, 115–130.
Syage, A., Ekiz, H., Skinner, D., Stone, C., O’Connell, R., and Lane, T. (2020). Single-cell RNA sequencing reveals the diversity of the immunological landscape following central nervous system infection by a murine coronavirus. J. Virol. 94, e01295–20. doi: 10.1128/JVI.01295-20
Thaweethai, T., Jolley, S., Karlson, E., Levitan, E., Levy, B., McComsey, G., et al. (2023). Development of a definition of postacute sequelae of SARS-CoV-2 infection. JAMA 329, 1934–1946.
Tyrrell, D., and Myint, S. (1996). “Coronaviruses,” in Medical microbiology, 4th Edn, ed. S. Baron (Galveston, TX: University of Texas Medical Branch at Galveston).
Ulrich, J., and Holtzman, D. (2016). TREM2 function in Alzheimer’s disease and neurodegeneration. ACS Chem. Neurosci. 7, 420–427.
Waltl, I., Kaufer, C., Gerhauser, I., Chhatbar, C., Ghita, L., Kalinke, U., et al. (2018). Microglia have a protective role in viral encephalitis-induced seizure development and hippocampal damage. Brain Behav. Immun. 74, 186–204. doi: 10.1016/j.bbi.2018.09.006
Wang, F., Hinton, D., Gilmore, W., Trousdale, M., and Fleming, J. (1992). Sequential infection of glial cells by the murine hepatitis virus JHM strain (MHV-4) leads to a characteristic distribution of demyelination. Lab. Invest. 66, 744–754.
Wang, Y., Grunewald, M., and Perlman, S. (2020). Coronaviruses: An updated overview of their replication and pathogenesis. Methods Mol. Biol. 2203, 1–29.
Wei, Z., Liang, K., and Shetty, A. (2023). Role of microglia, decreased neurogenesis and oligodendrocyte depletion in long COVID-mediated brain impairments. Aging Dis [Epub ahead of print]. doi: 10.14336/AD.2023.10918
Wheeler, D., Sariol, A., Meyerholz, D., and Perlman, S. (2018). Microglia are required for protection against lethal coronavirus encephalitis in mice. J. Clin. Invest. 128, 931–943. doi: 10.1172/JCI97229
Wlodarczyk, A., Holtman, I., Krueger, M., Yogev, N., Bruttger, J., Khorooshi, R., et al. (2017). A novel microglial subset plays a key role in myelinogenesis in developing brain. EMBO J. 36, 3292–3308. doi: 10.15252/embj.201696056
Wu, G., and Perlman, S. (1999). Macrophage infiltration, but not apoptosis, is correlated with immune-mediated demyelination following murine infection with a neurotropic coronavirus. J. Virol. 73, 8771–8780.
Wu, G., Dandekar, A., Pewe, L., and Perlman, S. (2000). CD4 and CD8 T cells have redundant but not identical roles in virus-induced demyelination. J. Immunol. 165, 2278–2286.
Ye, P., Li, L., Richards, R., DiAugustine, R., and D’Ercole, A. (2002). Myelination is altered in insulin-like growth factor-I null mutant mice. J. Neurosci. 22, 6041–6051. doi: 10.1523/JNEUROSCI.22-14-06041.2002
Ye, Q., Wang, B., and Mao, J. (2020). The pathogenesis and treatment of the;Cytokine Storm’ in COVID-19. J. Infect. 80, 607–613.
Yin, J., Valin, K., Dixon, M., and Leavenworth, J. (2017). The role of microglia and macrophages in CNS homeostasis, Autoimmunity, and Cancer. J. Immunol. Res. 2017:5150678.
Keywords: microglia, coronavirus, neuroinflammation, demyelination, remyelination
Citation: Syage A, Pachow C, Cheng Y, Mangale V, Green KN and Lane TE (2023) Microglia influence immune responses and restrict neurologic disease in response to central nervous system infection by a neurotropic murine coronavirus. Front. Cell. Neurosci. 17:1291255. doi: 10.3389/fncel.2023.1291255
Received: 08 September 2023; Accepted: 09 November 2023;
Published: 30 November 2023.
Edited by:
Sara Salinas, Institut National de la Santé et de la Recherche Médicale (INSERM), FranceReviewed by:
Fiona Limanaqi, University of Milan, ItalyCopyright © 2023 Syage, Pachow, Cheng, Mangale, Green and Lane. This is an open-access article distributed under the terms of the Creative Commons Attribution License (CC BY). The use, distribution or reproduction in other forums is permitted, provided the original author(s) and the copyright owner(s) are credited and that the original publication in this journal is cited, in accordance with accepted academic practice. No use, distribution or reproduction is permitted which does not comply with these terms.
*Correspondence: Thomas E. Lane, dGxhbmVAdWNpLmVkdQ==
†These authors have contributed equally to this work
Disclaimer: All claims expressed in this article are solely those of the authors and do not necessarily represent those of their affiliated organizations, or those of the publisher, the editors and the reviewers. Any product that may be evaluated in this article or claim that may be made by its manufacturer is not guaranteed or endorsed by the publisher.
Research integrity at Frontiers
Learn more about the work of our research integrity team to safeguard the quality of each article we publish.