- 1Department of Neurology, Nanjing Hospital of Chinese Medicine Affiliated to Nanjing University of Chinese Medicine, Nanjing University of Chinese Medicine, Nanjing, China
- 2School of Medicine & Holistic Integrative Medicine, Nanjing University of Chinese Medicine, Nanjing, China
Parkinson’s disease (PD) could be viewed as a proteinopathy caused by changes in lipids, whereby modifications in lipid metabolism may lead to protein alterations, such as the accumulation of alpha-synuclein (α-syn), ultimately resulting in neurodegeneration. Although the loss of dopaminergic neurons in the substantia nigra is the major clinical manifestation of PD, the etiology of it is largely unknown. Increasing evidence has highlighted the important role of lipids in the pathophysiology of PD. Sphingosine-1-phosphate (S1P), a signaling lipid, has been suggested to have a potential association with the advancement and worsening of PD. Therefore, better understanding the mechanisms and regulatory proteins is of high interest. Most interestingly, S1P appears to be an important target to offers a new strategy for the diagnosis and treatment of PD. In this review, we first introduce the basic situation of S1P structure, function and regulation, with a special focus on the several pathways. We then briefly describe the regulation of S1P signaling pathway on cells and make a special focused on the cell growth, proliferation and apoptosis, etc. Finally, we discuss the function of S1P as potential therapeutic target to improve the clinical symptoms of PD, and even prevent the progression of the PD. In the context of PD, the functions of S1P modulators have been extensively elucidated. In conclusion, S1P modulators represent a novel and promising therapeutic principle and therapeutic method for PD. However, more research is required before these drugs can be considered as a standard treatment option for PD.
1 Introduction
Parkinson’s disease (PD), described by James Parkinson in his 1817 “An essay on the shaking palsy” (Samii et al., 2004), is characterized by the typical symptoms include tremor, rigidity and postural instability, and bradykinesia, associated with non-motor symptoms such as cognitive impairment, sense of smell, gastrointestinal symptoms due to the degenerative loss of dopaminergic neurons and the formation of Lewy bodies (Pajares et al., 2020). With the global population aging at an accelerating pace and the expected average life span continuing to rise, neurodegenerative disease like PD is gaining attention from the scientific community. According to statistics from the Global Burden of Disease Study, the number of PD case will rise to 13 million in 2040 that is reaching epidemic proportions (GBD 2016 Parkinson’s Disease Collaborators, 2018). The treatment of PD is focused on the drug therapy including levodopa, catechol-o-methyltransferase inhibitors (COMT), monoamine oxidase type B inhibitor (MAOB), etc. (Ogawa et al., 2022). At present, levodopa is the most effective means for the motor symptoms of PD. However, levodopa, a natural stimulant, cannot cross blood brain barrier. And beyond that, Surgical treatment (deep brain stimulation) is also an effective way to improve the symptoms of PD patients (Tao and Liang, 2015). Nevertheless, these all methods cannot prevent, slow, or reverse the progress of the degenerative death of DA neuron as well as cannot effectively alleviate symptoms. Therefore, the realization is growing that novel PD targets and novel therapies are of upmost importance (Van Bulck et al., 2019).
Sphingosine-1-phosphate (S1P), a bioactive lysophospholipid produced by sphingosine kinases, plays a critical role in the regulation of cell growth, differentiation, and apoptosis through binding to a family of five G protein-coupled receptors (S1PR1-5) (Al Gadban et al., 2012; Ye et al., 2022). S1P exhibit a serious of biological effects, including oxidative stress, autophagy, anti-inflammatory, anti-apoptosis and improved mitochondrial function, and has a neuroprotective effect on PD (Sun et al., 2022). In addition, S1P is essential for vascularization and brain development (Van Echten-Deckert et al., 2014). Simultaneously, S1P can protect against dopamine depletion, neuroinflammation, and PD-associated symptoms by uncoupling the S1P1R from Gi-protein (Hait and Maiti, 2017; Lee et al., 2022). Most impressively, some studies have illustrated that S1P might regulate microglial phagocytosis (Xue et al., 2022). Hence, developing further understanding about the role of S1P in PD development and progression is of great significance and urgency to promote the timely and accurately diagnosis of PD.
2 Search strategy
We utilized PubMed and Web of science platforms for English language articles on this research. Meanwhile, two Chinese databases (China National Knowledge Infrastructure Database and Wanfang Database) will be searched for Chinese language articles. “Parkinson disease,” “S1P,” “Sphingosine-1-phosphate,” “modulators” as the key words used in database and many other keywords relevant to every section. We also reviewed bookson Parkinson’s disease or movement disorders published in the same period. We reviewed selected references from articles retrieved by the initial search. The contents of this article are based on reviewed published work, our judgment, consultation with experts in the area of PD.
3 S1P pathway overview
Sphingolipids are considered to serve principally as structural components of cell membranes conferring unique signaling functions. S1P (molecular weight: 379.47 g/mol), also known as sphing-4-enine-1-phosphate, is classified as a small molecular weight lipid and bioactive sphingolipid metabolite. S1P is a compound with potent bioactive actions in sphingolipid metabolism, the calcium signaling pathway, and neuroactive ligand-receptor interaction (Atkinson et al., 2017). Furthermore, S1P can be generated from the phosphorylation of sphingosine and catalyzed by sphingosine kinases (SphK1 and SphK2) in blood platelet, erythrocyte and endotheliocyte (Figure 1). In cells, S1P is recognized by a family of G-protein coupled receptors (GPCRs, S1PR1-5), degraded by the S1P lyase (S1PL) and reversibly conversion to sphingosine through S1P phosphatases (S1PP) (Mitrofanova et al., 2019; Hii et al., 2020). S1PL is an intracellular enzyme, which is a key enzyme to regulate the degradation pathway of sphingomyelin, as well as an important pathway of sphingomyelin metabolism (Choi and Saba, 2019). Abnormal function of S1PL can lead to accumulation of sphingomyelin in S1P and its upstream, leading to organ dysfunction (Tang et al., 2021). In contrast to the SphKs activity, S1PP activity is catalyzing the dephosphorylation of S1P into sphingosine (Figure 1). After binding with S1P, S1PRs can conjugate with different heterotrimer G protein subunits to activate downstream cell signaling pathways, such as PI3K/Akt (Liu and Tie, 2019) and MAPK (Liu et al., 2022) signaling pathway, so as to play the biological role of S1P. The S1P receptor is a G-protein-coupled cell membrane receptor that specifically binds to S1P, leading to the regulation of various biological effects mediated by S1P. It exhibits a high affinity for S1P and plays a crucial role in mediating important biological functions. S1PR1-3 is widely distributed in the body and highly expressed in lymphocytes, astrocytes, oligodendrocytes, etc. Alternatively, S1PR1-3 mainly regulates immunity, nerve cell migration, embryonic development of cardiovascular system and nervous system, and also plays a certain role in endothelial barrier function and angiogenesis. In addition, S1PR5 is mainly expressed in oligodendrocytes, astrocytes and other cells of the nervous system, which mainly regulates the function of oligodendrocytes and participates in the migration of natural killer cells (Chun et al., 2021).
4 The regulation of S1P signaling pathway on cells
Sphingosine-1-phosphate is produced by SphKs in blood platelet, erythrocyte and endotheliocyte and regulated by lyase and phosphohydrolase. For instance, the activation of SphK1 by abundant cytokines can cause the translocation of SphK1 from the cytoplasm to the cytomembrane. This translocation ultimately leads to the production and release of S1P (Jolly et al., 2005; Nayak et al., 2010). Currently, our understanding of the biological significance of S1P primarily revolves around the following two aspects: (1) it functions as a secondary messenger within cells and directly impacts downstream targets, leading to the regulation of diverse biological effects; (2) it is released into the extracellular environment through autocrine and paracrine mechanisms and travels in the bloodstream by binding to apolipoprotein. This apolipoprotein then interacts with specific S1P receptors, which are G protein-coupled, resulting in the regulation of various biological effects downstream. SphKs, widely existed in mammals, are the key enzymes to catalyze sphingosine to S1P. SphKs is a protein with an apparent molecular mass of 49 kDa, which originally purified from rat kidney (Obinata and Hla, 2012). There are two subtypes in the body (SphK1 and SphK2) and encoded by genes located on chromosome 17 (17q25.2) and chromosome 19 (19q13.2), respectively (Kihara et al., 2006). Interestingly, there are two SphK isozymes that differ in their temporal, spatial distribution and different biochemical properties. SphK1 was found to be located predominantly in the cytoplasm, while SphK2 is mainly exist in the nucleus (Hait and Maiti, 2017). SphK2 compensates for SphK1 in keeping tissue S1P in the SphK1 deficient state. SphK1 and SphK2 are show different functions in apoptosis, immune cell responses, inflammation, and cell survival, etc. (Li et al., 2020).
4.1 Effects of S1P signaling pathway on cell growth and proliferation
The overexpression of Sphk1 can induce cell proliferation through promoting the G1 phase transition to S phase and reducing doubling time in the cell cycle to regulate DNA synthesis (Olivera et al., 1999; Igarashi et al., 2003). After blocking Sphk1, cell cycle can be lengthened or arrested in G1 phase, pro-apoptotic proteins can be up-regulation to activate intracellular apoptotic pathways. In addition, SphK1 not only plays an important role in cell cycle but also blood vessel formation in the nervous system and inflammatory response. In contrast, Sphk2, a nuclear protein, can suppresses cell proliferation, induces cell cycle arrest and enhances apoptosis thus result in inhibiting DNA synthesis (Igarashi et al., 2003; Weigert et al., 2010). The nuclear localization sequence mutations of Sphk2 eliminates its inhibitory effect on cellular growth. SphKs inhibition could be contribution to the alteration of the S1P/ceramide inhibitor. S1P levels in the brain are mainly regulated via Sphk1 actions, and a dysregulation of this S1P/Sphk1 signaling has been reconditioned in numerous CNS pathologies (Ayub et al., 2021).
4.2 Effects of S1P signaling pathway on cell apoptosis
Apoptosis, which is characterized by morphological features, is the primary cause of pathophysiological cell death and regulated by a serious of pro-apoptotic, anti-apoptotic, and cell growth signals (Suzuki et al., 2013) in physiologic and pathologic conditions (Elmore, 2007). In addition, it plays an important role in development, aging, and defense mechanism via immune system. Apoptosis is the primary target for anti-cancer research through two pathways: intrinsic mitochondrial-dependent pathway and the extrinsic death receptor-mediated pathway. Among of them, caspases are the dominant enzymes and synchronized apoptosis events act as a cysteine-dependent aspartate-directed proteases, such as caspase-8 mediates extrinsic pathway (Ashkenazi, 2015), while caspase-3 mediates intrinsic pathway (Loison et al., 2014).
S1P acts as an intracellular second messenger to inhibit apoptosis by binding to the receptor S1PRs. The balance between ceramide and S1P, act as “sphingolipid rheostat “, plays a vital role in cell apoptosis (Harvald et al., 2015). S1P is produced via the expression of SphK1 and prevents apoptosis by activating signaling pathways involved in cell survival, including inositol-independent Ca2+ mobilization, ERK1/2, AKT and nuclear factors (Xia et al., 1999; Spiegel and Milstien, 2003; Figure 2; Table 1). S1P also inhibits apoptosis-related signal cascades (Figure 2; Table 1), such as cytochrome c release from mitochondria, activation of caspase, and activation of c-Jun N-terminal protein kinase (JNK) (Spiegel and Milstien, 2003). Interestingly, SphK2, the other isoform of the kinase, has the effect of pro-apoptotic via regulating cell cycle arrest and cell death, thus counteracting the positive effect of SphK1 on cell division. Furthermore, SphK2 is redirected to endoplasmic reticulum in the state of starvation (Igarashi et al., 2003). The re-localization of SphK2 illustrated that the pro-apoptotic role of it is due to the production of ceramide from external sources of sphingoid bases through the combined effects of SphK2 and lipid phosphohydrolases in the endoplasmic reticulum (Ding et al., 2007). In turn, up-regulation of SphK2 increases the conversion of sphingosine to ceramide, while up-regulation of SphK1 reduces it (Maceyka et al., 2005). Interestingly, S1P which is distributed in different intracellular pools might play different roles in metabolic and signaling pathways. The fact that mutation of the Bcl-2 homology-3 (BH3) primary reasons suppression of the SphK2 induced apoptosis. Ceramide, the converted product of S1P, coordinates programmed cell death via two pathways (type I and type II). Type I programmed cell death, apoptosis, is induced by elevated levels of ceramide, by activation of caspase-9 via inactivation of protein kinase B (PKB), activation of Bad via Ras and protein phosphatase 2A (PP2A), and activation of protein kinase Cζ, etc. (Ruvolo et al., 1998).
4.3 Effects of S1P signaling pathway on cell migration
The S1P signaling pathway plays a crucial role in regulating cell migration. S1P is a bioactive lipid that acts as a potent extracellular signaling molecule, binding to specific cell surface receptors and initiating intracellular signaling cascades (Rykowski et al., 2023). Especially, it is a complex process that plays a vital role in multiple physiological function, such as regeneration, angiogenesis, cell mediated immune responses, as well as tumor progression (Chi et al., 2022). Cell migration is a complex process that can be influenced by a variety of factors, which can roughly be divided into the following two categories: the chemical factors and the mechanical factors. The influence of S1P on cell migration is mainly manifested in the following aspects: promotion of cell migration, modulation of cytoskeletal dynamics (Donati and Bruni, 2006), regulation of cell adhesion, involvement in chemotaxis, and regulation of barrier function.
Cell migration can be regulated by S1P through blinding to S1P receptor, which is closely related to cell movement. Simultaneously, it is regulated by platelet-derived growth factor (PDGF), which is an effective activator of SphKs. Interestingly, PDGF signaling is fundamental to the development of brain vasculature and involved in variety neurodegenerative disease, especially in PD (Li S. Y. et al., 2022). PDGF tyrosine kinase receptors transactivate S1P pathway by stimulating the production of SphK1 and S1P, which is essential for PDGF to regulate cell movement. Once activated inside the cell, the generated S1P can regulate cell movement using “inside-out signaling” through its cell surface receptors. Nevertheless, the effect of S1P on migration is complex and may depend on the S1PRs expressed on the cell surface. Nicholas et al. (2017) found that the blinding of S1PR1 and S1PR3 with S1P many stimulate cell migration, whereas S1PR2 has adverse action.
Sphingosine-1-phosphate acts as a signaling molecule that binds to specific receptors on the cell surface, known as S1P receptors. Upon binding, the bioactive sphingolipid S1P induces significant reorganization of the cytoskeleton in diverse cell systems, primarily through a set of distinct cell surface receptors known as S1P receptors. Notably, S1P-induced alterations in cell morphology and/or movement through cytoskeletal remodeling play a functional role in the cell-specific biological effects exerted by S1P in diverse cellular systems, primarily mediated by a group of distinct cell surface receptors called S1P receptors (Donati and Bruni, 2006). S1P signaling affects cell adhesion molecules, such as integrins, which mediate cell-substrate interactions. It can enhance integrin activation, leading to increased cell adhesion to the extracellular matrix and facilitating cell migration (Mendelson et al., 2014). Remodeling plays a functional role in inducing chemotaxis of primary fibroblasts by the sphingolipid S1P, primarily mediated by S1P receptors (Gil et al., 2010; Li Y. et al., 2022). In line with the migratory reaction, S1P induced the elongation of lamellipodia at the outer edge of human fibroblast cells and reorganized the cytoskeleton. In fibroblasts, the chemotactic response mediated by S1P was impaired upon downregulation of decapentaplegic family member 3 (Smad3) (Huang et al., 2022). Therefore, it suggests that the downstream signaling pathways involving Smad3 are necessary for S1P to properly function in maintaining barrier function (Zhang and Wang, 2023).
4.4 Effects of S1P signaling pathway on immune responses
The innate immune system is the basis of host survival and overall health, the first line of defense against initial invading organisms and environmental challenges, and the fundamental for maintaining tissue homeostasis (Baumruker and Prieschl, 2002; Liaskou et al., 2012). The overall survival of the host relies on its ability to identify and alleviate appropriate defense signals to eliminate infectious microorganisms (Liaskou et al., 2012). The human brain organ is considered an immune privileged organ due to the blood-brain barrier (Tan et al., 2020). These findings illustrate that innate and adaptive immune responses could be contributed to the central nervous system (CNS) in neurodegenerative disease, especially PD (Harms et al., 2021). The increased risk of PD is associated with some autoimmune diseases, suggesting that autoimmunity have an effect on the pathogenesis of PD (Tan et al., 2020). The balance and downstream signal regulation of S1P and sphingosine also play an important role in the immune system (Baumruker and Prieschl, 2002). Especially in the immune response, high-level sphingosine significantly inhibits the synthesis of leukotriene and the production of cytokine in response to antigen-induced immunoglobulin in mast cells (Prieschl et al., 1999). S1P is a bioactive lipid molecule that binds to S1P receptors, and triggers a series of intracellular signaling events. These events ultimately affect the behavior and function of immune cells. One of the primary effects of S1P signaling on immune responses is the regulation of lymphocyte trafficking (Tsai and Han, 2016). Lymphocytes, such as T cells and B cells, are key players in the immune system and are involved in various immune processes. S1P acts as a chemoattractant, guiding lymphocytes out of lymphoid organs, such as the thymus and lymph nodes, and into the bloodstream. This process, called lymphocyte egress, is crucial for immune cell distribution and surveillance throughout the body.
Moreover, S1P signaling influences the migration of immune cells to specific sites of inflammation or infection (Tian et al., 2022). When immune cells encounter tissue damage or infection, S1P gradients are established, attracting immune cells toward the affected area. This helps in the recruitment of immune cells to sites of injury or infection, enabling an effective immune response. S1P signaling also modulates the function of various immune cell types. For example, S1P has been shown to regulate the activation and proliferation of T cells (Romani et al., 2018). It can enhance T cell activation by promoting the secretion of cytokines and increasing the expression of cell surface molecules involved in immune cell communication. S1P can also regulate the development and function of regulatory T cells, a specialized subset of T cells that play a crucial role in maintaining immune tolerance and preventing excessive immune responses.
Additionally, S1P signaling affects other immune cell populations, including dendritic cells (Zehra Okus et al., 2022), macrophages (Gong et al., 2023), and natural killer cells (Fettel et al., 2019). S1P can modulate the maturation, migration, and cytokine production of these cells, thereby influencing their ability to initiate and regulate immune responses (Fettel et al., 2019). Understanding the precise mechanisms underlying S1P signaling in immune responses is an active area of research. Further studies are needed to elucidate the downstream signaling pathways activated by S1P receptors and their interplay with other immune signaling molecules. Additionally, investigating the therapeutic potential of targeting the S1P signaling pathway in immune-related disorders, such as autoimmune diseases and inflammatory conditions, holds promise for the development of novel treatment strategies.
In conclusion, the S1P signaling pathway exerts profound effects on immune responses by regulating lymphocyte trafficking, immune cell migration, and immune cell function. Continued research in this field will contribute to our understanding of immune regulation and potentially lead to new therapeutic interventions for immune-related disorders.
5 Role of S1P in the central nervous system
Ceramide is a central hub for sphingolipid metabolism, and it could be catabolized and phosphorylated into S1P by a family of ceramidases which differ in their pH optimum and subcellular localization (Don-Doncow et al., 2019). In adult human brain, ceramidase is a primary glycoprotein and is primarily expressed in neurons (Don-Doncow et al., 2019). Interestingly, all S1PRs except S1PR4 are expressed in the CNS: oligodendrocytes mainly express S1PR1 and S1PR5, astrocytes S1PR1 and S1PR3, while microglia express S1PR1-S1PR3. A study conducted by Mizugishi group (Mizugishi et al., 2005) consistently showed that mice lacking both Sphk1 and Sphk2 had severe abnormalities in neurogenesis and angiogenesis, resulting in impaired brain development. S1P signaling is crucial during development of the nervous system and in neural progenitor cells by signaling a family of G protein-coupled receptors (Callihan et al., 2018). Based on all of this discussion, S1P is not only involved in vascularization and organ development in the periphery, but also essential for development of the brain (Mizugishi et al., 2005). Furthermore, S1P has anti-apoptotic and pro-growth effects among neural development and mediated by signaling from the S1P1 receptor (Don-Doncow et al., 2019). The activation of S1PRs via S1P contributes to a serious of physiologic processes which involved in neuronal plasticity including myelination, neurogenesis and neuroprotection (Chatzikonstantinou et al., 2021). S1P is more effective than fibroblast growth factor (FGF) in stimulating neurogenesis. Meanwhile, S1P mainly participate in the adjustion of growth cone formation, neurite extension and retraction by binding to S1PRs. In neurons, S1PR1 expression can enhance the neurite extension, while S1PR2 and S1PR5 inhibits neurite extension. Perhaps most interesting of all, S1P has a dual action because of the role of S1P in oligodendrocyte morphology. It enhances retraction function by the regulation of S1PR5, while it promotes process extension via the modulation of S1PR1. In addition, S1P can also act as a chemical attractant to promote the migration of neural stem and progenitor cells (NSPC) to the site of injury and promote the translocation of NSPC to the destination. However, the activation of S1PR5 on oligodendrocytes inhibits their migration. According to the above discussion, S1P can regulate the movement of NSPC and participate in the growth and development of central nervous system cells.
5.1 Implication for the role of S1P in brain-derived neurotrophic factors
Brain-derived neurotrophic growth factor (BDNF), one of the most important members of the neurotrophic factor family, was first isolated in 1982 from pig brain by German neurobiologist BARDE (Sandhya et al., 2013). BDNF exists as a homodimer and synthesized as a 32 kDa precursor molecule pro-BDNF, which is post-translationally cleaved to generate the mature and biologically active form of BDNF (m-BDNF, ∼13 kDa) (Barreda Tomás et al., 2020). At present, the biological activity of pro-BDNF is still controversial, but it is certain that m-BDNF plays an important role in maintaining the physiological function of the nervous system. There is a dynamic balance between different forms of BDNF, and the ratio of pro-BDNF to m-BDNF is different in specific stages and regions of brain development. During early development, the ratio of pro-BDNF to m-BDNF is considered to be an important factor in regulating brain function, while m-BDNF plays a crucial role in neuroprotection and synaptic plasticity after maturation. More to the point, BDNF is the second neurotrophin after nerve growth factor (NGF), and is critical for neuronal growth, survival of neurons and phenotypic expression of neuronal cells (Yoshii and Constantine-Paton, 2010). It is regarded as a general modulator of neurotransmitter release, including the release of γ-aminobutyric acid (GABA) which is the primary inhibitory neurotransmitter in the CNS.
BDNF has two binding receptors, high affinity receptor tyrosine kinase receptor B (tyrosine kinase receptor B) and low affinity neurotrophin receptor (p75 nerve growth factor receptor, p75 NTR). After binding with high-affinity tyrosine kinase receptor, tropomyosin-related kinase B (TrkB), receptor dimerization and intracellular tyrosine residue autophosphorylation were activated. Phosphorylated TrkB can promote neuronal survival, increase synaptic plasticity, and play a neurotrophic role via phospholipase C-γ/protein kinase C (PLC-γ/PKC), mitogen-activated protein kinase (MAPK), RAS/ERK, JAK/STAT and phosphatidylinositol-3-kinase (PI3K)/protein kinase B (PKB/AKT) pathways (Sandhya et al., 2013). PI3K/AKT signaling, stimulated by BDNF is crucial for proliferation, protection and survival of neuronal cells (Yamada et al., 2001; Sandhya et al., 2013). BDNF stimulation of ERK5/MEF pathway is essential for neuronal survival (Shalizi et al., 2003) and various cellular processes including growth (Sugimoto et al., 2001), differentiation (Yin et al., 2010), protection of neuronal cells (Szatmari et al., 2007). The activation of PLC also leads to intracellular calcium release and CREB phosphorylation (Finkbeiner et al., 1997), neuronal migration and increase synaptic plasticity (Zuccato et al., 2001). BDNF also binds to p75 NTR, albeit with a low affinity, and participate in the induction of some cell apoptosis and pathological injury process. Among the neurotrophins, BDNF has been become as a major regulator of synaptic plasticity, survival of neurons and neuronal differentiation, and also as a novel and valuable target for drug development in neurological disorders.
Sphingosine-1-phosphate enhances the expression of neurofilament and promote neuronal survival which induced by NGF, while inhibit SphK activity to eliminate neuronal cell differentiation (Edsall et al., 1997). The axon elongation of neuronal cells and dorsal root ganglion neuron induced by NGF depends on the activity of S1P (Toman et al., 2004). While, NGF can promote axon elongation to activate S1P through stimulates Sphk1 activity through TrkA receptor. S1P induced the expression and release of BDNF in neuronal cells while promoting the growth and proliferation of astrocytes (Yamagata et al., 2003). In addition, BDNF increases the expression of SphK1 in neuroblastoma cell lines, leading to the production and secretion of S1P (Murakami et al., 2011). Generally, it can be seen that there may be interaction and expression between S1P and BDNF. Upon binding to GPI-anchored coreceptors known as glial cell line-derived neurotrophic factor (GDNF) family alpha (GFRα), GDNF triggers the activation of the RET receptor tyrosine kinase, subsequently initiating the activation of various downstream signaling pathways (Murakami et al., 2011). The GDNF receptor RET induces the expression of the SPHK1 gene, and there are indications that SPHK1 plays a part in GDNF-induced differentiation. Therefore, the SPHK/S1P signaling pathways activated by neurotrophic factors play a crucial role in neural differentiation (Murakami et al., 2007). Elucidation of these mechanisms might also be helpful for the understanding of the clinical PD.
5.2 Implication for the role of S1P in neuroregulation
In the CNS, S1P can regulate the release of neurotransmitters and the excitability of neurons, and this regulation may be achieved through blinding to S1PR. Interestingly, S1P facilitated glutamate secretion in hippocampal neurons in a dose-dependent manner, indicating its potential involvement in the regulation of synaptic transmission by S1P/S1PR3 pathway (Kanno and Nishizaki, 2011; Sukocheva, 2018), while inhibited glutamatergic nerve transmission through S1PR1 (Sim-Selley et al., 2009). S1PR2-deficient mice showed a significant increase in excitatory postsynaptic enhancement, leading to spontaneous seizures in these animals. Consistent with these observations, S1P can enhance the excitability of small-diameter sensory neurons (Li et al., 2015). According to the above, the all evidence suggests that S1P may also be involved in the regulation of neuronal excitability.
5.3 Implication for the role of S1P in neuroprotection
Sphingosine-1-phosphate plays an important role in the brain as a neuromodulator and a neuroprotector. By far the greatest risk factor for neurodegenerative diseases including PD is aging, and mitochondria have been thought play an important role in aging by mitochondrial dysfunction, oxidative stress, and cell apoptosis. Mitochondria are double-membrane organelles responsible for oxidative energy metabolism in eukaryotic cells. In addition, it plays an important role in the maintenance of Ca2+ homeostasis (Witte et al., 2014; Figure 2; Table 1) and participates in the control generation of reactive oxygen species (ROS). SphK2, expressed in nucleus and mitochondria, is responsible for the local production of S1P in the mitochondria (Strub et al., 2011). Interestingly, S1P produced in the mitochondria own high affinity and specificity to prohibitin 2 (PHB2) which is a key factor in the normal development, morphogenesis and function of mitochondria as a highly conserved protein (Aoki et al., 2016). In mitochondria from SphK2-null mice, the interaction between PHB2 and subunit (I and IV) of cytochrome-c oxidase is greatly reduced (Strub et al., 2011). Most likely, S1P is necessary for the biogenesis of autophagosomal precursors via mitochondrial DNA replication and transcription (Shen et al., 2014).
Oxidative stress can activate apoptosis pathway by mitochondrial-dependent and non-mitochondrial-dependent ways. However, S1P inhibits the apoptosis pathway activated by mitochondrial-dependent method. Initial evidence implicating the effect of oxidative stress on cell fate is bidirectional. It will cause the activation of sphingomyelinase and shift the “sphingolipid rheostat” toward ceramide accumulation when the production rate of ROS exceeds the oxidation capacity of cells, thus triggering cell apoptosis (Petrache and Berdyshev, 2016). On the other hand, SphK1 can be activated by low-level oxidative stress which upregulated the level of S1P, thus facilitating cell survival (Motyl et al., 2021). S1P play a crucial role in cellular damage induced by oxidative stress and validated via human dopaminergic neuronal cell (SH-SY5Y) (Pyszko and Strosznajder, 2014). More interestingly, only S1P produced by SphK2 can cause cell apoptosis, suggesting the biological function of S1P in subcellular localization (Hao et al., 2020). Furthermore, the generate of ceramide mediated sphingomyelinase can be inhibited by S1P (Czubowicz et al., 2019).
Sphingosine-1-phosphate signal pathway can regulate autophagy which plays a crucial role in the cells survival such as neurons (Mitroi et al., 2017). Bioactive sphingolipids, such as ceramide and S1P, play a vital role in cellular apoptosis, senescence and growth arrest. Initial evidence implicating that the dynamic equilibrium between the formation, catalyzed by SphKs, and degradation, catalyzed by S1P phosphatases (SGPPs) and sphingosine phosphate lyase 1 (SGPL1), of S1P, is crucial to S1P-related autophagic processes (Hao et al., 2020). It is illustrated that ceramide can help to facilitate autophagy-associated cell death and can be blocked by S1P by transactivation of mTOR via S1PR1 and S1PR3 (Taniguchi et al., 2012). Also, similar results have been shown in SphK2, S1P-induced apoptosis can be prevented through the pretreatment with SphK2 inhibitors (Hao et al., 2020). Therefore, S1P-induced autophagy in intracellular can protect cells from death during nutrient deficiency and has the characteristics of apoptosis by reabsorption function (Lavieu et al., 2006). Supporting this concept, S1P-induced autophagy in intracellular is a cellular defense mechanism, which play a vital role in cell survival.
6 The relationship between S1P and Parkinson’s disease
6.1 Gene expression profiles and data preprocessing in the metabolism of S1P
The gene expression data was obtained from the Gene Expression Omnibus (GEO) database, comprising GSE8397 and GSE99039. One-way ANOVA test was performed on all selected samples to filter genes with a p-value less than 0.05. Then, the differentially expressed genes were converted to their gene IDs using omicshare gene ID tool. Additionally, the genes obtained will be subjected to metabolic pathway (KEGG) enrichment analysis by omicshare KEGG enrichment analysis tool. In our findings, we discovered a substantial connection between the development of PD and the metabolic processes of lipids (Figures 3A, D). What’s even more astonishing is the finding of an increased quantity of genes exhibiting a heightened association with S1P metabolism among differentially expressed genes. Dynamic heat maps and bar charts provide a visualization of the differentially expressed genes which related with S1P metabolism between healthy and PD patients (Figures 3B, C).
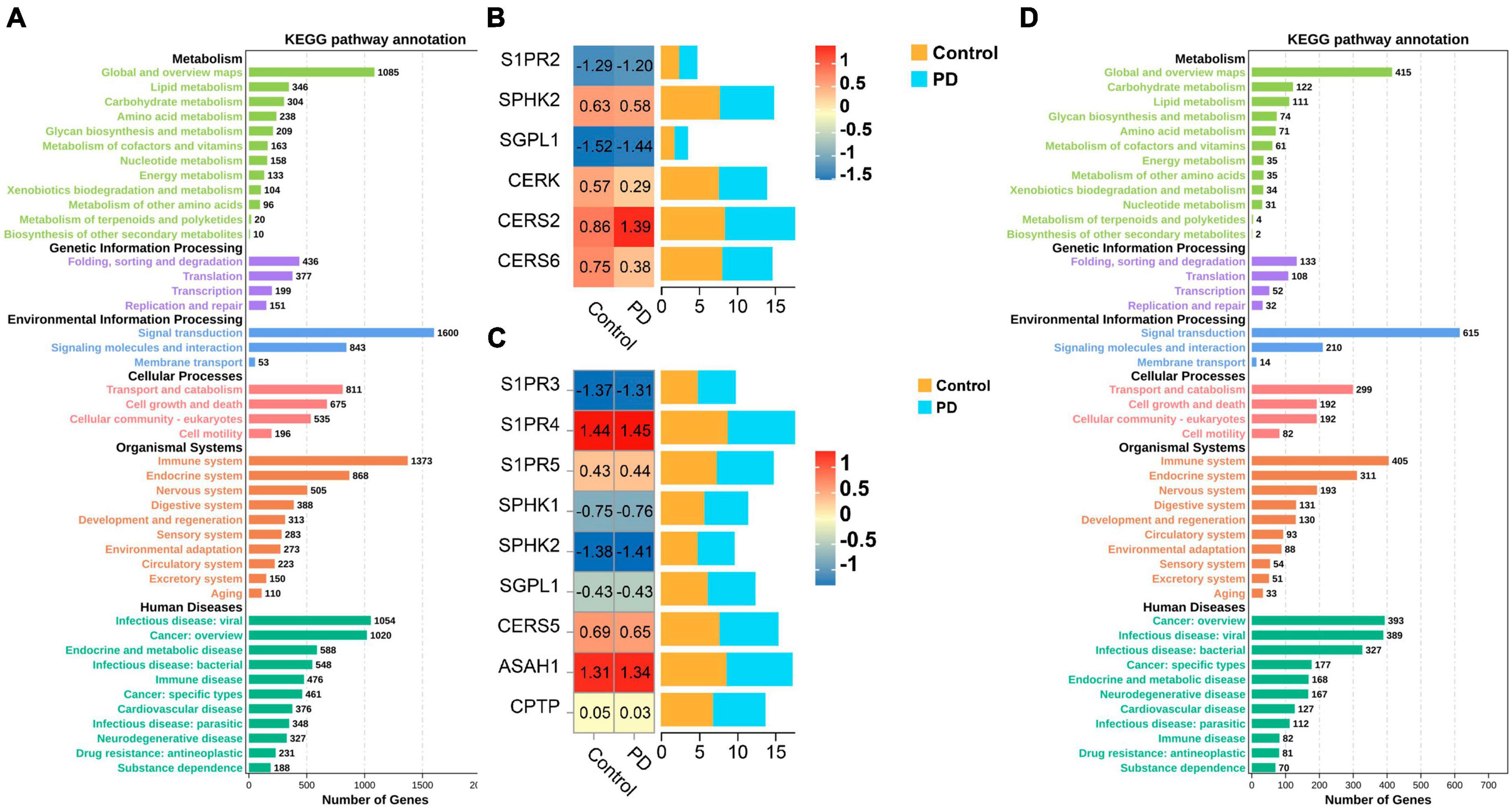
Figure 3. (A,D) The metabolic pathway (KEGG) enrichment analysis based on the GEO database which related with the development of PD; (B,C) Dynamic heat maps and bar charts provide a visualization of the differentially expressed genes which related with S1P metabolism between healthy and PD patients.
6.2 Crosstalk between S1P and the pathogenesis of PD
Parkinson’s disease is a neurodegenerative disease and the main pathological feature is the progressive degeneration or loss of dopaminergic neurons in the substantia nigra pars compacta (SNpc), which leads a lack of dopamine in the striatum (Samii et al., 2004). In addition to the loss of nigrostriatal dopamine neurons, accumulation of alpha-synuclein protein (α-syn, targeting dopamine neurons in the midbrain) in the form of Lewy bodies (LBs), leading the ability to synthesize dopamine reduce, is an important feature in PD (Su et al., 2021; Mahapatra et al., 2022). The function of S1P in the degradation of α-syn is to facilitate the breakdown and clearance of α-syn protein, potentially preventing its accumulation and the formation of aggregates through activation of the mTOR pathway (Mitroi et al., 2017). Autophagic flux is blocked at initial stages upon SGPL1 deficiency (Zhang et al., 2017). On the basis of these results, the balance between the acetylcholine loop and dopamine system is broken, leading to the motor symptoms and non-motor symptoms seen in patients (West et al., 2022). At present, the etiology and pathogenesis of PD is only partly understood. Notably, several observations suggest that oxidative stress, mitochondrial dysfunction, inflammation and protein misfolding are involved in the pathogenesis of PD (Wang et al., 2013; Li et al., 2021), which can be reversed by FTY720 (a structural sphingosine analog and S1PR modulator) (Zhang and Wang, 2020).
Increased oxidative stress, induced by the high basal rates of oxidative phosphorylation leading to the mitochondrial dysfunction in nigral neurons. The relationship between mitochondrial dysfunction and PD was first found by Schapira et al. (1990). Interestingly, decreased complex I activity in PD substantia nigra tissue appears to be specific to PD (Macdonald et al., 2018). Complex I, the largest mitochondrial complex, is a type-I NADH dehydrogenase which contain at least 44 subunits (Macdonald et al., 2018). The complex couples the transfer of two electrons from NADH to ubiquinone and translocates of four protons across the membrane (Schulte et al., 2019). Collectively, impairment of mitochondrial function result in decreased ATP levels, increased levels of reactive oxygen species (ROS), impaired calcium buffering capacity, elevated apoptosis, impaired mitochondrial membrane potential (MMP) and impaired calcium buffering capacity (Wang et al., 2019) which was related with age-associated muscle dysfunction (Gill et al., 2019). One particularly intriguing observation is that the activity of complex (I, II, III, or IV) remained unchanged before and after treatment (Shults et al., 1995). At present, data from the literature show that not only are mitochondrial deficiencies present before drug treatment (Punzi et al., 2018), but also that current drug therapy do not inhibit mitochondrial damage and resolve abnormal mitochondrial functions (Murthy et al., 2022). These findings suggest that targeting the S1P pathway might be therapeutically important for PD patients through regulating mitochondrial function.
Within the realm of translational medicine, it has become apparent to us that mitochondrial dysfunctions are the key factors in the mitochondrial biogenesis caused by the transcription-factor dysregulation, leading to the adverse effect on cellular bioenergetics. In particular, peroxisome proliferator-activated receptor-gamma coactivator-1α (PGC-1α), a coactivator of several transcription factors, play an important role in mitochondrial biogenesis (Xu et al., 2023). According to previous research findings, the overexpression of PGC-1α caused dopamine depletion is relative to low expression levels of paired-like homeodomain 3 (PITX3), increased susceptibility to MPTP (Corona and Duchen, 2015; Zhong et al., 2023) and reduced a-synuclein levels, whereas deficiency of PGC-1α is related with the propensity of a-synuclein to oligomerize (Punzi et al., 2018). On a functional site, the expression of PGC-1α reduced by the inhibition of Sphk2, down-regulated in the brain of PD patients (Sivasubramanian et al., 2015). Overall, therefore S1P/PGC-1α appears to play a neuroprotective role in PD. S1P modulates mitochondrial dysfunction by interacted with mitochondrial proteins, including Bak protein, and is also relevant to the release of cytochrome C (Pyszko and Strosznajder, 2014). The electron transport chain in the respiratory chain is blocked because of the deficiency of cytochrome C which is the important part of the mitochondrial respiratory chain, leading to the decrease of ATP synthesis and the excessive production of ROS caused by incomplete oxidation. It is well established that increased levels of mutations and deletions of mitochondrial DNA (mtDNA) are a common cause of PD patients (Siibak et al., 2017). Human mitochondrial DNA, consisting of 16,569 base pairs, is a double stranded molecule that is 15∼16 kb in size (Sun et al., 2018). MtDNA encodes for 13 proteins along with the 22 tRNAs and 2 rRNA (Hauser and Hastings, 2013). Another intriguing possibility that could underlie the mtDNA deletions are specific to nigral neurons which increase their contribute to in PD (Hauser and Hastings, 2013). However, the usage of S1P could obviously upregulate mitochondrial DNA replication and transcription, increase mitochondrial mass, and elevated adenosine triphosphate synthesis (Shen et al., 2014), leading to the improvement of PD patients.
Increasing evidence suggests that the accumulation of misfolded α-syn play an important role in neurodegenerative diseases including PD. To be gratified, the secreted of α-syn can be facilitated by inhibited SphKs, while α-syn can reduce the activity and protein expression level of SphK1, leading to molecular structure changes and cell death (Murphy et al., 2014). There is also some form of antagonism between S1P and its downstream proteins by α-syn. α-Syn can block the activity of essential proteins in downstream signal transduction of S1PR1, including BDNF/Akt kinase signal pathway (Safarian et al., 2015; Kang et al., 2017). In addition, the interaction between α-syn and S1P also influence mitochondrial homeostasis and protect DA neurons in MPTP/MPP + model.
6.3 S1P receptor modulators and the functions of it in PD
The most direct evidence in Schwedhelm et al. (2021) research, the level of serum S1P in patients with PD was lower than that in healthy controls, and decreased gradually with the increase of the severity of the disease by measuring the level of serum S1P in human body for the first time. This study demonstrated the correlation between S1P level and the severity of PD patients in human body. At present, S1P receptor modulators are mainly focused on FTY720, SEW2871 and CS0777, etc. FTY720 (2-Amino-2-[2-(4-octylphenyl) ethyl]propane-1,3-diol hydrochloride, Fingolimod, chemical structure in Figure 4), was the first S1P receptor modulator to be approved by United States Food and Drug Administration in 2010 for the treatment of multiple sclerosis, followed by siponimod, ozanimod, and ponesimod (Figure 4; Cohan et al., 2022). Emerging evidence have illustrated that Fingolimod can exert neuroprotective action to battle against PD (Pépin et al., 2020). The structure of fingolimod is similar to sphingosine and phosphorylated to fingolimod-P (an S1P analog) by SphK1/2 (Roy et al., 2021). Similar to S1P, fingolimod-P can bind to the S1P receptor, leading to the S1P receptor degraded (Roy et al., 2021). One of the most interesting findings, fingolimod promotes the levels of BDNF which is a crucial neurotrophic factor for dopaminergic neurons (Vidal-Martinez et al., 2019). In association with PD, BDNF is a major neurotrophin for SNc dopaminergic neurons, thereby using an agent to increase BDNF expression is a potential method is a promising PD therapeutic (Vidal-Martinez et al., 2019). Meanwhile, fingolimod significantly improved behavioral deficits, decreased the loss of dopaminergic neurons and promoted dopamine release by neuroinflammation pathway (Yao et al., 2019). Similar to the FTY720, CS0777 (1-{5-[(3R)-3-amino-4-hydroxy -3-methylbutyl]-1-methyl-1H-pyrrol-2-yl}-4-(4-methylphenyl) butan-1-one), a selective S1P1 modulator, is phosphorylated to an active S1P analog (Yao et al., 2019) (CS0777-P, Figure 4). SEW2871 (5-(4-phenyl-5-trifluoromethylthiophen-2-yl)-3-(3-trifluorometh ylphenyl)-1,2,4-oxadiazole, Figure 4), a selective agonist to subtype 1 of S1PRs, can protect dopaminergic neurons and improve motor deficits in PD (St-Cyr Giguère et al., 2017). Naturally, the other S1P modulator and the functions in PD were summarized in Table 2. Compared to FTY720, most other S1PR modulators provide safety advantages and fewer side effects because of the selective mode blinding to S1PR. However, the pharmacological and rational theory for the drug development of PD remain inadequate. Up to now, there is no targets of S1P reported in PD. Meanwhile it also shows that the S1P receptor modulators are an ideal therapeutic agent for PD.
7 Conclusion
Plasma S1P, which can improve PD development and progression, levels are reduced in PD patients compared to healthy controls. Meanwhile, S1P can protect against dopamine depletion, neuroinflammation, and PD-associated symptoms by uncoupling the S1P1R from Gi-protein. Most impressively, some studies have illustrated that S1P might regulate microglial phagocytosis. Thus, S1P receptor modulators are promising target in the therapy of PD. Hence, developing further understanding about the role of S1P in PD development and progression is of great significance and urgency to promote the timely and accurately diagnosis of PD. The drug development which focused on the S1P and S1P receptor modulators have implications for improving the diagnosis and therapy of the PD patients.
Author contributions
WW: Funding acquisition, Writing—original draft, Writing—review and editing. YZ: Conceptualization, Supervision, Writing—original draft, Writing—review and editing. GZ: Conceptualization, Funding acquisition, Supervision, Visualization, Writing—original draft, Writing—review and editing.
Funding
The author(s) declare financial support was received for the research, authorship, and/or publication of this article. This work was funded by the National Natural Science Foundation of China (82204375, 82003933), the Science and Technology Development Planning Project of Traditional Chinese Medicine of Jiangsu Province of China (QN202103), the Natural Science Foundation of Nanjing University of Chinese Medicine (XZR2021046), the Nanjing Medical Science and Technology Development Project (YKK20167), and the Nanjing Youth Talent Training Plan of TCM (ZYQ20006).
Conflict of interest
The authors declare that the research was conducted in the absence of any commercial or financial relationships that could be construed as a potential conflict of interest.
Publisher’s note
All claims expressed in this article are solely those of the authors and do not necessarily represent those of their affiliated organizations, or those of the publisher, the editors and the reviewers. Any product that may be evaluated in this article, or claim that may be made by its manufacturer, is not guaranteed or endorsed by the publisher.
References
Al Gadban, M. M., German, J., Truman, J. P., Soodavar, F., Riemer, E. C., Twal, W. O., et al. (2012). Lack of nitric oxide synthases increases lipoprotein immune complex deposition in the aorta and elevates plasma sphingolipid levels in lupus. Cell. Immunol. 276, 42–51. doi: 10.1016/j.cellimm.2012.03.007
Alexander, S. P., Benson, H. E., Faccenda, E., Pawson, A. J., Sharman, J. L., Spedding, M., et al. (2013). The concise guide to pharmacology 2013/14: G protein-coupled receptors. Br. J. Pharmacol. 170, 1459–1581.
Anwar, M., and Mehta, D. (2020). Post-translational modifications of S1PR1 and endothelial barrier regulation. Biochim. Biophys. Acta Mol. Cell Biol. Lipids 1865:158760. doi: 10.1016/j.bbalip.2020.158760
Aoki, M., Aoki, H., Ramanathan, R., Hait, N. C., and Takabe, K. (2016). Sphingosine-1-Phosphate signaling in immune cells and inflammation: Roles and therapeutic potential. Mediat. Inflamm. 2016:8606878.
Ashkenazi, A. (2015). Targeting the extrinsic apoptotic pathway in cancer: Lessons learned and future directions. J. Clin. Investig. 125, 487–489. doi: 10.1172/JCI80420
Atkinson, D., Nikodinovic Glumac, J., Asselbergh, B., Ermanoska, B., Blocquel, D., Steiner, R., et al. (2017). Sphingosine 1-phosphate lyase deficiency causes Charcot-Marie-Tooth neuropathy. Neurology 88, 533–542.
Ayub, M., Jin, H. K., and Bae, J. S. (2021). Novelty of sphingolipids in the central nervous system physiology and disease: Focusing on the sphingolipid hypothesis of neuroinflammation and neurodegeneration. Int. J. Mol. Sci. 22:7353. doi: 10.3390/ijms22147353
Barreda Tomás, F. J., Turko, P., Heilmann, H., Trimbuch, T., Yanagawa, Y., Vida, I., et al. (2020). BDNF expression in cortical GABAergic interneurons. Int. J. Mol. Sci. 21:1567.
Baumruker, T., and Prieschl, E. E. (2002). Sphingolipids and the regulation of the immune response. Semin. Immunol. 14, 57–63.
Callihan, P., Alqinyah, M., and Hooks, S. B. (2018). Sphingosine-1-phosphate (S1P) signaling in neural progenitors. Methods Mol. Biol. 1697, 141–151.
Chatzikonstantinou, S., Poulidou, V., Arnaoutoglou, M., Kazis, D., Heliopoulos, I., Grigoriadis, N., et al. (2021). Signaling through the S1P-S1PR axis in the gut, the immune and the central nervous system in multiple sclerosis: Implication for pathogenesis and treatment. Cells 10:3217. doi: 10.3390/cells10113217
Chen, L., Li, L., Song, Y., and Lv, T. (2021). Blocking SphK1/S1P/S1PR1 signaling pathway alleviates lung injury caused by sepsis in acute ethanol intoxication mice. Inflammation 44, 2170–2179. doi: 10.1007/s10753-021-01490-3
Chi, J., Wang, M., Chen, J., Hu, L., Chen, Z., Backman, L. J., et al. (2022). Topographic orientation of scaffolds for tissue regeneration: Recent advances in biomaterial design and applications. Biomimetics 7:131. doi: 10.3390/biomimetics7030131
Choi, Y. J., and Saba, J. D. (2019). Sphingosine phosphate lyase insufficiency syndrome (SPLIS): A novel inborn error of sphingolipid metabolism. Adv. Biol. Regul. 71, 128–140.
Chun, J., Giovannoni, G., and Hunter, S. F. (2021). Sphingosine 1-phosphate receptor modulator therapy for multiple sclerosis: Differential downstream receptor signalling and clinical profile effects. Drugs 81, 207–231. doi: 10.1007/s40265-020-01431-8
Cohan, S. L., Benedict, R. H. B., Cree, B. A. C., DeLuca, J., Hua, L. H., and Chun, J. (2022). The two sides of siponimod: Evidence for brain and immune mechanisms in multiple sclerosis. CNS Drugs 36, 703–719. doi: 10.1007/s40263-022-00927-z
Corona, J. C., and Duchen, M. R. (2015). Impaired mitochondrial homeostasis and neurodegeneration: Towards new therapeutic targets? J. Bioenerget. Biomembr. 47, 89–99. doi: 10.1007/s10863-014-9576-6
Czubowicz, K., Jęśko, H., Wencel, P., Lukiw, W. J., and Strosznajder, R. P. (2019). The role of ceramide and sphingosine-1-phosphate in alzheimer’s disease and other neurodegenerative disorders. Mol. Neurobiol. 56, 5436–5455.
D’Ambrosio, D., Freedman, M. S., and Prinz, J. (2016). Ponesimod, a selective S1P1 receptor modulator: A potential treatment for multiple sclerosis and other immune-mediated diseases. Therapeut. Adv. Chronic Dis. 7, 18–33. doi: 10.1177/2040622315617354
Dertschnig, S., Gergely, P., Finke, J., Schanz, U., Holler, E., Holtick, U., et al. (2023). Mocravimod, a selective sphingosine-1-phosphate receptor modulator, in allogeneic hematopoietic stem cell transplantation for malignancy. Transpl. Cell. Therapy 29, 41.e1–41.e9. doi: 10.1016/j.jtct.2022.10.029
Dhar, T. G., Xiao, H. Y., Xie, J., Lehman-McKeeman, L. D., Wu, D. R., Dabros, M., et al. (2016). Identification and preclinical pharmacology of BMS-986104: A differentiated S1P1 receptor modulator in clinical trials. ACS Med. Chem. Lett. 7, 283–288.
Ding, G., Sonoda, H., Yu, H., Kajimoto, T., Goparaju, S. K., Jahangeer, S., et al. (2007). Protein kinase D-mediated phosphorylation and nuclear export of sphingosine kinase 2. J. Biol. Chem. 282, 27493–27502. doi: 10.1074/jbc.M701641200
Donati, C., and Bruni, P. (2006). Sphingosine 1-phosphate regulates cytoskeleton dynamics: Implications in its biological response. Biochim. Biophys. Acta 1758, 2037–2048. doi: 10.1016/j.bbamem.2006.06.015
Don-Doncow, N., Vanherle, L., Zhang, Y., and Meissner, A. (2019). T-cell accumulation in the hypertensive brain: A role for sphingosine-1-phosphate-mediated chemotaxis. Int. J. Mol. Sci. 20:537. doi: 10.3390/ijms20030537
Edsall, L. C., Pirianov, G. G., and Spiegel, S. (1997). Involvement of sphingosine 1-phosphate in nerve growth factor-mediated neuronal survival and differentiation. J. Neurosci. 17, 6952–6960. doi: 10.1523/JNEUROSCI.17-18-06952.1997
Fettel, J., Kühn, B., Guillen, N. A., Sürün, D., Peters, M., Bauer, R., et al. (2019). Sphingosine-1-phosphate (S1P) induces potent anti-inflammatory effects in vitro and in vivo by S1P receptor 4-mediated suppression of 5-lipoxygenase activity. FASEB J. 33, 1711–1726. doi: 10.1096/fj.201800221R
Finkbeiner, S., Tavazoie, S. F., Maloratsky, A., Jacobs, K. M., Harris, K. M., and Greenberg, M. E. (1997). CREB: A major mediator of neuronal neurotrophin responses. Neuron 19, 1031–1047. doi: 10.1016/s0896-6273(00)80395-5
GBD 2016 Parkinson’s Disease Collaborators. (2018). Global, regional, and national burden of Parkinson’s disease, 1990-2016: A systematic analysis for the Global Burden of Disease Study 2016. Lancet Neurol. 17, 939–953.
Gil, P. R., Japtok, L., and Kleuser, B. (2010). Sphingosine 1-phosphate mediates chemotaxis of human primary fibroblasts via the S1P-receptor subtypes S1P1 and S1P3 and Smad-signalling. Cytoskeleton 67, 773–783. doi: 10.1002/cm.20486
Gill, J. F., Delezie, J., Santos, G., McGuirk, S., Schnyder, S., Frank, S., et al. (2019). Peroxisome proliferator-activated receptor γ coactivator 1α regulates mitochondrial calcium homeostasis, sarcoplasmic reticulum stress, and cell death to mitigate skeletal muscle aging. Aging Cell 18:e12993.
Gilmore, J. L., Xiao, H. Y., Dhar, T. G. M., Yang, M. G., Xiao, Z., Xie, J., et al. (2019). Identification and Preclinical Pharmacology of ((1 R,3 S)-1-Amino-3-((S)-6- (2-methoxyphenethyl)-5,6,7,8-tetrahydronaphthalen-2-yl)cyclopentyl)methanol (BMS-986166): A Differentiated Sphingosine-1-phosphate Receptor 1 (S1P1) Modulator Advanced into Clinical Trials. J. Med. Chem. 62, 2265–2285. doi: 10.1021/acs.jmedchem.8b01695
Gong, L., Shen, Y., Wang, S., Wang, X., Ji, H., Wu, X., et al. (2023). Nuclear SPHK2/S1P induces oxidative stress and NLRP3 inflammasome activation via promoting p53 acetylation in lipopolysaccharide-induced acute lung injury. Cell Death Discov. 9:12. doi: 10.1038/s41420-023-01320-5
Graeler, M., and Goetzl, E. J. (2002). Activation-regulated expression and chemotactic function of sphingosine 1-phosphate receptors in mouse splenic T cells. FASEB J. 16, 1874–1878. doi: 10.1096/fj.02-0548com
Gräler, M. H., Bernhardt, G., and Lipp, M. (1998). EDG6, a novel G-protein-coupled receptor related to receptors for bioactive lysophospholipids, is specifically expressed in lymphoid tissue. Genomics 53, 164–169. doi: 10.1006/geno.1998.5491
Guerrero, M., Urbano, M., Velaparthi, S., Zhao, J., Schaeffer, M. T., Brown, S., et al. (2011). Discovery, design and synthesis of the first reported potent and selective sphingosine-1-phosphate 4 (S1P4) receptor antagonists. Bioorgan. Med. Chem. Lett. 21, 3632–3636. doi: 10.1016/j.bmcl.2011.04.097
Hait, N. C., and Maiti, A. (2017). The role of sphingosine-1-phosphate and ceramide-1-phosphate in inflammation and cancer. Mediat. Inflamm. 2017:4806541.
Hao, Y., Guo, M., Feng, Y., Dong, Q., and Cui, M. (2020). Lysophospholipids and Their G-coupled protein signaling in Alzheimer’s Disease: From physiological performance to pathological impairment. Front. Mol. Neurosci. 13:58. doi: 10.3389/fnmol.2020.00058
Harms, A. S., Ferreira, S. A., and Romero-Ramos, M. (2021). Periphery and brain, innate and adaptive immunity in Parkinson’s disease. Acta Neuropathol. 141, 527–545.
Harvald, E. B., Olsen, A. S., and Færgeman, N. J. (2015). Autophagy in the light of sphingolipid metabolism. Apoptosis 20, 658–670.
Hauser, D. N., and Hastings, T. G. (2013). Mitochondrial dysfunction and oxidative stress in Parkinson’s disease and monogenic parkinsonism. Neurobiol. Dis. 51, 35–42.
Hii, L. W., Chung, F. F., Mai, C. W., Yee, Z. Y., Chan, H. H., Raja, V. J., et al. (2020). Sphingosine Kinase 1 regulates the survival of breast cancer stem cells and non-stem breast cancer cells by suppression of STAT1. Cells 9:886. doi: 10.3390/cells9040886
Huang, C., Hu, F., Song, D., Sun, X., Liu, A., Wu, Q., et al. (2022). EZH2-triggered methylation of SMAD3 promotes its activation and tumor metastasis. J. Clin. Investig. 132:e152394. doi: 10.1172/JCI152394
Igarashi, N., Okada, T., Hayashi, S., Fujita, T., Jahangeer, S., and Nakamura, S. (2003). Sphingosine kinase 2 is a nuclear protein and inhibits DNA synthesis. J. Biol. Chem. 278, 46832–46839.
Im, D. S., Heise, C. E., Ancellin, N., O’Dowd, B. F., Shei, G. J., Heavens, R. P., et al. (2000). Characterization of a novel sphingosine 1-phosphate receptor, Edg-8. J. Biol. Chem. 275, 14281–14286.
Ishii, I., Friedman, B., Ye, X., Kawamura, S., McGiffert, C., Contos, J. J., et al. (2001). Selective loss of sphingosine 1-phosphate signaling with no obvious phenotypic abnormality in mice lacking its G protein-coupled receptor, LP(B3)/EDG-3. J. Biol. Chem. 276, 33697–33704. doi: 10.1074/jbc.M104441200
Jaillard, C., Harrison, S., Stankoff, B., Aigrot, M. S., Calver, A. R., Duddy, G., et al. (2005). Edg8/S1P5: An oligodendroglial receptor with dual function on process retraction and cell survival. J. Neurosci. 25, 1459–1469. doi: 10.1523/JNEUROSCI.4645-04.2005
Jeon, W. J., Chung, K. W., Lee, J. H., and Im, D. S. (2021). Suppressive Effect of CYM50358 S1P4 antagonist on mast cell degranulation and allergic asthma in mice. Biomol. Therapeut. 29, 492–497. doi: 10.4062/biomolther.2020.206
Jo, E., Bhhatarai, B., Repetto, E., Guerrero, M., Riley, S., Brown, S. J., et al. (2012). Novel selective allosteric and bitopic ligands for the S1P(3) receptor. ACS Chem. Biol. 7, 1975–1983. doi: 10.1021/cb300392z
Jolly, P. S., Bektas, M., Watterson, K. R., Sankala, H., Payne, S. G., Milstien, S., et al. (2005). Expression of SphK1 impairs degranulation and motility of RBL-2H3 mast cells by desensitizing S1P receptors. Blood 105, 4736–4742. doi: 10.1182/blood-2004-12-4686
Kang, S. S., Zhang, Z., Liu, X., Manfredsson, F. P., Benskey, M. J., Cao, X., et al. (2017). TrkB neurotrophic activities are blocked by α-synuclein, triggering dopaminergic cell death in Parkinson’s disease. Proc. Natl. Acad. Sci. U. S. A. 114, 10773–10778. doi: 10.1073/pnas.1713969114
Kanno, T., and Nishizaki, T. (2011). Endogenous sphingosine 1-phosphate regulates spontaneous glutamate release from mossy fiber terminals via S1P(3) receptors. Life Sci. 89, 137–140. doi: 10.1016/j.lfs.2011.05.021
Karunakaran, I., Alam, S., Jayagopi, S., Frohberger, S. J., Hansen, J. N., Kuehlwein, J., et al. (2019). Neural sphingosine 1-phosphate accumulation activates microglia and links impaired autophagy and inflammation. Glia 67, 1859–1872. doi: 10.1002/glia.23663
Khattar, M., Deng, R., Kahan, B. D., Schroder, P. M., Phan, T., Rutzky, L. P., et al. (2013). Novel sphingosine-1-phosphate receptor modulator KRP203 combined with locally delivered regulatory T cells induces permanent acceptance of pancreatic islet allografts. Transplantation 95, 919–927. doi: 10.1097/TP.0b013e3182842396
Kihara, A., Anada, Y., and Igarashi, Y. (2006). Mouse sphingosine kinase isoforms SPHK1a and SPHK1b differ in enzymatic traits including stability, localization, modification, and oligomerization. J. Biol. Chem. 281, 4532–4539. doi: 10.1074/jbc.M510308200
Kihara, Y., Mizuno, H., and Chun, J. (2015). Lysophospholipid receptors in drug discovery. Exp. Cell Res. 333, 171–177.
Komiya, T., Sato, K., Shioya, H., Inagaki, Y., Hagiya, H., Kozaki, R., et al. (2013). Efficacy and immunomodulatory actions of ONO-4641, a novel selective agonist for sphingosine 1-phosphate receptors 1 and 5, in preclinical models of multiple sclerosis. Clin. Exp. Immunol. 171, 54–62. doi: 10.1111/j.1365-2249.2012.04669.x
Kunkel, G. T., Maceyka, M., Milstien, S., and Spiegel, S. (2013). Targeting the sphingosine-1-phosphate axis in cancer, inflammation and beyond. Nat. Rev. Drug Discov. 12, 688–702. doi: 10.1038/nrd4099
Lavieu, G., Scarlatti, F., Sala, G., Carpentier, S., Levade, T., Ghidoni, R., et al. (2006). Regulation of autophagy by sphingosine kinase 1 and its role in cell survival during nutrient starvation. J. Biol. Chem. 281, 8518–8527. doi: 10.1074/jbc.M506182200
Lee, H. J., Choe, K., Park, J. S., Khan, A., Kim, M. W., Park, T. J., et al. (2022). O-Cyclic phytosphingosine-1-phosphate protects against motor dysfunctions and glial cell mediated neuroinflammation in the Parkinson’s Disease mouse models. Antioxidants 11:2107. doi: 10.3390/antiox11112107
Li, C., Li, J. N., Kays, J., Guerrero, M., and Nicol, G. D. (2015). Sphingosine 1-phosphate enhances the excitability of rat sensory neurons through activation of sphingosine 1-phosphate receptors 1 and/or 3. J. Neuroinflamm. 12:70.
Li, S. Y., Johnson, R., Smyth, L. C., and Dragunow, M. (2022). Platelet-derived growth factor signalling in neurovascular function and disease. Int. J. Biochem. Cell Biol. 145:106187.
Li, X., Liu, T., Wu, T. T., Feng, Y., Peng, S. J., Yin, H., et al. (2021). SIRT1 Deacetylates TET2 and promotes its ubiquitination degradation to achieve neuroprotection against Parkinson’s Disease. Front. Neurol. 12:652882. doi: 10.3389/fneur.2021.652882
Li, Y., Tang, Y., Liu, J., Meng, X., Wang, Y., Min, Q., et al. (2022). Glia maturation factor-γ is involved in S1P-induced marginal zone B-cell chemotaxis and optimal IgM production to type II T-independent antigen. Int. Immunol. 34, 35–43.
Li, Y., Zhang, W., Li, J., Sun, Y., Yang, Q., Wang, S., et al. (2020). The imbalance in the aortic ceramide/sphingosine-1-phosphate rheostat in ovariectomized rats and the preventive effect of estrogen. Lipids Health Dis. 19:95. doi: 10.1186/s12944-020-01279-7
Liaskou, E., Wilson, D. V., and Oo, Y. H. (2012). Innate immune cells in liver inflammation. Mediat. Inflamm. 2012:949157.
Liu, H., Li, L., Chen, Z., Song, Y., Liu, W., Gao, G., et al. (2021). S1PR2 inhibition attenuates allergic asthma possibly by regulating autophagy. Front. Pharmacol. 11:598007. doi: 10.3389/fphar.2020.598007
Liu, K., Sun, T., Luan, Y., Chen, Y., Song, J., Ling, L., et al. (2022). Berberine ameliorates erectile dysfunction in rats with streptozotocin-induced diabetes mellitus through the attenuation of apoptosis by inhibiting the SPHK1/S1P/S1PR2 and MAPK pathways. Andrology 10, 404–418. doi: 10.1111/andr.13119
Liu, Y., and Tie, L. (2019). Apolipoprotein M and sphingosine-1-phosphate complex alleviates TNF-α-induced endothelial cell injury and inflammation through PI3K/AKT signaling pathway. BMC Cardiovasc. Disord. 19:279. doi: 10.1186/s12872-019-1263-4
Loison, F., Zhu, H., Karatepe, K., Kasorn, A., Liu, P., Ye, K., et al. (2014). Proteinase 3-dependent caspase-3 cleavage modulates neutrophil death and inflammation. J. Clin. Investig. 124, 4445–4458. doi: 10.1172/JCI76246
Lory, W., Wellslager, B., Sun, C., Yilmaz, Ö, and Yu, H. (2023). Inhibition of sphingosine-1-phosphate receptor 2 by JTE013 enhanced alveolar bone regeneration by promoting angiogenesis. Int. J. Mol. Sci. 24:3401. doi: 10.3390/ijms24043401
Macdonald, R., Barnes, K., Hastings, C., and Mortiboys, H. (2018). Mitochondrial abnormalities in Parkinson’s disease and Alzheimer’s disease: Can mitochondria be targeted therapeutically? Biochem. Soc. Transac. 46, 891–909.
Maceyka, M., Sankala, H., Hait, N. C., Le Stunff, H., Liu, H., Toman, R., et al. (2005). SphK1 and SphK2, sphingosine kinase isoenzymes with opposing functions in sphingolipid metabolism. J. Biol. Chem. 280, 37118–37129.
Mahapatra, M. K., Karuppasamy, M., and Sahoo, B. M. (2022). Therapeutic potential of semaglutide, a newer GLP-1 receptor agonist, in abating obesity, non-alcoholic steatohepatitis and neurodegenerative diseases: A narrative review. Pharmaceut. Res. 39, 1233–1248. doi: 10.1007/s11095-022-03302-1
Malek, R. L., Toman, R. E., Edsall, L. C., Wong, S., Chiu, J., Letterle, C. A., et al. (2001). Nrg-1 belongs to the endothelial differentiation gene family of G protein-coupled sphingosine-1-phosphate receptors. J. Biol. Chem. 276, 5692–5699. doi: 10.1074/jbc.M003964200
McGinley, M. P., and Cohen, J. A. (2021). Sphingosine 1-phosphate receptor modulators in multiple sclerosis and other conditions. Lancet 398, 1184–1194.
Mendelson, K., Evans, T., and Hla, T. (2014). Sphingosine 1-phosphate signalling. Development 141, 5–9.
Mirzaei, M., Abyadeh, M., Turner, A. J., Wall, R. V., Chick, J. M., Paulo, J. A., et al. (2022). Fingolimod effects on the brain are mediated through biochemical modulation of bioenergetics, autophagy, and neuroinflammatory networks. Proteomics 22:e2100247. doi: 10.1002/pmic.202100247
Mitrofanova, A., Mallela, S. K., Ducasa, G. M., Yoo, T. H., Rosenfeld-Gur, E., Zelnik, I. D., et al. (2019). SMPDL3b modulates insulin receptor signaling in diabetic kidney disease. Nat. Commun. 10:2692. doi: 10.1038/s41467-019-10584-4
Mitroi, D. N., Karunakaran, I., Gräler, M., Saba, J. D., Ehninger, D., Ledesma, M. D., et al. (2017). SGPL1 (sphingosine phosphate lyase 1) modulates neuronal autophagy via phosphatidylethanolamine production. Autophagy 13, 885–899. doi: 10.1080/15548627.2017.1291471
Mizugishi, K., Yamashita, T., Olivera, A., Miller, G. F., Spiegel, S., and Proia, R. L. (2005). Essential role for sphingosine kinases in neural and vascular development. Mol. Cell. Biol. 25, 11113–11121. doi: 10.1128/MCB.25.24.11113-11121.2005
Motyl, J., Przykaza, Ł, Boguszewski, P. M., Kosson, P., and Strosznajder, J. B. (2018). Pramipexole and Fingolimod exert neuroprotection in a mouse model of Parkinson’s disease by activation of sphingosine kinase 1 and Akt kinase. Neuropharmacology 135, 139–150. doi: 10.1016/j.neuropharm.2018.02.023
Motyl, J. A., Strosznajder, J. B., Wencel, A., and Strosznajder, R. P. (2021). Recent insights into the interplay of alpha-synuclein and sphingolipid signaling in Parkinson’s Disease. Int. J. Mol. Sci. 22:6277. doi: 10.3390/ijms22126277
Murakami, M., Ichihara, M., Sobue, S., Kikuchi, R., Ito, H., Kimura, A., et al. (2007). RET signaling-induced SPHK1 gene expression plays a role in both GDNF-induced differentiation and MEN2-type oncogenesis. J. Neurochem. 102, 1585–1594. doi: 10.1111/j.1471-4159.2007.04673.x
Murakami, M., Ito, H., Hagiwara, K., Kobayashi, M., Hoshikawa, A., Takagi, A., et al. (2011). Sphingosine kinase 1/S1P pathway involvement in the GDNF-induced GAP43 transcription. J. Cell. Biochem. 112, 3449–3458. doi: 10.1002/jcb.23275
Murphy, K. E., Gysbers, A. M., Abbott, S. K., Tayebi, N., Kim, W. S., Sidransky, E., et al. (2014). Reduced glucocerebrosidase is associated with increased α-synuclein in sporadic Parkinson’s disease. Brain 137, 834–848. doi: 10.1093/brain/awt367
Murthy, A. S. N., Das, S., Singh, T., Kim, T. W., Sepay, N., Jeon, S., et al. (2022). Mitochondria targeting molecular transporters: Synthesis, lipophilic effect, and ionic complex. Drug Delivery 29, 270–283. doi: 10.1080/10717544.2021.2023696
Nayak, D., Huo, Y., Kwang, W. X., Pushparaj, P. N., Kumar, S. D., Ling, E. A., et al. (2010). Sphingosine kinase 1 regulates the expression of proinflammatory cytokines and nitric oxide in activated microglia. Neuroscience 166, 132–144.
Nicholas, S. E., Rowsey, T. G., Priyadarsini, S., Mandal, N. A., and Karamichos, D. (2017). Unravelling the interplay of sphingolipids and TGF-β signaling in the human corneal stroma. PLoS One 12:e0182390. doi: 10.1371/journal.pone.0182390
Nishi, T., Miyazaki, S., Takemoto, T., Suzuki, K., Iio, Y., Nakajima, K., et al. (2011). Discovery of CS-0777: A potent, selective, and orally active S1P1 Agonist. ACS Med. Chem. Lett. 2, 368–372. doi: 10.1021/ml100301k
Obinata, H., and Hla, T. (2012). Sphingosine 1-phosphate in coagulation and inflammation. Semin. Immunopathol. 34, 73–91.
Ogawa, M., Murae, M., Gemba, R., Irie, T., Shimojima, M., Saijo, M., et al. (2022). L-DOPA, a treatment for Parkinson’s disease, and its enantiomer D-DOPA inhibit severe fever with thrombocytopenia syndrome virus infection in vitro. J. Infect. Chemother. 28, 373–376. doi: 10.1016/j.jiac.2021.11.005
Olivera, A., Kohama, T., Edsall, L., Nava, V., Cuvillier, O., Poulton, S., et al. (1999). Sphingosine kinase expression increases intracellular sphingosine-1-phosphate and promotes cell growth and survival. J. Cell Biol. 147, 545–558.
Pajares, M. I, Rojo, A., Manda, G., Boscá, L., and Cuadrado, A. (2020). Inflammation in Parkinson’s Disease: Mechanisms and therapeutic implications. Cells 9:1687.
Paradiso, E., Lazzaretti, C., Sperduti, S., Antoniani, F., Fornari, G., Brigante, G., et al. (2021). Sphingosine-1 phosphate induces cAMP/PKA-independent phosphorylation of the cAMP response element-binding protein (CREB) in granulosa cells. Mol. Cell. Endocrinol. 520:111082. doi: 10.1016/j.mce.2020.111082
Pépin, É, Jalinier, T., Lemieux, G. L., Massicotte, G., and Cyr, M. (2020). Sphingosine-1-phosphate receptors modulators decrease signs of neuroinflammation and prevent Parkinson’s Disease symptoms in the 1-Methyl-4-Phenyl-1,2,3,6-Tetrahydropyridine mouse model. Front. Pharmacol. 11:77. doi: 10.3389/fphar.2020.00077
Petrache, I., and Berdyshev, E. V. (2016). Ceramide signaling and metabolism in pathophysiological states of the lung. Annu. Rev. Physiol. 78, 463–480.
Prieschl, E. E., Csonga, R., Novotny, V., Kikuchi, G. E., and Baumruker, T. (1999). The balance between sphingosine and sphingosine-1-phosphate is decisive for mast cell activation after Fc epsilon receptor I triggering. J. Exp. Med. 190, 1–8. doi: 10.1084/jem.190.1.1
Punzi, G., Porcelli, V., Ruggiu, M., Hossain, M. F., Menga, A., Scarcia, P., et al. (2018). SLC25A10 biallelic mutations in intractable epileptic encephalopathy with complex I deficiency. Hum. Mol. Genet. 27, 499–504. doi: 10.1093/hmg/ddx419
Pyszko, J., and Strosznajder, J. B. (2014). Sphingosine kinase 1 and sphingosine-1-phosphate in oxidative stress evoked by 1-methyl-4-phenylpyridinium (MPP+) in human dopaminergic neuronal cells. Mol. Neurobiol. 50, 38–48.
Radeke, H. H., Stein, J., Van Assche, G., Rogler, G., Lakatos, P. L., Muellershausen, F., et al. (2020). A multicentre, double-blind, placebo-controlled, parallel-group study to evaluate the efficacy, safety, and tolerability of the S1P receptor agonist KRP203 in patients with moderately active refractory ulcerative colitis. Inflamm. Intest. Dis. 5, 180–190. doi: 10.1159/000509393
Ren, M., Han, M., Wei, X., Guo, Y., Shi, H., Zhang, X., et al. (2017). FTY720 Attenuates 6-OHDA-associated dopaminergic degeneration in cellular and mouse Parkinsonian models. Neurochem. Res. 42, 686–696. doi: 10.1007/s11064-016-2125-4
Romani, R., Manni, G., Donati, C., Pirisinu, I., Bernacchioni, C., Gargaro, M., et al. (2018). S1P promotes migration, differentiation and immune regulatory activity in amniotic-fluid-derived stem cells. Eur. J. Pharmacol. 833, 173–182. doi: 10.1016/j.ejphar.2018.06.005
Roy, R., Alotaibi, A. A., and Freedman, M. S. (2021). Sphingosine 1-phosphate receptor modulators for multiple sclerosis. CNS Drugs 35, 385–402.
Ruggieri, S., Quartuccio, M. E., and Prosperini, L. (2022). Ponesimod in the treatment of relapsing forms of multiple sclerosis: An update on the emerging clinical data. Degenerat. Neurol. Neuromusc. Dis. 12, 61–73. doi: 10.2147/DNND.S313825
Ruvolo, P. P., Deng, X., Carr, B. K., and May, W. S. (1998). A functional role for mitochondrial protein kinase Calpha in Bcl2 phosphorylation and suppression of apoptosis. J. Biol. Chem. 273, 25436–25442. doi: 10.1074/jbc.273.39.25436
Rykowski, S., Gurda-Woźna, D., Fedoruk-Wyszomirska, A., Orlicka-Płocka, M., Kowalczyk, A., Stączek, P., et al. (2023). Carboranyl-1,8-naphthalimide intercalators induce lysosomal membrane permeabilization and ferroptosis in cancer cell lines. J. Enzyme Inhibit. Med. Chem. 38:2171028. doi: 10.1080/14756366.2023.2171028
Safarian, F., Khallaghi, B., Ahmadiani, A., and Dargahi, L. (2015). Activation of S1P1 receptor regulates PI3K/Akt/FoxO3a pathway in response to oxidative stress in PC12 cells. J. Mol. Neurosci. 56, 177–187. doi: 10.1007/s12031-014-0478-1
Samuvel, D. J., Saxena, N., Dhindsa, J. S., Singh, A. K., Gill, G. S., Grobelny, D. W., et al. (2015). AKP-11 - A Novel S1P1 agonist with favorable safety profile attenuates experimental autoimmune encephalomyelitis in rat model of multiple sclerosis. PLoS One 10:e0141781. doi: 10.1371/journal.pone.0141781
Sandborn, W. J., Peyrin-Biroulet, L., Zhang, J., Chiorean, M., Vermeire, S., Lee, S. D., et al. (2020). Efficacy and safety of etrasimod in a phase 2 randomized trial of patients with ulcerative colitis. Gastroenterology 158, 550–561. doi: 10.1053/j.gastro.2019.10.035
Sandhya, V. K., Raju, R., Verma, R., Advani, J., Sharma, R., Radhakrishnan, A., et al. (2013). A network map of BDNF/TRKB and BDNF/p75NTR signaling system. J. Cell Commun. Signal. 7, 301–307. doi: 10.1007/s12079-013-0200-z
Schapira, A. H., Cooper, J. M., Dexter, D., Clark, J. B., Jenner, P., and Marsden, C. D. (1990). Mitochondrial complex I deficiency in Parkinson’s disease. J. Neurochem. 54, 823–827.
Schulte, M., Frick, K., Gnandt, E., Jurkovic, S., Burschel, S., Labatzke, R., et al. (2019). A mechanism to prevent production of reactive oxygen species by Escherichia coli respiratory complex I. Nat. Commun. 10:2551. doi: 10.1038/s41467-019-10429-0
Schwedhelm, E., Englisch, C., Niemann, L., Lezius, S., von Lucadou, M., Marmann, K., et al. (2021). Sphingosine-1-phosphate, motor severity, and progression in Parkinson’s Disease (MARK-PD). Move. Disord. 36, 2178–2182. doi: 10.1002/mds.28652
Scott, F. L., Clemons, B., Brooks, J., Brahmachary, E., Powell, R., Dedman, H., et al. (2016). Ozanimod (RPC1063) is a potent sphingosine-1-phosphate receptor-1 (S1P1) and receptor-5 (S1P5) agonist with autoimmune disease-modifying activity. Br. J. Pharmacol. 173, 1778–1792. doi: 10.1111/bph.13476
Shalizi, A., Lehtinen, M., Gaudilliere, B., Donovan, N., Han, J., Konishi, Y., et al. (2003). Characterization of a neurotrophin signaling mechanism that mediates neuron survival in a temporally specific pattern. J. Neurosci. 23, 7326–7336. doi: 10.1523/JNEUROSCI.23-19-07326.2003
Shen, Z., Liu, C., Liu, P., Zhao, J., and Xu, W. (2014). Sphingosine 1-phosphate (S1P) promotes mitochondrial biogenesis in Hep G2 cells by activating Peroxisome proliferator-activated receptor γ coactivator 1α (PGC-1α). Cell Stress Chaperones 19, 541–548.
Shults, C. W., Nasirian, F., Ward, D. M., Nakano, K., Pay, M., Hill, L. R., et al. (1995). Carbidopa/levodopa and selegiline do not affect platelet mitochondrial function in early Parkinsonism. Neurology 45, 344–348.
Siibak, T., Clemente, P., Bratic, A., Bruhn, H., Kauppila, T. E. S., Macao, B., et al. (2017). A multi-systemic mitochondrial disorder due to a dominant p.Y955H disease variant in DNA polymerase gamma. Hum. Mol. Genet. 26, 2515–2525. doi: 10.1093/hmg/ddx146
Sim-Selley, L. J., Goforth, P. B., Mba, M. U., Macdonald, T. L., Lynch, K. R., Milstien, S., et al. (2009). Sphingosine-1-phosphate receptors mediate neuromodulatory functions in the CNS. J. Neurochem. 110, 1191–1202. doi: 10.1111/j.1471-4159.2009.06202.x
Sivasubramanian, M., Kanagaraj, N., Dheen, S. T., and Tay, S. S. (2015). Sphingosine kinase 2 and sphingosine-1-phosphate promotes mitochondrial function in dopaminergic neurons of mouse model of Parkinson’s disease and in MPP+ -treated MN9D cells in vitro. Neuroscience 290, 636–648. doi: 10.1016/j.neuroscience.2015.01.032
Spampinato, S. F., Costantino, G., Merlo, S., Canonico, P. L., and Sortino, M. A. (2022). Microglia Contributes to BAF-312 effects on blood-brain barrier stability. Biomolecules 12:1174. doi: 10.3390/biom12091174
Spiegel, S., and Milstien, S. (2003). Sphingosine-1-phosphate: An enigmatic signalling lipid. Nat. Rev. Mol. Cell Biol. 4, 397–407.
St-Cyr Giguère, F., Attiori Essis, S., Chagniel, L., Germain, M., Cyr, M., and Massicotte, G. (2017). The sphingosine-1-phosphate receptor 1 agonist SEW2871 reduces Tau-Ser262 phosphorylation in rat hippocampal slices. Brain Res. 1658, 51–59. doi: 10.1016/j.brainres.2017.01.014
Strub, G. M., Paillard, M., Liang, J., Gomez, L., Allegood, J. C., Hait, N. C., et al. (2011). Sphingosine-1-phosphate produced by sphingosine kinase 2 in mitochondria interacts with prohibitin 2 to regulate complex IV assembly and respiration. FASEB J. 25, 600–612.
Su, W. M., Gu, X. J., Hou, Y. B., Zhang, L. Y., Cao, B., Ou, R. W., et al. (2021). Association Analysis of WNT3, HLA-DRB5 and IL1R2 polymorphisms in Chinese patients with Parkinson’s disease and multiple system atrophy. Front. Genet. 12:765833. doi: 10.3389/fgene.2021.765833
Sugahara, K., Maeda, Y., Shimano, K., Mogami, A., Kataoka, H., Ogawa, K., et al. (2017). Amiselimod, a novel sphingosine 1-phosphate receptor-1 modulator, has potent therapeutic efficacy for autoimmune diseases, with low bradycardia risk. Br. J. Pharmacol. 174, 15–27. doi: 10.1111/bph.13641
Sugahara, K., Maeda, Y., Shimano, K., Murase, M., Mochiduki, S., Takemoto, K., et al. (2019). Amiselimod (MT-1303), a novel sphingosine 1-phosphate receptor-1 modulator, potently inhibits the progression of lupus nephritis in two murine SLE models. J. Immunol. Res. 2019:5821589. doi: 10.1155/2019/5821589
Sugimoto, T., Kuroda, H., Horii, Y., Moritake, H., Tanaka, T., and Hattori, S. (2001). Signal transduction pathways through TRK-A and TRK-B receptors in human neuroblastoma cells. Jpn. J. Cancer Res. 92, 152–160.
Sukocheva, O. A. (2018). Expansion of sphingosine kinase and sphingosine-1-phosphate receptor function in normal and cancer cells: From membrane restructuring to mediation of estrogen signaling and stem cell programming. Int. J. Mol. Sci. 19:420. doi: 10.3390/ijms19020420
Sun, X., Johnson, J., and St John, J. C. (2018). Global DNA methylation synergistically regulates the nuclear and mitochondrial genomes in glioblastoma cells. Nucleic Acids Res. 46, 5977–5995. doi: 10.1093/nar/gky339
Sun, X., Xue, L., Wang, Z., and Xie, A. (2022). Update to the treatment of Parkinson’s Disease based on the gut-brain axis mechanism. Front. Neurosci. 16:878239. doi: 10.3389/fnins.2022.878239
Suzuki, Y., Hasegawa, H., Tsuji, T., Tsuruda, K., Sasaki, D., Ishihara, K., et al. (2013). Relationships of diverse apoptotic death process patterns to mitochondrial membrane potential (Δψ(m)) evaluated by three-parameter flow cytometric analysis. Cytotechnology 65, 59–70.
Szatmari, E., Kalita, K. B., Kharebava, G., and Hetman, M. (2007). Role of kinase suppressor of Ras-1 in neuronal survival signaling by extracellular signal-regulated kinase 1/2. J. Neurosci. 27, 11389–11400. doi: 10.1523/JNEUROSCI.3473-07.2007
Tan, E. K., Chao, Y. X., West, A., Chan, L. L., Poewe, W., and Jankovic, J. (2020). Parkinson disease and the immune system - associations, mechanisms and therapeutics. Nat. Rev. Neurol. 16, 303–318.
Tanaka, Y., Kondo, K., Ichibori, A., Yanai, Y., Susuta, Y., Inoue, S., et al. (2020). Amiselimod, a sphingosine 1-phosphate receptor-1 modulator, for systemic lupus erythematosus: A multicenter, open-label exploratory study. Lupus 29, 1902–1913. doi: 10.1177/0961203320966385
Tang, H., Xu, Y., Liu, L., He, L., Huang, J., Pan, J., et al. (2020). Nogo-A/S1PR2 signaling pathway inactivation decreases microvascular damage and enhances microvascular regeneration in PDMCI Mice. Neuroscience 449, 21–34. doi: 10.1016/j.neuroscience.2020.09.057
Tang, Y., Plötz, T., Gräler, M. H., and Gurgul-Convey, E. (2021). Sphingosine-1 phosphate lyase regulates sensitivity of pancreatic beta-cells to lipotoxicity. Int. J. Mol. Sci. 22:10893. doi: 10.3390/ijms221910893
Taniguchi, M., Kitatani, K., Kondo, T., Hashimoto-Nishimura, M., Asano, S., Hayashi, A., et al. (2012). Regulation of autophagy and its associated cell death by “sphingolipid rheostat”: Reciprocal role of ceramide and sphingosine 1-phosphate in the mammalian target of rapamycin pathway. J. Biol. Chem. 287, 39898–39910. doi: 10.1074/jbc.M112.416552
Tao, Y., and Liang, G. (2015). Effect of subthalamic nuclei electrical stimulation in the treatment of Parkinson’s disease. Cell Biochem. Biophys. 71, 113–117.
Tian, L., Wu, Y., Choi, H. J., Sui, X., Li, X., Sofi, M. H., et al. (2022). S1P/S1PR1 signaling differentially regulates the allogeneic response of CD4 and CD8 T cells by modulating mitochondrial fission. Cell. Mol. Immunol. 19, 1235–1250. doi: 10.1038/s41423-022-00921-x
Toman, R. E., Payne, S. G., Watterson, K. R., Maceyka, M., Lee, N. H., Milstien, S., et al. (2004). Differential transactivation of sphingosine-1-phosphate receptors modulates NGF-induced neurite extension. J. Cell Biol. 166, 381–392. doi: 10.1083/jcb.200402016
Tsai, H. C., and Han, M. H. (2016). Sphingosine-1-phosphate (S1P) and S1P signaling pathway: Therapeutic targets in autoimmunity and inflammation. Drugs 76, 1067–1079.
Van Bulck, M., Sierra-Magro, A., Alarcon-Gil, J., Perez-Castillo, A., and Morales-Garcia, J. A. (2019). Novel approaches for the treatment of Alzheimer’s and Parkinson’s Disease. Int. J. Mol. Sci. 20:719.
Van Echten-Deckert, G., Hagen-Euteneuer, N., Karaca, I., and Walter, J. (2014). Sphingosine-1-phosphate: Boon and bane for the brain. Cell Physiol. Biochem. 34, 148–157. doi: 10.1159/000362991
Vermeire, S., Chiorean, M., Panés, J., Peyrin-Biroulet, L., Zhang, J., Sands, B. E., et al. (2021). Long-term safety and efficacy of etrasimod for ulcerative colitis: Results from the open-label extension of the OASIS Study. J. Crohn’s Colitis 15, 950–959. doi: 10.1093/ecco-jcc/jjab016
Vidal-Martinez, G., Najera, K., Miranda, J. D., Gil-Tommee, C., Yang, B., Vargas-Medrano, J., et al. (2019). FTY720 improves behavior, increases brain derived neurotrophic factor levels and reduces α-synuclein pathology in Parkinsonian GM2+/- Mice. Neuroscience 411, 1–10. doi: 10.1016/j.neuroscience.2019.05.029
Wamhoff, B. R., Lynch, K. R., Macdonald, T. L., and Owens, G. K. (2008). Sphingosine-1-phosphate receptor subtypes differentially regulate smooth muscle cell phenotype. Arterioscl. Thromb. Vasc. Biol. 28, 1454–1461. doi: 10.1161/ATVBAHA.107.159392
Wang, F., Zhang, X., Liu, Y., Li, Z., Wei, R., Zhang, Y., et al. (2022). Neuroprotection by ozanimod following intracerebral hemorrhage in mice. Front. Mol. Neurosci. 15:927150. doi: 10.3389/fnmol.2022.927150
Wang, H., Huang, H., and Ding, S. F. (2018). Sphingosine-1-phosphate promotes the proliferation and attenuates apoptosis of Endothelial progenitor cells via S1PR1/S1PR3/PI3K/Akt pathway. Cell Biol. Int. 42, 1492–1502. doi: 10.1002/cbin.10991
Wang, Y. H., Yu, H. T., Pu, X. P., and Du, G. H. (2013). Baicalein prevents 6-hydroxydopamine-induced mitochondrial dysfunction in SH-SY5Y cells via inhibition of mitochondrial oxidation and up-regulation of DJ-1 protein expression. Molecules 18, 14726–14738. doi: 10.3390/molecules181214726
Wang, Z. T., Lu, M. H., Zhang, Y., Ji, W. L., Lei, L., Wang, W., et al. (2019). Disrupted-in-schizophrenia-1 protects synaptic plasticity in a transgenic mouse model of Alzheimer’s disease as a mitophagy receptor. Aging Cell 18:e12860. doi: 10.1111/acel.12860
Weigert, A., Cremer, S., Schmidt, M. V., von Knethen, A., Angioni, C., Geisslinger, G., et al. (2010). Cleavage of sphingosine kinase 2 by caspase-1 provokes its release from apoptotic cells. Blood 115, 3531–3540. doi: 10.1182/blood-2009-10-243444
West, T. O., Magill, P. J., Sharott, A., Litvak, V., Farmer, S. F., and Cagnan, H. (2022). Stimulating at the right time to recover network states in a model of the cortico-basal ganglia-thalamic circuit. PLoS Comput. Biol. 18:e1009887. doi: 10.1371/journal.pcbi.1009887
Wils, P., and Peyrin-Biroulet, L. (2023). Etrasimod for the treatment of ulcerative colitis. Immunotherapy 15, 311–321.
Witte, M. E., Mahad, D. J., Lassmann, H., and van Horssen, J. (2014). Mitochondrial dysfunction contributes to neurodegeneration in multiple sclerosis. Trends Mol. Med. 20, 179–187.
Xia, P., Wang, L., Gamble, J. R., and Vadas, M. A. (1999). Activation of sphingosine kinase by tumor necrosis factor-alpha inhibits apoptosis in human endothelial cells. J. Biol. Chem. 274, 34499–34505.
Xiang, P., Chew, W. S., Seow, W. L., Lam, B. W. S., Ong, W. Y., and Herr, D. R. (2021). The S1P2 receptor regulates blood-brain barrier integrity and leukocyte extravasation with implications for neurodegenerative disease. Neurochem. Int. 146:105018. doi: 10.1016/j.neuint.2021.105018
Xu, D. C., Chen, Y., Xu, Y., ShenTu, C., and Peng, L. H. (2023). Signaling pathways in Parkinson’s disease: Molecular mechanisms and therapeutic interventions. Signal Transduct. Target. Ther. 8:73.
Xue, T., Ji, J., Sun, Y., Huang, X., Cai, Z., Yang, J., et al. (2022). Sphingosine-1-phosphate, a novel TREM2 ligand, promotes microglial phagocytosis to protect against ischemic brain injury. Acta Pharm. Sin. B 12, 1885–1898. doi: 10.1016/j.apsb.2021.10.012
Yamada, M., Tanabe, K., Wada, K., Shimoke, K., Ishikawa, Y., Ikeuchi, T., et al. (2001). Differences in survival-promoting effects and intracellular signaling properties of BDNF and IGF-1 in cultured cerebral cortical neurons. J. Neurochem. 78, 940–951. doi: 10.1046/j.1471-4159.2001.00497.x
Yamagata, K., Tagami, M., Torii, Y., Takenaga, F., Tsumagari, S., Itoh, S., et al. (2003). Sphingosine 1-phosphate induces the production of glial cell line-derived neurotrophic factor and cellular proliferation in astrocytes. Glia 41, 199–206. doi: 10.1002/glia.10180
Yang, M. G., Xiao, Z., Dhar, T. G., Xiao, H. Y., Gilmore, J. L., Marcoux, D., et al. (2016). Asymmetric Hydroboration Approach to the Scalable Synthesis of ((1R,3S)-1-Amino-3-((R)-6-hexyl-5,6,7,8-tetrahydronaphthalen-2-yl)cyclopentyl)methanol (BMS-986104) as a Potent S1P1 Receptor Modulator. J. Med. Chem. 59, 11138–11147. doi: 10.1021/acs.jmedchem.6b01433
Yao, S., Li, L., Sun, X., Hua, J., Zhang, K., Hao, L., et al. (2019). FTY720 inhibits MPP+-induced microglial activation by affecting NLRP3 inflammasome activation. J. Neuroimmune Pharmacol. 14, 478–492. doi: 10.1007/s11481-019-09843-4
Ye, L., Song, J., Zheng, Y., Zhong, M., Liu, J., Zhu, D., et al. (2022). New mechanism for mesenchymal stem cell microvesicle to restore lung permeability: Intracellular S1P signaling pathway independent of S1P receptor-1. Stem Cell Res. Therapy 13:496. doi: 10.1186/s13287-022-03177-4
Yin, Y. X., Sun, Z. P., Huang, S. H., Zhao, L., Geng, Z., and Chen, Z. Y. (2010). RanBPM contributes to TrkB signaling and regulates brain-derived neurotrophic factor-induced neuronal morphogenesis and survival. J. Neurochem. 114, 110–121. doi: 10.1111/j.1471-4159.2010.06745.x
Yonesu, K., Kubota, K., Tamura, M., Inaba, S., Honda, T., Yahara, C., et al. (2011). Purification and identification of activating enzymes of CS-0777, a selective sphingosine 1-phosphate receptor 1 modulator, in erythrocytes. J. Biol. Chem. 286, 24765–24775. doi: 10.1074/jbc.M110.217299
Yoshii, A., and Constantine-Paton, M. (2010). Postsynaptic BDNF-TrkB signaling in synapse maturation, plasticity, and disease. Dev. Neurobiol. 70, 304–322.
Yuan, L., Basdeo, S., and Ji, Q. C. (2018). Overcoming the stability, solubility and extraction challenges in reversed-phase UHPLC-MS/MS bioanalysis of a phosphate drug and its prodrug in blood lysate. J. Pharm. Biomed. Anal. 157, 36–43. doi: 10.1016/j.jpba.2018.05.006
Zehra Okus, F., Busra Azizoglu, Z., Canatan, H., and Eken, A. (2022). S1P analogues SEW2871, BAF312 and FTY720 affect human Th17 and Treg generation ex vivo. Int. Immunopharmacol. 107:108665. doi: 10.1016/j.intimp.2022.108665
Zhang, L., Okada, T., Badawy, S. M. M., Hirai, C., Kajimoto, T., and Nakamura, S. I. (2017). Extracellular α-synuclein induces sphingosine 1-phosphate receptor subtype 1 uncoupled from inhibitory G-protein leaving β-arrestin signal intact. Sci. Rep. 7:44248.
Zhang, L., and Wang, H. (2020). FTY720 in CNS injuries: Molecular mechanisms and therapeutic potential. Brain Res. Bull. 164, 75–82. doi: 10.1016/j.brainresbull.2020.08.013
Zhang, Y., and Wang, J. (2023). Cellular and molecular mechanisms in idiopathic pulmonary fibrosis. Adv. Respir. Med. 91, 26–48.
Zhong, M., Wang, Y., Lin, G., Liao, F. F., and Zhou, F. M. (2023). Dopamine-independent development and maintenance of mouse striatal medium spiny neuron dendritic spines. Neurobiol. Dis. 181:106096. doi: 10.1016/j.nbd.2023.106096
Zhou, C., Kuang, Y., Li, Q., Duan, Y., Liu, X., Yue, J., et al. (2022). Endothelial S1pr2 regulates post-ischemic angiogenesis via AKT/eNOS signaling pathway. Theranostics 12, 5172–5188. doi: 10.7150/thno.71585
Keywords: Parkinson’s disease, sphingosine-1-phosphate, S1P metabolism, S1P modulators, cellular neuropathology
Citation: Wang W, Zhao Y and Zhu G (2023) The role of sphingosine-1-phosphate in the development and progression of Parkinson’s disease. Front. Cell. Neurosci. 17:1288437. doi: 10.3389/fncel.2023.1288437
Received: 04 September 2023; Accepted: 27 November 2023;
Published: 21 December 2023.
Edited by:
Marco Aurelio M. Freire, University of the State of Rio Grande do Norte, BrazilReviewed by:
José Ronaldo dos Santos, Federal University of Sergipe, BrazilSonia Luz Albarracin, Pontifical Javeriana University, Colombia
Maxwell Barbosa Santana, Federal University of Western Pará, Brazil
Copyright © 2023 Wang, Zhao and Zhu. This is an open-access article distributed under the terms of the Creative Commons Attribution License (CC BY). The use, distribution or reproduction in other forums is permitted, provided the original author(s) and the copyright owner(s) are credited and that the original publication in this journal is cited, in accordance with accepted academic practice. No use, distribution or reproduction is permitted which does not comply with these terms.
*Correspondence: Yang Zhao, eWFuZ3poYW90Y21Abmp1Y20uZWR1LmNu; Guoxue Zhu, emd4dWUwMTIyQG5qdWNtLmVkdS5jbg==