- 1Department of Biomedical Engineering, Rensselaer Polytechnic Institute, Troy, NY, United States
- 2Center for Biotechnology and Interdisciplinary Studies, Rensselaer Polytechnic Institute, Troy, NY, United States
- 3Department of Materials Science and Engineering, Rensselaer Polytechnic Institute, Troy, NY, United States
- 4Albany Stratton Veterans Affairs Medical Center, Albany, NY, United States
- 5Department of Biochemistry and Molecular Pharmacology, Mario Negri Institute for Pharmacological Research IRCCS, Milan, Italy
Central nervous system (CNS) glia, including astrocytes, microglia, and oligodendrocytes, play prominent roles in traumatic injury and degenerative disorders. Due to their importance, active pharmaceutical ingredients (APIs) are being developed to modulate CNS glia in order to improve outcomes in traumatic injury and disease. While many of these APIs show promise in vitro, the majority of APIs that are systemically delivered show little penetration through the blood–brain barrier (BBB) or blood-spinal cord barrier (BSCB) and into the CNS, rendering them ineffective. Novel nanomaterials are being developed to deliver APIs into the CNS to modulate glial responses and improve outcomes in injury and disease. Nanomaterials are attractive options as therapies for central nervous system protection and repair in degenerative disorders and traumatic injury due to their intrinsic capabilities in API delivery. Nanomaterials can improve API accumulation in the CNS by increasing permeation through the BBB of systemically delivered APIs, extending the timeline of API release, and interacting biophysically with CNS cell populations due to their mechanical properties and nanoscale architectures. In this review, we present the recent advances in the fields of both locally implanted nanomaterials and systemically administered nanoparticles developed for the delivery of APIs to the CNS that modulate glial activity as a strategy to improve outcomes in traumatic injury and disease. We identify current research gaps and discuss potential developments in the field that will continue to translate the use of glia-targeting nanomaterials to the clinic.
1. Introduction
Glia, which constitute roughly half the cells in the central nervous system (CNS), have essential yet distinct roles in supporting neuronal homeostasis and signal transduction (Allen and Lyons, 2018). In particular, astrocytes, microglia, and oligodendrocytes are necessary for regulating synaptic function, contributing to metabolic support, creating myelin sheaths for signal transduction, and in the CNS immune response (Somjen, 1988; Tomassy et al., 2016; von Bartheld et al., 2016). Glia also are critical players in disease and after traumatic injury, as microglia are the primary source of pro-inflammatory cytokines, astrocytes are regulators of synaptic homeostasis and glial scar formation after injury, and demyelination or changes in myelin thickness by oligodendrocytes alters signal conduction speed (Colonna and Butovsky, 2017; Liddelow and Barres, 2017; Wang S. S. et al., 2018). Due to the fact that neuronal regeneration is highly restricted following CNS injury and disease, glia have begun to emerge as important targets in the development of active pharmaceutical ingredients (APIs) in order to improve clinical outcomes. However, since many APIs do not readily cross the blood–brain barrier (BBB) to impart their action on glia, nanomaterials have been engineered to carry APIs to the site of action, extend the API release timeframe, and also to impact cellular behavior based on their architectural features (Zhang et al., 2016; Dai et al., 2021).
Nanomaterials, including nanoparticles and nanostructured scaffolds, offer many advantages in the delivery of APIs to CNS glia. Nanoparticles (NPs), composed of polymers, liposomes, inorganic materials, and extracellular vesicles, are used to deliver APIs to glia because they can be administered systemically, engineered to cross the BBB, carry and protect sensitive APIs, and be targeted to cells or regions of interest using antibodies, targeting peptides, and even nucleic acids (Patel et al., 2012; Mann et al., 2016; Zuidema et al., 2016; Furtado et al., 2018; Zhou et al., 2018; Ciciriello et al., 2022; Waggoner et al., 2022). The advantage of NP technologies is that they can be administered systemically; however, in some cases, such as TBI, BBB permeability can decrease over time, which can reduce the accumulation of the API in the CNS at longer time points after injury (Werner and Engelhard, 2007; Mann et al., 2016). In CNS disorders, BBB breakdown often occurs prior to neurodegeneration and persists as the disease progresses (Sweeney et al., 2018a,b). While this can be advantageous for API delivery to the CNS, there are also complex mechanisms, including disrupted BBB transporter expression, inflammation, immune products, and impaired solute transport, which can limit API accumulation in these regions (Sweeney et al., 2018b). NP design needs to consider this when attempting to traverse the injured or diseased BBB to deliver APIs to regions of interest. Nanostructured scaffolds have other advantages, even though they generally must be surgically implanted or injected into the site of interest. The nanoscale topographical features of nanomaterial scaffolds can influence cellular function, migration, and growth; APIs can be delivered from either the surface of the scaffold or incorporated into the scaffold to extend release, and, since the scaffolds are implanted directly at the site, APIs are released locally to glia (Tsui et al., 2019; Puhl et al., 2020, 2022). Here, we present the current state-of-the-art in API delivery to CNS glia using nanomaterials, point out the existing gaps in the research, and discuss the potential future developments and advances of this field that will drive nanomaterial delivery of APIs to CNS glia towards the clinic.
2. Nanomaterials for API delivery to CNS glia
2.1. Nanoparticle API delivery to astrocytes
Astrocytes are CNS glia that perform core homeostatic functions and whose radiating processes can contact upwards of 1 million synapses in humans (Hasel and Liddelow, 2021). They are integral parts of the BBB where they uptake metabolites such as glucose to fuel active neurons, modulate neurotransmitter concentration in synapses, phagocytose synapses, form part of the glymphatic system, and aid in the homeostatic control of neuronal redox stress (Sofroniew and Vinters, 2010; Hasel and Liddelow, 2021). In the event of injury or pathology, including stab wound injuries, experimental autoimmune encephalomyelitis (EAE), middle cerebral occlusion (MCAO), hypertrophic ciliary neurotrophic factor induction, cortical lesion, spinal cord injury (SCI), Alzheimer’s disease, Parkinson’s disease, prion disease, Huntington’s disease, multiple sclerosis (MS), and amyotrophic lateral sclerosis (ALS), astrocytes respond via a process termed reactive astrogliosis (Anderson et al., 2014; Pekny et al., 2016; Escartin et al., 2019). This process can be protective, but persistent reactive astrogliosis can become maladaptive, making it a target for APIs (Pekny et al., 2016). Therefore, APIs that act on astrocytes have been developed that target metabolic pathways, transporters and receptors, cell–cell interactions, and even as glia-to-neuron conversion therapies (Lee et al., 2022). Their role in disease and trauma, as well as recent advances in nanomaterial API delivery, make astrocytes a critical cell to target in order to improve clinical outcomes in CNS disease and injury.
Nanoparticles (NPs), including polymer, dendrimer, lipid, and inorganic nanoparticles, have all been developed to deliver APIs to astrocytes. Specific nanoparticle types, their payloads, the model studied, and the outcomes are listed in Table 1. The majority of astrocyte research to date has employed either polymer NPs or dendrimers to deliver APIs (Newland et al., 2014; Serramia et al., 2015; Lozic et al., 2016; Kong et al., 2017; Chowdhury et al., 2018; Surnar et al., 2018; Holmkvist et al., 2020; Proulx et al., 2020; Vismara et al., 2020; Wang et al., 2020; Clementino A. et al., 2021; Gu et al., 2022; Huang et al., 2022; Narsineni et al., 2023; Perumal et al., 2023; Sabourian et al., 2023; Zhang F. et al., 2023). API payloads range from small molecule drugs to proteins, plasmid DNA, and siRNA (Montenegro et al., 2011; Kannan et al., 2012; Chen and Foldvari, 2016; Tickle and Chari, 2019; Porkolab et al., 2020; Gu et al., 2022). The goal of most APIs is to push astrocytes towards a more protective phenotype, improving their ability to protect neurons in these environments. This includes reducing reactive oxygen species, decreasing astrocyte inflammatory response, and reducing inflammatory cytokine release (Table 1). NP API delivery to astrocytes has been shown to improve outcomes in cerebral palsy, blast-induced hearing loss, neural implants, ALS, and SCI models (Table 1), demonstrating the potential of these therapeutic strategies in future clinical applications.
2.2. Nanomaterial API delivery to astrocytes
Nanomaterial strategies targeting astrocytes, including electrospun fibers, composite hydrogels, and hybrid materials, address two occurrences in the astrocytic response to injury: (1) to reduce glial scar formation, or (2) to mitigate an established glial scar (Jarrin et al., 2021). Often, these materials contain anti-inflammatory API payloads or exogenous stem cells which assist in promoting a neuroprotective phenotype, by diminishing the inhibitory chemical barrier and promoting restoration of the blood-spinal cord barrier. Polymer nanofibers are fabricated by electrospinning, a process that allows for the creation of biodegradable and biocompatible scaffolds that can be used in neural tissue engineering (Schaub et al., 2016; Cheng et al., 2021). Due to their high surface area and structure, electrospun nanofibers mimic the native extracellular matrix of neural tissue and are hence suited to promote neural regeneration (Tian et al., 2015). Nanofibers are attractive as drug depots for astrocytes because their intrinsic material properties can alter astrocyte activation or direct astrocyte growth (Zuidema et al., 2014, 2018). Improved astrocyte activation outcomes have been demonstrated by employing nanofiber scaffolds alone, incorporating stem cells with nanofibers, and even with conductive nanofibers (Zhao et al., 2018; Shu et al., 2019; Yan et al., 2020; Dai et al., 2023; Xu et al., 2023). As a means to further promote neural repair and mitigation of secondary injury, nanofibers can be loaded with APIs that act on astrocytes due to their porous nature (Zhang et al., 2021). Growth factors and small molecule drugs released from nanofibers have shown the ability to reduce astrocyte activation (Zhang et al., 2018; Bighinati et al., 2020; Sun et al., 2020), decreasing GFAP expression and improving outcomes (Table 2).
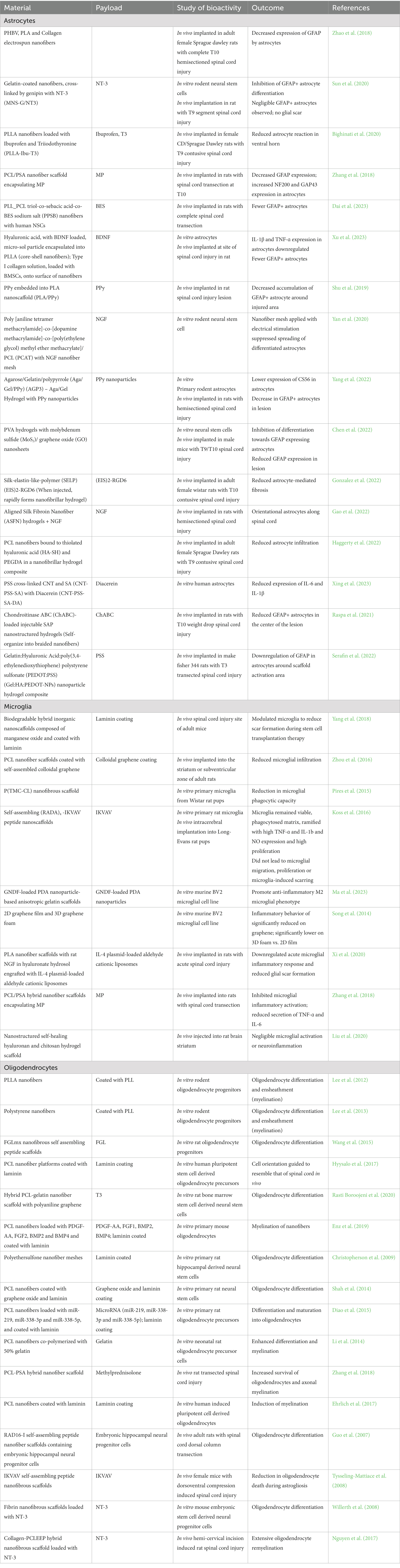
Table 2. Studies that use nanomaterials and nanomaterial delivered APIs to target central nervous system glia.
Nanocomposite hydrogel constructs are used frequently in neural tissue engineering to promote cell adhesion and proliferation, incorporate guidance cues, and provide electrical conductivity in the tissue-supporting scaffold (Madhusudanan et al., 2020). These properties make them especially attractive as injectable materials to deliver APIs to astrocytes. Conductive hydrogels with nanoparticles (Yang et al., 2022) or nanosheets (Chen et al., 2022) following stimulated spinal cord injury demonstrated decreases in GFAP-labeled astrocytes, as well as decreases in chondroitin sulfate proteoglycans and increased neuronal markers (Table 2). Studies using nanofiber hydrogels (NFH) have shown different outcomes, with NFH alone demonstrating no induction of astrocytes (Gonzalez et al., 2022), while increased amounts of astrocytes at the injury site were seen using an NFH construct combined with BMSCs (Li et al., 2020; Haggerty et al., 2022). Nanoparticle hydrogel composites have also shown varying results (Serafin et al., 2022). Hydrogel nanohybrids releasing NGF, diacerein, or chondroitinase ABC have reduced astrocyte activity (Raspa et al., 2021; Gao et al., 2022; Xing et al., 2023), demonstrating the potential of these API-releasing nanomaterials to improve astrocyte outcomes.
2.3. Nanoparticle API delivery to microglia
Microglia constitute 5%–10% of total brain cells and are the only true CNS parenchymal macrophages (Aguzzi et al., 2013). Upon CNS injury or disease, microglia adopt an “amoeboid” morphology and are responsible for phagocytosis and elimination of microbes, dead cells, and protein aggregates, and the secretion of soluble factors, including chemoattractants, cytokines, and neurotrophic factors (Colonna and Butovsky, 2017; Li and Barres, 2018). These polarized cells were traditionally categorized as having either toxic (M1) or protective (M2) states; however, accumulating evidence suggests microglial polarization is complex and multidimensional (Ransohoff, 2016a). In fact, single cell sequencing suggests that depending upon their anatomical compartment and pathological environment, microglia display an entire spectrum of functional states, ranging from highly inflammatory and phagocytic to anti-inflammatory and neuroprotective (Sankowski et al., 2022) Persistent pro-inflammatory microglial activation is a component of almost all neurodegenerative diseases (Ransohoff, 2016b). Because of this, many APIs have been developed to target microglia in order to improve outcomes in CNS disorders or after injury. This has prompted researchers to employ nanomaterials as an engineering approach to amplify further the impact of APIs designed for microglia.
NPs, including polymer, dendrimer, lipid, extracellular vesicles, and inorganic nanoparticles, have been designed to deliver APIs to microglia in many different CNS disorders and studied in various models, including autism spectrum disorder, cerebral palsy, neuropathic pain, SCI, Alzheimer’s, experimental autoimmune encephalomyelitis (EAE), TBI, retinal degeneration, Rett syndrome, and stroke (Iezzi et al., 2012; Sharma et al., 2017, 2020; Ganbold et al., 2020; Khoury et al., 2020; Liao et al., 2020; Gao et al., 2021; Kim et al., 2021; Liu et al., 2021; He et al., 2022; Sepasi et al., 2023) (Table 1). API payloads range from small molecule drugs to proteins, peptides, and siRNA (Lee et al., 2021; Liu et al., 2021; Hernando et al., 2022; Zhang M. et al., 2023). Most NP API therapies aim to modulate the microglial inflammatory response, polarizing microglia towards the more neuronally protective M2 phenotype to alleviate the inflammatory response and improve functional outcomes (Papa et al., 2013, 2016; Lu et al., 2014; Nance et al., 2015, 2017; Saxena et al., 2015; Kim et al., 2017; Wang Y. et al., 2018; Ellert-Miklaszewska et al., 2019; Cahalane et al., 2020; Cho et al., 2021; Xiao et al., 2021; Baghbanbashi et al., 2022; Ganbold et al., 2022; Guo et al., 2022; Hollinger et al., 2022; Shin et al., 2022; Ishida et al., 2023; Kalashnikova et al., 2023; Pu et al., 2023). NP API delivery to microglia has been shown to improve functional outcomes in many in vivo models of CNS disorders (Table 1), demonstrating that these cells have important implications across CNS pathologies and that modulating their response to injury and disease using NPs has immense potential in improving clinical outcomes.
2.4. Nanomaterial API delivery to microglia
Nanomaterial strategies targeting microglia are focused on nanofibrous scaffolds and hybrid nanostructured materials, often with an immunomodulatory payload to polarize microglia towards an anti-inflammatory phenotype in order to promote neuronal protection and repair (Table 2). Microglia have diverse, complex reactions to nanomaterials. 3D biodegradable hybrid inorganic nanoscaffolds modulated microglia in vivo to reduce scar formation during stem cell transplantation therapy for SCI (Yang et al., 2018). PCL nanofiber scaffolds coated with self-assembled colloidal graphene implanted in the striatum or subventricular zone of adult rats promoted reduced microglial infiltration (Watson et al., 2017). On the other hand, when primary microglia were cultured on poly(trimethylene carbonate-co-1-caprolactone) nanofibrous scaffolds, there was a reduction in phagocytic capacity, which indicates an inflammatory phenotype (Pires et al., 2015). Microglia were studied with engineered self-assembling (RADA)4-IKVAV peptide nanoscaffolds, and in vitro remained viable, phagocytosed the matrix, and remained ramified with high levels of TNF-ɑ and IL-1b and NO expression. When injected intracerebrally, however, the nanoscaffold did not lead to microglial migration, proliferation, or microglia-induced scarring (Koss et al., 2016). The inflammatory behavior of BV2 microglia was significantly reduced when interfaced with graphene nanomaterials compared to conventional polystyrene tissue culture substrates, and 3D graphene foams elicited a significantly milder neuroinflammatory response compared to a 2D graphene film (Fabbri et al., 2021). Nanostructured self-healing hyaluronan and chitosan hydrogel scaffolds injected into the rat brain striatum had negligible microglial activation or neuroinflammation (Liu et al., 2020).
The ability of nanomaterials to alter microglial response led researchers to include APIs during development to add a further level of control. Glial cell-derived neurotrophic factor (GNDF)-loaded polydopamine (PDA) nanoparticle-based anisotropic gelatin scaffolds efficiently deliver PDA nanoparticles to scavenge reactive oxygen species and promote the M2 anti-inflammatory polarization in the murine BV2 microglial cell line (Ma et al., 2023). Poly(lactic acid) nanofiber scaffolds with incorporated rat NGF in hyaluronate hydrosol were engrafted with immunoregulatory IL-4 plasmid-loaded aldehyde cationic liposomes and implanted into rats with acute SCI, resulting in a downregulated acute microglial inflammatory response and reduced glial scar formation (Xi et al., 2020). PCL/PSA hybrid nanofiber scaffolds encapsulating methylprednisolone (MP) implanted after transection SCI inhibited microglial inflammatory activation as evidenced by reduced secretion of TNF-ɑ and IL-6 (Zhang et al., 2018). As more advanced nanomaterials are developed, the ability to deliver APIs that modulate the microglial response has therapeutic potential in many CNS disorders.
2.5. Nanoparticle API delivery to oligodendrocytes
Oligodendrocytes generate myelin to increase the speed of propagation of axon potentials and provide metabolic support to neurons in the CNS (Simons and Nave, 2015). Unfortunately, oligodendrocytes are vulnerable to reactive oxygen species, hydrogen peroxide, and excitotoxicity from glutamate, and as such, are detrimentally impacted in a range of CNS disorders (Matute et al., 1997; Juurlink et al., 1998; Kuhn et al., 2019; Kenigsbuch et al., 2022; Pandey et al., 2022). The most common causes of oligodendrocyte death in the CNS are trauma, ischemia, or autoimmune attacks, such as multiple sclerosis. However, white matter pathology is also characteristic of other CNS diseases, including Alzheimer’s (Love, 2006; Fancy et al., 2011; Assinck et al., 2017; McAleese et al., 2017). Remyelination is a natural regenerative process that has been shown to prevent neurodegeneration and restore function (Duncan et al., 2009, 2018). Therefore, APIs have been studied in order to promote oligodendrocyte remyelination in CNS trauma and disease. Nanomaterial design for API delivery to oligodendrocytes is being studied to capitalize on the synergy between the advantageous properties of the API and those of the material (Russell and Lampe, 2017; Murphy and Lampe, 2018).
Nanoparticles, including polymer, lipid, extracellular vesicles, and inorganic nanoparticles, have been designed to deliver APIs to oligodendrocytes in models of TBI, EAE, focal CNS demyelination, cuprizone-induced demyelination, SCI, and ischemia (Table 1) (Rittchen et al., 2015; Kumar et al., 2018; Lin et al., 2019; Zhang et al., 2019; Osorio-Querejeta et al., 2020; Robinson et al., 2020; Pu et al., 2021). API payloads range from small molecule drugs to proteins, peptides, and miRNA (Fressinaud et al., 2020; Farhangi et al., 2023; Moura et al., 2023). The goal of most NP API therapies directed towards oligodendrocytes is to reduce myelin loss and induce remyelination after injury or disease in order to improve functional outcomes (Table 1). Importantly, NP API delivery to oligodendrocytes has been shown to rejuvenate myelin and improve outcomes in in vivo models of CNS injury and demyelinating disorders (Table 1). While NP API delivery to oligodendrocytes is the least studied of the three most prominent glia in the CNS, the functional benefits demonstrate the potential for developing these NP therapies to improve myelin outcomes in many different CNS pathologies in order to push these treatments towards the clinic.
2.6. Nanomaterial API delivery to oligodendrocytes
Nanomaterial strategies that target oligodendrocytes have focused on engineered nanofibrous materials due to their ability to provide an axon-like substrate to promote oligodendrocyte differentiation and myelination. Two fundamental studies pioneered this approach by demonstrating that rat oligodendrocyte progenitor cells (OPCs) cultured on electrospun nanofibers of diameter 500-800 nm proliferated and differentiated into oligodendrocytes and ensheathed the fibers, resembling myelination (Lee et al., 2012). The same group also reported similarly compacted myelination on polystyrene electrospun nanofibers cultured with rodent oligodendrocytes (Lee et al., 2013). Further studies have shown that nanofibers can guide oligodendrocyte orientation that more closely resembles in vivo morphologies, preferentially drive neural stem cells to oligodendrocytes, induce compact myelination, and protect oligodendrocytes following traumatic CNS injury (Tysseling-Mattiace et al., 2008; Cao et al., 2009; Li et al., 2014; Shah et al., 2014; Wang et al., 2015; Ehrlich et al., 2017; Hyysalo et al., 2017; Tupone et al., 2021; Zhang et al., 2022) (Table 2). The ability to mimic the in vivo environment and alter oligodendrocyte response has led to the design of nanomaterials that release APIs to act more specifically on these glia.
Further, hybrid PCL-gelatin nanofiber scaffolds, combined with polyaniline graphene nanocomposites, were incorporated in gelatin to lend conductive properties similar to axons. Chitosan nanoparticles loaded with T3 were incorporated into PCL for sustained release, and these nanohybrids led to the differentiation of rat bone marrow-derived neural stem cells towards an oligodendrocyte lineage with high expression of PDGFRα, O4. Olig2, O1, MOG, and MBP (Rasti Boroojeni et al., 2020). Primary oligodendrocytes isolated from B16 mice were able to myelinate aligned PCL nanofibers that released PDGF-AA, FGF2, BMP2, and BMP4 (Enz et al., 2019). PCL nanofibers loaded with miR-219, miR-338-3p, and miR-338-5p enhanced the differentiation of primary rat oligodendrocyte progenitor cells and their maturation into RIP+ oligodendrocytes (Diao et al., 2015).
Moreover, when hybrid PCL-PSA (polysialic acid) nanofiber scaffolds encapsulating glucocorticoid methylprednisolone were implanted into a transected rat SCI, the methylprednisolone delivered by the hybrid scaffold led to increased survival of oligodendrocytes and enhanced axonal myelination (Zhang et al., 2018). NT-3 is another API that has been used to act on oligodendrocytes, and fibrin nanofibrous scaffolds releasing NT-3 increase oligodendrocyte differentiation of neural progenitor cells (Willerth et al., 2008), while PCLEEP(PCL-co-ethyl ethylene phosphate)-collagen hybrid nanofibrous scaffolds releasing NT-3 showed extensive oligodendrocyte remyelination with MAG+ structures when implanted into a hemi-cervical incision induced rat spinal cord injury (Nguyen et al., 2017). Future oligodendrocyte-targeting nanomaterial design will seek to devise API-releasing strategies that specifically improve re-myelination after injury and improve myelin integrity in nervous system disorders.
3. Discussion
Nanomaterials designed to deliver APIs to CNS glia are beginning to emerge as viable therapies to improve outcomes in CNS disorders or after CNS injury. While many API-releasing nanomaterials are still being designed to focus their action on neurons (Kwon et al., 2016; Bruggeman et al., 2018; Zuidema et al., 2020), there is growing evidence that glia should not be overlooked as targets to improve outcomes in CNS injury and disorders (Tables 1, 2). However, in order for nanomaterial-mediated API delivery to glia to become a standard clinical intervention, further advances in engineering such materials are necessary.
Nanoparticle-mediated API delivery holds promise as a systemically administered approach to treat neurological disorders and CNS injuries where direct implantation into the site of action would be detrimental. For such treatments to become commonplace, one of the main areas of improvement is in traversing the BBB and delivering APIs directly to the relevant site of action. This will require a greater understanding of the mechanisms of nanoparticle permeation into the brain, including the importance of NP composition, size, charge, and shape, engineering the adsorbed biomolecular corona to not obstruct NP targeting, targeting the proper cell type once the NPs enter the brain, design of better-targeting moieties on the external surface of nanoparticles through such processes as in vivo phage display screening, exact API release timelines that induce desired outcomes, and, importantly, a more complete understanding of how to modulate glia to produce desired clinical outcomes (Salvati et al., 2013; Mann et al., 2016; Furtado et al., 2018; Waggoner et al., 2023; Wu et al., 2023). More personalized therapies can be envisioned, where each individual may respond to NPs differently. This may require a battery of different NP constructs to first be administered systematically, and once it is known which NP accumulates to the desired location, potential by using an imaging modality such as magnetic resonance imaging, then that NP construct can be incorporated with the desired API and delivered to the individual. Still, much research is needed to make NP-delivered APIs that act on glia a standard therapy to treat CNS disorders and injuries.
Nanomaterial-mediated API delivery has shown promise in areas where surgical intervention or injection into the site of action to act on glia can be used. These combinatorial nanomaterial-based therapies can simultaneously provide biophysical and biochemical cues to glial cells, eliciting their bioactive responses to facilitate robust neuronal repair and protection in the CNS. For these therapies to be used in the clinic, advances in API release paradigms must be realized, nanomaterial modulation of glia needs to be better understood, surgical implantation techniques optimized, degradation of the implanted material engineered based on the application, and the immune response accounted for not to impart adverse clinical outcomes (Nunes et al., 2012; Huang et al., 2017; Dai et al., 2021). We also envision the potential for nanomaterial therapies to be tailored to each patient to maximize therapeutic efficacy and minimize off-target adverse effects – by varying API release rates, compositions and coatings in nanomaterials design, and even the timeline of the surgical intervention. As advances in NPs, nanomaterials, and API design for targeting glia continue to be realized, there are many avenues for such therapies to improve clinical outcomes in CNS disorders and after CNS injury.
Author contributions
JS: Conceptualization, Investigation, Writing – original draft, Writing – review & editing. AH: Conceptualization, Funding acquisition, Investigation, Writing – original draft, Writing – review & editing. RG: Conceptualization, Funding acquisition, Project administration, Supervision, Writing – review & editing. JZ: Conceptualization, Funding acquisition, Project administration, Supervision, Writing – original draft, Writing – review & editing.
Funding
The author(s) declare financial support was received for the research, authorship, and/or publication of this article. This project received funding through the European Union’s Horizon 2021 research and innovation program under the Marie Skłodowska-Curie Actions grant agreement No. 101067770 (“PACMAN”). JZ is a Marie Skłodowska-Curie Actions Fellow. AH is supported by an NIH T32 Grant (#T32GM141865) and funding support is provided to RG via Veterans Affairs grants (I01RX003502 and I21RX004406), the National Science Foundation (2217513), and New York State Spinal Cord Injury Research Board (C38335GG).
Conflict of interest
The authors declare that the research was conducted in the absence of any commercial or financial relationships that could be construed as a potential conflict of interest.
The author(s) declared that they were an editorial board member of Frontiers, at the time of submission. This had no impact on the peer review process and the final decision.
Publisher’s note
All claims expressed in this article are solely those of the authors and do not necessarily represent those of their affiliated organizations, or those of the publisher, the editors and the reviewers. Any product that may be evaluated in this article, or claim that may be made by its manufacturer, is not guaranteed or endorsed by the publisher.
References
Aguzzi, A., Barres, B. A., and Bennett, M. L. (2013). Microglia: scapegoat, saboteur, or something else? Science 339, 156–161. doi: 10.1126/science.1227901
Allen, N. J., and Lyons, D. A. (2018). Glia as architects of central nervous system formation and function. Science 362, 181–185. doi: 10.1126/science.aat0473
Anderson, M. A., Ao, Y., and Sofroniew, M. V. (2014). Heterogeneity of reactive astrocytes. Neurosci. Lett. 565, 23–29. doi: 10.1016/j.neulet.2013.12.030
Assinck, P., Duncan, G. J., Plemel, J. R., Lee, M. J., Stratton, J. A., Manesh, S. B., et al. (2017). Myelinogenic plasticity of oligodendrocyte precursor cells following spinal cord contusion injury. J. Neurosci. 37, 8635–8654. doi: 10.1523/JNEUROSCI.2409-16.2017
Baghbanbashi, M., Yong, H. W., Zhang, I., Lotocki, V., Yuan, Z., Pazuki, G., et al. (2022). Stimuli-responsive miktoarm polymer-based formulations for fisetin delivery and regulatory effects in hyperactive human microglia. Macromol. Biosci. 22:e2200174. doi: 10.1002/mabi.202200174
Bighinati, A., Focarete, M. L., Gualandi, C., Pannella, M., Giuliani, A., Beggiato, S., et al. (2020). Improved functional recovery in rat spinal cord injury induced by a drug combination administered with an implantable polymeric delivery system. J. Neurotrauma 37, 1708–1719. doi: 10.1089/neu.2019.6949
Bruggeman, K. F., Williams, R. J., and Nisbet, D. R. (2018). Dynamic and responsive growth factor delivery from electrospun and hydrogel tissue engineering materials. Adv. Healthc. Mater. 7, 1–14. doi: 10.1002/adhm.201700836
Cahalane, C., Bonezzi, J., Shelestak, J., Clements, R., Boika, A., Yun, Y. H., et al. (2020). Targeted delivery of anti-inflammatory and imaging agents to microglial cells with polymeric nanoparticles. Mol. Pharm. 17, 1816–1826. doi: 10.1021/acs.molpharmaceut.9b00489
Cao, H., Liu, T., and Chew, S. Y. (2009). The application of nanofibrous scaffolds in neural tissue engineering. Adv. Drug Deliv. Rev. 61, 1055–1064. doi: 10.1016/j.addr.2009.07.009
Chen, D. W., and Foldvari, M. (2016). In vitro bioassay model for screening non-viral neurotrophic factor gene delivery systems for glaucoma treatment. Drug Deliv. Transl. Res. 6, 676–685. doi: 10.1007/s13346-016-0324-9
Chen, L., Wang, W., Lin, Z., Lu, Y., Chen, H., Li, B., et al. (2022). Conducting molybdenum sulfide/graphene oxide/polyvinyl alcohol nanocomposite hydrogel for repairing spinal cord injury. J. Nanobiotechnology 20:210. doi: 10.1186/s12951-022-01396-8
Cheng, Y., Zhang, Y., and Wu, H. (2021). Polymeric fibers as scaffolds for spinal cord injury: a systematic review. Front. Bioeng. Biotechnol. 9:807533. doi: 10.3389/fbioe.2021.807533
Cho, H., Kambhampati, S. P., Lai, M. J., Zhou, L., Lee, G., Xie, Y., et al. (2021). Dendrimer-triamcinolone acetonide reduces neuroinflammation, pathological angiogenesis, and neuroretinal dysfunction in ischemic retinopathy. Adv. Ther. (Weinh) 4:2000181. doi: 10.1002/adtp.202000181
Chowdhury, H. H., Cerqueira, S. R., Sousa, N., Oliveira, J. M., Reis, R. L., and Zorec, R. (2018). The uptake, retention and clearance of drug-loaded dendrimer nanoparticles in astrocytes – electrophysiological quantification. Biomater. Sci. 6, 388–397. doi: 10.1039/C7BM00886D
Christopherson, G. T., Song, H., and Mao, H. Q. (2009). The influence of fiber diameter of electrospun substrates on neural stem cell differentiation and proliferation. Biomaterials 30, 556–564. doi: 10.1016/j.biomaterials.2008.10.004
Ciciriello, A. J., Surnar, B., Medy, G. D., Su, X., Dhar, S., and Dumont, C. M. (2022). Biomaterial-targeted precision nanoparticle delivery to the injured spinal cord. Acta Biomater. 152, 532–545. doi: 10.1016/j.actbio.2022.08.077
Clementino, A. R., Marchi, C., Pozzoli, M., Bernini, F., Zimetti, F., and Sonvico, F. (2021). Anti-inflammatory properties of statin-loaded biodegradable lecithin/chitosan nanoparticles: a step toward nose-to-brain treatment of neurodegenerative diseases. Front. Pharmacol. 12:716380. doi: 10.3389/fphar.2021.716380
Clementino, A., Velasco-Estevez, M., Buttini, F., Sonvico, F., and Dev, K. K. (2021). Hybrid nanoparticles as a novel tool for regulating psychosine-induced neuroinflammation and demyelination in vitro and ex vivo. Neurotherapeutics 18, 2608–2622. doi: 10.1007/s13311-021-01109-3
Colonna, M., and Butovsky, O. (2017). Microglia function in the central nervous system during health and neurodegeneration. Annu. Rev. Immunol. 35, 441–468. doi: 10.1146/annurev-immunol-051116-052358
Dai, D., He, L., Chen, Y., and Zhang, C. (2021). Astrocyte responses to nanomaterials: functional changes, pathological changes and potential applications. Acta Biomater. 122, 66–81. doi: 10.1016/j.actbio.2020.12.013
Dai, Y., Wang, W. Z., Zhou, X. J., Li, L. L., Tang, Y. Y., Shao, M. H., et al. (2023). Biomimetic electrospun PLLA/PPSB nanofibrous scaffold combined with human neural stem cells for spinal cord injury repair. ACS Appl. Nano Mater. 6, 5980–5993. doi: 10.1021/acsanm.3c00374
Diao, H. J., Low, W. C., Milbreta, U., Lu, Q. R., and Chew, S. Y. (2015). Nanofiber-mediated microRNA delivery to enhance differentiation and maturation of oligodendroglial precursor cells. J. Control. Release 208, 85–92. doi: 10.1016/j.jconrel.2015.03.005
Duncan, I. D., Brower, A., Kondo, Y., Curlee, J. F. Jr., and Schultz, R. D. (2009). Extensive remyelination of the CNS leads to functional recovery. Proc. Natl. Acad. Sci. U. S. A. 106, 6832–6836. doi: 10.1073/pnas.0812500106
Duncan, I. D., Radcliff, A. B., Heidari, M., Kidd, G., August, B. K., and Wierenga, L. A. (2018). The adult oligodendrocyte can participate in remyelination. Proc. Natl. Acad. Sci. U. S. A. 115, E11807–E11816. doi: 10.1073/pnas.1808064115
Ehrlich, M., Mozafari, S., Glatza, M., Starost, L., Velychko, S., Hallmann, A. L., et al. (2017). Rapid and efficient generation of oligodendrocytes from human induced pluripotent stem cells using transcription factors. Proc. Natl. Acad. Sci. U. S. A. 114, E2243–E2252. doi: 10.1073/pnas.1614412114
Ellert-Miklaszewska, A., Ochocka, N., Maleszewska, M., Ding, L., Laurini, E., Jiang, Y., et al. (2019). Efficient and innocuous delivery of small interfering RNA to microglia using an amphiphilic dendrimer nanovector. Nanomedicine (Lond.) 14, 2441–2458. doi: 10.2217/nnm-2019-0176
Enz, L. S., Zeis, T., Hauck, A., Linington, C., and Schaeren-Wiemers, N. (2019). Combinatory multifactor treatment effects on primary nanofiber oligodendrocyte cultures. Cells 8:1422. doi: 10.3390/cells8111422
Escartin, C., Guillemaud, O., and Carrillo-de Sauvage, M. A. (2019). Questions and (some) answers on reactive astrocytes. Glia 67, 2221–2247. doi: 10.1002/glia.23687
Fabbri, R., Saracino, E., Treossi, E., Zamboni, R., Palermo, V., and Benfenati, V. (2021). Graphene glial-interfaces: challenges and perspectives. Nanoscale 13, 4390–4407. doi: 10.1039/D0NR07824G
Fancy, S. P., Harrington, E. P., Yuen, T. J., Silbereis, J. C., Zhao, C., Baranzini, S. E., et al. (2011). Axin2 as regulatory and therapeutic target in newborn brain injury and remyelination. Nat. Neurosci. 14, 1009–1016. doi: 10.1038/nn.2855
Farhangi, S., Karimi, E., Khajeh, K., Hosseinkhani, S., and Javan, M. (2023). Peptide mediated targeted delivery of gold nanoparticles into the demyelination site ameliorates myelin impairment and gliosis. Nanomedicine 47:102609. doi: 10.1016/j.nano.2022.102609
Fressinaud, C., Thomas, O., Umerska, A. M., and Saulnier, P. (2020). Lipid nanoparticles vectorized with NFL-TBS.40-63 peptide target oligodendrocytes and promote neurotrophin-3 effects after demyelination in vitro. Neurochem. Res. 45, 2732–2748. doi: 10.1007/s11064-020-03122-y
Furtado, D., Bjornmalm, M., Ayton, S., Bush, A. I., Kempe, K., and Caruso, F. (2018). Overcoming the blood-brain barrier: the role of nanomaterials in treating neurological diseases. Adv. Mater. 30:e1801362. doi: 10.1002/adma.201801362
Ganbold, T., Bao, Q., Xiao, H., Zurgaanjin, D., Liu, C., Han, S., et al. (2022). Peptidomimetic lipid-nanoparticle-mediated knockdown of TLR4 in CNS protects against cerebral ischemia/reperfusion injury in mice. Nanomaterials (Basel) 12:2072. doi: 10.3390/nano12122072
Ganbold, T., Bao, Q., Zandan, J., Hasi, A., and Baigude, H. (2020). Modulation of microglia polarization through silencing of NF-kappaB p65 by functionalized curdlan nanoparticle-mediated RNAi. ACS Appl. Mater. Interfaces 12, 11363–11374. doi: 10.1021/acsami.9b23004
Gao, X., Cheng, W., Zhang, X., Zhou, Z., Ding, Z., Zhou, X., et al. (2022). Nerve growth factor-laden anisotropic silk nanofiber hydrogels to regulate neuronal/astroglial differentiation for scarless spinal cord repair. ACS Appl. Mater. Interfaces 14, 3701–3715. doi: 10.1021/acsami.1c19229
Gao, Z. S., Zhang, C. J., Xia, N., Tian, H., Li, D. Y., Lin, J. Q., et al. (2021). Berberine-loaded M2 macrophage-derived exosomes for spinal cord injury therapy. Acta Biomater. 126, 211–223. doi: 10.1016/j.actbio.2021.03.018
Gonzalez, P., Gonzalez-Fernandez, C., Maqueda, A., Perez, V., Escalera-Anzola, S., Rodriguez de Lope, A., et al. (2022). Silk-elastin-like polymers for acute intraparenchymal treatment of the traumatically injured spinal cord: a first systematic experimental approach. Pharmaceutics. 14:2713. doi: 10.3390/pharmaceutics14122713
Gu, Y., Zhang, R., Jiang, B., Xu, X., Guan, J. J., Jiang, X. J., et al. (2022). Repair of spinal cord injury by inhibition of PLK4 expression through local delivery of siRNA-loaded nanoparticles. J. Mol. Neurosci. 72, 544–554. doi: 10.1007/s12031-021-01871-1
Guo, S., Cazarez-Marquez, F., Jiao, H., Foppen, E., Korpel, N. L., Grootemaat, A. E., et al. (2022). Specific silencing of microglial gene expression in the rat brain by nanoparticle-based small interfering RNA delivery. ACS Appl. Mater. Interfaces 14, 5066–5079. doi: 10.1021/acsami.1c22434
Guo, J., Su, H., Zeng, Y., Liang, Y. X., Wong, W. M., Ellis-Behnke, R. G., et al. (2007). Reknitting the injured spinal cord by self-assembling peptide nanofiber scaffold. Nanomed-Nanotechnol. 3, 311–321. doi: 10.1016/j.nano.2007.09.003
Haggerty, A. E., Maldonado-Lasuncion, I., Nitobe, Y., Yamane, K., Marlow, M. M., You, H., et al. (2022). The effects of the combination of mesenchymal stromal cells and nanofiber-hydrogel composite on repair of the contused spinal cord. Cells-Basel. 11:1137. doi: 10.3390/cells11071137
Hasel, P., and Liddelow, S. A. (2021). Astrocytes. Curr. Biol. 31, R326–R327. doi: 10.1016/j.cub.2021.01.056
He, X., Xie, J., Zhang, J., Wang, X., Jia, X., Yin, H., et al. (2022). Acid-responsive dual-targeted nanoparticles encapsulated aspirin rescue the immune activation and phenotype in autism spectrum disorder. Adv. Sci. (Weinh). 9:e2104286. doi: 10.1002/advs.202104286
Hernando, S., Nikolakopoulou, P., Voulgaris, D., Hernandez, R. M., Igartua, M., and Herland, A. (2022). Dual effect of TAT functionalized DHAH lipid nanoparticles with neurotrophic factors in human BBB and microglia cultures. Fluids Barriers CNS. 19:22. doi: 10.1186/s12987-022-00315-1
Hollinger, K. R., Sharma, A., Tallon, C., Lovell, L., Thomas, A. G., Zhu, X., et al. (2022). Dendrimer-2PMPA selectively blocks upregulated microglial GCPII activity and improves cognition in a mouse model of multiple sclerosis. Nano 6, 126–142. doi: 10.7150/ntno.63158
Holmkvist, A. D., Agorelius, J., Forni, M., Nilsson, U. J., Linsmeier, C. E., and Schouenborg, J. (2020). Local delivery of minocycline-loaded PLGA nanoparticles from gelatin-coated neural implants attenuates acute brain tissue responses in mice. J. Nanobiotechnology 18:27. doi: 10.1186/s12951-020-0585-9
Huang, L., Hu, J., Huang, S., Wang, B., Siaw-Debrah, F., Nyanzu, M., et al. (2017). Nanomaterial applications for neurological diseases and central nervous system injury. Prog. Neurobiol. 157, 29–48. doi: 10.1016/j.pneurobio.2017.07.003
Huang, W., Wang, L., Zou, Y., Ding, X., Geng, X., Li, J., et al. (2022). Preparation of gastrodin-modified dendrimer-entrapped gold nanoparticles as a drug delivery system for cerebral ischemia-reperfusion injury. Brain Behav. 12:e2810. doi: 10.1002/brb3.2810
Hyysalo, A., Ristola, M., Joki, T., Honkanen, M., Vippola, M., and Narkilahti, S. (2017). Aligned poly(epsilon-caprolactone) nanofibers guide the orientation and migration of human pluripotent stem cell-derived neurons, astrocytes, and oligodendrocyte precursor cells in vitro. Macromol. Biosci. 17, 1–8. doi: 10.1002/mabi.201600517
Iezzi, R., Guru, B. R., Glybina, I. V., Mishra, M. K., Kennedy, A., and Kannan, R. M. (2012). Dendrimer-based targeted intravitreal therapy for sustained attenuation of neuroinflammation in retinal degeneration. Biomaterials 33, 979–988. doi: 10.1016/j.biomaterials.2011.10.010
Ishida, T., Kawada, K., Jobu, K., Morisawa, S., Kawazoe, T., Nishimura, S., et al. (2023). Exosome-like nanoparticles derived from Allium tuberosum prevent neuroinflammation in microglia-like cells. J. Pharm. Pharmacol. 75, 1322–1331. doi: 10.1093/jpp/rgad062
Jarrin, S., Cabre, S., and Dowd, E. (2021). The potential of biomaterials for central nervous system cellular repair. Neurochem. Int. 144:104971. doi: 10.1016/j.neuint.2021.104971
Juurlink, B. H., Thorburne, S. K., and Hertz, L. (1998). Peroxide-scavenging deficit underlies oligodendrocyte susceptibility to oxidative stress. Glia 22, 371–378. doi: 10.1002/(SICI)1098-1136(199804)22:4<371::AID-GLIA6>3.0.CO;2-6
Kalashnikova, I., Cambell, H., Kolpek, D., and Park, J. (2023). Optimization and characterization of miRNA-129-5p-encapsulated poly (lactic-co-glycolic acid) nanoparticles to reprogram activated microglia. Nanoscale Adv. 5, 3439–3452. doi: 10.1039/D3NA00149K
Kannan, S., Dai, H., Navath, R. S., Balakrishnan, B., Jyoti, A., Janisse, J., et al. (2012). Dendrimer-based postnatal therapy for neuroinflammation and cerebral palsy in a rabbit model. Sci. Transl. Med. 4:130ra46. doi: 10.1126/scitranslmed.3003162
Kenigsbuch, M., Bost, P., Halevi, S., Chang, Y., Chen, S., Ma, Q., et al. (2022). A shared disease-associated oligodendrocyte signature among multiple CNS pathologies. Nat. Neurosci. 25, 876–886. doi: 10.1038/s41593-022-01104-7
Khoury, E. S., Sharma, A., Ramireddy, R. R., Thomas, A. G., Alt, J., Fowler, A., et al. (2020). Dendrimer-conjugated glutaminase inhibitor selectively targets microglial glutaminase in a mouse model of Rett syndrome. Theranostics. 10, 5736–5748. doi: 10.7150/thno.41714
Kim, H., Choi, B., Lim, H., Min, H., Oh, J. H., Choi, S., et al. (2017). Polyamidoamine dendrimer-conjugated triamcinolone acetonide attenuates nerve injury-induced spinal cord microglia activation and mechanical allodynia. Mol. Pain 13:1744806917697006. doi: 10.1177/1744806917697006
Kim, S. I., Shin, J., Tran, Q., Park, H., Kwon, H. H., Shin, N., et al. (2021). Application of PLGA nanoparticles to enhance the action of duloxetine on microglia in neuropathic pain. Biomater. Sci. 9, 6295–6307. doi: 10.1039/D1BM00486G
Kong, F., Liu, G., Zhou, S., Guo, J., Chen, S., and Wang, Z. (2017). Superior transfection efficiency of phagocytic astrocytes by large chitosan/DNA nanoparticles. Int. J. Biol. Macromol. 105, 1473–1481. doi: 10.1016/j.ijbiomac.2017.06.061
Koss, K. M., Churchward, M. A., Nguyen, A. T., Yager, J. Y., Todd, K. G., and Unsworth, L. D. (2016). Brain biocompatibility and microglia response towards engineered self-assembling (RADA)4 nanoscaffolds. Acta Biomater. 35, 127–137. doi: 10.1016/j.actbio.2016.02.001
Kuhn, S., Gritti, L., Crooks, D., and Dombrowski, Y. (2019). Oligodendrocytes in development, myelin generation and beyond. Cells 8:1424. doi: 10.3390/cells8111424
Kumar, P., Sharma, G., Gupta, V., Kaur, R., Thakur, K., Malik, R., et al. (2018). Preclinical explorative assessment of dimethyl fumarate-based biocompatible nanolipoidal carriers for the management of multiple sclerosis. ACS Chem. Neurosci. 9, 1152–1158. doi: 10.1021/acschemneuro.7b00519
Kwon, E. J., Skalak, M., Lo, B. R., and Bhatia, S. N. (2016). Neuron-targeted nanoparticle for siRNA delivery to traumatic brain injuries. ACS Nano 10, 7926–7933. doi: 10.1021/acsnano.6b03858
Lee, S., Chong, S. Y., Tuck, S. J., Corey, J. M., and Chan, J. R. (2013). A rapid and reproducible assay for modeling myelination by oligodendrocytes using engineered nanofibers. Nat. Protoc. 8, 771–782. doi: 10.1038/nprot.2013.039
Lee, S., Leach, M. K., Redmond, S. A., Chong, S. Y., Mellon, S. H., Tuck, S. J., et al. (2012). A culture system to study oligodendrocyte myelination processes using engineered nanofibers. Nat. Methods 9, 917–922. doi: 10.1038/nmeth.2105
Lee, S., Shin, H. J., Noh, C., Kim, S. I., Ko, Y. K., Lee, S. Y., et al. (2021). IKBKB siRNA-encapsulated poly (lactic-co-glycolic acid) nanoparticles diminish neuropathic pain by inhibiting microglial activation. Int. J. Mol. Sci. 22:5657. doi: 10.3390/ijms22115657
Lee, H. G., Wheeler, M. A., and Quintana, F. J. (2022). Function and therapeutic value of astrocytes in neurological diseases. Nat. Rev. Drug Discov. 21, 339–358. doi: 10.1038/s41573-022-00390-x
Li, Q., and Barres, B. A. (2018). Microglia and macrophages in brain homeostasis and disease. Nat. Rev. Immunol. 18, 225–242. doi: 10.1038/nri.2017.125
Li, Y., Ceylan, M., Shrestha, B., Wang, H., Lu, Q. R., Asmatulu, R., et al. (2014). Nanofibers support oligodendrocyte precursor cell growth and function as a neuron-free model for myelination study. Biomacromolecules 15, 319–326. doi: 10.1021/bm401558c
Li, X., Zhang, C., Haggerty, A. E., Yan, J., Lan, M., Seu, M., et al. (2020). The effect of a nanofiber-hydrogel composite on neural tissue repair and regeneration in the contused spinal cord. Biomaterials 245:119978. doi: 10.1016/j.biomaterials.2020.119978
Liao, K., Niu, F., Dagur, R. S., He, M., Tian, C., and Hu, G. (2020). Intranasal delivery of lincRNA-Cox2 siRNA loaded extracellular vesicles decreases lipopolysaccharide-induced microglial proliferation in mice. J. Neuroimmune Pharmacol. 15, 390–399. doi: 10.1007/s11481-019-09864-z
Liddelow, S. A., and Barres, B. A. (2017). Reactive astrocytes: production, function, and therapeutic potential. Immunity 46, 957–967. doi: 10.1016/j.immuni.2017.06.006
Lin, Y., Li, C., Li, J., Deng, R., Huang, J., Zhang, Q., et al. (2019). NEP(1-40)-modified human serum albumin nanoparticles enhance the therapeutic effect of methylprednisolone against spinal cord injury. J. Nanobiotechnology 17:12. doi: 10.1186/s12951-019-0449-3
Liu, Y., Hsu, Y. H., Huang, A. P., and Hsu, S. H. (2020). Semi-interpenetrating polymer network of Hyaluronan and chitosan self-healing hydrogels for central nervous system repair. ACS Appl. Mater. Interfaces 12, 40108–40120. doi: 10.1021/acsami.0c11433
Liu, P., Zhang, T., Chen, Q., Li, C., Chu, Y., Guo, Q., et al. (2021). Biomimetic dendrimer-peptide conjugates for early multi-target therapy of Alzheimer's disease by inflammatory microenvironment modulation. Adv. Mater. 33:e2100746. doi: 10.1002/adma.202100746
Love, S. (2006). Demyelinating diseases. J. Clin. Pathol. 59, 1151–1159. doi: 10.1136/jcp.2005.031195
Lozic, I., Hartz, R. V., Bartlett, C. A., Shaw, J. A., Archer, M., Naidu, P. S., et al. (2016). Enabling dual cellular destinations of polymeric nanoparticles for treatment following partial injury to the central nervous system. Biomaterials 74, 200–216. doi: 10.1016/j.biomaterials.2015.10.001
Lu, Y. M., Huang, J. Y., Wang, H., Lou, X. F., Liao, M. H., Hong, L. J., et al. (2014). Targeted therapy of brain ischaemia using Fas ligand antibody conjugated PEG-lipid nanoparticles. Biomaterials 35, 530–537. doi: 10.1016/j.biomaterials.2013.09.093
Ma, J., Li, J., Wang, X., Li, M., Teng, W., Tao, Z., et al. (2023). GDNF-loaded Polydopamine nanoparticles-based anisotropic scaffolds promote spinal cord repair by modulating inhibitory microenvironment. Adv. Healthc. Mater. 12:e2202377. doi: 10.1002/adhm.202202377
Madhusudanan, P., Raju, G., and Shankarappa, S. (2020). Hydrogel systems and their role in neural tissue engineering. J. R. Soc. Interface 17:20190505. doi: 10.1098/rsif.2019.0505
Mann, A. P., Scodeller, P., Hussain, S., Joo, J., Kwon, E., Braun, G. B., et al. (2016). A peptide for targeted, systemic delivery of imaging and therapeutic compounds into acute brain injuries. Nat. Commun. 7:11980. doi: 10.1038/ncomms11980
Matute, C., Sanchez-Gomez, M. V., Martinez-Millan, L., and Miledi, R. (1997). Glutamate receptor-mediated toxicity in optic nerve oligodendrocytes. Proc. Natl. Acad. Sci. U. S. A. 94, 8830–8835. doi: 10.1073/pnas.94.16.8830
McAleese, K. E., Walker, L., Graham, S., Moya, E. L. J., Johnson, M., Erskine, D., et al. (2017). Parietal white matter lesions in Alzheimer's disease are associated with cortical neurodegenerative pathology, but not with small vessel disease. Acta Neuropathol. 134, 459–473. doi: 10.1007/s00401-017-1738-2
Montenegro, L., Campisi, A., Sarpietro, M. G., Carbone, C., Acquaviva, R., Raciti, G., et al. (2011). In vitro evaluation of idebenone-loaded solid lipid nanoparticles for drug delivery to the brain. Drug Dev. Ind. Pharm. 37, 737–746. doi: 10.3109/03639045.2010.539231
Moura, R. P., Carvalho, E. D., Martins, C., des Rieux, A., Pêgo, A. P., and Sarmento, B. (2023). Functionalized retinoic acid lipid nanocapsules promotes a two-front attack on inflammation and lack of demyelination on neurodegenerative disorders. J. Control. Release 358, 43–58. doi: 10.1016/j.jconrel.2023.04.034
Murphy, N. P., and Lampe, K. J. (2018). Fabricating PLGA microparticles with high loads of the small molecule antioxidant N-acetylcysteine that rescue oligodendrocyte progenitor cells from oxidative stress. Biotechnol. Bioeng. 115, 246–256. doi: 10.1002/bit.26443
Nance, E., Kambhampati, S. P., Smith, E. S., Zhang, Z., Zhang, F., Singh, S., et al. (2017). Dendrimer-mediated delivery of N-acetyl cysteine to microglia in a mouse model of Rett syndrome. J. Neuroinflammation 14:252. doi: 10.1186/s12974-017-1004-5
Nance, E., Porambo, M., Zhang, F., Mishra, M. K., Buelow, M., Getzenberg, R., et al. (2015). Systemic dendrimer-drug treatment of ischemia-induced neonatal white matter injury. J. Control. Release 214, 112–120. doi: 10.1016/j.jconrel.2015.07.009
Narsineni, L., Chen, D. W., and Foldvari, M. (2023). BDNF gene delivery to the retina by cell adhesion peptide-conjugated gemini nanoplexes in vivo. J. Control. Release 359, 244–256. doi: 10.1016/j.jconrel.2023.05.033
Newland, B., Aied, A., Pinoncely, A. V., Zheng, Y., Zhao, T., Zhang, H., et al. (2014). Untying a nanoscale knotted polymer structure to linear chains for efficient gene delivery in vitro and to the brain. Nanoscale 6, 7526–7533. doi: 10.1039/C3NR06737H
Nguyen, L. H., Gao, M., Lin, J., Wu, W., Wang, J., and Chew, S. Y. (2017). Three-dimensional aligned nanofibers-hydrogel scaffold for controlled non-viral drug/gene delivery to direct axon regeneration in spinal cord injury treatment. Sci. Rep. 7:42212. doi: 10.1038/srep42212
Nunes, A., Al-Jamal, K. T., and Kostarelos, K. (2012). Therapeutics, imaging and toxicity of nanomaterials in the central nervous system. J. Control. Release 161, 290–306. doi: 10.1016/j.jconrel.2012.03.026
Osorio-Querejeta, I., Carregal-Romero, S., Ayerdi-Izquierdo, A., Mäger, I., Nash, L. A., Wood, M., et al. (2020). MiR-219a-5p enriched extracellular vesicles induce OPC differentiation and EAE improvement more efficiently than liposomes and polymeric nanoparticles. Pharmaceutics. 12:186. doi: 10.3390/pharmaceutics12020186
Pandey, S., Shen, K., Lee, S. H., Shen, Y. A., Wang, Y., Otero-Garcia, M., et al. (2022). Disease-associated oligodendrocyte responses across neurodegenerative diseases. Cell Rep. 40:111189. doi: 10.1016/j.celrep.2022.111189
Papa, S., Caron, I., Erba, E., Panini, N., De Paola, M., Mariani, A., et al. (2016). Early modulation of pro-inflammatory microglia by minocycline loaded nanoparticles confers long lasting protection after spinal cord injury. Biomaterials 75, 13–24. doi: 10.1016/j.biomaterials.2015.10.015
Papa, S., Rossi, F., Ferrari, R., Mariani, A., De Paola, M., Caron, I., et al. (2013). Selective nanovector mediated treatment of activated proinflammatory microglia/macrophages in spinal cord injury. ACS Nano 7, 9881–9895. doi: 10.1021/nn4036014
Patel, T., Zhou, J., Piepmeier, J. M., and Saltzman, W. M. (2012). Polymeric nanoparticles for drug delivery to the central nervous system. Adv. Drug Deliv. Rev. 64, 701–705. doi: 10.1016/j.addr.2011.12.006
Pekny, M., Pekna, M., Messing, A., Steinhauser, C., Lee, J. M., Parpura, V., et al. (2016). Astrocytes: a central element in neurological diseases. Acta Neuropathol. 131, 323–345. doi: 10.1007/s00401-015-1513-1
Perumal, V., Ravula, A. R., Shao, N., and Chandra, N. (2023). Effect of minocycline and its nano-formulation on central auditory system in blast-induced hearing loss rat model. J Otol. 18, 38–48. doi: 10.1016/j.joto.2022.09.002
Pires, L. R., Rocha, D. N., Ambrosio, L., and Pego, A. P. (2015). The role of the surface on microglia function: implications for central nervous system tissue engineering. J. R. Soc. Interface 12:20141224. doi: 10.1098/rsif.2014.1224
Porkolab, G., Meszaros, M., Toth, A., Szecsko, A., Harazin, A., Szegletes, Z., et al. (2020). Combination of alanine and glutathione as targeting ligands of nanoparticles enhances cargo delivery into the cells of the neurovascular unit. Pharmaceutics. 12:635. doi: 10.3390/pharmaceutics12070635
Proulx, J., Joshi, C., Vijayaraghavalu, S., Saraswathy, M., Labhasetwar, V., Ghorpade, A., et al. (2020). Arginine-modified polymers facilitate poly (Lactide-co-Glycolide)-based nanoparticle gene delivery to primary human astrocytes. Int. J. Nanomedicine 15, 3639–3647. doi: 10.2147/IJN.S250865
Pu, H., Wang, Y., Yang, T., Leak, R. K., Stetler, R. A., Yu, F., et al. (2023). Interleukin-4 mitigates anxiety-like behavior and loss of neurons and fiber tracts in limbic structures in a microglial PPARgamma-dependent manner after traumatic brain injury. Neurobiol. Dis. 180:106078. doi: 10.1016/j.nbd.2023.106078
Pu, H., Zheng, X., Jiang, X., Mu, H., Xu, F., Zhu, W., et al. (2021). Interleukin-4 improves white matter integrity and functional recovery after murine traumatic brain injury via oligodendroglial PPARgamma. J. Cereb. Blood Flow Metab. 41, 511–529. doi: 10.1177/0271678X20941393
Puhl, D. L., Funnell, J. L., Nelson, D. W., Gottipati, M. K., and Gilbert, R. J. (2020). Electrospun Fiber scaffolds for engineering glial cell behavior to promote neural regeneration. Bioengineering (Basel) 8:4. doi: 10.3390/bioengineering8010004
Puhl, D. L., Mohanraj, D., Nelson, D. W., and Gilbert, R. J. (2022). Designing electrospun fiber platforms for efficient delivery of genetic material and genome editing tools. Adv. Drug Deliv. Rev. 183:114161. doi: 10.1016/j.addr.2022.114161
Ransohoff, R. M. (2016a). A polarizing question: do M1 and M2 microglia exist? Nat. Neurosci. 19, 987–991. doi: 10.1038/nn.4338
Ransohoff, R. M. (2016b). How neuroinflammation contributes to neurodegeneration. Science 353, 777–783. doi: 10.1126/science.aag2590
Raspa, A., Carminati, L., Pugliese, R., Fontana, F., and Gelain, F. (2021). Self-assembling peptide hydrogels for the stabilization and sustained release of active chondroitinase ABC in vitro and in spinal cord injuries. J. Control. Release 330, 1208–1219. doi: 10.1016/j.jconrel.2020.11.027
Rasti Boroojeni, F., Mashayekhan, S., Abbaszadeh, H. A., Ansarizadeh, M., Khoramgah, M. S., and Rahimi, M. V. (2020). Bioinspired nanofiber scaffold for differentiating bone marrow-derived neural stem cells to oligodendrocyte-like cells: design, fabrication, and characterization. Int. J. Nanomedicine 15, 3903–3920. doi: 10.2147/IJN.S248509
Rittchen, S., Boyd, A., Burns, A., Park, J., Fahmy, T. M., Metcalfe, S., et al. (2015). Myelin repair in vivo is increased by targeting oligodendrocyte precursor cells with nanoparticles encapsulating leukaemia inhibitory factor (LIF). Biomaterials 56, 78–85. doi: 10.1016/j.biomaterials.2015.03.044
Robinson, A. P., Zhang, J. Z., Titus, H. E., Karl, M., Merzliakov, M., Dorfman, A. R., et al. (2020). Nanocatalytic activity of clean-surfaced, faceted nanocrystalline gold enhances remyelination in animal models of multiple sclerosis. Sci. Rep. 10:1936. doi: 10.1038/s41598-020-58709-w
Russell, L. N., and Lampe, K. J. (2017). Oligodendrocyte precursor cell viability, proliferation, and morphology is dependent on mesh size and storage Modulus in 3D poly(ethylene glycol)-based hydrogels. ACS Biomater Sci. Eng. 3, 3459–3468. doi: 10.1021/acsbiomaterials.7b00374
Sabourian, P., Frounchi, M., Kiani, S., Mashayekhan, S., Kheirabadi, M. Z., Heydari, Y., et al. (2023). Targeting reactive astrocytes by pH-responsive ligand-bonded polymeric nanoparticles in spinal cord injury. Drug Deliv. Transl. Res. 13, 1842–1855. doi: 10.1007/s13346-023-01300-3
Salvati, A., Pitek, A. S., Monopoli, M. P., Prapainop, K., Bombelli, F. B., Hristov, D. R., et al. (2013). Transferrin-functionalized nanoparticles lose their targeting capabilities when a biomolecule corona adsorbs on the surface. Nat. Nanotechnol. 8, 137–143. doi: 10.1038/nnano.2012.237
Sankowski, R., Monaco, G., and Prinz, M. (2022). Evaluating microglial phenotypes using single-cell technologies. Trends Neurosci. 45, 133–144. doi: 10.1016/j.tins.2021.11.001
Saxena, T., Loomis, K. H., Pai, S. B., Karumbaiah, L., Gaupp, E., Patil, K., et al. (2015). Nanocarrier-mediated inhibition of macrophage migration inhibitory factor attenuates secondary injury after spinal cord injury. ACS Nano 9, 1492–1505. doi: 10.1021/nn505980z
Schaub, N. J., Johnson, C. D., Cooper, B., and Gilbert, R. J. (2016). Electrospun fibers for spinal cord injury research and regeneration. J. Neurotrauma 33, 1405–1415. doi: 10.1089/neu.2015.4165
Sepasi, T., Ghadiri, T., Ebrahimi-Kalan, A., Bani, F., Talebi, M., Rahbarghazi, R., et al. (2023). CDX-modified chitosan nanoparticles remarkably reduce therapeutic dose of fingolimod in the EAE model of mice. Int. J. Pharm. 636:122815. doi: 10.1016/j.ijpharm.2023.122815
Serafin, A., Rubio, M. C., Carsi, M., Ortiz-Serna, P., Sanchis, M. J., Garg, A. K., et al. (2022). Electroconductive PEDOT nanoparticle integrated scaffolds for spinal cord tissue repair. Biomater Res. 26:63. doi: 10.1186/s40824-022-00310-5
Serramia, M. J., Alvarez, S., Fuentes-Paniagua, E., Clemente, M. I., Sanchez-Nieves, J., Gomez, R., et al. (2015). In vivo delivery of siRNA to the brain by carbosilane dendrimer. J. Control. Release 200, 60–70. doi: 10.1016/j.jconrel.2014.12.042
Shah, S., Yin, P. T., Uehara, T. M., Chueng, S. T., Yang, L., and Lee, K. B. (2014). Guiding stem cell differentiation into oligodendrocytes using graphene-nanofiber hybrid scaffolds. Adv. Mater. 26, 3673–3680. doi: 10.1002/adma.201400523
Sharma, R., Kambhampati, S. P., Zhang, Z., Sharma, A., Chen, S., Duh, E. I., et al. (2020). Dendrimer mediated targeted delivery of sinomenine for the treatment of acute neuroinflammation in traumatic brain injury. J. Control. Release 323, 361–375. doi: 10.1016/j.jconrel.2020.04.036
Sharma, R., Kim, S. Y., Sharma, A., Zhang, Z., Kambhampati, S. P., Kannan, S., et al. (2017). Activated microglia targeting dendrimer-minocycline conjugate as therapeutics for neuroinflammation. Bioconjug. Chem. 28, 2874–2886. doi: 10.1021/acs.bioconjchem.7b00569
Shin, H. J., Lee, K. Y., Kang, J. W., Choi, S. G., Kim, D. W., and Yi, Y. Y. (2022). Perampanel reduces brain damage via induction of M2 microglia in a neonatal rat stroke model. Int. J. Nanomedicine 17, 2791–2804. doi: 10.2147/IJN.S361377
Shu, B., Sun, X., Liu, R., Jiang, F., Yu, H., Xu, N., et al. (2019). Restoring electrical connection using a conductive biomaterial provides a new therapeutic strategy for rats with spinal cord injury. Neurosci. Lett. 692, 33–40. doi: 10.1016/j.neulet.2018.10.031
Simons, M., and Nave, K. A. (2015). Oligodendrocytes: myelination and axonal support. Cold Spring Harb. Perspect. Biol. 8:a020479. doi: 10.1101/cshperspect.a020479
Sofroniew, M. V., and Vinters, H. V. (2010). Astrocytes: biology and pathology. Acta Neuropathol. 119, 7–35. doi: 10.1007/s00401-009-0619-8
Somjen, G. G. (1988). Nervenkitt: notes on the history of the concept of neuroglia. Glia 1, 2–9. doi: 10.1002/glia.440010103
Song, Q., Jiang, Z. Y., Li, N., Liu, P., Liu, L. W., Tang, M. L., et al. (2014). Anti-inflammatory effects of three-dimensional graphene foams cultured with microglial cells. Biomaterials 35, 6930–6940. doi: 10.1016/j.biomaterials.2014.05.002
Sun, X., Zhang, C., Xu, J., Zhai, H., Liu, S., Xu, Y., et al. (2020). Neurotrophin-3-loaded multichannel nanofibrous scaffolds promoted anti-inflammation, neuronal differentiation, and functional recovery after spinal cord injury. ACS Biomater Sci. Eng. 6, 1228–1238. doi: 10.1021/acsbiomaterials.0c00023
Surnar, B., Basu, U., Banik, B., Ahmad, A., Marples, B., Kolishetti, N., et al. (2018). Nanotechnology-mediated crossing of two impermeable membranes to modulate the stars of the neurovascular unit for neuroprotection. Proc. Natl. Acad. Sci. U. S. A. 115, E12333–E12342. doi: 10.1073/pnas.1816429115
Surnar, B., Shah, A. S., Park, M., Kalathil, A. A., Kamran, M. Z., Ramirez Jaime, R., et al. (2021). Brain-accumulating nanoparticles for assisting astrocytes to reduce human immunodeficiency virus and drug abuse-induced Neuroinflammation and oxidative stress. ACS Nano 15, 15741–15753. doi: 10.1021/acsnano.0c09553
Sweeney, M. D., Kisler, K., Montagne, A., Toga, A. W., and Zlokovic, B. V. (2018a). The role of brain vasculature in neurodegenerative disorders. Nat. Neurosci. 21, 1318–1331. doi: 10.1038/s41593-018-0234-x
Sweeney, M. D., Sagare, A. P., and Zlokovic, B. V. (2018b). Blood-brain barrier breakdown in Alzheimer disease and other neurodegenerative disorders. Nat. Rev. Neurol. 14, 133–150. doi: 10.1038/nrneurol.2017.188
Tian, L., Prabhakaran, M. P., and Ramakrishna, S. (2015). Strategies for regeneration of components of nervous system: scaffolds, cells and biomolecules. Regen. Biomater. 2, 31–45. doi: 10.1093/rb/rbu017
Tickle, J. A., and Chari, D. M. (2019). Less is more: investigating the influence of cellular nanoparticle load on transfection outcomes in neural cells. J. Tissue Eng. Regen. Med. 13, 1732–1737. doi: 10.1002/term.2909
Tomassy, G. S., Dershowitz, L. B., and Arlotta, P. (2016). Diversity matters: a revised guide to myelination. Trends Cell Biol. 26, 135–147. doi: 10.1016/j.tcb.2015.09.002
Tsui, C., Koss, K., Churchward, M. A., and Todd, K. G. (2019). Biomaterials and glia: Progress on designs to modulate neuroinflammation. Acta Biomater. 83, 13–28. doi: 10.1016/j.actbio.2018.11.008
Tupone, M. G., d'Angelo, M., Castelli, V., Catanesi, M., Benedetti, E., and Cimini, A. (2021). A state-of-the-art of functional scaffolds for 3D nervous tissue regeneration. Front. Bioeng. Biotechnol. 9:639765. doi: 10.3389/fbioe.2021.639765
Tysseling-Mattiace, V. M., Sahni, V., Niece, K. L., Birch, D., Czeisler, C., Fehlings, M. G., et al. (2008). Self-assembling nanofibers inhibit glial scar formation and promote axon elongation after spinal cord injury. J. Neurosci. 28, 3814–3823. doi: 10.1523/JNEUROSCI.0143-08.2008
Vismara, I., Papa, S., Veneruso, V., Mauri, E., Mariani, A., De Paola, M., et al. (2020). Selective modulation of A1 astrocytes by drug-loaded Nano-structured gel in spinal cord injury. ACS Nano 14, 360–371. doi: 10.1021/acsnano.9b05579
von Bartheld, C. S., Bahney, J., and Herculano-Houzel, S. (2016). The search for true numbers of neurons and glial cells in the human brain: a review of 150 years of cell counting. J. Comp. Neurol. 524, 3865–3895. doi: 10.1002/cne.24040
Waggoner, L. E., Kang, J. Y., Zuidema, J. M., Vijayakumar, S., Hurtado, A. A., Sailor, M. J., et al. (2022). Porous silicon nanoparticles targeted to the extracellular matrix for therapeutic protein delivery in traumatic brain injury. Bioconjug. Chem. 33, 1685–1697. doi: 10.1021/acs.bioconjchem.2c00305
Waggoner, L. E., Miyasaki, K. F., and Kwon, E. J. (2023). Analysis of PEG-lipid anchor length on lipid nanoparticle pharmacokinetics and activity in a mouse model of traumatic brain injury. Biomater. Sci. 11, 4238–4253. doi: 10.1039/D2BM01846B
Wang, Y., Li, S. Y., Shen, S., and Wang, J. (2018). Protecting neurons from cerebral ischemia/reperfusion injury via nanoparticle-mediated delivery of an siRNA to inhibit microglial neurotoxicity. Biomaterials 161, 95–105. doi: 10.1016/j.biomaterials.2018.01.039
Wang, D., Wang, K., Liu, Z., Wang, Z., and Wu, H. (2020). Valproic acid-labeled chitosan nanoparticles promote recovery of neuronal injury after spinal cord injury. Aging (Albany NY) 12, 8953–8967. doi: 10.18632/aging.103125
Wang, S. S., Zhang, Z., Zhu, T. B., Chu, S. F., He, W. B., and Chen, N. H. (2018). Myelin injury in the central nervous system and Alzheimer's disease. Brain Res. Bull. 140, 162–168. doi: 10.1016/j.brainresbull.2018.05.003
Wang, J. A., Zheng, J., Zheng, Q. X., Wu, Y. C., Wu, B., Huang, S. A., et al. (2015). FGL-functionalized self-assembling nanofiber hydrogel as a scaffold for spinal cord-derived neural stem cells. Mat. Sci. Eng. C-Mater. 46, 140–147. doi: 10.1016/j.msec.2014.10.019
Watson, P. M. D., Kavanagh, E., Allenby, G., and Vassey, M. (2017). Bioengineered 3D glial cell culture systems and applications for neurodegeneration and neuroinflammation. SLAS Discov. 22, 583–601. doi: 10.1177/2472555217691450
Werner, C., and Engelhard, K. (2007). Pathophysiology of traumatic brain injury. Br. J. Anaesth. 99, 4–9. doi: 10.1093/bja/aem131
Willerth, S. M., Rader, A., and Sakiyama-Elbert, S. E. (2008). The effect of controlled growth factor delivery on embryonic stem cell differentiation inside fibrin scaffolds. Stem Cell Res. 1, 205–218. doi: 10.1016/j.scr.2008.05.006
Wu, J. R., Hernandez, Y., Miyasaki, K. F., and Kwon, E. J. (2023). Engineered nanomaterials that exploit blood-brain barrier dysfunction for delivery to the brain. Adv. Drug Deliv. Rev. 197:114820. doi: 10.1016/j.addr.2023.114820
Xi, K., Gu, Y., Tang, J., Chen, H., Xu, Y., Wu, L., et al. (2020). Microenvironment-responsive immunoregulatory electrospun fibers for promoting nerve function recovery. Nat. Commun. 11:4504. doi: 10.1038/s41467-020-18265-3
Xiao, H., Han, S., and Baigude, H. (2021). Regulation of microglia polarization via mannose receptor-mediated delivery of siRNA by ligand-functionalized DoGo LNP. RSC Adv. 11, 32549–32558. doi: 10.1039/D1RA04293A
Xing, S., Yan, M. Z., Yang, Y., Wang, Y. G., Hu, X. C., Ma, B., et al. (2023). Diacerein loaded poly (styrene sulfonate) and carbon nanotubes injectable hydrogel: an effective therapy for spinal cord injury regeneration. J. Clust. Sci. 34, 565–576. doi: 10.1007/s10876-022-02240-7
Xu, J., Xi, K., Tang, J., Wang, J., Tang, Y., Wu, L., et al. (2023). Engineered living oriented electrospun fibers regulate stem cell Para-secretion and differentiation to promote spinal cord repair. Adv. Healthc. Mater. 12:e2202785. doi: 10.1002/adhm.202202785
Yan, H., Wang, Y., Li, L., Zhou, X., Shi, X., Wei, Y., et al. (2020). A micropatterned conductive electrospun nanofiber mesh combined with electrical stimulation for synergistically enhancing differentiation of rat neural stem cells. J. Mater. Chem. B 8, 2673–2688. doi: 10.1039/C9TB02864A
Yang, L., Chueng, S. D., Li, Y., Patel, M., Rathnam, C., Dey, G., et al. (2018). A biodegradable hybrid inorganic nanoscaffold for advanced stem cell therapy. Nat. Commun. 9:3147. doi: 10.1038/s41467-018-05599-2
Yang, B., Liang, C., Chen, D., Cheng, F., Zhang, Y., Wang, S., et al. (2022). A conductive supramolecular hydrogel creates ideal endogenous niches to promote spinal cord injury repair. Bioact Mater. 15, 103–119. doi: 10.1016/j.bioactmat.2021.11.032
Zhang, M., Chen, H., Zhang, W., Liu, Y., Ding, L., Gong, J., et al. (2023). Biomimetic remodeling of microglial riboflavin metabolism ameliorates cognitive impairment by modulating neuroinflammation. Adv. Sci. (Weinh). 10:e2300180. doi: 10.1002/advs.202300180
Zhang, X. D., Gong, B. W., Zhai, J. L., Zhao, Y., Lu, Y. L., Zhang, L. Q., et al. (2021). A perspective: electrospun fibers for repairing spinal cord injury. Chem. Res. Chin. Univ. 37, 404–410. doi: 10.1007/s40242-021-1162-y
Zhang, F., Lin, Y. A., Kannan, S., and Kannan, R. M. (2016). Targeting specific cells in the brain with nanomedicines for CNS therapies. J. Control. Release 240, 212–226. doi: 10.1016/j.jconrel.2015.12.013
Zhang, X., Meng, Y., Gong, B., Wang, T., Lu, Y., Zhang, L., et al. (2022). Electrospun nanofibers for manipulating soft tissue regeneration. J. Mater. Chem. B 10, 7281–7308. doi: 10.1039/D2TB00609J
Zhang, S., Wang, X. J., Li, W. S., Xu, X. L., Hu, J. B., Kang, X. Q., et al. (2018). Polycaprolactone/polysialic acid hybrid, multifunctional nanofiber scaffolds for treatment of spinal cord injury. Acta Biomater. 77, 15–27. doi: 10.1016/j.actbio.2018.06.038
Zhang, F., Zhang, Z., Alt, J., Kambhampati, S. P., Sharma, A., Singh, S., et al. (2023). Dendrimer-enabled targeted delivery attenuates glutamate excitotoxicity and improves motor function in a rabbit model of cerebral palsy. J. Control. Release 358, 27–42. doi: 10.1016/j.jconrel.2023.04.017
Zhang, Q., Zhu, W., Xu, F., Dai, X., Shi, L., Cai, W., et al. (2019). The interleukin-4/PPARgamma signaling axis promotes oligodendrocyte differentiation and remyelination after brain injury. PLoS Biol. 17:e3000330. doi: 10.1371/journal.pbio.3000330
Zhao, T., Jing, Y., Zhou, X., Wang, J., Huang, X., Gao, L., et al. (2018). PHBV/PLA/col-based nanofibrous scaffolds promote recovery of locomotor function by decreasing reactive astrogliosis in a hemisection spinal cord injury rat model. J. Biomed. Nanotechnol. 14, 1921–1933. doi: 10.1166/jbn.2018.2622
Zhou, K., Motamed, S., Thouas, G. A., Bernard, C. C., Li, D., Parkington, H. C., et al. (2016). Graphene functionalized scaffolds reduce the inflammatory response and supports endogenous neuroblast migration when implanted in the adult brain. PLoS One 11:e0151589. doi: 10.1371/journal.pone.0151589
Zhou, Y., Peng, Z., Seven, E. S., and Leblanc, R. M. (2018). Crossing the blood-brain barrier with nanoparticles. J. Control. Release 270, 290–303. doi: 10.1016/j.jconrel.2017.12.015
Zuidema, J. M., Dumont, C. M., Wang, J., Batchelor, W. M., Lu, Y. S., Kang, J., et al. (2020). Porous silicon nanoparticles embedded in poly(lactic-co-glycolic acid) nanofiber scaffolds deliver neurotrophic payloads to enhance neuronal growth. Adv. Funct. Mater. 30:2002560. doi: 10.1002/adfm.202002560
Zuidema, J. M., Gilbert, R. J., and Osterhout, D. J. (2016). Nanoparticle technologies in the spinal cord. Cells Tissues Organs 202, 102–115. doi: 10.1159/000446647
Zuidema, J. M., Hyzinski-Garcia, M. C., Van Vlasselaer, K., Zaccor, N. W., Plopper, G. E., Mongin, A. A., et al. (2014). Enhanced GLT-1 mediated glutamate uptake and migration of primary astrocytes directed by fibronectin-coated electrospun poly-L-lactic acid fibers. Biomaterials 35, 1439–1449. doi: 10.1016/j.biomaterials.2013.10.079
Keywords: nanomaterial, nanoparticle, astrocyte, oligodendrocyte, microglia, drug delivery, central nervous system, glia
Citation: Saksena J, Hamilton AE, Gilbert RJ and Zuidema JM (2023) Nanomaterial payload delivery to central nervous system glia for neural protection and repair. Front. Cell. Neurosci. 17:1266019. doi: 10.3389/fncel.2023.1266019
Edited by:
Maria Grazia Mola, University of Bari Aldo Moro, ItalyReviewed by:
Zhijian Cheng, Xi'an Jiaotong University, ChinaCopyright © 2023 Saksena, Hamilton, Gilbert and Zuidema. This is an open-access article distributed under the terms of the Creative Commons Attribution License (CC BY). The use, distribution or reproduction in other forums is permitted, provided the original author(s) and the copyright owner(s) are credited and that the original publication in this journal is cited, in accordance with accepted academic practice. No use, distribution or reproduction is permitted which does not comply with these terms.
*Correspondence: Jonathan M. Zuidema, am9uYXRoYW4uenVpZGVtYUBtYXJpb25lZ3JpLml0
†These authors have contributed equally to this work and share first authorship