- 1Precision Medicine Research Institute, Samsung Medical Center, Seoul, Republic of Korea
- 2Division of Hematology-Oncology, Department of Medicine, Sungkyunkwan University, Samsung Medical Center, Seoul, Republic of Korea
- 3Graduate School of Cancer Science and Policy, Cancer Biomedical Science, National Cancer Center, Goyang-si, Gyeonggi-do, Republic of Korea
- 4DAWINBIO Inc., Hanam-si, Gyeonggi-do, Republic of Korea
Optogenetic techniques combine optics and genetics to enable cell-specific targeting and precise spatiotemporal control of excitable cells, and they are increasingly being employed. One of the most significant advantages of the optogenetic approach is that it allows for the modulation of nearby cells or circuits with millisecond precision, enabling researchers to gain a better understanding of the complex nervous system. Furthermore, optogenetic neuron activation permits the regulation of information processing in the brain, including synaptic activity and transmission, and also promotes nerve structure development. However, the optimal conditions remain unclear, and further research is required to identify the types of cells that can most effectively and precisely control nerve function. Recent studies have described optogenetic glial manipulation for coordinating the reciprocal communication between neurons and glia. Optogenetically stimulated glial cells can modulate information processing in the central nervous system and provide structural support for nerve fibers in the peripheral nervous system. These advances promote the effective use of optogenetics, although further experiments are needed. This review describes the critical role of glial cells in the nervous system and reviews the optogenetic applications of several types of glial cells, as well as their significance in neuron–glia interactions. Together, it briefly discusses the therapeutic potential and feasibility of optogenetics.
Introduction
Optogenetics is an innovative technology for controlling the complex nervous system, allowing for two-way interactions between neurons and glial cells. Numerous studies have shown that optogenetic treatment of neuronal cells promotes cell excitability (Adamantidis et al., 2007; Carter et al., 2010; Park et al., 2015; Hyung et al., 2019) and synaptic response (Lindsay et al., 2011; Mahn et al., 2016; Sinnen et al., 2017). These phenomena are followed by the firing of action potentials, thereby supporting the maintenance of nervous system health. Thus, optogenetics shows great promise for application in neuroscience research. But, studies on neuronal cells have reported limitations in controlling neuron–glia crosstalk and repairing injured nerves. Indeed, neuronal cells cannot spontaneously regenerate without the support of glial cells, which transform them into dedifferentiated phenotypes to promote nerve damage repair. As the importance of glial cells in neuroscience research has increased, advanced optogenetic tools targeting glial cells are needed to overcome the difficulty of regeneration.
Glial cells in the central nervous system (CNS) and peripheral nervous system (PNS) are known to play essential roles as structural and metabolic components of the complex nervous system. In particular, glial cells have been shown to play roles in the regulation of synaptic formation and activation, the propagation of action potentials for physical responses, and the secretion of neurotrophic factors for trophic support (Jessen and Mirsky, 2005; Zuchero and Barres, 2015). Recently, neuroscientists have predicted that optogenetics of glial cells might contribute to structural and functional maturation of the nervous system (Perea et al., 2014; Pelluru et al., 2016; Jung et al., 2019), which holds promise for improving nervous system function. Although glial cells lack the membrane components required to fire an action potential, they respond well to ion flux via voltage-sensitive ion channels and neurotransmitter receptors. Importantly, Ca2+ in optogenetically stimulated glial cells plays a critical role in modulating and developing the neural network, synaptic activity, and plasticity (Sasaki et al., 2012; Perea et al., 2014; Jung et al., 2019; Balachandar et al., 2020). Increasing evidence supports optogenetic stimulation of glial cells to enhance neuronal maturation and physiology, but the initial findings require verification, and further exploration using diverse experimental designs is needed.
Optogenetics
Optogenetics is an innovative technology that integrates genetic and optical methods to precisely control the activity of excitable neurons using light. Through optogenetic manipulation, neurons can be genetically modified so that effector activity is induced by light. The principle of the optogenetic method is that a genetic component that responds to light, called opsin, is inserted into a neuron or a specific region of the brain, and related activities can be turned on or off using light (Deisseroth, 2010, 2011). One of the most widely used opsins is channelrhodopsin-2 (ChR2), which permits transmembrane movement of ions in and out of light-sensitive cells. This method can be used to depolarize neurons, resulting in precisely timed action potentials that propagate like electrical signals along the axon. Conversely, halorhodopsin (NpHR) is known as an inhibitory opsin and functions as a light-sensitive inward chloride pump, causing hyperpolarization of neurons. Using an opsin, researchers can easily manipulate the activity of a specific network, thereby elucidating cell–cell interactions in highly complex neural circuits. This is particularly useful for controlling one type of cell among numerous brain cells, and because it allows for photoactivation of biological processes at the millisecond timescale. Compared to electrical stimulation, which is insufficient for discriminating among nearby cells, optogenetics provides an unprecedented level of spatial and temporal resolution, making it advantageous for the precise control of neuronal activation.
Numerous studies have shown that optogenetics could be used to modulate cellular and molecular signaling pathways in real time with high precision (Boyden et al., 2005; Li et al., 2005; Bi et al., 2006; Stirman et al., 2011). In particular, this method has been investigated in the context of neurobiology, ophthalmology (Busskamp et al., 2012; Wu et al., 2013), and cardiac systems (Arrenberg et al., 2010; Bruegmann et al., 2010), and it has shown promise for improving organ dysfunction. Optogenetic control of well-defined biological events has allowed the monitoring of the complicated functions of nerve structures in living tissues. Optogenetics has provided new insight into the influence of individual cells on neighboring ones and on other cell types in a given region, which could help clarify the bidirectionality of communication between neurons and between neurons and glia. However, a deeper understanding of how optogenetic activation of a specific cell contributes to the control of neuronal activities in a wider region is still needed. Moreover, the best strategies for modulating neural circuits remain to be identified.
Optogenetics in neurons
The importance of optogenetics has been in the spotlight for understanding how neurons (Adamantidis et al., 2007; Carter et al., 2010), neural circuits (Stuber et al., 2012; Sparta et al., 2013), and the nervous system (Alilain et al., 2008; Lindsay et al., 2011) operate under both normal and pathological conditions. In contrast to other techniques, optogenetics offers both high temporal precision and cell-type specificity. As a result, targeted neurons can be excited or inhibited rapidly, leading to the stimulation of neighboring cells and other neurons. To date, most optogenetic experiments have focused on direct neuronal excitability and inhibition, as well as their effects on neuromodulation (Stuber et al., 2012; Land et al., 2014), synaptic responses (Mahn et al., 2016; Sinnen et al., 2017), and behavior (Caggiano et al., 2014; Iyer et al., 2014). These studies have demonstrated that optogenetics is a powerful tool for behavioral analysis. Extensive optogenetics research has been conducted both in vivo and in vitro. In vitro studies have mainly investigated connectivity among individual cells, changes in cellular processes (Park et al., 2015; Lee et al., 2016), and neurotrophic factors secreted at the cellular level (Cheng et al., 2014; Park et al., 2015). In vivo research has assessed changes in behavior achieved through the excitation or inhibition of target neurons with an optical fiber connected to a laser or light-emitting diode (LED) implanted in freely moving animals (Towne et al., 2013; Bonin et al., 2016). This method enables the study of functional connectivity at multiple scales, from the neuron to the entire brain level, under physiological or pathological conditions.
In recent years, optogenetics has been increasingly used to explore the mechanisms underlying dysfunction caused by neurological disorders and injuries (Krook-Magnuson et al., 2013; Xie et al., 2013; Cheng et al., 2014; Lim et al., 2014). Using an optogenetics approach, Lim et al. (2014) studied neural network changes in both cortical hemispheres after a cortical stroke and reported heterogeneous recovery of the injured region, even in the intact hemisphere, as reflected in changes in functional connections. These results are consistent with the view that stroke affects networks throughout the brain and supports the involvement of intact regions in stroke recovery. In addition, optogenetics has been used to investigate cell types associated with cortical excitability and recovery. Xie et al. (2013) assessed responses to optogenetic stimulation of cortical neurons and electrical stimulation of the forelimb. Electroencephalography and electromyography were used to examine sensory processing after global ischemia, and the acute effects of global ischemia were also investigated using optogenetics. Optogenetics has also been applied to neurological disorders in studies investigating the role of GABAergic cells in the hippocampus in temporal lobe epilepsy (Krook-Magnuson et al., 2013). Optogenetic interventions involving the stimulation of parvalbumin (PV)-expressing GABAergic neurons with ChR2 were found to attenuate electrical seizures and reduce the frequency of behavioral seizures. These results demonstrate the potential application of optogenetics in therapeutic interventions to control spontaneous seizures in cases of temporal lobe epilepsy.
Furthermore, optogenetics has been widely used in pre-clinical studies as a rehabilitative and therapeutic tool for neurodegenerative diseases (Gradinaru et al., 2009; Yoon et al., 2014; Seeger-Armbruster et al., 2015). In the ventroanterior motor thalamus, glutamatergic neurons can be activated through optogenetics to modulate movement in acute drug-induced Parkinsonian rats. An optogenetic stimulation pattern that mimicked real neuronal activity or theta-burst patterns showed significantly improved effectiveness (Seeger-Armbruster et al., 2015). Moreover, optogenetic inhibition of the subthalamic nucleus improved the motor symptoms of Parkinson’s, including forelimb akinesia, by reducing the firing of inhibitory neurons in areas such as the internal globus pallidus and substantia nigra pars reticulata (Yoon et al., 2014). Optogenetics has also been used to study Alzheimer’s disease (Yamamoto et al., 2015; Etter et al., 2019). For example, Etter et al. (2019) reported that optogenetic activation of PV neurons restored slow gamma oscillations in the hippocampus and rescued spatial memory in a mouse model of Alzheimer’s disease. It has also been reported that optogenetic stimulation of dentate gyrus neurons reduced memory impairment and promoted the reactivation of previously activated neural ensembles during memory encoding (Tonegawa et al., 2015). These results suggest that optogenetic stimulation could serve as an innovative treatment for neurodegenerative diseases.
Several optogenetic studies have focused both on individual neurons (Park et al., 2015) and their interactions with surrounding cells, especially glial cells, which promote myelination (Gibson et al., 2014; Lee et al., 2016; Hyung et al., 2019). The myelin sheath is an electrical insulator formed by oligodendrocytes in the CNS and Schwann cells in the PNS. It wraps around axons to provide electrical insulation, enveloping and protecting the axons while also increasing the propagation speed of the action potential and providing neurotrophic factors to the axons. When the myelin sheath is damaged, signal conduction of the affected nerve is impaired, which can affect sensation, movement, cognition, and other functions depending on the nerve involved; in severe cases, this can lead to demyelinating diseases such as multiple sclerosis (Genain et al., 1999; Zhou et al., 2006; Fancy et al., 2010), Charcot–Marie–Tooth disease (Patel et al., 1992; Sereda et al., 2003; Berger et al., 2006), and Guillain−Barré syndrome (Koski, 1990; Khalili-Shirazi et al., 1993; Ruts et al., 2012). Reforming a myelin sheath following injury is difficult; numerous methods have been investigated, including use of neurotrophic factors (McTigue et al., 1998; Chan et al., 2001; Gingras et al., 2008), stem cells (Gupta et al., 2012; Uchida et al., 2012), autologous (den Dunnen and Meek, 2001; Zhang et al., 2010) or artificial nerve grafts (Xu et al., 2003; Huang et al., 2012), and electrical stimulation (Brushart et al., 2002; Ishibashi et al., 2006; Huang et al., 2010). However, their therapeutic efficiency has been limited by indiscriminate stimulation of the injured area. Therefore, optogenetic techniques are increasingly being used to promote myelination, and have shown remarkable efficacy. Several studies have reported that the number of oligodendrocytes and myelin thickness in the CNS increased with optogenetic stimulation, both in vivo and in vitro (Gibson et al., 2014; Lee et al., 2016; Yamazaki et al., 2019). For example, Gibson et al. (2014) demonstrated that optogenetic stimulation of neurons in the premotor cortex induced the proliferation of oligodendrocyte precursor cells (OPCs), some of which differentiated into mature oligodendrocytes and increased myelin thickness in active neuronal circuits. In addition, using a three-dimensional microfluidic chip, Hyung et al. (2019) demonstrated that optogenetic stimulation of motor neurons promoted the differentiation of Schwann cells and enhanced axon outgrowth. Moreover, they showed that the thickness of the myelin sheath was similar to that under normal conditions.
Optogenetics in glia
Astrocytes
Astrocytes are the most abundant non-neuronal cells in the brain, composing approximately 50% of the volume of the adult mammalian brain. For a century, studies considered astrocytes merely as structural supporters for neuronal cells. Astrocytes typically extend their processes, tightly contacting the synapses and blood vessels in their surroundings, positioning them well to maintain the structural integrity of the blood–brain barrier (Abbott, 2002; Cabezas et al., 2014). They express transporters on their membranes for ions, enabling them to maintain brain extracellular homeostasis by regulating extracellular K+ and pH levels (Walz, 2000; Perea et al., 2014). Furthermore, they express glutamate transporters, allowing them to clear away excess released glutamate (Rothstein et al., 1994; Sutherland et al., 1996; Danbolt, 2001; Huang and Bergles, 2004; Tzingounis and Wadiche, 2007).
During the last few decades, studies have indisputably demonstrating that astrocytes not only support and maintain the neuronal cells but also contribute significantly to the regulation of synaptic transmission. Astrocytes express many neurotransmitter receptors, including metabotropic (mGluR) and ionotropic (AMPAR) glutamate receptors, ATP receptors, and GABA receptors (Porter and McCarthy, 1997; Hamilton and Attwell, 2010; Kimelberg and Nedergaard, 2010; Allen, 2014). These receptors indicate that not only neurons but also astrocytes can be activated by the neurotransmitters released from the presynaptic terminal. Supporting studies have shown that the activation of synaptic transmission induces intracellular calcium increases in the astrocytes (Wang et al., 2009; Bazargani and Attwell, 2016). Furthermore, it is established that astrocytes can release some neurotransmitters such as glutamate, D-serine, ATP, and GABA, collectively referred to as “gliotransmitters” (Parpura et al., 1994; Mothet et al., 2000; Yoon and Lee, 2014). The release of these gliotransmitters often occurs when G protein-coupled receptors (GPCR) are activated, leading to sequential downstream signaling pathways like phospholipase C, adenylate cyclase, and inositol 1,4,5-trisphosphate (IP3), which can cause an increase in intracellular calcium in astrocytes (Sheppard et al., 1997; Agulhon et al., 2008; Petravicz et al., 2008). Since they are connected via gap junctions composed of connexin channels (Theis et al., 2003), which allow cAMP and calcium to move around (Harris, 2007; Orthmann-Murphy et al., 2008), the intracellular calcium increase in a single astrocyte can be passed to other astrocytes, initiating the calcium waves (Hofer et al., 2002; Theis et al., 2003; Scemes and Giaume, 2006; Theis and Giaume, 2012). Increased intracellular calcium in astrocytes is considered one of the common factors that drive the release of gliotransmitter (Allen, 2014; Bazargani and Attwell, 2016). Gliotransmitters released from astrocytes are able to facilitate the excitability of neighboring neurons (Perea et al., 2009; Araque and Navarrete, 2010; Allen, 2014; Sahlender et al., 2014). The consequences depend on the location and the activated receptors of the neurons (Araque et al., 2001; Bezzi and Volterra, 2001; Liu et al., 2004; Perea et al., 2009; Sasaki et al., 2011). Based on these evidences of interaction and communication between astrocytes and neurons, a concept of the “tripartite synapse” has been proposed (Agulhon et al., 2008).
As mentioned previously, astrocytes were for a long time known to be non-excitable cells and therefore were assumed not to be influenced by membrane potential or by neurotransmitters. However, with the development of optogenetics, many studies have shown that astrocytes can truly be a practical tool to investigate the role of astrocytes in deeper aspects of higher brain functions such as respiration, depression, sleep, memory, disease, pain, and much more to be investigated in the near future (Li et al., 2012; Berlinguer-Palmini et al., 2014; Figueiredo et al., 2014; Ono et al., 2014; Bang et al., 2016).
In optogenetic manipulation studies involving astrocytes, both primary astrocytes culture and immortal cell lines have demonstrated that transfected opsins can respond to photostimulation, leading to intracellular calcium increases and gliotransmitter release in vitro (Figure 1; Table 1).
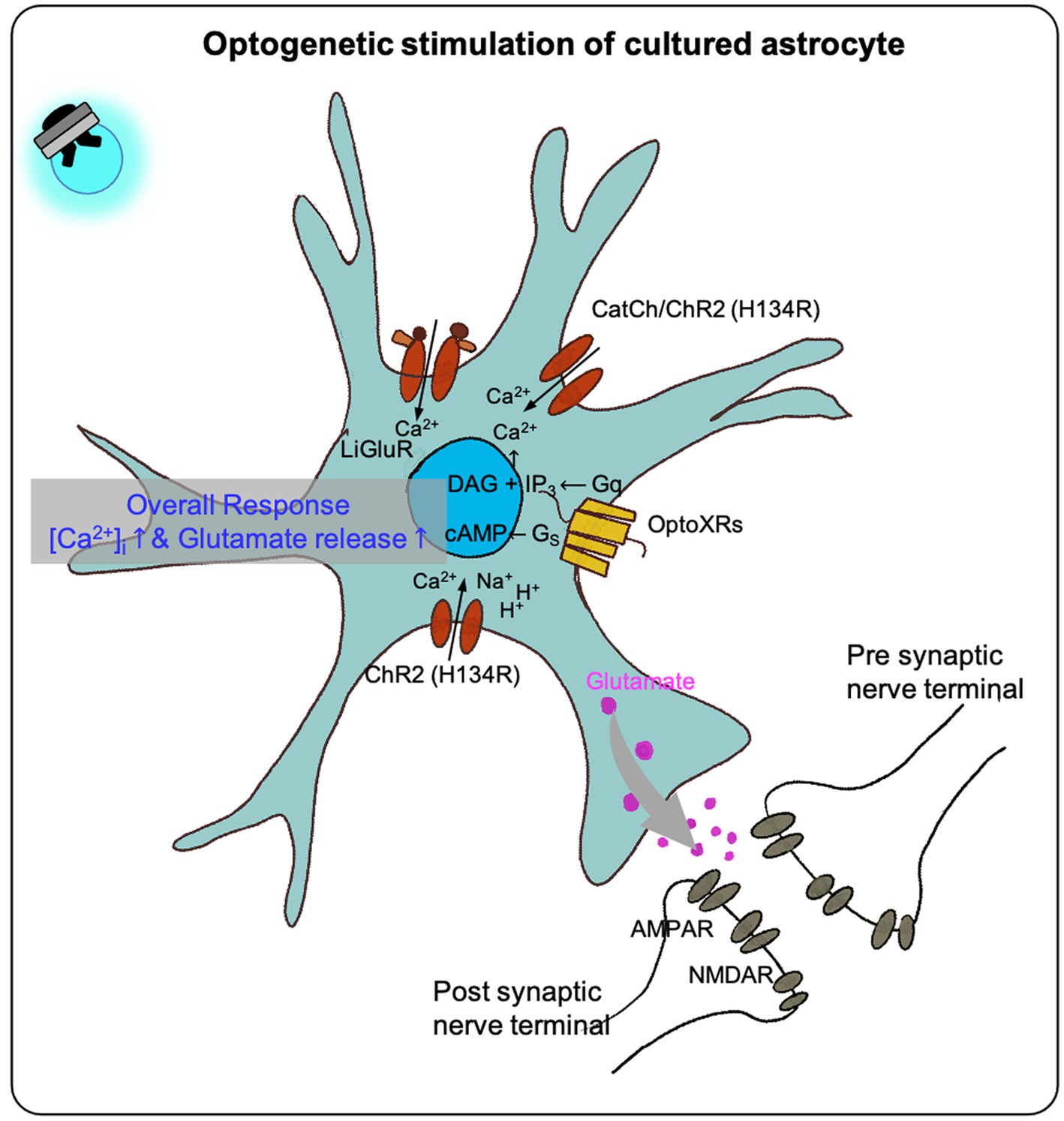
Figure 1. The effect of optogenetic stimulation on cultured astrocytes. Previous studies shown that the optogenetic stimulation on the cultured astrocytes transfected with different optogenetic effectors (LiGluR, CatCh, ChR and its variants, OptoXR) showed increased intracellular calcium concentration in overall, some resulted in glutamate release (see Table 1).
Two main approaches have been employed for the in vivo expression of astrocytes-specific opsins (Figure 2).
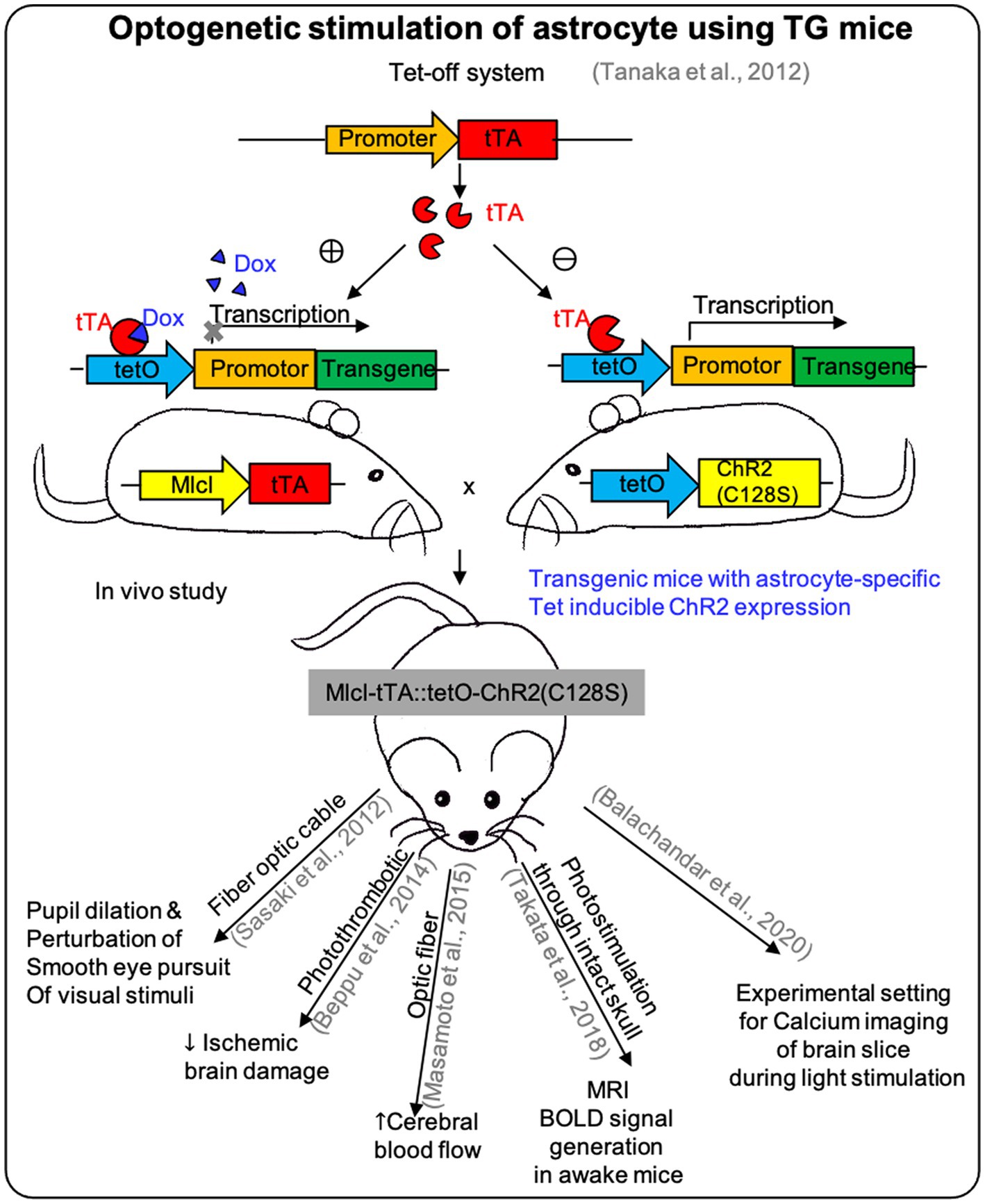
Figure 2. The effect of optogenetic stimulation on astrocytes using TG mice. Researches used the Tet-off system on TG mice for expressing ChR2 specifically on astrocytes to demonstrate the in vivo effect of optogenetic stimulation and showed different responses depending on the site of effectors (see Table 2).
One is to use the astrocytes-specific opsin transgenic or knock-in mice (Table 2). Both Cre/loxP and tetO-tTA systems can be used for astrocytes-specific opsin-transgenic mice, which can be generated by mating astrocytes-specific Cre or tTA mice with floxed-stop-opsin knock-in mice or tetO-opsin transgenic mice (Cho et al., 2016).
The other approach is to use recombinant adeno-associated virus (AAV) for opsin construct expression (Figure 3).
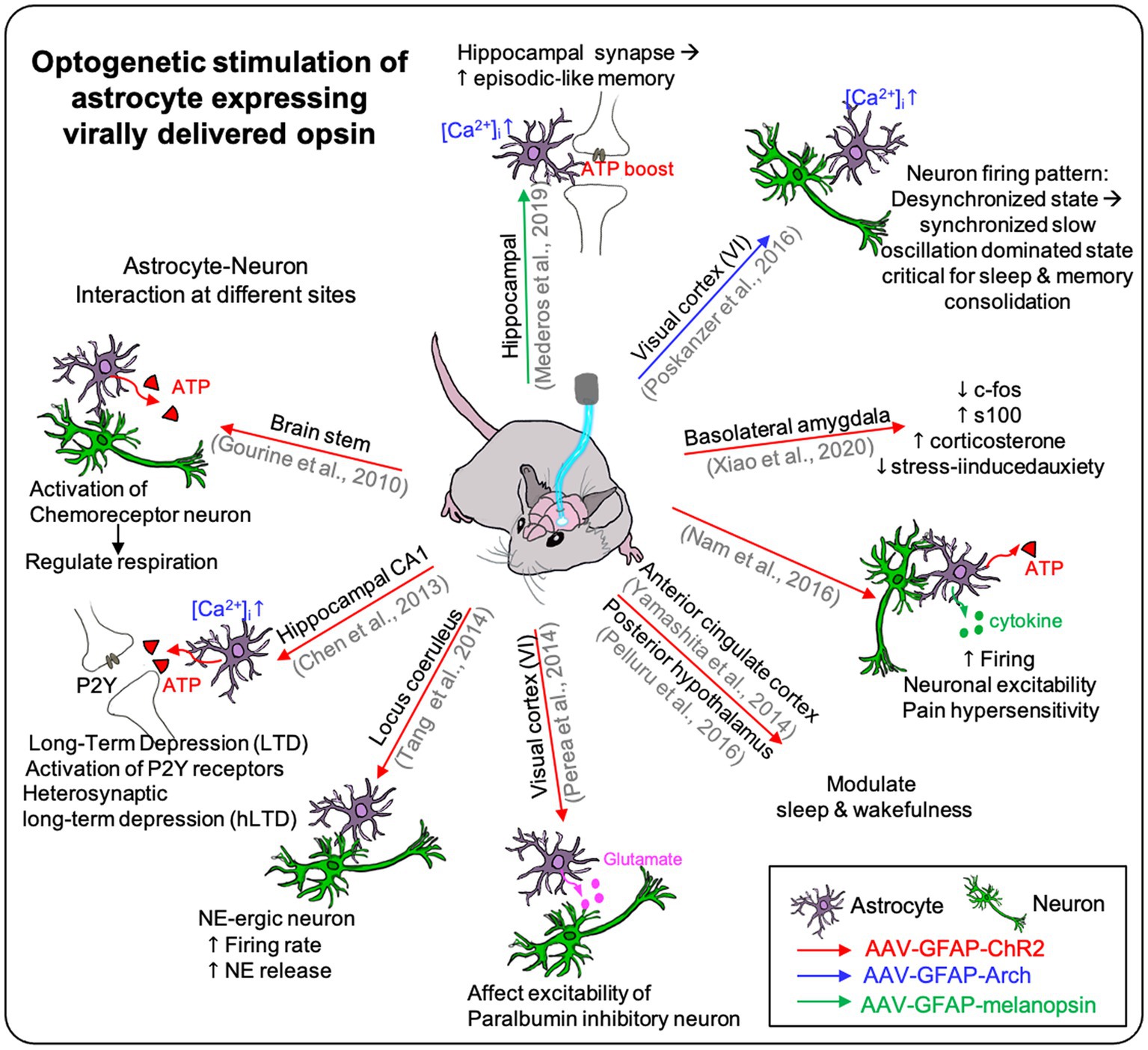
Figure 3. The effect of optogenetic stimulation on astrocyte expressing virally delivered opsin. Virally delivered opsins are used on many different sites on the brain in the previous studies. It showed the effect of optogenetic stimulation on the opsin-expressing astrocyte itself and showed the effect on the neighboring neurons affected by the photostimulation of the astrocytes. Furthermore, it demonstrated the optogenetic stimulation could affect higher brain function such as respiration, depression, sleep, pain, and memory (see Table 3).
For example, viral vectors encoding opsin genes under the astrocyte-specific promoter (e.g., GFAP) are used to express astrocyte-specific ChR2 in the mouse brain (Table 3).
Studies have been demonstrating clearly that the optogenetic manipulation can regulate astrocyte gliotransmitter release and subsequent synaptic transmission. Of course, there are certain limitations of current optogenetic astrocyte manipulation. For example, the intracellular calcium increase in astrocytes via optogenetic ChR2 activation does not exactly recapitulate physiological astrocyte calcium elevation, which is mostly due to GPCRs activation. Additionally, astrocytic calcium responses are distinct depending on which compartment of the cell is involved because different compartments have different expression of receptors and sources for calcium. In this regard, some review papers suggest the utilization and development of more optogenetic tools, such as optoXR (Airan et al., 2009) or OptoSTIM1 (Kyung et al., 2015). These tools include cell-signaling modifying opsins, such as chimeric opsins (the Gq-coupled optoa1AR and the Gs-coupled optobAR), which have been shown to induce calcium increases in cultured astrocytes as efficiently, if not more efficiently, as ChR2 (Figueiredo et al., 2014; Adamsky and Goshen, 2018).
While neuronal optogenetics allows for precise control of membrane potential and the timing of action potentials, astrocyte optogenetics involves influencing processes like neurotransmitter regulation, synaptic plasticity, and the ionic environment, which are critical for overall brain homeostasis. However, glial optogenetics may not achieve the same level of timing precision as neuronal optogenetics, since it often involves modulating slower processes such as calcium signaling in astrocytes. One notable finding (Figueiredo et al., 2014; Gerasimov et al., 2021) is related to the potentiation of field potentials during optogenetic activation of astrocytes expressing Opto-a1AR (metabotropic opsin). This potentiation is associated not only with the release of glutamate but also gliotransmitters like D-serine, crucial for long-term changes in plasticity. The release of D-serine occurs through calcium-dependent exocytosis and other pathways. Activation of Opto-a1AR significantly increases intracellular calcium concentrations, facilitating D-serine release from astrocytes. This differs from ChR2 activation, which does not produce a comparable increase in intracellular calcium.
The significance of astrocyte optogenetics in this context lies in its ability to influence neuronal networks in a nuanced and precise manner. Astrocytes are pivotal in modulating synaptic transmission, plasticity, and neurovascular coupling. Targeting astrocytes with optogenetics not only allows for the modulation of neurotransmitter release but also affects gliotransmitter dynamics like D-serine, critical for long-term plasticity. This approach holds promise for correcting network dysfunctions seen in neurodegenerative disorders.
However, it is essential to acknowledge the potential drawbacks of astrocyte stimulation, including the conversion of astrocytes into reactive glia with cytotoxic effects. This conversion can harm neurons and exacerbate neuroinflammation. In vivo experiments are necessary to validate the study’s parameters and refine the approach to mitigate potential negative effects on brain function. Even with some restrictions and limitations of the current optogenetic technique, it is truly undeniable that optogenetics is a valuable tool for elucidating the more physiological roles of astrocytes in higher-order brain functions.
Microglia
Microglia are immune cells of central nervous system, composing 5–10% of total brain cells. They constantly survey the microenvironment in the brain and spinal cord through their ramified processes. Under normal healthy conditions, microglia sensitively observe their surrounding area with their long and thin branches, which spans about 50 μm in diameter. Ramified microglia in the resting state under normal physiological conditions are able to search for and identify immune threats while maintaining homeostasis in the CNS (Davis et al., 1994; Aloisi, 2001; Christensen et al., 2006). The resting potential of microglia is around -40 mV, maintained by THIK-1 (Tandem of P domains in a Weak Inward rectifying K+ channel 1) and potentially by TRP (transient receptor potential) and chloride-conducting channels (Newell and Schlichter, 2005; Madry et al., 2018). THIK-1 is identified as the primary potassium (K+) channel expressed in microglia, and it is a constitutively active, with its activity being enhanced by P2Y12 receptors. Inhibiting THIK-1 function through pharmacological means or gene knockout results in the depolarization of microglia, reducing their ramification, and thus impairing their surveillance capabilities. Interestingly, blocking P2Y12 receptors does not affect membrane potential, ramification, or surveillance. This suggests that THIK-1 plays a vital role in maintaining microglial membrane potential, which is crucial for immune surveillance and the release of pro-inflammatory cytokines like interleukin-1β from activated microglia. Besides, TRP channels are a family of ion channels that include both cation and anion channels. Some TRP channels can allow the flow of calcium ions (Ca2+) into microglia, which can influence membrane potential and cellular responses. These channels may be involved in detecting changes in the extracellular environment, such as injury or inflammation, and can contribute to microglial activation. Chloride channels are responsible for regulating the flow of chloride ions (Cl−) into or out of the cell membrane. The movement of chloride ions can affect the overall membrane potential of microglia. These channels play a role in maintaining the electrochemical balance within the cell, which is essential for normal cellular functions, including immune responses.
Microglia was previously classified as non-excitable cells, but they do exhibit strong electrophysiological stimulus–response features. Unlike neurons and astrocytes, microglia have no reported studies showing direct optogenetic microglia manipulation so far. There are studies showing correlation between the microglia activation and its membrane potential, but it has not been clearly investigated if changes in the membrane potential are the cause or the consequence of the microglia activation.
It has been especially challenging to specifically investigate the role of activated microglia in their native environment due to their interactions with other types of glial cells and neurons. However, studies have demonstrated that optogenetic astrocyte manipulation has successfully shown the possibilities of cell-type specific opsin expression with the transgenic mice and viral delivery of opsin, opening up the potential for many interesting future research avenues in optogenetic microglia manipulation.
Microglia play various critical roles in the CNS such as regulating synapse formation/elimination through BDNF release (Coull et al., 2005; Gottmann et al., 2009; Ferrini and De Koninck, 2013), and phagocytic activity induced by chloride influx (Averaimo et al., 2010), among others. Intracellular calcium increase in microglia is a common signaling pathway leading to expression of the proinflammatory genes including cytokines and chemokines such as TNF-α and ROS. As many previous studies in neurons and astrocytes have shown that optogenetic ChR2 stimulation increases intracellular calcium, it is now possible to investigate the optogenetically induced calcium increase and its effect on microglia.
In the near future, we anticipate that additional optogenetic manipulations utilizing different receptors/activators, such as opto-XR (Airan et al., 2009) or optoSTIM1 (Kyung et al., 2015), will be employed to delve deeper into the specific roles and underlying mechanisms of microglia in brain functions.
Oligodendrocytes
Oligodendrocytes wrap tightly around axons (referred to as myelin sheaths) to facilitate the transmission of electrical signals to the terminal, thus providing structural and functional support to neural networks of the CNS. Myelin sheaths created by oligodendrocytes reduce ion leakage and cell membrane capacitance, leading to an increase in the impulse speed and faster signal propagation along insulated axons. Destruction of myelinating oligodendrocytes causes several types of nervous system dysfunction, including motor circuit deficits and delayed sensory processing (Tkachev et al., 2003; Takahashi et al., 2011; Philips et al., 2013). As with other types of glial cells, therapeutic strategies for protecting oligodendrocytes to support maintenance of a healthy myelin structure and reconstruction in response to injury are needed.
Despite the importance of oligodendrocytes as morphological and functional supports in the nervous system, only a few studies describing direct optogenetic manipulation of oligodendrocytes (Yamazaki et al., 2014, 2019). In these studies, selective ChR2 expression in oligodendrocytes was achieved using transgenic mouse models. They used the proteolipid protein (PLP) promoter to create tTA protein specifically in oligodendrocytes. Then, this tTA protein activated the tetO promoter, responsible for ChR2 expression, resulting in ChR2(C128S)-EYFP fusion protein production within oligodendrocytes. This approach ensured precise and targeted ChR2 expression in these cells. This emphasizes the significance of optogenetic approaches in comprehending neural circuit function and plasticity (Yamazaki et al., 2019). Oligodendrocyte depolarization, achieved through optogenetics, leads to significant increases in excitatory synaptic responses and enhances long-term potentiation (LTP) at specific synapses in the hippocampus. These results suggest that optogenetic manipulation of oligodendrocytes can finely tune synaptic activity and influence information transfer between different regions of the nervous system, highlighting the role of oligodendrocyte depolarization in modulating synaptic activity in a region- and cell type-specific manner.
Moreover, oligodendrocytes expressing ChR2 were applied to study the functional plasticity of white matter in the hippocampus (Yamazaki et al., 2014). Researchers observed that light-induced depolarization of oligodendrocytes led to both short-term and long-term facilitation of axonal conduction, depending on the magnitude of oligodendrocyte depolarization. These findings demonstrate that oligodendrocyte depolarization actively induces functional plastic changes in white matter. Therefore, optogenetic control of oligodendrocytes can significantly contribute to our understanding of how white matter responds to neural activity and how it influences brain functions.
As neurons lack sufficient spontaneous repair capacity, the control of the nervous system using oligodendrocytes is necessary to ensure functional recovery from damage to the CNS. In contrast to neurons, oligodendrocytes cannot generate action potentials; however, they respond to ion influx. In particular, the influx of calcium ions through the calcium channels of oligodendrocyte progenitor cells promotes their migration, differentiation, and myelination (Soliven, 2001; Cheli et al., 2015), indicating that the regulation of calcium ion fluctuations in oligodendrocytes may play a key role in nervous system maturation. Optogenetic manipulation of oligodendrocytes through the introduction of calcium-permeable ChR2 may enable precise control of the reciprocal interactions between neurons and glia.
Schwann cells
The Schwann cell is a type of glial cell in the PNS, with equivalent functions to those of oligodendrocytes in the CNS. However, the structure of Schwann cells differs from that of oligodendrocytes. An individual Schwann cell can enclose only one axon, forming a myelin sheath, whereas a single oligodendrocyte forms myelin sheaths around segments of several adjacent axons. Studies on the functions of Schwann cells have extensively reported their pivotal role in increasing the conduction of electrical signals via myelination (Salzer, 2015). Additionally, they supply nutrients to neurons (Frostick et al., 1998; Boyd and Gordon, 2003) and modulate synaptic activity (Cao and Ko, 2007; Feng and Ko, 2008). Moreover, Schwann cells recognize damaged areas of the PNS and rapidly transform their phenotype into dedifferentiated and redifferentiated forms to assist in the regeneration of damaged neurons. Despite limitations regarding the functional recovery of regenerated nerves, peripheral nerves are more readily regenerated after injury than those of the CNS, due to the functions of Schwann cells in the PNS repair process.
A few studies have reported that optogenetic manipulation of Schwann cells promotes their proliferation and differentiation, and accelerates the myelination of neurons cocultured with Schwann cells (Jung et al., 2019). Through LED irradiation of optogenetically modified Schwann cells, the number of proliferating Schwann cells increased substantially over time in the absence of neurons (Figure 4). Furthermore, optogenetically treated Schwann cells showed increased expression of myelin-related proteins, such as early growth response protein 2 and myelin basic protein, resulting in the formation of a compact myelin sheath in the presence of neurons (Figure 4). Surprisingly, these effects depended on intracellular calcium signaling in optogenetically modified Schwann cells; increased calcium levels were induced in these cells through T-type voltage-gated calcium channels and mobilization of inositol 1,4,5-trisphosphate-, caffeine-, and ryanodine-sensitive stores. Although controlling optogenetically modified Schwann cells is essential for optimizing their growth and function, the most effective method of manipulating optogenetic Schwann cell has not yet been determined. Moreover, there is a need for studies on the functions of optogenetically modified Schwann cells cocultured with other types of neurons and on their activities under in vivo conditions.
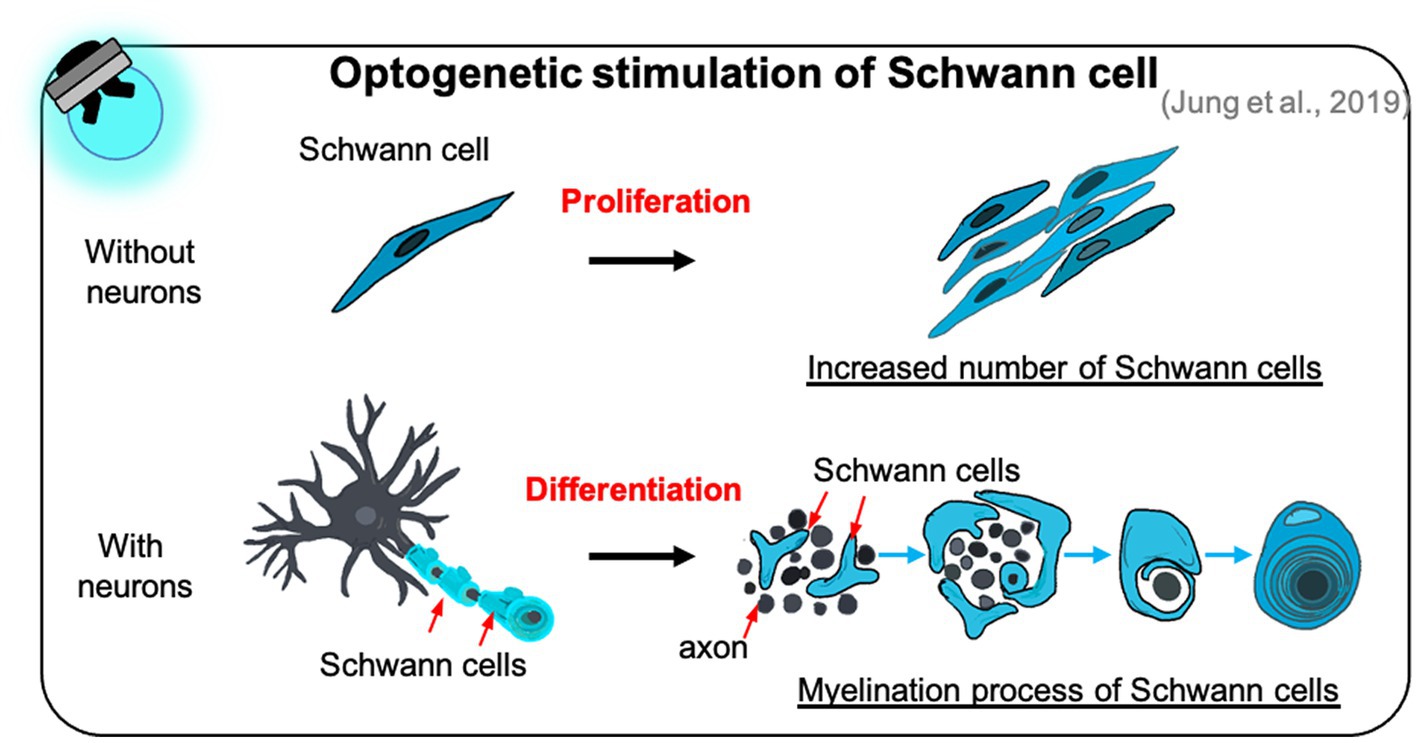
Figure 4. The effect of optogenetic Schwann cell manipulation on proliferation and differentiation. Optogenetic Schwann cells stimulate their proliferation in the Schwann cell monoculture without neuron (top) and differentiation to form myelin sheath in the Schwann cells-neuron coculture (bottom).
Recent research has demonstrated that PNS myelination is accelerated when optogenetic Schwann cells are used rather than optogenetic neurons (Jung et al., 2020). When LED irradiation was applied to optogenetically stimulated Schwann cells and neurons in a neuron-Schwann cell coculture model, the expression of several myelin-promoting factors increased more sharply in the Schwann cells, and the g-ratio (the ratio of the inner diameter to the total outer diameter) of myelinated axons in optogenetically stimulated Schwann cells was similar to the theoretical value for optimal fiber conduction. Similarly, optogenetic glial manipulation strongly promoted PNS myelin sheath maturation compared to the use of optogenetic neurons. These findings indicate that appropriate cell selection for optogenetic applications may improve our understanding of developmental and neurodegenerative diseases.
Furthermore, optogenetic techniques were applied to nociceptive Schwann cells, resulting in pain-like responses in experimental animals. This approach allowed precise manipulation of Schwann cell activity without directly stimulating sensory nerves (Abdo et al., 2019). This discovery highlights the intricate role of glial cells in sensory perception, particularly in sensing mechanical stimuli and contributing to pain modulation. The potential application of optogenetics introduced in controlling gene expression in Schwann cells for spinal cord regeneration (Muna, 2020). In this study, Schwann cells were optogenetically engineered to respond to near-infrared light (NIRW) stimulation, offering a non-invasive and precise approach to the production of therapeutic agents. These Schwann cells were stably engineered to express a NIRW light-responsive adenylyl cyclase called IlaM5. Upon exposure to NIRW irradiation, these engineered Schwann cells activated the expression of a reporter luciferase from a cAMP-dependent promoter, resulting in a several-fold increase in luciferase levels following 1 h of NIRW irradiation. Notably, the activation levels were even higher when biliverdin, the chromophore for IlaM5, was externally added to the Schwann cells. This innovative approach has significant implications for translational applications in spinal cord regeneration therapies, offering a promising avenue for precise and non-invasive control over gene expression in Schwann cells, which can support axon regeneration in cases of spinal cord injuries associated with permanent neurological deficits.
Challenges and future considerations
Advances in optogenetic glial cell manipulation have opened up new perspectives on nervous system development. Moreover, these techniques hold excellent potential for enhancing nerve function and uncovering the mechanisms of neuron–glia communication. However, these approaches have only been applied to a limited number of glial cell types, and further studies are required to determine the molecular pathways through which each type of optogenetic glial cell regulates the physiology of the CNS and PNS.
It is important to note that achieving long-term and stable opsin expression in glial cells for clinical applications can be challenging, and there may be limitations to how long expression can be maintained. Achieving long-term and stable opsin expression in astrocytes for clinical applications presents challenges, with the duration of expression influenced by factors such as the viral vector, promoter, delivery method, and individual patient factors.
1. Viral vector selection: Utilizing a viral vector known for stable, long-term transgene expression is crucial. Adeno-associated viruses (AAV), renowned for their relatively enduring expression, hold paramount importance in astrocyte optogenetics for clinical use. Certain AAV serotypes have received regulatory approval for clinical gene therapy, facilitating potential clinical trials and novel therapy development.
2. Cell-specific promoters: The choice of a promoter directing opsin expression specifically in astrocytes ensures precise targeting. Cell type-specific promoters minimize off-target effects and unintended interactions between different cell types in the brain. Customizable to target specific astrocyte populations, these promoters tailor the therapy to the underlying mechanisms of neurological disorders.
3. Delivery method: Precision in targeting astrocytes while safeguarding neighboring cells is essential. Minimally invasive techniques prioritize patient safety, reduce tissue damage, and enhance accessibility and ease of administration. Real-time monitoring and control, ethical and regulatory compliance, translatability, and compatibility with combination therapies collectively underpin the development of safe and effective clinical astrocyte optogenetics.
4. Patient-specific considerations: Recognizing the variability in neurological disorders, treatment responses, and individual characteristics, personalized approaches are crucial. Tailoring glial cell optogenetic interventions allows for precise adjustments, prediction of long-term effects, and optimization of patient outcomes, advancing precision medicine in neuroscience and enhancing the quality of life for individuals affected by neurological disorders.
On the other hand, chemogenetic technologies, such as Designer Receptors Exclusively Activated by Designer Drugs (DREADDs), are widely employed by neuroscientists to identify the specific neural circuits and cellular signals that govern behaviors, perceptions, emotions, innate drives, and motor functions in various species from flies to nonhuman primates (Roth, 2016; Meister et al., 2021; Bossuyt et al., 2023). One key factor is that glial cells, including astrocytes and microglia, often communicate through G-protein-coupled receptors (GPCRs). The DREADD system, which relies on GPCRs engineered to respond to specific ligands, aligns well with the signaling pathways commonly found in glial cells. This makes it a more natural choice for modulating glial activity when compared to optogenetics. Additionally, chemogenetic manipulation can induce sustained changes in cellular activity as long as the ligand is present, allowing researchers to study longer-term effects. In contrast, optogenetic manipulation typically requires continuous light exposure, which may not be suitable for all experimental designs. Furthermore, chemogenetics is often preferred for in vivo studies because it can be more easily applied to freely moving animals. Researchers can administer the ligand systemically, allowing for precise control of glial activity in behaving animals. Moreover, chemogenetics, particularly when using systemically administered ligands, can penetrate deeply into tissues, making it suitable for manipulating glial cells in different brain regions. But, optogenetic stimulation may have limitations in terms of light penetration and tissue scattering. Lastly, the DREADD system offers flexibility in terms of temporal control. Researchers can administer the ligand at specific time points, allowing for controlled activation or inhibition of glial cells during particular phases of an experiment. DREADDs, while valuable, can have limitations. It exhibits poor reactivity with the endogenous ligand, potentially acting as dominant-negative forms of muscarinic receptors. This limitation can hinder their ability to effectively modulate cellular responses. Moreover, there are reported instances of toxic effects associated with long-term DREADD expression, although this may not be exclusive to glial cells (Goossens et al., 2021). Additionally, DREADDs, as engineered receptors, have the potential to interfere with endogenous signaling cascades, which could disrupt normal cellular processes. In contrast, optogenetic proteins, though exogenous, are less likely to disrupt endogenous signaling cascades. The choice between DREADDs and optogenetics should consider the specific research goals and potential drawbacks associated with each method.
Nevertheless, evidence supporting the importance of optogenetic glial manipulation is mounting. Given that damaged neurons cannot regenerate spontaneously and require structural and trophic support from glial cells, optogenetic strategies involving glial cells are reasonable for repair of damaged nerves. Ongoing research is aiming to determine (1) whether optogenetic manipulation of all types of glial cells can better control nerve function organization than optogenetic neuronal activation, and (2) the optimal expression level of the opsin gene to initiate the excitation of glial cells and maintain the activity of excitable cells. In addition, several challenges remain, including elucidating (1) whether optogenetic glial cells can be applied to correct nervous system dysfunction and (2) whether the effects of optogenetically activated cells and tissues are similar in vitro and in vivo and, ultimately, in the context of clinical application.
To apply optogenetics therapeutically in the clinical setting, it is important to focus on the delivery and safety of optogenetic tools. An in-vitro optogenetic study using human-derived cells provided critical evidence that the application of optogenetics to humans is possible (Zhuge et al., 2014; Klapper et al., 2017). According to high-quality review papers (Williams and Denison, 2013; Cheng et al., 2016; Shen et al., 2020), the therapeutic potential of optogenetic techniques appears to be substantial. Nevertheless, for clinical application of opsin gene activation in a specific cell type or brain region, invasive “gene conveyance” is required via viral injection into the target location. This injection leads to scar tissue formation and is susceptible to infection, which can cause immunogenic conditions. Additionally, a method for assessing the expression of the implanted opsin gene in human cells, and for mitigating damage caused by the temperature increase induced by the lights used for optogenetic stimulation, is still urgently needed.
Author contributions
SH: conceptualization ideas, formulation or evolution of overarching research goals and aims. KJ, J-HP, and SH: writing (original draft) preparation, creation and/or presentation of the published work, specifically writing the initial draft. J-HP and SH: visualization, preparation, creation and/or presentation of the published work, specifically visualization/data presentation. All authors contributed to the article and approved the submitted version.
Funding
This research was supported by the [Biomedical Technology Development Program] of the National Research Foundation (NRF) funded by the Korean government (MSIT; no. RS-2023-00222838).
Conflict of interest
KJ was employed by DAWINBIO Inc.
The remaining authors declare that the research was conducted in the absence of any commercial or financial relationships that could be construed as a potential conflict of interest.
Publisher’s note
All claims expressed in this article are solely those of the authors and do not necessarily represent those of their affiliated organizations, or those of the publisher, the editors and the reviewers. Any product that may be evaluated in this article, or claim that may be made by its manufacturer, is not guaranteed or endorsed by the publisher.
References
Abbott, N. J. (2002). Astrocyte-endothelial interactions and blood-brain barrier permeability. J. Anat. 200, 629–638. doi: 10.1046/j.1469-7580.2002.00064.x
Abdo, H., Calvo-Enrique, L., Lopez, J. M., Song, J., Zhang, M. D., Usoskin, D., et al. (2019). Specialized cutaneous Schwann cells initiate pain sensation. Science 365, 695–699. doi: 10.1126/science.aax6452
Adamantidis, A. R., Zhang, F., Aravanis, A. M., Deisseroth, K., and de Lecea, L. (2007). Neural substrates of awakening probed with optogenetic control of hypocretin neurons. Nature 450, 420–424. doi: 10.1038/nature06310
Adamsky, A., and Goshen, I. (2018). Astrocytes in memory function: pioneering findings and future directions. Neuroscience 370, 14–26. doi: 10.1016/j.neuroscience.2017.05.033
Agulhon, C., Petravicz, J., McMullen, A. B., Sweger, E. J., Minton, S. K., Taves, S. R., et al. (2008). What is the role of astrocyte calcium in neurophysiology? Neuron 59, 932–946. doi: 10.1016/j.neuron.2008.09.004
Airan, R. D., Thompson, K. R., Fenno, L. E., Bernstein, H., and Deisseroth, K. (2009). Temporally precise in vivo control of intracellular signalling. Nature 458, 1025–1029. doi: 10.1038/nature07926
Alilain, W. J., Li, X., Horn, K. P., Dhingra, R., Dick, T. E., Herlitze, S., et al. (2008). Light-induced rescue of breathing after spinal cord injury. J. Neurosci. 28, 11862–11870. doi: 10.1523/JNEUROSCI.3378-08.2008
Allen, N. J. (2014). Astrocyte regulation of synaptic behavior. Annu. Rev. Cell Dev. Biol. 30, 439–463. doi: 10.1146/annurev-cellbio-100913-013053
Araque, A., Carmignoto, G., and Haydon, P. G. (2001). Dynamic signaling between astrocytes and neurons. Annu. Rev. Physiol. 63, 795–813. doi: 10.1146/annurev.physiol.63.1.795
Araque, A., and Navarrete, M. (2010). Glial cells in neuronal network function. Philos. Trans. R. Soc. Lond. Ser. B Biol. Sci. 365, 2375–2381. doi: 10.1098/rstb.2009.0313
Arrenberg, A. B., Stainier, D. Y., Baier, H., and Huisken, J. (2010). Optogenetic control of cardiac function. Science 330, 971–974. doi: 10.1126/science.1195929
Averaimo, S., Milton, R. H., Duchen, M. R., and Mazzanti, M. (2010). Chloride intracellular channel 1 (CLIC1): sensor and effector during oxidative stress. FEBS Lett. 584, 2076–2084. doi: 10.1016/j.febslet.2010.02.073
Balachandar, L., Montejo, K. A., Castano, E., Perez, M., Moncion, C., Chambers, J. W., et al. (2020). Simultaneous ca(2+) imaging and Optogenetic stimulation of cortical astrocytes in adult murine brain slices. Curr. Protoc. Neurosci. 94:e110. doi: 10.1002/cpns.110
Bang, J., Kim, H. Y., and Lee, H. (2016). Optogenetic and Chemogenetic approaches for studying astrocytes and Gliotransmitters. Exp Neurobiol 25, 205–221. doi: 10.5607/en.2016.25.5.205
Bazargani, N., and Attwell, D. (2016). Astrocyte calcium signaling: the third wave. Nat. Neurosci. 19, 182–189. doi: 10.1038/nn.4201
Beppu, K., Sasaki, T., Tanaka, K. F., Yamanaka, A., Fukazawa, Y., Shigemoto, R., et al. (2014). Optogenetic countering of glial acidosis suppresses glial glutamate release and ischemic brain damage. Neuron 81, 314–320. doi: 10.1016/j.neuron.2013.11.011
Berger, P., Niemann, A., and Suter, U. (2006). Schwann cells and the pathogenesis of inherited motor and sensory neuropathies (Charcot-Marie-tooth disease). Glia 54, 243–257. doi: 10.1002/glia.20386
Berlinguer-Palmini, R., Narducci, R., Merhan, K., Dilaghi, A., Moroni, F., Masi, A., et al. (2014). Arrays of microLEDs and astrocytes: biological amplifiers to optogenetically modulate neuronal networks reducing light requirement. PLoS One 9:e108689. doi: 10.1371/journal.pone.0108689
Bezzi, P., and Volterra, A. (2001). A neuron-glia signalling network in the active brain. Curr. Opin. Neurobiol. 11, 387–394. doi: 10.1016/S0959-4388(00)00223-3
Bi, A., Cui, J., Ma, Y. P., Olshevskaya, E., Pu, M., Dizhoor, A. M., et al. (2006). Ectopic expression of a microbial-type rhodopsin restores visual responses in mice with photoreceptor degeneration. Neuron 50, 23–33. doi: 10.1016/j.neuron.2006.02.026
Bonin, R. P., Wang, F., Desrochers-Couture, M., Ga Secka, A., Boulanger, M. E., Cote, D. C., et al. (2016). Epidural optogenetics for controlled analgesia. Mol. Pain 12:1744806916629051. doi: 10.1177/1744806916629051
Bossuyt, J., Van Den Herrewegen, Y., Nestor, L., Buckinx, A., De Bundel, D., and Smolders, I. (2023). Chemogenetic modulation of astrocytes and microglia: state-of-the-art and implications in neuroscience. Glia 71, 2071–2095. doi: 10.1002/glia.24390
Boyd, J. G., and Gordon, T. (2003). Glial cell line-derived neurotrophic factor and brain-derived neurotrophic factor sustain the axonal regeneration of chronically axotomized motoneurons in vivo. Exp. Neurol. 183, 610–619. doi: 10.1016/S0014-4886(03)00183-3
Boyden, E. S., Zhang, F., Bamberg, E., Nagel, G., and Deisseroth, K. (2005). Millisecond-timescale, genetically targeted optical control of neural activity. Nat. Neurosci. 8, 1263–1268. doi: 10.1038/nn1525
Bruegmann, T., Malan, D., Hesse, M., Beiert, T., Fuegemann, C. J., Fleischmann, B. K., et al. (2010). Optogenetic control of heart muscle in vitro and in vivo. Nat. Methods 7, 897–900. doi: 10.1038/nmeth.1512
Brushart, T. M., Hoffman, P. N., Royall, R. M., Murinson, B. B., Witzel, C., and Gordon, T. (2002). Electrical stimulation promotes motoneuron regeneration without increasing its speed or conditioning the neuron. J. Neurosci. 22, 6631–6638. doi: 10.1523/JNEUROSCI.22-15-06631.2002
Busskamp, V., Picaud, S., Sahel, J. A., and Roska, B. (2012). Optogenetic therapy for retinitis pigmentosa. Gene Ther. 19, 169–175. doi: 10.1038/gt.2011.155
Cabezas, R., Avila, M., Gonzalez, J., El-Bacha, R. S., Baez, E., Garcia-Segura, L. M., et al. (2014). Astrocytic modulation of blood brain barrier: perspectives on Parkinson’s disease. Front. Cell. Neurosci. 8:211. doi: 10.3389/fncel.2014.00211
Caggiano, V., Sur, M., and Bizzi, E. (2014). Rostro-caudal inhibition of hindlimb movements in the spinal cord of mice. PLoS One 9:e100865. doi: 10.1371/journal.pone.0100865
Cao, G., and Ko, C. P. (2007). Schwann cell-derived factors modulate synaptic activities at developing neuromuscular synapses. J. Neurosci. 27, 6712–6722. doi: 10.1523/JNEUROSCI.1329-07.2007
Carter, M. E., Yizhar, O., Chikahisa, S., Nguyen, H., Adamantidis, A., Nishino, S., et al. (2010). Tuning arousal with optogenetic modulation of locus coeruleus neurons. Nat. Neurosci. 13, 1526–1533. doi: 10.1038/nn.2682
Chan, J. R., Cosgaya, J. M., Wu, Y. J., and Shooter, E. M. (2001). Neurotrophins are key mediators of the myelination program in the peripheral nervous system. Proc. Natl. Acad. Sci. U. S. A. 98, 14661–14668. doi: 10.1073/pnas.251543398
Cheli, V. T., Santiago Gonzalez, D. A., Spreuer, V., and Paez, P. M. (2015). Voltage-gated Ca2+ entry promotes oligodendrocyte progenitor cell maturation and myelination in vitro. Exp. Neurol. 265, 69–83. doi: 10.1016/j.expneurol.2014.12.012
Chen, J., Tan, Z., Zeng, L., Zhang, X., He, Y., Gao, W., et al. (2013). Heterosynaptic long-term depression mediated by ATP released from astrocytes. Glia 61, 178–191. doi: 10.1002/glia.22425
Cheng, M. Y., Aswendt, M., and Steinberg, G. K. (2016). Optogenetic approaches to target specific neural circuits in post-stroke recovery. Neurotherapeutics 13, 325–340. doi: 10.1007/s13311-015-0411-5
Cheng, M. Y., Wang, E. H., Woodson, W. J., Wang, S., Sun, G., Lee, A. G., et al. (2014). Optogenetic neuronal stimulation promotes functional recovery after stroke. Proc. Natl. Acad. Sci. U. S. A. 111, 12913–12918. doi: 10.1073/pnas.1404109111
Cho, W. H., Barcelon, E., and Lee, S. J. (2016). Optogenetic glia manipulation: possibilities and future prospects. Exp Neurobiol 25, 197–204. doi: 10.5607/en.2016.25.5.197
Christensen, R. N., Ha, B. K., Sun, F., Bresnahan, J. C., and Beattie, M. S. (2006). Kainate induces rapid redistribution of the actin cytoskeleton in ameboid microglia. J. Neurosci. Res. 84, 170–181. doi: 10.1002/jnr.20865
Coull, J. A., Beggs, S., Boudreau, D., Boivin, D., Tsuda, M., Inoue, K., et al. (2005). BDNF from microglia causes the shift in neuronal anion gradient underlying neuropathic pain. Nature 438, 1017–1021. doi: 10.1038/nature04223
Danbolt, N. C. (2001). Glutamate uptake. Prog. Neurobiol. 65, 1–105. doi: 10.1016/S0301-0082(00)00067-8
Davis, E. J., Foster, T. D., and Thomas, W. E. (1994). Cellular forms and functions of brain microglia. Brain Res. Bull. 34, 73–78. doi: 10.1016/0361-9230(94)90189-9
Deisseroth, K. (2010). Controlling the brain with light. Sci. Am. 303, 48–55. doi: 10.1038/scientificamerican1110-48
den Dunnen, W. F., and Meek, M. F. (2001). Sensory nerve function and auto-mutilation after reconstruction of various gap lengths with nerve guides and autologous nerve grafts. Biomaterials 22, 1171–1176. doi: 10.1016/S0142-9612(00)00339-2
Etter, G., van der Veldt, S., Manseau, F., Zarrinkoub, I., Trillaud-Doppia, E., and Williams, S. (2019). Optogenetic gamma stimulation rescues memory impairments in an Alzheimer’s disease mouse model. Nat. Commun. 10:5322. doi: 10.1038/s41467-019-13260-9
Fancy, S. P., Kotter, M. R., Harrington, E. P., Huang, J. K., Zhao, C., Rowitch, D. H., et al. (2010). Overcoming remyelination failure in multiple sclerosis and other myelin disorders. Exp. Neurol. 225, 18–23. doi: 10.1016/j.expneurol.2009.12.020
Feng, Z., and Ko, C. P. (2008). Schwann cells promote synaptogenesis at the neuromuscular junction via transforming growth factor-beta1. J. Neurosci. 28, 9599–9609. doi: 10.1523/JNEUROSCI.2589-08.2008
Ferrini, F., and De Koninck, Y. (2013). Microglia control neuronal network excitability via BDNF signalling. Neural Plast. 2013:429815. doi: 10.1155/2013/429815
Figueiredo, M., Lane, S., Stout, R. F. Jr., Liu, B., Parpura, V., Teschemacher, A. G., et al. (2014). Comparative analysis of optogenetic actuators in cultured astrocytes. Cell Calcium 56, 208–214. doi: 10.1016/j.ceca.2014.07.007
Frostick, S. P., Yin, Q., and Kemp, G. J. (1998). Schwann cells, neurotrophic factors, and peripheral nerve regeneration. Microsurgery 18, 397–405. doi: 10.1002/(SICI)1098-2752(1998)18:7<397::AID-MICR2>3.0.CO;2-F
Genain, C. P., Cannella, B., Hauser, S. L., and Raine, C. S. (1999). Identification of autoantibodies associated with myelin damage in multiple sclerosis. Nat. Med. 5, 170–175. doi: 10.1038/5532
Gerasimov, E., Erofeev, A., Borodinova, A., Bolshakova, A., Balaban, P., Bezprozvanny, I., et al. (2021). Optogenetic activation of astrocytes-effects on neuronal network function. Int. J. Mol. Sci. 22:9613. doi: 10.3390/ijms22179613
Gibson, E. M., Purger, D., Mount, C. W., Goldstein, A. K., Lin, G. L., Wood, L. S., et al. (2014). Neuronal activity promotes oligodendrogenesis and adaptive myelination in the mammalian brain. Science 344:1252304. doi: 10.1126/science.1252304
Gingras, M., Beaulieu, M. M., Gagnon, V., Durham, H. D., and Berthod, F. (2008). In vitro study of axonal migration and myelination of motor neurons in a three-dimensional tissue-engineered model. Glia 56, 354–364. doi: 10.1002/glia.20617
Goossens, M. G., Larsen, L. E., Vergaelen, M., Wadman, W., van den Haute, C., Brackx, W., et al. (2021). Level of hM4D(Gi) DREADD expression determines inhibitory and neurotoxic effects in the Hippocampus. eNeuro. 8:ENEURO.0105-21. doi: 10.1523/ENEURO.0105-21.2021
Gottmann, K., Mittmann, T., and Lessmann, V. (2009). BDNF signaling in the formation, maturation and plasticity of glutamatergic and GABAergic synapses. Exp. Brain Res. 199, 203–234. doi: 10.1007/s00221-009-1994-z
Gourine, A. V., Kasymov, V., Marina, N., Tang, F., Figueiredo, M. F., Lane, S., et al. (2010). Astrocytes control breathing through pH-dependent release of ATP. Science 329, 571–575. doi: 10.1126/science.1190721
Gradinaru, V., Mogri, M., Thompson, K. R., Henderson, J. M., and Deisseroth, K. (2009). Optical deconstruction of parkinsonian neural circuitry. Science 324, 354–359. doi: 10.1126/science.1167093
Gupta, N., Henry, R. G., Strober, J., Kang, S. M., Lim, D. A., Bucci, M., et al. (2012). Neural stem cell engraftment and myelination in the human brain. Sci. Transl. Med. 4:155ra137. doi: 10.1126/scitranslmed.3004373
Hamilton, N. B., and Attwell, D. (2010). Do astrocytes really exocytose neurotransmitters? Nat. Rev. Neurosci. 11, 227–238. doi: 10.1038/nrn2803
Harris, A. L. (2007). Connexin channel permeability to cytoplasmic molecules. Prog. Biophys. Mol. Biol. 94, 120–143. doi: 10.1016/j.pbiomolbio.2007.03.011
Hofer, T., Venance, L., and Giaume, C. (2002). Control and plasticity of intercellular calcium waves in astrocytes: a modeling approach. J. Neurosci. 22, 4850–4859. doi: 10.1523/JNEUROSCI.22-12-04850.2002
Huang, W., Begum, R., Barber, T., Ibba, V., Tee, N. C., Hussain, M., et al. (2012). Regenerative potential of silk conduits in repair of peripheral nerve injury in adult rats. Biomaterials 33, 59–71. doi: 10.1016/j.biomaterials.2011.09.030
Huang, Y. H., and Bergles, D. E. (2004). Glutamate transporters bring competition to the synapse. Curr. Opin. Neurobiol. 14, 346–352. doi: 10.1016/j.conb.2004.05.007
Huang, J., Ye, Z., Hu, X., Lu, L., and Luo, Z. (2010). Electrical stimulation induces calcium-dependent release of NGF from cultured Schwann cells. Glia 58, 622–631. doi: 10.1002/glia.20951
Hyung, S., Lee, S. R., Kim, Y. J., Bang, S., Tahk, D., Park, J. C., et al. (2019). Optogenetic neuronal stimulation promotes axon outgrowth and myelination of motor neurons in a three-dimensional motor neuron-Schwann cell coculture model on a microfluidic biochip. Biotechnol. Bioeng. 116, 2425–2438. doi: 10.1002/bit.27083
Ishibashi, T., Dakin, K. A., Stevens, B., Lee, P. R., Kozlov, S. V., Stewart, C. L., et al. (2006). Astrocytes promote myelination in response to electrical impulses. Neuron 49, 823–832. doi: 10.1016/j.neuron.2006.02.006
Iyer, S. M., Montgomery, K. L., Towne, C., Lee, S. Y., Ramakrishnan, C., Deisseroth, K., et al. (2014). Virally mediated optogenetic excitation and inhibition of pain in freely moving nontransgenic mice. Nat. Biotechnol. 32, 274–278. doi: 10.1038/nbt.2834
Jessen, K. R., and Mirsky, R. (2005). The origin and development of glial cells in peripheral nerves. Nat. Rev. Neurosci. 6, 671–682. doi: 10.1038/nrn1746
Jung, K., Kim, H. N., Jeon, N. L., and Hyung, S. (2020). Comparison of the efficacy of Optogenetic stimulation of glia versus neurons in myelination. ACS Chem. Neurosci. 11, 4280–4288. doi: 10.1021/acschemneuro.0c00542
Jung, K., Park, J. H., Kim, S. Y., Jeon, N. L., Cho, S. R., and Hyung, S. (2019). Optogenetic stimulation promotes Schwann cell proliferation, differentiation, and myelination in vitro. Sci. Rep. 9:3487. doi: 10.1038/s41598-019-40173-w
Khalili-Shirazi, A., Atkinson, P., Gregson, N., and Hughes, R. A. (1993). Antibody responses to P0 and P2 myelin proteins in Guillain-Barre syndrome and chronic idiopathic demyelinating polyradiculoneuropathy. J. Neuroimmunol. 46, 245–251. doi: 10.1016/0165-5728(93)90255-W
Kimelberg, H. K., and Nedergaard, M. (2010). Functions of astrocytes and their potential as therapeutic targets. Neurotherapeutics 7, 338–353. doi: 10.1016/j.nurt.2010.07.006
Klapper, S. D., Sauter, E. J., Swiersy, A., Hyman, M. A. E., Bamann, C., Bamberg, E., et al. (2017). On-demand optogenetic activation of human stem-cell-derived neurons. Sci. Rep. 7:14450. doi: 10.1038/s41598-017-14827-6
Koski, C. L. (1990). Characterization of complement-fixing antibodies to peripheral nerve myelin in Guillain-Barre syndrome. Ann. Neurol. 27, S44–S47. doi: 10.1002/ana.410270712
Krook-Magnuson, E., Armstrong, C., Oijala, M., and Soltesz, I. (2013). On-demand optogenetic control of spontaneous seizures in temporal lobe epilepsy. Nat. Commun. 4:1376. doi: 10.1038/ncomms2376
Kyung, T., Lee, S., Kim, J. E., Cho, T., Park, H., Jeong, Y. M., et al. (2015). Optogenetic control of endogenous ca(2+) channels in vivo. Nat. Biotechnol. 33, 1092–1096. doi: 10.1038/nbt.3350
Land, B. B., Brayton, C. E., Furman, K. E., Lapalombara, Z., and Dileone, R. J. (2014). Optogenetic inhibition of neurons by internal light production. Front. Behav. Neurosci. 8:108. doi: 10.3389/fnbeh.2014.00108
Lee, H. U., Nag, S., Blasiak, A., Jin, Y., Thakor, N., and Yang, I. H. (2016). Subcellular Optogenetic stimulation for activity-dependent myelination of axons in a novel microfluidic compartmentalized platform. ACS Chem. Neurosci. 7, 1317–1324. doi: 10.1021/acschemneuro.6b00157
Li, X., Gutierrez, D. V., Hanson, M. G., Han, J., Mark, M. D., Chiel, H., et al. (2005). Fast noninvasive activation and inhibition of neural and network activity by vertebrate rhodopsin and green algae channelrhodopsin. Proc. Natl. Acad. Sci. U. S. A. 102, 17816–17821. doi: 10.1073/pnas.0509030102
Li, D., Herault, K., Isacoff, E. Y., Oheim, M., and Ropert, N. (2012). Optogenetic activation of LiGluR-expressing astrocytes evokes anion channel-mediated glutamate release. J. Physiol. 590, 855–873. doi: 10.1113/jphysiol.2011.219345
Lim, D. H., LeDue, J. M., Mohajerani, M. H., and Murphy, T. H. (2014). Optogenetic mapping after stroke reveals network-wide scaling of functional connections and heterogeneous recovery of the peri-infarct. J. Neurosci. 34, 16455–16466. doi: 10.1523/JNEUROSCI.3384-14.2014
Lindsay, T. H., Thiele, T. R., and Lockery, S. R. (2011). Optogenetic analysis of synaptic transmission in the central nervous system of the nematode Caenorhabditis elegans. Nat. Commun. 2:306. doi: 10.1038/ncomms1304
Liu, Q. S., Xu, Q., Arcuino, G., Kang, J., and Nedergaard, M. (2004). Astrocyte-mediated activation of neuronal kainate receptors. Proc. Natl. Acad. Sci. U. S. A. 101, 3172–3177. doi: 10.1073/pnas.0306731101
Madry, C., Kyrargyri, V., Arancibia-Carcamo, I. L., Jolivet, R., Kohsaka, S., Bryan, R. M., et al. (2018). Microglial ramification, surveillance, and interleukin-1beta release are regulated by the two-pore domain K(+) channel THIK-1. Neuron 97, 299–312 e6. doi: 10.1016/j.neuron.2017.12.002
Mahn, M., Prigge, M., Ron, S., Levy, R., and Yizhar, O. (2016). Biophysical constraints of optogenetic inhibition at presynaptic terminals. Nat. Neurosci. 19, 554–556. doi: 10.1038/nn.4266
Masamoto, K., Unekawa, M., Watanabe, T., Toriumi, H., Takuwa, H., Kawaguchi, H., et al. (2015). Unveiling astrocytic control of cerebral blood flow with optogenetics. Sci. Rep. 5:11455. doi: 10.1038/srep11455
McTigue, D. M., Horner, P. J., Stokes, B. T., and Gage, F. H. (1998). Neurotrophin-3 and brain-derived neurotrophic factor induce oligodendrocyte proliferation and myelination of regenerating axons in the contused adult rat spinal cord. J. Neurosci. 18, 5354–5365. doi: 10.1523/JNEUROSCI.18-14-05354.1998
Mederos, S., Hernandez-Vivanco, A., Ramirez-Franco, J., Martin-Fernandez, M., Navarrete, M., Yang, A., et al. (2019). Melanopsin for precise optogenetic activation of astrocyte-neuron networks. Glia 67, 915–934. doi: 10.1002/glia.23580
Meister, J., Wang, L., Pydi, S., and Wess, J. (2021). Chemogenetic approaches to identify metabolically important GPCR signaling pathways: therapeutic implications. J. Neurochem. 158, 603–620. doi: 10.1111/jnc.15314
Mothet, J. P., Parent, A. T., Wolosker, H., Brady, R. O. Jr., Linden, D. J., Ferris, C. D., et al. (2000). D-serine is an endogenous ligand for the glycine site of the N-methyl-D-aspartate receptor. Proc. Natl. Acad. Sci. U. S. A. 97, 4926–4931. doi: 10.1073/pnas.97.9.4926
Muna, T. H. (2020). Engineeering optogenetically controlled Schwann cells. [dissertation/master’s thesis]: University of Wyoming
Nam, Y., Kim, J. H., Kim, J. H., Jha, M. K., Jung, J. Y., Lee, M. G., et al. (2016). Reversible induction of pain hypersensitivity following Optogenetic stimulation of spinal astrocytes. Cell Rep. 17, 3049–3061. doi: 10.1016/j.celrep.2016.11.043
Newell, E. W., and Schlichter, L. C. (2005). Integration of K+ and cl- currents regulate steady-state and dynamic membrane potentials in cultured rat microglia. J. Physiol. 567, 869–890. doi: 10.1113/jphysiol.2005.092056
Ono, K., Suzuki, H., Higa, M., Tabata, K., and Sawada, M. (2014). Glutamate release from astrocyte cell-line GL261 via alterations in the intracellular ion environment. J. Neural Transm. (Vienna) 121, 245–257. doi: 10.1007/s00702-013-1096-8
Orthmann-Murphy, J. L., Abrams, C. K., and Scherer, S. S. (2008). Gap junctions couple astrocytes and oligodendrocytes. J. Mol. Neurosci. 35, 101–116. doi: 10.1007/s12031-007-9027-5
Park, S., Koppes, R. A., Froriep, U. P., Jia, X., Achyuta, A. K., McLaughlin, B. L., et al. (2015). Optogenetic control of nerve growth. Sci. Rep. 5:9669. doi: 10.1038/srep09669
Parpura, V., Basarsky, T. A., Liu, F., Jeftinija, K., Jeftinija, S., and Haydon, P. G. (1994). Glutamate-mediated astrocyte-neuron signalling. Nature 369, 744–747. doi: 10.1038/369744a0
Patel, P. I., Roa, B. B., Welcher, A. A., Schoener-Scott, R., Trask, B. J., Pentao, L., et al. (1992). The gene for the peripheral myelin protein PMP-22 is a candidate for Charcot-Marie-tooth disease type 1A. Nat. Genet. 1, 159–165. doi: 10.1038/ng0692-159
Pelluru, D., Konadhode, R. R., Bhat, N. R., and Shiromani, P. J. (2016). Optogenetic stimulation of astrocytes in the posterior hypothalamus increases sleep at night in C57BL/6J mice. Eur. J. Neurosci. 43, 1298–1306. doi: 10.1111/ejn.13074
Perea, G., Navarrete, M., and Araque, A. (2009). Tripartite synapses: astrocytes process and control synaptic information. Trends Neurosci. 32, 421–431. doi: 10.1016/j.tins.2009.05.001
Perea, G., Yang, A., Boyden, E. S., and Sur, M. (2014). Optogenetic astrocyte activation modulates response selectivity of visual cortex neurons in vivo. Nat. Commun. 5:3262. doi: 10.1038/ncomms4262
Petravicz, J., Fiacco, T. A., and McCarthy, K. D. (2008). Loss of IP3 receptor-dependent Ca2+ increases in hippocampal astrocytes does not affect baseline CA1 pyramidal neuron synaptic activity. J. Neurosci. 28, 4967–4973. doi: 10.1523/JNEUROSCI.5572-07.2008
Philips, T., Bento-Abreu, A., Nonneman, A., Haeck, W., Staats, K., Geelen, V., et al. (2013). Oligodendrocyte dysfunction in the pathogenesis of amyotrophic lateral sclerosis. Brain 136, 471–482. doi: 10.1093/brain/aws339
Porter, J. T., and McCarthy, K. D. (1997). Astrocytic neurotransmitter receptors in situ and in vivo. Prog. Neurobiol. 51, 439–455. doi: 10.1016/S0301-0082(96)00068-8
Poskanzer, K. E., and Yuste, R. (2016). Astrocytes regulate cortical state switching in vivo. Proc. Natl. Acad. Sci. U. S. A. 113, E2675–E2684. doi: 10.1073/pnas.1520759113
Roth, B. L. (2016). DREADDs for neuroscientists. Neuron 89, 683–694. doi: 10.1016/j.neuron.2016.01.040
Rothstein, J. D., Martin, L., Levey, A. I., Dykes-Hoberg, M., Jin, L., Wu, D., et al. (1994). Localization of neuronal and glial glutamate transporters. Neuron 13, 713–725. doi: 10.1016/0896-6273(94)90038-8
Ruts, L., van Doorn, P. A., Lombardi, R., Haasdijk, E. D., Penza, P., Tulen, J. H. M., et al. (2012). Unmyelinated and myelinated skin nerve damage in Guillain-Barre syndrome: correlation with pain and recovery. Pain 153, 399–409. doi: 10.1016/j.pain.2011.10.037
Sahlender, D. A., Savtchouk, I., and Volterra, A. (2014). What do we know about gliotransmitter release from astrocytes? Philos. Trans. R. Soc. Lond. Ser. B Biol. Sci. 369:20130592. doi: 10.1098/rstb.2013.0592
Salzer, J. L. (2015). Schwann cell myelination. Cold Spring Harb. Perspect. Biol. 7:a020529. doi: 10.1101/cshperspect.a020529
Sasaki, T., Beppu, K., Tanaka, K. F., Fukazawa, Y., Shigemoto, R., and Matsui, K. (2012). Application of an optogenetic byway for perturbing neuronal activity via glial photostimulation. Proc. Natl. Acad. Sci. U. S. A. 109, 20720–20725. doi: 10.1073/pnas.1213458109
Sasaki, T., Kuga, N., Namiki, S., Matsuki, N., and Ikegaya, Y. (2011). Locally synchronized astrocytes. Cereb. Cortex 21, 1889–1900. doi: 10.1093/cercor/bhq256
Scemes, E., and Giaume, C. (2006). Astrocyte calcium waves: what they are and what they do. Glia 54, 716–725. doi: 10.1002/glia.20374
Seeger-Armbruster, S., Bosch-Bouju, C., Little, S. T., Smither, R. A., Hughes, S. M., Hyland, B. I., et al. (2015). Patterned, but not tonic, optogenetic stimulation in motor thalamus improves reaching in acute drug-induced parkinsonian rats. J. Neurosci. 35, 1211–1216. doi: 10.1523/JNEUROSCI.3277-14.2015
Sereda, M. W., Meyer zu Hörste, G., Suter, U., Uzma, N., and Nave, K. A. (2003). Therapeutic administration of progesterone antagonist in a model of Charcot-Marie-tooth disease (CMT-1A). Nat. Med. 9, 1533–1537. doi: 10.1038/nm957
Shen, Y., Campbell, R. E., Cote, D. C., and Paquet, M. E. (2020). Challenges for therapeutic applications of opsin-based Optogenetic tools in humans. Front Neural Circuits 14:41. doi: 10.3389/fncir.2020.00041
Sheppard, C. A., Simpson, P. B., Sharp, A. H., Nucifora, F. C., Ross, C. A., Lange, G. D., et al. (1997). Comparison of type 2 inositol 1,4,5-trisphosphate receptor distribution and subcellular Ca2+ release sites that support Ca2+ waves in cultured astrocytes. J. Neurochem. 68, 2317–2327. doi: 10.1046/j.1471-4159.1997.68062317.x
Sinnen, B. L., Bowen, A. B., Forte, J. S., Hiester, B. G., Crosby, K. C., Gibson, E. S., et al. (2017). Optogenetic control of synaptic composition and function. Neuron 93, 646–660 e5. doi: 10.1016/j.neuron.2016.12.037
Soliven, B. (2001). Calcium signalling in cells of oligodendroglial lineage. Microsc. Res. Tech. 52, 672–679. doi: 10.1002/jemt.1051
Sparta, D. R., Jennings, J. H., Ung, R. L., and Stuber, G. D. (2013). Optogenetic strategies to investigate neural circuitry engaged by stress. Behav. Brain Res. 255, 19–25. doi: 10.1016/j.bbr.2013.05.007
Stirman, J. N., Crane, M. M., Husson, S. J., Wabnig, S., Schultheis, C., Gottschalk, A., et al. (2011). Real-time multimodal optical control of neurons and muscles in freely behaving Caenorhabditis elegans. Nat. Methods 8, 153–158. doi: 10.1038/nmeth.1555
Stuber, G. D., Britt, J. P., and Bonci, A. (2012). Optogenetic modulation of neural circuits that underlie reward seeking. Biol. Psychiatry 71, 1061–1067. doi: 10.1016/j.biopsych.2011.11.010
Sutherland, M. L., Delaney, T. A., and Noebels, J. L. (1996). Glutamate transporter mRNA expression in proliferative zones of the developing and adult murine CNS. J. Neurosci. 16, 2191–2207. doi: 10.1523/JNEUROSCI.16-07-02191.1996
Takahashi, N., Sakurai, T., Davis, K. L., and Buxbaum, J. D. (2011). Linking oligodendrocyte and myelin dysfunction to neurocircuitry abnormalities in schizophrenia. Prog. Neurobiol. 93, 13–24. doi: 10.1016/j.pneurobio.2010.09.004
Takata, N., Sugiura, Y., Yoshida, K., Koizumi, M., Hiroshi, N., Honda, K., et al. (2018). Optogenetic astrocyte activation evokes BOLD fMRI response with oxygen consumption without neuronal activity modulation. Glia 66, 2013–2023. doi: 10.1002/glia.23454
Tanaka, K. F., Matsui, K., Sasaki, T., Sano, H., Sugio, S., Fan, K., et al. (2012). Expanding the repertoire of optogenetically targeted cells with an enhanced gene expression system. Cell Rep. 2, 397–406. doi: 10.1016/j.celrep.2012.06.011
Tang, F., Lane, S., Korsak, A., Paton, J. F., Gourine, A. V., Kasparov, S., et al. (2014). Lactate-mediated glia-neuronal signalling in the mammalian brain. Nat. Commun. 5:3284. doi: 10.1038/ncomms4284
Theis, M., and Giaume, C. (2012). Connexin-based intercellular communication and astrocyte heterogeneity. Brain Res. 1487, 88–98. doi: 10.1016/j.brainres.2012.06.045
Theis, M., Jauch, R., Zhuo, L., Speidel, D., Wallraff, A., Doring, B., et al. (2003). Accelerated hippocampal spreading depression and enhanced locomotory activity in mice with astrocyte-directed inactivation of connexin43. J. Neurosci. 23, 766–776. doi: 10.1523/JNEUROSCI.23-03-00766.2003
Tkachev, D., Mimmack, M. L., Ryan, M. M., Wayland, M., Freeman, T., Jones, P. B., et al. (2003). Oligodendrocyte dysfunction in schizophrenia and bipolar disorder. Lancet 362, 798–805. doi: 10.1016/S0140-6736(03)14289-4
Tonegawa, S., Pignatelli, M., Roy, D. S., and Ryan, T. J. (2015). Memory engram storage and retrieval. Curr. Opin. Neurobiol. 35, 101–109. doi: 10.1016/j.conb.2015.07.009
Towne, C., Montgomery, K. L., Iyer, S. M., Deisseroth, K., and Delp, S. L. (2013). Optogenetic control of targeted peripheral axons in freely moving animals. PLoS One 8:e72691. doi: 10.1371/journal.pone.0072691
Tzingounis, A. V., and Wadiche, J. I. (2007). Glutamate transporters: confining runaway excitation by shaping synaptic transmission. Nat. Rev. Neurosci. 8, 935–947. doi: 10.1038/nrn2274
Uchida, N., Chen, K., Dohse, M., Hansen, K. D., Dean, J., Buser, J. R., et al. (2012). Human neural stem cells induce functional myelination in mice with severe dysmyelination. Sci. Transl. Med. 4:155ra136. doi: 10.1126/scitranslmed.3004371
Walz, W. (2000). Role of astrocytes in the clearance of excess extracellular potassium. Neurochem. Int. 36, 291–300. doi: 10.1016/S0197-0186(99)00137-0
Wang, X., Takano, T., and Nedergaard, M. (2009). Astrocytic calcium signaling: mechanism and implications for functional brain imaging. Methods Mol. Biol. 489, 93–109. doi: 10.1007/978-1-59745-543-5_5
Williams, J. C., and Denison, T. (2013). From optogenetic technologies to neuromodulation therapies. Sci. Transl. Med. 5:177ps6. doi: 10.1126/scitranslmed.3003100
Wu, C., Ivanova, E., Zhang, Y., and Pan, Z. H. (2013). rAAV-mediated subcellular targeting of optogenetic tools in retinal ganglion cells in vivo. PLoS One 8:e66332. doi: 10.1371/journal.pone.0066332
Xiao, Q., Xu, X., and Tu, J. (2020). Chronic optogenetic manipulation of basolateral amygdala astrocytes rescues stress-induced anxiety. Biochem. Biophys. Res. Commun. 533, 657–664. doi: 10.1016/j.bbrc.2020.09.106
Xie, Y., Chen, S., Anenberg, E., and Murphy, T. H. (2013). Resistance of optogenetically evoked motor function to global ischemia and reperfusion in mouse in vivo. J. Cereb. Blood Flow Metab. 33, 1148–1152. doi: 10.1038/jcbfm.2013.89
Xu, X., Yee, W. C., Hwang, P. Y., Yu, H., Wan, A. C., Gao, S., et al. (2003). Peripheral nerve regeneration with sustained release of poly(phosphoester) microencapsulated nerve growth factor within nerve guide conduits. Biomaterials 24, 2405–2412. doi: 10.1016/S0142-9612(03)00109-1
Yamamoto, K., Tanei, Z. I., Hashimoto, T., Wakabayashi, T., Okuno, H., Naka, Y., et al. (2015). Chronic optogenetic activation augments abeta pathology in a mouse model of Alzheimer disease. Cell Rep. 11, 859–865. doi: 10.1016/j.celrep.2015.04.017
Yamashita, A., Hamada, A., Suhara, Y., Kawabe, R., Yanase, M., Kuzumaki, N., et al. (2014). Astrocytic activation in the anterior cingulate cortex is critical for sleep disorder under neuropathic pain. Synapse 68, 235–247. doi: 10.1002/syn.21733
Yamazaki, Y., Abe, Y., Shibata, S., Shindo, T., Fujii, S., Ikenaka, K., et al. (2019). Region- and cell type-specific facilitation of synaptic function at destination synapses induced by oligodendrocyte depolarization. J. Neurosci. 39, 4036–4050. doi: 10.1523/JNEUROSCI.1619-18.2019
Yamazaki, Y., Fujiwara, H., Kaneko, K., Hozumi, Y., Xu, M., Ikenaka, K., et al. (2014). Short- and long-term functional plasticity of white matter induced by oligodendrocyte depolarization in the hippocampus. Glia 62, 1299–1312. doi: 10.1002/glia.22681
Yoon, B. E., and Lee, C. J. (2014). GABA as a rising gliotransmitter. Front Neural Circuits 8:141. doi: 10.3389/fncir.2014.00141
Yoon, H. H., Park, J. H., Kim, Y. H., Min, J., Hwang, E., Lee, C. J., et al. (2014). Optogenetic inactivation of the subthalamic nucleus improves forelimb akinesia in a rat model of Parkinson disease. Neurosurgery 74, 533–540. doi: 10.1227/NEU.0000000000000297
Zhang, Y., Luo, H., Zhang, Z., Lu, Y., Huang, X., Yang, L., et al. (2010). A nerve graft constructed with xenogeneic acellular nerve matrix and autologous adipose-derived mesenchymal stem cells. Biomaterials 31, 5312–5324. doi: 10.1016/j.biomaterials.2010.03.029
Zhou, D., Srivastava, R., Nessler, S., Grummel, V., Sommer, N., Bruck, W., et al. (2006). Identification of a pathogenic antibody response to native myelin oligodendrocyte glycoprotein in multiple sclerosis. Proc. Natl. Acad. Sci. U. S. A. 103, 19057–19062. doi: 10.1073/pnas.0607242103
Zhuge, Y., Patlolla, B., Ramakrishnan, C., Beygui, R. E., Zarins, C. K., Deisseroth, K., et al. (2014). Human pluripotent stem cell tools for cardiac optogenetics. Annu Int Conf IEEE Eng Med Biol Soc 2014, 6171–6174. doi: 10.1109/EMBC.2014.6945038
Keywords: optogenetics, astrocyte, microglia, oligodendrocyte, Schwann cell, neuron–glia crosstalk
Citation: Hyung S, Park J-H and Jung K (2023) Application of optogenetic glial cells to neuron–glial communication. Front. Cell. Neurosci. 17:1249043. doi: 10.3389/fncel.2023.1249043
Edited by:
Maria Grazia Mola, University of Bari Aldo Moro, ItalyReviewed by:
Ken Berglund, Emory University, United StatesHyungju Park, Korea Brain Research Institute, Republic of Korea
Copyright © 2023 Hyung, Park and Jung. This is an open-access article distributed under the terms of the Creative Commons Attribution License (CC BY). The use, distribution or reproduction in other forums is permitted, provided the original author(s) and the copyright owner(s) are credited and that the original publication in this journal is cited, in accordance with accepted academic practice. No use, distribution or reproduction is permitted which does not comply with these terms.
*Correspondence: Sujin Hyung, cy5oeXVuZ0BzYW1zdW5nLmNvbQ==