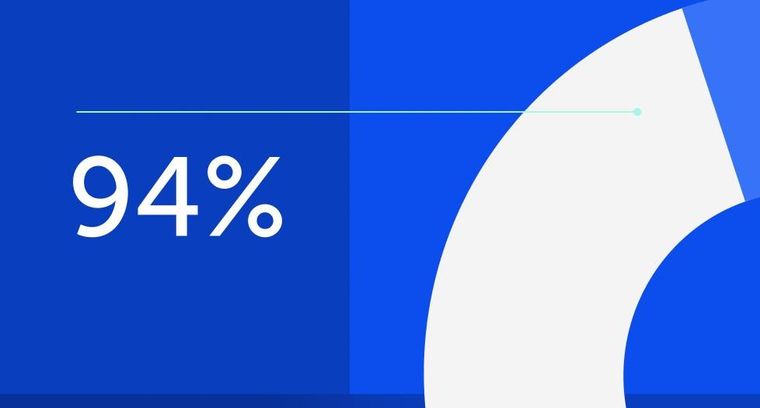
94% of researchers rate our articles as excellent or good
Learn more about the work of our research integrity team to safeguard the quality of each article we publish.
Find out more
MINI REVIEW article
Front. Cell. Neurosci., 13 July 2023
Sec. Cellular Neurophysiology
Volume 17 - 2023 | https://doi.org/10.3389/fncel.2023.1237589
Presynaptic plasticity is an activity-dependent change in the neurotransmitter release and plays a key role in dynamic modulation of synaptic strength. Particularly, presynaptic potentiation mediated by cyclic adenosine monophosphate (cAMP) is widely seen across the animals and thought to contribute to learning and memory. Hippocampal mossy fiber-CA3 pyramidal cell synapses have been used as a model because of robust presynaptic potentiation in short- and long-term forms. Moreover, direct presynaptic recordings from large mossy fiber terminals allow one to dissect the potentiation mechanisms. Recently, super-resolution microscopy and flash-and-freeze electron microscopy have revealed the localizations of release site molecules and synaptic vesicles during the potentiation at a nanoscale, identifying the molecular mechanisms of the potentiation. Incorporating these growing knowledges, we try to present plausible mechanisms underlying the cAMP-mediated presynaptic potentiation.
Neurotransmitters are released from presynaptic terminals and bound to postsynaptic receptors, carrying neuronal signals from one cell to another. When an action potential (AP) arrives at the presynaptic terminal, voltage-gated Ca2+ channels are activated and mediate Ca2+ influx that initiates exocytosis of synaptic vesicles (SVs) filled with neurotransmitters. This process takes place in the nanoscopic domains called active zones (AZs), defined and structured by a set of molecules organizing the release (Südhof, 2012; Sakamoto et al., 2018; Walter et al., 2018). The amounts of SV release, together with postsynaptic factors such as the number and the density of receptors, sets weighting of the synaptic transmission (Atwood and Karunanithi, 2002). The SV release is dynamically regulated by neuronal activities, shaping presynaptic plasticity, which brings a plastic nature to neural circuit computation (Jackman and Regehr, 2017; Monday et al., 2018). For transmitter release, the processes of SV docking/priming, Ca2+ sensing and fusion are crucial. However, the molecular changes underlying presynaptic plasticity largely remains elusive.
Glutamatergic synapses between mammalian hippocampal mossy fibers and CA3 pyramidal cells (MF-CA3 synapses) show cAMP-mediated presynaptic potentiation (Weisskopf et al., 1994; Nicoll and Schmitz, 2005), one of the well documented types of plasticity across the animals thought to underlie learning and memory (Kandel, 2001; Heisenberg, 2003; Castillo, 2012). The cAMP-mediated presynaptic potentiation at the MF-CA3 synapses is induced by tetanic stimulation of presynaptic neurons, dentate gyrus granule cells (GCs), via protein kinase A (PKA) activation (Zalutsky and Nicoll, 1990; Weisskopf et al., 1994; Huang and Kandel, 1996). One CA3 pyramidal cell receives a single synaptic contact from one GC (Acsády et al., 1998; Delvendahl et al., 2013), and a large size of hippocampal mossy fiber boutons (hMFBs) allows for direct presynaptic patch-clamp recordings (Geiger and Jonas, 2000; Hallermann et al., 2003) and live imaging (Regehr et al., 1994; Kamiya et al., 2002; Midorikawa and Sakaba, 2017). Due to the simple induction protocols and the unique anatomical features, the MF-CA3 synapse has been used as a model for presynaptic potentiation.
It is generally considered that SVs are released from the SV population ready to be released by stimulation (readily-releasable pool; RRP) (Zucker and Regehr, 2002; Rizzoli and Betz, 2005; Kaeser and Regehr, 2017). The neurotransmitter release is often described as a function of the number of SVs within the RRP (NRRP) and release probabilities of SVs in the RRP (Pr). It is unclear whether NRRP is the same as N (the number of release sites) defined from the quantal hypothesis of SV release (del Castillo and Katz, 1954; Kaeser and Regehr, 2017; Pulido and Marty, 2017; Sakaba, 2018). Here, we use the term NRRP as the number of SVs released by presynaptic depolarization, as described below. Electrophysiological analyses are used to examine if either an increase in NRRP or Pr is responsible for cAMP-mediated potentiation at MF-CA3 synapses. However, the results are equivocal: Some studies rather support an increase of NRRP (Vandael et al., 2020), while others support an increase in Pr (Weisskopf et al., 1994; Fukaya et al., 2023), or both (Reid et al., 2004). Recent developments of super-resolution microscopy and flash-and-freeze electron microscopy have allowed linking the physiological outcomes with localizations of AZ molecules and SVs during the potentiation (Imig et al., 2020; Vandael et al., 2020; Fukaya et al., 2023). We here incorporate these recent findings and try to present a coherent view of mechanistic changes underlying cAMP-mediated presynaptic potentiation.
Recent conceptual advances offer molecular mechanisms of Pr. SVs undergo tethering and docking/priming at the release sites (Sakaba, 2018). It has been considered that the docked SVs are classified into loosely- and tightly-docked SVs, detected as a difference in distances from presynaptic plasma membrane, and that Pr increases in the course of SVs getting docked loosely and then tightly to the release sites (Neher and Brose, 2018; Figure 1A). The tightening might advance molecular priming and increase fusion competence, presumably corresponding to progression of zippering of SNARE complex (Neher and Brose, 2018). The idea of loose and tight docking states may be comparable to such concepts as “slow and fast releasing pools” (Sakaba, 2006; Ritzau-Jost et al., 2014), “primed and super-primed SVs” (Lee et al., 2012; Taschenberger et al., 2016) and “replacement and docking sites” (Miki et al., 2016). However, correspondence among these terms needs further characterization.
Figure 1. SV release parameters at hMFBs. (A) Schematic illustration of SV dynamics in the RRP at hMFBs. As a tethered SV becomes docked loosely then tightly, Pr of the SV is thought to increase with progression of molecular priming. It has been proposed that tethered and loosely- and tightly-docked SVs are located ∼50 nm, ∼8–10 nm, and within 2–4 nm from the presynaptic plasma membrane, respectively (Neher and Brose, 2018). (B) Scheme of direct presynaptic patch-clamp recordings at MF-CA3 synapses. Membrane capacitance (Cm) recorded under voltage clamp condition allows for quantification of SV release, because an increase in Cm linearly correlates with the number of exocytosed SVs estimated from the EPSCs (∼0.1 fF/SV). (C) Cm increases under the basal condition are plotted against the durations of depolarization (circles). The release can be dissected into exponential (solid) and linear components (dashed), representing release from the RRP and the following replenishment of the RRP, respectively. The amplitude of the exponential component provides an estimate of NRRP. Note that a faster time course of the exponential component represents higher Pr. In panels (B,C), the trace and the plotted data are presented in Fukaya et al. (2023).
The fusion process requires Ca2+ binding to the sensor proteins of the SVs (Eggermann et al., 2011; Jackman and Regehr, 2017). In addition to the Ca2+ sensing properties, the coupling distance between Ca2+ channels and docked SVs is also crucial for Pr (“positional priming”) (Wadel et al., 2007; Neher and Sakaba, 2008). Tighter coupling is mechanistically realized by shorter physical distances between Ca2+ channels and SVs and larger Ca2+ influx in the AZs. It follows that fusion competence and Ca2+ channel-SV release coupling synergistically determine Pr.
Direct presynaptic patch-clamp recording in hMFBs allows one to quantify the SV release as an increase in membrane capacitance caused by SV exocytosis (Hallermann et al., 2003). Depolarization strong enough to deplete the RRP provides estimation of the NRRP around 500–1,000 SVs/bouton (Hallermann et al., 2003; Miyano et al., 2019; Fukaya et al., 2023; Figures 1B, C). The RRP is depleted with a time constant of ∼10–40 ms and refilled with that of hundreds of milliseconds (Miyano et al., 2019; Fukaya et al., 2023). NRRP seems larger than the number of tightly-docked SVs (∼300 SVs/bouton) and rather matches the number of SVs localized within ∼50 nm of the AZs (∼900 SVs/bouton), which includes both docked and undocked (i.e., tethered) SVs (Rollenhagen et al., 2007; Maus et al., 2020; Figures 1A, 2). Nevertheless, whether undocked/tethered SVs are included in the RRP or not is a matter of debate (Kaeser and Regehr, 2017). It is appreciated that docked SVs might be a major SV source for AP-evoked release (Imig et al., 2014, 2020; Borges-Merjane et al., 2020). One AP triggers release of ∼2–20 SVs/bouton in most cases (Jonas et al., 1993; Chamberland et al., 2014; Vyleta and Jonas, 2014; Vandael et al., 2020). This value seems equivalent to at most ∼10% of the total NRRP. The basal low Pr of the hMFBs has been explained by loose Ca2+ channel-docked SV coupling, characterized by relatively long physical distances in between (∼80 nm) and fast Ca2+ buffering in the AZs (Vyleta and Jonas, 2014).
Figure 2. Possible mechanistic changes underlying mfPTP and mfLTP. Schematic illustrations of the RRP in the basal condition (top) and during mfPTP (middle) and mfLTP (bottom). In (top), equilibriums between the reserve pool and RRP (a), between undocked/tethered and docked states of the SVs in the RRP (b) and between loose and tight states of the docked SVs (c) are indicated. The mfPTP phase would return to the basal situation, if tetanic stimulation is not strong enough to permit mfLTP, where the potentiation seems more solidified by the increases of AZ proteins and less reversible. At the PTP phase, NRRP and the number of docked SVs are increased. SV replenishment to the RRP (arrow 1) and/or docking actions of SVs (arrow 2) could be promoted, helping some docked SVs to become tightly docked (arrow 3). It is also possible that the docking states are directly modulated (arrow 3). Actin could be involved in these three steps potentially (see text). At the LTP phase, Munc13-1 and RIM1 are increased, increasing fusion competence of the docked SVs. These increased AZ components might stabilize the docked SVs in a tight state, by regulating docking state directly (arrow ii) and/or promoting docking of SVs that helps docked SVs to roll into a tight state (arrows i→ii). In ether scenario, NRRP is unchanged, and Pr is increased.
A large NRRP with low Pr of MF-CA3 synapses is a typical feature of high-pass filtering “tonic” synapses (Neher and Brose, 2018), showing prominent synaptic facilitation during train stimulation (Salin et al., 1996; Geiger and Jonas, 2000). Pr increases as the repetitive stimulation goes on via broadening of an AP waveform (Geiger and Jonas, 2000; but see Chamberland et al., 2014). Activation of presynaptic kainate (Lauri et al., 2001; Schmitz et al., 2001; but see Kwon and Castillo, 2008) or NMDA receptors (Lituma et al., 2021), Ca2+ release from intracellular organelles (Shimizu et al., 2008) or saturation of Ca2+ buffers in the AZs (Vyleta and Jonas, 2014) can also contribute to the facilitation.
Tetanic stimulation has been applied by bulk electrical stimulation at the mossy fiber tract. Recent studies have used single-bouton stimulation with a presynaptic patch-clamp electrode (Vyleta and Jonas, 2014; Vyleta et al., 2016; Vandael et al., 2020, 2021) and optogenetic tools enabling tetanic stimulation by light illumination (Ben-Simon et al., 2015; Fukaya et al., 2023), successfully inducing robust potentiation. Ca2+ entry at hMFBs during tetanic stimulation plays a role in induction of potentiation afterward (Castillo et al., 1994; Tong et al., 1996; Breustedt et al., 2003), presumably leading to cAMP/PKA activation mediated by adenylate cyclase 1 (Huang and Kandel, 1996) and 8 (Wang et al., 2003). After tetanic stimulation is applied to GC axons, the synaptic response immediately amplifies ∼5-fold and then attenuates to the basal level in several minutes (Griffith, 1990), showing post-tetanic potentiation (PTP). When repetition and length of the train stimulation are increased to a sufficient level, PTP is followed by ∼2-fold potentiation lasting for at least tens of minutes, shaping long-term potentiation (LTP) (Zalutsky and Nicoll, 1990). Both PTP and LTP are suppressed by PKA inhibitors at MF-CA3 synapses (Weisskopf et al., 1994; Vandael et al., 2020), while, at other synapses, presynaptic PTP and LTP can be induced by different molecular pathways such as protein kinase C cascade (Alle et al., 2001; Korogod et al., 2007; Castillo, 2012). For clarification, PTP and LTP in hMFBs are herein termed mfPTP and mfLTP, respectively.
In addition to tetanic stimulation, pharmacological activation of cAMP/PKA pathway with cAMP analogues or an adenylate cyclase activator, forskolin, has also been used for potentiation (“chemical potentiation”) (Weisskopf et al., 1994; Midorikawa and Sakaba, 2017; Fukaya et al., 2021; Orlando et al., 2021). Although the chemical potentiation is robust and prevails throughout the preparation, it unlikely shares the induction pathway completely with mfPTP or mfLTP: Some presynaptic molecules, such as Rab3a and RIM1alpha, are responsible for mfLTP, not for chemical potentiation (Castillo et al., 1997, 2002), and vice versa (Shahoha et al., 2022).
Vandael et al. (2020) induced mfPTP via a cell-attached presynaptic patch electrode and analyzed postsynaptic currents recorded from the paired CA3 pyramidal cell. They concluded that mfPTP is attributed largely to an increase in NRRP, while a slight increase in Pr can also contribute. In addition, the flash-and-freeze electron microscopy revealed that the number of the docked SVs is increased within a minute after the optical tetanic stimulation. Interestingly, such changes have been also observed, when chemical potentiation is induced by 15 min forskolin application (Orlando et al., 2021).
The increased docked SVs during mfPTP might be caused by (1) increased refilling rate from the reserve pool to the RRP (arrow 1 in Figure 2) and/or (2) increased rate of SV docking (arrow 2 in Figure 2). It is also possible that (3) promoting loosely-docked SVs into tightly-docked ones (arrow 3 in Figure 2) contributes to mfPTP (Taschenberger et al., 2016). In addition to the increased forward rates, the increased number of docked SVs may be caused by a shift in the equilibrium between undocked and docked states (arrows b in Figure 2, top, Hosoi et al., 2007). At the calyx of Held, Lee et al. (2010) have proposed such a scenario. Vandael et al. (2020) have suggested that actin depolymerization reduced the steady state of the EPSC and PTP, which led them to suggest that the enhanced SV refilling to the RRP was responsible for mfPTP (arrow 1 in Figure 2). The studies at other synapses rather suggest that actin polymerization is required for docking (arrow 2 in Figure 2, Lee et al., 2012) or SV supply from replacement sites to docking sites (Miki et al., 2016). There is no direct evidence that actin is involved in transition from loose to tight states defined by Neher and Brose (2018) (arrow 3 in Figure 2).
Fukaya et al. (2021) and Midorikawa and Sakaba (2017) have reported that a short application of forskolin or cAMP analogues (<10 min, the time course similar or slightly longer than PTP) increases Pr with unchanged NRRP. This potentiation is induced mainly by tightening Ca2+ channel-SV release coupling via accumulation of Ca2+ channels near the release sites. The mechanism is different from mfPTP and longer application of forskolin, involving increases in NRRP and the number of docked SVs (Vandael et al., 2020; Orlando et al., 2021). It remains to be seen if Ca2+ channel accumulation happens physiologically.
Fukaya et al. (2023) have introduced photo-activated cation channels to GCs to induce mfLTP by optical tetanic stimulation. After the optical LTP induction, we performed presynaptic patch-clamp recordings and membrane capacitance measurements in the photo-sensitive hMFBs. We found that, in contrast to mfPTP, Pr is increased by mfLTP induction, while NRRP is not changed. Nevertheless, mfLTP is not caused by a change in the coupling between Ca2+ channels and SVs, but by an increase in fusion competence of the vesicles in the RRP. Importantly, stimulated emission deletion microscopy suggested that Munc13-1 and RIM1, which are involved in docking/priming of the SVs (Betz et al., 2001), are increased in the AZs after mfLTP induction (Fukaya et al., 2023), consistent with contributions of these proteins to mfLTP (Castillo et al., 2002; Yang and Calakos, 2011; but see Kaeser et al., 2008). These proteins are putative release site molecules and rather control the number of release sites and NRRP at the synapses with high Pr (Sakamoto et al., 2018). How can changes of the docking/priming molecules account for an increase in Pr, not in NRRP?
NRRP in hMFBs is ∼20–40 SVs/AZ while the RRP likely contains both undocked and docked SVs (Maus et al., 2020), meaning that only limited number of SVs in the RRP can access to and be clamped at the release sites, a process essential for molecular priming. It is presumed that the increased priming molecules improve the accessibility and/or stabilize the SV-release site complex, consequently increasing the number of docked SVs in a tight state. The increased Munc13-1/RIM1 during mfLTP might act on (1) docking of the SVs (arrow i in Figure 2), which in turn supplies tightly-docked SVs (arrow ii in Figure 2), and/or (2) tightening of the docked SVs (arrow ii in Figure 2), which could be accompanied with compensatory enhancement of SV docking (arrow i in Figure 2). A change in the docking state may underlie presynaptic LTP at other hippocampal synapses (Jung et al., 2021). The faster replenishment of RRP is also triggered during the mfLTP phase (Fukaya et al., 2023), but the change does not seem large enough to influence NRRP at rest. Rather, this acceleration could help SV supply to maintain evoked release during repetitive APs.
Recent advances have started to identify molecular mechanisms of presynaptic potentiation at mossy fiber synapses. The studies mentioned above suggest involvements of actin in mfPTP and Munc13-1/RIM1 in mfLTP, but these are not exclusive, and other molecules may contribute to mfPTP and mfLTP. It should be also noted that Figure 2 is a mechanistic proposal at the current stage. More detailed electrophysiological analyses along with molecular or pharmacological perturbations and applications of dynamic release models (Pan and Zucker, 2009; Vyleta and Jonas, 2014; Miki et al., 2016; Taschenberger et al., 2016; Ritzau-Jost et al., 2018; Miyano et al., 2019; Kobbersmed et al., 2020) will help to understand the cellular/molecular mechanisms of plasticity quantitively. In consequence of mfPTP and mfLTP, the synaptic filtering properties can be modified dynamically (Monday et al., 2018), influencing the circuit computation. Identification of molecular mechanisms for the potentiation will help to elucidate a plastic nature of the circuit function.
Potentiation mechanisms via cAMP/PKA cascade seem different between tetanic stimulation and chemical induction, and are also different depending on the intensity of the inductions, as described above (mfPTP vs. mfLTP). Variations in spatiotemporal patterns of cAMP concentration and resultant enzymatic activities likely contribute, in line with the fact that genetic ablation of a PKA-anchoring protein, AKAP7, suppresses chemical potentiation, but not mfPTP and mfLTP (Jones et al., 2016). Note that another cAMP-dependent protein, Epac2, can also contribute to mfPTP and mfLTP (Fernandes et al., 2015).
During mfLTP, Munc13-1 and RIM1 are increased in the AZs, while other presynaptic molecules such as Rab3a (Castillo et al., 1997), Synaptotagmin 12 (Kaeser-Woo et al., 2013), and Tomosyn (Ben-Simon et al., 2015) can also contribute to mfLTP. However, how these proteins cooperate for the potentiation entailing AZ reorganization has not yet been resolved. It has been reported that cytoskeletal activities and protein synthesis are involved in mfLTP (Barnes et al., 2010; Monday et al., 2022). A recent study revealed that local actin synthesis at hMFBs increases the terminal volume, underlying mfLTP over an hour after the induction (Monday et al., 2022). It is interesting to see if the AZ reorganization is accompanied with this enlargement. AZ reorganization has been also observed during homeostatic presynaptic potentiation at hippocampal synapses (Glebov et al., 2017; Müller et al., 2022) and at Drosophila neuromuscular junctions (Böhme et al., 2019; Mrestani et al., 2021; Ghelani et al., 2023). This Drosophila homeostatic potentiation recruits presynaptic molecules involved in olfactory associative memory formation in flies (Turrel et al., 2022). This may warrant investigations of how AZ reorganization operates presynaptic plasticity, to elucidate molecular mechanisms underlying learning and memory in the animals.
All authors wrote the manuscript and prepared the figures.
This work is supported by JSPS KAKENHI (grant numbers: JP21H02598, JP20KK0171 to TS), JSPS Core-to-Core Program A. Advanced Research Networks (grant number: JPJSCCA20220007 to TS) and Takeda Science Foundation (to TS).
RF acknowledges support from JSPS Overseas Research Fellowship. HH acknowledges support from JST, SPRING (JPMJSP2129).
The authors declare that the research was conducted in the absence of any commercial or financial relationships that could be construed as a potential conflict of interest.
All claims expressed in this article are solely those of the authors and do not necessarily represent those of their affiliated organizations, or those of the publisher, the editors and the reviewers. Any product that may be evaluated in this article, or claim that may be made by its manufacturer, is not guaranteed or endorsed by the publisher.
Acsády, L., Kamondi, A., Sík, A., Freund, T., and Buzsáki, G. (1998). GABAergic cells are the major postsynaptic targets of mossy fibers in the rat hippocampus. J. Neurosci. 18, 3386–3403. doi: 10.1523/JNEUROSCI.18-09-03386.1998
Alle, H., Jonas, P., and Geiger, J. R. (2001). PTP and LTP at a hippocampal mossy fiber-interneuron synapse. Proc. Natl. Acad. Sci. U.S.A. 98, 14708–14713. doi: 10.1073/pnas.251610898
Atwood, H. L., and Karunanithi, S. (2002). Diversification of synaptic strength: Presynaptic elements. Nat. Rev. Neurosci. 3, 497–516. doi: 10.1038/nrn876
Barnes, S. J., Opitz, T., Merkens, M., Kelly, T., von der Brelie, C., Krueppel, R., et al. (2010). Stable mossy fiber long-term potentiation requires calcium influx at the granule cell soma, protein synthesis, and microtubule-dependent axonal transport. J. Neurosci. 30, 12996–13004. doi: 10.1523/JNEUROSCI.1847-10.2010
Ben-Simon, Y., Rodenas-Ruano, A., Alviña, K., Lam, A. D., Stuenkel, E. L., Castillo, P. E., et al. (2015). A combined optogenetic-knockdown strategy reveals a major role of tomosyn in mossy fiber synaptic plasticity. Cell Rep. 12, 396–404. doi: 10.1016/j.celrep.2015.06.037
Betz, A., Thakur, P., Junge, H. J., Ashery, U., Rhee, J. S., Scheuss, V., et al. (2001). Functional interaction of the active zone proteins Munc13-1 and RIM1 in synaptic vesicle priming. Neuron 30, 183–196. doi: 10.1016/s0896-6273(01)00272-0
Böhme, M. A., McCarthy, A. W., Grasskamp, A. T., Beuschel, C. B., Goel, P., Jusyte, M., et al. (2019). Rapid active zone remodeling consolidates presynaptic potentiation. Nat. Commun. 10:1085. doi: 10.1038/s41467-019-08977-6
Borges-Merjane, C., Kim, O., and Jonas, P. (2020). Functional electron microscopy, “flash and freeze,” of identified cortical synapses in acute brain slices. Neuron 105, 992–1006.e6. doi: 10.1016/j.neuron.2019.12.022
Breustedt, J., Vogt, K. E., Miller, R. J., Nicoll, R. A., and Schmitz, D. (2003). Alpha1E-containing Ca2+ channels are involved in synaptic plasticity. Proc. Natl. Acad. Sci. U.S.A. 100, 12450–12455. doi: 10.1073/pnas.2035117100
Castillo, P. E. (2012). Presynaptic LTP and LTD of excitatory and inhibitory synapses. Cold Spring Harb. Perspect. Biol. 4:a005728. doi: 10.1101/cshperspect.a005728
Castillo, P. E., Janz, R., Südhof, T. C., Tzounopoulos, T., Malenka, R. C., and Nicoll, R. A. (1997). Rab3A is essential for mossy fibre long-term potentiation in the hippocampus. Nature 388, 590–593. doi: 10.1038/41574
Castillo, P. E., Schoch, S., Schmitz, F., Südhof, T. C., and Malenka, R. C. (2002). RIM1alpha is required for presynaptic long-term potentiation. Nature 415, 327–330. doi: 10.1038/415327a
Castillo, P. E., Weisskopf, M. G., and Nicoll, R. A. (1994). The role of Ca2+ channels in hippocampal mossy fiber synaptic transmission and long-term potentiation. Neuron 12, 261–269. doi: 10.1016/0896-6273(94)90269-0
Chamberland, S., Evstratova, A., and Tóth, K. (2014). Interplay between synchronization of multivesicular release and recruitment of additional release sites support short-term facilitation at hippocampal mossy fiber to CA3 pyramidal cells synapses. J. Neurosci. 34, 11032–11047. doi: 10.1523/JNEUROSCI.0847-14.2014
del Castillo, J., and Katz, B. (1954). Quantal components of the end-plate potential. J. Physiol. 124, 560–573. doi: 10.1113/jphysiol.1954.sp005129
Delvendahl, I., Weyhersmüller, A., Ritzau-Jost, A., and Hallermann, S. (2013). Hippocampal and cerebellar mossy fibre boutons - same name, different function. J. Physiol. 591, 3179–3188. doi: 10.1113/jphysiol.2012.248294
Eggermann, E., Bucurenciu, I., Goswami, S. P., and Jonas, P. (2011). Nanodomain coupling between Ca2 + channels and sensors of exocytosis at fast mammalian synapses. Nat. Rev. Neurosci. 13, 7–21. doi: 10.1038/nrn3125
Fernandes, H. B., Riordan, S., Nomura, T., Remmers, C. L., Kraniotis, S., Marshall, J. J., et al. (2015). Epac2 Mediates cAMP-dependent potentiation of neurotransmission in the hippocampus. J. Neurosci. 35, 6544–6553. doi: 10.1523/JNEUROSCI.0314-14.2015
Fukaya, R., Hirai, H., Sakamoto, H., Hashimotodani, Y., Hirose, K., and Sakaba, T. (2023). Increased vesicle fusion competence underlies long-term potentiation at hippocampal mossy fiber synapses. Sci. Adv. 9:eadd3616. doi: 10.1126/sciadv.add3616
Fukaya, R., Maglione, M., Sigrist, S. J., and Sakaba, T. (2021). Rapid Ca2+ channel accumulation contributes to cAMP-mediated increase in transmission at hippocampal mossy fiber synapses. Proc. Natl. Acad. Sci. U.S.A. 118:e2016754118. doi: 10.1073/pnas.2016754118
Geiger, J. R., and Jonas, P. (2000). Dynamic control of presynaptic Ca2+ inflow by fast-inactivating K+ channels in hippocampal mossy fiber boutons. Neuron 28, 927–939. doi: 10.1016/s0896-6273(00)00164-1
Ghelani, T., Escher, M., Thomas, U., Esch, K., Lützkendorf, J., Depner, H., et al. (2023). Interactive nanocluster compaction of the ELKS scaffold and Cacophony Ca2+ channels drives sustained active zone potentiation. Sci. Adv. 9:eade7804. doi: 10.1126/sciadv.ade7804
Glebov, O. O., Jackson, R. E., Winterflood, C. M., Owen, D. M., Barker, E. A., Doherty, P., et al. (2017). Nanoscale structural plasticity of the active zone matrix modulates presynaptic function. Cell Rep. 18, 2715–2728. doi: 10.1016/j.celrep.2017.02.064
Griffith, W. H. (1990). Voltage-clamp analysis of posttetanic potentiation of the mossy fiber to CA3 synapse in hippocampus. J. Neurophysiol. 63, 491–501. doi: 10.1152/jn.1990.63.3.491
Hallermann, S., Pawlu, C., Jonas, P., and Heckmann, M. (2003). A large pool of releasable vesicles in a cortical glutamatergic synapse. Proc. Natl. Acad. Sci. U.S.A. 100, 8975–8980. doi: 10.1073/pnas.1432836100
Heisenberg, M. (2003). Mushroom body memoir: From maps to models. Nat. Rev. Neurosci. 4, 266–275. doi: 10.1038/nrn1074
Hosoi, N., Sakaba, T., and Neher, E. (2007). Quantitative analysis of calcium-dependent vesicle recruitment and its functional role at the calyx of Held synapse. J. Neurosci. 27, 14286–14298. doi: 10.1523/JNEUROSCI.4122-07.2007
Huang, Y. Y., and Kandel, E. R. (1996). Modulation of both the early and the late phase of mossy fiber LTP by the activation of beta-adrenergic receptors. Neuron 16, 611–617. doi: 10.1016/s0896-6273(00)80080-x
Imig, C., López-Murcia, F. J., Maus, L., García-Plaza, I. H., Mortensen, L. S., Schwark, M., et al. (2020). Ultrastructural imaging of activity-dependent synaptic membrane-trafficking events in cultured brain slices. Neuron 108, 843e–860.e8. doi: 10.1016/j.neuron.2020.09.004
Imig, C., Min, S. W., Krinner, S., Arancillo, M., Rosenmund, C., Südhof, T. C., et al. (2014). The morphological and molecular nature of synaptic vesicle priming at presynaptic active zones. Neuron 84, 416–431. doi: 10.1016/j.neuron.2014.10.009
Jackman, S. L., and Regehr, W. G. (2017). The mechanisms and functions of synaptic facilitation. Neuron 94, 447–464. doi: 10.1016/j.neuron.2017.02.047
Jonas, P., Major, G., and Sakmann, B. (1993). Quantal components of unitary EPSCs at the mossy fibre synapse on CA3 pyramidal cells of rat hippocampus. J. Physiol. 472, 615–663. doi: 10.1113/jphysiol.1993.sp019965
Jones, B. W., Deem, J., Younts, T. J., Weisenhaus, M., Sanford, C. A., Slack, M. C., et al. (2016). Targeted deletion of AKAP7 in dentate granule cells impairs spatial discrimination. eLife 5:e20695. doi: 10.7554/eLife.20695
Jung, J. H., Kirk, L. M., Bourne, J. N., and Harris, K. M. (2021). Shortened tethering filaments stabilize presynaptic vesicles in support of elevated release probability during LTP in rat hippocampus. Proc. Natl. Acad. Sci. U.S.A. 118, e2018653118. doi: 10.1073/pnas.2018653118
Kaeser, P. S., and Regehr, W. G. (2017). The readily releasable pool of synaptic vesicles. Curr. Opin. Neurobiol. 43, 63–70. doi: 10.1016/j.conb.2016.12.012
Kaeser, P. S., Kwon, H. B., Blundell, J., Chevaleyre, V., Morishita, W., Malenka, R. C., et al. (2008). RIM1alpha phosphorylation at serine-413 by protein kinase A is not required for presynaptic long-term plasticity or learning. Proc. Natl. Acad. Sci. U.S.A. 105, 14680–14685. doi: 10.1073/pnas.0806679105
Kaeser-Woo, Y. J., Younts, T. J., Yang, X., Zhou, P., Wu, D., Castillo, P. E., et al. (2013). Synaptotagmin-12 phosphorylation by cAMP-dependent protein kinase is essential for hippocampal mossy fiber LTP. J. Neurosci. 33, 9769–9780. doi: 10.1523/JNEUROSCI.5814-12.2013
Kamiya, H., Umeda, K., Ozawa, S., and Manabe, T. (2002). Presynaptic Ca2+ entry is unchanged during hippocampal mossy fiber long-term potentiation. J. Neurosci. 22, 10524–10528. doi: 10.1523/JNEUROSCI.22-24-10524.2002
Kandel, E. R. (2001). The molecular biology of memory storage: A dialogue between genes and synapses. Science 294, 1030–1038. doi: 10.1126/science.1067020
Kobbersmed, J. R., Grasskamp, A. T., Jusyte, M., Böhme, M. A., Ditlevsen, S., Sørensen, J. B., et al. (2020). Rapid regulation of vesicle priming explains synaptic facilitation despite heterogeneous vesicle:Ca2+ channel distances. eLife 9:e51032. doi: 10.7554/eLife.51032
Korogod, N., Lou, X., and Schneggenburger, R. (2007). Posttetanic potentiation critically depends on an enhanced Ca2+ sensitivity of vesicle fusion mediated by presynaptic PKC. Proc. Natl. Acad. Sci. U.S.A. 104, 15923–15928. doi: 10.1073/pnas.0704603104
Kwon, H. B., and Castillo, P. E. (2008). Role of glutamate autoreceptors at hippocampal mossy fiber synapses. Neuron 60, 1082–1094. doi: 10.1016/j.neuron.2008.10.045
Lauri, S. E., Bortolotto, Z. A., Bleakman, D., Ornstein, P. L., Lodge, D., Isaac, J. T., et al. (2001). A critical role of a facilitatory presynaptic kainate receptor in mossy fiber LTP. Neuron 32, 697–709. doi: 10.1016/s0896-6273(01)00511-6
Lee, J. S., Ho, W. K., and Lee, S. H. (2010). Post-tetanic increase in the fast-releasing synaptic vesicle pool at the expense of the slowly releasing pool. J. Gen. Physiol. 136, 259–272. doi: 10.1085/jgp.201010437
Lee, J. S., Ho, W. K., and Lee, S. H. (2012). Actin-dependent rapid recruitment of reluctant synaptic vesicles into a fast-releasing vesicle pool. Proc. Natl. Acad. Sci. U.S.A. 109, E765–E774. doi: 10.1073/pnas.1114072109
Lituma, P. J., Kwon, H. B., Alviña, K., Luján, R., and Castillo, P. E. (2021). Presynaptic NMDA receptors facilitate short-term plasticity and BDNF release at hippocampal mossy fiber synapses. eLife 10:e66612. doi: 10.7554/eLife.66612
Maus, L., Lee, C., Altas, B., Sertel, S. M., Weyand, K., Rizzoli, S. O., et al. (2020). Ultrastructural correlates of presynaptic functional heterogeneity in hippocampal synapses. Cell Rep. 30, 3632–3643.e8. doi: 10.1016/j.celrep.2020.02.083
Midorikawa, M., and Sakaba, T. (2017). Kinetics of releasable synaptic vesicles and their plastic changes at hippocampal mossy fiber synapses. Neuron 96, 1033–1040.e3. doi: 10.1016/j.neuron.2017.10.016
Miki, T., Malagon, G., Pulido, C., Llano, I., Neher, E., and Marty, A. (2016). Actin- and myosin-dependent vesicle loading of presynaptic docking sites prior to exocytosis. Neuron 91, 808–823. doi: 10.1016/j.neuron.2016.07.033
Miyano, R., Miki, T., and Sakaba, T. (2019). Ca-dependence of synaptic vesicle exocytosis and endocytosis at the hippocampal mossy fibre terminal. J. Physiol. 597, 4373–4386. doi: 10.1113/JP278040
Monday, H. R., Kharod, S. C., Yoon, Y. J., Singer, R. H., and Castillo, P. E. (2022). Presynaptic FMRP and local protein synthesis support structural and functional plasticity of glutamatergic axon terminals. Neuron 110, 2588–2606.e6. doi: 10.1016/j.neuron.2022.05.024
Monday, H. R., Younts, T. J., and Castillo, P. E. (2018). Long-term plasticity of neurotransmitter release: Emerging mechanisms and contributions to brain function and disease. Annu. Rev. Neurosci. 41, 299–322. doi: 10.1146/annurev-neuro-080317-062155
Mrestani, A., Pauli, M., Kollmannsberger, P., Repp, F., Kittel, R. J., Eilers, J., et al. (2021). Active zone compaction correlates with presynaptic homeostatic potentiation. Cell Rep. 37:109770. doi: 10.1016/j.celrep.2021.109770
Müller, J. A., Betzin, J., Santos-Tejedor, J., Mayer, A., Oprişoreanu, A. M., Engholm-Keller, K., et al. (2022). A presynaptic phosphosignaling hub for lasting homeostatic plasticity. Cell Rep. 39:110696. doi: 10.1016/j.celrep.2022.110696
Neher, E., and Brose, N. (2018). Dynamically primed synaptic vesicle states: Key to understand synaptic short-term plasticity. Neuron 100, 1283–1291. doi: 10.1016/j.neuron.2018.11.024
Neher, E., and Sakaba, T. (2008). Multiple roles of calcium ions in the regulation of neurotransmitter release. Neuron 59, 861–872. doi: 10.1016/j.neuron.2008.08.019
Nicoll, R. A., and Schmitz, D. (2005). Synaptic plasticity at hippocampal mossy fibre synapses. Nat. Rev. Neurosci. 6, 863–876. doi: 10.1038/nrn1786
Orlando, M., Dvorzhak, A., Bruentgens, F., Maglione, M., Rost, B. R., Sigrist, S. J., et al. (2021). Recruitment of release sites underlies chemical presynaptic potentiation at hippocampal mossy fiber boutons. PLoS Biol. 19:e3001149. doi: 10.1371/journal.pbio.3001149
Pan, B., and Zucker, R. S. (2009). A general model of synaptic transmission and short-term plasticity. Neuron 62, 539–554. doi: 10.1016/j.neuron.2009.03.025
Pulido, C., and Marty, A. (2017). Quantal fluctuations in central mammalian synapses: Functional role of vesicular docking sites. Physiol. Rev. 97, 1403–1430. doi: 10.1152/physrev.00032.2016
Regehr, W. G., Delaney, K. R., and Tank, D. W. (1994). The role of presynaptic calcium in short-term enhancement at the hippocampal mossy fiber synapse. J. Neurosci. 14, 523–537. doi: 10.1523/JNEUROSCI.14-02-00523.1994
Reid, C. A., Dixon, D. B., Takahashi, M., Bliss, T. V., and Fine, A. (2004). Optical quantal analysis indicates that long-term potentiation at single hippocampal mossy fiber synapses is expressed through increased release probability, recruitment of new release sites, and activation of silent synapses. J. Neurosci. 24, 3618–3626. doi: 10.1523/JNEUROSCI.3567-03.2004
Ritzau-Jost, A., Delvendahl, I., Rings, A., Byczkowicz, N., Harada, H., Shigemoto, R., et al. (2014). Ultrafast action potentials mediate kilohertz signaling at a central synapse. Neuron 84, 152–163. doi: 10.1016/j.neuron.2014.08.036
Ritzau-Jost, A., Jablonski, L., Viotti, J., Lipstein, N., Eilers, J., and Hallermann, S. (2018). Apparent calcium dependence of vesicle recruitment. J. Physiol. 596, 4693–4707. doi: 10.1113/JP275911
Rizzoli, S. O., and Betz, W. J. (2005). Synaptic vesicle pools. Nat. Rev. Neurosci. 6, 57–69. doi: 10.1038/nrn1583
Rollenhagen, A., Sätzler, K., Rodríguez, E. P., Jonas, P., Frotscher, M., and Lübke, J. H. (2007). Structural determinants of transmission at large hippocampal mossy fiber synapses. J. Neurosci. 27, 10434–10444. doi: 10.1523/JNEUROSCI.1946-07.2007
Sakaba, T. (2006). Roles of the fast-releasing and the slowly releasing vesicles in synaptic transmission at the calyx of Held. J. Neurosci. 26, 5863–5871. doi: 10.1523/JNEUROSCI.0182-06.2006
Sakaba, T. (2018). Kinetics of transmitter release at the calyx of Held synapse. Proc. Jpn. Acad. Ser. B Phys. Biol. Sci. 94, 139–152. doi: 10.2183/pjab.94.010
Sakamoto, H., Ariyoshi, T., Kimpara, N., Sugao, K., Taiko, I., Takikawa, K., et al. (2018). Synaptic weight set by Munc13-1 supramolecular assemblies. Nat. Neurosci. 21, 41–49. doi: 10.1038/s41593-017-0041-9
Salin, P. A., Scanziani, M., Malenka, R. C., and Nicoll, R. A. (1996). Distinct short-term plasticity at two excitatory synapses in the hippocampus. Proc. Natl. Acad. Sci. U.S.A. 93, 13304–13309. doi: 10.1073/pnas.93.23.13304
Schmitz, D., Mellor, J., and Nicoll, R. A. (2001). Presynaptic kainate receptor mediation of frequency facilitation at hippocampal mossy fiber synapses. Science 291, 1972–1976. doi: 10.1126/science.1057105
Shahoha, M., Cohen, R., Ben-Simon, Y., and Ashery, U. (2022). cAMP-Dependent synaptic plasticity at the hippocampal mossy fiber terminal. Front. Synaptic Neurosci. 14:861215. doi: 10.3389/fnsyn.2022.861215
Shimizu, H., Fukaya, M., Yamasaki, M., Watanabe, M., Manabe, T., and Kamiya, H. (2008). Use-dependent amplification of presynaptic Ca2+ signaling by axonal ryanodine receptors at the hippocampal mossy fiber synapse. Proc. Natl. Acad. Sci. U.S.A. 105, 11998–12003. doi: 10.1073/pnas.0802175105
Südhof, T. C. (2012). The presynaptic active zone. Neuron 75, 11–25. doi: 10.1016/j.neuron.2012.06.012
Taschenberger, H., Woehler, A., and Neher, E. (2016). Superpriming of synaptic vesicles as a common basis for intersynapse variability and modulation of synaptic strength. Proc. Natl. Acad. Sci. U.S.A. 113, E4548–E4557. doi: 10.1073/pnas.1606383113
Tong, G., Malenka, R. C., and Nicoll, R. A. (1996). Long-term potentiation in cultures of single hippocampal granule cells: A presynaptic form of plasticity. Neuron 16, 1147–1157. doi: 10.1016/s0896-6273(00)80141-5
Turrel, O., Ramesh, N., Escher, M. J. F., Pooryasin, A., and Sigrist, S. J. (2022). Transient active zone remodeling in the Drosophila mushroom body supports memory. Curr. Biol. 32, 4900–4913.e4. doi: 10.1016/j.cub.2022.10.017
Vandael, D., Borges-Merjane, C., Zhang, X., and Jonas, P. (2020). Short-term plasticity at hippocampal mossy fiber synapses is induced by natural activity patterns and associated with vesicle pool engram formation. Neuron 107, 509–521.e7. doi: 10.1016/j.neuron.2020.05.013
Vandael, D., Okamoto, Y., and Jonas, P. (2021). Transsynaptic modulation of presynaptic short-term plasticity in hippocampal mossy fiber synapses. Nat. Commun. 12:2912. doi: 10.1038/s41467-021-23153-5
Vyleta, N. P., and Jonas, P. (2014). Loose coupling between Ca2+ channels and release sensors at a plastic hippocampal synapse. Science 343, 665–670. doi: 10.1126/science.1244811
Vyleta, N. P., Borges-Merjane, C., and Jonas, P. (2016). Plasticity-dependent, full detonation at hippocampal mossy fiber-CA3 pyramidal neuron synapses. eLife 5:e17977. doi: 10.7554/eLife.17977
Wadel, K., Neher, E., and Sakaba, T. (2007). The coupling between synaptic vesicles and Ca2+ channels determines fast neurotransmitter release. Neuron 53, 563–575. doi: 10.1016/j.neuron.2007.01.021
Walter, A. M., Böhme, M. A., and Sigrist, S. J. (2018). Vesicle release site organization at synaptic active zones. Neurosci. Res. 127, 3–13. doi: 10.1016/j.neures.2017.12.006
Wang, H., Pineda, V. V., Chan, G. C., Wong, S. T., Muglia, L. J., and Storm, D. R. (2003). Type 8 adenylyl cyclase is targeted to excitatory synapses and required for mossy fiber long-term potentiation. J. Neurosci. 23, 9710–9718. doi: 10.1523/JNEUROSCI.23-30-09710.2003
Weisskopf, M. G., Castillo, P. E., Zalutsky, R. A., and Nicoll, R. A. (1994). Mediation of hippocampal mossy fiber long-term potentiation by cyclic AMP. Science 265, 1878–1882. doi: 10.1126/science.7916482
Yang, Y., and Calakos, N. (2011). Munc13-1 is required for presynaptic long-term potentiation. J. Neurosci. 31, 12053–12057. doi: 10.1523/JNEUROSCI.2276-11.2011
Zalutsky, R. A., and Nicoll, R. A. (1990). Comparison of two forms of long-term potentiation in single hippocampal neurons. Science 248, 1619–1624. doi: 10.1126/science.2114039
Keywords: hippocampal mossy fiber synapses, cAMP-mediated potentiation, presynaptic plasticity, synaptic vesicle release, readily-releasable pool
Citation: Fukaya R, Miyano R, Hirai H and Sakaba T (2023) Mechanistic insights into cAMP-mediated presynaptic potentiation at hippocampal mossy fiber synapses. Front. Cell. Neurosci. 17:1237589. doi: 10.3389/fncel.2023.1237589
Received: 09 June 2023; Accepted: 30 June 2023;
Published: 13 July 2023.
Edited by:
Haruyuki Kamiya, Hokkaido University, JapanReviewed by:
Suk-Ho Lee, Seoul National University, Republic of KoreaCopyright © 2023 Fukaya, Miyano, Hirai and Sakaba. This is an open-access article distributed under the terms of the Creative Commons Attribution License (CC BY). The use, distribution or reproduction in other forums is permitted, provided the original author(s) and the copyright owner(s) are credited and that the original publication in this journal is cited, in accordance with accepted academic practice. No use, distribution or reproduction is permitted which does not comply with these terms.
*Correspondence: Ryota Fukaya, cmZ1a2F5YUB6ZWRhdC5mdS1iZXJsaW4uZGU=; Takeshi Sakaba, dHNha2FiYUBtYWlsLmRvc2hpc2hhLmFjLmpw
Disclaimer: All claims expressed in this article are solely those of the authors and do not necessarily represent those of their affiliated organizations, or those of the publisher, the editors and the reviewers. Any product that may be evaluated in this article or claim that may be made by its manufacturer is not guaranteed or endorsed by the publisher.
Research integrity at Frontiers
Learn more about the work of our research integrity team to safeguard the quality of each article we publish.