- Amity Institute of Neuropsychology and Neurosciences, Amity University, Noida, Uttar Pradesh, India
Inherited progressive degeneration of photoreceptors such as retinitis pigmentosa (RP) is the most common cause of blindness leading to severe vision impairment affecting ~1 in 5,000 people worldwide. Although the function and morphology of the photoreceptors get disrupted, there is evidence that the inner retinal neurons such as bipolar cells and the retinal ganglion cells are left intact until later stages. Among several innovative therapeutic options aiming to restore vision, optogenetic therapy can bestow light sensitivity to remaining retinal neurons by ectopic expression of light-sensitive proteins. Since the advent of this technique, a diverse class of opsins (microbial and mammalian opsins), chimeric proteins, ligand-gated ion channels, and switchable opsins have been used to study their potential in vision restoration. These proteins differ in their excitation spectra, response kinetics, and signal amplification cascade. Although most of the studies have reported high fidelity of responses in the retina, only a handful of them have achieved functional vision in the visual cortex. This review is a summary of the visuocortical and behavioral responses after optogenetic treatment of the degenerated retina. This clarifies to what extent improved and meaningful vision can be obtained for therapeutic efficacy and continued clinical progress.
Introduction
The retina is a light-sensitive tissue at the back of the eye consisting of several layers of neurons for processing of light information (photoreceptor-bipolar cells–retinal ganglion cells). When light strikes the retina, the photoreceptors convert the light into an electrical impulse through a cascade of biochemical events. The retinal output is relayed through the optic nerve, optic chiasm, optic tract, dorsal lateral geniculate nucleus, and finally to the visual cortex for image processing and interpretation (Erskine and Herrera, 2014). Retinal degeneration (RD) is the major cause of loss of vision characterized by the progressive degeneration of photoreceptor cells and neuronal remodeling. The most common forms of RD are age-related macular degeneration (AMD) and retinitis pigmentosa (RP) (Katherine et al., 2014). According to reports from the World Health Organization, over 2.2 billion people globally are affected with vision problems, of which 1 billion cases of vision impairment are preventable (Trott et al., 2022). In the past few years, the focus has been to develop innovative experimental therapies for the restoration of naturalistic vision. Among several therapeutic options, optogenetic vision restoration has been successful in restoring vision at the cellular level and has successfully entered clinical trials. The advent of optogenetics has benefitted neuroscience researchers in providing a toolbox that allows optical control of neural circuitry using genetically encoded proteins in target cells (Boyden et al., 2005; Smedemark-Margulies and Trapani, 2013; Sakai et al., 2022). The retina undergoes progressive changes upon the death of the photoreceptors such as sprouting, dendritic retraction of the horizontal cells and bipolar cells, remodeling of neural and glial cells, and the inner nuclear layer changing to a thinner and irregular form (Strettoi et al., 2002). The remnant cones after the loss of outer segments make ectopic synapses with rod bipolar cells (Peng et al., 2000) that form clusters of synchronized spontaneous activity (Haq et al., 2014). A similar increase in spontaneous activity has been shown in the primary visual cortex that results in a lower signal-to-noise ratio and reduced capacity to discriminate stimulus (Wang et al., 2016). Despite the pathologic remodeling in the retina with the onset of photoreceptor loss, the bipolar and retinal ganglion cells (RGCs) survive even in the later stages of inherited RD (Saraf and Olmos de Koo, 2019). The optogenetic strategy of vision restoration offers a unique opportunity to make use of surviving retinal cells by vector-based [adeno-associated virus (AAV) delivery of a transgene encoding a light-sensitive protein (McClements et al., 2020; Lindner et al., 2022; Figure 1). Until now, research has shown successful restoration of light sensitivity at the electrophysiological and behavioral level in animal models of retinitis pigmentosa (RP), a heterogeneous group of retinal disorder resulting in progressive loss of photoreceptor cells leading to impaired vision (Gilhooley et al., 2022; Prosseda et al., 2022).
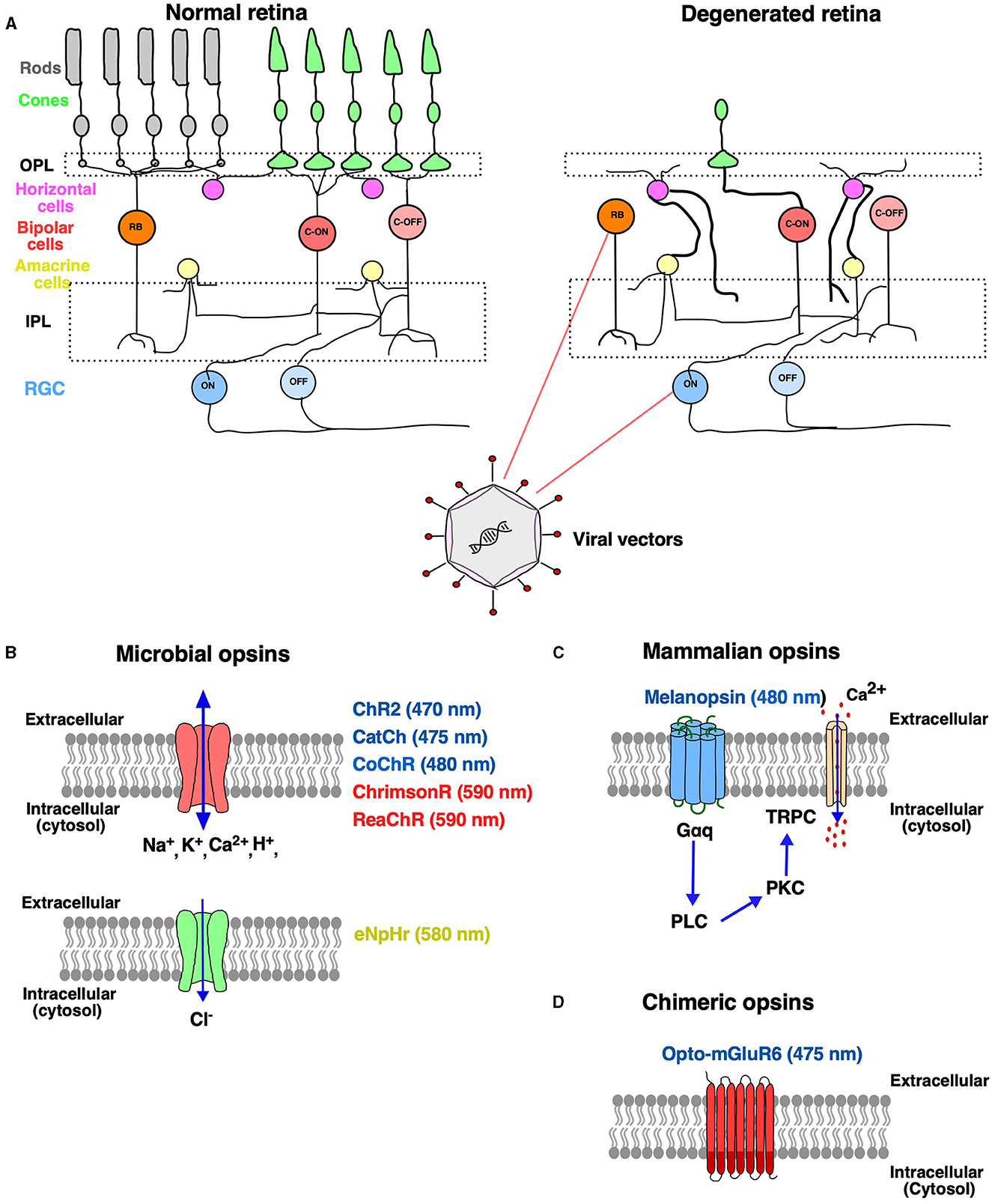
Figure 1. Optogenetic therapy to treat retinal degenerative disease. (A) Schematic representation of a normal healthy retina consisting of different types of neurons: photoreceptors (rods and cones), bipolar cells and retinal ganglion cells (RGCs), horizontal cells and amacrine cells. In inherited retinal degeneration, there is a progressive loss of rods and cones, and the retinal circuitry undergoes aberrant changes, while the deeper retinal layers [bipolar cells (BP) and retinal ganglion cells (RGCs)] remain largely intact. Aberrant synaptic connections are formed and represented in bold with infiltration of glial cells. The BP and RGCs have become potential targets for optogenetic therapy turning them into functional photoreceptors. Viral vectors are used for the expression of the opsin gene to the target cell comprising rod and cone bipolar cells and RGCs through intravitreal or subretinal administration (B–D). The optogenetic toolkit comprises of different classes of opsins such as microbial opsins and mammalian opsins. (B) The microbial opsins are of two types: depolarizing and hyperpolarizing. Depolarizing opsins (denoted by red color): light-gated ion channels upon light activation allow the entry of cations inside the cell-causing depolarization. Hyperpolarizing opsins (denoted by green color): light-gated chloride pump that inhibits neurons by hyperpolarization in response to yellow or green light stimulation. (C) Mammalian opsins, such as melanopsin, a G-protein-coupled receptor (GPCR), which upon light stimulation triggers Gaq activation, followed by phospholipase C (PLC) and protein kinase C (PKC) signaling that further triggers the opening of transient receptor potential ion channel (TRPC), thereby increasing cytosolic Ca2+. (D) GPCRs have been engineered for enhanced light sensitivity, Opto-mGluR6, which is a chimeric fusion protein composed of the light sensing extracellular and transmembrane domains of melanopsin and the intracellular, G protein coupling domains of the ON-bipolar cell-specific, metabotropic glutamate receptor, mGluR6. OPL, outer plexiform layer; IPL, inner plexiform layer; ChR2, channelrhodopsin; CatCh, calcium-translocating channelrhodopsin; eNpHR, enhanced Natromonas halorhodopsin; CoChR, chloromonas oogama.
The first attempt of inducing light sensitivity in vertebrate neurons was demonstrated by the genetic expression of opsin from the fruit fly retina that elicited action potentials upon illumination (Zemelman et al., 2002). Since then, optogenetics has gained momentum by using microbial (type I) or vertebrate opsins (type II) and converting secondary/tertiary retinal neurons into primary neurons. The initial studies involved viral-mediated expression of channelrhodopsin-2 (ChR2), where ectopic expression of this protein in RGCs and bipolar cells led to light-induced membrane permeability restoring visual function in the rd1 mouse model (Bi et al., 2006; Cehajic-Kapetanovic et al., 2015; Simunovic et al., 2019). The same has also been tested in patients with advanced RP initiated by Retrosense Therapeutics and is currently in Phase I/IIa of the clinical trial (NCT02556736). These studies presented some major challenges such as short wavelength and the requirement of high-intensity light which is toxic for the retina limiting its application in clinical studies. Since then, the focus has been to use human opsins that execute their effect through the native G-protein coupled cascade amplifying the signals and increasing sensitivity. The major disadvantage of this method is the slower kinetics of light response not suited for tracking moving objects (Lin et al., 2008). A recent focus has been more on targeting the bipolar cells compared to retinal ganglion cells to evaluate restored vision where differences in the visual output have been observed (Gilhooley et al., 2021). The visual output is evaluated from visually evoked potentials (VEPs) that have an initial negative deflection, a wave, resulting from hyperpolarization of photoreceptors and a positive deflection, b wave, due to depolarization of ON-bipolar cells (Mahroo, 2023). The amplitude (from negative trough to subsequent positive peak) and latency (time from onset of light stimulation to the negative trough) components of the waves are measured to assess the recovery of vision after therapy. It is important to understand how visual responses differ and to what extent visual acuity can be achieved using optogenetic therapy for maximal translational benefit (Figure 2).
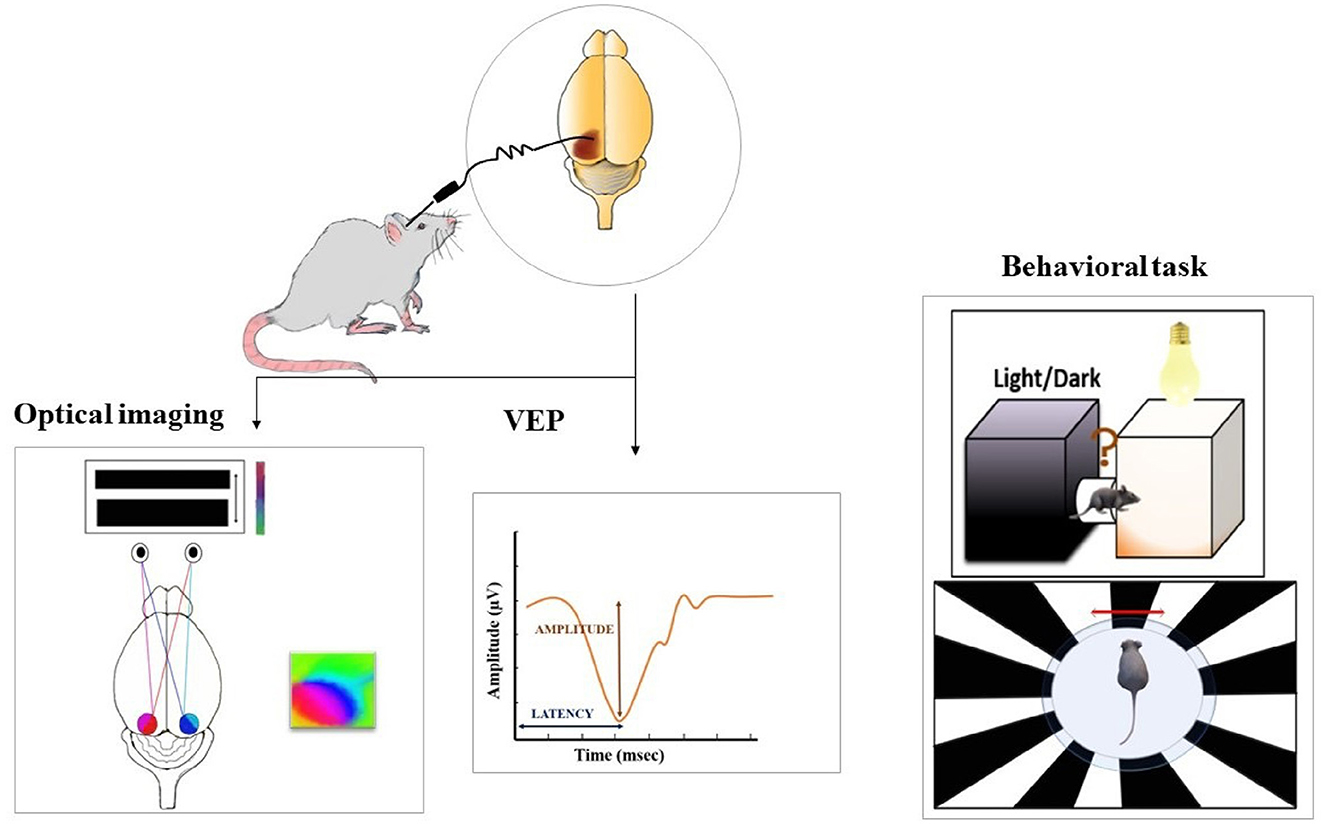
Figure 2. Post-treatment evaluation of the restored vision is assessed using various methods such as optical imaging (measure cortical activity represented by polar maps on the right), electrophysiological recordings (measure the amplitude and latency of visually evoked potential (VEP), and behavioral paradigms. For optical imaging, the intrinsic signal images are acquired as a measure of cortical responses from both hemispheres, while the presentation of continuous visual stimulus to the animal. Visually evoked responses from the visual cortex are evaluated to assess if the transduced retina is able to transmit the information to higher visual centers. The potentials are measured from the entire depth or middle layers of the visual cortex while presentation of a light stimulus to the retina. Light-induced behavioral responses are tested with the help of a light dark box where an increase or decrease in locomotor activity in response to light is measured. Behavioral assessment is also done with an optokinetic drum presenting rotating black and white stripes. This test is performed to measure contrast sensitivity and visual acuity.
Visual cortical responses with expression in inner retinal neurons
There are several strategies to impart light sensitivity to the surviving cells of the retina: one is by selective targeted expression of specific cell types and the other is by ubiquitous expression in surviving retinal cells.
To determine the efficacy of optogenetic treatment as a viable option for vision restoration, preliminary studies were conducted by expressing channelrhodopsin-2 (ChR2- Chop2) in inner retinal neurons in rd1 mice (Bi et al., 2006). The expression profile of the transduced protein was checked by retinal wholemounts and brain sections where RGCs showed predominant expression. The protein expression was stable and helped restore the retina's capacity in encoding light signals and relayed them to the visual cortex. The VEPs had smaller amplitude with shorter latency compared to WT mice, which could be due to lower transfection of the protein in the inner retinal neurons and lack of amplification cascade for signal propagation to higher visual areas. Similar experiments were carried out in another animal model with inherited retinal degenerations, a Royal College of Surgeon (RCS) rat at advanced stages of RD (Tomita et al., 2007). The action of the transgene (AAV-Chop2V) led to low-amplitude VEPs in response to blue light but not to red light stimulation, unlike non-dystrophic rats. Later, the same group tested intravitreal administration with a modified version of the previous transgene, Volvox-channelrhodopsin-1 (mVChR1) having a broader, red-shifted action spectrum suitable for vision restoration (Tomita et al., 2014). The modified vector helped to elicit evoked responses from LED stimulation in longer wavelengths (468–640 nm) which were retained for a year. Ongoing efforts in making channel rhodopsin more light-sensitive led to the development of modified ChR2, ex3mV1CO which is more sensitive than mVChR1 (Watanabe et al., 2021). Blind rats transfected with this gene led to VEP responses at wider wavelengths between 460 and 650 nm at a threshold intensity of 1.0 × 1011 photons/cm2/s, making it a suitable candidate for daylight vision.
Researchers have demonstrated that ectopic expression of human opsins such as melanopsin (hMel) on the retinal cells can safely restore visual function in blind rd1 mice at an advanced stage of degeneration (Liu et al., 2016). Although the visual function of the treated mice was restored, the effects were temporary as it was not visible after 45 days of injection. The loss of human melanopsin expression due to species differences could contribute to the loss of visual rescue. Cone photoreceptors also offer the possibility of intervention due to their lifespan. This advantage has offered the benefit of reactivating the cones with light-activated chloride pumps via the AAV virus (Busskamp et al., 2010). The transduced retinas showed sustained, fast, and large cone-driven light responses elicited by reactivated cones to light increments and decrements. In contrast, evoked responses could not be measured from the cortex. The problem with targeting cones is that after the death of rods, the cones start to lose their outer segments leaving a narrow time window to use them for intervention. The progressive degeneration is unlikely to stop even after optogenetic treatment of cones which may lead to improper computation of sensory information. Therefore, targeting cones may not be the best-suited option for patients with end-stage RD where cones are barely left.
Targeting the inner retinal neurons established the proof of concept that optogenetics has the potential to restore visual processing even after retinal degeneration. The drawback in the above studies was that the recovery of vision was mostly suboptimal and not long term. A list of studies in inner retinal neurons is given in Table 1.
Visual responses with expression in bipolar cells
During RD, the cell loss is mostly confined to the outer retinal photoreceptors while the BCs and RGCs are spared and remain intact even at the end stage (Bi et al., 2006). Targeting the BCs offers the advantage of providing natural signaling via inherent retinal circuitry and demonstrates reliable, stable cortical responses upon treatment. The signal convergence from bipolar cells to retinal ganglion cells enhances light sensitivity due to their potential for photon catch. These upstream cells have the advantage of not being affected by significant remodeling at end-stage RD (Gaub et al., 2015). A summary of the studies is given in Table 1.
Initial attempts to preserve inner retinal signaling were made by Lagali et al., where ChR2 was introduced in the ON-bipolar cells (both rod and cone) of the retina via electroporation in neonatal rd1 mice (Lagali et al., 2008). To determine if the photo responses were transmitted downstream to the cortex, the visually evoked potentials (VEPs) were recorded from the primary visual cortex (V1). The amplitude of the VEP responses from the treated mice was significantly larger as compared to untreated blind mice. Interestingly, the shape of the VEP was different from the WT mice which could be due to the difference in the retinal output as one eye of the mice was electroporated. With the groundbreaking demonstration of light sensitivity achieved at the cortical level by expression of a genetically encoded neuromodulator in different subtypes of ON-bipolar cells, successive studies were carried out to check the efficacy at late-stage RD (Doroudchi et al., 2011). Targeted expression in the ON bipolar cells (rod and cone) with ChR2 transgene led to robust expression that lasted almost 10 months post-injection. The long-lasting expression of the transgene enabled the treated mice for improved behavioral performance in a visually guided behavior. The mice did not perform well at lower light intensities but improved remarkably with increments in light intensity that was close to wild-type (WT) mice (Doroudchi et al., 2011). Furthermore, Mace et al. developed an ON-bipolar cell-specific promoter with a novel AAV vector, 7m8 that had improved transduction efficiency (both cone and rod) compared to AAV2 parental serotype used in previous studies (Macé et al., 2015). The recovery of visual responses was measured by the peak amplitude of the visually evoked potentials (VEPs) and multiunit activity (MUA) from V1. The VEP amplitude was lower but had a similar shape as compared to wild-type mice. Spikes were elicited in the cortex in response to both light increments and decrements across several repetitions, and the latency of ON responses was shorter, whereas the OFF responses were similar in both treated and WT mice. The difference in latency for ON responses can be attributed to direct synaptic transmission by cone bipolar cells to RGCs and further downstream the visual pathway. The other plausible reason could be the amplified signals originating from rod bipolar cells activating several cone bipolar cells. The observed OFF responses may result from the activation of AII amacrine cells by the rod ON bipolar cells that have glycinergic synapses with OFF bipolar cells. The physiological responses of the cortex were consistent with a light avoidance task where the mice showed quick locomotory behavior in response to the light stimulus. This study demonstrated that optogenetic treatment of bipolar cells retains the ability to transmit visual information even after full photoreceptor degeneration. Despite favorable results with microbial opsins at the cortical and behavioral level, they have the disadvantage of requiring higher intensities of light, while native opsins can function with lower intensities of light possibly due to signal amplification cascades, and pose less risks to the immune response. The selective expression of human rod opsin in the ON-bipolar cells (cone and rod) has been shown to effectively restore vision in blind mice (Cehajic-Kapetanovic et al., 2015). Electrophysiological recordings from retinal explants and the visual thalamus exhibited changes in firing (increase and decrease) induced by simple light pulses, luminance change, and naturalistic movies in treated mice. The overall responses were of mixed type with variability in their amplitude, latency, and duration of responses. The animals demonstrated an improved ability to discriminate between light increment and decrement in a visual discrimination task, and detect flicker and gratings of different frequencies and contrast. In addition, there was an increase in visual responses to natural movies that involved changes in elements of natural scene. A further addition to the already existing optogenetic toolkit was a newly engineered melanopsin-glutamate receptor chimera, Opto-mGluR6 (van Wyk et al., 2015). Melanopsin is a blue light-sensitive photopigment responsible for the pupillary light reflex and circadian rhythm. It resides in a small population of retinal ganglion cells called intrinsically photosensitive retinal ganglion cells (ipRGCs) (Hattar et al., 2002). The modified G protein-coupled receptor (GPCR) consists of a transmembrane domain of melanopsin and an intracellular domain of ON-bipolar cell-specific mGluR6. The transduction of this chimeric gene in the retina (both rod and cone) of rd1 mice showed elevated activity in response to visual stimulus. The animal had better visual performance in a visually guided swim task after a few days of training. Learning was not affected even at subthreshold light intensities as the animal performed equally well in this behavioral task, demonstrating high sensitivity of this protein (van Wyk et al., 2015). Melanopsin-based chimeras expressed in both rod and cone ON bipolar cells have been demonstrated to restore cortical light responses (Kralik et al., 2022) but have a higher latency of evoked response unlike MW opsin (Berry et al., 2019) and do not elicit OFF responses different from previous studies (Macé et al., 2015).
Overall, targeting the bipolar cells with microbial opsins showed VEPs with different shapes and higher amplitude compared to WT mice. On the other hand, bipolar cells expressing vertebrate opsins had smaller amplitude VEPs exhibiting ON and sometimes OFF responses. Expression of chimeric constructs in the bipolar cells led to improved light sensitivity attributed to intracellular signal amplification by the G-protein metabotropic signal cascade. The inconsistency of OFF responses could arise from a compromised rod bipolar pathway. The observed variability in the responses could result from different contributing factors such as the method of vector delivery, age of the mice, efficiency in transducing bipolar cells, and non-specific labeling of cells (amacrine and RGCs). Until now, the focus had been to target only the ON-bipolar cells (rod and cone), and the OFF pathway has not been explored so far. Simultaneous targeting of both the cell types would help to attain better visual perception.
Visual responses with expression in retinal ganglion cells
The RGCs are the output neurons of the retina responsible for transmitting visual information to the cortex. The visual capability upon transduction of a transgene in RGCs has been investigated by several research groups. ChR2-YFP expressing in roughly 30% of RGCs in blind mice (ChR2rd1/rd1) could discriminate between bright and dark stimuli with no measurable cortical activation even with high-intensity stimulus similar to rd1/rd1 mice (Thyagarajan et al., 2010). The lack of visible responses from the cortex could arise from the restricted expression only in the ganglion cell class that project to the superior colliculus and not to the downstream visual structures. The transduction of ChR2 in RGCs of aged dystrophic RCS rats (rdy/rdy) was evaluated for visual function (Tomita et al., 2010). The amplitude of the VEPs in rats increased with an increase in light intensities in comparison to dystrophic rats where no responses were observed. The latency was shorter as the transduced signals in the RGCs were transmitted directly to the cortex and not through the inner retinal network. The optomotor responses of the treated rat also showed better responses with increasing light intensities. Since ChR2 requires high light intensities and stimulation with blue light increases the risk of inducing photochemical damage in the retina, better alternatives were devised to use red-shifted channel rhodopsin variants (ReaChR) by increasing the range of light intensity (Lin et al., 2013; Sengupta et al., 2016). ReaChR-treated mice were stimulated with light intensities below the safety limit threshold of the human eye. The cortex was activated by light ON but not to light OFF as all RGCs turned into ON cells and the ON-OFF pathway could not be restored. Another attempt to deal with the problem of potential cell damage was to use a ChR2 mutant that has a higher permeability for Ca2+ ions, named CatCh (Kleinlogel et al., 2011). The protein is 70 times more light-sensitive than ChR2 and can generate fast action potentials with lower light intensity. Selective targeting of this protein in the RGCs of rd1 mice was evaluated for cortical responses to increasing light intensities. The treated blind mice responded to lower light intensities (1015 photons/cm2/s), with short latency compared to the ON responses of wild-type mice. CatCh had a higher transduction efficiency in the peri-foveolar RGCs in macaques exhibiting robust-spiking activity from a population of RGCs with light intensities from 1014 photons/cm2/s intensity and above (Chaffiol et al., 2017). More variants of ChR2, CoChR (Chloromonas oogama) were developed in terms of improved light sensitivity by optimizing the kinetics such as an increase in deactivation time or off rate (Ganjawala et al., 2019). The efficacy of this variant was tested in triple knock-out transgenic blind mice via a multielectrode array (MEA) recordings and optomotor behavioral assays. The threshold light intensity for RGC response was almost 2 log units lower than ChR2 and ReaChR (1.9 × 1012 photons/cm2/s). This was consistent with optomotor responses elicited at ambient light intensities similar to the LCD monitor.
With the proof of concept established with an expression of ChR2 in RGCs, researchers explored the potential of vertebrate opsins such as melanopsin and cone opsins as new biological prosthetics in restoring vision as modification of existing ones. In a previous study, the expression of mouse melanopsin in the ganglion cells rendered them intrinsically light responsive and showed uniform responses driven by melanopsin (Lin et al., 2008). These mice performed well in a two-choice visual discrimination task. The pupillary light reflex was stable and lasted until 11 months of injection showing the capacity of ectopic expression of melanopsin protein in mediating visually driven information. Subsequently, the outcome of the human melanopsin gene (OPN4) was tested for long-term vision restoration at later stages of RD in blind mice (De Silva et al., 2017). Subretinal injection of the construct led to widespread expression in the retina and increased blood flow induced by light stimulation. The injected mice showed improved performance in an object recognition task and the effects lasted 13 months after injection.
A recent strategy to endow light sensitivity was by using cone opsins, middle wave opsin (MW-opsin) which are G-protein-coupled receptors that are very sensitive to light. The goal was to achieve faster kinetics, sensitivity, and better adaptation to ambient light (Berry et al., 2019). The rd1 mice expressing the MW-opsin showed a strong preference for staying in the dark compartment in a light avoidance task. Furthermore, these mice could discriminate between constant and flashing light, distinguish moving lines of different spatial frequencies, and explore novel objects over a wide range of natural light conditions. Electrophysiology experiments from the V1 showed better detection of stimulus with changes in response to changes in brightness. With notable achievements obtained from the above methods, researchers are now trying for preclinical validation in primates. In non-human primates, the spatial resolution of the retina has been estimated to determine the visual acuity treated by optogenetic therapy (Ferrari et al., 2020). The size of the receptive field of ganglion cells was measured in monkeys injected with optogenetic proteins. Most cells had a smaller receptive field indicating the whole neuron of the axon not being light-sensitive after reactivation. The ganglion cells are sensitive to stimulation of only their axon and dendritic field. The finding is promising and suggests optogenetic therapy as a better strategy compared to retinal implants where electrical stimulation can activate distant ganglion cells thereby limiting visual acuity. In rd10 mice whose visual responses decline at an advanced age (P99), upon treatment with a red-shifted channelrhodopsin, ChrimsonR showed robust, long-lasting responses to UV light and red light stimulation (Cheong et al., 2018). This was due to the expression of the protein lasting for an extended time in the ganglion cells. The therapeutic efficacy of ChrimsonR in efficient signal transmission to the higher visual areas of the brain has been demonstrated in primates as well (Gauvain et al., 2021; Chaffiol et al., 2022; McGregor et al., 2022). In primates, the responses compared before and after stimulation using synaptic blockers (block glutamatergic transmission of the retina) allowed the selected stimulation of transduced cells and not the entire retinal pathway. The evoked potentials followed the train of pulses of specific frequencies more frequently after blocker administration. Responses were fast, and the amplitude increased with the increase in light intensity. The protein expression lasted ~20 months after injection in the perifoveal region of the eye. In a recent clinical study, Chrimson R was targeted to foveal retinal ganglion cells into the worse-seeing eye of a patient suffering from retinitis pigmentosa (RP). The therapy was combined with specialized light-stimulating goggles (Sahel et al., 2021). The patient was trained 4.5 months after injection, allowing the stabilization of the expression of the transduced gene. After 7 months of training, the patient started to show signs of visual improvement in a visual detection task that enabled him to perceive, locate, and touch different objects with the help of goggles. The quality of vision restored cannot be compared with natural vision since there are multiple subtypes of RGCs that encode diverse features and send highly processed images to the brain. Further research into developing tools targeting more varied cell types of RGCs would help to restore more natural vision.
The functional outcome of differential targeting of the BCs and RGCs of AAV-mediated optogenetic gene therapy was compared in a triple knock-out mouse model (Lu et al., 2020). Electrophysiological recordings from the retina showed RGCs to have a light intensity (2.0 × 1013 photons/cm2/s) that was approximately a log unit lower than that of BCs (2.4 × 1014 photons/cm2/s). Better performance with RGC transfection was also observed with optomotor responses displaying higher sensitivity with a lower threshold of light intensity. In a recent study, both mammalian (human melanopsin, hOPN4) and microbial opsin (ReaChR) were targeted to the RGCs and bipolar cells to compare their kinetics and sensitivity (Gilhooley et al., 2022). As compared to RGCs, targeting ON bipolar cells with hOPN4 exhibited higher light sensitivity being able to encode a range of light intensities and rapid decay kinetics. ReaChR had a limited dynamic range of encoding, but it exhibited faster decay kinetics selectively only in ON bipolar cells. Until now, there is no common consensus on the preferred cell type for targeting as each of them offers elements of advantages and disadvantages, and further comparative studies are necessary to determine the best choice. A summary of the above studies is given in Table 1.
Conclusion
Optogenetic strategies for vision restoration have gained impetus using a wide variety of transgene and vector combinations. The success of this approach has been through the continuous process of improving the transduction mechanism and cell-specific expression profile of the optogene. The initial studies that started with ChR2 gradually expanded to a safer and broader spectrum in addition to the G protein signaling cascade for better temporal resolution. With the proof of principle demonstrated by superior spatial and temporal visual resolution in animals and primates, these tools are utilized in clinical trials as viable treatment for inherited retinal degeneration. The outcome has been promising as the patients have shown partial functional recovery being able to recognize the pattern and presence of large objects. However, there are several factors that have limited the outcome of gene therapy. One such challenge is the selective targeting of the retinal cell types in healthy primates and degenerated human retina and its potential immune response with microbial opsins. Until now, there are no data about the performance of vertebrate opsins in primates or humans. Further development is required in designing promoters and engineering opsins will enable reliable targeting of different cell types yielding increased light sensitivity and better temporal kinetics. Next, extensive inner retinal rewiring at advanced stages of RD leads to the emergence of rhythmic activity and oscillations of the local field potentials in the retina that further affects the central visual circuitry (Biswas et al., 2014; Goo et al., 2015; Kalloniatis et al., 2016). Currently, there is a lack of information on whether early- or late-stage degeneration yields the best therapeutic result. This necessitates more functional studies to understand the implications of RD on the response properties of the central visual circuitry at different stages of degeneration. Recent studies are therefore trying to determine the proper stage of intervention to achieve the maximal benefits of optogenetics in obtaining complete visual restoration.
Author contributions
AB contributed to conception, design of the study, and wrote the manuscript. AB and KP did literature collection and made the figures and tables. Both authors approved the submitted version.
Acknowledgments
AB was supported by the Ramanujan Fellowship of Science and Engineering Research Board (SERB), Department of Science and Technology (DST), Government of India (Grant: RJF/2019/000040), and the Core Research Grant (CRG) of SERB, DST (CRG/2021/003472).
Conflict of interest
The authors declare that the research was conducted in the absence of any commercial or financial relationships that could be construed as a potential conflict of interest.
Publisher's note
All claims expressed in this article are solely those of the authors and do not necessarily represent those of their affiliated organizations, or those of the publisher, the editors and the reviewers. Any product that may be evaluated in this article, or claim that may be made by its manufacturer, is not guaranteed or endorsed by the publisher.
References
Batabyal, S., Gajjeraman, S., Pradhan, S., Bhattacharya, S., Wright, W., and Mohanty, S. (2021). Sensitization of ON-bipolar cells with ambient light activatable multi-characteristic opsin rescues vision in mice. Gene Ther. 28, 162–176. doi: 10.1038/s41434-020-00200-2
Berry, M. H., Holt, A., Salari, A., Veit, J., Visel, M., Levitz, J., et al. (2019). Restoration of high-sensitivity and adapting vision with a cone opsin. Nat. Commun. 10, 1221. doi: 10.1038/s41467-019-09124-x
Bi, A., Cui, J., Ma, Y. P., Olshevskaya, E., Pu, M., Dizhoor, A. M., et al. (2006). Ectopic expression of a microbial-type rhodopsin restores visual responses in mice with photoreceptor degeneration. Neuron 50, 23–33. doi: 10.1016/j.neuron.2006.02.026
Biswas, S., Haselier, C., Mataruga, A., Thumann, G., Walter, P., and Muller, F. (2014). Pharmacological analysis of intrinsic neuronal oscillations in rd10 retina. PLoS ONE 9, e99075. doi: 10.1371/journal.pone.0099075
Boyden, E. S., Zhang, F., Bamberg, E., Nagel, G., and Deisseroth, K. (2005). Millisecond-timescale, genetically targeted optical control of neural activity. Nat. Neurosci. 8, 1263–1268. doi: 10.1038/nn1525
Busskamp, V., Duebel, J., Balya, D., Fradot, M., Viney, T. J., Siegert, S., et al. (2010). Genetic reactivation of cone photoreceptors restores visual responses in retinitis pigmentosa. Science 329, 413–417. doi: 10.1126/science.1190897
Cehajic-Kapetanovic, J., Eleftheriou, C., Allen, A. E., Milosavljevic, N., Pienaar, A., Bedford, R., et al. (2015). Restoration of vision with ectopic expression of human rod opsin. Curr. Biol. 25, 2111–2122. doi: 10.1016/j.cub.2015.07.029
Chaffiol, A., Caplette, R., Jaillard, C., Brazhnikova, E., Desrosiers, M., Dubus, E., et al. (2017). A new promoter allows optogenetic vision restoration with enhanced sensitivity in macaque retina. Mol. Ther. 25, 2546–2560. doi: 10.1016/j.ymthe.2017.07.011
Chaffiol, A., Provansal, M., Joffrois, C., Blaize, K., Labernede, G., Goulet, R., et al. (2022). In vivo optogenetic stimulation of the primate retina activates the visual cortex after long-term transduction. Mol. Ther. Methods Clin. Dev. 24, 1–10. doi: 10.1016/j.omtm.2021.11.009
Cheong, S. K., Strazzeri, J. M., Williams, D. R., and Merigan, W. H. (2018). All-optical recording and stimulation of retinal neurons in vivo in retinal degeneration mice. PLoS ONE 13, e0194947. doi: 10.1371/journal.pone.0194947
De Silva, S. R., Barnard, A. R., Hughes, S., Tam, S. K. E., Martin, C., Singh, M. S., et al. (2017). Long-term restoration of visual function in end-stage retinal degeneration using subretinal human melanopsin gene therapy. Proc. Natl. Acad. Sci. U. S. A. 114, 11211–11216. doi: 10.1073/pnas.1701589114
Doroudchi, M. M., Greenberg, K. P., Liu, J., Silka, K. A., Boyden, E. S., Lockridge, J. A., et al. (2011). Virally delivered channelrhodopsin-2 safely and effectively restores visual function in multiple mouse models of blindness. Mol. Ther. 19, 1220–1229. doi: 10.1038/mt.2011.69
Erskine, L., and Herrera, E. (2014). Connecting the retina to the brain. ASN Neuro 6, 1759091414562107. doi: 10.1177/1759091414562107
Ferrari, U., Deny, S., Sengupta, A., Caplette, R., Trapani, F., Sahel, J. A., et al. (2020). Towards optogenetic vision restoration with high resolution. PLoS Comput. Biol. 16, e1007857. doi: 10.1371/journal.pcbi.1007857
Ganjawala, T. H., Lu, Q., Fenner, M. D., Abrams, G. W., and Pan, Z. H. (2019). Improved CoChR variants restore visual acuity and contrast sensitivity in a mouse model of blindness under ambient light conditions. Mol. Ther. 27, 1195–1205. doi: 10.1016/j.ymthe.2019.04.002
Gaub, B. M., Berry, M. H., Holt, A. E., Isacoff, E. Y., and Flannery, J. G. (2015). Optogenetic vision restoration using rhodopsin for enhanced sensitivity. Mol. Ther. 23, 1562–1571. doi: 10.1038/mt.2015.121
Gauvain, G., Akolkar, H., Chaffiol, A., Arcizet, F., Khoei, M. A., Desrosiers, M., et al. (2021). Optogenetic therapy: high spatiotemporal resolution and pattern discrimination compatible with vision restoration in non-human primates. Commun. Biol. 4, 125. doi: 10.1038/s42003-020-01594-w
Gilhooley, M. J., Hickey, D. G., Lindner, M., Palumaa, T., Hughes, S., Peirson, S. N., et al. (2021). ON-bipolar cell gene expression during retinal degeneration: implications for optogenetic visual restoration. Exp. Eye Res. 207, 108553. doi: 10.1016/j.exer.2021.108553
Gilhooley, M. J., Lindner, M., Palumaa, T., Hughes, S., Peirson, S. N., and Hankins, M. W. (2022). A systematic comparison of optogenetic approaches to visual restoration. Mol. Ther. Methods Clin. Dev. 25, 111–123. doi: 10.1016/j.omtm.2022.03.003
Goo, Y. S., Park, D. J., Ahn, J. R., and Senok, S. S. (2015). Spontaneous oscillatory rhythms in the degenerating mouse retina modulate retinal ganglion cell responses to electrical stimulation. Front. Cell Neurosci. 9, 512. doi: 10.3389/fncel.2015.00512
Haq, W., Arango-Gonzalez, B., Zrenner, E., Euler, T., and Schubert, T. (2014). Synaptic remodeling generates synchronous oscillations in the degenerated outer mouse retina. Front. Neural Circuit. 8, 108. doi: 10.3389/fncir.2014.00108
Hattar, S., Liao, H. W., Takao, M., Berson, D. M., and Yau, K. W. (2002). Melanopsin-containing retinal ganglion cells: architecture, projections, and intrinsic photosensitivity. Science 295, 1065–1070. doi: 10.1126/science.1069609
Kalloniatis, M., Nivison-Smith, L., Chua, J., Acosta, M. L., and Fletcher, E. L. (2016). Using the rd1 mouse to understand functional and anatomical retinal remodelling and treatment implications in retinitis pigmentosa: a review. Exp. Eye Res. 150, 106–121. doi: 10.1016/j.exer.2015.10.019
Katherine, J., Werta, J. H. L., and Tsang, S. H. (2014). General pathophysiology in retinal degeneration. Dev. Ophthalmol. 53, 33–43. doi: 10.1159/000357294
Kleinlogel, S., Feldbauer, K., Dempski, R. E., Fotis, H., Wood, P. G., Bamann, C., et al. (2011). Ultra light-sensitive and fast neuronal activation with the Ca(2)+-permeable channelrhodopsin CatCh. Nat. Neurosci. 14, 513–518. doi: 10.1038/nn.2776
Kralik, J., van Wyk, M., Stocker, N., and Kleinlogel, S. (2022). Bipolar cell targeted optogenetic gene therapy restores parallel retinal signaling and high-level vision in the degenerated retina. Commun. Biol. 5, 1116. doi: 10.1038/s42003-022-04016-1
Lagali, P. S., Balya, D., Awatramani, G. B., Münch, T. A., Kim, D. S., Busskamp, V., et al. (2008). Light-activated channels targeted to ON bipolar cells restore visual function in retinal degeneration. Nat. Neurosci. 11, 667–675. doi: 10.1038/nn.2117
Lin, B., Koizumi, A., Tanaka, N., Panda, S., and Masland, R. H. (2008). Restoration of visual function in retinal degeneration mice by ectopic expression of melanopsin. Proc. Natl. Acad. Sci. U. S. A. 105, 16009–16014. doi: 10.1073/pnas.0806114105
Lin, J. Y., Knutsen, P. M., Muller, A., Kleinfeld, D., and Tsien, R. Y. (2013). ReaChR: a red-shifted variant of channelrhodopsin enables deep transcranial optogenetic excitation. Nat. Neurosci. 16, 1499–1508. doi: 10.1038/nn.3502
Lindner, M., Gilhooley, M. J., Hughes, S., and Hankins, M. W. (2022). Optogenetics for visual restoration: from proof of principle to translational challenges. Prog. Retin. Eye Res. 91, 101089. doi: 10.1016/j.preteyeres.2022.101089
Liu, M. M., Dai, J. M., Liu, W. Y., Zhao, C. J., Lin, B., and Yin, Z. Q. (2016). Human melanopsin-AAV2/8 transfection to retina transiently restores visual function in rd1 mice. Int. J. Ophthalmol. 9, 655–661. doi: 10.18240/ijo.2016.05.03
Lu, Q., Ganjawala, T. H., Krstevski, A., Abrams, G. W., and Pan, Z. H. (2020). Comparison of AAV-mediated optogenetic vision restoration between retinal ganglion cell expression and ON bipolar cell targeting. Mol. Ther. Methods Clin. Dev. 18, 15–23. doi: 10.1016/j.omtm.2020.05.009
Macé, E., Caplette, R., Marre, O., Sengupta, A., Chaffiol, A., Barbe, P., et al. (2015). Targeting channelrhodopsin-2 to ON-bipolar cells with vitreally administered AAV Restores ON and OFF visual responses in blind mice. Mol. Ther. 23, 7–16. doi: 10.1038/mt.2014.154
Mahroo, O. A. (2023). Visual electrophysiology and “the potential of the potentials”. Eye 23, 2. doi: 10.1038/s41433-023-02491-2
McClements, M. E., Staurenghi, F., MacLaren, R. E., and Cehajic-Kapetanovic, J. (2020). Optogenetic gene therapy for the degenerate retina: recent advances. Front. Neurosci. 14, 570909. doi: 10.3389/fnins.2020.570909
McGregor, J. E., Kunala, K., Xu, Z., Murphy, P. J., Godat, T., Strazzeri, J. M., et al. (2022). Optogenetic therapy restores retinal activity in primate for at least a year following photoreceptor ablation. Mol. Ther. 30, 1315–1328. doi: 10.1016/j.ymthe.2021.09.014
Peng, Y. W., Hao, Y., Petters, R. M., and Wong, F. (2000). Ectopic synaptogenesis in the mammalian retina caused by rod photoreceptor-specific mutations. Nat. Neurosci. 3, 1121–1127. doi: 10.1038/80639
Prosseda, P. P., Tran, M., Kowal, T., Wang, B., and Sun, Y. (2022). Advances in ophthalmic optogenetics: approaches and applications. Biomolecules 12, 20269. doi: 10.3390/biom12020269
Sahel, J. A., Boulanger-Scemama, E., Pagot, C., Arleo, A., Galluppi, F., Martel, J. N., et al. (2021). Partial recovery of visual function in a blind patient after optogenetic therapy. Nat. Med. 27, 1223–1229. doi: 10.1038/s41591-021-01351-4
Sakai, D., Tomita, H., and Maeda, A. (2022). Optogenetic therapy for visual restoration. Int. J. Mol. Sci. 23, 15041. doi: 10.3390/ijms232315041
Saraf, S. S., and Olmos de Koo, L. C. (2019). Vision restoration in outer retinal degenerations: current approaches and future directions. Int. Ophthalmol. Clin. 59, 59–69. doi: 10.1097/IIO.0000000000000257
Sengupta, A., Chaffiol, A., Mace, E., Caplette, R., Desrosiers, M., Lampic, M., et al. (2016). Red-shifted channelrhodopsin stimulation restores light responses in blind mice, macaque retina, and human retina. EMBO Mol. Med. 8, 1248–1264. doi: 10.15252/emmm.201505699
Simunovic, M. P., Shen, W., Lin, J. Y., Protti, D. A., Lisowski, L., and Gillies, M. C. (2019). Optogenetic approaches to vision restoration. Exp. Eye Res. 178, 15–26. doi: 10.1016/j.exer.2018.09.003
Smedemark-Margulies, N., and Trapani, J. G. (2013). Tools, methods, and applications for optophysiology in neuroscience. Front. Mol. Neurosci. 6, 18. doi: 10.3389/fnmol.2013.00018
Strettoi, E., Porciatti, V., Falsini, B., Pignatelli, V., and Rossi, C. (2002). Morphological and functional abnormalities in the inner retina of the rd/rd mouse. J. Neurosci. 22, 5492–5504. doi: 10.1523/JNEUROSCI.22-13-05492.2002
Thyagarajan, S., van Wyk, M., Lehmann, K., Löwel, S., Feng, G., and Wässle, H. (2010). Visual function in mice with photoreceptor degeneration and transgenic expression of channelrhodopsin 2 in ganglion cells. J. Neurosci. 30, 8745–8758. doi: 10.1523/JNEUROSCI.4417-09.2010
Tomita, H., Sugano, E., Isago, H., Hiroi, T., Wang, Z., Ohta, E., et al. (2010). Channelrhodopsin-2 gene transduced into retinal ganglion cells restores functional vision in genetically blind rats. Exp. Eye Res. 90, 429–436. doi: 10.1016/j.exer.2009.12.006
Tomita, H., Sugano, E., Murayama, N., Ozaki, T., Nishiyama, F., Tabata, K., et al. (2014). Restoration of the majority of the visual spectrum by using modified Volvox channelrhodopsin-1. Mol. Ther. 22, 1434–1440. doi: 10.1038/mt.2014.81
Tomita, H., Sugano, E., Yawo, H., Ishizuka, T., Isago, H., Narikawa, S., et al. (2007). Restoration of visual response in aged dystrophic RCS rats using AAV-mediated channelopsin-2 gene transfer. Invest. Ophthalmol. Vis. Sci. 48, 3821–3826. doi: 10.1167/iovs.06-1501
Trott, M., Smith, L., Veronese, N., Pizzol, D., Barnett, Y., Gorely, T., et al. (2022). Eye disease and mortality, cognition, disease, and modifiable risk factors: an umbrella review of meta-analyses of observational studies. Eye 36, 369–378. doi: 10.1038/s41433-021-01684-x
van Wyk, M., Pielecka-Fortuna, J., Lowel, S., and Kleinlogel, S. (2015). Restoring the ON switch in blind retinas: opto-mGluR6, a next-generation, cell-tailored optogenetic tool. PLoS Biol. 13, e1002143. doi: 10.1371/journal.pbio.1002143
Wang, Y., Chen, K., Xu, P., Ng, T. K., and Chan, L. L. (2016). Spontaneous neural activity in the primary visual cortex of retinal degenerated rats. Neurosci. Lett. 623, 42–46. doi: 10.1016/j.neulet.2016.04.062
Watanabe, Y., Sugano, E., Tabata, K., Hatakeyama, A., Sakajiri, T., Fukuda, T., et al. (2021). Development of an optogenetic gene sensitive to daylight and its implications in vision restoration. NPJ Regen. Med. 6, 64. doi: 10.1038/s41536-021-00177-5
Keywords: vision restoration, primary visual cortex, optogenetic, bipolar cells, retinal ganglion cells
Citation: Parnami K and Bhattacharyya A (2023) Current approaches to vision restoration using optogenetic therapy. Front. Cell. Neurosci. 17:1236826. doi: 10.3389/fncel.2023.1236826
Received: 08 June 2023; Accepted: 24 July 2023;
Published: 16 August 2023.
Edited by:
John O'Brien, University of Houston, United StatesReviewed by:
Christianne E. Strang, University of Alabama at Birmingham, United StatesHiroshi Tomita, Iwate University, Japan
Copyright © 2023 Parnami and Bhattacharyya. This is an open-access article distributed under the terms of the Creative Commons Attribution License (CC BY). The use, distribution or reproduction in other forums is permitted, provided the original author(s) and the copyright owner(s) are credited and that the original publication in this journal is cited, in accordance with accepted academic practice. No use, distribution or reproduction is permitted which does not comply with these terms.
*Correspondence: Anwesha Bhattacharyya, YWJoYXR0YWNoYXJ5eWFAYW1pdHkuZWR1; YW53ZXNoYS5iaGF0dGFjaGFyeXlhMkBnbWFpbC5jb20=
†ORCID: Anwesha Bhattacharyya orcid.org/0000-0001-5334-1341