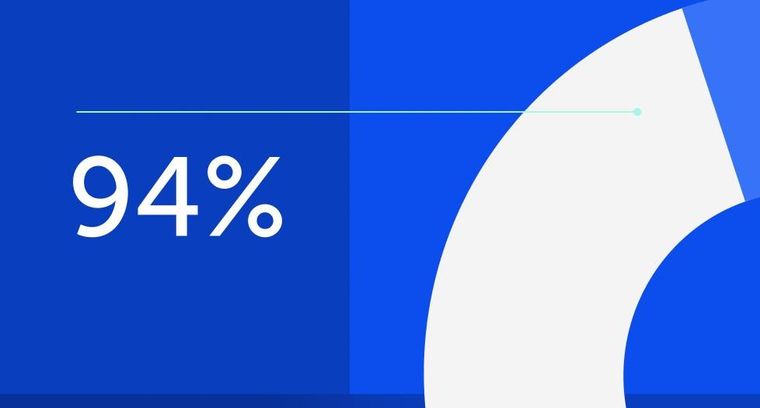
94% of researchers rate our articles as excellent or good
Learn more about the work of our research integrity team to safeguard the quality of each article we publish.
Find out more
REVIEW article
Front. Cell. Neurosci., 20 July 2023
Sec. Cellular Neuropathology
Volume 17 - 2023 | https://doi.org/10.3389/fncel.2023.1228282
This article is part of the Research Topic15 Years of Frontiers in Cellular Neuroscience: Myelination and Remyelination ProcessesView all 5 articles
Peripheral nerve injury (PNI) is associated with delayed repair of the injured nerves in elderly patients, resulting in loss of nerve function, chronic pain, muscle atrophy, and permanent disability. Therefore, the mechanism underlying the delayed repair of peripheral nerves in aging patients should be investigated. Schwann cells (SCs) play a crucial role in repairing PNI and regulating various nerve-repair genes after injury. SCs also promote peripheral nerve repair through various modalities, including mediating nerve demyelination, secreting neurotrophic factors, establishing Büngner bands, clearing axon and myelin debris, and promoting axon remyelination. However, aged SCs undergo structural and functional changes, leading to demyelination and dedifferentiation disorders, decreased secretion of neurotrophic factors, impaired clearance of axonal and myelin debris, and reduced capacity for axon remyelination. As a result, aged SCs may result in delayed repair of nerves after injury. This review article aimed to examine the mechanism underlying the diminished neural repair ability of aging SCs.
Peripheral nerve injury (PNI) involves the impairment of the peripheral nerve trunk and its ramifications resulting from various factors, such as trauma, tumor, metabolic disease, or iatrogenic intervention, especially external trauma (De la Rosa et al., 2018). Axon and myelin sheath rupture and disintegrate at the site of injury after PNI, leading to the degeneration of distal segment of the nerve, which is known as Wallerian degeneration (Li et al., 2021). The peripheral nervous system (PNS) has a regeneration capability in mammals. Axons regenerate at 1–3 mm daily after PNI to reach the motor endplates and innervate corresponding muscles (Slavin et al., 2021). However, incomplete recovery of axons occurs in about one-third of PNI patients, leading to inadequate restoration of function (loss of sensory and motor functions), chronic pain, muscle atrophy, and even permanent disability (Wang et al., 2019).
Aging is one of the major causes of incomplete recovery of the peripheral nerve (Büttner et al., 2018). The impairment of peripheral nerve repair in elderly patients poses a significant burden on families and society due to a gradual increase in the aging population in industrialized countries and thus requires an urgent solution. Research has demonstrated that age-related changes such as persistent inflammation, delayed macrophage response to injury, Schwann cells (SCs) dysfunction, and changes in the microenvironment reduce the regenerative capability of the PNS in murine models. In aged animals, there are fewer axonal protrusion buds and an altered chromatolytic response in neurons. The distribution of axon microtubules and microfilaments and nerve regeneration at the terminals are also altered (Tanaka et al., 1992). The number of macrophages in sciatic nerves of old mice is significantly increased without injury, indicating a chronic inflammatory microenvironment. In aged mice, macrophage numbers and cytokine markedly decreased on day 3 after injury but were considerably higher at 8 weeks than in young mice, which reflects a delayed acute immune response with a persistent chronic inflammatory response. Because of the delayed macrophage response, the clearance of myelin and chemotaxis of other fundamental molecules for successful Wallerian degeneration is reduced in old mice. The persistence of proinflammatory macrophages and higher cytokine expression also becomes one of the inhibitory elements of nerve regeneration in aged mice. Additionally, SCs dysfunction is an important factor in delayed repair of PNI (Büttner et al., 2018; Maita et al., 2023).
Schwann cells are glial cells found in the PNS involved in repairing injuries to peripheral nerves (Galino et al., 2019). SCs originate from multipotent neural crest precursors, which migrate within nascent peripheral nerves and undergo two distinct conformations: myelinating Schwann cells (mSCs) and non-myelinating Schwann cells (nmSCs) – mSC establishes an exclusive relationship with one axon, while nmSC (Remak Schwann cells) enclose multiple axons of smaller caliber (Monje, 2020; Muppirala et al., 2021). SCs initiate a regenerative response after PNI by dramatically changing the expression of various genes involved in nerve repair. SCs acquire a repair phenotype (Repair Schwann Cells; RSCs) after PNI to promote nerve regeneration and functional recovery (Figure 1; Bolívar et al., 2020). However, SCs undergo various changes during aging that can potentially reduce their repair abilities. For example, SCs aging is associated with decreased cytoplasmic volume occupied by mitochondria and increased residual bodies and myelin debris, which can reduce energy availability and hinder nerve regeneration, leading to delayed functional recovery (Pannese, 2021). Changes in metabolic patterns, reduced stress adaptation ability, accumulation of damaged proteins, lipids and DNA, as well as pathological and traumatic factors can also affect the repair ability of SCs (Sardella-Silva et al., 2021). In an aged environment, SCs exhibit a slow activation of the transcriptional repair system, which results in diminished dedifferentiation, macrophage recruitment, and myelin clearance ability (Maita et al., 2023). Therefore, the mechanisms underlying SCs aging should be evaluated to develop effective strategies for nerve repair after PNI.
Figure 1. The development process of Schwann cells during development and after injury. SCs mature in two distinct conformations: myelinating Schwann cells (mSC) and non-myelinating Schwann cells (nmSC). SCs acquire a repair phenotype (repair Schwann cells; RSCs) after injury as a regenerative response, after which the repair of nerve RSCs returns into the phenotype of mSCs and nmSCs.
Although numerous recent studies have investigated the underlying mechanism of SCs in repairing damage to the PNS. The pathophysiological mechanism underlying delayed nerve repair of aging SCs is unclear. Therefore, further research should elucidate how SCs transform with age and how these changes impact their repair ability. This article aimed to review the relevant literature evaluating the pathophysiological mechanism underlying the decreased neural repair capacity of aging SCs.
Studies cited in this review were searched on the PubMed database. These studies were published between 1999 and 2022. Literature retrieval was performed using the following keywords: peripheral nerve injury, aging Schwann cell, nerve repair, aging nerve, c-Jun, Schwann cell dedifferentiation, neurotrophic factors, myelin clearance, macrophages, and remyelination. The aim of this review is to explore the mechanisms by which aging SCs cause delayed nerve repair. Therefore, we selected “peripheral nerve injury,” “aging Schwann cell,” “nerve repair,” and “aging nerve” as search keywords. Previous literature has shown that c-Jun functions as a single independent initiator of the repair SC phenotype, which is essential for the dedifferentiation and transdifferentiation of SCs and subsequent regeneration (McMorrow et al., 2022). Therefore, exploring the changes in c-Jun in aging SCs is extremely important. In addition, secretion of neurotrophic factors combined with macrophage clearance of myelin and remyelination are important ways for SCs to play a role in repair (Nocera and Jacob, 2020). Therefore, we also selected “neurotrophic factors,” “myelin clearance,” “macrophages,” and “remyelination” as search keywords. The search was completed on June 2023 (Table 1).
Schwann cells undergo various changes, such as changes in the expression of genes related to nerve repair, after PNI to promote the repair process. Nerve repair after PNI involves two successive and partially overlapping stages. In the initial stage, SCs detach from the distal segment of axons and demyelinate, followed by dedifferentiation and trans-differentiation of SCs into RSCs (Jessen and Mirsky, 2016; Brosius Lutz et al., 2022), thus activating a series of repair functions. Welleford et al. (2020) analyzed the whole transcriptome profile of the human peripheral nerve after injury. They found that genes related to the cell cycle, cell proliferation, immune cell function, synaptic structure, and neuron function revealed significant changes, myelination-related gene transcripts were downregulated and genes related to growth factor activity were upregulated. Besides, SCs transdifferentiated or reprogrammed from a mature form into a repair phenotype. Chau et al. (2022) reached a similar conclusion in a study of transection injury to the human sural nerve.
Several pro-myelinating genes, such as Early Growth Response 2 (Erg2 or Krox20) and other myelin-related genes, including Myelin Basic Protein (MBP), Myelin Protein Zero (Mpz or P0), Peripheral Myelin Protein 22 (Pmp22), and Myelin Associated Glycoprotein (Mag) are down-regulated after PNI (Nocera and Jacob, 2020). Furthermore, the upregulation of F-actin within SCs that accumulate at the myelin Schmidt-Lantermann incisures (SLI) occurs after PNI, damaging E-cadherin/catenin complexes, thus leading to the demyelination of injured nerves (Jung et al., 2011; Tricaud and Park, 2017).
Schwann cells undergo dedifferentiation after PNI, leading to a state reminiscent of neonatal SC progenitors, and thus they are converted into RSCs (Xu et al., 2020; Stassart and Woodhoo, 2021). The transcription factor, which rapidly increases 80 to 100-fold after PNI, regulates SCs reprogramming by directly or indirectly modulating the expression of at least 172 genes within SCs post-injury (Arthur-Farraj et al., 2017). These genes are involved in several neural repair processes, including SCs dedifferentiation, myelin clearance, neuronal survival, and axonal regeneration (Norrmén et al., 2018; Della-Flora Nunes et al., 2021). These processes promote nerve repair by triggering the secretion of neurotrophic factors, formation of bands of Büngner, clearance of damaged axons and myelin, and stimulation of axonal remyelination (Glenn and Talbot, 2013; Fornaro et al., 2021).
Repair Schwann cells secrete various neurotrophic factors involved in the repair process after PNI, including nerve growth factor (NGF), brain-derived neurotrophic factor (BDNF), glial cell line-derived neurotrophic factor (GDNF), neurotrophin-3 (NT-3), neurotrophin-4/5 (NT-4/5), ciliary neurotrophic factor (CNTF), and fibroblast growth factor (FGF) (Fontana et al., 2012; Huang et al., 2019).
The accumulation of axon and myelin debris at the site of nerve injury can impede nerve repair. SCs play a critical role in accelerating the clearance of debris. SCs can phagocytose axonal debris and digest myelin after injury, establishing a favorable environment for neural repair and axon regeneration (Vaquié et al., 2019; Wang et al., 2020). Myelin clearance involves intracellular and extracellular components. For intracellular components, RSCs can clear debris through autophagic destruction (myelinophagy) (Reed et al., 2020). RSCs can initiate myelin breakdown, attracting macrophages for debris phagocytosis (extracellular component) (Gomez-Sanchez et al., 2015). RSCs up-regulate several cytokines, such as tumor necrosis factor α (TNFα), interleukin-1α (IL-1α), IL-1β, leukemia inhibitory factor (LIF), monocyte chemotactic protein 1 (MCP-1), and fibroblast growth factor 9 (FGF9), during myelin clearance to activate the innate immune response (Jessen and Mirsky, 2016; Qu et al., 2021).
Repair Schwann cells are shortened by about sevenfold after conversion from the repair phenotype to the myelin phenotype, thereby generating typically short internodes in regenerated nerves (Gomez-Sanchez et al., 2017). RSCs up-regulate myelin proteins, including neuregulin-1 (NGR-1) type III, adhesion G protein-coupled receptor (GPCR), and tyrosine kinase (Fyn) after reconnecting with axons through activation of multiple signaling pathways, including Par-3/Par-6/aPKC, PI3K/Akt/mTOR, Ga6-Tyro3, NRG-1/ErbB2/3 (Birchmeier and Bennett, 2016; Torii et al., 2019). As a result, RSCs redifferentiate into mSCs, facilitating remyelination of large-caliber axons, while Remak SCs stimulate ensheathing of small-caliber fibers to reform Remak bundles (Ulrichsen et al., 2022).
The diminished repair ability of aging SCs is associated with impaired nerve repair after PNI in older animals (Kang and Lichtman, 2013). Recent studies have shown that several factors promote delayed nerve repair in aging SCs, including demyelination and dedifferentiation disorders, decreased secretion of neurotrophic factors, obstruction of axon and myelin clearance, and reduced remyelination ability (Figure 2; Fazal et al., 2017).
Figure 2. Mechanism by which aging SCs delay peripheral nerve repair. Demyelination is a disorderly process associated with impeded PNI repair. Additionally, disorders involving SC dedifferentiation have detrimental effects on the repair process. The secretion of neurotrophic factors is reduced in aging SCs, affecting all phases of nerve repair. Moreover, the reduced secretion affects the clearing of axons and myelin by SCs and macrophages at the distal end of injured nerves. Finally, decreased remyelination ability of aging SCs results in inefficient remyelination of new axons.
Genes related to myelin production in aging SCs, such as Pmp22, Mpz, Mal, and Egr2 are overexpressed after PNI. As a result, these genes have decreased myelinating ability, leading to delayed demyelination of injured nerves (Painter et al., 2014). Demyelination is the first step involved in nerve repair after PNI, and thus delayed demyelination can delay subsequent physiological processes, ultimately impeding the repair of injured nerve.
Additionally, delayed demyelination weakens the ability of aging SCs to transdifferentiate into RSCs, thus limiting nerve repair. Significant downregulation of mitosis-related genes (Kif2c, Pbk, Birc5, and Cdc20) and growth factor genes (Btc, Ngfr, and Bdnf) can also delay demyelination (Painter et al., 2014; Sardella-Silva et al., 2021). The reduced neural repair ability of aging SCs is caused by the failure to maintain or activate high levels of the transcription factor c-Jun, which promotes SC dedifferentiation. c-Jun also acts as a global amplifier of the RSC phenotype (Chen et al., 2017; Wagstaff et al., 2021). Wagstaff et al. (2021) reported that c-Jun protein levels within the distal nerve stump are about 50% lower in aged mice than in young mice on the fourth day after PNI. Furthermore, increased c-Jun levels in SCs reversed age-related defects in nerve regeneration. As a result, the same number of regenerated neurons and CGRP+ nerve fibers were similar between the older and younger animals. Painter et al. (2014) also reported that c-Jun protein levels in the nerves are significantly different between the aged and young animals immediately after injury. c-Jun expression was significantly higher in the nerves of young animals 1 day after injury (about fivefold higher) than in aged animals. In an analysis involving 173 c-Jun-regulated injury genes, 138 genes were differentially expressed between young and aged mice in both uninjured and injured nerves. c-Jun down-regulation inhibits the expression of multiple genes closely related to SC proliferation and differentiation, including Gpr37L1, Igfbp2, and Olig1 (Boerboom et al., 2017; Wagstaff et al., 2021). As a result, the repair ability of SCs is inhibited and neural cell adhesion molecule (NCAM), p75 neurotrophin receptor (p75NTR), and glial fibrillary acidic protein (GFAP) (Jessen and Arthur-Farraj, 2019) are down-regulated, thus delaying the repair of injured nerves.
Decreased secretion of neurotrophic factors after injury also affects the repair capacity of aged SCs. Besides, recent research has elucidated that aging SCs cannot efficiently secrete various neurotrophic factors, such as GDNF, BDNF, NGF, CNTF, NT-3, and β-cellulin (Btc) (Jessen and Mirsky, 2021).
Glial cell line-derived neurotrophic factor can promote the survival of motor neurons, neurite outgrowth, myelination, and remodeling of neuromuscular junctions (Cintron-Colon et al., 2022). GDNF down-regulation in aging SCs delays neural repair. BDNF is rapidly up-regulated in the early phase of nerve injury and mediates early nerve repair. BDNF has two receptors, tropomyosin receptor kinase B (trkB) and p75NTR. The BDNF/trkB signaling pathway promotes the transport of actin filaments and associated proteins toward the growth cone, thereby promoting axon outgrowth. p75NTR mediates remyelination through a BDNF-dependent mechanism. p75NTR down-regulation delays functional recovery and axonal growth (McGregor and English, 2018). Overall, BDNF down-regulation in aging SCs delays neural repair. Additionally, NGF can enhance autophagic activities in SCs via the p75NTR/AMPK/mTOR dependent pathways, thus promoting the clearance of axons and myelin in the early stage of PNI (Li et al., 2020). CNTF mediates a neuroimmune cascade by activating signal transducer and activator of transcription 3 (STAT3) and inducing interleukin 6 (IL-6) (Hu et al., 2020), thus promoting the survival of nerve cells and the regeneration of axons through the ERK1/2/MAPK pathway (Fan et al., 2017). Other neurotrophic factors, such as NT-3, are also involved in nerve repair. Unlike BDNF/p75NTR, which inhibits SC migration and enhances myelin formation, NT-3/TrkC promotes SCs migration while inhibiting myelin formation. NT-3 also mediates the activation of downstream MAKP/ERK and AKT signaling pathways, thus facilitating in vitro SC migration. NT-3 down-regulation can decrease the myelination of regenerating axons. Furthermore, NT-3/TrkC participates in the regeneration of Remak bundles (Ulrichsen et al., 2022), indicating that reduced NT-3 levels in aging SCs can significantly delay nerve regeneration after PNI. SCs-secreted Btc is significantly up-regulated in injured segments, driving SCs proliferation, thus stimulating their migration, regulating their phenotype, influencing neuron behavior, increasing neurite length, and mediating nerve regeneration. In one study (Wang et al., 2021), the scratch area of SCs transfected with siRNA control decreased by nearly twofold compared with the scratch area SCs transfected with siRNA against Btc, consistent with transwell migration assay. Furthermore, neurons co-cultured with SCs transfected with siRNA control had longer neurites than those with siRNA against Btc. These assays suggest that Btc is essential after PNI.
Therefore, down-regulation of the above neurotrophic factors secreted by SCs can affect the stages of nerve repair, thus delaying PNI-related repair.
Senescent SCs have decreased ability to assimilate and digest axons and myelin sheaths at the distal end of injured nerves, limiting Wallerian degeneration in aged nerves. Therefore, inefficient removal of axons and myelin sheaths inhibits axon extension (Scheib and Höke, 2016). Painter et al. (2014) showed that axons in the nerve distal to the injury are degenerated after PNI and cleared. Although myelin sheaths are degenerated in young mice within 3 days post-PNI, aged mice have more axons with intact myelin sheaths (about four times) than young mice, indicating that myelin and axon clearance is delayed in aging mice. Scheib and Höke (2016) also showed that myelin debris increases in distal-aged rats. They also showed that aged grafts in aged rats had more debris than young grafts in young rats, indicating that age affects debris clearance. Moreover, Painter et al. (2014) revealed that phagocytosis of myelin is decreased by 35% in aged compared with young SCs based on Florescent-activated cell sorting, which was not attributable to reduced viability of aged SCs. Scheib and Höke (2016) demonstrated that SCs from young rats engulf more myelin than those from aged rats, indicating that SCs from young rats are more phagocytic than those from aged rats. The SCs aging is associated with gradual decrease in various autophagy functions, thus obstructing axon and myelin clearance (Longo et al., 2015; Shi et al., 2021).
Furthermore, aging SCs secrete fewer cytokines, impeding prompt recruitment of macrophages, further hindering clearance of damaged axons and myelin. The expression of cytokines, such as pro-inflammatory IL-6, anti-inflammatory IL-10, and arginase-1, is decreased in aging nerves, slowing down macrophage recruitment at the injury site and reducing clearance of axons and myelin. The secretion of CCL2, a key chemoattractant for macrophages, s decreased in aging SCs (Scheib and Höke, 2016). Phagocytosis assays have indicated that macrophages from young rats are more phagocytic than those from aged ones. Although there are many macrophages in aged rats, only a few may infiltrate the injury site after PNI. More macrophages enter young nerve grafts in young rats than aged grafts in aged rats (Scheib and Höke, 2016; Lu et al., 2022). Cantuti-Castelvetri et al. (2018) also reported that myelin debris, lipid droplets, and needle-shaped cholesterol crystals accumulate in phagocyte lysosomes in old mice, a typical hallmark of cholesterol overloading found in numerous foam cells. Lesion restitution failure in old mice could be due to the inability to clear excessive myelin-derived cholesterol from phagocytes.
In conclusion, impaired phagocytosis and decreased cytokine secretion in aging SCs affect axon and myelin clearance, resulting in physical blockage of regenerated axons, thus slowing the repair of damaged nerves.
Schwann cells aging causes myelination abnormalities in mice. Furthermore, the number of myelinated nerve fibers is significantly decreased in older mice. Myelinated fiber diameter, axon perimeter, myelin thickness, and myelin perimeter are also significantly decreased in older mice compared with those in younger mice (Sakita et al., 2016, 2018). Tanaka et al. (1992) reported that after crush injury, nerves from aging mice contained significantly fewer regenerating myelinated fibers, with smaller axons and thinner myelin sheaths. The length and thickness of the myelin sheath are controlled by axolemmal “myelinating” signals and their receptors. Aging SCs may have an abnormal number or distribution of receptors, which delays remyelination of regenerating axons (Tanaka et al., 1992). Sakita et al. (2020) also reported that the structure of myelin sheaths and axons in myelinated fibers is altered in aged mice, with many irregularities stemming from degeneration. Furthermore, they showed that the mean fiber diameter, axon diameter, and myelin thickness are significantly lower in the aged group than in the young and middle-aged groups. Moreover, aging mice have tiny vacuoles within the myelin sheath, accompanied by thinning and some dislodged myelin walls. Fiber loss and massive disorganization also occur within the myelin sheath of aging mice, culminating in irregular disintegrated and dysmyelinated fibers (Djuanda et al., 2021). These changes are mainly caused by down-regulation of myelin proteins. Djuanda et al. (2021) also showed that MBP is significantly down-regulated in healthy aging nerves at 6 months, which remained low until the late stages of life. Other myelin proteins, such as MAG, MPZ, and PMP22, are also significantly down-regulated in aged SCs (Shen et al., 2011).
Furthermore, aging SCs lead to inefficient remyelination of newborn axons. Myelin-associated proteins, such as PMP22, MPZ, MAG, and EGR2 are down-regulated in aged SCs during the myelin repair stage after PNI, reducing remyelination ability (Liu et al., 2018). Giorgetti et al. (2019) showed that myelin and axons are highly damaged and disorganized in both young and aging mice, with the ring structure of myelin disappearing and intense myelin accumulation occurring at week 1 after injury. Although the young mice fully recovered by week 6, the level of myelin in older mice remained low, ultimately delaying nerve repair in older mice. Additionally, myelin trophic factors, such as prosaposin (PSAP) and prosaptide are secreted after PNI, thus promoting sulfide synthesis in the myelin sheath, mRNA expression of MPZ, and UDP-galactose expression (ceramide galactosyltransferase; GalT), thereby promoting repair of myelinated nerves (Hiraiwa et al., 1999). Although G protein-coupled receptors GPR37 and GPR37L1 serve as receptors for PSAP and prosaptide (Taniguchi et al., 2021; Massimi et al., 2022), the expression of these receptors is low in aging SCs (Wagstaff et al., 2021), leading to peripheral nerve remyelination disorders and delayed nerve repair. Cantuti-Castelvetri et al. (2018) indicated that inadequate clearance of damaged myelin results in the accumulation of cholesterol crystals, eventually impairing remyelination. Cholesterol crystals can induce inflammation by phagolysosomal membrane rupture, followed by stimulation of the caspase-1-activating NLRP3 (NALP3 or cryopyrin) inflammasome and secretion of IL-1 cytokine, thus activating NLRP3 inflammasome in macrophages. Uncontrolled inflammation impairs inflammation resolution and subsequent repair processes in aging animals, thus delaying remyelination (Cantuti-Castelvetri et al., 2018).
Peripheral nerve injury induces protracted delays in the repair of nerve damage among elderly patients. The morphological, physiological, and biochemical transformations caused by the aging of peripheral nerves affect the efficacy of regeneration. Therefore, the neural repair impairment mechanism in aging individuals should be evaluated due to the increasing human longevity and the number of elderly citizens. SCs are crucial in the developmental and regenerative responses of peripheral nerves. Therefore, understanding SCs is crucial for exploring homeostasis in peripheral nerves and regeneration mechanisms. Furthermore, the mechanism underlying the delayed repair of PNI should be investigated. The role of senescent SCs in the restoration of damaged nerves should also be analyzed.
Several studies have examined the pathophysiological mechanism of aging SCs and their impact on delayed nerve repair. This review focused on the principal mechanisms underlying the diminished neural repair capabilities of aging SCs in the latest research. The disorderly process of demyelination affects the expression of various myelin genes in senescent SCs, thus impeding the repair process of PNI. Additionally, disorderly dedifferentiation of SCs further delays the repair process, primarily due to c-Jun down-regulation. Furthermore, aging SCs reduce the secretion of neurotrophic factors, including GDNF, BDNF, NGF, CNTF, NT-3, and Btc, thus affecting all stages of nerve repair. Moreover, SCs and macrophages cannot efficiently clear axons and myelin at the distal end of injured nerves, thus affecting the physical regeneration of axons and resulting in uncontrolled inflammation that hinders remyelination. Senescent SCs mediate myelination irregularities, which cause inefficient remyelination of nascent axons.
Nonetheless, a comprehensive network of mechanisms by which aging SCs delay neural repair after PNI should be evaluated. Furthermore, the pathophysiology of aging SCs should be evaluated. Numerous studies have been conducted on therapeutic strategies for aging cells. These include conventional senotherapeutics, prodrugs, protein degraders, nanocarriers, and immunotherapies. The most common senotherapeutic strategy is selectively killing aging cells, known as senolytics. Another way is senomorphics, which reduces the detrimental effects of the senescence-associated secretory phenotype (SASP). In addition, senoreverters, galactose-based prodrugs, proteolysis-targeting chimera, nanocarriers, immunotherapy based on the senescent cell surfaceome are all important therapeutic strategies for aging cells (Birch and Gil, 2020; Zhang et al., 2022). Potential therapies against aging SCs have been proposed, such as maintenance of c-Jun levels to reverse age-related defects, transplantation of SCs and use of sphingosine-1-phosphate receptor agonist Fingolimod to enhance the repair phenotype (Han et al., 2019; Fuertes-Alvarez and Izeta, 2021; Wagstaff et al., 2021; Schira-Heinen et al., 2022). However, there are still few therapeutic strategies for aging SCs. Therefore, gaining insight into the biology of aging SCs may provide new research directions and therapeutic strategies for preventing and repairing PNI among older people.
HZ and HL: review design. HZ and ZZ: data collection. HZ, ZZ, and HL: manuscript draft and revision. All authors approved the final version of the manuscript.
The study was supported by the National Natural Science Foundation of China (82271407).
Figures were created with Medpeer.cn.
The authors declare that the research was conducted in the absence of any commercial or financial relationships that could be construed as a potential conflict of interest.
All claims expressed in this article are solely those of the authors and do not necessarily represent those of their affiliated organizations, or those of the publisher, the editors and the reviewers. Any product that may be evaluated in this article, or claim that may be made by its manufacturer, is not guaranteed or endorsed by the publisher.
Arthur-Farraj, P. J., Morgan, C. C., Adamowicz, M., Gomez-Sanchez, J. A., Fazal, S. V., Beucher, A., et al. (2017). Changes in the coding and non-coding transcriptome and DNA methylome that define the schwann cell repair phenotype after nerve injury. Cell Rep. 20, 2719–2734. doi: 10.1016/j.celrep.2017.08.064
Birch, J., and Gil, J. (2020). Senescence and the SASP: many therapeutic avenues. Genes Dev. 34, 1565–1576. doi: 10.1101/gad.343129.120
Birchmeier, C., and Bennett, D. L. (2016). Neuregulin/ErbB signaling in developmental myelin formation and nerve repair. Curr. Top. Dev. Biol. 116, 45–64. doi: 10.1016/bs.ctdb.2015.11.009
Boerboom, A., Dion, V., Chariot, A., and Franzen, R. (2017). Molecular mechanisms involved in schwann cell plasticity. Front. Mol. Neurosci. 10:38. doi: 10.3389/fnmol.2017.00038
Bolívar, S., Navarro, X., and Udina, E. (2020). Schwann cell role in selectivity of nerve regeneration. Cells 9:2131. doi: 10.3390/cells9092131
Brosius Lutz, A., Lucas, T. A., Carson, G. A., Caneda, C., Zhou, L., Barres, B. A., et al. (2022). An RNA-sequencing transcriptome of the rodent Schwann cell response to peripheral nerve injury. J. Neuroinflamm. 19:105. doi: 10.1186/s12974-022-02462-6
Büttner, R., Schulz, A., Reuter, M., Akula, A. K., Mindos, T., Carlstedt, A., et al. (2018). Inflammaging impairs peripheral nerve maintenance and regeneration. Aging Cell 17:e12833. doi: 10.1111/acel.12833
Cantuti-Castelvetri, L., Fitzner, D., Bosch-Queralt, M., Weil, M. T., Su, M., Sen, P., et al. (2018). Defective cholesterol clearance limits remyelination in the aged central nervous system. Science 359, 684–688. doi: 10.1126/science.aan4183
Chau, M. J., Quintero, J. E., Blalock, E., Byrum, S., Mackintosh, S. G., Samaan, C., et al. (2022). Transection injury differentially alters the proteome of the human sural nerve. PLoS One 17:e0260998. doi: 10.1371/journal.pone.0260998
Chen, W. A., Luo, T. D., Barnwell, J. C., Smith, T. L., and Li, Z. (2017). Age-dependent schwann cell phenotype regulation following peripheral nerve injury. J. Hand Surg. Asian Pac. 22, 464–471. doi: 10.1142/s0218810417500514
Cintron-Colon, A. F., Almeida-Alves, G., VanGyseghem, J. M., and Spitsbergen, J. M. (2022). GDNF to the rescue: GDNF delivery effects on motor neurons and nerves, and muscle re-innervation after peripheral nerve injuries. Neural Regen. Res. 17, 748–753. doi: 10.4103/1673-5374.322446
De la Rosa, M. B., Kozik, E. M., and Sakaguchi, D. S. (2018). Adult stem cell-based strategies for peripheral nerve regeneration. Adv. Exp. Med. Biol. 1119, 41–71. doi: 10.1007/5584_2018_254
Della-Flora Nunes, G., Wilson, E. R., Hurley, E., He, B., O’Malley, B. W., Poitelon, Y., et al. (2021). Activation of mTORC1 and c-Jun by Prohibitin1 loss in Schwann cells may link mitochondrial dysfunction to demyelination. Elife 10:e66278. doi: 10.7554/eLife.66278
Djuanda, D., He, B., Liu, X., Xu, S., Zhang, Y., Xu, Y., et al. (2021). Comprehensive analysis of age-related changes in lipid metabolism and myelin sheath formation in sciatic nerves. J. Mol. Neurosci. 71, 2310–2323. doi: 10.1007/s12031-020-01768-5
Fan, L., Xiong, Y., Fu, Z., Xu, D., Wang, L., Chen, Y., et al. (2017). Polyaniline promotes peripheral nerve regeneration by enhancement of the brain-derived neurotrophic factor and ciliary neurotrophic factor expression and activation of the ERK1/2/MAPK signaling pathway. Mol. Med. Rep. 16, 7534–7540. doi: 10.3892/mmr.2017.7534
Fazal, S. V., Gomez-Sanchez, J. A., Wagstaff, L. J., Musner, N., Otto, G., Janz, M., et al. (2017). Graded elevation of c-Jun in schwann cells in vivo: gene dosage determines effects on development, remyelination, tumorigenesis, and hypomyelination. J. Neurosci. 37, 12297–12313. doi: 10.1523/jneurosci.0986-17.2017
Fontana, X., Hristova, M., Da Costa, C., Patodia, S., Thei, L., Makwana, M., et al. (2012). c-Jun in Schwann cells promotes axonal regeneration and motoneuron survival via paracrine signaling. J. Cell Biol. 198, 127–141. doi: 10.1083/jcb.201205025
Fornaro, M., Marcus, D., Rattin, J., and Goral, J. (2021). Dynamic environmental physical cues activate mechanosensitive responses in the repair schwann cell phenotype. Cells 10:425. doi: 10.3390/cells10020425
Fuertes-Alvarez, S., and Izeta, A. (2021). Terminal schwann cell aging: implications for age-associated neuromuscular dysfunction. Aging Dis. 12, 494–514. doi: 10.14336/ad.2020.0708
Galino, J., Cervellini, I., Zhu, N., Stöberl, N., Hütte, M., Fricker, F. R., et al. (2019). RalGTPases contribute to Schwann cell repair after nerve injury via regulation of process formation. J. Cell Biol. 218, 2370–2387. doi: 10.1083/jcb.201811002
Giorgetti, E., Obrecht, M., Ronco, M., Panesar, M., Lambert, C., Accart, N., et al. (2019). Magnetic resonance imaging as a biomarker in rodent peripheral nerve injury models reveals an age-related impairment of nerve regeneration. Sci. Rep. 9:13508. doi: 10.1038/s41598-019-49850-2
Glenn, T. D., and Talbot, W. S. (2013). Signals regulating myelination in peripheral nerves and the Schwann cell response to injury. Curr. Opin. Neurobiol. 23, 1041–1048. doi: 10.1016/j.conb.2013.06.010
Gomez-Sanchez, J. A., Carty, L., Iruarrizaga-Lejarreta, M., Palomo-Irigoyen, M., Varela-Rey, M., Griffith, M., et al. (2015). Schwann cell autophagy, myelinophagy, initiates myelin clearance from injured nerves. J. Cell Biol. 210, 153–168. doi: 10.1083/jcb.201503019
Gomez-Sanchez, J. A., Pilch, K. S., van der Lans, M., Fazal, S. V., Benito, C., Wagstaff, L. J., et al. (2017). After nerve injury, lineage tracing shows that myelin and remak schwann cells elongate extensively and branch to form repair schwann cells, which shorten radically on remyelination. J. Neurosci. 37, 9086–9099. doi: 10.1523/jneurosci.1453-17.2017
Han, G. H., Peng, J., Liu, P., Ding, X., Wei, S., Lu, S., et al. (2019). Therapeutic strategies for peripheral nerve injury: decellularized nerve conduits and Schwann cell transplantation. Neural Regen. Res. 14, 1343–1351. doi: 10.4103/1673-5374.253511
Hiraiwa, M., Campana, W. M., Mizisin, A. P., Mohiuddin, L., and O’Brien, J. S. (1999). Prosaposin: a myelinotrophic protein that promotes expression of myelin constituents and is secreted after nerve injury. Glia 26, 353–360.
Hu, Z., Deng, N., Liu, K., Zhou, N., Sun, Y., and Zeng, W. (2020). CNTF-STAT3-IL-6 axis mediates neuroinflammatory cascade across schwann cell-neuron-microglia. Cell Rep. 31:107657. doi: 10.1016/j.celrep.2020.107657
Huang, L., Xia, B., Shi, X., Gao, J., Yang, Y., Xu, F., et al. (2019). Time-restricted release of multiple neurotrophic factors promotes axonal regeneration and functional recovery after peripheral nerve injury. FASEB J. 33, 8600–8613. doi: 10.1096/fj.201802065RR
Jessen, K. R., and Arthur-Farraj, P. (2019). Repair Schwann cell update: adaptive reprogramming, EMT, and stemness in regenerating nerves. Glia 67, 421–437. doi: 10.1002/glia.23532
Jessen, K. R., and Mirsky, R. (2016). The repair Schwann cell and its function in regenerating nerves. J. Physiol. 594, 3521–3531. doi: 10.1113/jp270874
Jessen, K. R., and Mirsky, R. (2021). The role of c-Jun and autocrine signaling loops in the control of repair schwann cells and regeneration. Front. Cell Neurosci. 15:820216. doi: 10.3389/fncel.2021.820216
Jung, J., Cai, W., Lee, H. K., Pellegatta, M., Shin, Y. K., Jang, S. Y., et al. (2011). Actin polymerization is essential for myelin sheath fragmentation during Wallerian degeneration. J. Neurosci. 31, 2009–2015. doi: 10.1523/jneurosci.4537-10.2011
Kang, H., and Lichtman, J. W. (2013). Motor axon regeneration and muscle reinnervation in young adult and aged animals. J. Neurosci. 33, 19480–19491. doi: 10.1523/jneurosci.4067-13.2013
Li, R., Li, D., Wu, C., Ye, L., Wu, Y., Yuan, Y., et al. (2020). Nerve growth factor activates autophagy in Schwann cells to enhance myelin debris clearance and to expedite nerve regeneration. Theranostics 10, 1649–1677. doi: 10.7150/thno.40919
Li, Y., Ma, Z., Ren, Y., Lu, D., Li, T., Li, W., et al. (2021). Tissue engineering strategies for peripheral nerve regeneration. Front. Neurol. 12:768267. doi: 10.3389/fneur.2021.768267
Liu, J. H., Tang, Q., Liu, X. X., Qi, J., Zeng, R. X., Zhu, Z. W., et al. (2018). Analysis of transcriptome sequencing of sciatic nerves in Sprague-Dawley rats of different ages. Neural Regen. Res. 13, 2182–2190. doi: 10.4103/1673-5374.241469
Longo, V. D., Antebi, A., Bartke, A., Barzilai, N., Brown-Borg, H. M., Caruso, C., et al. (2015). Interventions to slow aging in humans: are we ready? Aging Cell 14, 497–510. doi: 10.1111/acel.12338
Lu, R. J., Wang, E. K., and Benayoun, B. A. (2022). Functional genomics of inflamm-aging and immunosenescence. Brief Funct. Genomics 21, 43–55. doi: 10.1093/bfgp/elab009
Maita, K. C., Garcia, J. P., Avila, F. R., Torres-Guzman, R. A., Ho, O., Chini, C. C. S., et al. (2023). Evaluation of the aging effect on peripheral nerve regeneration: a systematic review. J. Surg. Res. 288, 329–340. doi: 10.1016/j.jss.2023.03.017
Massimi, M., Di Pietro, C., La Sala, G., and Matteoni, R. (2022). Mouse mutants of Gpr37 and Gpr37l1 receptor genes: disease modeling applications. Int. J. Mol .Sci. 23:4288. doi: 10.3390/ijms23084288
McGregor, C. E., and English, A. W. (2018). The role of BDNF in peripheral nerve regeneration: activity-dependent treatments and Val66Met. Front. Cell Neurosci. 12:522. doi: 10.3389/fncel.2018.00522
McMorrow, L. A., Kosalko, A., Robinson, D., Saiani, A., and Reid, A. J. (2022). Advancing our understanding of the chronically denervated schwann cell: a potential therapeutic target? Biomolecules 12:1128. doi: 10.3390/biom12081128
Monje, P. V. (2020). Schwann cell cultures: biology, technology and therapeutics. Cells 9:1848. doi: 10.3390/cells9081848
Muppirala, A. N., Limbach, L. E., Bradford, E. F., and Petersen, S. C. (2021). Schwann cell development: from neural crest to myelin sheath. Wiley Interdiscip Rev. Dev. Biol. 10:e398. doi: 10.1002/wdev.398
Nocera, G., and Jacob, C. (2020). Mechanisms of Schwann cell plasticity involved in peripheral nerve repair after injury. Cell Mol Life Sci. 77, 3977–3989. doi: 10.1007/s00018-020-03516-9
Norrmén, C., Figlia, G., Pfistner, P., Pereira, J. A., Bachofner, S., and Suter, U. (2018). mTORC1 is transiently reactivated in injured nerves to promote c-Jun elevation and schwann cell dedifferentiation. J. Neurosci. 38, 4811–4828. doi: 10.1523/jneurosci.3619-17.2018
Painter, M. W., Brosius Lutz, A., Cheng, Y. C., Latremoliere, A., Duong, K., Miller, C. M., et al. (2014). Diminished Schwann cell repair responses underlie age-associated impaired axonal regeneration. Neuron 83, 331–343. doi: 10.1016/j.neuron.2014.06.016
Pannese, E. (2021). Quantitative, structural and molecular changes in neuroglia of aging mammals: a review. Eur. J. Histochem. 65:3249. doi: 10.4081/ejh.2021.3249
Qu, W. R., Zhu, Z., Liu, J., Song, D. B., Tian, H., Chen, B. P., et al. (2021). Interaction between Schwann cells and other cells during repair of peripheral nerve injury. Neural Regen. Res. 16, 93–98. doi: 10.4103/1673-5374.286956
Reed, C. B., Frick, L. R., Weaver, A., Sidoli, M., Schlant, E., Feltri, M. L., et al. (2020). Deletion of calcineurin in schwann cells does not affect developmental myelination, but reduces autophagy and delays myelin clearance after peripheral nerve injury. J. Neurosci. 40, 6165–6176. doi: 10.1523/jneurosci.0951-20.2020
Sakita, M., Murakami, S., and Fujino, H. (2016). Age-related morphological regression of myelinated fibers and capillary architecture of distal peripheral nerves in rats. BMC Neurosci. 17:39. doi: 10.1186/s12868-016-0277-4
Sakita, M., Murakami, S., Fujino, H., Hayashi, S., Kameyama, K., Saito, T., et al. (2018). Remodeling of myelinated fibers and internal capillaries in distal peripheral nerves following aerobic exercise in aged rats. J. Appl. Physiol. 125, 1051–1061. doi: 10.1152/japplphysiol.00257.2018
Sakita, M., Murakami, S., Nonaka, K., Sakamoto, R., Saito, T., Isobe, W., et al. (2020). Different patterns in age-related morphometric alteration of myelinated fibers and capillaries of the tibial nerve: a longitudinal study in normal rats. J. Anat. 236, 1101–1111. doi: 10.1111/joa.13168
Sardella-Silva, G., Mietto, B. S., and Ribeiro-Resende, V. T. (2021). Four seasons for schwann cell biology, revisiting key periods: development, homeostasis, repair, and aging. Biomolecules 11:1887. doi: 10.3390/biom11121887
Scheib, J. L., and Höke, A. (2016). An attenuated immune response by Schwann cells and macrophages inhibits nerve regeneration in aged rats. Neurobiol. Aging 45, 1–9. doi: 10.1016/j.neurobiolaging.2016.05.004
Schira-Heinen, J., Wang, L., Akgün, S., Blum, S., Ziegler, B., Heinen, A., et al. (2022). Modulation of specific sphingosine-1-phosphate receptors augments a repair mediating schwann cell phenotype. Int. J. Mol. Sci. 23:10311. doi: 10.3390/ijms231810311
Shen, D., Zhang, Q., Gao, X., Gu, X., and Ding, F. (2011). Age-related changes in myelin morphology, electrophysiological property and myelin-associated protein expression of mouse sciatic nerves. Neurosci. Lett. 502, 162–167. doi: 10.1016/j.neulet.2011.07.034
Shi, G., Hao, D., Zhang, L., Qin, J., Tian, G., Ma, B., et al. (2021). Endocytosis-associated patterns in nerve regeneration after peripheral nerve injury. J. Orthop. Translat. 31, 10–19. doi: 10.1016/j.jot.2021.09.004
Slavin, B. R., Sarhane, K. A., von Guionneau, N., Hanwright, P. J., Qiu, C., Mao, H. Q., et al. (2021). Insulin-like growth factor-1: a promising therapeutic target for peripheral nerve injury. Front. Bioeng. Biotechnol. 9:695850. doi: 10.3389/fbioe.2021.695850
Stassart, R. M., and Woodhoo, A. (2021). Axo-glial interaction in the injured PNS. Dev. Neurobiol. 81, 490–506. doi: 10.1002/dneu.22771
Tanaka, K., Zhang, Q. L., and Webster, H. D. (1992). Myelinated fiber regeneration after sciatic nerve crush: morphometric observations in young adult and aging mice and the effects of macrophage suppression and conditioning lesions. Exp. Neurol. 118, 53–61. doi: 10.1016/0014-4886(92)90022-i
Taniguchi, M., Nabeka, H., Yamamiya, K., Khan, M. S. I., Shimokawa, T., Islam, F., et al. (2021). The expression of prosaposin and its receptors, GRP37 and GPR37L1, are increased in the developing dorsal root ganglion. PLoS One 16:e0255958. doi: 10.1371/journal.pone.0255958
Torii, T., Miyamoto, Y., and Yamauchi, J. (2019). Cellular Signal-Regulated Schwann Cell Myelination and Remyelination. Adv. Exp. Med. Biol. 1190, 3–22. doi: 10.1007/978-981-32-9636-7_1
Tricaud, N., and Park, H. T. (2017). Wallerian demyelination: chronicle of a cellular cataclysm. Cell Mol. Life Sci. 74, 4049–4057. doi: 10.1007/s00018-017-2565-2
Ulrichsen, M., Gonçalves, N. P., Mohseni, S., Hjæresen, S., Lisle, T. L., Molgaard, S., et al. (2022). Sortilin Modulates schwann cell signaling and remak bundle regeneration following nerve injury. Front. Cell Neurosci. 16:856734. doi: 10.3389/fncel.2022.856734
Vaquié, A., Sauvain, A., Duman, M., Nocera, G., Egger, B., Meyenhofer, F., et al. (2019). Injured axons instruct schwann cells to build constricting actin spheres to accelerate axonal disintegration. Cell Rep. 27, 3152–3166.e7. doi: 10.1016/j.celrep.2019.05.060
Wagstaff, L. J., Gomez-Sanchez, J. A., Fazal, S. V., Otto, G. W., Kilpatrick, A. M., Michael, K., et al. (2021). Failures of nerve regeneration caused by aging or chronic denervation are rescued by restoring Schwann cell c-Jun. Elife 10:e62232. doi: 10.7554/eLife.62232
Wang, M. L., Rivlin, M., Graham, J. G., and Beredjiklian, P. K. (2019). Peripheral nerve injury, scarring, and recovery. Connect Tissue Res. 60, 3–9. doi: 10.1080/03008207.2018.1489381
Wang, Y., Du, S., Liu, T., Ren, J., Zhang, J., Xu, H., et al. (2020). Schwann cell migration through magnetic actuation mediated by fluorescent-magnetic bifunctional Fe(3)O(4)⋅Rhodamine 6G@Polydopamine Superparticles. ACS Chem. Neurosci. 11, 1359–1370. doi: 10.1021/acschemneuro.0c00116
Wang, Y., Zhang, F., Zhang, Y., Shan, Q., Liu, W., Zhang, F., et al. (2021). Betacellulin regulates peripheral nerve regeneration by affecting Schwann cell migration and axon elongation. Mol. Med. 27:27. doi: 10.1186/s10020-021-00292-5
Welleford, A. S., Quintero, J. E., Seblani, N. E., Blalock, E., Gunewardena, S., Shapiro, S. M., et al. (2020). RNA sequencing of human peripheral nerve in response to injury: distinctive analysis of the nerve repair pathways. Cell Transplant 29:963689720926157. doi: 10.1177/0963689720926157
Xu, Z., Orkwis, J. A., DeVine, B. M., and Harris, G. M. (2020). Extracellular matrix cues modulate Schwann cell morphology, proliferation, and protein expression. J. Tissue Eng. Regen. Med. 14, 229–242. doi: 10.1002/term.2987
Keywords: peripheral nerve injury, aging, repair Schwann cells, c-Jun, dedifferentiation, neurotrophic factors, myelin clearance, remyelination
Citation: Zhang H, Zhang Z and Lin H (2023) Research progress on the reduced neural repair ability of aging Schwann cells. Front. Cell. Neurosci. 17:1228282. doi: 10.3389/fncel.2023.1228282
Received: 24 May 2023; Accepted: 06 July 2023;
Published: 20 July 2023.
Edited by:
Yongjun Wang, Nantong University, ChinaReviewed by:
Chun Yao, Nantong University, ChinaCopyright © 2023 Zhang, Zhang and Lin. This is an open-access article distributed under the terms of the Creative Commons Attribution License (CC BY). The use, distribution or reproduction in other forums is permitted, provided the original author(s) and the copyright owner(s) are credited and that the original publication in this journal is cited, in accordance with accepted academic practice. No use, distribution or reproduction is permitted which does not comply with these terms.
*Correspondence: Haodong Lin, aGFvZG9uZ2xpbkBob3RtYWlsLmNvbQ==
Disclaimer: All claims expressed in this article are solely those of the authors and do not necessarily represent those of their affiliated organizations, or those of the publisher, the editors and the reviewers. Any product that may be evaluated in this article or claim that may be made by its manufacturer is not guaranteed or endorsed by the publisher.
Research integrity at Frontiers
Learn more about the work of our research integrity team to safeguard the quality of each article we publish.