Corrigendum: Neurovascular crosstalk and cerebrovascular alterations: an underestimated therapeutic target in autism spectrum disorders
- 1Queen Mary School, Jiangxi Medical College, Nanchang University, Nanchang, China
- 2Department of Psychosomatic Medicine, The First Affiliated Hospital of Nanchang University, Nanchang, Jiangxi, China
Normal brain development, function, and aging critically depend on unique characteristics of the cerebrovascular system. Growing evidence indicated that cerebrovascular defects can have irreversible effects on the brain, and these defects have been implicated in various neurological disorders, including autism spectrum disorder (ASD). ASD is a neurodevelopmental disorder with heterogeneous clinical manifestations and anatomical changes. While extensive research has focused on the neural abnormalities underlying ASD, the role of brain vasculature in this disorder remains poorly understood. Indeed, the significance of cerebrovascular contributions to ASD has been consistently underestimated. In this work, we discuss the neurovascular crosstalk during embryonic development and highlight recent findings on cerebrovascular alterations in individuals with ASD. We also discuss the potential of vascular-based therapy for ASD. Collectively, these investigations demonstrate that ASD can be considered a neurovascular disease.
1. Introduction
Autism spectrum disorder (ASD), also known as autism, is a heterogeneous neurodevelopmental disorder characterized by deficits in social interaction and communication, accompanied by repetitive behaviors (Schafer et al., 2019). In this review, we will use the term “autism” to refer to ASD in general for brevity. In 2020, the estimated prevalence of ASD among 8-year-old children in America was approximately one in 36 (Maenner et al., 2023). ASD is typically diagnosed in early childhood and is considered a lifelong condition (Lord et al., 2018). Despite the high prevalence and substantial impact of ASD, the development of effective therapies is hindered by our limited understanding of its underlying pathogenesis. Behavioral interventions are the primary approach for managing ASD. Although antipsychotics such as risperidone and aripiprazole are used for ASD-related irritability, there are no approved medications specifically targeting the core symptoms of autism, such as social communication deficits and repetitive behaviors (Neul and Sahin, 2015; Xu et al., 2019). The lifespan cost of supporting a person with ASD in the United States was about $2.4 million, and by 2025, the financial burden of ASD may far exceed the costs of diabetes and attention deficit hyperactivity disorder (Buescher et al., 2014; Leigh and Du, 2015). ASD places a significant burden on families, society, and the economy, suggesting that further research into the pathogenesis of ASD and finding a safe, effective, reliable, and inexpensive treatment strategy is urgent (Buescher et al., 2014). According to many epidemiological reports, ASD is caused by a complex interplay of genetic susceptibility and/or environmental factors, and potentially immunological influences (Theoharides, 2013; Colvert et al., 2015; Geschwind and State, 2015; Modabbernia et al., 2017). Current research on the etiology of ASD has mainly focused on identifying risk genes and neurological abnormalities (Hazlett et al., 2017; Satterstrom et al., 2020). Neuropathological investigations have revealed abnormal brain development during the early prenatal period in individuals with ASD (Rodier, 2002), involving disrupted functional connectivity (Courchesne and Pierce, 2005), cortical hyperexpansion (Hazlett et al., 2017), altered neuronal plasticity (Ardalan et al., 2022), and extensive atypical synaptic function (Johnson et al., 2015). However, the precise pathogenesis of ASD remains elusive.
The brain accounts for at least 20% of the body’s resting metabolic energy consumption, primarily dedicated to supporting neuronal activity (Magistretti and Allaman, 2015). The brain vasculature develops into a unique and efficient vascular system different from the peripheral vascular system, to maintain enormous energy consumption and normal brain function. Recent insights have expanded our understanding of the neurovascular interactions beyond traditional oxygen and nutrient supply. Cerebrovascular elements also emerge as critical scaffolds and paracrine signaling mediators, regulating the development, function, and maintenance of various neural cell populations (Paredes et al., 2018; Segarra et al., 2019). The precise interaction between the nervous and cerebrovascular systems is essential for normal development, functional maintenance, and aging of the central nervous system (CNS) (Peguera et al., 2021). In recent years, there has been growing interest in exploring the role of cerebrovascular abnormalities in neurodevelopmental and neurodegenerative diseases, such as schizophrenia and Alzheimer’s disease (Nation et al., 2019; Ouellette and Lacoste, 2021). The potential contribution of cerebrovascular abnormalities to ASD has only recently been gradually described (Ouellette et al., 2020). Although some studies have reported the presence of cerebrovascular abnormalities in individuals with ASD, its contribution to the pathophysiology of ASD remains incompletely understood. In this work, starting from the process of cerebrovascular formation during embryonic development, we review research findings on cerebrovascular alteration in ASD patients. By exploring the association of specific cerebrovascular characteristic defects with neurological abnormalities and behavioral disturbances in ASD, this review aims to enhance our understanding of ASD’s pathogenesis and provide a theoretical foundation for the development of novel therapeutic interventions.
2. Neurovascular development in the central nervous system
Neurovascular development refers to the parallel emergence and formation of the neural and cerebrovascular during early embryonic stages (Bautch and James, 2009). While the vascular and nervous systems each establish distinct specialized networks, they also share overlapping mechanisms. This integration of signaling pathways and cellular responses is crucial for normal brain development, function, and aging (Ouellette and Lacoste, 2021). In mice, between embryonic day 8.5 (E8.5) and 10 (E10), the dorsal ectoderm region containing neuroectodermal cells undergoes specialization into the neural plate. Subsequently, the neural plate elongates, folds, and closes, forming the neural tube (Nikolopoulou et al., 2017). Incipient neural tubes have no vessels, this mild “physiological hypoxic” environment promotes the proliferation and differentiation of neural stem cells (NSCs). This process is likely mediated through the hypoxia-inducible factor-1 (HIF-1) signal pathway (Zhu et al., 2005). Studies involving conditional knockdown of HIF1-α in mouse midbrain-derived neural precursor cells (mNPCs) have demonstrated an impaired midbrain-specific proliferation of mNPCs and a significant reduction in dopaminergic differentiation in vitro. Notably, this phenotype could be rescued by 50 ng/ml VEGF treatment (Milosevic et al., 2007). Conversely, the increasing oxygen and nutrient demands of immature neurons serve as signals to promote angiogenesis (Ouellette and Lacoste, 2021). Simultaneously to the closure of neural tube, the angioblasts (endothelial cell precursors) in the adjacent presomitic mesoderm are recruited by neural tube to form perineural vascular plexus (PNVP) which constitutes the initial vascular network in CNS (James and Mukouyama, 2011). Vascular endothelial growth factor A (VEGF-A), a downstream signal of the transcription factor HIF-1 primarily secreted by the CNS-resident NPCs, acts as the initial driver to trigger angiogenesis (Ferrara et al., 2003; James et al., 2009; Peguera et al., 2021). Thereafter CNS vascularization accompanies its development, followed by massive neurovascular molecular communications. VEGF-A facilitates tip cell migration and enhances stalk cell proliferation at the tips of vascular sprouts, promoting vascular growth into non-vascular regions (Gerhardt et al., 2003). Around E10.5, vessel sprouts from the PNVP invade the neuroectoderm, extending from the pial surface to the luminal surface, resulting in the formation of the intraneural vascular plexus (INVP) (James and Mukouyama, 2011). In general, numerous angiogenic sprouts originating from the IVNP follow the radial glia cells (RGCs) fibers, branch laterally, and anastomose to form the periventricular vascular plexus (PVP) within the ventricular zone (VZ) (Tata and Ruhrberg, 2018). Subsequently, the periventricular and perineural vessels coalesce, forming a complex network of blood vessels intertwined with neural cells that undergo dynamic remodeling until postnatal stage. The instructive role of the vascular system in CNS fate has been largely overlooked in the past but is now garnering increasing attention.
At the same time as neural tube vascularization begins, RGCs transformed from NSCs, present a bipolar morphology, with a long in basal side vessels in the meningeal surface (Sun and Hevner, 2014). RGCs are a major class of neural progenitor cells as well as serve as scaffolds for pyramidal neuron migration in cortical formation (Sun and Hevner, 2014). A 2018 study identified the significant role of CNS vascular endothelial cells (ECs) in regulating neuronal migration and differentiation (Segarra et al., 2018). Reelin, a large glycoprotein secreted by Cajal-Retzius cells, plays a crucial role in neocortex development. Knockdown of reelin/Dab1 signaling specifically in the vascular system impaired the anchoring of RGCs fibers on pial vessels, induced altered pyramidal neuron location, and affect the differentiation of glial cells to neuronal cells (Segarra et al., 2018). This suggests that the vasculature may direct neuronal migration through the function of Dab1 on ECs. Moreover, reelin interacts with the VEGF/VEGF2 pathway to control EC proliferation and vascular filopodia expansion, exerting a powerful proangiogenic effect (Segarra et al., 2018). As a good example, indicated that Reelin/Dab1 signals perform bivalent functions to coordinate neurovascular communication. In addition, vascular ECs around the stem cell niche can promote NPCs proliferation by secreting Neuropilin 1 (NRP1), as one way of vascular regulation of neurogenesis (Tata et al., 2016). The neuropilin (NRP) family members NRP1 and NRP2 also work as receptors or co-receptors of VEGF and semaphorins to modulates angiogenesis (Simons et al., 2016). Knockdown of endothelial uncoupling protein 2 (UCP2) in ECs inhibited NPCs differentiation into neuronal but promoted differentiation into astrocyte, this suggests that blood vessels near NPCs influence neurogenic-to-gliogenic transition (Wang et al., 2022). Conversely, Wnt signaling is crucial for guiding cerebral angiogenesis during neural development. NPCs expressing Wnt7a/b ligand stimulate sprouting angiogenesis of the INVP and PNVP, promoting invasion of the neural tube and inducing and maintaining blood-brain barrier (BBB) properties (Stenman et al., 2008; Daneman et al., 2009). This process involves the interaction of Gpr124 (an orphan GPCR) expressed by ECs with the Norrin/Frizzled4 signaling pathway (Zhou and Nathans, 2014). Throughout the embryonic and postnatal stages, NPCs undergo a highly active process of cell division, differentiation, and migration to establish the intricate and organized CNS. Overall, balanced neurovascular interactions are essential to support the development of the CNS and cerebrovascular systems.
During vertebrate development, specialized blood vessels are formed to meet the specific requirements of different tissues. The formation of the BBB coincides with the growth of vasculature into the CNS. BBB is a specialized vascular barrier between circulating blood and nervous system, acting as a gatekeeper to maintain homeostasis (Langen et al., 2019). It consists of a specialized monolayer of brain capillary ECs. These cells exhibit unique cytological features that differ from those of peripheral ECs, including specialized tight junctions (TJs) between cells, designated transporters that highly selectively control specific substrates and low rates of transcellular vesicle trafficking (Langen et al., 2019). However, early transplantation experiments demonstrated that the BBB properties are induced by the perivascular cellular (pericytes and astrocytes) and CNS environment, rather than the intrinsic properties of CNS endothelial cells (Stewart and Wiley, 1981; Daneman et al., 2010). During embryonic development, the BBB gradually forms from the vascularization of the CNS. At an early stage of cerebrovascular development (sprouts from PNVP), they already acquire TJs under the regulation of canonical Wnt signaling (Risau, 1991) and express barrier function-related genes such as CLDN-5 (Morita et al., 1999). However, blood vessels do not exhibit barrier properties in the early stages of development due to the continued active transcellular transport of ECs. The inhibition of EC endocytosis gradually occurs as the barrier forms (Langen et al., 2019).
Previous physiological studies on cerebral blood flow (CBF) dynamics have demonstrated intensive intercellular communication between cells of the vasculature and nearby neurons and glial cells (McConnell et al., 2017). The BBB is now understood to function as part of a multicellular unit called the neurovascular unit (NVU) rather than operating independently (Figure 1) (McConnell et al., 2017). Endothelial cells are connected by tight junctions, surrounded by pericytes, and embedded in the basement membrane, the end-foot processes of astrocytes surround blood vessels, forming NVU (Abbott et al., 2006; Tam and Watts, 2010). Compared with peripheral blood vessels, cerebral vessels require dense vascularization to maintain the supply of nutrients and oxygen and precise control of CBF (Ouellette and Lacoste, 2021). In the mammalian brain, cerebral vessels respond to the needs of the nervous system by increasing the rate of CBF and oxygen transport, a mechanism known as neurovascular coupling (Iadecola, 2017). The integrity of the NVU is critical for this normal function (Stobart and Anderson, 2013). Besides, the NVU induces and regulates BBB establish (Abbott et al., 2006), and controls the selective transportation of substances in the CNS (Attwell et al., 2010; Daneman and Prat, 2015; Iadecola, 2017). Therefore, the interaction among the cellular elements of the NVU is crucial for maintaining CNS homeostasis (Segarra et al., 2019).
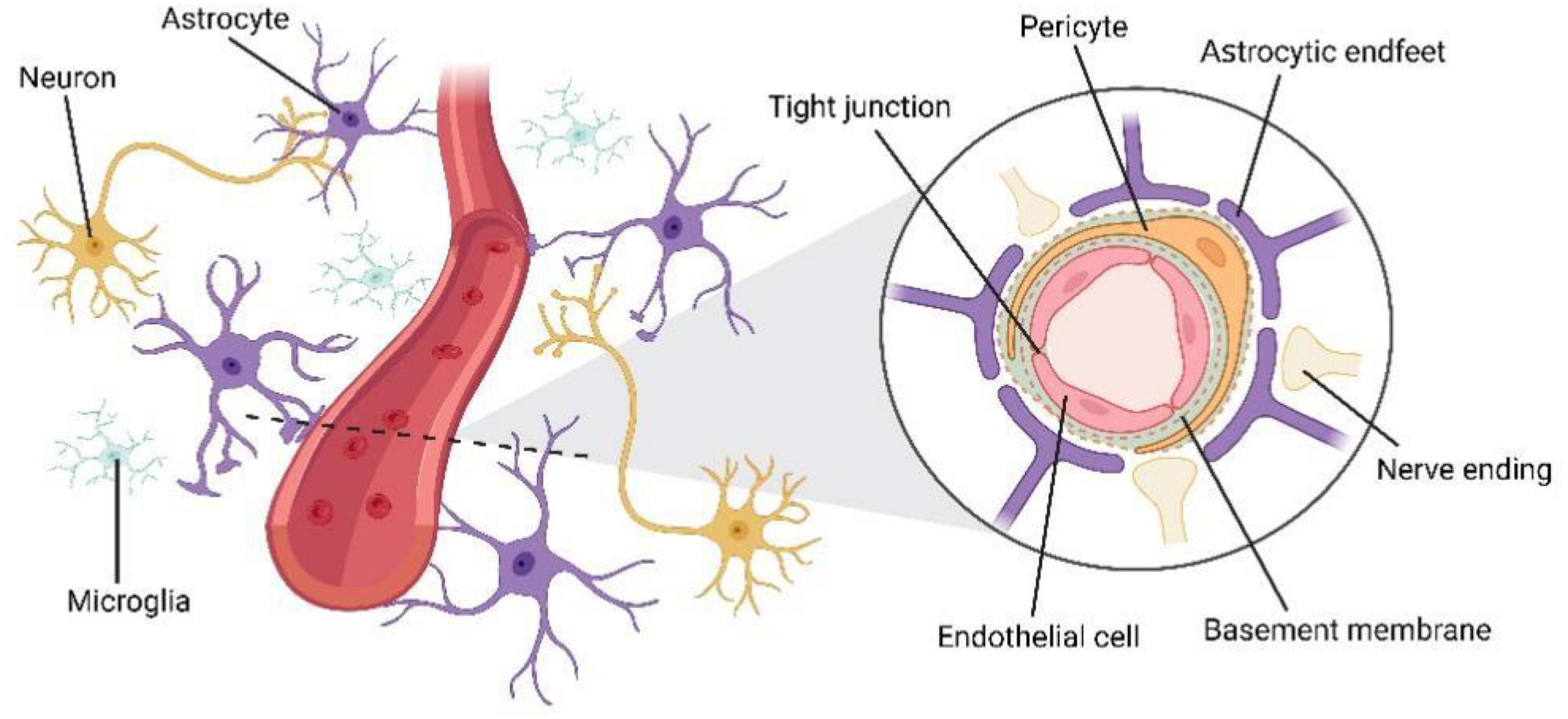
Figure 1. The structure of neurovascular unit (NVU). Endothelial cells are connected by tight junctions, surrounded by pericytes, and embedded in the basement membrane, the end-foot processes of astrocytes surround blood vessels, forming NVU.
The neuronal activity also ensures the development of the cerebrovascular network. In the stress model of rats with social isolation, occurs BBB damage early, manifested as increased BBB permeability accompanied by increased IL-6 expression (Schiavone et al., 2017). Chronic stimulation of neonatal but not adult mice, such as repetitive sounds, whisker deflection, and chemically induced seizures, reduced endothelial proliferation and vascular sprouting, which may be attributed to inhibition of vascular growth by abnormally active interneurons and glial cells through the release of nitric oxide (Whiteus et al., 2014). Exosomes provide another mechanism for mediating neurovascular communication in the CNS. Exosomes are extracellular vesicles enriched in proteins and lipids that are produced by nearly every cell type and play a significant role in neuron-glial cell communication (Frühbeis et al., 2012; Tkach and Théry, 2016). These exosomes carry microRNAs, which can cross the BBB and regulate gene expression in various physiological and pathological processes of the CNS through the RNA-induced silencing complex (Tkach and Théry, 2016). In zebrafish larvae, embryonic neurons express a microRNA called miR-132 in exosomes, which regulates BBB integrity by controlling the expression of endothelial VE-cadherin. When miR-132 is antagonized, zebrafish larvae exhibit severe intracranial hemorrhage and impaired BBB integrity. This suggests exosome-mediated long-range communication between neurons and brain ECs (Xu et al., 2017). Overall, the neural and cerebrovascular systems develop and mature simultaneously. Molecular pathways shared by both systems converge during CNS development to regulate the complex processes of morphogenesis and functional formation in the brain.
3. Cerebrovascular deficits in autism spectrum disorders
Prior research has suggested that the etiology of ASD might involve a complex interaction between genetic and environmental factors (Geschwind and State, 2015; Modabbernia et al., 2017). However, the molecular and cellular mechanisms underlying ASD pathogenesis remain poorly understood. While most studies have focused on neuronal aspects, ASD is now thought to be associated with abnormal vasculature. The healthy development and functioning of the brain rely heavily on a well-functioning vascular system. Given the extensive crosstalk between the vasculature and nervous system during embryonic development, it is reasonable to hypothesize that structural and functional abnormalities in the cerebral vasculature could have detrimental effects on neurodevelopment. Surprisingly, few studies have explored the neurological consequences of vascular abnormalities during the embryonic period in the context of ASD. Furthermore, the brain cannot store amounts of energy and therefore depends on a constant supply of oxygen and nutrients of cerebral vasculature, to buffer its high but variable metabolic demand. The unique characteristics of the cerebral vasculature ensure a stable microenvironment for neural activity and provide adequate oxygen and nutrients. These characteristics distinguish it from the peripheral vasculature including a dense vasculature to maintain adequate perfusion, a functional BBB to maintain brain homeostasis, as well as regulating stable local CBF in response to neuronal demand (Ouellette and Lacoste, 2021). Defects in the brain vascular system can have severe and irreversible impacts on the CNS. Increasing evidence indicates that these critical features of the cerebral vasculature are altered in individuals with ASD (Figure 2). It is not clear whether cerebrovascular abnormalities are the cause of autism or the result of pathophysiologic changes. The association between genetic, environmental factors and cerebrovascular abnormalities in ASD needs to be further explored.
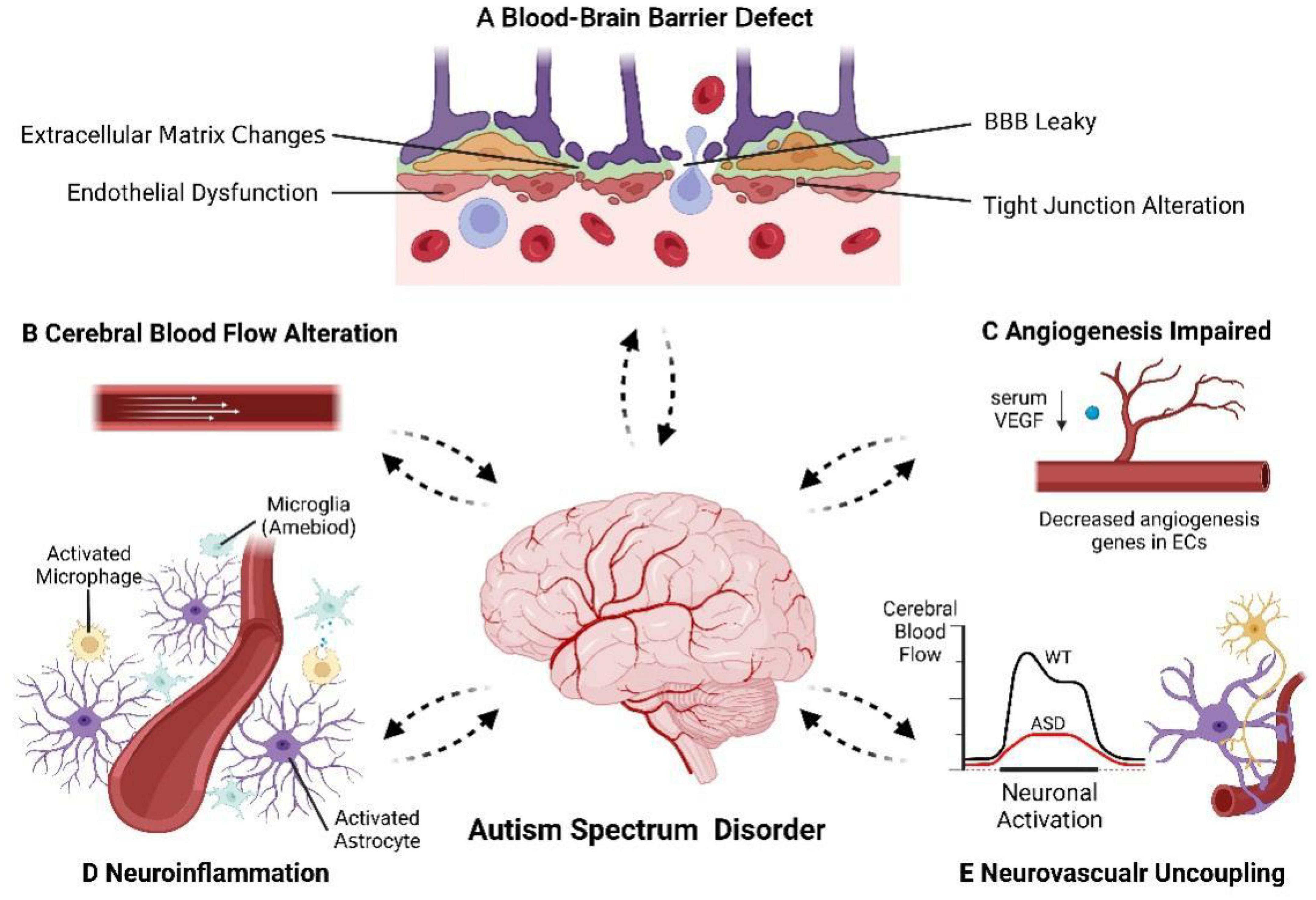
Figure 2. The cerebrovascular alteration in autism spectrum disorder. The cerebrovascular, especially BBB and NVU, appears to have deficits in ASD patients where we see: (A) BBB defect. (B) CBF alteration in specific brain regions. (C) Angiogenesis impaired. (D) Neuroinflammation. (E) Neurovascular uncoupling and decreased hemodynamic response. Dotted arrows indicated that the causal relationship between cerebrovascular abnormalities and the pathogenesis of ASD is unclear.
3.1. Impaired cerebral blood supply in autism spectrum disorders
3.1.1. Insights into cerebral blood flow changes in autism spectrum disorders
Common imaging techniques such as Functional magnetic resonance imaging (fMRI), Arterial Spin Labeling (ASL), single-photon emission computed tomography (SPECT), Positron Emission Tomography/Computerized Tomography (PET/CT), and an emerging technique Diffuse correlation spectroscopy (DCS) (Lin et al., 2023) have been used to detect regional cerebral blood flow (rCBF) and can significantly enhance our understanding of the contribution of brain vasculature to ASD.
A case-control study showed that significantly higher rCBF values were prevalent in specific regions in patients with ASD, and the higher the rCBF value, the more severe the socialization deficit. This may be due to alterations in metabolism and axonal function that reduce the role of nerves in cognitive and social functioning, which provokes a compensatory response from glial cells, that results in rCBF increase (Peterson et al., 2019).
3.1.2. Altered vasculogenesis and angiogenesis in autism spectrum disorders
Early in embryonic development, angioblasts are recruited by the neural tube and form the perineural vascular plexus covering the entire neural tube by vasculogenesis (Risau and Wolburg, 1990). After that, the vessel branches from pre-existing vessels establishes a more complex vascular network by angiogenesis. During this process, vascular sprouts from PNVP ingress and extend from the pial surface toward luminal part (Greenberg and Jin, 2005). Subsequently the nascent cerebral vasculature recruits mural cells and forms the extracellular matrix, which undergoes formation, stabilization, branching, pruning, and specialization to acquire features adapted to the brain (Jain, 2003). E15 exposure of rats to the angiogenesis inhibitor and teratogen thalidomide causes abnormal, leaky blood vessels throughout the brain, especially in the cortex, resulting in cortical and hippocampal region malformations, which are implicated in the pathophysiology of various neurological disorders including autism (Hallene et al., 2006). This finding suggests that normal neuronal migration and maturation are reliant on embryonic angiogenesis. Drugs or events that affect vasculogenesis may promote the development of neurological deficits. The role of neuroinflammation on angiogenesis will be mentioned later. Notably, the potent anti-inflammatory and immunomodulatory effects of thalidomide, through suppression of TNF-α and NF-κB, may also underlie this effect (Talaat et al., 2015). There is growing evidence of abnormal angiogenesis in ASD. Chromosomal domain helicase DNA-binding protein 8 (Chd8) is one of the ASD risk genes (Satterstrom et al., 2020). Chd8 haploinsufficient old mice exposed to the insecticide deltamethrin showed downregulation of the vascular endothelium-associated genes Kdr and Ptprb in the cerebral cortex (Jiménez et al., 2022). However, immunohistochemical staining did not reveal significant changes in vessel length or density in the deltamethrin-exposed mice. Moreover, de novo mutations in brain-specific angiogenesis inhibitor (BAI1/ADGRB1) were identified in ASD patients (Satterstrom et al., 2020; Shiu et al., 2022). VEGF is an important pro-angiogenic factor that also acts as a key molecule for neuronal survival and axonal growth (Yasuhara et al., 2004; Takahashi and Shibuya, 2005). Decreased serum VEGF concentrations and increased sVEGFR-1 levels were found in patients with severe ASD (Emanuele et al., 2010). Although it is uncertain whether peripheral concentrations respond to CNS expression levels, this result predicts a reduced rate of angiogenesis. This demonstrates the presence of an anti-angiogenic environment in ASD, which may ultimately lead to abnormal perfusion as well as impaired neuronal function (Emanuele et al., 2010). However, the limited sample size in this study poses certain limitations, and other studies have yielded differing results (Kajizuka et al., 2010). It is worth noting that 16p11.2df/ + mice exhibited impaired cerebral angiogenesis at postnatal day 14 as well as lower the density and branching of endothelial networks throughout the cerebral cortex (Ouellette et al., 2020). This is due to endothelial dysfunction as characterized by downregulation of angiogenic genes in brain ECs from primary 16p11.2df + mice and induced-pluripotent-stem-cell-derived ECs from 16p11.2-deficient human vectors (Ouellette et al., 2020). Interestingly, in addition to reduced vasculogenesis and angiogenesis, hypervascularization was also found to be present in patients with ASD. A 2016 immunocytochemical study of postmortem brain sections from ASD revealed significantly elevated angiogenic markers associated with perivascular pericytes and angiogenic endothelial precursors throughout superior temporal cortex, fusiform cortex, pons/ midbrain and cerebellum (Azmitia et al., 2016). This study indicated that angiogenesis continues after normal development in ASD patients (Azmitia et al., 2016). This persistent microvascular rearrangement in ASD may contribute to brain neuroplasticity, helping to maintain short, localized connections but inhibiting the development of long, complex brain connections required for language and social interactions (Azmitia et al., 2016). These studies collectively suggest the presence of abnormal angiogenesis in ASD and may explain the decrease/increase in CBF in different brain regions as well as the association with various ASD behavioral deficits. CNS vasculature supporter proper neuronal migration and axon pathfinding (Peguera et al., 2021). During embryonic development, vascular defects may affect many vascular-dependent neurodevelopmental processes as well as CBF in ASD.
3.2. Altered blood-brain barrier in autism spectrum disorders
3.2.1. Blood-brain barrier permeability markers in autism spectrum disorders
The BBB is a vital boundary of the brain which consists of brain capillary endothelial cells, and its dysfunction is associated with various neurological disorders (Sweeney et al., 2019). Animal models have provided evidence linking BBB dysfunction to ASD. Solute carrier transporter 7a5 (SLC7A5) is an amino acid transporter on the BBB, which is important for maintaining the level of branched-chain amino acids in the brain. A 2016 study found that deletion of SLC7A5 in BBB ECs in mice leads to severe neurological abnormalities (Tărlungeanu et al., 2016). Homozygous mutations in the SLC7A5 gene have also been observed in patients with ASD, who exhibited unbalanced neuronal activity, autistic behavior, and motor delay (Tărlungeanu et al., 2016). Moreover, in valproic acid-induced ASD mouse model, leakage to Evans blue dye was found in the cerebellum, indicating increased BBB permeability (Kumar and Sharma, 2016). Mounting studies suggest potential alterations in the BBB in ASD individuals. Genetic screens have shown that some autism-related genes regulate BBB function to some extent. Activation of Sonic Hedgehog (Shh) and WNT/β-catenin signaling is critical for angiogenesis, BBB formation, and neurodevelopment (Alvarez et al., 2011). Multiple gene mutations associated with this pathway have been identified as ASD risk mutations that may lead to reduced and impaired regulation of CBF, BBB impairment, leakage of toxic components and defective molecular clearance, neurological dysfunction, and possibly increased incidence of ASD (Gozal et al., 2021). Markers of BBB permeability, including autoantibodies against brain endothelial cells (ECs), S100B protein, platelet-endothelial adhesion molecule-1 (PECAM-1), and vascular cell adhesion molecule-1 (VCAM-1), have been found to be altered in individuals with ASD. A subset of children with ASD had significantly higher serum levels of autoantibodies against brain ECs compared to typically developing individuals, indicating the presence of BBB defect (Connolly et al., 1999). Elevated levels of S100B, a protein produced by brain astrocytes and considered a marker of BBB breakdown, have also been observed in ASD individuals (Marchi et al., 2004; Al-Ayadhi and Mostafa, 2012). Other studies have shown that serum PECAM-1 and VCAM-1 level are lower in ASD and PECAM-1 levels are negatively correlated with head circumference of infants at birth (Tsuchiya et al., 2007; Onore et al., 2012). These adhesion molecules mediate leukocyte infiltration and regulate BBB permeability, cluing that components of the BBB may play a role in ASD (Lee and Benveniste, 1999; Blankenberg et al., 2003).
3.2.2. Altered blood-brain barrier structure in autism spectrum disorders
A small number of studies directly examined BBB properties to further demonstrate BBB dysfunction in ASD. Fiorentino et al. (2016) conducted a study of the post-mortem brain of ASD patients, indicating that the expression of gene associated with BBB integrity are altered including claudin (CLDN)-5 and -12 as well as increased neuroinflammation. Tight junction proteins CLDN-3, -5, and -12 in ECs all contribute to the BBB, in particular, CLDN-5 is now recognized as the predominant isoform (Nitta et al., 2003; Greene et al., 2019). CLDN-5 deficiency is implicated in BBB disorders and has been associated with psychiatric disorders, such as depression, schizophrenia, neurodegenerative disorders, and neuroinflammatory disorders (Menard et al., 2017; Greene et al., 2019). Interestingly, elevated CLDN-5 gene expression and protein content have been consistently observed in the cortex and cerebellum of individuals with ASD (Fiorentino et al., 2016). This may suggest a compensatory mechanism for the destruction of the BBB by neuroinflammation (Fiorentino et al., 2016). An alternative explanation is that due to endothelial trafficking or protein mutations, CLDN proteins fail to be incorporated into tight junctions but continue to be produced compensatively (Fiorentino et al., 2016). Moreover, the CLDN associated with pore formation is increased in the intestine of ASD patients, but the expression of tight junction components of barrier formation is decreased, confirming the possibility of gastrointestinal barrier dysfunction in ASD (Fiorentino et al., 2016). Also in this study, matrix metalloproteinase (MMP)-9 gene expression was increased in ASD subjects and its secretion induced BBB destruction, aligning with the hypothesis of BBB damage (Fiorentino et al., 2016; Vafadari et al., 2016). Overall, this study shows directly evidences of structural alterations in the BBB in the ASD population.
3.2.3. Pathological conditions of autism spectrum disorders associated with blood-brain barrier dysfunction
Although BBB impairment in individuals with ASD has been demonstrated, further research is needed to investigate the mechanisms and the extent of BBB disruption in ASD. Immune dysfunction has also been found in some patients with ASD, characterized by microglia activation and increased cytokines, as well as high expression of immune/inflammatory genes (Vargas et al., 2005; Voineagu et al., 2011; Onore et al., 2012; Gesundheit et al., 2013; Pape et al., 2019). Studies have shown significant upregulation of TSPO gene expression in the brains of ASD individuals, indicating increased microglia activation and reactive astrocyte expression, which are molecular markers of brain inflammation (Lavisse et al., 2012; Fiorentino et al., 2016). Consistent with this, individuals with ASD exhibit decreased levels of brain-derived neurotrophic factor (BDNF) and increased levels of inflammatory biomarkers and acute phase proteins in serum and/or cerebrospinal fluid, hinting at the early neurodevelopmental and altered immune responses in ASD (Pardo et al., 2005; Zimmerman et al., 2005; Skogstrand et al., 2019). Neuroinflammation and oxidative stress of ASD trigger cell connection breakdown and cytoskeletal recombination of ECs, damaging the BBB (Kumar et al., 2009; Daneman and Prat, 2015). Under stressful conditions, the hypothalamus secretes corticotropin-releasing hormone (CRH) and neurotensin (NT) to stimulate the release of VEGF from mast cells in the brain, leading to the increase of BBB permeability, resulting in focal encephalitis that may be associated with an increased incidence of ASD (Theoharides, 2013). Some pathological states associated with the development of ASD for example, gastrointestinal abnormalities and perinatal infections may also lead to abnormalities in the cerebrovascular and BBB. A hypothesis based on the gut-brain axis suggested that antigen and immune complex impairment of the gut barrier and BBB may act as part of subsequent inflammatory activation and neurological disease (Fiorentino et al., 2016; Margolis et al., 2021; Socała et al., 2021). Bile acids and lipopolysaccharide (LPS) produced by bacteria can induce the release of cytokines to affect BBB permeability (Quinn et al., 2014; Goyal et al., 2021). Interestingly, bacterial fermentation products short-chain fatty acids (SCFAs) affect the maturation of CNS microglia as signaling molecules within the nervous system (Erny et al., 2015). Moreover, maternal viral infection can be directly or indirectly involved in the mechanism of BBB defects. Dengue virus disrupts the glycocalyx of endodermal (Puerta-Guardo et al., 2016), and Zika virus and Herpes Simplex Virus release cytokine TNFα, both of which can downregulate the tight junction protein expression and alter BBB integrity (Chiu et al., 2020; He et al., 2020). Inflammatory cytokines (e.g., IL-6 and TNF) released by maternal viral infection during pregnancy can cross the placenta (Meyer et al., 2009) and act as a neuroimmune “switch” for ASD, beginning to activate the inflammatory cascade of microglia, affecting brain and BBB development with behavioral abnormalities (Brudnak, 2001; Zawadzka et al., 2021). Collectively, abnormal neuroinflammation and oxidative stress in the brain are consistently observed as co-morbid conditions in ASD, closely associated with BBB dysfunction, and collectively contribute to the pathophysiology of ASD. Abnormal immune activation in the CNS is involved to some extent in BBB damage, while systemic inflammation may impact normal brain development due to BBB dysfunction in individuals with ASD.
3.3. Altered neurovascular unit in autism spectrum disorders
3.3.1. Altered hemodynamic responses in autism spectrum disorders
Although localized to CNS endothelial cells, its function also relies on the collaboration of other cell types, including pericytes and astrocytes. These cells together with neurons and smooth muscle cells form a multicellular system, termed NVU, to regulate CBF as needed to coordinate brain function (Langen et al., 2019). Individuals with ASD have been observed to exhibit smaller hemodynamic responses in specific brain regions, such as the dorsolateral prefrontal cortex, bilateral ventrolateral prefrontal cortex, and anterior temporal cortex, during various tasks (Kawakubo et al., 2009; Hosokawa et al., 2015; Uratani et al., 2019; Ohtani et al., 2021). This change was reported in children with ASD during color-word task, auditory tasks, and self-face recognition using near-infrared spectroscopy (NIRS) measurements (Kita et al., 2011; Funabiki et al., 2012; Uratani et al., 2019). In the letter fluency task, measurement of relative hemoglobin concentrations in the prefrontal cortex revealed no significant change in the child group with ASD, but instead decreased in the adult group (Kawakubo et al., 2009). However, there are studies showing different result (Feczko et al., 2012; Xiao et al., 2012). Hemodynamics itself is heterogeneous across brain regions and subjects and is influenced by both neural activity and non-neural factors (Reynell and Harris, 2013; Yan et al., 2018). Therefore, it is uncertain whether hemodynamic changes in these studies directly reflect abnormalities in NVU. Notably, a recent study reliably confirmed the presence of neurovascular uncoupling in 16p11.2df/ + mouse model of ASD. The experiment increased neuronal activation by stimulating whiskers, but induced a weaker vascular response in mutant young adult male mice (Ouellette et al., 2020). After excluding abnormalities in vascular smooth muscle cell function, it was postulated that these neurovascular impairments may be a result of defective vasodilation caused by endothelial deficit (Ouellette et al., 2020). Overall, these studies suggest that hemodynamic abnormalities are found in patients with ASD, affecting the supply of oxygen and nutrients to some brain regions, possibly due to NVU defect.
3.3.2. Altered elements of neurovascular unit in autism spectrum disorders
The contribution of the composition of NVU, including ECs, pericytes, astrocytes, and microglia, to proper brain development and function, is being increasingly elucidated (Carrier et al., 2020). Various elements of the NVU have been found to be disrupted in recent years in ASD. The potential impairment of vascular endothelial in ASD has been mentioned in individual research, characterized by downregulation of angiogenic genes in ECs and endothelium-dependent vasoconstriction abnormalities (Ouellette et al., 2020). In addition, it has been reported that oxidative stress in ASD increases the formation of F2-isoprenaline leading to platelet and vascular endothelial activation (Yao et al., 2006). The interaction of ECs and nerve cells ensures proper brain function (Segarra et al., 2018). Although VEGF-A is a pro-angiogenic substance secreted primarily by neuronal cells, it is also expressed in ECs to regulate the migration and localization of neurons during early development, as well as the interneuron spatial association to vessels. Vascular-specific knockout of VEGF-A contributed to impaired angiogenesis, defects in cortical cytoarchitecture, and axonal tracts in the telencephalon (Li et al., 2013). Similarly, GABA is also partly secreted by the vascular system and influences the migration of cortical neurons during embryonic development (Li et al., 2018). When the secretion of GABA in vascular ECs is deficient, the interruption of GABA signal in ECs and neurons leads to impaired forebrain development, affecting normal angiogenesis, neurogenesis, projection neurons, and the migration of GABAergic neurons, resulting in autistic behaviors (Li et al., 2018; Choi and Vasudevan, 2019). Considering the importance of the vascular endothelium, its contribution in ASD is gaining more and more attention. Moreover, pericytes are embedded in the basement membrane outside the endothelium of small vessels, including capillaries (Winkler et al., 2011). Pericytes have been reported to be involved in regulating NVU and BBB integrity, angiogenesis, regulation of CBF, and neuroinflammation (Zehendner et al., 2013; Sweeney et al., 2016). A 2016 immunocytochemical study of post-mortem brain sections from ASD identified elevated expression of nestin in pericytes in specific brain region, indirectly indicating continued angiogenesis after normal development in ASD patients (Azmitia et al., 2016). Mercier et al. (2011) also found vascular alterations and associated extracellular matrix changes in the lateral ventricular neurogenic area of adult BTBR T+ tf/J mice, showing arterial enlargement and choroid plexus atrophy. Furthermore, astrocytes are the most abundant cells in the CNS. In addition to the traditional function of providing support to neurons, differentiated astrocytes can release signaling molecules such as Ang-1 and TGF-B to promote BBB maturation (Lee et al., 2003; Daneman et al., 2009). The characteristic structure of the perivascular astrocyte foot processes, orthogonal arrays of particles (OAPs), plays an important role in the homeostasis of the glial-endothelial interface (Wolburg et al., 2011). Two markers of abnormal glial-neuronal communication, aquaporin 4 (an important component of OAP) and connexin 43, both were found to be altered in ASD subjects, possibly associated with increased BBB permeability and neuroinflammation (Fatemi et al., 2008). Besides, S100B and glial fibrillary acidic protein (GFAP) values expressed by astrocytes in the serum of children with ASD are higher than those of normal controls and are expected to be an indicator of the severity of neurological damage (Al-Ayadhi and Mostafa, 2012; Esnafoglu et al., 2017). In addition, microglia is a special cell type in NVU, which are resident macrophages of brain tissue that develop from hematopoietic progenitor cells in yolk sac before the BBB closure (Nayak et al., 2014; Colonna and Butovsky, 2017). Microglia also can affect neuronal proliferation, migration, and programmed death, promotes the construction of neural circuits and myelination, now are also thought to have the ability to promote vascular germination and branching (Fantin et al., 2010; Rymo et al., 2011; Ganguli and Chavali, 2021). Recent studies suggest that microglia in ASD patients are more active, and show characteristic amoeboid patterns that can present antigens, release more cytokines, and recruit white blood cells in blood vessels (Zlokovic, 2008; Morgan et al., 2010; Suzuki et al., 2013). Some scholars attribute the stereotypic behavior and social dysfunction of ASD patients to the effect of microglial activation and leukocyte infiltration on neural development, leading to neurological dysfunction (Zhan et al., 2014). Platelets are recruited under the damaged endodermis during neuroinflammation, releasing inflammatory mediators and recruiting other inflammatory cells, exacerbating the inflammatory process (Deppermann, 2018). The CNS has long been regarded as an immune privilege site due to the presence of BBB, but the brain cannot be separated from the peripheral immune system (Zengeler and Lukens, 2021). The effects of neuroimmune on CNS development are multifaceted. Normal immune cells are necessary for CNS development, such as astrocytes can participate in synapse formation and regulation (Volterra and Meldolesi, 2005). However, abnormal neuroinflammation in the ASD brain can cause extensive damage to the constituent cells of the NVU. Therefore, it is reasonable to hypothesize that impairments in cerebrovascular features and immune activation interactions play a significant role in the pathophysiology of ASD.
4. Discussion and prospect
Indeed, these cerebrovascular characteristics mentioned earlier are not independent in ASD. Proper cerebral blood supply relies on both normal cerebrovascular network and regulation of neurovascular coupling. Disruptions in either of these factors can impair brain maturation by compromising the oxygen and blood supply necessary for proper brain function. Furthermore, systemic inflammation and immune activation have long been associated with the pathogenesis of ASD as co-morbid conditions. One potential mechanism is inflammation, derived from environmental factors (e.g., gut-brain axis dysbiosis, perinatal maternal infections, vaccines) and genetic susceptibility, as a source of cerebrovascular susceptibility (Theoharides, 2013; Zengeler and Lukens, 2021). Indeed, neuroinflammation could damage the component cells of NVC. Defective NVC cells are unable to maintain the integrity of the BBB, resulting in BBB leakage and inflammatory mediators and cells from the periphery easily entering and damaging the brain. This mechanism has been demonstrated in other psychiatric disorders, such as schizophrenia and Alzheimer’s disease (Carrier et al., 2020; Korte et al., 2020).
The vascular and nervous systems construct a highly specialized system separately, but share overlapping mechanisms during embryonic development. It is plausible that abnormalities in vascular development could adversely affect associated neurodevelopmental processes. Regrettably, these studies that have explored the neurovascular interactions of ASD are largely non-developmental, providing only a snapshot at a single point in time (Azmitia et al., 2016; Fiorentino et al., 2016). For example, studies on cerebrovascular alterations in patients with ASD have been conducted using post-mortem brains (Azmitia et al., 2016; Fiorentino et al., 2016), making it challenging to determine the timing of these defects. Although these studies have identified cerebrovascular vasculature as an important component of ASD, it is unclear whether changes in vascular structure and function are a cause or a consequence of neuropathy in ASD. More research is needed to further explore the pivotal role of cerebrovascular defects in the neuropathology of ASD. Additionally, the limited sample sizes in these studies warrant caution, considering the complex pathophysiology and heterogeneous phenotype of ASD.
However, recent studies have provided evidence of cerebrovascular alterations in individuals with ASD (Azmitia et al., 2016; Fiorentino et al., 2016; Bjørklund et al., 2018). Hypoperfusion and neuroinflammation caused by cerebrovascular alterations may emerge as a new potential pathogenesis of ASD. Thus, conceptually, treatments that improve intracerebral hypoperfusion or restore defective BBB and NVU may show beneficial effects in patients with ASD. In fact, several studies based on the repair of cerebrovascular features have made many new advances. As previously described, perfusion abnormalities in specific cortical regions are associated with key features of ASD (Ohnishi et al., 2000; Bjørklund et al., 2018). Improve hypoperfusion through vasodilatation or pro-angiogenesis may be useful. Considering that the VEGF pathway plays an important role in neurovascular communication, more and more studies have focused on this pathway as a potential therapeutic target of neurodevelopmental disorders and neurodegenerative diseases. In mice experiment, VEGF-C injections were found to dilate blood vessel diameter, restore cerebrospinal fluid flow, and ultimately improve cognitive test results (Da Mesquita et al., 2018). Besides, transplantation of VEGF-A-producing cells has been shown to promote the repair of brain damage caused by ischemia in neonatal rats (Yao et al., 2016). Other studies indicate that intrathecal transplantation of autologous bone marrow mononuclear cells (BMMNCs) in 32 patients with autism has shown significant clinical effects by promoting angiogenesis to improve perfusion and balance inflammation through immunomodulation (Sharma et al., 2013). Notably, the persistent proliferation of blood vessels in the brain of ASD patients after normal development may be related to the disorder of brain functional connectivity and the increased incidence of epilepsy. Anti-VEGF therapies may reduce abnormal neuronal activity in ASD patients by inhibiting vascular plasticity (Greenberg and Jin, 2005; Shibuya, 2009; Azmitia et al., 2016). However, given the high heterogeneity of pathophysiological conditions in individuals with ASD, further studies are warranted to evaluate the feasibility and effectiveness of pro/anti-angiogenic therapies in this population.
In addition, restoring the integrity of the BBB may also benefit patients with ASD by re-establishing vascular function and providing neuroprotection. Study has demonstrated that once-daily administration of P7C3-A20, a compound that stabilizes cellular energy levels, is beneficial to the recovery of BBB endothelial injury, neuroinflammation reduction, and cognitive recovery after traumatic brain injury (TBI) (Vázquez-Rosa et al., 2020). Alvarez et al. (2011) claimed that shh secreted by astrocytes binds to receptors in BBB ECs, has a BBB protective effect. Tumor necrosis-alpha (TNF-α) and interferon-gamma (IFN-γ) -treated astrocytes increased Shh production, to promote BBB repair and balance inflammation induced during BBB injury (Alvarez et al., 2011). This study provides new avenues for designing treatments to repair BBB. Balancing neuroinflammation in the brain is also beneficial for defective BBB and NVU. For example, pioglitazone is a non-steroidal anti-inflammatory drug (NSAID) was found can improve ASD symptoms by reducing microglial activation and subsequent inflammation in children with ASD (Emanuele et al., 2007). Immunoregulatory therapy may be an effective option as a personalized treatment for patients with a distinct inflammatory phenotype (Pape et al., 2019).
Moreover, interventions targeting ASD risk genes associated with neurovascular development, are also expected to provide potential therapeutic benefits in the management of ASD symptoms (Baranova et al., 2021). The Wnt/Shh pathway is critical for controlling neural progenitor cell differentiation, neuronal migration, synaptogenesis, NVU development, and BBB formation, the disruption of the pathway may lead to neurodevelopmental disorders including ASD (Gozal et al., 2021). Therapeutic interventions based on components of the Wnt/Shh pathway are currently being tested or used for the treatment of various neurological diseases (Baranova et al., 2021; Rahi and Mehan, 2022).
5. Conclusion
This review focuses on neurovascular communication during embryonic development and recent research advancements regarding cerebrovascular alterations in ASD, aiming to deepen our understanding of ASD pathogenesis. We also discuss the possibility of these findings as a mechanistic basis for vascular-based therapy for ASD. As we remarked above, these treatment attempts have yielded some positive results, which further confirm the presence of cerebrovascular defects in ASD individuals. Although these current treatments targeting cerebrovascular structure and function do not provide a cure for autism, it is certain that they have great potential to control disease progression and improve quality of life. The investigation of ASD has increasingly emphasized the role of the cerebrovascular component. These studies provide additional evidence for exploring the mechanisms underlying ASD and demonstrate that ASD can be considered as a neurovascular disease. The cerebral vasculature is crucial for normal brain function, and emerging evidence has linked it to various neurological disorders. It is curious that similar vascular abnormalities are found in neurological diseases with different pathogenesis and clinical features. Cerebrovascular defects make the brain more vulnerable, and it is reasonable to assume that the vascular plays a more important role than previously thought. However, much of the evidence supporting the vascular basis of neurological disease needs to be explored in additional causal studies, to determine the extent to which cerebrovascular alterations are involved in pathogenesis or whether these changes do as a result of pathophysiological changes.
Author contributions
YW: writing-original draft. YW, SY, and ML: writing-review and editing. All authors have read and agreed to the published version of the manuscript.
Acknowledgments
Special thanks to Dingfa Liang for providing support and guidance. The graphical abstracts were created using BioRender software (biorender.com).
Conflict of interest
The authors declare that the research was conducted in the absence of any commercial or financial relationships that could be construed as a potential conflict of interest.
Publisher’s note
All claims expressed in this article are solely those of the authors and do not necessarily represent those of their affiliated organizations, or those of the publisher, the editors and the reviewers. Any product that may be evaluated in this article, or claim that may be made by its manufacturer, is not guaranteed or endorsed by the publisher.
References
Abbott, N. J., Rönnbäck, L., and Hansson, E. (2006). Astrocyte-endothelial interactions at the blood-brain barrier. Nat. Rev. Neurosci. 7, 41–53. doi: 10.1038/nrn1824
Al-Ayadhi, L. Y., and Mostafa, G. A. (2012). A lack of association between elevated serum levels of S100B protein and autoimmunity in autistic children. J. Neuroinflammation 9:54. doi: 10.1186/1742-2094-9-54
Alvarez, J. I., Dodelet-Devillers, A., Kebir, H., Ifergan, I., Fabre, P. J., Terouz, S., et al. (2011). The Hedgehog pathway promotes blood-brain barrier integrity and CNS immune quiescence. Science 334, 1727–1731. doi: 10.1126/science.1206936
Ardalan, M., Chumak, T., Quist, A., Hermans, E., Hoseinpoor Rafati, A., Gravina, G., et al. (2022). Reelin cells and sex-dependent synaptopathology in autism following postnatal immune activation. Br. J. Pharmacol. 179, 4400–4422. doi: 10.1111/bph.15859
Attwell, D., Buchan, A. M., Charpak, S., Lauritzen, M., MacVicar, B. A., and Newman, E. A. (2010). Glial and neuronal control of brain blood flow. Nature 468, 232–243.
Azmitia, E. C., Saccomano, Z. T., Alzoobaee, M. F., Boldrini, M., and Whitaker-Azmitia, P. M. (2016). Persistent angiogenesis in the autism brain: An immunocytochemical study of postmortem cortex, brainstem and cerebellum. J. Autism Dev. Disord. 46, 1307–1318. doi: 10.1007/s10803-015-2672-6
Baranova, J., Dragunas, G., Botellho, M. C. S., Ayub, A. L. P., Bueno-Alves, R., Alencar, R. R., et al. (2021). Autism spectrum disorder: Signaling pathways and prospective therapeutic targets. Cell. Mol. Neurobiol. 41, 619–649. doi: 10.1007/s10571-020-00882-7
Bautch, V. L., and James, J. M. (2009). Neurovascular development: The beginning of a beautiful friendship. Cell Adh. Migr. 3, 199–204. doi: 10.4161/cam.3.2.8397
Bjørklund, G., Kern, J. K., Urbina, M. A., Saad, K., El-Houfey, A. A., Geier, D. A., et al. (2018). Cerebral hypoperfusion in autism spectrum disorder. Acta Neurobiol. Exp. (Wars) 78, 21–29.
Blankenberg, S., Barbaux, S., and Tiret, L. (2003). Adhesion molecules and atherosclerosis. Atherosclerosis 170, 191–203. doi: 10.1016/s0021-9150(03)00097-2
Brudnak, M. A. (2001). Application of genomeceuticals to the molecular and immunological aspects of autism. Med. Hypotheses 57, 186–191. doi: 10.1054/mehy.2001.1331
Buescher, A. V., Cidav, Z., Knapp, M., and Mandell, D. S. (2014). Costs of autism spectrum disorders in the United Kingdom and the United States. JAMA Pediatr. 168, 721–728. doi: 10.1001/jamapediatrics.2014.210
Carrier, M., Guilbert, J., Lévesque, J. P., Tremblay, M., and Desjardins, M. (2020). Structural and functional features of developing brain capillaries, and their alteration in schizophrenia. Front. Cell. Neurosci. 14:595002. doi: 10.3389/fncel.2020.595002
Chiu, C. F., Chu, L. W., Liao, I. C., Simanjuntak, Y., Lin, Y. L., Juan, C. C., et al. (2020). The mechanism of the zika virus crossing the placental barrier and the blood-brain barrier. Front. Microbiol. 11:214. doi: 10.3389/fmicb.2020.00214
Choi, Y. K., and Vasudevan, A. (2019). Mechanistic insights into autocrine and paracrine roles of endothelial GABA signaling in the embryonic forebrain. Sci. Rep. 9:16256. doi: 10.1038/s41598-019-52729-x
Colonna, M., and Butovsky, O. (2017). Microglia function in the central nervous system during health and neurodegeneration. Annu. Rev. Immunol. 35, 441–468. doi: 10.1146/annurev-immunol-051116-052358
Colvert, E., Tick, B., McEwen, F., Stewart, C., Curran, S. R., Woodhouse, E., et al. (2015). Heritability of autism spectrum disorder in a UK population-based twin sample. JAMA Psychiatry 72, 415–423. doi: 10.1001/jamapsychiatry.2014.3028
Connolly, A. M., Chez, M. G., Pestronk, A., Arnold, S. T., Mehta, S., and Deuel, R. K. (1999). Serum autoantibodies to brain in Landau-Kleffner variant, autism, and other neurologic disorders. J. Pediatr. 134, 607–613. doi: 10.1016/s0022-3476(99)70248-9
Courchesne, E., and Pierce, K. (2005). Why the frontal cortex in autism might be talking only to itself: Local over-connectivity but long-distance disconnection. Curr. Opin. Neurobiol. 15, 225–230. doi: 10.1016/j.conb.2005.03.001
Da Mesquita, S., Louveau, A., Vaccari, A., Smirnov, I., Cornelison, R. C., Kingsmore, K. M., et al. (2018). Functional aspects of meningeal lymphatics in ageing and Alzheimer’s disease. Nature 560, 185–191. doi: 10.1038/s41586-018-0368-8
Daneman, R., Agalliu, D., Zhou, L., Kuhnert, F., Kuo, C. J., and Barres, B. A. (2009). Wnt/beta-catenin signaling is required for CNS, but not non-CNS, angiogenesis. Proc. Natl. Acad. Sci. U.S.A. 106, 641–646. doi: 10.1073/pnas.0805165106
Daneman, R., and Prat, A. (2015). The blood-brain barrier. Cold Spring Harb. Perspect. Biol. 7:a020412. doi: 10.1101/cshperspect.a020412
Daneman, R., Zhou, L., Kebede, A. A., and Barres, B. A. (2010). Pericytes are required for blood–brain barrier integrity during embryogenesis. Nature 468, 562–566. doi: 10.1038/nature09513
Deppermann, C. (2018). Platelets and vascular integrity. Platelets 29, 549–555. doi: 10.1080/09537104.2018.1428739
Emanuele, E., Lossano, C., Politi, P., and Barale, F. (2007). Pioglitazone as a therapeutic agent in autistic spectrum disorder. Med. Hypotheses 69:699. doi: 10.1016/j.mehy.2007.01.018
Emanuele, E., Orsi, P., Barale, F., di Nemi, S. U., Bertona, M., and Politi, P. (2010). Serum levels of vascular endothelial growth factor and its receptors in patients with severe autism. Clin. Biochem. 43, 317–319. doi: 10.1016/j.clinbiochem.2009.10.005
Erny, D., Hrabě de Angelis, A. L., Jaitin, D., Wieghofer, P., Staszewski, O., David, E., et al. (2015). Host microbiota constantly control maturation and function of microglia in the CNS. Nat. Neurosci. 18, 965–977. doi: 10.1038/nn.4030
Esnafoglu, E., Ayyıldız, S. N., Cırrık, S., Erturk, E. Y., Erdil, A., Daglı, A., et al. (2017). Evaluation of serum Neuron-specific enolase, S100B, myelin basic protein and glial fibrilliary acidic protein as brain specific proteins in children with autism spectrum disorder. Int. J. Dev. Neurosci. 61, 86–91. doi: 10.1016/j.ijdevneu.2017.06.011
Fantin, A., Vieira, J. M., Gestri, G., Denti, L., Schwarz, Q., Prykhozhij, S., et al. (2010). Tissue macrophages act as cellular chaperones for vascular anastomosis downstream of VEGF-mediated endothelial tip cell induction. Blood 116, 829–840. doi: 10.1182/blood-2009-12-257832
Fatemi, S. H., Folsom, T. D., Reutiman, T. J., and Lee, S. (2008). Expression of astrocytic markers aquaporin 4 and connexin 43 is altered in brains of subjects with autism. Synapse 62, 501–507. doi: 10.1002/syn.20519
Feczko, E., Miezin, F. M., Constantino, J. N., Schlaggar, B. L., Petersen, S. E., and Pruett, J. R. Jr. (2012). The hemodynamic response in children with Simplex Autism. Dev. Cogn. Neurosci. 2, 396–408. doi: 10.1016/j.dcn.2012.06.001
Ferrara, N., Gerber, H. P., and LeCouter, J. (2003). The biology of VEGF and its receptors. Nat. Med. 9, 669–676. doi: 10.1038/nm0603-669
Fiorentino, M., Sapone, A., Senger, S., Camhi, S. S., Kadzielski, S. M., Buie, T. M., et al. (2016). Blood-brain barrier and intestinal epithelial barrier alterations in autism spectrum disorders. Mol. Autism 7:49. doi: 10.1186/s13229-016-0110-z
Frühbeis, C., Fröhlich, D., and Krämer-Albers, E. M. (2012). Emerging roles of exosomes in neuron-glia communication. Front. Physiol. 3:119. doi: 10.3389/fphys.2012.00119
Funabiki, Y., Murai, T., and Toichi, M. (2012). Cortical activation during attention to sound in autism spectrum disorders. Res. Dev. Disabil. 33, 518–524. doi: 10.1016/j.ridd.2011.10.016
Ganguli, S., and Chavali, P. L. (2021). Intrauterine viral infections: Impact of inflammation on fetal neurodevelopment. Front. Neurosci. 15:771557. doi: 10.3389/fnins.2021.771557
Gerhardt, H., Golding, M., Fruttiger, M., Ruhrberg, C., Lundkvist, A., Abramsson, A., et al. (2003). VEGF guides angiogenic sprouting utilizing endothelial tip cell filopodia. J. Cell Biol. 161, 1163–1177. doi: 10.1083/jcb.200302047
Geschwind, D. H., and State, M. W. (2015). Gene hunting in autism spectrum disorder: On the path to precision medicine. Lancet Neurol. 14, 1109–1120. doi: 10.1016/s1474-4422(15)00044-7
Gesundheit, B., Rosenzweig, J. P., Naor, D., Lerer, B., Zachor, D. A., Procházka, V., et al. (2013). Immunological and autoimmune considerations of autism spectrum disorders. J. Autoimmun. 44, 1–7. doi: 10.1016/j.jaut.2013.05.005
Goyal, D., Ali, S. A., and Singh, R. K. (2021). Emerging role of gut microbiota in modulation of neuroinflammation and neurodegeneration with emphasis on Alzheimer’s disease. Prog. Neuropsychopharmacol. Biol. Psychiatry 106:110112. doi: 10.1016/j.pnpbp.2020.110112
Gozal, E., Jagadapillai, R., Cai, J., and Barnes, G. N. (2021). Potential crosstalk between sonic hedgehog-WNT signaling and neurovascular molecules: Implications for blood-brain barrier integrity in autism spectrum disorder. J. Neurochem. 159, 15–28. doi: 10.1111/jnc.15460
Greenberg, D. A., and Jin, K. (2005). From angiogenesis to neuropathology. Nature 438, 954–959. doi: 10.1038/nature04481
Greene, C., Hanley, N., and Campbell, M. (2019). Claudin-5: Gatekeeper of neurological function. Fluids Barriers CNS 16:3. doi: 10.1186/s12987-019-0123-z
Hallene, K. L., Oby, E., Lee, B. J., Santaguida, S., Bassanini, S., Cipolla, M., et al. (2006). Prenatal exposure to thalidomide, altered vasculogenesis, and CNS malformations. Neuroscience 142, 267–283. doi: 10.1016/j.neuroscience.2006.06.017
Hazlett, H. C., Gu, H., Munsell, B. C., Kim, S. H., Styner, M., Wolff, J. J., et al. (2017). Early brain development in infants at high risk for autism spectrum disorder. Nature 542, 348–351. doi: 10.1038/nature21369
He, Q., Liu, H., Huang, C., Wang, R., Luo, M., and Lu, W. (2020). Herpes simplex virus 1-induced blood-brain barrier damage involves apoptosis associated with GM130-mediated golgi stress. Front. Mol. Neurosci. 13:2. doi: 10.3389/fnmol.2020.00002
Hosokawa, M., Nakadoi, Y., Watanabe, Y., Sumitani, S., and Ohmori, T. (2015). Association of autism tendency and hemodynamic changes in the prefrontal cortex during facial expression stimuli measured by multi-channel near-infrared spectroscopy. Psychiatry Clin. Neurosci. 69, 145–152. doi: 10.1111/pcn.12240
Iadecola, C. (2017). The neurovascular unit coming of age: A journey through neurovascular coupling in health and disease. Neuron 96, 17–42. doi: 10.1016/j.neuron.2017.07.030
Jain, R. K. (2003). Molecular regulation of vessel maturation. Nat. Med. 9, 685–693. doi: 10.1038/nm0603-685
James, J. M., and Mukouyama, Y.-S. (2011). Neuronal action on the developing blood vessel pattern. Semin. Cell Dev. Biol. 22, 1019–1027.
James, J. M., Gewolb, C., and Bautch, V. L. (2009). Neurovascular development uses VEGF-A signaling to regulate blood vessel ingression into the neural tube. Development 136, 833–841. doi: 10.1242/dev.028845
Jiménez, J. A., Simon, J. M., Hu, W., Moy, S. S., Harper, K. M., Liu, C. W., et al. (2022). Developmental pyrethroid exposure and age influence phenotypes in a Chd8 haploinsufficient autism mouse model. Sci. Rep. 12:5555. doi: 10.1038/s41598-022-09533-x
Johnson, M. H., Jones, E. J., and Gliga, T. (2015). Brain adaptation and alternative developmental trajectories. Dev. Psychopathol. 27, 425–442. doi: 10.1017/s0954579415000073
Kajizuka, M., Miyachi, T., Matsuzaki, H., Iwata, K., Shinmura, C., Suzuki, K., et al. (2010). Serum levels of platelet-derived growth factor BB homodimers are increased in male children with autism. Prog. Neuropsychopharmacol. Biol. Psychiatry 34, 154–158. doi: 10.1016/j.pnpbp.2009.10.017
Kawakubo, Y., Kuwabara, H., Watanabe, K., Minowa, M., Someya, T., Minowa, I., et al. (2009). Impaired prefrontal hemodynamic maturation in autism and unaffected siblings. PLoS One 4:e6881. doi: 10.1371/journal.pone.0006881
Kita, Y., Gunji, A., Inoue, Y., Goto, T., Sakihara, K., Kaga, M., et al. (2011). Self-face recognition in children with autism spectrum disorders: A near-infrared spectroscopy study. Brain Dev. 33, 494–503. doi: 10.1016/j.braindev.2010.11.007
Korte, N., Nortley, R., and Attwell, D. (2020). Cerebral blood flow decrease as an early pathological mechanism in Alzheimer’s disease. Acta Neuropathol. 140, 793–810. doi: 10.1007/s00401-020-02215-w
Kumar, H., and Sharma, B. (2016). Memantine ameliorates autistic behavior, biochemistry & blood brain barrier impairments in rats. Brain Res. Bull. 124, 27–39. doi: 10.1016/j.brainresbull.2016.03.013
Kumar, P., Shen, Q., Pivetti, C. D., Lee, E. S., Wu, M. H., and Yuan, S. Y. (2009). Molecular mechanisms of endothelial hyperpermeability: Implications in inflammation. Expert Rev. Mol. Med. 11:e19. doi: 10.1017/s1462399409001112
Langen, U. H., Ayloo, S., and Gu, C. (2019). Development and cell biology of the blood-brain barrier. Annu. Rev. Cell Dev. Biol. 35, 591–613. doi: 10.1146/annurev-cellbio-100617-062608
Lavisse, S., Guillermier, M., Hérard, A. S., Petit, F., Delahaye, M., Van Camp, N., et al. (2012). Reactive astrocytes overexpress TSPO and are detected by TSPO positron emission tomography imaging. J. Neurosci. 32, 10809–10818. doi: 10.1523/jneurosci.1487-12.2012
Lee, S. J., and Benveniste, E. N. (1999). Adhesion molecule expression and regulation on cells of the central nervous system. J. Neuroimmunol. 98, 77–88. doi: 10.1016/s0165-5728(99)00084-3
Lee, S. W., Kim, W. J., Choi, Y. K., Song, H. S., Son, M. J., Gelman, I. H., et al. (2003). SSeCKS regulates angiogenesis and tight junction formation in blood-brain barrier. Nat. Med. 9, 900–906. doi: 10.1038/nm889
Leigh, J. P., and Du, J. (2015). Brief report: Forecasting the economic burden of autism in 2015 and 2025 in the United States. J. Autism Dev. Disord. 45, 4135–4139. doi: 10.1007/s10803-015-2521-7
Li, S., Haigh, K., Haigh, J. J., and Vasudevan, A. (2013). Endothelial VEGF sculpts cortical cytoarchitecture. J. Neurosci. 33, 14809–14815. doi: 10.1523/jneurosci.1368-13.2013
Li, S., Kumar, T. P., Joshee, S., Kirschstein, T., Subburaju, S., Khalili, J. S., et al. (2018). Endothelial cell-derived GABA signaling modulates neuronal migration and postnatal behavior. Cell Res. 28, 221–248. doi: 10.1038/cr.2017.135
Lin, F., Huang, W., Lu, S., and Li, J. (2023). Cerebral blood flow measured by diffuse correlation spectroscopy in children with autism spectrum disorder. J. Biophoton. 16:e202300151. doi: 10.1002/jbio.202300151
Lord, C., Elsabbagh, M., Baird, G., and Veenstra-Vanderweele, J. (2018). Autism spectrum disorder. Lancet 392, 508–520. doi: 10.1016/s0140-6736(18)31129-2
Maenner, M. J., Warren, Z., Williams, A. R., Amoakohene, E., Bakian, A. V., Bilder, D. A., et al. (2023). Prevalence and characteristics of autism spectrum disorder among children aged 8 years - autism and developmental disabilities monitoring network, 11 sites, United States, 2020. MMWR Surveill. Summ. 72, 1–14. doi: 10.15585/mmwr.ss7202a1
Magistretti, P. J., and Allaman, I. (2015). A cellular perspective on brain energy metabolism and functional imaging. Neuron 86, 883–901. doi: 10.1016/j.neuron.2015.03.035
Marchi, N., Cavaglia, M., Fazio, V., Bhudia, S., Hallene, K., and Janigro, D. (2004). Peripheral markers of blood-brain barrier damage. Clin. Chim. Acta 342, 1–12. doi: 10.1016/j.cccn.2003.12.008
Margolis, K. G., Cryan, J. F., and Mayer, E. A. (2021). The microbiota-gut-brain axis: From motility to mood. Gastroenterology 160, 1486–1501. doi: 10.1053/j.gastro.2020.10.066
McConnell, H. L., Kersch, C. N., Woltjer, R. L., and Neuwelt, E. A. (2017). The translational significance of the neurovascular unit. J. Biol. Chem. 292, 762–770. doi: 10.1074/jbc.R116.760215
Menard, C., Pfau, M. L., Hodes, G. E., Kana, V., Wang, V. X., Bouchard, S., et al. (2017). Social stress induces neurovascular pathology promoting depression. Nat. Neurosci. 20, 1752–1760. doi: 10.1038/s41593-017-0010-3
Mercier, F., Cho Kwon, Y., and Kodama, R. (2011). Meningeal/vascular alterations and loss of extracellular matrix in the neurogenic zone of adult BTBR T+ tf/J mice, animal model for autism. Neurosci. Lett. 498, 173–178. doi: 10.1016/j.neulet.2011.05.014
Meyer, U., Feldon, J., and Yee, B. K. (2009). A review of the fetal brain cytokine imbalance hypothesis of schizophrenia. Schizophr. Bull. 35, 959–972. doi: 10.1093/schbul/sbn022
Milosevic, J., Maisel, M., Wegner, F., Leuchtenberger, J., Wenger, R. H., Gerlach, M., et al. (2007). Lack of hypoxia-inducible factor-1α impairs midbrain neural precursor cells involving vascular endothelial growth factor signaling. J. Neurosci. 27, 412–421. doi: 10.1523/JNEUROSCI.2482-06.2007
Modabbernia, A., Velthorst, E., and Reichenberg, A. (2017). Environmental risk factors for autism: An evidence-based review of systematic reviews and meta-analyses. Mol. Autism 8:13. doi: 10.1186/s13229-017-0121-4
Morgan, J. T., Chana, G., Pardo, C. A., Achim, C., Semendeferi, K., Buckwalter, J., et al. (2010). Microglial activation and increased microglial density observed in the dorsolateral prefrontal cortex in autism. Biol. Psychiatry 68, 368–376. doi: 10.1016/j.biopsych.2010.05.024
Morita, K., Sasaki, H., Furuse, M., and Tsukita, S. (1999). Endothelial claudin: Claudin-5/TMVCF constitutes tight junction strands in endothelial cells. J. Cell Biol. 147, 185–194. doi: 10.1083/jcb.147.1.185
Nation, D. A., Sweeney, M. D., Montagne, A., Sagare, A. P., D’Orazio, L. M., Pachicano, M., et al. (2019). Blood-brain barrier breakdown is an early biomarker of human cognitive dysfunction. Nat. Med. 25, 270–276. doi: 10.1038/s41591-018-0297-y
Nayak, D., Roth, T. L., and McGavern, D. B. (2014). Microglia development and function. Annu. Rev. Immunol. 32, 367–402. doi: 10.1146/annurev-immunol-032713-120240
Neul, J. L., and Sahin, M. (2015). Therapeutic advances in autism and other neurodevelopmental disorders. Neurotherapeutics 12, 519–520. doi: 10.1007/s13311-015-0364-8
Nikolopoulou, E., Galea, G. L., Rolo, A., Greene, N. D., and Copp, A. J. (2017). Neural tube closure: Cellular, molecular and biomechanical mechanisms. Development 144, 552–566.
Nitta, T., Hata, M., Gotoh, S., Seo, Y., Sasaki, H., Hashimoto, N., et al. (2003). Size-selective loosening of the blood-brain barrier in claudin-5-deficient mice. J. Cell Biol. 161, 653–660. doi: 10.1083/jcb.200302070
Ohnishi, T., Matsuda, H., Hashimoto, T., Kunihiro, T., Nishikawa, M., Uema, T., et al. (2000). Abnormal regional cerebral blood flow in childhood autism. Brain 123(Pt 9), 1838–1844. doi: 10.1093/brain/123.9.1838
Ohtani, T., Wakabayashi, A., Sutoh, C., Oshima, F., Hirano, Y., and Shimizu, E. (2021). Ventrolateral prefrontal hemodynamic responses in autism spectrum disorder with and without depression. PLoS One 16:e0256780. doi: 10.1371/journal.pone.0256780
Onore, C., Careaga, M., and Ashwood, P. (2012). The role of immune dysfunction in the pathophysiology of autism. Brain Behav. Immun. 26, 383–392. doi: 10.1016/j.bbi.2011.08.007
Ouellette, J., and Lacoste, B. (2021). From neurodevelopmental to neurodegenerative disorders: The vascular continuum. Front. Aging Neurosci. 13:749026. doi: 10.3389/fnagi.2021.749026
Ouellette, J., Toussay, X., Comin, C. H., Costa, L. D. F., Ho, M., Lacalle-Aurioles, M., et al. (2020). Vascular contributions to 16p11.2 deletion autism syndrome modeled in mice. Nat. Neurosci. 23, 1090–1101. doi: 10.1038/s41593-020-0663-1
Pape, K., Tamouza, R., Leboyer, M., and Zipp, F. (2019). Immunoneuropsychiatry–novel perspectives on brain disorders. Nat. Rev. Neurol. 15, 317–328. doi: 10.1038/s41582-019-0174-4
Pardo, C. A., Vargas, D. L., and Zimmerman, A. W. (2005). Immunity, neuroglia and neuroinflammation in autism. Int. Rev. Psychiatry 17, 485–495. doi: 10.1080/02646830500381930
Paredes, I., Himmels, P., and Ruiz de Almodóvar, C. (2018). Neurovascular communication during CNS development. Dev. Cell 45, 10–32. doi: 10.1016/j.devcel.2018.01.023
Peguera, B., Segarra, M., and Acker-Palmer, A. (2021). Neurovascular crosstalk coordinates the central nervous system development. Curr. Opin. Neurobiol. 69, 202–213.
Peterson, B. S., Zargarian, A., Peterson, J. B., Goh, S., Sawardekar, S., Williams, S. C. R., et al. (2019). Hyperperfusion of frontal white and subcortical gray matter in autism spectrum disorder. Biol. Psychiatry 85, 584–595. doi: 10.1016/j.biopsych.2018.11.026
Puerta-Guardo, H., Glasner, D. R., and Harris, E. (2016). Dengue virus NS1 disrupts the endothelial glycocalyx, leading to hyperpermeability. PLoS Pathog. 12:e1005738. doi: 10.1371/journal.ppat.1005738
Quinn, M., McMillin, M., Galindo, C., Frampton, G., Pae, H. Y., and DeMorrow, S. (2014). Bile acids permeabilize the blood brain barrier after bile duct ligation in rats via Rac1-dependent mechanisms. Dig. Liver Dis. 46, 527–534. doi: 10.1016/j.dld.2014.01.159
Rahi, S., and Mehan, S. (2022). Understanding abnormal SMO-SHH signaling in autism spectrum disorder: Potential drug target and therapeutic goals. Cell. Mol. Neurobiol. 42, 931–953. doi: 10.1007/s10571-020-01010-1
Reynell, C., and Harris, J. J. (2013). The BOLD signal and neurovascular coupling in autism. Dev. Cogn. Neurosci. 6, 72–79. doi: 10.1016/j.dcn.2013.07.003
Risau, W. (1991). Induction of blood-brain barrier endothelial cell differentiation. Ann. N. Y. Acad. Sci. 633, 405–419. doi: 10.1111/j.1749-6632.1991.tb15630.x
Risau, W., and Wolburg, H. (1990). Development of the blood-brain barrier. Trends Neurosci. 13, 174–178. doi: 10.1016/0166-2236(90)90043-a
Rodier, P. M. (2002). Converging evidence for brain stem injury in autism. Dev. Psychopathol. 14, 537–557. doi: 10.1017/s0954579402003085
Rymo, S. F., Gerhardt, H., Wolfhagen Sand, F., Lang, R., Uv, A., and Betsholtz, C. (2011). A two-way communication between microglial cells and angiogenic sprouts regulates angiogenesis in aortic ring cultures. PLoS One 6:e15846. doi: 10.1371/journal.pone.0015846
Satterstrom, F. K., Kosmicki, J. A., Wang, J., Breen, M. S., De Rubeis, S., An, J. Y., et al. (2020). Large-scale exome sequencing study implicates both developmental and functional changes in the neurobiology of autism. Cell 180, 568–584.e523. doi: 10.1016/j.cell.2019.12.036
Schafer, S. T., Paquola, A. C. M., Stern, S., Gosselin, D., Ku, M., Pena, M., et al. (2019). Pathological priming causes developmental gene network heterochronicity in autistic subject-derived neurons. Nat. Neurosci. 22, 243–255. doi: 10.1038/s41593-018-0295-x
Schiavone, S., Mhillaj, E., Neri, M., Morgese, M. G., Tucci, P., Bove, M., et al. (2017). Early loss of blood-brain barrier integrity precedes NOX2 elevation in the prefrontal cortex of an animal model of psychosis. Mol. Neurobiol. 54, 2031–2044. doi: 10.1007/s12035-016-9791-8
Segarra, M., Aburto, M. R., Cop, F., Llaó-Cid, C., Härtl, R., Damm, M., et al. (2018). Endothelial Dab1 signaling orchestrates neuro-glia-vessel communication in the central nervous system. Science 361:eaao2861. doi: 10.1126/science.aao2861
Segarra, M., Aburto, M. R., Hefendehl, J., and Acker-Palmer, A. (2019). Neurovascular interactions in the nervous system. Annu. Rev. Cell Dev. Biol. 35, 615–635. doi: 10.1146/annurev-cellbio-100818-125142
Sharma, A., Gokulchandran, N., Sane, H., Nagrajan, A., Paranjape, A., Kulkarni, P., et al. (2013). Autologous bone marrow mononuclear cell therapy for autism: An open label proof of concept study. Stem Cells Int. 2013:623875. doi: 10.1155/2013/623875
Shibuya, M. (2009). Brain angiogenesis in developmental and pathological processes: Therapeutic aspects of vascular endothelial growth factor. FEBS J. 276, 4636–4643. doi: 10.1111/j.1742-4658.2009.07175.x
Shiu, F. H., Wong, J. C., Yamamoto, T., Lala, T., Purcell, R. H., Owino, S., et al. (2022). Mice lacking full length Adgrb1 (Bai1) exhibit social deficits, increased seizure susceptibility, and altered brain development. Exp. Neurol. 351:113994. doi: 10.1016/j.expneurol.2022.113994
Simons, M., Gordon, E., and Claesson-Welsh, L. (2016). Mechanisms and regulation of endothelial VEGF receptor signalling. Nat. Rev. Mol. Cell Biol. 17, 611–625. doi: 10.1038/nrm.2016.87
Skogstrand, K., Hagen, C. M., Borbye-Lorenzen, N., Christiansen, M., Bybjerg-Grauholm, J., Bækvad-Hansen, M., et al. (2019). Reduced neonatal brain-derived neurotrophic factor is associated with autism spectrum disorders. Transl. Psychiatry 9:252. doi: 10.1038/s41398-019-0587-2
Socała, K., Doboszewska, U., Szopa, A., Serefko, A., Włodarczyk, M., Zielińska, A., et al. (2021). The role of microbiota-gut-brain axis in neuropsychiatric and neurological disorders. Pharmacol. Res. 172:105840. doi: 10.1016/j.phrs.2021.105840
Stenman, J. M., Rajagopal, J., Carroll, T. J., Ishibashi, M., McMahon, J., and McMahon, A. P. (2008). Canonical Wnt signaling regulates organ-specific assembly and differentiation of CNS vasculature. Science 322:1247–1250. doi: 10.1126/science.1164594
Stewart, P. A., and Wiley, M. J. (1981). Developing nervous tissue induces formation of blood-brain barrier characteristics in invading endothelial cells: A study using quail–chick transplantation chimeras. Dev. Biol. 84, 183–192. doi: 10.1016/0012-1606(81)90382-1
Stobart, J. L., and Anderson, C. M. (2013). Multifunctional role of astrocytes as gatekeepers of neuronal energy supply. Front. Cell. Neurosci. 7:38. doi: 10.3389/fncel.2013.00038
Sun, T., and Hevner, R. F. (2014). Growth and folding of the mammalian cerebral cortex: From molecules to malformations. Nat. Rev. Neurosci. 15, 217–232. doi: 10.1038/nrn3707
Suzuki, K., Sugihara, G., Ouchi, Y., Nakamura, K., Futatsubashi, M., Takebayashi, K., et al. (2013). Microglial activation in young adults with autism spectrum disorder. JAMA Psychiatry 70, 49–58. doi: 10.1001/jamapsychiatry.2013.272
Sweeney, M. D., Ayyadurai, S., and Zlokovic, B. V. (2016). Pericytes of the neurovascular unit: Key functions and signaling pathways. Nat. Neurosci. 19, 771–783. doi: 10.1038/nn.4288
Sweeney, M. D., Zhao, Z., Montagne, A., Nelson, A. R., and Zlokovic, B. V. (2019). Blood-brain barrier: From physiology to disease and back. Physiol. Rev. 99, 21–78. doi: 10.1152/physrev.00050.2017
Takahashi, H., and Shibuya, M. (2005). The vascular endothelial growth factor (VEGF)/VEGF receptor system and its role under physiological and pathological conditions. Clin. Sci. (Lond.) 109, 227–241. doi: 10.1042/cs20040370
Talaat, R., El-Sayed, W., Agwa, H. S., Gamal-Eldeen, A. M., Moawia, S., and Zahran, M. A. (2015). Anti-inflammatory effect of thalidomide dithiocarbamate and dithioate analogs. Chem. Biol. Interact. 238, 74–81. doi: 10.1016/j.cbi.2015.05.017
Tam, S. J., and Watts, R. J. (2010). Connecting vascular and nervous system development: Angiogenesis and the blood-brain barrier. Annu. Rev. Neurosci. 33, 379–408.
Tărlungeanu, D. C., Deliu, E., Dotter, C. P., Kara, M., Janiesch, P. C., Scalise, M., et al. (2016). Impaired amino acid transport at the blood brain barrier is a cause of autism spectrum disorder. Cell 167, 1481–1494.e1418. doi: 10.1016/j.cell.2016.11.013
Tata, M., and Ruhrberg, C. (2018). Cross-talk between blood vessels and neural progenitors in the developing brain. Neuronal Signal. 2:NS20170139.
Tata, M., Wall, I., Joyce, A., Vieira, J. M., Kessaris, N., and Ruhrberg, C. (2016). Regulation of embryonic neurogenesis by germinal zone vasculature. Proc. Natl. Acad. Sci. U.S.A. 113, 13414–13419. doi: 10.1073/pnas.1613113113
Theoharides, T. C. (2013). Is a subtype of autism an allergy of the brain? Clin. Ther. 35, 584–591. doi: 10.1016/j.clinthera.2013.04.009
Tkach, M., and Théry, C. (2016). Communication by extracellular vesicles: Where we are and where we need to go. Cell 164, 1226–1232. doi: 10.1016/j.cell.2016.01.043
Tsuchiya, K. J., Hashimoto, K., Iwata, Y., Tsujii, M., Sekine, Y., Sugihara, G., et al. (2007). Decreased serum levels of platelet-endothelial adhesion molecule (PECAM-1) in subjects with high-functioning autism: A negative correlation with head circumference at birth. Biol. Psychiatry 62, 1056–1058. doi: 10.1016/j.biopsych.2006.12.018
Uratani, M., Ota, T., Iida, J., Okazaki, K., Yamamuro, K., Nakanishi, Y., et al. (2019). Reduced prefrontal hemodynamic response in pediatric autism spectrum disorder measured with near-infrared spectroscopy. Child Adolesc. Psychiatry Ment. Health 13:29. doi: 10.1186/s13034-019-0289-9
Vafadari, B., Salamian, A., and Kaczmarek, L. (2016). MMP-9 in translation: From molecule to brain physiology, pathology, and therapy. J. Neurochem. 139(Suppl. 2), 91–114. doi: 10.1111/jnc.13415
Vargas, D. L., Nascimbene, C., Krishnan, C., Zimmerman, A. W., and Pardo, C. A. (2005). Neuroglial activation and neuroinflammation in the brain of patients with autism. Ann. Neurol. 57, 67–81. doi: 10.1002/ana.20315
Vázquez-Rosa, E., Shin, M. K., Dhar, M., Chaubey, K., Cintrón-Pérez, C. J., Tang, X., et al. (2020). P7C3-A20 treatment one year after TBI in mice repairs the blood-brain barrier, arrests chronic neurodegeneration, and restores cognition. Proc. Natl. Acad. Sci. U.S.A. 117, 27667–27675. doi: 10.1073/pnas.2010430117
Voineagu, I., Wang, X., Johnston, P., Lowe, J. K., Tian, Y., Horvath, S., et al. (2011). Transcriptomic analysis of autistic brain reveals convergent molecular pathology. Nature 474, 380–384. doi: 10.1038/nature10110
Volterra, A., and Meldolesi, J. (2005). Astrocytes, from brain glue to communication elements: The revolution continues. Nat. Rev. Neurosci. 6, 626–640. doi: 10.1038/nrn1722
Wang, W., Su, L., Wang, Y., Li, C., Ji, F., and Jiao, J. (2022). Endothelial cells mediated by UCP2 control the neurogenic-to-astrogenic neural stem cells fate switch during brain development. Adv. Sci. (Weinh) 9:e2105208. doi: 10.1002/advs.202105208
Whiteus, C., Freitas, C., and Grutzendler, J. (2014). Perturbed neural activity disrupts cerebral angiogenesis during a postnatal critical period. Nature 505, 407–411. doi: 10.1038/nature12821
Winkler, E. A., Bell, R. D., and Zlokovic, B. V. (2011). Central nervous system pericytes in health and disease. Nat. Neurosci. 14, 1398–1405. doi: 10.1038/nn.2946
Wolburg, H., Wolburg-Buchholz, K., Fallier-Becker, P., Noell, S., and Mack, A. F. (2011). Structure and functions of aquaporin-4-based orthogonal arrays of particles. Int. Rev. Cell Mol. Biol. 287, 1–41. doi: 10.1016/b978-0-12-386043-9.00001-3
Xiao, T., Xiao, Z., Ke, X., Hong, S., Yang, H., Su, Y., et al. (2012). Response inhibition impairment in high functioning autism and attention deficit hyperactivity disorder: Evidence from near-infrared spectroscopy data. PLoS One 7:e46569. doi: 10.1371/journal.pone.0046569
Xu, B., Zhang, Y., Du, X. F., Li, J., Zi, H. X., Bu, J. W., et al. (2017). Neurons secrete miR-132-containing exosomes to regulate brain vascular integrity. Cell Res. 27, 882–897. doi: 10.1038/cr.2017.62
Xu, G., Strathearn, L., Liu, B., O’Brien, M., Kopelman, T. G., Zhu, J., et al. (2019). Prevalence and treatment patterns of autism spectrum disorder in the United States, 2016. JAMA Pediatr. 173, 153–159. doi: 10.1001/jamapediatrics.2018.4208
Yan, W., Rangaprakash, D., and Deshpande, G. (2018). Aberrant hemodynamic responses in autism: Implications for resting state fMRI functional connectivity studies. Neuroimage Clin. 19, 320–330. doi: 10.1016/j.nicl.2018.04.013
Yao, Y., Walsh, W. J., McGinnis, W. R., and Praticò, D. (2006). Altered vascular phenotype in autism: Correlation with oxidative stress. Arch. Neurol. 63, 1161–1164. doi: 10.1001/archneur.63.8.1161
Yao, Y., Zheng, X. R., Zhang, S. S., Wang, X., Yu, X. H., Tan, J. L., et al. (2016). Transplantation of vascular endothelial growth factor-modified neural stem/progenitor cells promotes the recovery of neurological function following hypoxic-ischemic brain damage. Neural Regen. Res. 11, 1456–1463. doi: 10.4103/1673-5374.191220
Yasuhara, T., Shingo, T., and Date, I. (2004). The potential role of vascular endothelial growth factor in the central nervous system. Rev. Neurosci. 15, 293–307. doi: 10.1515/revneuro.2004.15.4.293
Zawadzka, A., Cieślik, M., and Adamczyk, A. (2021). The role of maternal immune activation in the pathogenesis of autism: A review of the evidence, proposed mechanisms and implications for treatment. Int. J. Mol. Sci. 22:11516. doi: 10.3390/ijms222111516
Zehendner, C. M., Wedler, H. E., and Luhmann, H. J. (2013). A novel in vitro model to study pericytes in the neurovascular unit of the developing cortex. PLoS One 8:e81637. doi: 10.1371/journal.pone.0081637
Zengeler, K. E., and Lukens, J. R. (2021). Innate immunity at the crossroads of healthy brain maturation and neurodevelopmental disorders. Nat. Rev. Immunol. 21, 454–468. doi: 10.1038/s41577-020-00487-7
Zhan, Y., Paolicelli, R. C., Sforazzini, F., Weinhard, L., Bolasco, G., Pagani, F., et al. (2014). Deficient neuron-microglia signaling results in impaired functional brain connectivity and social behavior. Nat. Neurosci. 17, 400–406. doi: 10.1038/nn.3641
Zhou, Y., and Nathans, J. (2014). Gpr124 controls CNS angiogenesis and blood-brain barrier integrity by promoting ligand-specific canonical wnt signaling. Dev. Cell 31, 248–256. doi: 10.1016/j.devcel.2014.08.018
Zhu, L.-L., Wu, L.-Y., Yew, D. T., and Fan, M. (2005). Effects of hypoxia on the proliferation and differentiation of NSCs. Mol. Neurobiol. 31, 231–242.
Zimmerman, A. W., Jyonouchi, H., Comi, A. M., Connors, S. L., Milstien, S., Varsou, A., et al. (2005). Cerebrospinal fluid and serum markers of inflammation in autism. Pediatr. Neurol. 33, 195–201. doi: 10.1016/j.pediatrneurol.2005.03.014
Keywords: autism spectrum disorder (ASD), cerebrovascular, blood-brain barrier, neurovascular unit, neurovascular crosstalk
Citation: Wang Y, Yu S and Li M (2023) Neurovascular crosstalk and cerebrovascular alterations: an underestimated therapeutic target in autism spectrum disorders. Front. Cell. Neurosci. 17:1226580. doi: 10.3389/fncel.2023.1226580
Received: 21 May 2023; Accepted: 08 August 2023;
Published: 24 August 2023.
Edited by:
Jian Shen, Zhejiang University, ChinaReviewed by:
Maryam Ardalan, University of Gothenburg, SwedenEvelyne Gozal, University of Louisville, United States
Copyright © 2023 Wang, Yu and Li. This is an open-access article distributed under the terms of the Creative Commons Attribution License (CC BY). The use, distribution or reproduction in other forums is permitted, provided the original author(s) and the copyright owner(s) are credited and that the original publication in this journal is cited, in accordance with accepted academic practice. No use, distribution or reproduction is permitted which does not comply with these terms.
*Correspondence: Mengqian Li, bWVuZ3FpYW5saUBuY3UuZWR1LmNu