- Department of Neurobiology and Anatomy, Wake Forest School of Medicine, Winston-Salem, NC, United States
During early development, brains undergo profound changes in structure at the molecular, synaptic, cellular and circuit level. At the same time, brains need to perform adaptive function. How do structurally immature brains process information? How do brains perform stable and reliable function despite massive changes in structure? The rodent olfactory system presents an ideal model for approaching these poorly understood questions. Rodents are born deaf and blind, and rely completely on their sense of smell to acquire resources essential for survival during the first 2 weeks of life, such as food and warmth. Here, we review decades of work mapping structural changes in olfactory circuits during early development, as well as more recent studies performing in vivo electrophysiological recordings to characterize functional activity patterns generated by these circuits. The findings demonstrate that neonatal olfactory processing relies on an interacting network of brain areas including the olfactory bulb and piriform cortex. Circuits in these brain regions exhibit varying degrees of structural maturity in neonatal animals. However, despite substantial ongoing structural maturation of circuit elements, the neonatal olfactory system produces dynamic network-level activity patterns that are highly stable over protracted periods during development. We discuss how these findings inform future work aimed at elucidating the circuit-level mechanisms underlying information processing in the neonatal olfactory system, how they support unique neonatal behaviors, and how they transition between developmental stages.
Introduction
Brains undergo extensive structural changes on multiple time scales throughout life. This is particularly apparent during early development when neurons migrate, differentiate, change morphology, and form balanced excitatory and inhibitory connections (Tau and Peterson, 2010; Sarma et al., 2011). Eventually, this process establishes mature processing circuits that are the subject of study for the bulk of neuroscience research. However, long before reaching their adult state, brains are highly functional and capable of mediating adaptive behaviors in some domains. Thus, despite structural immaturity at the cellular, molecular and synaptic level, “immature” fails to capture certain functional capabilities of the developing brain. Moreover, whereas the extant literature has focused extensively on how brain structure develops from immature to mature, our understanding of how developing brains process information to mediate functional interactions with the environment in vivo is severely lagging (Avitan and Goodhill, 2018). We argue that the olfactory system presents an ideal model for studying information processing in the developing brain. In many species, sensory systems like vision and audition undergo postnatal development largely uncoupled from adaptive behavioral output (Mooney et al., 1996; Hanganu et al., 2006; Colonnese et al., 2010). For example, rodents—a major model system for studying the neural basis of sensory processing and behavior—remain deaf and blind during the first 2 weeks of life. Proper early life development of sensory processing capabilities in the visual and auditory systems relies on internally-generated spontaneous activity patterns (i.e., retinal and cochlear waves, respectively) (Katz and Shatz, 1996; Mooney et al., 1996; Weliky and Katz, 1999; Tritsch et al., 2007, 2010). The contribution of natural sensory input to circuit development in the visual system finds its onset after the second week of life, during the so-called critical period (Crair et al., 1998; Rochefort et al., 2009; Hoy and Niell, 2015; Hooks and Chen, 2020). In the olfactory system, early development and maintenance of proper connectivity also relies in part on spontaneously-generated activity patterns [(Yu et al., 2004; Lorenzon et al., 2015; Nakashima et al., 2019), reviewed in Redolfi and Lodovichi (2021)], suggesting a shared principle. However, unlike vision and audition, processing of external sensory input to the olfactory system is crucial for survival already at birth. Odor signals support the formation of bonds between pups and their caregivers, and guide behaviors that help pups acquire essential resources such as food and warmth (Landers and Sullivan, 2012).
Here, we review research on the structure and function of the neonatal olfactory system in rodents. Early work established the importance of olfaction for neonatal behavior and identified the brain regions involved in neonatal odor processing. Ongoing work at the molecular, cellular and synaptic level provides further insight into the local circuit motifs that characterize the neonatal olfactory network. Finally, recent studies started to characterize how these circuits interact to generate dynamic activity patterns that reflect sensory processing in vivo. The highlighted findings reveal the challenges of performing functional sensory processing in the structurally immature brain, and shed light on the strategies the brain has evolved to resolve these challenges at multiple levels of organization: from molecular to systems.
Unique odor-guided behavior in neonates
A long history of elegant behavioral work in rats illustrates the crucial role of olfaction for survival of neonatal rodents. The ability to learn about the olfactory environment starts already at prenatal stages. Rats learn preferences for both natural and artificial odorants experienced via the amniotic fluid and in the first hours after birth (Pedersen and Blass, 1982; Miller and Spear, 2008, 2009). Similar patterns of prenatal olfactory learning have been observed in human infants (Schaal et al., 2020). At the time of birth, rats are capable of using prenatally exposed odors to guide suckling behavior (Leon et al., 1977; Alberts and Brunjes, 1978; Galef and Kaner, 1980; Pedersen and Blass, 1982; Rosenblatt, 1983; Leon, 1992), and show increased head movements in the context of exposed odors (Miller and Spear, 2008). The importance of olfaction for survival during this early developmental period is underscored by work on mice rendered anosmic through transgenics. Anosmia drastically decreases pups’ survival chances in the first couple of days after birth as a result of impaired suckling behavior (Brunet et al., 1996; Contos et al., 2000). Unique olfactory learning and behavior continues during the first 2 weeks of life, when rats are completely dependent on their mother for survival. Pups learn preferences for arbitrary odors associated with maternal care, or signals that mimic maternal care (warmth, tactile stimulation, milk infusion), after only 10 min of exposure (Caza and Spear, 1984; Sullivan and Leon, 1987; Kraebel and Spear, 2000; Miller and Spear, 2009). Subsequent exposure to the same odor evoked orienting and approach behavior. Thus, behavioral work suggests a highly functional sense of smell in neonatal rodents—one that is uniquely adapted to the needs of the animal at the earliest postnatal developmental stages.
The brain network supporting olfaction during early life
To support neonatal odor-guided behaviors, a functional olfactory system emerges early in development. First order neurons in the olfactory epithelium (olfactory sensory neurons) innervate the olfactory bulb (OB) in anatomically segregated clusters called glomeruli and form functional synapses with mitral cells (the principal output neurons of the OB) prenatally (Hinds and Hinds, 1972; Mair and Gesteland, 1982; Malun and Brunjes, 1996; Treloar et al., 1999; Walz et al., 2006; Illig, 2007). The axons of mitral cells form the lateral olfactory tract (LOT) and innervate the piriform cortex by the time of birth (Schwob and Price, 1984; Walz et al., 2006). Thus, a primary olfactory circuit consisting of the OB and PCX that processes odor-driven input is established at the time of birth (Schwob et al., 1984; Illig, 2007; Zhang et al., 2020). Modulatory input to the OB-PCX circuit comes from several brainstem nuclei, including the locus coeruleus (LC) and Raphe nuclei, which release the neuromodulators norepinephrine (NE) and serotonin (5-HT), respectively. Figure 1 shows a schematic of the neonatal olfactory circuit and its main bottom-up and top-down input sources.
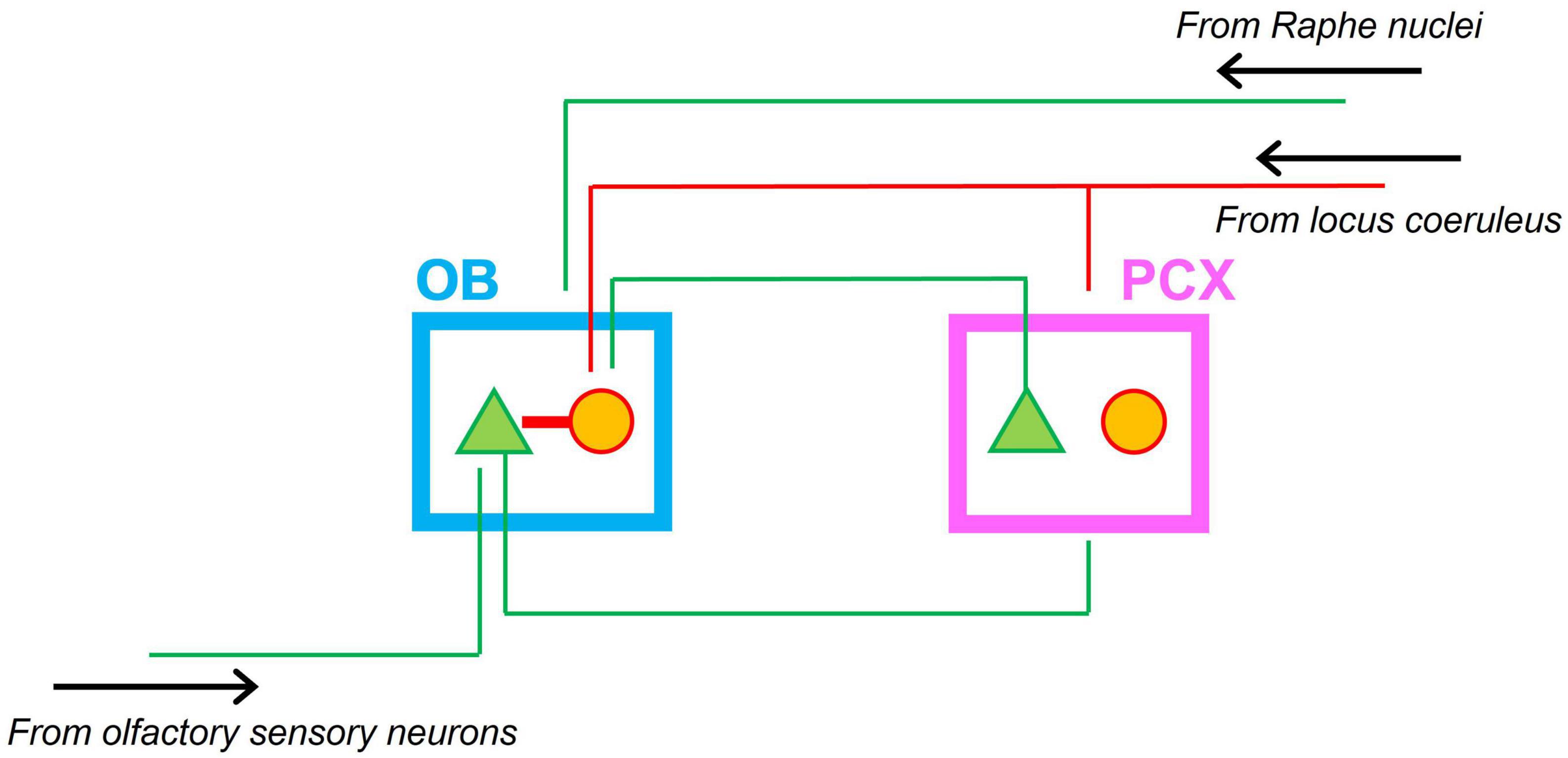
Figure 1. Schematic of the neonatal olfactory circuit. Afferent input from olfactory sensory neurons in the nasal epithelium targets mitral cells in the olfactory bulb (OB). Mitral cells in turn project to the piriform olfactory cortex (PCX). Feedback from the PCX targets granule cells in the OB. Granule cells in turn inhibit mitral cells in the OB. Neonatal interneurons in the PCX do not form functional inhibitory synapses. Both OB and PCX receive modulatory noradrenergic input from the locus coeruleus. Green triangles indicate excitatory projection neurons; red circles indicate interneurons. Green lines indicate excitatory projections; red lines indicate inhibitory projections. The OB also receives excitatory inputs from the Raphe nuclei (not shown).
All regions in the network depicted in Figure 1 are involved in neonatal olfaction. Exposure to an artificial odor in the context of (signals associated with) maternal care produces selective changes in responsiveness of both OB (Coopersmith and Leon, 1984; Wilson et al., 1985, 1987; Wilson and Leon, 1988) and PCX (Roth and Sullivan, 2005; Morrison et al., 2013; Mukherjee et al., 2014) neurons, as measured by metabolic markers, in vitro recordings, and single unit recordings in anesthetized animals. Modulatory systems play an important role in driving plastic changes in the olfactory circuit. Blocking NE receptors in the OB (Sullivan et al., 1992) impairs neonatal preference learning, and so does lesioning the LC (Sullivan et al., 1994). Depletion of 5-HT in the OB has a comparable effect on odor preference learning (McLean et al., 1993). Moreover, pairing an odor stimulus with direct stimulation of the LC is sufficient to instill preferences for the paired odor (Sullivan et al., 2000). Similarly, infusion of the adrenergic antagonist propranolol to the PCX impairs preference learning for an odor stimulus in the context of signals mimicking maternal care; infusion of the adrenergic agonist isoproterenol induces a preference for a concurrent odor stimulus in the absence of signals mimicking maternal care.
Circuit-level mechanisms underlying neonatal olfactory processing
Although circuit-level function in the neonatal olfactory system remains poorly understood, the extant literature suggests a set of unique sensory processing mechanisms that rely on a combination of mature and immature circuit motifs. Much of the work on the mechanisms underlying neonatal olfactory processing has focused on cellular, molecular and synaptic characteristics of circuit motifs locally within the OB and PCX.
The neonatal OB exhibits some remarkably mature features during the first week of postnatal life, most notably the presence of functional inhibitory synapses (Wilson and Leon, 1986; Wang et al., 2005; Mack-Bucher et al., 2007; Dietz et al., 2011). Early anatomical studies noted the presence of granule cells—the main inhibitory cell type in the OB (Hinds, 1968; Mair et al., 1982)—at birth. However, the vast majority of granule cells are of postnatal origin [∼90%, (Rosselli-Austin and Altman, 1979)], and numbers steadily increase until the third week of life (Hinds, 1968). Despite the low numbers of granule cells, subsequent work employing extracellular recordings in the OB of anesthetized neonatal rats showed that mitral cells show a phase of strong inhibition in response to antidromic electrical stimulation of the LOT (Wilson and Leon, 1986, 1987), and suggested that this inhibition is mediated via reciprocal mitral cell-granule cell synapses. This idea was supported by recent molecular and in vitro work demonstrating that GABA-mediated inhibition develops more quickly in the OB compared to the rest of the brain (Wang et al., 2005; Mack-Bucher et al., 2007; Dietz et al., 2011). Due to low expression levels of the potassium chloride co-transporter KCC2 in neonatal neurons, intracellular chloride concentrations are generally high, and GABA has a depolarizing, excitatory effect on postsynaptic cells. This phenomenon is widely observed in the neocortex and hippocampus of rats up to around P8 (Ben-Ari et al., 1989; Cherubini et al., 1991). In contrast, KCC2 levels in the neonatal OB are closer to adult levels, allowing for functional inhibition in the circuit (Wang et al., 2005).
In adults, granule cell-mediated inhibition plays a key role on in shaping odor representations in the OB through lateral inhibition (Schoppa and Urban, 2003). Granule cells are also the main recipient of centrifugal and modulatory projections in the adult (Price and Powell, 1970), which have been shown to affect odor discriminability (Otazu et al., 2015). In neonatal animals, feedback projections from the PCX (Schwob and Price, 1984) and LC (McLean and Shipley, 1991) mainly target the granule cell layer. Findings regarding the effect of adrenergic modulators on mitral cell excitability are consistent with the idea that modulatory inputs to the OB target inhibitory granule cells, thereby modulating OB output. It is currently unclear how inhibition in the neonatal OB affects odor representations. Future behavioral work will characterize to what extent neonatal animals are able to discriminate and/or generalize between odors, and what the role of cortical feedback and inhibition is. The observation that inhibition in the neonatal OB is stronger than in the adult suggests a unique adaptation to the neonatal state: enabling fast and robust learning of maternal odors by creating highly specific odor representations. Mechanistically, enhanced inhibition may be accomplished by dense feedback projections, since the number of granule cells is low in neonates.
Compared to the neonatal OB, inhibition in the PCX remains immature until the third week of life. In line with developmental dynamics previously observed in the neocortex and hippocampus, GABA has a depolarizing effect on postsynaptic PCX neurons until at least P8 (Pardo et al., 2018). Despite the lack of inhibition, PCX plays an important role in mediating neonatal odor preference learning, as reviewed above. In vitro work suggests a unique excitatory mechanism underlying experience-dependent plasticity in the PCX. Prior to maturation of the local piriform cortical circuit, feedforward OB→PCX synapses are highly plastic and rely on NMDA receptors (Franks and Isaacson, 2005). After the second week of life, the strength of NMDA-mediated OB→PCX synapse plasticity declines and makes way for mature intra-cortical plasticity involving AMPA receptors (Poo and Isaacson, 2007) and balanced excitatory/inhibitory synapses (Canto-Bustos et al., 2022).
Dynamic activity patterns in the neonatal olfactory system in vivo
The studies reviewed above demonstrate that the neonatal olfactory system features both mature and immature local circuit motifs that support unique neonatal olfactory function. However, it remains largely unknown how these circuits interact to process odor input in awake behaving animals. In adult rodents, processing of odor inputs in awake behaving animals is characterized by coherent oscillatory activity at multiple spatial scales across the olfactory network (Kay et al., 2009). To gain insight into how neonatal circuits function to generate dynamic neural activity patterns reflective of information processing in vivo, a recent study applied electrophysiological recordings in awake rat pups during the first 3 weeks of life while they actively sampled odor stimuli (Zhang et al., 2020). Figure 2A shows traces of local field potential activity recorded simultaneously from the OB and PCX at P5. Orthonasal presentation of an odor stimulus evoked bursts of 10–20 Hz oscillatory activity with each inhalation in both OB and PCX. Oscillatory activity is apparent as a distinct peak in the frequency representation of the local field potential shown in Figure 2B. Oscillations in the 10–20 Hz range were present at birth (see Figure 3A), and similar oscillations were observed in a different study that recorded from the OB of anesthetized rat pups at P7 (Fletcher et al., 2005). Neonatal oscillations are phenomenologically analogous to beta frequency (20–30 Hz) oscillations observed in the olfactory system of adult rodents (Gray and Skinner, 1988; Kay and Freeman, 1998; Martin et al., 2004, 2006; David et al., 2015; Frederick et al., 2016). Both types of oscillations are evoked by odor stimuli, locked to the respiration cycle, coherent across the OB and PCX, and entrain cortical spiking activity. However, the underlying circuit and functional significance of neonatal oscillations remain unknown.
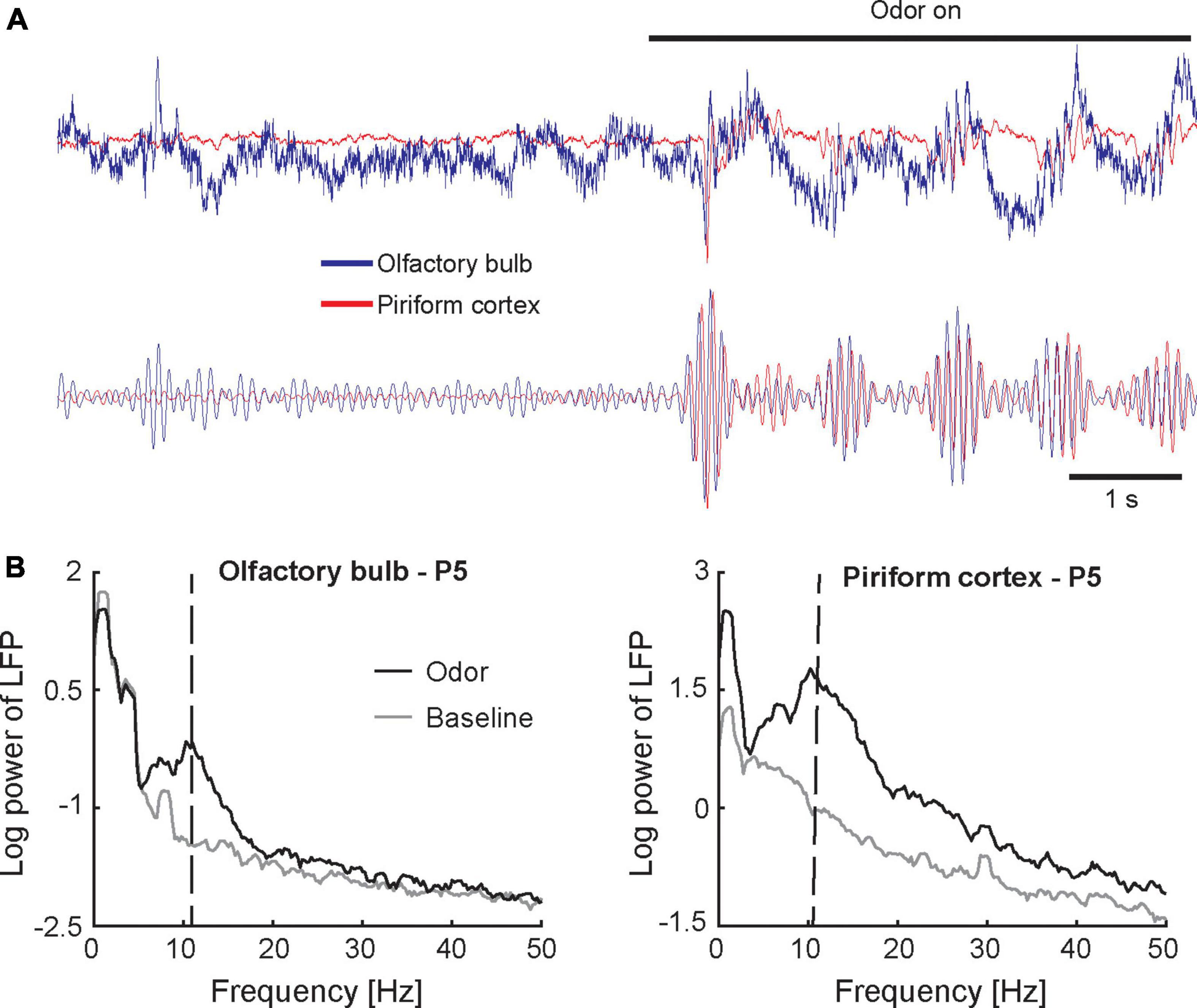
Figure 2. Oscillatory activity in the neonatal olfactory network. (A) Traces represent the raw (upper panel) and band-pass filtered (lower panel) local field potential recorded simultaneously from the OB (blue) and PCX (red) in a 5-day old awake rat pup (single trial). Inhaling an odor stimulus (amyl acetate; presence of odor stimulus is indicated by the horizontal black bar) elicits a burst of oscillations in the 10–20 Hz frequency range. Oscillations are highly coherent across the OB and PCX. Slight time lag of the PCX signal relative to the OB signal indicates that oscillations originate in the OB. (B) Frequency-amplitude representations (spectra) of the local field potential recorded simultaneously from the olfactory bulb (left panel) and piriform cortex (right panel) in a 5-day old awake rat pup. Spectra are based on 10 odor presentations. The peak around 1 Hz indicates respiration-induced modulation. Vertical dashed lines in panel (B) indicate peak frequency of odor-evoked activity. Figures adapted from Zhang et al. (2020).
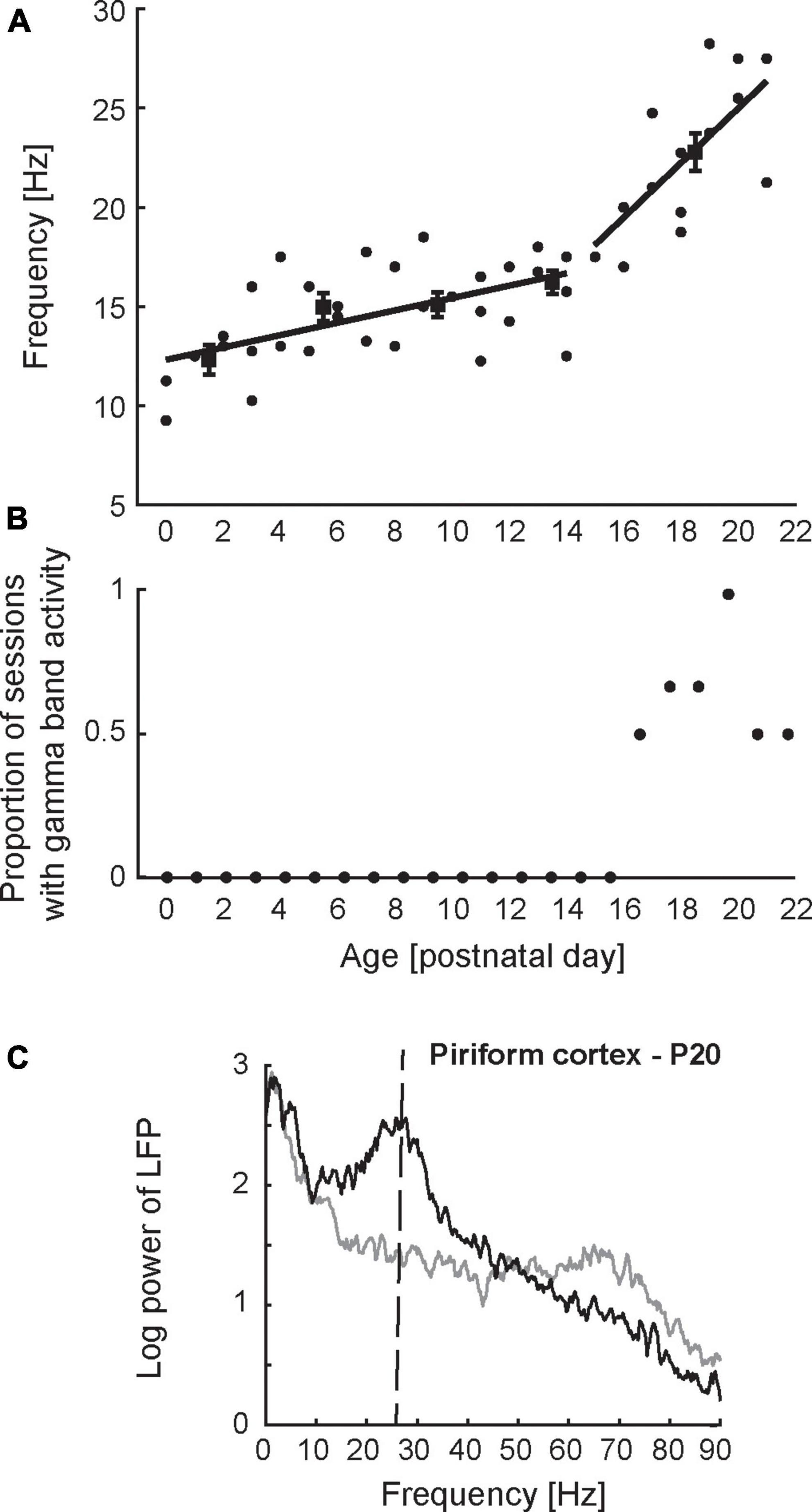
Figure 3. Developmental dynamics of network-level activity in the piriform cortex. (A) Peak frequency of odor-evoked activity in PCX as a function of age. Each datapoint represents a single recording session from a unique animal. Peak frequency was determined based on the spectrum obtained from the local field potential recorded during odor presentation (n = 10 trials). Lines represent the best piecewise linear fit to the data. (B) Proportion of recording sessions exhibiting spontaneous gamma-band oscillations (60–90 Hz) in the PCX as a function of age. The presence of gamma-band oscillations was determined based on the spectrum obtained from the local field potential recorded before stimulus presentation (n = 10 trials/session). Gamma band oscillations were not observed in the PCX prior to postnatal day 16. (C) Spectrum of local field potential activity recorded from the PCX in a 20-day old rat pup. Mature network activity in the PCX emerges after P15 and is characterized by odor-evoked beta oscillations (20–30 Hz) and spontaneous gamma oscillations (60–90 Hz). Vertical dashed line in panel (C) indicates peak frequency of odor-evoked activity. Figures adapted from Zhang et al. (2020).
In adult rodents, two major types of oscillations that have been implicated in odor processing are beta and gamma oscillations (Kay et al., 2009). Both oscillations are generated in the OB, but their expression is modulated by cortical feedback projections (Gray and Skinner, 1988; Martin et al., 2004, 2006; David et al., 2015). As laid out above, cortical feedback in adults targets inhibitory granule cells (Price and Powell, 1970), which form reciprocal synapses with mitral cells (Strowbridge, 2009), thereby controlling excitability. In the presence of odor input—when cortical feedback is strong—mitral cells are in a low-excitability state and the OB and PCX exhibit coherent beta oscillations. In the absence of odor stimuli—when cortical feedback is weak—mitral cells are in a high-excitability state and exhibit gamma frequency oscillations. Functionally, beta oscillations reflect long-range functional interactions between the OB and PCX necessary for olfactory learning (Martin et al., 2004). Gamma oscillations on the other hand reflect local interactions between mitral and granule cells, and aid in fine discrimination between bulbar odor representations (Beshel et al., 2007; Martin and Ravel, 2014; Frederick et al., 2016). In the neonatal olfactory system, 10–20 Hz oscillations also originate from the OB (Zhang et al., 2020). Moreover, the work reviewed above shows that the neonatal OB receives cortical feedback projections and features inhibitory mitral-granule cell synapses. This leaves open the possibility that the neonatal OB can generate oscillations that exceed the 10–20 Hz range. Regarding beta (20–30 Hz) oscillations, one possibility is that cortical feedback (and as a result: inhibition in the OB) is particularly strong in neonates, suppressing oscillations to a low (10–20 Hz) frequency range. This may be adaptive in allowing functional interactions of the relatively mature OB with a relatively immature PCX that is incapable of sustaining beta frequency oscillations. Another possibility (not mutually exclusive with the former) is that the neonatal OB can switch between long-range (10–20 Hz) and local scale (gamma) processing modes depending on context. Alternatively, some aspects of local mitral-granule cell circuitry may not yet have fully matured in neonates, preventing the expression of gamma oscillations. To date, gamma oscillations have not been observed in neonatal animals, but future work combining in vivo recordings and circuit-breaking techniques in awake behaving neonatal animals will test the context-dependence of neonatal oscillatory activity, and the role of cortical feedback.
A third network-level activity pattern that occurs in the olfactory system in vivo are oscillations in the theta frequency range (8–12 Hz). Like beta oscillations, theta oscillations reflect long-range functional interactions, and have been observed across olfactory and limbic areas such as the entorhinal cortex and hippocampus in adult rats (Kay, 2005). A recent study in neonatal mouse pups demonstrated coherent theta oscillations across the OB and entorhinal cortex (Gretenkord et al., 2019). In general, functional interactions between olfactory and limbic circuits may facilitate learning and memory. Additionally, the authors speculate that activity patterns originating from the OB may affect the maturation of downstream systems. Given the accelerated developmental profile of the OB, a relatively mature OB that continuously generates structured activity patterns in response to external input may play an instructional or permissive role in the development of connected systems. Indeed, Gretenkord et al. (2019) showed that experimentally-induced anosmia during early life led to immediate loss of coherent oscillatory activity between the OB and entorhinal cortex, and to long-term disruption of neural activity patterns within the entorhinal cortex.
Developmental changes in olfactory circuit function
Few studies have tracked in vivo neural activity patterns in the olfactory system [or any other sensory system for that matter, see Colonnese et al. (2010) for an exceptional rare example] across early development, and it remains largely unknown how the system transitions from the neonatal state described above to a mature state. Major developmental milestones occur during the third week of life, including eye opening and the onset of autonomous exploratory behavior (Bolles and Woods, 1964; Welker, 1964; Zhang et al., 2020). Roughly aligned with these milestones, Zhang et al. (2020) observed profound changes in in vivo network-level activity pattern. Figure 3A shows peak frequency of neonatal odor-evoked oscillations as a function of age. Prior to P15, olfactory network oscillations in response to odor stimuli are remarkably stable, consisting of the 10–20 Hz oscillations described above. After P15, the frequency of odor-evoked oscillations undergoes an abrupt increase from 10–20 Hz to mature beta frequency range (20–30 Hz). Aligned with this rapid increase in odor-evoked oscillations is the appearance of mature spontaneous gamma oscillations (40–90 Hz) in the PCX (Figure 3B). Figure 3C shows the spectrum of mature network-level activity in the PCX, characterized by odor-evoked beta and spontaneous gamma oscillations. Future work will test whether the appearance of gamma oscillations in PCX occurs in parallel with the OB or whether the two brain regions follow independent developmental time courses.
Changes in network-level activity patterns are certainly the result of changes in brain structure. However, developmental changes in structure and function appear to follow divergent dynamics. Whereas network activity patterns recorded in vivo are stable for protracted periods of development and undergo abrupt changes, maturation at the molecular, cellular and synaptic level typically progresses continuously. In the OB, the number of glomeruli gradually increases (LaMantia and Purves, 1989), and mitral cells undergo gradual changes in biophysical properties during the first 3 weeks of life (Yu et al., 2015). The number of granule cells also gradually increases during the first 2 weeks of life (Hinds, 1968; Mair et al., 1982), paralleled by an increase in inhibitory synapse strength, and followed by a decrease in inhibition after 2 weeks (Wilson and Leon, 1986, 1987; Dietz et al., 2011). In the PCX, myelination of the lateral olfactory tract (which carries the afferent axons from mitral cells to the PCX) (Schwob et al., 1984) and anterior commissure (which connects the PCX across the two hemispheres) (Martin-Lopez et al., 2018) reaches adult levels by 2 weeks of life. Pyramidal cells continue to differentiate and undergo gradual changes in morphology during the first 3 weeks of life (Poo and Isaacson, 2007; Sarma et al., 2011; Oruro et al., 2020). The number of inhibitory neurons in the PCX decreases during the first 2 weeks, followed by an increase in the number of inhibitory synapses after 2 weeks of life (Schwob et al., 1984; Westenbroek et al., 1988; Sarma et al., 2011; Pardo et al., 2018).
It remains unclear how network-level activity remains stable during the first 2 weeks despite profound, gradual structural changes. Previous work on the developing visual system suggests that abrupt developmental changes in network-level activity patterns can result from dynamic interactions between gradually maturing circuit elements (Romagnoni et al., 2020). Abrupt changes in network-level activity patterns would ensure stable function during early developmental stages while incorporating new functionality in preparation for the next developmental stage. Indeed, developmental changes in network-level activity in the visual system occur abruptly and in a coordinated manner around the time of eye opening (Golshani et al., 2009; Rochefort et al., 2009; Colonnese et al., 2010; Colonnese, 2014; Hoy and Niell, 2015). Another unanswered question is whether abrupt developmental changes in olfactory function are driven by internal factors, or dependent on sensory experience. Relevant in this respect is the potential role inhibitory synapse maturation plays in this process. As reviewed above, both the OB and PCX undergo substantial changes in the strength of inhibitory synapses after 2 weeks of life: the OB shows a marked decrease in inhibition starting at P15; the PCX a marked increase around the same time. A role for the maturation of inhibition in shaping network-level function is supported by findings regarding the development of oscillatory activity in the PCX. The increase in inhibitory synapse density in the PCX after P15 parallels the temporal profile of rapid increases in oscillation frequency from 10–20 Hz to beta frequency (20–30 Hz), as well as the emergence of gamma oscillations (Figure 3B). Both beta and gamma oscillations in the PCX critically rely on balanced intracortical excitatory-inhibitory interactions (Poo and Isaacson, 2009; Gonzalez et al., 2023). The finding that beta and gamma oscillations in the PCX emerge simultaneously (Figures 3A, B) further suggests that maturation of inhibition may serve as a common factor driving these developmental processes in a coordinated manner. The idea that maturation of inhibition shapes developmental changes in network-level activity is also consistent with previous work in neocortical sensory systems, and some of these studies indicate a role of sensory experience in shaping the maturation of inhibition in cortical circuits (Miao et al., 2016; Guan et al., 2017; Tatti et al., 2017). Future work using sensory deprivation protocols will elucidate the role of experience in the development of information processing in the olfactory system.
Conclusion
The research reviewed above demonstrates that the neonatal olfactory system uses unique, developmentally-transient processing mechanisms that are adapted to immature structural features, yet perform age-appropriate function. In vitro preparations and work in anesthetized animals continue to provide mechanistic insight into how various components of the neonatal olfactory circuit function at the molecular, cellular and synaptic level. In addition, recent efforts to studying neonatal olfactory processing using in vivo techniques in awake animals is starting to gain unique insight into how components of the neonatal olfactory circuit interact to generate network-level activity patterns in response to odor stimuli. We also highlight that network-level function exhibits unique developmental dynamics across age, and that network-level activity can provide insight into how the olfactory system may affect the development of extra-olfactory circuits. Future work will combine phenomenological observation of network-level activity with controlled optogenetic and pharmacological manipulations to gain mechanistic understanding of the relation between molecular, cellular, and synaptic processes and network-level function. Finally, placing in vivo preparations in different environmental contexts will provide a detailed account of how neural function at different levels of description supports neonatal olfactory perception and behavior.
Author contributions
JM prepared draft of the text and figures and contributed to the ideas. ZZ edited the text, drafted figures, and contributed to the ideas. Both authors contributed to the article and approved the submitted version.
Funding
This work was supported by NIH R01 DC016063 and DC020212 (to JM).
Conflict of interest
The authors declare that the research was conducted in the absence of any commercial or financial relationships that could be construed as a potential conflict of interest.
Publisher’s note
All claims expressed in this article are solely those of the authors and do not necessarily represent those of their affiliated organizations, or those of the publisher, the editors and the reviewers. Any product that may be evaluated in this article, or claim that may be made by its manufacturer, is not guaranteed or endorsed by the publisher.
References
Alberts, J. R., and Brunjes, P. C. (1978). Ontogeny of thermal and olfactory determinants of huddling in the rat. J. Comp. Physiol. Psychol. 92, 897–906. doi: 10.1037/h0077533
Avitan, L., and Goodhill, G. J. (2018). Code under construction: Neural coding over development. Trends Neurosci. 41, 599–609. doi: 10.1016/j.tins.2018.05.011
Ben-Ari, Y., Cherubini, E., Corradetti, R., and Gaiarsa, J. L. (1989). Giant synaptic potentials in immature rat CA3 hippocampal neurones. J. Physiol. 416, 303–325. doi: 10.1113/jphysiol.1989.sp017762
Beshel, J., Kopell, N., and Kay, L. M. (2007). Olfactory bulb gamma oscillations are enhanced with task demands. J. Neurosci. 27, 8358–8365. doi: 10.1523/JNEUROSCI.1199-07.2007
Bolles, R., and Woods, P. (1964). Ontogeny of behaviour in albino rat. Anim. Behav. 12, 427–441. doi: 10.1016/0003-3472(64)90062-4
Brunet, L. J., Gold, G. H., and Ngai, J. (1996). General anosmia caused by a targeted disruption of the mouse olfactory cyclic nucleotide-gated cation channel. Neuron 17, 681–693. doi: 10.1016/s0896-6273(00)80200-7
Canto-Bustos, M., Friason, F. K., Bassi, C., and Oswald, A. M. (2022). Disinhibitory circuitry gates associative synaptic plasticity in olfactory cortex. J. Neurosci. 42, 2942–2950. doi: 10.1523/JNEUROSCI.1369-21.2021
Caza, P., and Spear, N. (1984). Short-term exposure to an odor increases its subsequent preference in preweanling rats - a descriptive profile of the phenomenon. Dev. Psychobiol. 17, 407–422. doi: 10.1002/dev.420170407
Cherubini, E., Gaiarsa, J. L., and Ben-Ari, Y. (1991). GABA: An excitatory transmitter in early postnatal life. Trends Neurosci. 14, 515–519. doi: 10.1016/0166-2236(91)90003-d
Colonnese, M. T. (2014). Rapid developmental emergence of stable depolarization during wakefulness by inhibitory balancing of cortical network excitability. J. Neurosci. 34, 5477–5485. doi: 10.1523/JNEUROSCI.3659-13.2014
Colonnese, M. T., Kaminska, A., Minlebaev, M., Milh, M., Bloem, B., Lescure, S., et al. (2010). A conserved switch in sensory processing prepares developing neocortex for vision. Neuron 67, 480–498. doi: 10.1016/j.neuron.2010.07.015
Contos, J. J., Fukushima, N., Weiner, J. A., Kaushal, D., and Chun, J. (2000). Requirement for the lpA1 lysophosphatidic acid receptor gene in normal suckling behavior. Proc. Natl. Acad. Sci. U.S.A. 97, 13384–13389. doi: 10.1073/pnas.97.24.13384
Coopersmith, R., and Leon, M. (1984). Enhanced neural response to familiar olfactory cues. Science 225, 849–851. doi: 10.1126/science.6474157
Crair, M. C., Gillespie, D. C., and Stryker, M. P. (1998). The role of visual experience in the development of columns in cat visual cortex. Science 279, 566–570. doi: 10.1126/science.279.5350.566
David, F., Courtiol, E., Buonviso, N., and Fourcaud-Trocmé, N. (2015). Competing mechanisms of gamma and beta oscillations in the olfactory bulb based on multimodal inhibition of mitral cells over a respiratory cycle. eNeuro 2:ENEURO.0018-15.2015. doi: 10.1523/ENEURO.0018-15.2015
Dietz, S. B., Markopoulos, F., and Murthy, V. N. (2011). Postnatal development of dendrodendritic inhibition in the Mammalian olfactory bulb. Front. Cell. Neurosci. 5:10. doi: 10.3389/fncel.2011.00010
Fletcher, M. L., Smith, A. M., Best, A. R., and Wilson, D. A. (2005). High-frequency oscillations are not necessary for simple olfactory discriminations in young rats. J. Neurosci. 25, 792–798. doi: 10.1523/JNEUROSCI.4673-04.2005
Franks, K. M., and Isaacson, J. S. (2005). Synapse-specific downregulation of NMDA receptors by early experience: A critical period for plasticity of sensory input to olfactory cortex. Neuron 47, 101–114. doi: 10.1016/j.neuron.2005.05.024
Frederick, D., Brown, A., Brim, E., Mehta, N., Vujovic, M., and Kay, L. (2016). Gamma and beta oscillations define a sequence of neurocognitive modes present in odor processing. J. Neurosci. 36, 7750–7767. doi: 10.1523/JNEUROSCI.0569-16.2016
Galef, B. G., and Kaner, H. C. (1980). Establishment and maintenance of preference for natural and artificial olfactory stimuli in juvenile rats. J. Comp. Physiol. Psychol. 94, 588–595. doi: 10.1037/h0077693
Golshani, P., Gonçalves, J. T., Khoshkhoo, S., Mostany, R., Smirnakis, S., and Portera-Cailliau, C. (2009). Internally mediated developmental desynchronization of neocortical network activity. J. Neurosci. 29, 10890–10899. doi: 10.1523/JNEUROSCI.2012-09.2009
Gonzalez, J., Torterolo, P., and Tort, A. B. L. (2023). Mechanisms and functions of respiration-driven gamma oscillations in the primary olfactory cortex. Elife 12:e83044. doi: 10.7554/eLife.83044
Gray, C. M., and Skinner, J. E. (1988). Centrifugal regulation of neuronal activity in the olfactory bulb of the waking rabbit as revealed by reversible cryogenic blockade. Exp. Brain Res. 69, 378–386. doi: 10.1007/BF00247583
Gretenkord, S., Kostka, J. K., Hartung, H., Watznauer, K., Fleck, D., Minier-Toribio, A., et al. (2019). Coordinated electrical activity in the olfactory bulb gates the oscillatory entrainment of entorhinal networks in neonatal mice. PLoS Biol. 17:e2006994. doi: 10.1371/journal.pbio.2006994
Guan, W., Cao, J. W., Liu, L. Y., Zhao, Z. H., Fu, Y., and Yu, Y. C. (2017). Eye opening differentially modulates inhibitory synaptic transmission in the developing visual cortex. Elife 6:e32337. doi: 10.7554/eLife.32337
Hanganu, I., Ben-Ari, Y., and Khazipov, R. (2006). Retinal waves trigger spindle bursts in the neonatal rat visual cortex. J. Neurosci. 26, 6728–6736. doi: 10.1523/JNEUROSCI.0752-06.2006
Hinds, J. (1968). Autoradiographic study of histogenesis in mouse olfactory bulb .i. time of origin of neurons and neuroglia. J. Comp. Neurol. 134, 287–304. doi: 10.1002/cne.901340304
Hinds, J. W., and Hinds, P. L. (1972). Reconstruction of dendritic growth cones in neonatal mouse olfactory bulb. J. Neurocytol. 1, 169–187. doi: 10.1007/BF01099183
Hooks, B. M., and Chen, C. (2020). Circuitry underlying experience-dependent plasticity in the mouse visual system. Neuron 106, 21–36. doi: 10.1016/j.neuron.2020.01.031
Hoy, J. L., and Niell, C. M. (2015). Layer-specific refinement of visual cortex function after eye opening in the awake mouse. J. Neurosci. 35, 3370–3383. doi: 10.1523/JNEUROSCI.3174-14.2015
Illig, K. R. (2007). Developmental changes in odor-evoked activity in rat piriform cortex. Neuroscience 145, 370–376. doi: 10.1016/j.neuroscience.2006.11.049
Katz, L. C., and Shatz, C. J. (1996). Synaptic activity and the construction of cortical circuits. Science 274, 1133–1138. doi: 10.1126/science.274.5290.1133
Kay, L. M. (2005). Theta oscillations and sensorimotor performance. Proc. Natl. Acad. Sci. U.S.A. 102, 3863–3868. doi: 10.1073/pnas.0407920102
Kay, L. M., and Freeman, W. J. (1998). Bidirectional processing in the olfactory-limbic axis during olfactory behavior. Behav. Neurosci. 112, 541–553. doi: 10.1037//0735-7044.112.3.541
Kay, L., Beshel, J., Brea, J., Martin, C., Rojas-Libano, D., and Kopell, N. (2009). Olfactory oscillations: The what, how and what for. Trends Neurosci. 32, 207–214. doi: 10.1016/j.tins.2008.11.008
Kraebel, K. S., and Spear, N. E. (2000). Infant rats are more likely than adolescents to orient differentially to amodal (intensity-based) features of single-element and compound stimuli. Dev. Psychobiol. 36, 49–66.
LaMantia, A. S., and Purves, D. (1989). Development of glomerular pattern visualized in the olfactory bulbs of living mice. Nature 341, 646–649. doi: 10.1038/341646a0
Landers, M. S., and Sullivan, R. M. (2012). The development and neurobiology of infant attachment and fear. Dev. Neurosci. 34, 101–114. doi: 10.1159/000336732
Leon, M. (1992). Neuroethology of olfactory preference development. J. Neurobiol. 23, 1557–1573. doi: 10.1002/neu.480231012
Leon, M., Galef, B., and Behse, J. (1977). Establishment of pheromonal bonds and diet choice in young-rats by odor pre-exposure. Physiol. Behav. 18, 387–391. doi: 10.1016/0031-9384(77)90248-7
Lorenzon, P., Redolfi, N., Podolsky, M. J., Zamparo, I., Franchi, S. A., Pietra, G., et al. (2015). Circuit formation and function in the olfactory bulb of mice with reduced spontaneous afferent activity. J. Neurosci. 35, 146–160. doi: 10.1523/JNEUROSCI.0613-14.2015
Mack-Bucher, J. A., Li, J., and Friedrich, R. W. (2007). Early functional development of interneurons in the zebrafish olfactory bulb. Eur. J. Neurosci. 25, 460–470. doi: 10.1111/j.1460-9568.2006.05290.x
Mair, R. G., and Gesteland, R. C. (1982). Response properties of mitral cells in the olfactory bulb of the neonatal rat. Neuroscience 7, 3117–3125. doi: 10.1016/0306-4522(82)90234-2
Mair, R. G., Gellman, R. L., and Gesteland, R. C. (1982). Postnatal proliferation and maturation of olfactory bulb neurons in the rat. Neuroscience 7, 3105–3116. doi: 10.1016/0306-4522(82)90233-0
Malun, D., and Brunjes, P. C. (1996). Development of olfactory glomeruli: Temporal and spatial interactions between olfactory receptor axons and mitral cells in opossums and rats. J. Comp. Neurol. 368, 1–16.
Martin, C., and Ravel, N. (2014). Beta and gamma oscillatory activities associated with olfactory memory tasks: Different rhythms for different functional networks? Front. Behav. Neurosci. 8:218. doi: 10.3389/fnbeh.2014.00218
Martin, C., Gervais, R., Hugues, E., Messaoudi, B., and Ravel, N. (2004). Learning modulation of odor-induced oscillatory responses in the rat olfactory bulb: A correlate of odor recognition? J. Neurosci. 24, 389–397. doi: 10.1523/JNEUROSCI.3433-03.2004
Martin, C., Gervais, R., Messaoudi, B., and Ravel, N. (2006). Learning-induced oscillatory activities correlated to odour recognition: A network activity. Eur. J. Neurosci. 23, 1801–1810. doi: 10.1111/j.1460-9568.2006.04711.x
Martin-Lopez, E., Meller, S. J., and Greer, C. A. (2018). Development of piriform cortex interhemispheric connections via the anterior commissure: Progressive and regressive strategies. Brain Struct. Funct. 223, 4067–4085. doi: 10.1007/s00429-018-1741-y
McLean, J. H., and Shipley, M. T. (1991). Postnatal development of the noradrenergic projection from locus coeruleus to the olfactory bulb in the rat. J. Comp. Neurol. 304, 467–477. doi: 10.1002/cne.903040310
McLean, J. H., Darby-King, A., Sullivan, R. M., and King, S. R. (1993). Serotonergic influence on olfactory learning in the neonate rat. Behav. Neural Biol. 60, 152–162. doi: 10.1016/0163-1047(93)90257-i
Miao, Q., Yao, L., Rasch, M. J., Ye, Q., Li, X., and Zhang, X. (2016). Selective maturation of temporal dynamics of intracortical excitatory transmission at the critical period onset. Cell Rep. 16, 1677–1689. doi: 10.1016/j.celrep.2016.07.013
Miller, S. S., and Spear, N. E. (2008). Olfactory learning in the rat neonate soon after birth. Dev. Psychobiol. 50, 554–565. doi: 10.1002/dev.20318
Miller, S., and Spear, N. (2009). Olfactory learning in the rat immediately after birth: Unique salience of first odors. Dev. Psychobiol. 51, 488–504. doi: 10.1002/dev.20388
Mooney, R., Penn, A., Gallego, R., and Shatz, C. (1996). Thalamic relay of spontaneous retinal activity prior to vision. Neuron 17, 863–874. doi: 10.1016/S0896-6273(00)80218-4
Morrison, G., Fontaine, C., Harley, C., and Yuan, Q. (2013). A role for the anterior piriform cortex in early odor preference learning: Evidence for multiple olfactory learning structures in the rat pup. J. Neurophysiol. 110, 141–152. doi: 10.1152/jn.00072.2013
Mukherjee, B., Morrison, G. L., Fontaine, C. J., Hou, Q., Harley, C. W., and Yuan, Q. (2014). Unlearning: NMDA receptor-mediated metaplasticity in the anterior piriform cortex following early odor preference training in rats. J. Neurosci. 34, 5143–5151. doi: 10.1523/JNEUROSCI.0128-14.2014
Nakashima, A., Ihara, N., Shigeta, M., Kiyonari, H., Ikegaya, Y., and Takeuchi, H. (2019). Structured spike series specify gene expression patterns for olfactory circuit formation. Science 365:eaaw5030. doi: 10.1126/science.aaw5030
Oruro, E. M., Pardo, G. V. E., Lucion, A. B., Calcagnotto, M. E., and Idiart, M. A. P. (2020). Maturation of pyramidal cells in anterior piriform cortex may be sufficient to explain the end of early olfactory learning in rats. Learn. Mem. 27, 20–32. doi: 10.1101/lm.050724.119
Otazu, G. H., Chae, H., Davis, M. B., and Albeanu, D. F. (2015). Cortical feedback decorrelates olfactory bulb output in awake mice. Neuron 86, 1461–1477. doi: 10.1016/j.neuron.2015.05.023
Pardo, G. V. E., Lucion, A. B., and Calcagnotto, M. E. (2018). Postnatal development of inhibitory synaptic transmission in the anterior piriform cortex. Int. J. Dev. Neurosci. 71, 1–9. doi: 10.1016/j.ijdevneu.2018.07.008
Pedersen, P. E., and Blass, E. M. (1982). Prenatal and postnatal determinants of the 1st suckling episode in albino rats. Dev. Psychobiol. 15, 349–355. doi: 10.1002/dev.420150407
Poo, C., and Isaacson, J. S. (2007). An early critical period for long-term plasticity and structural modification of sensory synapses in olfactory cortex. J. Neurosci. 27, 7553–7558. doi: 10.1523/JNEUROSCI.1786-07.2007
Poo, C., and Isaacson, J. S. (2009). Odor representations in olfactory cortex: “sparse” coding, global inhibition, and oscillations. Neuron 62, 850–861. doi: 10.1016/j.neuron.2009.05.022
Price, J. L., and Powell, T. P. (1970). An experimental study of the origin and the course of the centrifugal fibres to the olfactory bulb in the rat. J. Anat. 107(Pt 2), 215–237.
Redolfi, N., and Lodovichi, C. (2021). Spontaneous afferent activity carves olfactory circuits. Front. Cell. Neurosci. 15:637536. doi: 10.3389/fncel.2021.637536
Rochefort, N. L., Garaschuk, O., Milos, R. I., Narushima, M., Marandi, N., Pichler, B., et al. (2009). Sparsification of neuronal activity in the visual cortex at eye-opening. Proc. Natl. Acad. Sci. U.S.A. 106, 15049–15054. doi: 10.1073/pnas.0907660106
Romagnoni, A., Colonnese, M. T., Touboul, J. D., and Gutkin, B. S. (2020). Progressive alignment of inhibitory and excitatory delay may drive a rapid developmental switch in cortical network dynamics. J. Neurophysiol. 123, 1583–1599. doi: 10.1152/jn.00402.2019
Rosenblatt, J. S. (1983). Olfaction mediated developmental transition in the altricial newborn of selected species of mammals. Dev. Psychobiol. 16, 347–375. doi: 10.1002/dev.420160502
Rosselli-Austin, L., and Altman, J. (1979). The postnatal development of the main olfactory bulb of the rat. J. Dev. Physiol. 1, 295–313.
Roth, T., and Sullivan, R. (2005). Memory of early maltreatment: Neonatal behavioral and neural correlates of maternal maltreatment within the context of classical conditioning. Biol. Psychiatry 57, 823–831. doi: 10.1016/j.biopsych.2005.01.032
Sarma, A., Richard, M., and Greer, C. (2011). Developmental dynamics of piriform cortex. Cereb. Cortex 21, 1231–1245. doi: 10.1093/cercor/bhq199
Schaal, B., Saxton, T. K., Loos, H., Soussignan, R., and Durand, K. (2020). Olfaction scaffolds the developing human from neonate to adolescent and beyond. Philos. Trans. R. Soc. Lond. B Biol. Sci. 375:20190261. doi: 10.1098/rstb.2019.0261
Schoppa, N. E., and Urban, N. N. (2003). Dendritic processing within olfactory bulb circuits. Trends Neurosci. 26, 501–506. doi: 10.1016/S0166-2236(03)00228-5
Schwob, J. E., and Price, J. L. (1984). The development of axonal connections in the central olfactory system of rats. J. Comp. Neurol. 223, 177–202. doi: 10.1002/cne.902230204
Schwob, J., Haberly, L., and Price, J. (1984). The development of physiological-responses of the piriform cortex in rats to stimulation of the lateral olfactory tract. J. Comp. Neurol. 223, 223–237. doi: 10.1002/cne.902230206
Strowbridge, B. W. (2009). Role of cortical feedback in regulating inhibitory microcircuits. Ann. N. Y. Acad. Sci. 1170, 270–274. doi: 10.1111/j.1749-6632.2009.04018.x
Sullivan, R. M., and Leon, M. (1987). One-trial olfactory learning enhances olfactory bulb responses to an appetitive conditioned odor in 7-day-old rats. Brain Res. 432, 307–311. doi: 10.1016/0165-3806(87)90056-3
Sullivan, R. M., Stackenwalt, G., Nasr, F., Lemon, C., and Wilson, D. A. (2000). Association of an odor with activation of olfactory bulb noradrenergic beta-receptors or locus coeruleus stimulation is sufficient to produce learned approach responses to that odor in neonatal rats. Behav. Neurosci. 114, 957–962. doi: 10.1037/0735-7044.114.5.957
Sullivan, R. M., Wilson, D. A., Lemon, C., and Gerhardt, G. A. (1994). Bilateral 6-OHDA lesions of the locus coeruleus impair associative olfactory learning in newborn rats. Brain Res. 643, 306–309. doi: 10.1016/0006-8993(94)90038-8
Sullivan, R. M., Zyzak, D. R., Skierkowski, P., and Wilson, D. A. (1992). The role of olfactory bulb norepinephrine in early olfactory learning. Brain Res. Dev. Brain Res. 70, 279–282. doi: 10.1016/0165-3806(92)90207-d
Tatti, R., Swanson, O. K., Lee, M. S. E., and Maffei, A. (2017). Layer-specific developmental changes in excitation and inhibition in rat primary visual cortex. eNeuro 4:ENEURO.0402-17.2017. doi: 10.1523/ENEURO.0402-17.2017
Tau, G. Z., and Peterson, B. S. (2010). Normal development of brain circuits. Neuropsychopharmacology 35, 147–168. doi: 10.1038/npp.2009.115
Treloar, H., Purcell, A., and Greer, C. (1999). Glomerular formation in the developing rat olfactory bulb. J. Comp. Neurol. 413, 289–304.
Tritsch, N. X., Rodríguez-Contreras, A., Crins, T. T., Wang, H. C., Borst, J. G., and Bergles, D. E. (2010). Calcium action potentials in hair cells pattern auditory neuron activity before hearing onset. Nat. Neurosci. 13, 1050–1052. doi: 10.1038/nn.2604
Tritsch, N., Yi, E., Gale, J., Glowatzki, E., and Bergles, D. (2007). The origin of spontaneous activity in the developing auditory system. Nature 450, 50–55. doi: 10.1038/nature06233
Walz, A., Omura, M., and Mombaerts, P. (2006). Development and topography of the lateral olfactory tract in the mouse: Imaging by genetically encoded and injected fluorescent markers. J. Neurobiol. 66, 835–846. doi: 10.1002/neu.20266
Wang, C., Ohno, K., Furukawa, T., Ueki, T., Ikeda, M., Fukuda, A., et al. (2005). Differential expression of KCC2 accounts for the differential GABA responses between relay and intrinsic neurons in the early postnatal rat olfactory bulb. Eur. J. Neurosci. 21, 1449–1455. doi: 10.1111/j.1460-9568.2005.03975.x
Weliky, M., and Katz, L. (1999). Correlational structure of spontaneous neuronal activity in the developing lateral geniculate nucleus in vivo. Science 285, 599–604. doi: 10.1126/science.285.5427.599
Westenbroek, R. E., Westrum, L. E., Hendrickson, A. E., and Wu, J. Y. (1988). Ultrastructural localization of immunoreactivity in the developing piriform cortex. J. Comp. Neurol. 274, 319–333. doi: 10.1002/cne.902740303
Wilson, D. A., and Leon, M. (1986). Early appearance of inhibition in the neonatal rat olfactory bulb. Brain Res. 391, 289–292. doi: 10.1016/0165-3806(86)90295-6
Wilson, D. A., and Leon, M. (1987). Abrupt decrease in synaptic inhibition in the postnatal rat olfactory bulb. Brain Res. 430, 134–138. doi: 10.1016/0165-3806(87)90183-0
Wilson, D. A., and Leon, M. (1988). Spatial patterns of olfactory bulb single-unit responses to learned olfactory cues in young rats. J. Neurophysiol. 59, 1770–1782. doi: 10.1152/jn.1988.59.6.1770
Wilson, D. A., Sullivan, R. M., and Leon, M. (1985). Odor familiarity alters mitral cell response in the olfactory bulb of neonatal rats. Brain Res. 354, 314–317. doi: 10.1016/0165-3806(85)90186-5
Wilson, D. A., Sullivan, R. M., and Leon, M. (1987). Single-unit analysis of postnatal olfactory learning: Modified olfactory bulb output response patterns to learned attractive odors. J. Neurosci. 7, 3154–3162. doi: 10.1523/JNEUROSCI.07-10-03154.1987
Yu, C. R., Power, J., Barnea, G., O’Donnell, S., Brown, H. E., Osborne, J., et al. (2004). Spontaneous neural activity is required for the establishment and maintenance of the olfactory sensory map. Neuron 42, 553–566. doi: 10.1016/s0896-6273(04)00224-7
Yu, Y., Burton, S. D., Tripathy, S. J., and Urban, N. N. (2015). Postnatal development attunes olfactory bulb mitral cells to high-frequency signaling. J. Neurophysiol. 114, 2830–2842. doi: 10.1152/jn.00315.2015
Keywords: piriform cortex, olfactory bulb, neonatal, local field potential, oscillation, inhibition
Citation: Maier JX and Zhang Z (2023) Early development of olfactory circuit function. Front. Cell. Neurosci. 17:1225186. doi: 10.3389/fncel.2023.1225186
Received: 18 May 2023; Accepted: 29 June 2023;
Published: 26 July 2023.
Edited by:
Heiko J. Luhmann, Johannes Gutenberg University Mainz, GermanyReviewed by:
Claudia Lodovichi, National Research Council (CNR), ItalyHaruyuki Kamiya, Hokkaido University, Japan
Copyright © 2023 Maier and Zhang. This is an open-access article distributed under the terms of the Creative Commons Attribution License (CC BY). The use, distribution or reproduction in other forums is permitted, provided the original author(s) and the copyright owner(s) are credited and that the original publication in this journal is cited, in accordance with accepted academic practice. No use, distribution or reproduction is permitted which does not comply with these terms.
*Correspondence: Joost X. Maier, am1haWVyQHdha2VoZWFsdGguZWR1