- Department of Pharmacology and Pharmaceutical Sciences, Alfred E. Mann School of Pharmacy and Pharmaceutical Sciences, University of Southern California, Los Angeles, CA, United States
Translocator protein (TSPO), a 18 kDa protein found in the outer mitochondrial membrane, has historically been associated with the transport of cholesterol in highly steroidogenic tissues though it is found in all cells throughout the mammalian body. TSPO has also been associated with molecular transport, oxidative stress, apoptosis, and energy metabolism. TSPO levels are typically low in the central nervous system (CNS), but a significant upregulation is observed in activated microglia during neuroinflammation. However, there are also a few specific regions that have been reported to have higher TSPO levels than the rest of the brain under normal conditions. These include the dentate gyrus of the hippocampus, the olfactory bulb, the subventricular zone, the choroid plexus, and the cerebellum. These areas are also all associated with adult neurogenesis, yet there is no explanation of TSPO’s function in these cells. Current studies have investigated the role of TSPO in microglia during neuron degeneration, but TSPO’s role in the rest of the neuron lifecycle remains to be elucidated. This review aims to discuss the known functions of TSPO and its potential role in the lifecycle of neurons within the CNS.
Introduction
Translocator protein (TSPO) is an 18 kD five transmembrane-domain protein found in the outer mitochondrial membrane (OMM) and distributed throughout the mammalian body (Papadopoulos et al., 2006). This protein contains both cholesterol- and drug-binding domains and was previously known as the peripheral benzodiazepine receptor (PBR) due to its ability to bind the benzodiazepine diazepam (Marangos et al., 1982). TSPO forms a complex with voltage-dependent anion-selective channel 1 (VDAC1), spanning the outer mitochondrial membrane (Veenman et al., 2008; Gatliff et al., 2014), and interacts with several proteins, including ATPase family AAA domain-containing protein 3A (ATAD3), steroidogenic acute regulatory protein (STAR), acyl-CoA binding domain 1 (ACBD1), or diazepam binding inhibitor (DBI) and ACBD3 (Fan and Papadopoulos, 2013) to form complexes (Figure 1). It is most known for facilitating cholesterol transport from the cytosol into the mitochondria (Krueger and Papadopoulos, 1990; Papadopoulos et al., 2015; Biswas et al., 2018; Li et al., 2021). Studies have also supported its contribution to mediating ion transport and metabolites, oxidative stress, inflammation, apoptosis, and energy metabolism through downstream pathways (Stoebner et al., 2001; Santidrián et al., 2007; Veenman et al., 2008; Shargorodsky et al., 2012; Mendonça-Torres and Roberts, 2013).
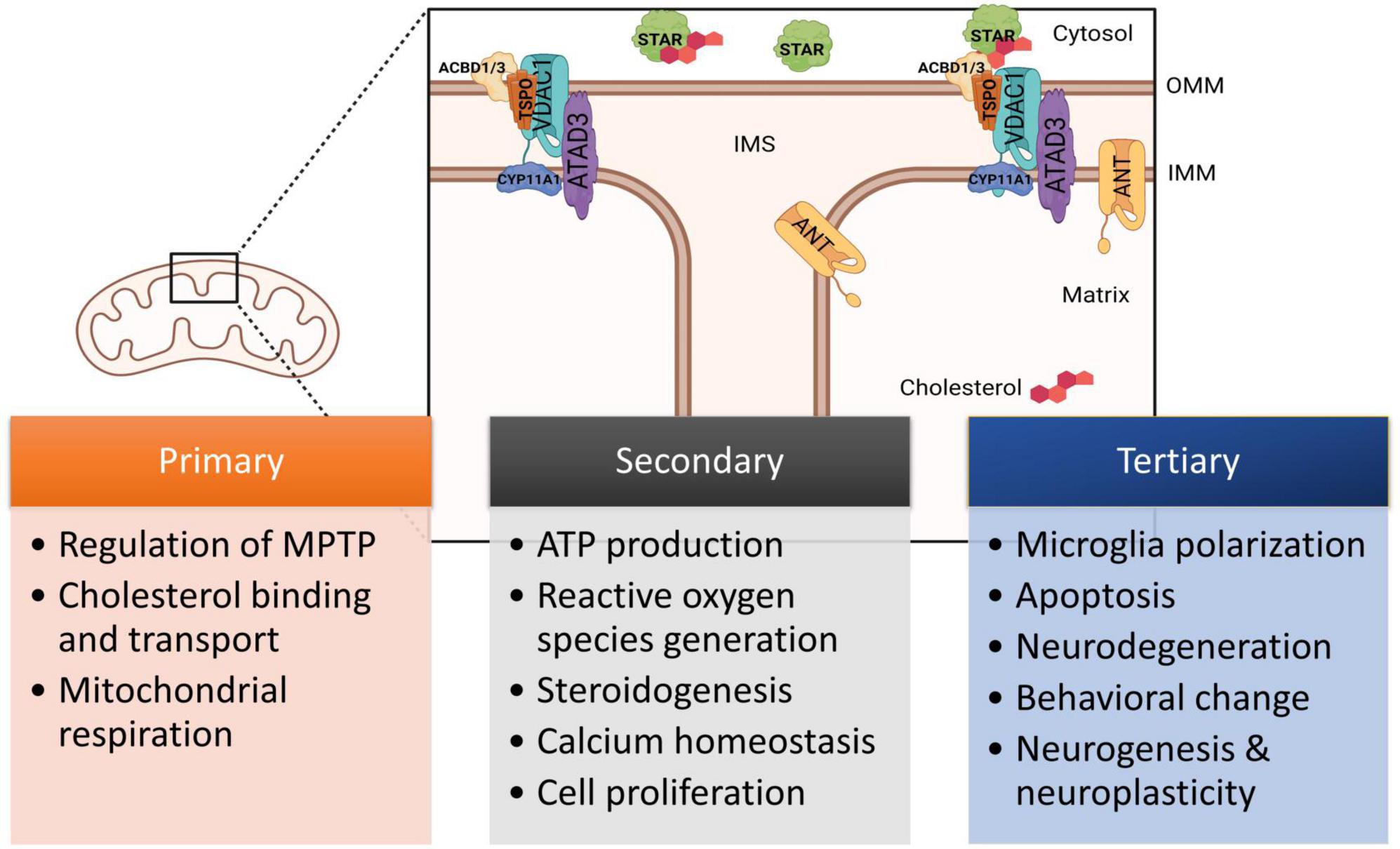
Figure 1. Diagram of TSPO and associated proteins located in the mitochondria demonstrating structural organization and the transport of cholesterol. Figure not drawn to scale. Currently known relationships with TPSO and downstream functions are indicated, including primary, secondary, and tertiary effects. ACBD1, acyl-CoA binding domain 1; ACBD3, acyl-CoA binding domain 3; ANT, adenine nucleotide translocator; ATAD3, ATPase family AAA domain-containing protein 3A; CYP11A1, cytochrome P450 11A1 cholesterol side-chain cleavage enzyme; IMM, inner mitochondrial membrane; OMM, outer mitochondrial membrane; STAR, steroidogenic acute regulatory protein; TSPO, translocator protein; VDAC1, voltage-dependent anion-selective channel 1. This figure created from biorender.com.
Translocator protein contains a cholesterol-recognition amino acid consensus sequence (CRAC) (Li and Papadopoulos, 1998; Jamin et al., 2005; Jaipuria et al., 2017), ubiquitously found in transmembrane proteins that interact with cholesteryl groups (Vincent et al., 2002). Administration of a CRAC peptide prevents the opening of the mitochondrial permeability transition pore (MPTP) (Azarashvili et al., 2016). Both cholesterol and TSPO ligands have been shown to bind to MPTP with nanomolar affinity across species (Papadopoulos et al., 1992; Lacapère et al., 2001). Nevertheless, despite extensive study of TSPO’s facilitation of cholesterol transport into the mitochondria (Li and Papadopoulos, 1998; Li et al., 2001), recent knockout studies dispute this claim (Morohaku et al., 2014). A review of the alternative results along with all prior literature, suggests that the conservation of TSPO across all mammalian species increases the likelihood that redundant mechanisms are available to compensate for its removal (Papadopoulos et al., 2018), making the study of TSPO and its functions more complex.
Classically, TSPO’s high levels in steroidogenic organs have been associated with the production of steroids in the endocrine system (Papadopoulos et al., 1990, 2015). However, TSPO is found in cells all over the body. The central nervous system (CNS) normally expresses TSPO at low levels, making it notable when there is significant upregulation detected during neuroinflammation associated with dementia and other neurodegenerative diseases. Although higher levels of TSPO in the CNS are generally detected during inflammation, there are five specific regions in the adult brain where TSPO has been detectable under normal conditions. These include the olfactory bulb (OB), the subventricular zone (SVZ), the dentate gyrus of the hippocampus, the choroid plexus, and the cerebellum (Betlazar et al., 2018; Nutma et al., 2021). One common characteristic of these regions of the brain is that they are all associated with adult neurogenesis. While studies have examined the clinical application of TSPO, specifically with inflammation, only limited research has explored TSPO’s role in the neuronal life cycle. Currently, it is still unknown why TSPO is highly expressed in these brain regions. This review aims to discuss the possible function of TSPO in the life cycle of neurons, both directly and indirectly, through interactions with other cell types in the CNS.
Current known functions of TSPO
Translocator protein was initially found in the pursuit of other proteins that benzodiazepines would bind to, and [3H]Ro5-4864 was used to characterize it (Marangos et al., 1982). As a result, Ro5-4864 (Ki: 1.02–20.04 nM) (Kita et al., 2004; Costa et al., 2016) was used as an exploratory treatment to determine the effect of ligand binding to the protein. Since then, other ligands have been developed in attempts to increase the binding specificity and affinity. Initial ligands included PK 11195 (Ki: 0.602–3.60 nM) (Kita et al., 2004; Costa et al., 2016) and FGIN-1-27 (Ki: 3.25 nM) (Kita et al., 2004). These were followed by XBD173 (Ki: 0.297 nM) (Kita et al., 2004), Etifoxine (Ki: 7.8–9.0 μM) (Costa et al., 2017; Bloms-Funke et al., 2022; Owen et al., 2022), SSR-180 575, and PIGA (N,N-Dialkyl-2-phenylindol-3-ylglyoxylamide; Ki: 0.31 to 343.01 nM) (Costa et al., 2016). Most recently GRT-X [(N-[(3-fluorophenyl)-methyl]-1-(2-methoxyethyl)-4-methyl-2-oxo-(7-trifluoromethyl)-1H-quinoline-3-caboxylic acid amide]; Ki: 0.07–4.60 μM) (Bloms-Funke et al., 2022), 2a (Ki: 40-200 nM) (Hallé et al., 2017), 2b (Ki: 5.5–6 nM) (Hallé et al., 2017), and ZBD-2 (N-benzyl-N-ethyl-2-(7,8-dihydro-7-benzyl-8-oxo-2-phenyl-9H-purin-9-yl) acetamide; Ki: 0.463 nM) (Wang D. et al., 2015) have also been used as TSPO ligands (Table 1). These ligands exhibit varying binding affinities depending on whether mitochondrial homogenates, cells or whole organ tissue was used for the assay. The introduction of these ligands allows for examining the function of TSPO when it is present. However, a genetic knockout or knockdown model can assess the basal biological function of TSPO and determine any compensatory mechanisms when the protein is removed from the system. These methods are not mutually exclusive and are important to be used in conjunction to fully understand the role of TSPO. This section outlines and explains the current known functions of TSPO.
Steroidogenesis
The most prominent role of TSPO in the periphery, steroidogenesis, has been extensively studied with data supporting the increase of steroid production through administration of TSPO ligands PK 11195, FGIN-1-27, PIGA, and Ro5-4864 (Papadopoulos et al., 1990; Chung et al., 2013; Da Pozzo et al., 2016; Chen et al., 2019; Table 1). Treatment of Leydig cells, the primary testosterone-producing cells in the male reproductive system, with diazepam binding inhibitor (DBI) increased steroid production (Papadopoulos et al., 1991; Garnier et al., 1994). DBI is an endogenous TSPO ligand isolated from rat brains. This increase in steroid production was also observed in rodent studies with adult rats treated with other TSPO ligands (Chung et al., 2013; Liere et al., 2017).
The involvement of TSPO in steroidogenesis is supported by genetic TSPO knockouts both in vitro and in vivo. However, recent studies have also challenged TSPO’s role in steroidogenesis from various knockout models (Morohaku et al., 2014; Tu et al., 2015). Nevertheless, additional studies utilizing CRISPR/Cas9-mediated knockout of TSPO in mouse Leydig cells showed a marked reduction in mitochondrial membrane potential and steroid formation (Fan et al., 2018). Similar steroidogenic abnormalities were also observed in TSPO knockout mice and rats, where total steroid production was decreased, and an increase in steroidogenic flux was observed (Owen et al., 2017; Barron et al., 2018).
It should also be noted that studies challenging the role of TSPO in steroidogenesis with TSPO knockout models observed the highest steroid production at drug concentrations 10–100 times greater than previous publications. The most noticeable increase occurred at 100 times higher concentrations; however, it is not certain if this increase was significant since the statistics were not published (Tu et al., 2015). This increase could be attributed to off-target binding of the ligand used (PK 11195), as it was given at a much higher concentration than needed for observable steroidogenic effects found in previous studies.
In addition to the effect on peripheral steroidogenesis, TSPO ligands have been reported to increase steroid production within the CNS as well. Ligands XBD173 and Ro5-4864 increased pregnenolone synthesis in the BV-2 microglia cell line (Bader et al., 2019). The same effect was observed when TSPO ligands were used to treat human neuroblastoma SH-SY5Y cells (Hallé et al., 2017; Lejri et al., 2019), human microglia cell lines (Da Pozzo et al., 2016; Lin et al., 2022), human astrocyte cell line (Lin et al., 2022), human glioblastoma cells (Lin et al., 2022), and rat glioma C6 cells (Da Pozzo et al., 2016; Santoro et al., 2016). Along with in vitro studies, TSPO ligands XBD173, SSR-180,575, PK 11195, and FGIN-1-27 were found to increase brain neurosteroid levels in vivo as well (Rupprecht et al., 2009; Porcu et al., 2016). These data show that TSPO, though barely expressed in the CNS under normal conditions, is still present and functional in the production of neurosteroids.
Energy metabolism
Translocator protein influences energy metabolism in the mitochondria, specifically the membrane potential and oxygen consumption rate, playing a role in cellular respiration. TSPO has been shown to also interact with the MPTP, consisting of VDAC1 and the adenine nucleotide translocator (ANT), and contributes to the generation of reactive oxygen species (ROS), moderation of Ca2+ homeostasis, and ATP production (Veenman et al., 2008; Banati et al., 2014; Gatliff et al., 2014, 2017; Papadopoulos et al., 2015; Biswas et al., 2018; Farhan et al., 2021). Though there has been contention with the interaction of TSPO, VDAC1, and ANT as the MPTP opened in the absence of these proteins, evidence suggests that they are still involved potentially with the MPTP pore formation and in the apoptosis cascade (Veenman et al., 2008; Camara et al., 2017; Shoshan-Barmatz et al., 2019). Previous studies reporting changes in mitochondrial membrane potential have found altered ATP production (Vojtíšková et al., 2004) and oxygen consumption rates (Schuchmann et al., 2005) in vivo and in vitro (Zorova et al., 2018). TSPO deficiency in fibroblasts exhibited a decrease in oxygen consumption rate and mitochondrial membrane potential (Zhao et al., 2016). Similarly, microglia isolated from TSPO knockout animals had lower ATP synthesis (Banati et al., 2014), while TSPO insertion into human leukemia cell lines resulted in increased cellular excitability and ATP production (Liu et al., 2017). TSPO ligands had a similar effect in improving ATP production impaired by mitochondrial injury (Palzur et al., 2021).
Additionally, TSPO is involved in mitochondrial respiration within the CNS. An increase in ATP synthesis was observed when human neuroblastoma SH-SY5Y cells were treated with TSPO ligands (Lejri et al., 2019; Grimm et al., 2020). A reverse effect was observed with a CRISPR-Cas9 TSPO knockout, decreasing cellular respiratory function and mitochondrial membrane potential (Milenkovic et al., 2019). The impaired mitochondrial functions were rescued with lentiviral overexpression of TSPO in the knockout cells. As a result, it appears that TSPO in the CNS may be responsible for both overall mitochondrial health and ATP production.
Translocator protein has also been reported to play a role in apoptosis and has been investigated as a possible treatment in oncology. Depending on the stage of breast cancer progression, changes in TSPO have both proliferative and decreased viability effects (Wu and Gallo, 2013). An increase in TSPO has also been linked to both increases in expression and gene amplification likely part of a feedback system with ROS induction (Batarseh and Papadopoulos, 2010). Interactions with VDAC1 may also be a way TSPO contributes to apoptosis. TSPO ligands PK 11195 and Ro5-4864 altered MPTP opening and were shown to inhibit the pro-apoptotic function of erucylphosphohomocholine (an alkylphosphocholine antitumor agent) at high concentrations, preventing the collapse of the mitochondrial membrane potential (Veenman et al., 2008). TSPO ligands have also been shown to increase cell survival (Ferzaz et al., 2002) and decrease Caspase-3 apoptotic cells (Shehadeh et al., 2019). This was further supported with studies showing that the downregulation of TSPO expression inhibited oxidative stress from anoxia/reoxygenation insult (Meng et al., 2020). Treatment with TSPO ligands PIGA also protected photoreceptor-like cells from damage induced by lipopolysaccharide (LPS)-driven inflammation, resulting in a noticeable increase in cell viability at nanomolar doses (Corsi et al., 2022). On the other hand, one paper found that treatment with ligand FGIN-1-27 induced apoptosis in HT29 cancer cells and correlated with decreased membrane potential, while ligand PK 11195 induced apoptosis through Bcl-2 downregulation (Maaser et al., 2005). Nevertheless, there is little understanding of how TSPO may be influencing viability and regulated cell death. Possible explanations include altering the mitochondrial membrane potential or modulation of ROS, leading to apoptosis.
Reactive oxygen species are essential for cell regulation and are highly involved with cellular signal transduction, particularly in metabolism and apoptosis. ROS are byproducts of mitochondrial respiration but are also found altered in disease and cause proinflammatory cytokine release (Bulua et al., 2011). Oxidative stress leads to elevated inflammation, which is associated with cell death and apoptosis. Specifically, ROS play a role in LPS-driven inflammation caused by cytokine release (Bulua et al., 2011). Injury caused by anoxia/reoxygenation injury in cardiomyocytes has also resulted in increased TSPO expression and subsequent increased oxidative stress (Meng et al., 2020). On the other hand, downregulation of TSPO expression with RNA interference or the administration of various TSPO ligands has been found to decrease oxidative stress (Biswas et al., 2018; Meng et al., 2020). Additional studies have also reported the inhibition of TSPO by FGIN-1-27-amended Ca2+ overload caused by chemical ischemia in a cardiac cell line (Li et al., 2010). As a result, TSPO can alter ROS production, influence the signaling cascade, and lead to expedited progression of disease.
Neurological disorders
Translocator protein has been closely tied to neurodegenerative diseases like Alzheimer’s Disease (AD) and Parkinson’s Disease (PD) (Mcgeer et al., 1988; Zimmer et al., 2014; Edison et al., 2018; Zhou et al., 2021), due to the presence of dysfunctional mitochondria, and the increased inflammation as the innate response to degradation. AD and PD have both been linked to dysfunctional mitochondria, and TSPO has been reported to be capable of modulating mitochondrial health and mitophagy (Gatliff et al., 2014, 2017; Wang et al., 2020; Frison et al., 2021). Previous publications have also reported that the overexpression of TSPO led to impaired mitophagy, similar to those seen in PD and amyotrophic lateral sclerosis (ALS) (Frison et al., 2021; Magrì et al., 2023). This altered mitophagy and increased TSPO levels were also observed when mitochondrial respiration was significantly reduced. It is hypothesized that dysfunctional mitochondria may be involved in the progression of neurodegeneration, but the cause of the dysfunction remains to be elucidated.
The association between neurodegeneration and TSPO is also linked to the activation of microglia, the CNS’s resident macrophages. As a result, TSPO ligands have been used as fluorescent PET markers for inflammation in diagnosing AD and PD (Dheen et al., 2007; Karlstetter et al., 2014; Hamelin et al., 2016; James et al., 2017; Yokokura et al., 2017; Janssen et al., 2018; Daniela Femminella et al., 2019; Werry et al., 2019). Confusingly, the binding of [11C]PK 11195 failed in some patients known to have neuroinflammation. This has been attributed to the reduced binding affinity of [11C]PK 11195 to TSPO caused by a common polymorphism, where the 147th amino acid alanine is replaced with a threonine (Berroterán-Infante et al., 2019). New fluorescent TSPO ligands have been developed to bind regardless of the polymorphism (Vettermann et al., 2021). This polymorphism has been shown to have a 30% prevalence in individuals with European ancestry (Kreisl et al., 2013) and influences diurnal cortisol rhythm in individuals diagnosed with bipolar disorder (Prossin et al., 2018). The mutation not only affects ligand binding but also alters the binding of cholesterol to the CRAC domain (Li et al., 2015). On the other hand, this polymorphism has been shown to have no effect on the endogenous TSPO ligand DBI (Berroterán-Infante et al., 2019). These findings indicate that mutations in TSPO can have far-reaching consequences with endocrine signaling and lead to behavior alterations.
Translocator protein ligands have also been studied for their potential effects on limiting neurological disorders. PK 11195 was found to improve AD-related behavior and β-amyloid pathology in 3xTg-AD rats (Christensen and Pike, 2018), and XBD173 improved clinical symptoms of multiple sclerosis (MS) (Leva et al., 2017). Ro5-4864 also showed protection against β-amyloid toxicity in organotypic hippocampal cultures (Arbo et al., 2017a). However, the mechanism by which TSPO affects neurodegenerative disorders remains to be elucidated.
The anxiolytic and anti-depressive effects of TSPO ligands have been investigated as an alternative to conventional benzodiazepines. An anxiolytic effect was seen in chronic unpredictable mild stress-conditioned mice when TSPO ligand, ZBD-2 [N-benzyl-N-ethyl2-(7,8-dihydro-7-benzyl-8-oxo-2-phenyl-9H-purin-9-yl) acetamide], was administered (Wang et al., 2017). This effect was prevented by the coadministration of PK 11195. Administration of ZBD-2 also decreased depression in the same mice and depressive behavior in mouse models of postpartum depression (Li et al., 2018). This ligand was also reported to decrease anxiety-like behaviors caused by chronic inflammatory pain (Wang D. et al., 2015). Similar results were observed with an elevated-plus maze anxiety test in rats treated with PIGA (da Settimo et al., 2008). Anti-depressive effects were also seen with the administration of TSPO ligands FGIN-1-27 in non-mammalian models (Lima-Maximino et al., 2018) and XBD173 in rodent models of chronic stress and depression associated with diabetes mellitus (Qiu et al., 2016; Shang et al., 2020). It is believed that TSPO ligands alter neurological function by increasing the production of neurosteroids, which can act as inhibitory neurotransmitters (Longone et al., 2011). Neurosteroids have similarly been closely tied to the anxiolytic effects of TSPO, with ligands etifoxine being approved for treating anxiety (Nguyen et al., 2006) and brexanolone, the aqueous formulation of allopregnanolone, for treatment of postpartum depression (Scarff, 2019). Despite TSPO’s effect on anxiety and depression, the exact mechanism by which it acts is still unknown.
TSPO role in neurons
Although the functions of TSPO have been extensively studied in the periphery, it remains to be elucidated in neurons. The current knowledge of TSPO functions (such as steroid production, energy, and apoptosis) could be used as a basis to theorize and explore the potential means that TSPO can affect the lifecycle of neurons, either directly or indirectly (Figure 2).
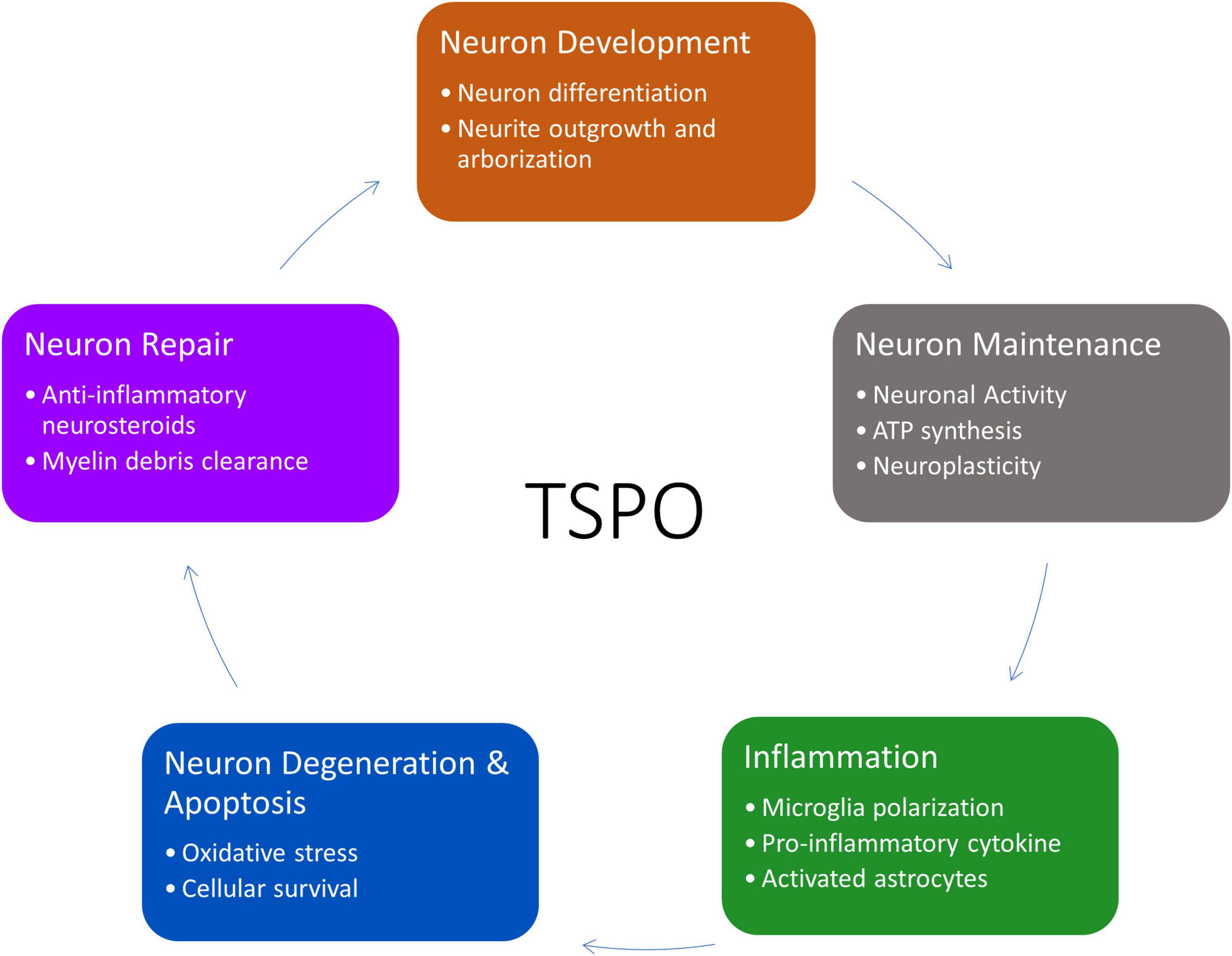
Figure 2. Overview of TSPO’s involvement in the life cycle of neurons from neurogenesis to apoptosis and recycling. This figure created from biorender.com.
Neurogenesis
Translocator protein has been difficult to detect in mature neurons, particularly in the normal brain. Recent studies have pointed toward a role for TSPO in neurogenesis, particularly as the few CNS regions with high TSPO are associated with neurogenesis. This was demonstrated by the discovery of TSPO in neural stem cells (NSC) by Varga et al. (2009). Moreover, the endogenous TSPO ligand DBI increased stem cell growth and expansion in neurogenesis (Dumitru et al., 2017), further indicating that TSPO may play a role in stem cell proliferation and differentiation. However, it may be related to GABA receptor function, as DBI can bind to GABA receptors (Bormann, 1991) and modulate GABA signaling (Everlien et al., 2022).
The role of TSPO in neurogenesis has also been investigated in neuronal differentiation with experiments using embryonic stem cell lines. Previous studies have found that the TSPO ligand PK 11195 could induce neuronal lineages. In PC12, a rat cell line of embryonic neural crest origin, PK 11195 treatment induced the development of neurites and increased expression of β-III tubulin, the neuron-specific tubulin in PC12 cells, without any additional supplements (Yasin et al., 2017). A study with N1E-115 cells, a mouse neuroblastoma cell line, also showed an increase in neuron-like morphology, but the cells were not tested for other neuronal markers (Krestinina et al., 2017). When PK 11195 was administered to P19 cells, a mouse embryonic carcinoma cell line, all neuronal markers were reported to increase, though not to the same level as cells treated with retinoic acid, the traditional cytokine used for differentiation (González-Blanco et al., 2021). Other TSPO ligands have also been shown to increase rates of neuron differentiation and neurite arborization in ex vivo models (Arbo et al., 2017b).
Although it appears that TSPO is directly involved in neurogenesis, different authors came to different conclusions. One study determined that the effect was not a result of TSPO but instead with mitochondrial 2′,3′-cyclo-nucleotide-3′-phosphodiesterase (Krestinina et al., 2017), while the other two studies either state that TSPO was directly involved in neuronal differentiation (González-Blanco et al., 2021) or that it indirectly led to differentiation through modulation of gene expression (Yasin et al., 2017). It should be noted that these experiments utilize only embryonic stem cells and cell lines. Studies have shown that genes and growth factors can have varying roles and effects depending on age and stage of development (Urbán and Guillemot, 2014). For example, γ-aminobutyric acid (GABA) promotes proliferation of embryonic NSCs (Haydar et al., 2000) but reduces proliferation in adult NSCs (Liu et al., 2005). As a result, TSPO’s function in stem cell survival and differentiation in adults remains to be elucidated.
Neuronal maintenance
As neurogenesis is not limited to the developmental period of organisms, TSPO may be involved in adult neurogenesis as well. Due to the high energy requirement of differentiation, TSPO and its interaction with cellular respiration could be important in modulating energy metabolism and ATP production. In adult hippocampal neurons, an increase in TSPO was found in response to increased neuronal activity (Notter et al., 2020). The upregulation of TSPO could also be involved in the increased cellular respiration and ATP production needed for the high energy demands of action potential generation (Aiello and Bach-Y-Rita, 2000; Berndt and Holzhütter, 2013).
Translocator protein may also contribute to the control of neurogenesis in adults through modulation of apoptosis in mammalian adult brains. In the adult, neural stem cells created through neurogenesis are not all incorporated into the neural network, and less than 2% of all mature neurons are replaced regularly (Ryu et al., 2016). In the rat, dentate gyrus, over 50% of all neural stem cells generated through adult neurogenesis will undergo apoptosis before they develop into mature neurons (Cameron and Mckay, 2001; Dayer et al., 2003). Similarly, only 40% of mature cells from adult neurogenesis integrate into the olfactory bulb (Winner et al., 2002). As TSPO has been linked to programmed cell death in other areas of the body, the relationship of TSPO with adult neurogenesis may also reflect this linkage. However, the exact role of TSPO in neural stem cell apoptosis has not been explored.
Maintenance of developing neurons is beneficial to overall cognitive health, but limiting the degradation of existing mature neurons may prevent additional problems as well. TSPO overexpression in the hippocampus, achieved through lentiviral transfection, reduced cognitive impairments caused by LPS-induced inflammation in mice (Wang et al., 2016). This indicates that TSPO may play a neuroprotective role by modulating apoptosis, as seen in other examples in the periphery. Though there have been data published on the presence of TSPO in neurons and predicted explanations, the role of TSPO in neurons remains to be fully established.
Another possibility is that the presence of TSPO in neurons is related to its anxiolytic effect, specifically in the management of anxiety and neuroplasticity. Overexpression of TSPO in the hippocampal dentate gyrus resulted in anxiolytic effects in a post-traumatic stress-disorder mouse model (Zhang et al., 2018). However, it remains to be seen if this is a result of increased rewiring neurons in the memory processing centers of the brain or of TSPO’s direct anxiolytic effect discussed above. Neurosteroids have been shown to modulate GABA receptors (Akk et al., 2001; Hosie et al., 2007) and improve learning and memory in rodents (Marx et al., 2009). As a result, the effects of TSPO in neurons may be due to neurosteroid production and metabolism.
Neuronal repair
Translocator protein may also be important in repair and regeneration, though most effects of TSPO ligands on neurons have primarily been studied in the peripheral nervous system (PNS). The TSPO ligand Ro5-4864 improved repair and remyelination of PNS neurons along with elevating brain-derived neurotropic factor (BDNF) levels in the dorsal root ganglion and sciatic nerve (Ma et al., 2018). However, a separate study using the same ligand found that TSPO did not alter BDNF levels between treatment conditions (Palzur et al., 2016). Therefore, it is not certain through which mechanism Ro5-4864 acts to promote recovery. Ro5-4864, along with etifoxine, was also shown to improve peripheral nerve injury repair and regeneration (Girard et al., 2008; Mills et al., 2008). Moreover, Ro5-4864 protected neonatal dorsal root ganglion neurons from apoptosis, though it had limited success in improving survival of mature neurons (Mills et al., 2008). Etifoxine also improved neurite outgrowth in PC12 cells, a cell line derived from a pheochromocytoma of the rat adrenal medulla (Girard et al., 2008). Thus, TSPO may play a role in neonatal development and survival of neural progenitors and might facilitate the repair of adult peripheral neurons.
Interestingly, all effects on neurons seen with TSPO ligands have been found in the PNS or in neurons taken during embryonic development. However, neurons in the PNS are capable of spontaneous regeneration after injury, while CNS neurons fail to do so (Huebner and Strittmatter, 2009). On the other hand, previous studies have shown CNS neuron regeneration when a PNS nerve graft was placed over them, indicating that CNS neurons do have the capacity to regrow when placed in a supportive environment (Stouffer et al., 1980). A recent study utilized another TSPO ligand GRT-X (N-[(3-fluorophenyl)-methyl]-1-[2-methoxyethyl]-4-methyl-2-oxo-[7-trifluoromethyl]-1H-quinoline-3-caboxylic acid amide), which is also an agonist for the voltage-gated potassium channel of the Kv7 family, to treat chronic neuropathic pain caused by traumatic injury (Bloms-Funke et al., 2022). It was effective in promoting the survival and repair of sensory and motor neurons. However, the injury in this study was also in the periphery, distal to the dorsal root ganglions, and indicates that TSPO ligands can influence peripheral nerve regeneration, but it did not explore any effects on the CNS. Alternatively, a study utilizing Ro5-4864 and its effect on traumatic brain injury in rats showed that TSPO protein was not detectable in control neurons but appeared to be upregulated in injured neurons (Palzur et al., 2016). This study confirmed the presence of TSPO during neuronal injury but again did not explore its function. As a result, the role of TSPO within CNS neurons remains to be elucidated despite the presence of the protein in individual CNS niches.
TSPO in glial regulation of neuronal health
The study of TSPO in the CNS has primarily focused on neuroinflammation and neurodegeneration, primarily the involvement of microglia. Microglia are unique brain macrophages that differ in roles and functions from peripheral macrophages. Specifically, microglia act as the first line of defense within the CNS and respond to calcium waves (Olson and Miller, 2004; Sieger et al., 2012), while peripheral macrophages do not. Microglia respond to brain-damage Ca2+ and ATP signals, T helper cytokines, and LPS, activating to produce pro-inflammatory cytokines and inducing an M1 polarization (proinflammatory) state (Sieger et al., 2012; Franco and Fernández-Suárez, 2015). Microglia are also crucial for neuronal repair as they remove damaged cells and debris via phagocytosis to prepare the area for regeneration (Liu et al., 2012; Cai et al., 2019; Berghoff et al., 2021). In the event of injuries to the CNS, common inflammatory cytokines and ROS are released to initiate wound repair.
Translocator protein is involved in the phagocytotic ability of microglia. Microglia taken from TSPO knockout mice had impaired phagocytosis, which led to the accumulation of β-amyloid that was not properly cleared and removed (Zhang et al., 2021). This may be a result of the high energy demand of cytoskeletal reorganization during phagocytosis, where a decrease in energy production was accompanied by impaired phagocytosis (Yao et al., 2020; Fairley et al., 2023). These studies suggest that a shift toward glycolysis caused by TSPO knockout could lead to lower ATP production. This was also supported in experiments using TSPO knockout cells, TSPO knockout animals, and reduced TSPO levels found in aging animals (Garza et al., 2022). Alternatively, a shift toward glycolysis would also increase lactate production and lower pH levels. Cellular pH has been shown to alter the ability of macrophages to phagocytose (Grinstein et al., 1991). Moreover, modulation of microglia polarization via TSPO can also affect phagocytosis, as TSPO induces M1 polarization over M2. M2 polarization increases the production of scavenger receptors for phagocytosis and is more effective in its ability to uptake and degrade β-amyloid than M1 microglia (Cherry et al., 2014). Therefore, TSPO appears to be involved in the polarization of microglia required for phagocytosis, which is needed to remove apoptotic neural stem cells in the neurogenic niches. TSPO ligands have been found to reduce inflammation and β-amyloid generation in vitro (Xue et al., 2022) and in vivo (Ma et al., 2016; Simon-O’Brien et al., 2016) and reduce quinolinic-acid-induced degeneration (Leaver et al., 2012) and LPS-induced cognitive dysfunction in rats (Lan et al., 2021). Previous studies have also reported that TSPO ligands direct microglia toward M2 polarization to promote anti-inflammatory cytokines (Zhou J. et al., 2020) and increase microglia phagocytotic ability (Fairley et al., 2023).
Microglia activation increases cytokine release and is involved in the phagocytosis of cellular debris (Lampron et al., 2015). This response initiates the clearing of degraded myelin, leading to neuron recovery and repair (Lloyd et al., 2019; Berghoff et al., 2021). Microglia are also responsible for secreting anti-inflammatory cytokines to promote regeneration after removing the source of inflammation. This may also explain the reduction of β-amyloid accumulation with Ro5-4864 treatment (Barron et al., 2013), as it may increase microglia clearance of debris. The protective effects of TSPO ligands have also been investigated as possible treatments for neurodegenerative diseases, primarily by inhibiting the inflammatory response (Karlstetter et al., 2014; Scholz et al., 2015; Leva et al., 2017). For example, treatment with etifoxine reduced clinical pathology in a mouse model of MS by lowering the number of peripheral immune cells infiltrating the CNS and promoting remyelination after peripherally induced demyelination (Daugherty et al., 2013).
Translocator protein-modulated microglia polarization and neurogenesis are also connected via cytokines secreted by microglia that aid in the removal of apoptotic cells and promote neuron differentiation, depending on their polarization state. Initial pro-inflammatory activation of microglia to the M1 state is required for myelin clearance after demyelination (Cunha et al., 2020). M1 polarization further produces pro-inflammatory cytokines (Wang W. Y. et al., 2015) and increases ROS production necessary for macrophage myelin phagocytosis (Van Der Goes et al., 1998). Once there is sufficient breakdown, microglia shift to M2 state through anti-inflammatory signaling (Zhou D. et al., 2020; Guo et al., 2022). They can also secrete anti-inflammatory cytokines to increase the signal as well as begin phagocytosis and breakdown of myelin debris (Tang and Le, 2016; Guo et al., 2022). TSPO’s role in modulating the pro-inflammatory M1 and anti-inflammatory M2 polarization of microglia (da Pozzo et al., 2019) directly impacts the degradation and clearance of myelin and cellular debris that inhibits neurogenesis and promotes the regeneration process.
Translocator protein may also influence neuronal development via its function in glial cells. De novo neurosteroid synthesis can play a role in both the development and regulation of neuronal health and function and is primarily observed in glial cells. Progesterone directly influences neurogenesis, as progesterone treatment can increase the number of dopamine neurons during neuronal differentiation from mouse embryonic stem cells (Díaz et al., 2009). Similar effects were observed with human umbilical cord mesenchymal stem cell differentiation into neuron-like cells (Wang et al., 2012). De novo synthesized progesterone also increased dendritic spine formation in developing rat Purkinje cells (Sakamoto et al., 2002). Moreover, not only are neurosteroids involved in development, but they are also neuromodulatory. They can interact with extracellular GABA and potentiate GABA receptors on synaptic terminals. Specifically, progesterone, allopregnanolone, and deoxycorticosterone have been shown to promote the activation of GABA (Kokate et al., 1994; Reddy and Rogawski, 2002). Given the neurosteroid effects on neurogenesis and cognitive processes, in addition to TSPO’s demonstrated function in steroidogenesis, TSPO’s role in the CNS may include neurosteroid production used for the regeneration and maintenance of neurons.
Conclusion
Translocator protein has long been studied in the PNS, particularly its involvement in steroidogenesis and energy metabolism, but few studies have delved into its function in the development and regulation of cells in the central nervous system. It is important to determine if the effects seen in the PNS are applicable to the CNS, as the behavior of cells varies depending on the environment and location. This is particularly evident in neurogenesis, where only specific areas within the adult brain are involved, and neuronal repair and regrowth, which occurs spontaneously in the PNS but not the CNS. Crucially, the CNS areas with significant neurogenesis are also where TSPO has been reported at higher levels than the rest of the CNS. While most neurogenic studies have focused on embryonic development, more investigation is needed to determine if factors affecting neurogenesis stay consistent throughout the development of the neural system into adulthood. TSPO has been shown to be involved in the development of cells but is also important in controlled cell death, repair, and regeneration of neurons (Figure 2). Further studies investigating the detailed involvement of TSPO in each of these functions specific to CNS neurons may provide more clarity on how it affects neuronal development and health, adding to our understanding of neuron degradation and turnover.
Author contributions
GC, YL, and VP: conceptualization and writing–review and editing. GC: writing–original draft. VP: funding acquisition. All authors contributed to the article and approved the submitted version.
Funding
This work was supported by the USC Alfred E. Mann School of Pharmacy and Pharmaceutical Sciences and the John Stauffer Dean’s Chair in Pharmaceutical Sciences at USC to VP.
Conflict of interest
The authors declare that the research was conducted in the absence of any commercial or financial relationships that could be construed as a potential conflict of interest.
Publisher’s note
All claims expressed in this article are solely those of the authors and do not necessarily represent those of their affiliated organizations, or those of the publisher, the editors and the reviewers. Any product that may be evaluated in this article, or claim that may be made by its manufacturer, is not guaranteed or endorsed by the publisher.
References
Aiello, G. L., and Bach-Y-Rita, P. (2000). The cost of an action potential. J. Neurosci. Methods 103, 145–149. doi: 10.1016/s0165-0270(00)00308-3
Akk, G., Bracamontes, J., and Steinbach, J. H. (2001). Pregnenolone sulfate block of GABAA receptors: Mechanism and involvement of a residue in the M2 region of the α subunit. J. Physiol. 532, 673–684.
Arbo, B. D., Hoppe, J. B., Rodrigues, K., Garcia-Segura, L. M., Salbego, C. G., and Ribeiro, M. F. (2017a). 4’-Chlorodiazepam is neuroprotective against amyloid-beta in organotypic hippocampal cultures. J. Steroid Biochem. Mol. Biol. 171, 281–287. doi: 10.1016/j.jsbmb.2017.04.010
Arbo, B. D., Vieira-Marques, C., Ruiz-Palmero, I., Ortiz-Rodriguez, A., Arevalo, M. A., Garcia-Segura, L. M., et al. (2017b). 4′-Chlorodiazepam modulates the development of primary hippocampal neurons in a sex-dependent manner. Neurosci. Lett. 639, 98–102. doi: 10.1016/j.neulet.2016.12.067
Azarashvili, T., Krestinina, O., Baburina, Y., Odinokova, I., Akatov, V., Beletsky, I., et al. (2016). Effect of the CRAC Peptide, VLNYYVW, on mPTP opening in rat brain and liver mitochondria. Int. J. Mol. Sci. 17:2096. doi: 10.3390/ijms17122096
Bader, S., Wolf, L., Milenkovic, V. M., Gruber, M., Nothdurfter, C., Rupprecht, R., et al. (2019). Differential effects of TSPO ligands on mitochondrial function in mouse microglia cells. Psychoneuroendocrinology 106, 65–76. doi: 10.1016/j.psyneuen.2019.03.029
Banati, R. B., Middleton, R. J., Chan, R., Hatty, C. R., Wai-Ying Kam, W., Quin, C., et al. (2014). Positron emission tomography and functional characterization of a complete PBR/TSPO knockout. Nat. Commun. 5:5452. doi: 10.1038/NCOMMS6452
Barron, A. M., Garcia-Segura, L. M., Caruso, D., Jayaraman, A., Lee, J. W., Melcangi, R. C., et al. (2013). Ligand for translocator protein reverses pathology in a mouse model of Alzheimer’s disease. J. Neurosci. 33, 8891–8897. doi: 10.1523/JNEUROSCI.1350-13.2013
Barron, A. M., Ji, B., Kito, S., Suhara, T., and Higuchi, M. (2018). Steroidogenic abnormalities in translocator protein knockout mice and significance in the aging male. Biochem. J. 475, 75–85. doi: 10.1042/BCJ20170645
Batarseh, A., and Papadopoulos, V. (2010). Regulation of translocator protein 18kDa (TSPO) expression in health and disease states. Mol. Cell Endocrinol. 327, 1–12. doi: 10.1016/j.mce.2010.06.013
Berghoff, S. A., Spieth, L., Sun, T., Hosang, L., Schlaphoff, L., Depp, C., et al. (2021). Microglia facilitate repair of demyelinated lesions via post-squalene sterol synthesis. Nat. Neurosci. 24, 47–60. doi: 10.1038/s41593-020-00757-6
Berndt, N., and Holzhütter, H. G. (2013). The high energy demand of neuronal cells caused by passive leak currents is not a waste of energy. Cell Biochem. Biophys. 67, 527–535. doi: 10.1007/s12013-013-9538-3
Berroterán-Infante, N., Tadić, M., Hacker, M., Wadsak, W., and Mitterhauser, M. (2019). Binding affinity of some endogenous and synthetic TSPO ligands regarding the rs6971 polymorphism. Int. J. Mol. Sci. 20:563. doi: 10.3390/IJMS20030563
Betlazar, C., Harrison-Brown, M., Middleton, R. J., Banati, R., and Liu, G. J. (2018). Cellular sources and regional variations in the expression of the neuroinflammatory marker translocator protein (TSPO) in the normal brain. Int. J. Mol. Sci. 19:2707. doi: 10.3390/ijms19092707
Biswas, L., Farhan, F., Reilly, J., Bartholomew, C., and Shu, X. (2018). TSPO ligands promote cholesterol efflux and suppress oxidative stress and inflammation in choroidal endothelial cells. Int. J. Mol. Sci. 19:3740. doi: 10.3390/IJMS19123740
Bloms-Funke, P., Schumacher, M., Liu, S., Su, D., Li, J., Liere, P., et al. (2022). A novel dual mode-of-action anti-hyperalgesic compound in rats which is neuroprotective and promotes neuroregeneration. Eur. J. Pharmacol. 923:174935. doi: 10.1016/j.ejphar.2022.174935
Bormann, J. (1991). Electrophysiological characterization of diazepam binding inhibitor (DBI) on GABAa receptors. Neuropharmacology 30, 1387–1389. doi: 10.1016/S0028-3908(11)80006-7
Bulua, A. C., Simon, A., Maddipati, R., Pelletier, M., Park, H., Kim, K. Y., et al. (2011). Mitochondrial reactive oxygen species promote production of proinflammatory cytokines and are elevated in TNFR1-associated periodic syndrome (TRAPS). J. Exp. Med. 208, 519–533. doi: 10.1084/JEM.20102049
Cai, W., Dai, X., Chen, J., Zhao, J., Xu, M., Zhang, L., et al. (2019). STAT6/Arg1 promotes microglia/macrophage efferocytosis and inflammation resolution in stroke mice. JCI Insight 4:e131355. doi: 10.1172/JCI.INSIGHT.131355
Camara, A. K. S., Zhou, Y. F., Wen, P. C., Tajkhorshid, E., and Kwok, W. M. (2017). Mitochondrial VDAC1: A key gatekeeper as potential therapeutic target. Front. Physiol. 8:460. doi: 10.3389/fphys.2017.00460
Cameron, H. A., and Mckay, R. D. G. (2001). Adult neurogenesis produces a large pool of new granule cells in the dentate gyrus. J. Comp. Neurol. 435, 406–417. doi: 10.1002/cne.1040
Chen, F., Lu, H., Chen, P., Zhao, X., Guan, X., Liang, Q., et al. (2019). Acute effects of the translocator protein drug ligand FGIN-1-27 on serum testosterone and luteinizing hormone levels in male Sprague-Dawley rats. Biol. Reprod. 100, 824–832. doi: 10.1093/biolre/ioy220
Cherry, J. D., Olschowka, J. A., and O’Banion, M. K. (2014). Neuroinflammation and M2 microglia: The good, the bad, and the inflamed. J. Neuroinflammation 11:98. doi: 10.1186/1742-2094-11-98
Christensen, A., and Pike, C. J. (2018). TSPO ligand PK11195 improves Alzheimer-related outcomes in aged female 3xTg-AD mice. Neurosci. Lett. 683, 7–12. doi: 10.1016/j.neulet.2018.06.029
Chung, J. Y., Chen, H., Midzak, A., Burnett, A. L., Papadopoulos, V., and Zirkin, B. R. (2013). Drug ligand-induced activation of translocator protein (TSPO) stimulates steroid production by aged Brown Norway rat Leydig cells. Endocrinology 154, 2156–2165. doi: 10.1210/en.2012-2226
Corsi, F., Baglini, E., Barresi, E., Salerno, S., Cerri, C., Martini, C., et al. (2022). Targeting TSPO reduces inflammation and apoptosis in an in vitro photoreceptor-like model of retinal degeneration. ACS Chem. Neurosci. 13, 3188–3197. doi: 10.1021/acschemneuro.2c00582
Costa, B., Cavallini, C., Da Pozzo, E., Taliani, S., Da Settimo, F., and Martini, C. (2017). The Anxiolytic etifoxine binds to TSPO Ro5-4864 binding site with long residence time showing a high neurosteroidogenic activity. ACS Chem. Neurosci. 8, 1448–1454. doi: 10.1021/acschemneuro.7b00027
Costa, B., Da Pozzo, E., Giacomelli, C., Barresi, E., Taliani, S., Da Settimo, F., et al. (2016). TSPO ligand residence time: A new parameter to predict compound neurosteroidogenic efficacy. Sci. Rep. 6:18164. doi: 10.1038/srep18164
Cunha, M. I., Su, M., Cantuti-Castelvetri, L., Müller, S. A., Schifferer, M., Djannatian, M., et al. (2020). Pro-inflammatory activation following demyelination is required for myelin clearance and oligodendrogenesis. J. Exp. Med. 217:e20191390. doi: 10.1084/jem.20191390
Da Pozzo, E., Giacomelli, C., Costa, B., Cavallini, C., Taliani, S., Barresi, E., et al. (2016). TSPO PIGA ligands promote neurosteroidogenesis and human astrocyte well-being. Int. J. Mol. Sci. 17:1028. doi: 10.3390/ijms17071028
da Pozzo, E., Tremolanti, C., Costa, B., Giacomelli, C., Milenkovic, V. M., Bader, S., et al. (2019). Microglial pro-inflammatory and anti-inflammatory phenotypes are modulated by translocator protein activation. Int. J. Mol. Sci. 20:4467. doi: 10.3390/ijms20184467
da Settimo, F., Simorini, F., Taliani, S., la Motta, C., Marini, A. M., Salerno, S., et al. (2008). Anxiolytic-like effects of N,N-dialkyl-2-phenylindol-3-ylglyoxylamides by modulation of translocator protein promoting neurosteroid biosynthesis. J. Med. Chem. 51, 5798–5806. doi: 10.1021/jm8003224
Daniela Femminella, G., Dani, M., Wood, M., Fan, Z., Calsolaro, V., Atkinson, R., et al. (2019). Microglial activation in early Alzheimer trajectory is associated with higher gray matter volume. Neurology 92, e1331–e1343. doi: 10.1212/WNL.0000000000007133
Daugherty, D. J., Selvaraj, V., Chechneva, O. V., Liu, X. B., Pleasure, D. E., and Deng, W. (2013). A TSPO ligand is protective in a mouse model of multiple sclerosis. EMBO Mol. Med. 5, 891–903. doi: 10.1002/EMMM.201202124
Dayer, A. G., Ford, A. A., Cleaver, K. M., Yassaee, M., and Cameron, H. A. (2003). Short-term and long-term survival of new neurons in the rat dentate gyrus. J. Comp. Neurol. 460, 563–572. doi: 10.1002/cne.10675
Dheen, S. T., Kaur, C., and Ling, E.-A. (2007). Microglial activation and its implications in the brain diseases. Curr. Med. Chem. 14, 1189–1197. doi: 10.2174/092986707780597961
Díaz, N. F., Díaz-Martínez, N. E., Velasco, I., and Camacho-Arroyo, I. (2009). Progesterone increases dopamine neurone number in differentiating mouse embryonic stem cells. J. Neuroendocrinol. 21, 730–736. doi: 10.1111/j.1365-2826.2009.01891.x
Dumitru, I., Neitz, A., Alfonso, J., and Monyer, H. (2017). Diazepam binding inhibitor promotes stem cell expansion controlling environment-dependent neurogenesis. Neuron 94, 125–137.e5. doi: 10.1016/j.neuron.2017.03.003
Edison, P., Donat, C. K., and Sastre, M. (2018). In vivo imaging of glial activation in alzheimer’s disease. Front. Neurol. 9:625. doi: 10.3389/fneur.2018.00625
Everlien, I., Yen, T. Y., Liu, Y. C., di Marco, B., Vázquez-Marín, J., Centanin, L., et al. (2022). Diazepam binding inhibitor governs neurogenesis of excitatory and inhibitory neurons during embryonic development via GABA signaling. Neuron 10, 3139–3153.e6. doi: 10.1016/j.neuron.2022.07.022
Fairley, L. H., Lai, K. O., Wong, J. H., Chong, W. J., Vincent, A. S., D’Agostino, G., et al. (2023). Mitochondrial control of microglial phagocytosis by the translocator protein and hexokinase 2 in Alzheimer’s disease. Proc. Natl. Acad. Sci. U. S. A. 120:e2209177120. doi: 10.1073/pnas.2209177120
Fan, J., and Papadopoulos, V. (2013). Evolutionary origin of the mitochondrial cholesterol transport machinery reveals a universal mechanism of steroid hormone biosynthesis in animals. PLoS One 8:e76701. doi: 10.1371/journal.pone.0076701
Fan, J., Wang, K., Zirkin, B., and Papadopoulos, V. (2018). CRISPR/Cas9-Mediated tspo gene mutations lead to reduced mitochondrial membrane potential and steroid formation in MA-10 mouse tumor leydig cells. Endocrinology 159, 1130–1146. doi: 10.1210/en.2017-03065
Farhan, F., Almarhoun, M., Wong, A., Findlay, A. S., Bartholomew, C., Williams, M. T. S., et al. (2021). Deletion of TSPO causes dysregulation of cholesterol metabolism in mouse retina. Cells 10:3066. doi: 10.3390/cells10113066
Ferzaz, B., Brault, E., Bourliaud, G., Robert, J.-P., Poughon, G., Claustre, Y., et al. (2002). SSR180575 (7-Chloro-N,N,5-trimethyl-4-oxo-3-phenyl-3,5-dihydro-4H-pyridazino[4,5-b]indole-1-acetamide), a peripheral benzodiazepine receptor ligand, promotes neuronal survival and repair. J. Pharmacol. Exp. Ther. 301, 1068–1078. doi: 10.1124/jpet.301.3.1067
Franco, R., and Fernández-Suárez, D. (2015). Alternatively activated microglia and macrophages in the central nervous system. Prog. Neurobiol. 131, 65–86. doi: 10.1016/J.PNEUROBIO.2015.05.003
Frison, M., Faccenda, D., Abeti, R., Rigon, M., Strobbe, D., England-Rendon, B. S., et al. (2021). The translocator protein (TSPO) is prodromal to mitophagy loss in neurotoxicity. Mol. Psychiatry 26, 2721–2739. doi: 10.1038/s41380-021-01050-z
Garnier, M., Boujrad, N., Ogwuegbu, S. O., Hudson, J. R., and Papadopoulos, V. (1994). The polypeptide diazepam-binding inhibitor and a higher affinity mitochondrial peripheral-type benzodiazepine receptor sustain constitutive steroidogenesis in the R2C Leydig tumor cell line. J. Biol. Chem. 269, 22105–22112. doi: 10.1016/s0021-9258(17)31762-3
Garza, S., Chen, L., Galano, M., Cheung, G., Sottas, C., Li, L., et al. (2022). Mitochondrial dynamics, Leydig cell function, and age-related testosterone deficiency. FASEB J. 36:e22637. doi: 10.1096/fj.202201026R
Gatliff, J., East, D., Crosby, J., Abeti, R., Harvey, R., Craigen, W., et al. (2014). TSPO interacts with VDAC1 and triggers a ROS-mediated inhibition of mitochondrial quality control. Autophagy 10, 2279–2296. doi: 10.4161/15548627.2014.991665
Gatliff, J., East, D. A., Singh, A., Alvarez, M. S., Frison, M., Matic, I., et al. (2017). A role for TSPO in mitochondrial Ca2+ homeostasis and redox stress signaling. Cell Death Dis. 8:e2896. doi: 10.1038/CDDIS.2017.186
Girard, C., Liu, S., Cadepond, F., Adams, D., Lacroix, C., Verleye, M., et al. (2008). Etifoxine improves peripheral nerve regeneration and functional recovery. Proc. Natl. Acad. Sci. U. S. A. 105, 20505–20510.
González-Blanco, L., Bermejo-Millo, J. C., Oliveira, G., Potes, Y., Antuña, E., Menéndez-Valle, I., et al. (2021). Neurogenic potential of the 18-kda mitochondrial translocator protein (TSPO) in pluripotent P19 stem cells. Cells 10:2784. doi: 10.3390/cells10102784
Grimm, A., Lejri, I., Hallé, F., Schmitt, M., Götz, J., Bihel, F., et al. (2020). Mitochondria modulatory effects of new TSPO ligands in a cellular model of tauopathies. J. Neuroendocrinol. 32:e12796. doi: 10.1111/jne.12796
Grinstein, S., Swallow, C. J., and Rotstein, O. D. (1991). Regulation of cytoplasmic pH in phagocytic cell function and dysfunction. Clin. Biochem. 24, 241–247. doi: 10.1016/0009-9120(91)80014-t
Guo, S., Wang, H., and Yin, Y. (2022). Microglia Polarization From M1 to M2 in Neurodegenerative Diseases. Front. Aging Neurosci. 14:815347. doi: 10.3389/fnagi.2022.815347
Hallé, F., Lejri, I., Abarghaz, M., Grimm, A., Klein, C., Maitre, M., et al. (2017). Discovery of imidazoquinazolinone derivatives as TSPO ligands modulating neurosteroidogenesis and cellular bioenergetics in neuroblastoma cells expressing amyloid precursor protein. ChemistrySelect 2, 6452–6457. doi: 10.1002/slct.201701565
Hamelin, L., Lagarde, J., Dorothée, G., Leroy, C., Labit, M., Comley, R. A., et al. (2016). Early and protective microglial activation in Alzheimer’s disease: A prospective study using 18 F-DPA-714 PET imaging. Brain 139, 1252–1264. doi: 10.1093/BRAIN/AWW017
Haydar, T. F., Wang, F., Schwartz, M. L., and Rakic, P. (2000). Differential modulation of proliferation in the neocortical ventricular and subventricular zones. J. Neurosci. 20, 5764–5774.
Hosie, A. M., Wilkins, M. E., and Smart, T. G. (2007). Neurosteroid binding sites on GABAA receptors. Pharmacol. Ther. 116, 7–19. doi: 10.1016/j.pharmthera.2007.03.011
Huebner, E. A., and Strittmatter, S. M. (2009). Axon regeneration in the peripheral and central nervous systems. Results Probl. Cell Differ. 48, 339–351. doi: 10.1007/400_2009_19
Jaipuria, G., Leonov, A., Giller, K., Vasa, S. K., Jaremko, Á, Jaremko, M., et al. (2017). Cholesterol-mediated allosteric regulation of the mitochondrial translocator protein structure. Nat. Commun. 8:14893. doi: 10.1038/ncomms14893
James, M. L., Belichenko, N. P., Shuhendler, A. J., Hoehne, A., Andrews, L. E., Condon, C., et al. (2017). [18F]GE-180 PET detects reduced microglia activation after LM11A-31 therapy in a mouse model of Alzheimer’s disease. Theranostics 7, 1422–1436. doi: 10.7150/thno.17666
Jamin, N., Neumann, J. M., Ostuni, M. A., Vu, T. K. N., Yao, Z. X., Murail, S., et al. (2005). Characterization of the cholesterol recognition amino acid consensus sequence of the peripheral-type benzodiazepine receptor. Mol. Endocrinol. 19, 588–594. doi: 10.1210/ME.2004-0308
Janssen, B., Vugts, D. J., Windhorst, A. D., and Mach, R. H. (2018). PET imaging of microglial activation - Beyond targeting TSPO. Molecules 23:607. doi: 10.3390/molecules23030607
Karlstetter, M., Nothdurfter, C., Aslanidis, A., Moeller, K., Horn, F., Scholz, R., et al. (2014). Translocator protein (18 kDa) (TSPO) is expressed in reactive retinal microglia and modulates microglial inflammation and phagocytosis. J. Neuroinflammation 11:3. doi: 10.1186/1742-2094-11-3
Kita, A., Kohayakawa, H., Kinoshita, T., Ochi, Y., Nakamichi, K., Kurumiya, S., et al. (2004). Antianxiety and antidepressant-like effects of AC-5216, a novel mitochondrial benzodiazepine receptor ligand. Br. J. Pharmacol. 142, 1059–1072. doi: 10.1038/sj.bjp.0705681
Kokate, T. G., Svensson, B. E., and Rogawski, M. A. (1994). Anticonvulsant activity of neurosteroids: Correlation with ’y-aminobutyric acid-evoked chloride current potentiation. J. Pharmacol. Exp. Ther. 270, 1223–1229.
Kreisl, W. C., Jenko, K. J., Hines, C. S., Hyoung Lyoo, C., Corona, W., Morse, C. L., et al. (2013). A genetic polymorphism for translocator protein 18 kDa affects both in vitro and in vivo radioligand binding in human brain to this putative biomarker of neuroinflammation. J. Cereb. Blood Flow Metab. 33, 53–58. doi: 10.1038/jcbfm.2012.131
Krestinina, O. V., Myakisheva, S. N., Baburina, Y. L., Fadeev, R. S., Azarashvili, T. S., and Akatov, V. S. (2017). The effects of isoquinoline carboxamide and melatonin on the differentiation of N1Å-115 mouse neuroblastoma cells (clone C-1300) and on the expression of the TSPO translocation protein and 2’,3’-cyclonucleotide-3’-phosphodiesterase in these cells. Neurochem. J. 11, 31–37. doi: 10.1134/S1819712417010044
Krueger, K. E., and Papadopoulos, V. (1990). Peripheral-type benzodiazepine receptors mediate translocation of cholesterol from outer to inner mitochondrial membranes in adrenocortical cells. J. Biol. Chem. 265, 15015–15022. doi: 10.1016/s0021-9258(18)77217-7
Lacapère, J. J., Delavoie, F., Li, H., Péranzi, G., Maccario, J., Papadopoulos, V., et al. (2001). Structural and functional study of reconstituted peripheral benzodiazepine receptor. Biochem. Biophys. Res. Commun. 284, 536–541. doi: 10.1006/bbrc.2001.4975
Lampron, A., Larochelle, A., Laflamme, N., Préfontaine, P., Plante, M. M., Sánchez, M. G., et al. (2015). Inefficient clearance of myelin debris by microglia impairs remyelinating processes. J. Exp. Med. 212, 481–495. doi: 10.1084/jem.20141656
Lan, N., Liu, Y., Juan, Z., Zhang, R., Ma, B., Xie, K., et al. (2021). The TSPO-specific ligand PK11195 protects against LPS-induced cognitive dysfunction by inhibiting cellular autophagy. Front. Pharmacol. 11:615543. doi: 10.3389/fphar.2020.615543
Leaver, K. R., Reynolds, A., Bodard, S., Guilloteau, D., Chalon, S., and Kassiou, M. (2012). Effects of translocator protein (18 kDa) ligands on microglial activation and neuronal death in the quinolinic-acid-injected rat striatum. ACS Chem. Neurosci. 3, 114–119. doi: 10.1021/cn200099e
Lejri, I., Grimm, A., Hallé, F., Abarghaz, M., Klein, C., Maitre, M., et al. (2019). TSPO ligands boost mitochondrial function and pregnenolone synthesis. J. Alzheimers Dis. 72:1045. doi: 10.3233/JAD-190127
Leva, G., Klein, C., Benyounes, J., Hallé, F., Bihel, F., Collongues, N., et al. (2017). The translocator protein ligand XBD173 improves clinical symptoms and neuropathological markers in the SJL/J mouse model of multiple sclerosis. Biochim. Biophys. Acta Mol. Basis Dis. 1863, 3016–3027. doi: 10.1016/j.bbadis.2017.09.007
Li, F., Liu, J., Valls, L., Hiser, C., and Ferguson-Miller, S. (2015). Identification of a key cholesterol binding enhancement motif in translocator protein 18 kDa. Biochemistry 54, 1441–1443. doi: 10.1021/bi5015453
Li, H., and Papadopoulos, V. (1998). Peripheral-Type Benzodiazepine Receptor Function in Cholesterol Transport. Identification of a Putative Cholesterol Recognition/Interaction Amino Acid Sequence and Consensus Pattern. Endocrinology 139, 4991–4997. doi: 10.1210/ENDO.139.12.6390
Li, H., Yao, Z. X., Degenhardt, B., Teper, G., and Papadopoulos, V. (2001). Cholesterol binding at the cholesterol recognition/interaction amino acid consensus (CRAC) of the peripheral-type benzodiazepine receptor and inhibition of steroidogenesis by an HIV TAT-CRAC peptide. Proc. Natl. Acad. Sci. U. S. A. 98, 1267–1272. doi: 10.1073/pnas.98.3.1267
Li, J., Xiao, J., Liang, D., Zhang, H., Zhang, G., Liu, Y., et al. (2010). Inhibition of mitochondrial translocator protein prevents atrial fibrillation. Eur. J. Pharmacol. 632, 60–64. doi: 10.1016/j.ejphar.2010.01.014
Li, X. B., Liu, A., Yang, L., Zhang, K., Wu, Y. M., Zhao, M. G., et al. (2018). Antidepressant-like effects of translocator protein (18 kDa) ligand ZBD-2 in mouse models of postpartum depression Tim Bliss. Mol. Brain 11:12. doi: 10.1186/s13041-018-0355-x
Li, Y., Chen, L., Li, L., and Stiles, B. (2021). Cholesterol-binding translocator protein TSPO regulates steatosis and bile acid synthesis in nonalcoholic fatty liver disease. iScience 24:102457. doi: 10.1016/j.isci.2021.102457
Liere, P., Pianos, A., Oudinet, J. P., Schumacher, M., and Akwa, Y. (2017). Differential effects of the 18-kDa translocator protein (TSPO) ligand etifoxine on steroidogenesis in rat brain, plasma and steroidogenic glands: Pharmacodynamic studies. Psychoneuroendocrinology 83, 122–134. doi: 10.1016/j.psyneuen.2017.05.022
Lima-Maximino, M. G., Cueto-Escobedo, J., Rodríguez-Landa, J. F., and Maximino, C. (2018). FGIN-1-27, an agonist at translocator protein 18 kDa (TSPO), produces anti-anxiety and anti-panic effects in non-mammalian models. Pharmacol. Biochem. Behav. 171, 66–73. doi: 10.1016/j.pbb.2018.04.007
Lin, Y. C., Cheung, G., Porter, E., and Papadopoulos, V. (2022). The neurosteroid pregnenolone is synthesized by a mitochondrial P450 enzyme other than CYP11A1 in human glial cells. J. Biol. Chem. 298:102110. doi: 10.1016/j.jbc.2022.102110
Liu, G., Fiala, M., Mizwicki, M. T., Sayre, J., Magpantay, L., Siani, A., et al. (2012). Neuronal phagocytosis by inflammatory macrophages in ALS spinal cord: Inhibition of inflammation by resolvin D1. Am. J. Neurodegener. Dis. 1, 60–74.
Liu, G. J., Middleton, R. J., Kam, W. W. Y., Chin, D. Y., Hatty, C. R., Chan, R. H. Y., et al. (2017). Functional gains in energy and cell metabolism after TSPO gene insertion. Cell Cycle 16:436. doi: 10.1080/15384101.2017.1281477
Liu, X., Wang, Q., Haydar, T. F., and Bordey, A. (2005). Nonsynaptic GABA signaling in postnatal subventricular zone controls GFAP-expressing progenitor proliferation. Nat. Neurosci. 8, 1179–1187.
Lloyd, A. F., Davies, C. L., Holloway, R. K., Labrak, Y., Ireland, G., Carradori, D., et al. (2019). Central nervous system regeneration is driven by microglia necroptosis and repopulation. Nat. Neurosci. 7, 1046–1052. doi: 10.1038/s41593-019-0418-z
Longone, P., di Michele, F., D’Agati, E., Romeo, E., Pasini, A., and Rupprecht, R. (2011). Neurosteroids as neuromodulators in the treatment of anxiety disorders. Front. Endocrinol. 2:55. doi: 10.3389/fendo.2011.00055
Ma, B., Liu, X., Huang, X., Ji, Y., Jin, T., and Ma, K. (2018). Translocator protein agonist Ro5-4864 alleviates neuropathic pain and promotes remyelination in the sciatic nerve. Mol. Pain 14:1744806917748019. doi: 10.1177/1744806917748019
Ma, L., Zhang, H., Liu, N., Wang, P., Guo, W., Fu, Q., et al. (2016). TSPO ligand PK11195 alleviates neuroinflammation and beta-amyloid generation induced by systemic LPS administration. Brain Res. Bull. 121, 192–200. doi: 10.1016/j.brainresbull.2016.02.001
Maaser, K., Sutter, A. P., and Scherübl, H. (2005). Mechanisms of mitochondrial apoptosis induced by peripheral benzodiazepine receptor ligands in human colorectal cancer cells. Biochem. Biophys. Res. Commun. 332, 646–652. doi: 10.1016/j.bbrc.2005.05.005
Magrì, A., Lipari, C. L. R., Risiglione, P., Zimbone, S., Guarino, F., Caccamo, A., et al. (2023). ERK1/2-dependent TSPO overactivation associates with the loss of mitophagy and mitochondrial respiration in ALS. Cell Death Dis. 14:122. doi: 10.1038/s41419-023-05643-0
Marangos, P. J., Patel, J., Boulenger, J.-P., and Clark-Rosenberg’, R. (1982). Characterization of Peripheral-Type Benzodiazepine Binding Sites in Brain Using [3H]Ro 5-4864. Mol. Pharmacol. 22, 26–32.
Marx, C. E., Keefe, R. S. E., Buchanan, R. W., Hamer, R. M., Kilts, J. D., Bradford, D. W., et al. (2009). Proof-of-concept trial with the neurosteroid pregnenolone targeting cognitive and negative symptoms in schizophrenia. Neuropsychopharmacology 34, 1885–1903. doi: 10.1038/npp.2009.26
Mcgeer, P. L., Itagaki, S., Boyes, B. E., and Mcgeer, E. G. (1988). Reactive microglia are positive for HLA-DR in the substantia nigra of Parkinson’s and Alzheimer’s disease brains. Neurology 38, 1285–1291. doi: 10.1212/wnl.38.8.1285
Mendonça-Torres, M. C., and Roberts, S. S. (2013). The translocator protein (TSPO) ligand PK11195 induces apoptosis and cell cycle arrest and sensitizes to chemotherapy treatment in pre- and post-relapse neuroblastoma cell lines. Cancer Biol. Ther. 14, 319–326. doi: 10.4161/cbt.23613
Meng, Y., Tian, M., Yin, S., Lai, S., Zhou, Y., Chen, J., et al. (2020). Downregulation of TSPO expression inhibits oxidative stress and maintains mitochondrial homeostasis in cardiomyocytes subjected to anoxia/reoxygenation injury. Biomed. Pharmacother. 121:109588. doi: 10.1016/J.BIOPHA.2019.109588
Milenkovic, V. M., Slim, D., Bader, S., Koch, V., Heinl, E. S., Alvarez-Carbonell, D., et al. (2019). CRISPR-cas9 mediated TSPO gene knockout alters respiration and cellular metabolism in human primary microglia cells. Int. J. Mol. Sci. 20:3359. doi: 10.3390/ijms20133359
Mills, C., Makwana, M., Wallace, A., Benn, S., Schmidt, H., Tegeder, I., et al. (2008). Ro5-4864 promotes neonatal motor neuron survival and nerve regeneration in adult rats. Eur. J. Neurosci. 27, 937–946. doi: 10.1111/j.1460-9568.2008.06065.x
Morohaku, K., Pelton, S. H., Daugherty, D. J., Butler, W. R., Deng, W., and Selvaraj, V. (2014). Translocator protein/peripheral benzodiazepine receptor is not required for steroid hormone biosynthesis. Endocrinology 155, 89–97. doi: 10.1210/EN.2013-1556
Nguyen, N., Fakra, E., Pradel, V., Jouve, E., Alquier, C., le Guern, M. E., et al. (2006). Efficacy of etifoxine compared to lorazepam monotherapy in the treatment of patients with adjustment disroders with anxiety: A double-blind controlled study in general practice. Hum. Psychopharmacol. 21, 139–149. doi: 10.1002/hup.757
Notter, T., Schalbetter, S. M., Clifton, N. E., Mattei, D., Richetto, J., Thomas, K., et al. (2020). Neuronal activity increases translocator protein (TSPO) levels. Mol. Psychiatry 26, 2025–2037. doi: 10.1038/s41380-020-0745-1
Nutma, E., Ceyzériat, K., Amor, S., Tsartsalis, S., Millet, P., Owen, D. R., et al. (2021). Cellular sources of TSPO expression in healthy and diseased brain. Eur. J. Nucl. Med. Mol. Imaging 49, 146–163. doi: 10.1007/s00259-020-05166-2
Olson, J. K., and Miller, S. D. (2004). Microglia initiate central nervous system innate and adaptive immune responses through multiple TLRs. J. Immunol. 173, 3916–3924. doi: 10.4049/JIMMUNOL.173.6.3916
Owen, D. R., Fan, J., Campioli, E., Venugopal, S., Midzak, A., Daly, E., et al. (2017). TSPO mutations in rats and a human polymorphism impair the rate of steroid synthesis. Biochem. J. 474, 3985–3999. doi: 10.1042/BCJ20170648
Owen, D. R., Phillips, A., O’Connor, D., Grey, G., Aimola, L., Nicholas, R., et al. (2022). Human pharmacokinetics of XBD173 and etifoxine distinguish their potential for pharmacodynamic effects mediated by translocator protein. Br. J. Clin. Pharmacol. 88, 4230–4236. doi: 10.1111/bcp.15392
Palzur, E., Edelman, D., Sakas, R., and Soustiel, J. F. (2021). Etifoxine restores mitochondrial oxidative phosphorylation and improves cognitive recovery following traumatic brain injury. Int. J. Mol. Sci. 22:12881. doi: 10.3390/ijms222312881
Palzur, E., Sharon, A., Shehadeh, M., and Soustiel, J. F. (2016). Investigation of the mechanisms of neuroprotection mediated by Ro5-4864 in brain injury. Neuroscience 329, 162–170. doi: 10.1016/j.neuroscience.2016.05.014
Papadopoulos, V., Aghazadeh, Y., Fan, J., Campioli, E., Zirkin, B., and Midzak, A. (2015). Translocator protein-mediated pharmacology of cholesterol transport and steroidogenesis. Mol. Cell Endocrinol. 408, 90–98. doi: 10.1016/J.MCE.2015.03.014
Papadopoulos, V., Baraldi, M., Guilarte, T. R., Knudsen, T. B., Lacapère, J. J., Lindemann, P., et al. (2006). Translocator protein (18 kDa): New nomenclature for the peripheral-type benzodiazepine receptor based on its structure and molecular function. Trends Pharmacol. Sci. 27, 402–409. doi: 10.1016/j.tips.2006.06.005
Papadopoulos, V., Berkovtch, A., and Krueger, K. E. (1991). The role of diazepam binding inhibitor and its processing products at mitochondrial benzodiazepine receptors: Regulation of steroid biosynthesis. Neuropharmacology 30, 1417–1423. doi: 10.1016/s0028-3908(11)80011-0
Papadopoulos, V., Fan, J., and Zirkin, B. (2018). Translocator protein (18 kDa): An update on its function in steroidogenesis. J. Neuroendocrinol. 30:e12500. doi: 10.1111/jne.12500
Papadopoulos, V., Guarneri, P., Krueger, K. E., Guidotti, A., and Costa, E. (1992). Pregnenolone biosynthesis in C6-2B glioma cell mitochondria: Regulation by a mitochondrial diazepam binding inhibitor receptor. Proc. Natl. Acad. Sci. U. S. A. 89, 5113–5117. doi: 10.1073/pnas.89.11.5113
Papadopoulos, V., Mukhinyl, A. G., Costa, E., and Krueger, K. (1990). The peripheral-type benzodiazepine receptor is functionally linked to leydig cell steroidogenesis. J. Biol. Chem. 265, 3772–3779. doi: 10.1016/S0021-9258(19)39661-9
Porcu, P., Barron, A. M., Frye, C. A., Walf, A. A., Yang, S. Y., He, X. Y., et al. (2016). Neurosteroidogenesis today: Novel targets for neuroactive steroid synthesis and action and their relevance for translational research. J. Neuroendocrinol. 28, 1–19. doi: 10.1111/jne.12351
Prossin, A. R., Chandler, M., Ryan, K. A., Saunders, E. F., Kamali, M., Papadopoulos, V., et al. (2018). Functional TSPO polymorphism predicts variance in the diurnal cortisol rhythm in bipolar disorder. Psychoneuroendocrinology 89, 194–202. doi: 10.1016/j.psyneuen.2018.01.013
Qiu, Z. K., He, J. L., Liu, X., Zhang, G. H., Zeng, J., Nie, H., et al. (2016). The antidepressant-like activity of AC-5216, a ligand for 18KDa translocator protein (TSPO), in an animal model of diabetes mellitus. Sci. Rep. 6:37345. doi: 10.1038/srep37345
Reddy, D. S., and Rogawski, M. A. (2002). Stress-induced deoxycorticosterone-derived neurosteroids modulate GABA a receptor function and seizure susceptibility. J. Neurosci. 22, 3795–3805.
Rupprecht, R., Rammes, G., Eser, D., Baghai, T. C., Schüle, C., Nothdurfter, C., et al. (2009). Translocator protein (18 kD) as target for anxiolytics without benzodiazepine-like side effects. Science 325, 490–493. doi: 10.1126/science.1172667
Ryu, J. R., Hong, C. J., Kim, J. Y., Kim, E. K., Sun, W., and Yu, S. W. (2016). Control of adult neurogenesis by programmed cell death in the mammalian brain. Mol. Brain 9:43. doi: 10.1186/s13041-016-0224-4
Sakamoto, H., Ukena, K., and Tsutsui, K. (2002). Dendritic spine formation in response to progesterone synthesized de novo in the developing Purkinje cell in rats. Neurosci. Lett. 322, 111–115.
Santidrián, A. F., Cosialls, A. M., Coll-Mulet, L., Iglesias-Serret, D., De Frias, M., González-Gironès, D. M., et al. (2007). The potential anticancer agent PK11195 induces apoptosis irrespective of p53 and ATM status in chronic lymphocytic leukemia cells. Haematologica 92, 1631–1638. doi: 10.3324/HAEMATOL.11194
Santoro, A., Mattace Raso, G., Taliani, S., da Pozzo, E., Simorini, F., Costa, B., et al. (2016). TSPO-ligands prevent oxidative damage and inflammatory response in C6 glioma cells by neurosteroid synthesis. Eur. J. Pharm. Sci. 88, 124–131. doi: 10.1016/j.ejps.2016.04.006
Scarff, J. R. (2019). Use of brexanolone for postpartum depression. Innov. Clin. Neurosci. 16, 32–35.
Scholz, R., Caramoy, A., Bhuckory, M. B., Rashid, K., Chen, M., Xu, H., et al. (2015). Targeting translocator protein (18 kDa) (TSPO) dampens pro-inflammatory microglia reactivity in the retina and protects from degeneration. J. Neuroinflammation 12:201. doi: 10.1186/s12974-015-0422-5
Schuchmann, S., Buchheim, K., Heinemann, U., Hosten, N., and Buttgereit, F. (2005). Oxygen consumption and mitochondrial membrane potential indicate developmental adaptation in energy metabolism of rat cortical neurons. Eur. J. Neurosci. 21, 2721–2732. doi: 10.1111/J.1460-9568.2005.04109.X
Shang, C., Yao, R. M., Guo, Y., Ding, Z. C., Sun, L. J., Ran, Y. H., et al. (2020). Translocator protein–mediated fast-onset antidepressant-like and memory-enhancing effects in chronically stressed mice. J. Psychopharmacol. 34, 441–451. doi: 10.1177/0269881119896304
Shargorodsky, L., Veenman, L., Caballero, B., Pe’er, Y., Leschiner, S., Bode, J., et al. (2012). The nitric oxide donor sodium nitroprusside requires the 18 kDa Translocator Protein to induce cell death. Apoptosis 17, 647–665. doi: 10.1007/s10495-012-0725-2
Shehadeh, M., Palzur, E., Apel, L., and Soustiel, J. F. (2019). Reduction of traumatic brain damage by Tspo ligand etifoxine. Int. J. Mol. Sci. 20:2639. doi: 10.3390/ijms20112639
Shoshan-Barmatz, V., Pittala, S., and Mizrachi, D. (2019). VDAC1 and the TSPO: Expression, interactions, and associated functions in health and disease states. Int. J. Mol. Sci. 20:3348. doi: 10.3390/ijms20133348
Sieger, D., Moritz, C., Ziegenhals, T., Prykhozhij, S., and Peri, F. (2012). Long-range Ca2+ waves transmit brain-damage signals to microglia. Dev. Cell 22, 1138–1148. doi: 10.1016/J.DEVCEL.2012.04.012
Simon-O’Brien, E., Gauthier, D., Riban, V., and Verleye, M. (2016). Etifoxine improves sensorimotor deficits and reduces glial activation, neuronal degeneration, and neuroinflammation in a rat model of traumatic brain injury. J. Neuroinflammation 13:203. doi: 10.1186/s12974-016-0687-3
Stoebner, P., Carayon, P., Casellas, P., Portier, M., Lavabre-Bertrand, T., Cuq, P., et al. (2001). Transient protection by peripheral benzodiazepine receptors during the early events of ultraviolet light-induced apoptosis. Cell Death Differ. 8, 747–753. doi: 10.1038/sj.cdd.4400861
Stouffer, R. L., Nixon, W. E., Gulyas, B. J., Hodgen, G. D., Williams, M. T., Roth, M. S., et al. (1980). Axons from CNS neurones regenerate into PNS grafts. Nature 264, 264–265. doi: 10.1038/284264a0
Tang, Y., and Le, W. (2016). Differential roles of M1 and M2 microglia in neurodegenerative diseases. Mol. Neurobiol. 53, 1181–1194. doi: 10.1007/s12035-014-9070-5
Tu, L. N., Zhao, A. H., Stocco, D. M., and Selvaraj, V. (2015). PK11195 effect on steroidogenesis is not mediated through the translocator protein (TSPO). Endocrinology 156, 1033–1039. doi: 10.1210/en.2014-1707
Urbán, N., and Guillemot, F. (2014). Neurogenesis in the embryonic and adult brain: Same regulators, different roles. Front. Cell Neurosci. 8:396. doi: 10.3389/fncel.2014.00396
Van Der Goes, A., Brouwer, J., Hoekstra, K., Roos, D., Van Den Berg, T. K., and Dijkstra, C. D. (1998). Reactive oxygen species are required for the phagocytosis of myelin by macrophages. J. Neuroimmunol. 1998, 67–75.
Varga, B., Markó, K., Hádinger, N., Jelitai, M., Demeter, K., Tihanyi, K., et al. (2009). Translocator protein (TSPO 18 kDa) is expressed by neural stem and neuronal precursor cells. Neurosci. Lett. 462, 257–262. doi: 10.1016/J.NEULET.2009.06.051
Veenman, L., Shandalov, Y., and Gavish, M. (2008). VDAC activation by the 18 kDa translocator protein (TSPO), implications for apoptosis. J. Bioenerg. Biomembr. 40, 199–205. doi: 10.1007/s10863-008-9142-1
Vettermann, F. J., Harris, S., Schmitt, J., Unterrainer, M., Lindner, S., Rauchmann, B.-S., et al. (2021). Impact of TSPO receptor polymorphism on [18 F]GE-180 binding in healthy brain and pseudo-reference regions of neurooncological and neurodegenerative disorders. Life 11:484. doi: 10.3390/life11060484
Vincent, N., Genin, C., and Malvoisin, E. (2002). Identification of a conserved domain of the HIV-1 transmembrane protein gp41 which interacts with cholesteryl groups. Biochim. Biophys. Acta Biomembr. 1567, 157–164. doi: 10.1016/S0005-2736(02)00611-9
Vojtíšková, A., Ješina, P., Kalous, M., Kaplanová, V., Houštěk, J., Tesařová, M., et al. (2004). Mitochondrial membrane potential and ATP production in primary disorders of ATP synthase. Toxicol. Mech. Methods 14, 7–11. doi: 10.1080/15376520490257347
Wang, D., Han, J., Li, S., Sun, T., Guo, Y., Kang, W., et al. (2017). Antidepressant-like and anxiolytic-like effects of ZBD-2, a novel ligand for the translocator protein (18 kDa). Neuromol. Med. 19, 57–68. doi: 10.1007/s12017-016-8425-7
Wang, D., Tian, Z., Guo, Y., Guo, H., Kang, W., Li, S., et al. (2015). Anxiolytic-like effects of translocator protein (TSPO) ligand ZBD-2 in an animal model of chronic pain. Mol. Pain 11:16. doi: 10.1186/s12990-015-0013-6
Wang, W. Y., Tan, M. S., Yu, J. T., and Tan, L. (2015). Role of pro-inflammatory cytokines released from microglia in Alzheimer’s disease. Ann. Transl. Med. 3:136. doi: 10.3978/j.issn.2305-5839.2015.03.49
Wang, W., Zhang, L., Zhang, X., Xue, R., Li, L., Zhao, W., et al. (2016). Lentiviral-mediated overexpression of the 18 kDa translocator protein (TSPO) in the hippocampal dentate gyrus ameliorates LPS-induced cognitive impairment in mice. Front. Pharmacol. 7:384. doi: 10.3389/FPHAR.2016.00384
Wang, W., Zhao, F., Ma, X., Perry, G., and Zhu, X. (2020). Mitochondria dysfunction in the pathogenesis of Alzheimer’s disease: Recent advances. Mol. Neurodegener. 15:30. doi: 10.1186/s13024-020-00376-6
Wang, X., Wu, H., Xue, G., and Hou, Y. (2012). Progesterone promotes neuronal differentiation of human umbilical cord mesenchymal stem cells in culture conditions that mimic the brain microenvironment. Neural Regen. Res. 7, 1925–1930. doi: 10.3969/j.issn.1673-5374.2012.25.001
Werry, E. L., Bright, F. M., Piguet, O., Ittner, L. M., Halliday, G. M., Hodges, J. R., et al. (2019). Molecular sciences recent developments in TSPO PET imaging as a biomarker of neuroinflammation in neurodegenerative disorders. Int. J. Mol. Sci. 20:3161. doi: 10.3390/ijms20133161
Winner, B., Cooper-Kuhn, C. M., Aigner, R., Winkler, J., and Kuhn, H. G. (2002). Long-term survival and cell death of newly generated neurons in the adult rat olfactory bulb. Eur. J. Neurosci. 16, 1681–1689. doi: 10.1046/j.1460-9568.2002.02238.x
Wu, X., and Gallo, K. A. (2013). The 18-kDa translocator protein (TSPO) disrupts mammary epithelial morphogenesis and promotes breast cancer cell migration. PLoS One 8:e71258. doi: 10.1371/journal.pone.0071258
Xue, X., Duan, R., Zheng, G., Chen, H., Zhang, W., and Shi, L. (2022). Translocator protein (18 kDa) regulates the microglial phenotype in Parkinson’s disease through P47. Bioengineered 13, 11061–11071. doi: 10.1080/21655979.2022.2068754
Yao, R., Pan, R., Shang, C., Li, X., Cheng, J., Xu, J., et al. (2020). Translocator protein 18 kDa (TSPO) deficiency inhibits microglial activation and impairs mitochondrial function. Front. Pharmacol. 11:986. doi: 10.3389/fphar.2020.00986
Yasin, N., Veenman, L., Singh, S., Azrad, M., Bode, J., Vainshtein, A., et al. (2017). Classical and novel TSPO ligands for the mitochondrial TSPO can modulate nuclear gene expression: Implications for mitochondrial retrograde signaling. Int. J. Mol. Sci. 18:786. doi: 10.3390/ijms18040786
Yokokura, M., Terada, T., Bunai, T., Nakaizumi, K., Takebayashi, K., Iwata, Y., et al. (2017). Depiction of microglial activation in aging and dementia: Positron emission tomography with [11 C]DPA713 versus [11 C](R)PK11195. J. Cereb. Blood Flow Metab. 37, 877–889. doi: 10.1177/0271678X16646788
Zhang, H., Wang, H., Gao, F., Yang, J., Xu, Y., Fu, Y., et al. (2021). TSPO deficiency accelerates amyloid pathology and neuroinflammation by impairing microglial phagocytosis. Neurobiol. Aging 106, 292–303. doi: 10.1016/J.NEUROBIOLAGING.2021.06.020
Zhang, X. Y., Wei, W., Zhang, Y. Z., Fu, Q., Mi, W. D., Zhang, L. M., et al. (2018). The 18 kDa translocator protein (TSPO) overexpression in hippocampal dentate gyrus elicits anxiolytic-like effects in a mouse model of post-traumatic stress disorder. Front. Pharmacol. 9:1364. doi: 10.3389/fphar.2018.01364
Zhao, A. H., Tu, L. N., Mukai, C., Sirivelu, M. P., Pillai, V. V., Morohaku, K., et al. (2016). Mitochondrial translocator protein (TSPO) function is not essential for heme biosynthesis. J. Biol. Chem. 291:1591. doi: 10.1074/JBC.M115.686360
Zhou, D., Ji, L., and Chen, Y. (2020). TSPO Modulates IL-4-Induced Microglia/Macrophage M2 Polarization via PPAR-γ Pathway. J. Mol. Neurosci. 70, 542–549. doi: 10.1007/S12031-019-01454-1/FIGURES/5
Zhou, J., Zhang, X., Peng, J., Xie, Y., Du, F., Guo, K., et al. (2020). TSPO ligand Ro5-4864 modulates microglia/macrophages polarization after subarachnoid hemorrhage in mice. Neurosci. Lett. 729:134977. doi: 10.1016/j.neulet.2020.134977
Zhou, R., Ji, B., Kong, Y., Qin, L., Ren, W., Guan, Y., et al. (2021). PET Imaging of Neuroinflammation in Alzheimer’s Disease. Front. Immunol. 12:3750. doi: 10.3389/FIMMU.2021.739130/BIBTEX
Zimmer, E. R., Leuzy, A., Benedet, A. L., Breitner, J., Gauthier, S., and Rosa-Neto, P. (2014). Tracking neuroinflammation in Alzheimer’s disease: The role of positron emission tomography imaging. J. Neuroinflammation 11:120. doi: 10.1186/1742-2094-11-120
Keywords: neurogenesis, neurodegeneration, mitochondria, ligands, 18 kDa translocator protein (TSPO)
Citation: Cheung G, Lin YC and Papadopoulos V (2023) Translocator protein in the rise and fall of central nervous system neurons. Front. Cell. Neurosci. 17:1210205. doi: 10.3389/fncel.2023.1210205
Received: 21 April 2023; Accepted: 07 June 2023;
Published: 21 June 2023.
Edited by:
Barbara Monti, University of Bologna, ItalyReviewed by:
Patrizia Longone, Santa Lucia Foundation (IRCCS), ItalyAngela Messina, University of Catania, Italy
Copyright © 2023 Cheung, Lin and Papadopoulos. This is an open-access article distributed under the terms of the Creative Commons Attribution License (CC BY). The use, distribution or reproduction in other forums is permitted, provided the original author(s) and the copyright owner(s) are credited and that the original publication in this journal is cited, in accordance with accepted academic practice. No use, distribution or reproduction is permitted which does not comply with these terms.
*Correspondence: Vassilios Papadopoulos, dnBhcGFkb3BAdXNjLmVkdQ==