- 1Key Laboratory of Neuroregeneration of Jiangsu and Ministry of Education, Jiangsu Province Co-innovation Center of Neuroregeneration, NMPA Key Laboratory for Research and Evaluation of Tissue Engineering Technology Products, Nantong University, Nantong, China
- 2School of Medicine, Nantong University, Nantong, China
Stroke ranks second as a leading cause of death and permanent disability globally. Microglia, innate immune cells in the brain, respond rapidly to ischemic injury, triggering a robust and persistent neuroinflammatory reaction throughout the disease’s progression. Neuroinflammation plays a critical role in the mechanism of secondary injury in ischemic stroke and is a significant controllable factor. Microglia activation takes on two general phenotypes: the pro-inflammatory M1 type and the anti-inflammatory M2 type, although the reality is more complex. The regulation of microglia phenotype is crucial to controlling the neuroinflammatory response. This review summarized the key molecules and mechanisms of microglia polarization, function, and phenotypic transformation following cerebral ischemia, with a focus on the influence of autophagy on microglia polarization. The goal is to provide a reference for the development of new targets for the treatment for ischemic stroke treatment based on the regulation of microglia polarization.
1. Introduction
Based on data from the WHO, stroke is the second most common cause of death and lifelong disability worldwide, with ischemic stroke accounting for 80–90% of all strokes (Fukuta et al., 2017; Henderson et al., 2018; Barthels and Das, 2020). The inflammatory response is an early event in the cascade of cerebral ischemia-reperfusion injury and is present throughout the disease course, including during convalescence (Chamorro et al., 2016). Microglia, which are innate immune cells in the brain, play a central role in the onset and persistence of inflammation (Hu et al., 2012). Selective ablation of proliferating microglia has been shown to worsen cerebral ischemic injury, indicating that the presence of microglia is necessary to mitigate ischemic injury (Lalancette-Hébert et al., 2007). In fact, microglia play dual and opposite roles in the progression of inflammatory responses in ischemic stroke (Huang and Feng, 2013). The activated microglia are classified into two phenotypes: the pro-inflammatory M1 phenotype and the anti-inflammatory M2 phenotype (Xue et al., 2021). However, an increasing number of studies have shown that this binary classification oversimplifies microglia activation, as the two polar phenotypes often overlap and can be transformed by both intracellular and extracellular factors during disease progression. These factors, which regulate the transformation of M1/M2 phenotype, act as molecular switches in signaling pathways and are crucial targets for regulating neuroinflammatory responses to improve prognosis in cerebral ischemia.
Autophagy is a crucial degradation system in eukaryotic cells, whereby autophagosomes enwrap damaged proteins or organelles within their bilayer structure and transport them to lysosomes (animals) or vacuoles (yeast and plants) for degradation (Parzych and Klionsky, 2014). In ischemic stroke, both oxidative stress and inflammation can directly trigger autophagy (Zang et al., 2020; Zhu Y. et al., 2021). Properly regulated autophagy can promote brain injury recovery by reducing oxidative stress and inflammatory responses. Dysfunctional autophagy can exacerbate brain damage, while excessive autophagy can lead to autophagy-dependent cell death (Wang P. et al., 2018; Denton and Kumar, 2019). Autophagy can regulate the polarization and function of microglia, but its mechanism remains controversial. Knocking out Atg5, an autophagy-associated protein, is sufficient to trigger the M1 phenotype without inflammatory stimulation. Autophagy was once thought to act as an endogenous brake on M1 polarization in microglia (Jin et al., 2018). However, recent studies have found that Atg5 in microglia does not play a role in autoimmune neuroinflammation in mice (Srimat Kandadai et al., 2021). Studies in ischemic stroke suggest that microglial autophagy may influence the pathological process of neuroinflammation by regulating polarization.
In this review, we examine the signaling molecules and mechanisms closely associated with the regulation of microglia polarization following cerebral ischemia, with a particular focus on the impact of autophagy. Our goal is to identify potential targets for the development of novel drugs based on the regulation of microglia polarization, which could ultimately improve the prognosis of cerebral ischemia.
2. Resting microglia and their normal function
Microglia originate from primitive myeloid progenitor cells, which leave the yolk sac at 8.5–9 days of embryo development and enter the neural tube through the primitive blood flow to the CNS. They acquire genealogy-specific gene expression and differentiate into mature microglia (Ginhoux et al., 2010). In the healthy CNS, microglia account for 5–12% of the total number of cells, with varying proportions in different brain regions (Lawson et al., 1990). Under stable condition, microglia are found in the CNS parenchyma, while CNS-associated macrophages (CAMs) are present in the CNS boundary region, such as the perivascular space, meninges, and choroid plexus. Microglia and CAMs express several common markers, including CD11b CX3CR1 (CX3 chemokine receptor 1), and CD45 (hematopoietic marker). Therefore, they are often grouped together as microglia/macrophages to discuss their activation and function (Hickman et al., 2013). However, recent findings from single-cell sequencing studies have revealed distinct expression profiles of microglia and CAMs. Microglia specifically express P2yr12, Tmem119, Fcrls, Siglech, Slc2a5, SalI1, Hexb, and Trem2, while CAMs specifically express Lyve1, Siglec1, and Mrc1. This highlights the transcriptional and epigenetic differences between microglia and other bone marrow-derived macrophages in the brain (Konishi et al., 2017; Masuda et al., 2020a,b). In this review, we will focus on microglia and their activation and function in cerebral ischemia.
Normal microglia have a highly branched morphology and are commonly referred to as resting microglia. However, recent in vivo two-photon microscopy studies have revealed that these so-called resting microglia are not completely stationary but are dynamic and highly responsive to their environment (Yu et al., 2020). The maintenance of microglial quiescence depends in part on neuronal and astrocytic signaling. Resting microglia establish direct contact with neuronal synapses approximately once per hour, enabling them to monitor synaptic function and act as organizers, facilitating synaptic formation and maturation (Nimmerjahn et al., 2005; Kato et al., 2019). Microglia-mediated synaptic pruning and plasticity play an important role in CNS physiology. During early brain development, approximately 20–80% of long axonal neurons undergo apoptosis and are rapidly eliminated by microglia (Sierra et al., 2010). Microglia secrete extracellular signals such as cytokines, hormones, neurotransmitters, or growth factors that bind to specific neuronal receptors to promote neuron survival or initiate programmed cell death, thereby regulating the number of CNS neurons (Aarum et al., 2003; Morgan et al., 2004).
Microglia are not only involved in regulating tissue development, structural refinement, and maintaining the neural environment but also maintain brain homeostasis by removing pathogens, dead cell debris, and abnormal proteins, as well as participating in injury response and subsequent remodeling and repair processes (Orihuela et al., 2016). Microglia use a series of immune receptors, such as NODs, NLRs, TLRs, and SRs, to identify adverse stimuli and withstand self and non-self injuries (Hickman et al., 2018). In monitoring mode, once they encounter a danger signal, microglia rapidly activate and undergo morphological changes, such as enlarging the cell body, shortening the process, and increasing phagocytic activity (Tsukahara et al., 2020). These functions determine the crucial role of microglia in the brain development and various CNS diseases.
3. Phenotype and morphological changes of activated microglia
Microglia activation is involved in the pathogenesis of almost all CNS diseases. Furthermore, the response of microglia to stimuli is influenced by age and sex. In C57BL/6 mice, the expression levels of iNOS, TNF-α, and Arg-1 were higher in microglia at 3 days of age, while the levels of CD11b, TLR4, and FcRγ increased in microglia at 21 days of age. The expression levels of pro-inflammatory factors in microglia were low in adult mice but increased with age. Interestingly, at 3 days of age, females expressed more inflammatory factors than males, even though there was no difference in estrogen levels between them at this age (Crain et al., 2013).
Activated microglia can polarize in two rather different directions, depending on the initial stimulus that caused the activation. Exposure to bacteria-derived products (such as LPS) or infection-associated signals (such as IFN-γ) promotes M1-type polarization of microglia, which is known as classic activation (Kalkman and Feuerbach, 2016). M1-type microglia produce pro-inflammatory cytokines such as IL-1α, IL-1β, IL-6, IL-12, and TNF-α, REDOX molecules like NADPH oxidase, phagocytic oxidase, and iNOS, as well as MHC-II, chemokines such as CCL2, CXCL9, CXCL10, and large amounts of ROS, which promote inflammatory responses and play neurotoxic roles (Ransohoff and Brown, 2012; Gyoneva and Ransohoff, 2015).
Interleukin 4 and IL-13 can induce alternative activation of microglia, also known as M2-type polarization, which plays a role in inflammation inhibition, tissue remodeling, angiogenesis, and immune regulation. Unlike classical activation, M2-type polarization leads to a phenotypic change that promotes neuroprotection. M2-type polarization can be further divided into M2a, M2b, and M2c subclasses (Walker and Lue, 2015). M2a microglia produce anti-inflammatory factors such as IL-10 and IGF-1, up-regulate the expression of Arg-1, CD206, and Ym1, inhibit NF-κB, and enhance phagocytosis (Martinez and Gordon, 2014). M2b microglia are induced by the triggering of immunoglobulin FC-γ receptors, TLRs, IL-1R, and immune complexes on the surface of microglia. They down-regulate the expression of IL-12 and increase the secretion of IL-10. The M2b subclass is considered to be a transitional activation state with some characteristics of both M1 and M2 type and is a potential regulator of M2 type (Gensel and Zhang, 2015). M2c polarization can be induced by IL-10 and glucocorticoid, up-regulating the expression of TGF-β, and participating in tissue remodeling and matrix deposition after inflammation (Siddiqui et al., 2016). Table 1 shows the triggers of microglia with different phenotypes and their typical markers.
Microglia exhibit a wide range of phenotypic diversity, and their morphology is closely related to their function. Microscopic observation reveals that resting microglia have small cell bodies and very fine, long ramifications, while activated microglia have enlarged cell bodies and shortened ramifications (Lier et al., 2021). Amoeboid microglia are characterized by swelling of the cell body and complete contraction of the protrusions (Doorn et al., 2014). The highly branched and amoeboid morphologies represent two extremes of the microglia morphological spectrum, with a variety of transitional states existing between them. These states likely reflect disease-specific functional cell states and are closely related to their precise role in the damaged brain (Salamanca et al., 2019).
Since most markers may be expressed simultaneously in different microglial functional states, it is impractical to identify phenotypes with a single marker. For example, while Iba-1 is a classic marker of microglial activation, it cannot distinguish between microglia with different polarizing phenotypes (Ito et al., 1998). Tmem119 is expressed in both resting and dystrophic microglia, while CD68 expression is significantly increased in both activated and amoeboid microglia. Similarly, ferritin is expressed in both activated and dystrophic microglia (Lier et al., 2021). Therefore, when differentiating microglia in different functional states, appropriate markers should be selected and combined with more than two markers to increase the accuracy of identification. Figure 1 displays the morphological changes of resting microglia after stimulation and the markers in different phases.
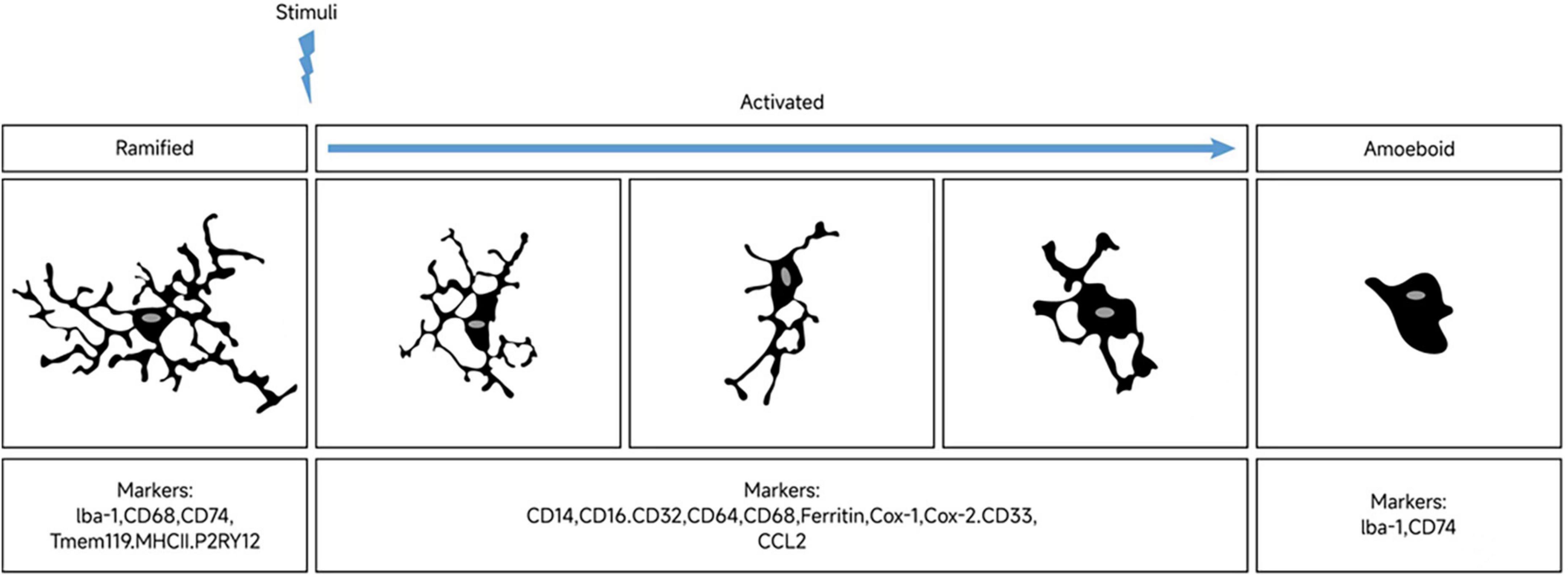
Figure 1. Morphological changes of microglia upon stimulation and markers expression across polarization phases. In their resting state, microglia display a highly branched morphology. Stimulation results in shorter branches and enlarged cell bodies. Microglia exhibit different polarization phenotypes in response to distinct triggering signals. At opposite ends of the morphological spectrum are the highly branched and amoeboid microglial phenotypes, with a diverse range of morphologies observed between them. Markers associated with microglial polarization are expressed differentially across these distinct phenotypic phases.
The classification of microglia function based on morphological characteristics often involves multiple quantitative parameters. However, this approach may be susceptible to biases arising from artificial selection, which can ultimately affect the accuracy of the classification (York et al., 2018; Kyriazis et al., 2019). Leyh et al. (2021) developed a novel method to classify microglia based on morphological assessment using convolutional neural networks. They focused on four microglia morphologies found in the hippocampus and cortex of mice: branched, rod-shaped, activated, and amoeboid microglia. This classification method was validated in a mouse model of ischemic stroke, indicating that machine learning can be employed as an objective and time-efficient approach to characterize microglial changes in healthy and diseased mouse models. Moreover, it may also serve as a valuable tool for analyzing human brain autopsy samples. Salamanca et al. (2019) developed a pipeline called the microglia and immune cells morphologies analyzer and classifier (MIC-MAC), which semi-automatically segments, extracts, and classifies all microglia and immune cells labeled in large 3D confocal image stacks of mouse and human brain samples. MIC-MAC is a precise diagnostic tool capable of automatically characterizing and classifying the 3D morphologies of thousands of individual microglial cells, enabling rapid, unbiased, large-scale analysis of microglia morphological states in mouse models and human brain autopsy samples.
Apart from variations in inflammatory factors and microenvironment, are there discernible morphological differences between the M1 and M2 phenotypes of microglia? Evidence suggests that a mixture of M1-type activation markers (CD74, CD40, CD86, and CCR7) and M2-type activation markers (CCL22 and CD209) can be detected in the brains of individuals with multiple sclerosis (MS), but it remains unclear whether these different activation states correspond to unique morphological features of microglia (Peferoen et al., 2015). This indicates that the expression of both M1-type and M2-type markers is dynamic and that microglia can switch between the M1 and M2 phenotypes. In the brain of patients with AD, the co-expression of both M1-type and M2-type markers, including M2a, M2b, and M2c, can also be observed (Sudduth et al., 2013). Therefore, as activated microglia, it is difficult to distinguish between the M1 and M2 phenotypes based solely on morphology. It is necessary to associate their morphology, markers, and functional states together for accurate classification.
4. Polarization dynamics of microglia after ischemic stroke
Microglial activation can be detected clinically in the acute, subacute, and chronic stages of ischemic stroke (Gulyás et al., 2012). In animal models of ischemic stroke, microglial activation varies dynamically over time and space at different stages, and is correlated with the severity of the stroke (Colton, 2009).
The initial hours after cerebral ischemia typically show a dominance of M2-type microglia in terms of activation, which gradually shifts to M1-type over time (Hu et al., 2012; Kanazawa et al., 2017; Liu et al., 2018). In the 12 h following experimental middle cerebral artery occlusion (MCAO), a mix of M1 and M2 microglia can be observed (Zhu et al., 2019). After stroke, M1-type microglia are mainly concentrated in the penumbra within 24 h, and the level of its marker CD68 significantly increases from 24 h after MCAO. At a later time point, M1-type microglia can be observed in both the penumbra and the ischemic center (Ma et al., 2017). On the other hand, the expression of M2-type microglia in the acute stage of stroke is limited to the ischemic core area and reaches its peak 3–5 days after MCAO. It gradually decreases over time and changes into M1-type microglia in the infarct margin area. By day 14, the level of M2-type microglia has returned to the its pre-injury level (Mabuchi et al., 2000; Hu et al., 2012). However, it has also been reported that the expression of genes associated with M2 phenotype returns to baseline at 28 days after ischemia (Liu et al., 2018; Venkat et al., 2019). In contrast, M1-type microglia continue to increase for 14 days after MCAO (Perego et al., 2011; Hu et al., 2012). Figure 2 illustrates the dynamics of microglia polarization after cerebral ischemia. It is worth noting that the activation of microglia may differ between the permanent ischemia model and the transient cerebral ischemia reperfusion model. In the permanent occlusion model, activated microglia are primarily located in the penumbra rather in the infarct center. However, in transient ischemic animals, activated microglia are found in both the infarct center and the penumbra (Zanier et al., 2015).
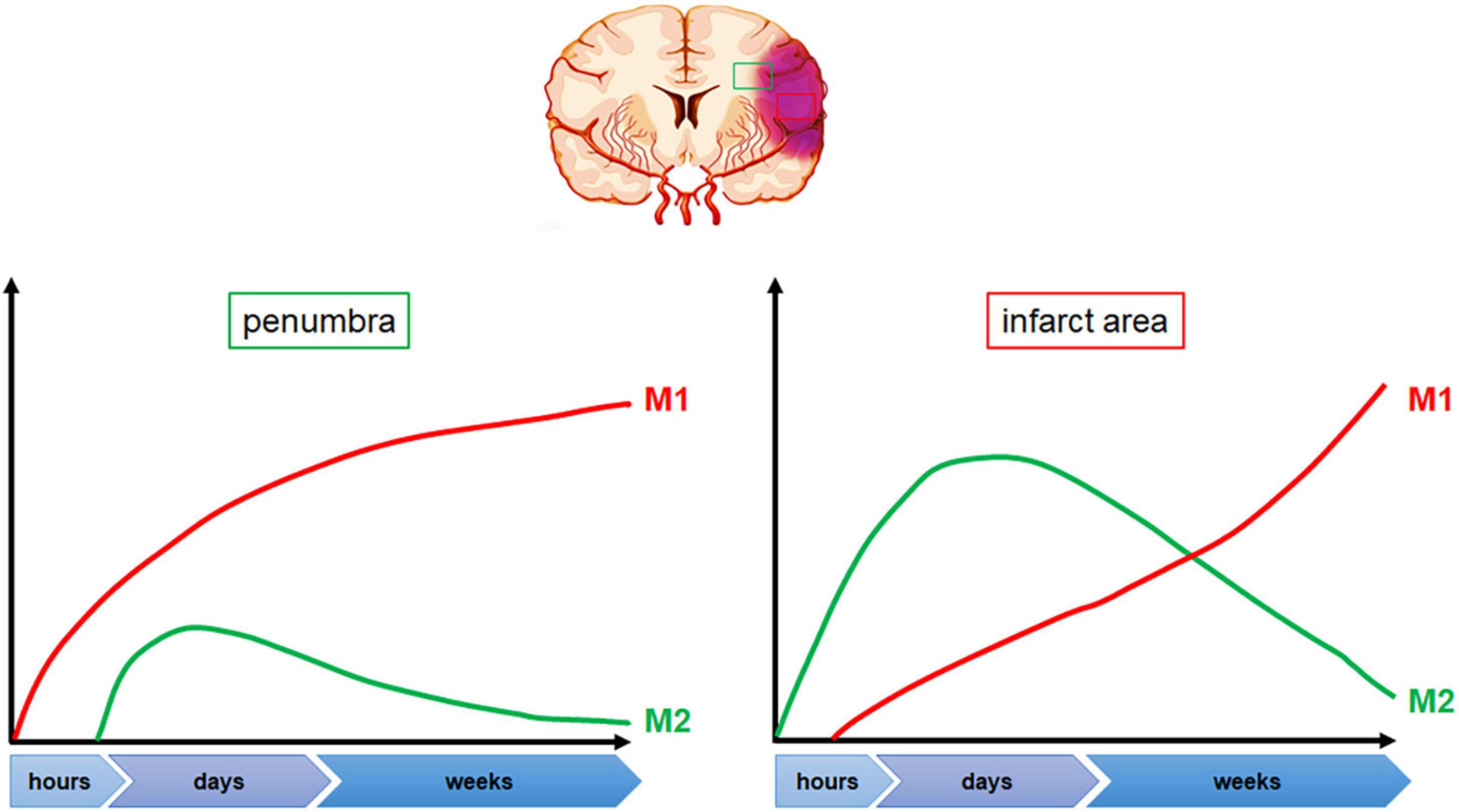
Figure 2. Dynamic changes in microglia polarization following cerebral ischemia. Following stroke onset, microglia polarization shifts toward the M2 phenotype within the first few hours, with predominant accumulation in the ischemic area. Peak M2 polarization is observed between 3 and 5 days post-stroke, following which microglia gradually transition toward the M1 phenotype, primarily at the penumbra edge. The levels of M1 polarized microglia increase significantly 24 h after stroke onset and continue to rise.
In the late stage of MCAO, M1-type microglia-mediated inflammation can cause further damage to neurons and blood-brain barrier, exacerbating tissue destruction and functional deterioration (Wimmer et al., 2018). Recombinant human fibroblast growth factor 21 (rhFGF21) has been found to decrease M1-type polarization of microglia after cerebral ischemia, promote M1-to-M2-type polarization transformation, and activate PPARγ in the ischemic penumbra (Jiang et al., 2018; Wang D. et al., 2020). Hypothermia is also known to protect brain from ischemic stroke. In the MCAO model, maintaining a low temperature between 32 and 34°C can reduce the number of CD16-positive M1 microglia and increase the number of CD206-positive M2 microglia, thereby alleviating brain injury (Liu et al., 2018). This effect is likely related to the promotion of M1-to-M2-type polarization transformation of brain microglia in ischemic mice by low temperature.
After 24 h of cerebral ischemia in mice, CD11b-positive cells often gather around neurons in the ischemic core and peripheral regions, indicating microglia activation. CD11b/CD68 double-positive cells appear in both regions, with round cell bodies, while the CD68-positive branch protrusions wrap NeuN-positive cells, suggesting a potential role in engulfing neurons. In contrast, Ym1-positive cells are only found in the ischemic core, and CD11b/Ym1 double-positive cells do not seem to participate in neuronal phagocytosis (Perego et al., 2013). There are sex differences in CD11b expression in microglia in adult mice, with higher levels observed in female mice compared to males. However, only male mice show an increase in CD11b immunoreactivity after MCAO. This suggests that the sex difference of CD11b expression levels may influence the different phagocytosis responses of male and female microglia to ischemic injury (Morrison and Filosa, 2016).
The inflammatory environment undergoes changes over time and plays a crucial role in orchestrating microglial polarization. Inhibition of M1-type microglia polarization has been found to reduce neurological deficits following cerebral ischemia (Zhang et al., 2019; Mota et al., 2020), while promoting the polarization of M2-type microglia can aid in tissue remodeling and repair (Choi et al., 2017; Jiang et al., 2021). Future research should aim to regulate the balance between M2 and M1 phenotypes, maintain inflammatory defense, and promote neuroprotection, rather than simply reducing the damage caused by M1-related chronic inflammation (Lian et al., 2020).
5. Transformation of M1 and M2 phenotypes
Microglia activation serves as both the initiating and continuing factor of the inflammatory response in ischemic stroke. The M1/M2 phenotype represents two distinct activation states, but in vivo, microglia may exist in a spectrum of different and overlapping polarized phenotypes that can be reversed or transformed based on microenvironmental signals (Mosser and Edwards, 2008; Colton and Wilcock, 2010; Tanaka et al., 2020). Transcription regulators, cytokines, and inflammatory signaling pathways are involved in regulating the M1 and M2 phenotypic transformations. Therefore, future research should explore the complexity of microglia activation and its regulation, as well as identify potential therapeutic targets to modulate microglia activation states for optimal recovery after ischemic stroke.
5.1. Peroxisome proliferation-activated receptor γ (PPARγ)
Peroxisome proliferation -activated receptor γ is a ligand-dependent nuclear transcription factor that exists in multiple subtypes, including PPARγ. PPARγ is expressed in both neurons and microglia, where it exerts neuroprotective and anti-inflammatory effects on ischemic injury. It plays a key role in polarizing microglia from M1 type to M2 phenotypes (Yu et al., 2015; Li L. et al., 2021) and neutrophils from N1 to N2 phenotypes (Cuartero et al., 2013). PPARγ activation has been detected during M2 polarization of microglia (Zhou et al., 2020). In non-ischemic tissues, the expression of PPARγ is low, but it increases significantly in ischemic neurons, peaking at 24 h after MCAO (Victor et al., 2006). Troglitazone and Pioglitazone, which are classic insulin sensitizers, have been shown to reduce neuroinflammation and infarct volume in transient cerebral ischemia by targeting PPARγ activation (Sundararajan et al., 2005). Rosiglitazone, another PPARγ agonist, has been found to reduce neuroinflammation and autophagy-induced cell death in rats with global cerebral ischemia (Shao and Liu, 2015). RhFGF21, on the other hand, reduced neuroinflammation after cerebral ischemia by inhibiting NF-κB and upregulating PPARγ, thereby inhibiting M1 polarization and pro-inflammatory cytokine expression in microglia (Wang D. et al., 2020). However, some studies have shown that antagonistic or knockout of PPARγ can inhibit the activation of NF-κB-IKKβ by activating the LKB1-AMPK signal and promote the LPS-induced M1 to M2 phenotype transformation of microglia (Ji et al., 2018). Therefore, PPARγ may act as a hub molecule and play different roles in regulating polarization through different signaling pathways.
5.2. Interferon regulatory factor (IRF)
Interferon regulatory factor is a multifunctional transcription factor involved in interferon transcription regulation, signal transduction, and immune regulation. IRF5 and IRF4 mediate M1 and M2 polarization of microglia, respectively (Al Mamun et al., 2018). IRF5 and IRF4 balance each other, and reducing the expression of IRF5 can promote M2-type activation, increase IRF4 expression, inhibit the inflammatory response, and improve outcomes in cerebral ischemia. Conversely, reducing IRF4 expression can lead to increased IRF5 expression, promote M1-type activation, exacerbate the inflammatory response, and lead to worse outcomes in cerebral ischemia (Al Mamun et al., 2020). Age has an impact on the regulatory effect of IRF4/5 on stroke-related inflammation. In young mice, the levels of IRF4 and CD206, anti-inflammatory factors such as TGF-β, IL-4, and IL-10, are significantly higher in the brain compared to old mice after cerebral ischemia. Conversely, in old mice, the levels of IRF5 and MHCII in the brain, as well as pro-inflammatory factors such as TNF-α, iNOS and IL-6 in the serum, are significantly higher than in young mice (Zhao et al., 2017). The expression of IRF4/5 in microglia is significantly higher in aged female mice compared to male mice, and this difference is further amplified by cerebral ischemia injury. In ischemic brain tissue of elderly female mice, the IRF4 gene is inhibited while IRF5 gene is activated. Consequently, older females tend to have higher mortality and poorer outcomes after stroke. This sex-specific phenotype is associated with epigenetic modification of IRF4 and IRF5 by the escape genes kdm5c and kdm6a, respectively, on the X chromosome (Qi et al., 2021). When considering the regulation of microglia polarization by targeting IRF, appropriate intervention timing, gender, and age should be taken into account.
5.3. Toll-like receptor 4 (TLR4)
Toll-like receptor 4 is primarily expressed on microglia and plays a crucial role in recognizing damage-associated molecular patterns (DAMPs). It is also one of the primary receptors for LPS binding and can activate NF-κB pathway to regulate inflammatory processes. Polyphenolic natural products, including quercetin (Le et al., 2020), curcumin (Liu et al., 2017), resveratrol (Mota et al., 2020), baicalin (Yang et al., 2019), can regulate microglia polarization by inhibiting TLR4/NF-κB pathway, leading to anti-inflammatory roles in ischemic stroke (Li et al., 2022). Stimulation of the vagus nerve during the acute stage of cerebral ischemia can inhibit M1-type polarization and promote M2-type polarization by blocking the TLR4/MyD88/NF-κB signaling pathway, leading to improved outcomes in ischemic stroke (Zhang et al., 2021). Analgecine (AGC), an analgesic for lumbar pain in patients with spinal degenerative diseases, has been shown to inhibit M1-type polarization and promote M2-type polarization by inhibiting TLR4/MyD88/NF-κB signaling pathway, which reduced neuroinflammation during cerebral ischemia (Yang et al., 2021). TLR4 deficiency has been shown to regulate the polarization of microglia toward the M2 phenotype, improving neurological function following cerebral ischemia and traumatic brain injury (Yao et al., 2017; Tian et al., 2019). Therefore, TLR4 is a promising target for stroke therapy. ApTOLL is an aptamer that antagonizes TLR4. In Hernández-Jiménez et al. (2022), a dose-ascending, randomized, placebo-controlled Phase I clinical trial was conducted to evaluate the safety and pharmacokinetic profile of ApTOLL in healthy volunteers. In Hernández-Jiménez et al. (2023), a multicenter, double-blind, randomized, placebo-controlled Phase Ib/IIa clinical study (NCT04734548) was initiated to evaluate the tolerability, safety, pharmacokinetic, and biological effects of ApTOLL in patients with acute ischemic stroke (AIS) who are eligible for endovascular therapy (EVT). The ongoing clinical study will provide a basis for the clinical application of ApTOLL.
5.4. Galectins
Galectins are a family of carbohydrate-binding proteins that have been identified as potential regulators of microglia polarization, immune surveillance, and neuroinflammation (da Rosa et al., 2021). The Galectin family consists of 15 members, of which Gal-1,3,4,8,9 are expressed in the brain and involved in neural regulation. Deletion of Gal-1 significantly enhances microglia activation, while Gal-1 promotes microglia inactivation through glycosylation-dependent mechanisms, thus playing a neuroprotective role (Aalinkeel and Mahajan, 2016). Additionally, Gal-1 also targets activated microglia, promoting phagocytosis and transformation to the M2 phenotype (Rinaldi et al., 2016).
In a model of cerebral ischemia, Gal-3 expression is dependent on microglia activation (Rabinovich and Toscano, 2009). Gal-3 is an endogenous mediator of injury-induced microglia activation and proliferation (Ladeby et al., 2005; Lalancette-Hébert et al., 2007). Deletion of Gal-3 gene inhibits microglia activation (Espinosa-Oliva et al., 2021), resulting in insufficient microglia proliferation after ischemic injury, which leads to an increased size of infarction and number of apoptotic neurons (Lalancette-Hébert et al., 2012). In one study, administrating a single dose of Gal-3 recombinant protein into the lateral ventricle 24 h after stroke resulted in increased immune reactivity of Ym1 and up-regulated expression of anti-inflammatory factor IL-4, while reducing the immune reactivity of iNOS and the expression of pro-inflammatory factors (Rahimian et al., 2019). This suggests that Gal-3 can promote M2-type polarization of microglia after cerebral ischemia. However, other studies have found that Gal-3 released by microglia after global ischemic injury can act as an endogenous paracrine TLR4 ligand, activating TLR4 and resulting in sustained activation of microglia and increased neuronal damage (Burguillos et al., 2015). Additionally, a protective mechanism against cerebral ischemia has been found in Gal-3 knockout mouse model, particularly in the hippocampus and striate cortex (Doverhag et al., 2010). Therefore, the role of Gal-3 in neuroinflammatory response is complex, and it may play an crucial role in the fine-tuning of neuroinflammation (Rabinovich and Toscano, 2009).
5.5. Cysteinyl leukotriene receptor (CysLTR)
Cysteinyl leukotrienes (CysLTs) are potent lipid inflammatory mediators that activate their receptors (CysLTR1 and CysLTR2), mediating pro-inflammatory effects (Gelosa et al., 2017). During the pathological process, CysLTR1 and CysLTR2 expression levels in cerebral vascular endothelial cells, astrocytes, microglia, and neurons were significantly up-regulated. Upregulation of CysLTRs in activated microglia in the ischemic core of MCAO rats was associated with inflammation and exacerbation in the subacute phase (Shi et al., 2012; Mansour et al., 2018; Wang Y. et al., 2020). Inhibition of CysLTR1 or CysLTR2 protects against LPS or ischemia-induced microglial inflammation and brain injury (Shi et al., 2015; Chen F. et al., 2017; Lin et al., 2017). Neuroprotection is provided by the CysLTR1 antagonist Montelukast and the CysLTR2 antagonist HAMI3379, as they inhibit ischemia-induced microglial activation and pro-inflammatory cytokine release (Shi et al., 2015). The effect of CysLTR1 siRNA is limited in comparison to that of Montelukast, suggesting that Montelukast may produce neuroprotective effects through both CysLTr2-dependent and independent mechanisms (Theron et al., 2014; Marschallinger et al., 2015; Chen F. et al., 2017). Activation of CysLTR2 promotes M1-type polarization and inhibits M2-type polarization through NF-κB pathway (Wang Y. et al., 2020). Specific blocking of CysLTR2 can inhibit M1-type polarization and reduce ischemic neuronal damage (Zhang et al., 2013; Shi et al., 2015). IL-4, in combination with HAMI3379 (a CysLTR2 inhibitor), has been shown to promote M2-type polarization of microglia (Zhao et al., 2019). Additionally, mesenchymal stem cell-derived exosomes have been found to inhibit M1-type polarization of microglia by inhibiting CysLTR2, thereby alleviating cerebral ischemia reperfusion injury (Zhao et al., 2020a,b). The combination of baicalin and Geniposide (BC/GP) has been shown to reverse the polarization state of microglia by down-regulating 5-LOX/CysLTRs pathway, promoting the transformation of M1-type to M2-type polarization, and inhibiting cerebral ischemia-induced inflammation and injury (Wu et al., 2019). These results suggest that CysLTR1 and CysLTR2 play a role in microglia activation and neuroinflammation after cerebral ischemia and that CysLTR1 and CysLTR2 antagonists may serve as new anti-inflammatory agents for the treatment of microglia inflammation and neurotoxicity following cerebral ischemia.
5.6. Nuclear factor erythroid 2-related factor 2 (NRF2)
Nuclear factor erythroid 2-related factor 2 is a key regulatory factors of the endogenous antioxidant stress defense system. While no significant NRF2 immunopositive signal is observed in the infarct core area in the early stage after MCAO, NRF2 can be detected in microglia, astrocytes, and neurons in the penumbra and reaches peak levels 24 h after MCAO (Dang et al., 2012; Takagi et al., 2014). Activation of NRF2 has been shown to promote M2-type polarization of microglia after cerebral ischemia (Wang Y. et al., 2018; Hsia et al., 2020; He et al., 2021). Sevoflurane preconditioning can protect against cerebral ischemia by increasing the anti-inflammatory phenotype of microglia through activating the GSK-3β/NRF2 pathway (Cai et al., 2021). Similarly, Magnolol, and Dexmedetomidine have been found to promote M2-type polarization of microglia by activating the NRF2/HO-1/NLRP3 pathway (Tao et al., 2021; Wang et al., 2022). Non-mitogenic fibroblast growth factor 1 (nmFGF1) regulates microglia polarization by activating the NRF2 pathway and inhibiting the NF-κB pathway to protect against ischemic stroke (Dordoe et al., 2022). Tanshinol borneol ester (DBZ) promotes the transformation of microglia from the M1 to M2 phenotype by activating the Akt/GSK-3β/NRF2 pathway and releasing a series of anti-inflammatory and antioxidant factors to protect against cerebral ischemia (Liao et al., 2020). Sinomenine also promotes M2-type polarization by activating NRF2 and alleviates cerebral ischemia injury in rats (Bi et al., 2021). The ability of NRF2 to promote M2-type polarization in microglia, in addition to its antioxidant effects, makes it a promising target for treating ischemic stroke and other CNS diseases.
5.7. Interleukin 4 (IL-4)
Interleukin 4 is a unique cytokine that can regulate microglia function and promote brain repair (Zhao et al., 2015). IL-4 induces microglia M2 polarization and improves long-term neurological function after stroke (Liu et al., 2016). After cerebral ischemia, neurons in the penumbral zone rapidly produce IL-4. This IL-4 induces the activation of M2-type microglia, up-regulates the expression of IL-4 receptors and trophic factors on the surface of microglia, and activates PPARγ to enhance PPARγ-mediated phagocytosis of apoptotic neurons (Zhao et al., 2015). It is worth noting that neurons with excitotoxic damage due to overactivation of NMDA receptors also produce IL-4, promoting microglia to neuroprotective M2-type polarization (Ting et al., 2020). In female mice, IL-4 is required for neuroprotective effects, and female mice protected by IL-4 after focal cerebral ischemia show dominant M2 activation and reduced inflammatory infiltration (Xiong et al., 2015).
However, a study showed that the simultaneous loss of IL-4, IL-5, IL-9, and IL-13 did not affect neuropathologic responses in subacute and chronic ischemic stroke (Perego et al., 2019a). Perego et al. (2019a) investigated the phenotype changes of microglia at 24 h, 7 days, and 5 weeks after pMCAO using transgenic mice with simultaneous deletion of IL-4, IL-5, IL-9 and IL-13 genes (4KO). They found that CD16/32 (M1 marker) expression was lower but CD206 (M2 marker) expression was higher in 4KO mice than in wild-type mice at 24 h and 7 days after pMCAO. However, the neuropathological results of the 4KO mice did not differ from those of wild-type mice. This conclusion caused some controversy. Xu et al. (2019) suggested that IL-4 is induced by injured neurons in the ischemic penumbra region, and the use of pMCAO models with less penumbra tissue may result in negative results for differences between genotypes (Zhao et al., 2015). Perego et al. (2019b) argued that they demonstrated that Th2 cytokines, such as IL-4, are not necessary neural immune signals to drive the outcome of cerebral ischemia, although penumbra extension may be different in pMCAO and tMCAO models, and the results may differ in cerebral ischemia models with a higher degree of penumbra.
5.8. Signal transducer and activator of transcription (STAT)
Excessive production of pro-inflammatory cytokines, including IL-1, IL-6, and TNF-α, during the acute phase following focal cerebral ischemia, is a major contributor to inflammatory injury. The effects of these cytokines are magnified by the JAK-STAT signaling pathway downstream. Here, phosphorylated STAT is translocated to the nucleus and regulates gene expression downstream. STAT1, STAT3, and STAT6 are all involved in the activation of microglia induced by ischemia. Cerebral ischemia can trigger the phosphorylation of both STAT1 and STAT3. Phosphorylated STAT1 can exacerbate ischemia-induced neuronal injury, while STAT1 knockout can decrease cerebral infarction volume in mice (Takagi et al., 2002). STAT activation can induce the transformation of microglia into an M1 phenotype, while STAT6 activation can induce an M2 phenotype (Deszo et al., 2004). Minocycline, an antibiotic medication, can inhibit the phosphorylation of STAT1 and promote the phosphorylation of STAT6 after cerebral ischemia. As a result, it can regulate the M1/M2 polarization of microglia by modulating the STAT1/6 pathway (Lu et al., 2021).
During cerebral ischemia, STAT3 is expressed in activated microglia, astrocytes, and endothelial cells, which are closely associated with inflammation. By upregulating the phosphorylation level of STAT3 and increasing the levels of neurotrophic factors and anti-inflammatory cytokines, IL-10 can promote the polarization of M2c microglia and facilitate tissue repair (Miki et al., 2021). Fingolimod (FTY720) is a high-affinity agonist of sphingosine 1 phosphate (S1P) receptor and is the first FDA-approved drug for the treatment of relapsing-remitting MS due to its immunosuppressive action (Fu et al., 2015). By activating STAT3 signaling pathway, Fingolimod promotes M2-type microglia activation and oligodendrocyte regeneration, which helps improve white matter integrity and alleviate cognitive dysfunction caused by hypoperfusion (Qin et al., 2017).
It’s worth noting that the JAK2/STAT3 signaling pathway likely has a biphasic in regulating cerebral ischemia (Zhong et al., 2021). The overactivation of STAT3 induced by ischemia primarily occurs in microglia (Chen S. et al., 2017). Inhibition of JAK2 prevents the phosphorylation and nuclear translocation of STAT3, thereby reducing cerebellar infarct volume and improving neurological deficits (Satriotomo et al., 2006). Stachydrine inhibits the phosphorylation of JAK2/STAT3 and improves neural deficits in rats with MCAO (Li et al., 2020). On the other hand, Xuesaitong down-regulates the transcription level of STAT3, promoting M2-type polarization of microglia and providing neuroprotection in MCAO mice (Li et al., 2019). Additionally, long non-coding RNA nuclear enriched transcript 1 (NEAT1) plays a vital role in immune regulation. Cerebral ischemia can significantly increase NEAT1 expression levels. Knocking down NEAT1 inhibits the Akt/STAT3 signaling pathway, promotes M2-type polarization of microglia, inhibits M1-type polarization, and ultimately reduces neuronal apoptosis caused by ischemia (Ni et al., 2020; Lian and Luo, 2021).
5.9. Triggering receptor expressed on myeloid cells-2 (TREM2)
Triggering receptor expressed on myeloid cells-2 is a single transmembrane receptor primarily localized in microglia. Like other phagocyte receptors, TREM2 moves between the cytoplasm and membrane and regulates microglial phagocytosis, migration, and survival. Additionally, TREM2 can directly induce microglial phenotypic transformation. A lack of TREM2 is harmful to microglial phagocytosis of synaptic components, while overexpression of TREM2 can reduce the pro-inflammatory response of microglia (Zhang et al., 2017; Ren et al., 2018; Gratuze et al., 2020; Ruganzu et al., 2021).
Triggering receptor expressed on myeloid cells-2 may have a neuroprotective role in experimental ischemic stroke by reducing neuroinflammation (Kawabori et al., 2013; Wu et al., 2017). Its expression is highly increased in microglia following cerebral ischemia, and its level of expression can be used to predict the prognosis of cerebral ischemia, rather than its expression level in circulating macrophages (Kurisu et al., 2019). This supports the idea that phagocytosis is mainly mediated by microglia in the brain, rather than macrophages of bone marrow origin in tMCAO model (Schilling et al., 2005). Microglia with TREM2 knocked out show reduced activity after ischemia, and their phagocytosis of injured neurons is also reduced (Kawabori et al., 2015).
Triggering receptor expressed on myeloid cells-2 plays a role in regulating the M1 to M2 phenotype transformation of microglia after ischemic stroke. In mouse models of MCAO, TREM2 activation and up-regulation promote the transition of microglia from a pro-inflammatory M1 phenotype to an anti-inflammatory M2 phenotype. Administering systemic TREM2 agonists or injecting TREM2 lentivirus directly into the ventricle accelerates neurological recovery in mice. These findings suggest that TREM2 may modulate microglial phenotypes after stroke, affecting the short-term prognosis of stroke in mice (Zhai et al., 2017).
Triggering receptor expressed on myeloid cells-2 has the ability to bind to different ligands, which regulates the direction of TREM2 signal transduction and produces different effects. As a novel regulator of microglial phenotype, TREM2 represents an attractive target for microglial regulation in the treatment of ischemic stroke. However, the mechanism and temporal dynamics of TREM2 in regulating phenotypes after stroke are currently unclear and require further investigation.
5.10. Cathepsin
Cathepsins are a group of cysteine proteolytic enzymes in the endosomal lysosome system that play a crucial role in the lysosomal protein degradation pathway. Activated microglia have a complex role that is related to its phenotypic changes, and the expression and secretion of various cathepsins are important in the M1-type polarization of microglia, chronic neuroinflammation, and neuronal death. The cathepsins associated with microglia polarization and neuroinflammatory regulation include Cathepsin B, C, E, and H (Nakanishi, 2020).
Cathepsin B in microglia can activate NLRP3 inflammasome, leading to the activation of caspase-1 and the subsequent release of IL-1β. Alternatively, it can also directly activate procaspase-11, independent of NLRP3, leading to the activation of caspase-1 and release of IL-1β from microglia (Sun et al., 2012; Chen et al., 2018; Campden and Zhang, 2019). After hypoxic ischemia, the deficiency of Cathepsin B results in a transient polarization of microglia toward the neuroprotective M2 phenotype instead of the M1 type (Ni et al., 2015). NF-κB is a crucial transcription factor that regulates multiple genes involved in M1-type polarization in microglia. Its activity is higher in M1-polarized microglia but lower in M2-polarized microglia (Karin, 2006; Biswas and Lewis, 2010; Dorrington and Fraser, 2019). NF-κB inhibitor α (IκBα) is an endogenous negative regulator of NF-κB, which prevents its translocation into the nucleus. IκBα undergoes proteolytic degradation upon signal induction, which is mediated by the ubiquitin-proteasome system. This degradation leads to the rapid and transient activation of NF-κB. During the early stage of hypoxic-ischemic brain injury, ubiquitinated IκBα is proteolytically degraded by the proteasome, which results in the activation of NF-κB (He et al., 2019). After the early activation of NF-κB, Cathepsin B in microglia autophagolysosomes induces delayed but persistent activation of NF-κB by degradation of IκBα. Subsequently, NF-κB further enhances the expression of Cathepsin B and Beclin 1, a key regulator of autophagy, which promotes autophagy in post-ischemic brain injury (Ni et al., 2015). Cathepsin B has been shown to play a role in the phenotypic switch of microglia from M2 to M1 by modulating the activity of NF-κB.
Cathepsin C is primarily induced in activated microglia after systemic injection of LPS, promoting microglia polarization toward neurotoxicity and exacerbating neuroinflammation. This effect is mainly achieved through the activation of the Ca2+ dependent protein kinase C/p38 MAPK/NF-κB pathway (Liu et al., 2019). The interaction between Cathepsin C and inflammatory cytokines in microglia amplifies neuroinflammation-related cellular events and is considered one of the underlying causes of persistent inflammation in the brain.
Cathepsin E is not normally detected in healthy brains and is only expressed in activated microglia in pathological brains, suggesting that its enzymatic activity is associated with activated microglia (Nakanishi et al., 1993; Ni et al., 2015). In neonatal mice with hypoxic-ischemic brain injury, activated microglia secreted Cathepsin E to hydrolyze tumor necrosis factor-associated apoptosis inducing ligand (TRAIL) on the surface of microglia. This leads to the activation of NF-κB via TRAIL death receptor DR5, which promotes M1-type polarization of microglia (Ni et al., 2015).
Interferon-β (IFN-β) secreted by microglia is known to have a neuroprotective effect (Lobo-Silva et al., 2017; Scheu et al., 2019). Deficiency of Cathepsin H leads to decreased levels of TLR3 and IFN-β expressed in splenic dendritic cells (Okada et al., 2018). TLR3 is involved in the activation of NF-κB and IRF3, which leads to the production of IFN-β and pro-inflammatory cytokines. The proteolysis of TLR3 is essential for its function, and Cathepsin H and B play a crucial role in the regulation of this process (Garcia-Cattaneo et al., 2012). In neonatal mice, the deficiency of Cathepsin H leads to prolonged neurotoxic M1-like polarization following hypoxia ischemia, which ultimately results in microglia death and subsequent neurotoxic A1 astrocyte proliferation and progressive neuronal death (Liddelow et al., 2017; Ni et al., 2021). However, in mice injected with LPS, upregulated expression of Cathepsin H in microglia exacerbates neuronal death in neuroinflammation (Fan et al., 2015). Based on the current evidence, it appears that maintaining appropriate levels of Cathepsin H may be important in regulating neuroinflammation and protecting against neurological damage. Both deficient and elevated levels of Cathepsin H have been associated with adverse effects on neuroinflammation, suggesting that a balanced level of Cathepsin H activity is necessary for maintaining healthy brain function. Further research is needed to fully understand the complex role of Cathepsin H in neuroinflammation and to explore potential therapeutic strategies targeting this enzyme in neurological disorders.
Figure 3 and Table 2 summarizes the molecules that regulate microglia polarization and their functions. These include various transcription factors, cytokines, chemokines, and receptors, which can promote either M1 or M2 polarization. Table 3 summarizes the factors and drugs that can modulate microglia polarization phenotypes. These include various natural compounds, such as curcumin and resveratrol, as well as drugs that are commonly used in clinical settings, such as minocycline and dexamethasone. These factors and drugs have been shown to promote either M1 or M2 polarization, and their effects on microglia polarization may have important implications for the treatment of neurological disorders.
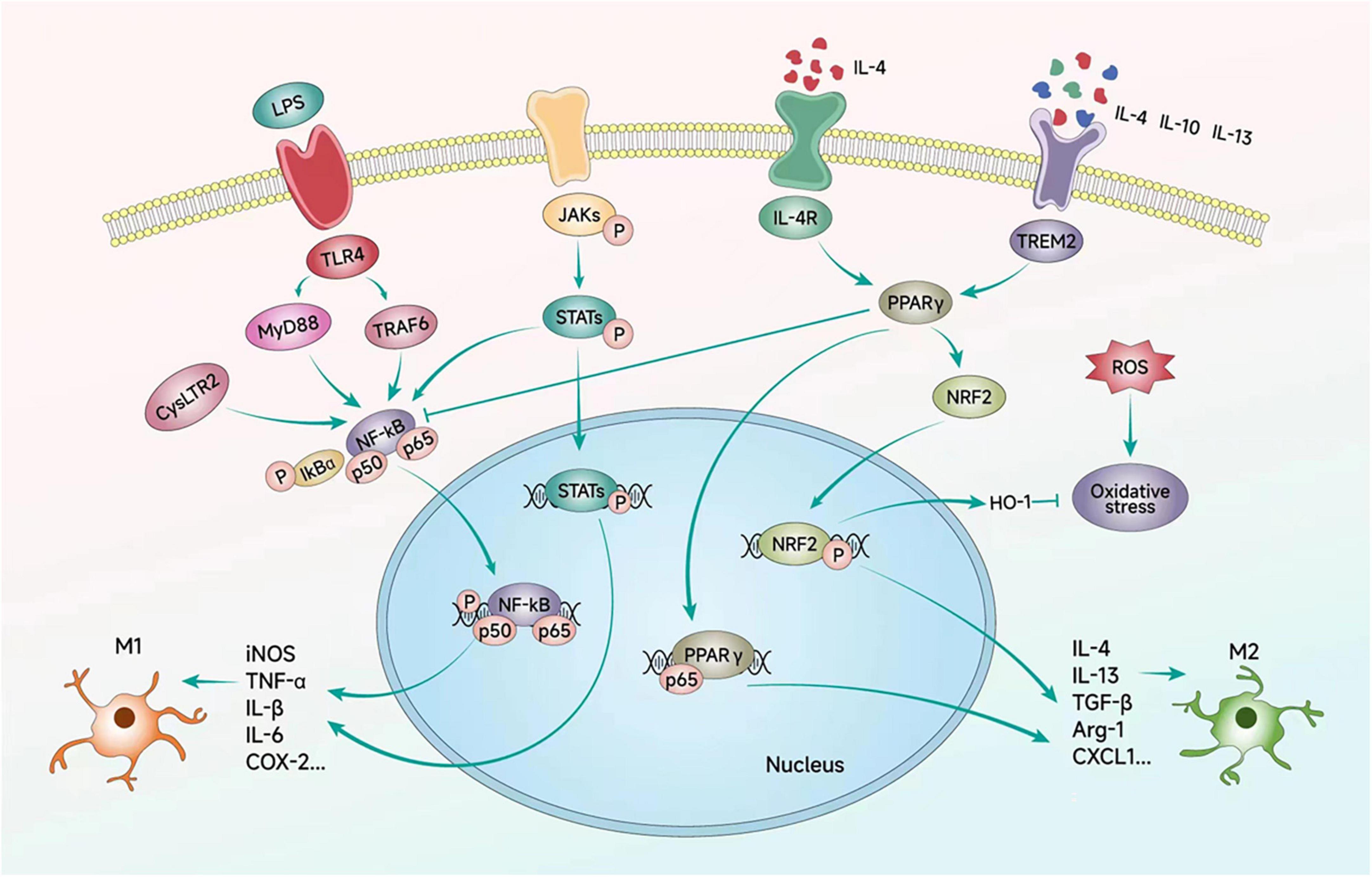
Figure 3. Signaling pathways regulating microglial polarization. Microglial polarization is triggered by extracellular factors such as LPS, cytokines, and ILs, which bind to their corresponding receptors on the microglial cell membrane. These signaling events initiate downstream cascades that transfer signals to the nucleus and regulate the expression of inflammatory factors through a series of transcription factors. Ultimately, these signaling pathways contribute to the emergence of different polarized phenotypes of microglial cells.
6. Autophagy and ischemia microglia polarization
The transition of cells from one phenotypic state to another is closely linked to the rapid degradation of protein involved in the previous homeostatic state. Autophagy is a major mechanism of large-scale protein degradation in eukaryotic cells and a critical step in cell reprogramming (Zubova et al., 2022). Following cerebral ischemia, autophagy can be either activated or inhibited in various cell types in the brain, including neurons, astrocytes, vascular endothelial cells, and microglia. Autophagy plays a role in the regulating inflammatory responses, blood-brain barrier permeability, and microglia polarization (Lu et al., 2022). Indeed, in many CNS diseases, autophagy dysfunction and neuroinflammation are intertwined and mutually affect each other (Plaza-Zabala et al., 2017).
6.1. Autophagy participates in regulating microglial inflammation
Lipopolysaccharide can activate PI3K/Akt/mTOR pathway in microglia, which inhibits autophagic flow and enhances the inflammatory response (Ye et al., 2020). Conversely, autophagy activation can inhibit the increase of LPS-induced microglia pro-inflammatory factors, such as iNOS and IL-6 expression, and thus suppress the inflammatory response (Han et al., 2013). Annexin-A1 (ANXA1) mediates the degradation of IκB kinase-α (Iκk-α) via selective autophagy, which is promoted by the interaction between ANXA1 and the autophagy receptor NBR1. This inhibits the activation of the NF-κB signaling pathway and reduces the production of pro-inflammatory factors in OGD/R-damaged microglia (Li X. et al., 2021). Additionally, selective autophagy regulated by Beclin1 is involved in the degrading of the NLRP3 inflammasome, which regulate the production of IL-1β and IL-18 and affects the activation of microglia. Therefore, impaired autophagy in microglia can contribute to the neuroinflammation (Houtman et al., 2019). Autophagy can act as a negative regulator of inflammation in acute cerebral ischemia by participating in the inhibition of NLRP3-mediated neuroinflammation through the involvement of Beclin1, LC3, and p62 (Fu et al., 2020; Huang et al., 2021; Lugovaya et al., 2022).
6.2. Autophagy affects the function of microglia
Beclin 1 plays a crucial role in promoting effective microglial phagocytosis in vitro and in the mouse brain. The decreased levels of autophagy in microglia in AD are associated with reduced ability to clear abnormal protein accumulation, which highlights the importance of autophagy in maintaining protein homeostasis (Lucin et al., 2013). Deletion of the microglia-specific autophagy regulator Atg7, instead of the typical macrophage protein ULK1, may cause progressive MS-like disease (Berglund et al., 2020). Moreover, microglial autophagy also regulates neuroinflammation and tau protein pathology. Atg7 deletion in microglia leads to a pro-inflammatory transformation of microglia and enhances the pathology and diffusion of tau protein in neurons (Xu et al., 2021). Microglial Atg5 deficiency results in PD-like symptoms in mice, which could be attributed to the up-regulation of pro-inflammatory macrophage migration inhibitory factor (MIF) expression in microglia due to autophagy dysfunction (Cheng et al., 2020).
6.3. Autophagy regulates microglia polarization
Autophagy is a crucial homeostasis mechanism that regulates microglial activation states. It can modulate the phenotype of OGD-damaged microglia by regulating the balance between NF-κB and cAMP-response element binding protein (CREB) (Xia et al., 2016). Molecular switches, including TLR4, STATs, and PPARγ, play a crucial role in regulating autophagy-induced phenotypic changes. After ischemic injury, TLR4 expression in microglia is significantly increased, resulting in the accumulation of autophagosomes in microglia. However, knocking out of TLR4 or using autophagy inhibitors can induce the transformation of microglia from pro-inflammatory M1 phenotype to anti-inflammatory M2 phenotype, thereby exerting a neuroprotective effect (Qin et al., 2018). Nevertheless, heavily activated autophagy can clear the anti-inflammatory phenotype of TLR4-deficient microglia (Qin et al., 2018). These findings suggest that the maintenance of appropriate autophagic flux is critical for regulating microglial functional phenotypes. LPS-induced upregulation of STAT1 expression and downregulation of STAT6 expression in microglia, which can be reversed by autophagy inhibitors (Qin et al., 2018). The MS drug Fengomode inhibits LPS-induced microglial autophagy and STAT1 activation and promotes the transformation of M1-type microglia into M2 type microglia (Hu et al., 2021). Specific inhibition of PPARγ can stimulate the formation of microglial autophagosomes and their degradation by lysosomes, promoting the transformation of microglia from M1 to M2 type (Ji et al., 2018).
The polarization of microglia affects autophagic flux as well. In the context of cerebral ischemia, activated M1-type microglia expresses the phosphodiesterase enzyme 1 (PDE1), and inhibiting PDE1 enhances autophagy in microglia. The PDE1 inhibitor Vinpocetine inhibits M1-type polarization and promotes M2-type polarization in microglia in an autophagy-dependent manner. However, inhibiting microglia autophagy can counteract the regulatory effect of Vinpocetine on polarization (Zang et al., 2020).
Notably, the M2 polarization of microglia is highly dependent on autophagy (Sanjurjo et al., 2018). Treatment with IL-4 enhances the autophagic flux of microglia, leading to autophagy-dependent M2 polarization (Tang et al., 2019). Inhibition of autophagy by chloroquine significantly reduces the expression of M2-specific genes, such as Arg-1, FIZZ1, and IL-10, in wild-type macrophages, but this effect is not observed in Cathepsin S–/– macrophages (Yang M. et al., 2014). This suggests that the regulation of microglia M2 polarization by autophagy may be linked to Cathepsin S.
6.4. Autophagy regulates microglia polarization at different phases after cerebral ischemia
The regulation of microglia autophagy flux and phenotype is dependent on the timing after ischemia and hypoxia. Studies have shown that the autophagy flux of microglia increases at 12, 24, and 48 h after OGD reperfusion but decreases at 72 h, during which most microglia show the M1 phenotype. If autophagy is inhibited by the lysosome protease inhibitor ammonium chloride (NH4Cl) 24 h after reperfusion of OGD, most microglia also show the M1 type (Xia et al., 2016). As mentioned earlier, the M2 phenotype is dominant within 24 h after ischemia reperfusion injury, indicating that autophagy flux can be appropriately increased at this stage to promote M2-type polarization of microglia. However, excessive autophagy can also lead to autophagic apoptosis or death of microglia. The M2 phenotype gradually decreases within 72 h after ischemia reperfusion, which may be related to the decrease of autophagy flux. Thus, it is crucial to maintain an appropriate level of autophagy flux at different phases after ischemia to regulate microglia polarization.
6.5. Key signaling molecules associated with autophagy and microglia polarization
Neuroinflammation and autophagy dysfunction are closely linked to the onset and progression of CNS diseases. However, the precise role of autophagy in microglia and neuroinflammation remains unclear. The regulation of microglial autophagy involves several critical factors, including mammalian target of rapamycin (mTOR), hypoxia-inducible factor-1 (HIF-1), and endoplasmic reticulum (ER) stress. These signaling pathways play a crucial role in modulating the autophagy flux and polarization of microglia, and dysfunction of these pathways has been implicated in the pathogenesis of various CNS disorders. Therefore, a better understanding of the molecular mechanisms underlying autophagy dysregulation in microglia could provide novel therapeutic targets for the treatment of neuroinflammatory diseases.
Mammalian target of rapamycin is a key regulator of cell metabolism and plays a critical role in modulating autophagy. mTOR exists in two signaling complexes, mTORC1 and mTORC2. While mTORC1 integrates various growth factor signals to promote protein, lipid, and nucleotide synthesis, it also blocks catabolic processes such as autophagy by post-transcriptional and transcriptional mechanisms (Laplante and Sabatini, 2013).
Activated mTORC1 is closely associated with autophagy induction. However, most autophagy-inducing conditions, such as nutrient or growth factor deprivation and low cellular energy levels, inhibit mTORC1 activity. mTORC1 inhibits autophagy induction by phosphorylation-dependent inhibition of unc-51-like kinase 1/2 (ULK1/2) and phosphatidylinositol 3-kinase catalytic subunit type 3 (Vps34). Additionally, it prevents overall expression of lysosome and autophagy genes by phosphorylating transcription factor EB (TFEB), which strictly regulates autophagy (McAlpine et al., 2013; Munson et al., 2015; Napolitano et al., 2018). The molecular mechanisms of upstream effector regulation of mTORC2 remain unclear. It is speculated that mTORC2 may indirectly inhibit autophagy by activating mTORC1 (Oh et al., 2010).
In transgenic mice with deletions of microglia-regulated proteins associated with mTOR, the inhibition of mTORC1 signaling significantly reduces the production of pro-inflammatory cytokines and chemokines, as well as the number of M1-type microglia, resulting in a significant reduction in infarct volume after MCAO (Li et al., 2016). TNF-α inhibits microglial autophagy through the Akt/mTOR signaling pathway, and enhancing autophagy promotes microglial polarization toward the M2 phenotype and inflammation regression (Jin et al., 2018). Sestrin2 plays a neuroprotective role in ischemic stroke mice by inhibiting mTOR and restoring autophagy flux to drive microglia toward the M2 phenotype (He et al., 2020).
Hypoxia-inducible factor-1 is a key transcription factor that regulates the response to anoxic environment. It is a heterodimer consisting of a functional α subunit and a structural β subunit, which is regulated by oxygen levels. During oxidative stress, HIF-1α forms a heterodimer with HIF-1β, translocates to the nucleus, binds to hypoxia response elements (HREs) in downstream gene promoters, and activates downstream BNIP3. BNIP3 can competitively bind with Beclin-1 to Bcl-2, releasing Beclin-1, a key autophagy protein, and inducing autophagy. Additionally, BNIP3 inhibits Rheb activity, which inhibits the mTOR pathway and promotes autophagy (Xu et al., 2020; Abo El-Ella, 2022). Hypoxia upregulates HIF-1α expression and increases autophagy flux in microglia, while the number of autophagosomes decreases significantly in cells transfected with HIF-1α-siRNA after hypoxia treatment. Pharmacological inhibition of HIF-1α can also down-regulate autophagy of hypoxic microglia (Wang et al., 2017). These findings suggest that HIF-1α mediates autophagy in microglia under hypoxia conditions, and it may be a regulatory target to maintain appropriate autophagy flux in microglia under such conditions.
Ischemic stroke leads to Ca2+ overload, which causes an increase in protein misfolding and results in ER stress. When ER stress occurs, GRP78 releases PERK, IRE1, and ATF6,which activate the UPR and induce autophagy to degrade misfolded or unfolded proteins, thereby mitigating ER stress (Chaudhari et al., 2014). During this process, oligo PERK phosphorylates EIF2α and selectively translates ATF4 to induce autophagy gene expression. Oligo IRE1α binds TRAF2, activating ASK1 and its downstream kinase p38 MAPK and JNK. Furthermore, the intrinsic ribonuclease activity of IRE1α enables it to selectively cut and activate XBP1, a transcription factor that induces UPR gene expression. ATF6 is transported to the Golgi apparatus for proteolysis, releasing active AtF6α. ATF4, XBP1, and ATF6α converge on the promoter of CHOP gene, stimulating CHOP activation. CHOP can inhibit the expression of Bcl-2 gene, while JNK can directly inhibit the activity of Bcl-2, thus reducing the inhibition of Bcl-2 on the autophagy protein Beclin-1 and promoting autophagy (Han et al., 2022). Following OGD/R injury, autophagy and ER stress are significantly increased in microglia. SC-222227, a protein tyrosine phosphatase 1B inhibitor, can inhibit microglia autophagy by suppressing PERK-regulated ER stress, indicating the involvement of ER stress in the regulation of microglia autophagy during cerebral ischemia (Zhu Y. et al., 2021). Figure 4 illustrates the key signaling pathways by which microglia autophagy participates in the regulation of polarization.
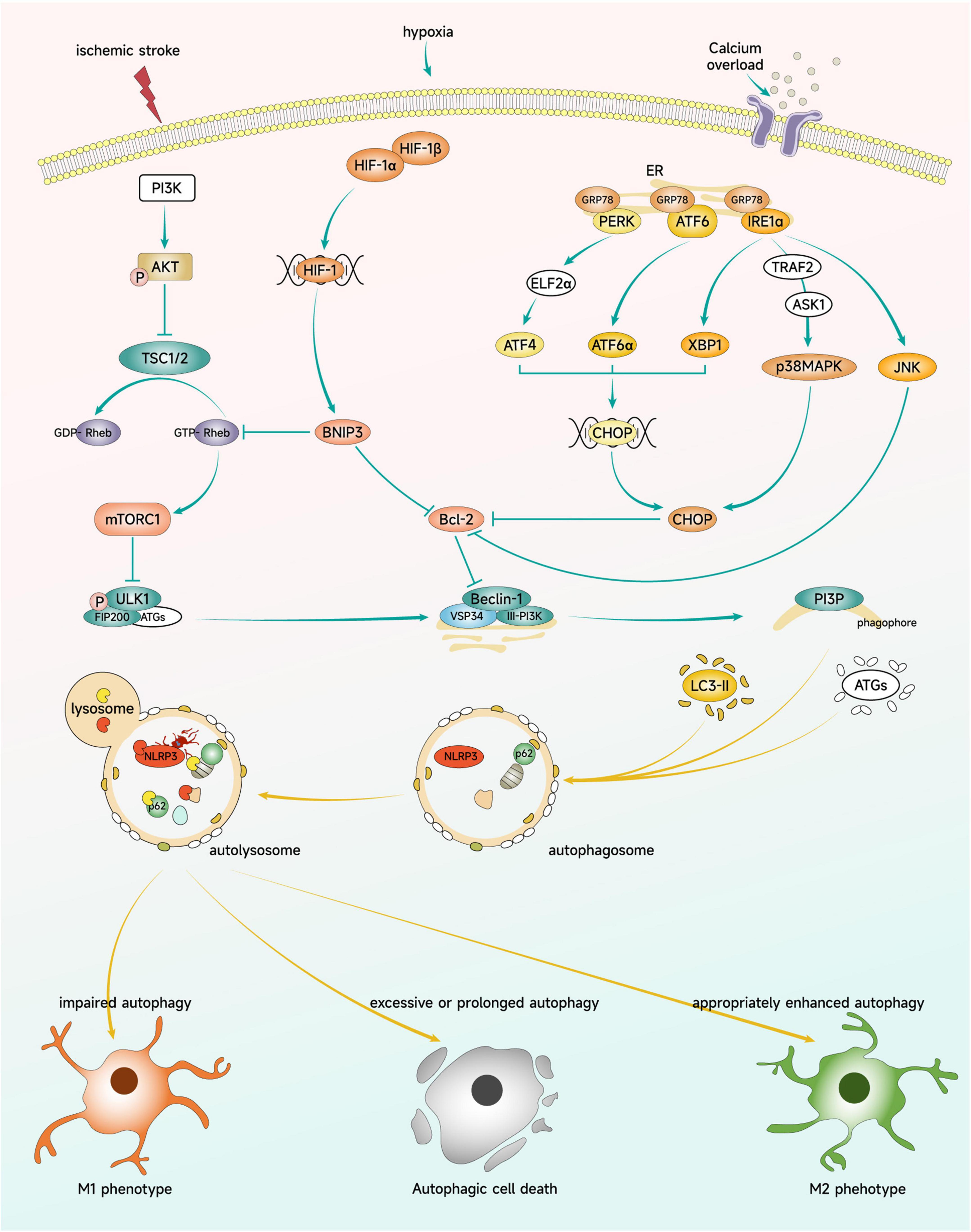
Figure 4. Key signaling pathways regulating microglial polarization through autophagy. Brain ischemia triggers signaling through mTOR, HIF-1, and ER stress pathways. These signaling molecules can impact microglial autophagy levels and modulate the polarization phenotype and cellular fate of microglia.
The mechanism of microglial autophagy in ischemic stroke and its impact on stroke outcome have yet to be fully elucidated. Activated microglia autophagy may influence phenotype changes while serving a scavenging role (Chen et al., 2016), and may also contribute to nerve injury (Yang Z. et al., 2014; Qin et al., 2018). The dynamic shift of microglia polarization phenotype is a well-known factor in nerve repair following ischemic stroke. By introducing the appropriate subtype at the appropriate time, it may be possible to enhance CNS repair.
7. Conclusion and perspective
Despite significant progress, there are still important questions that need to be addressed in understanding the regulation of microglia polarization. For instance, how to effectively regulate the polarization of microglia using a large number of different types of receptors and signaling molecules, and how to adjust it over time during the progression of the disease to achieve the best balance between M1/M2 polarization. Furthermore, while modulation of microglia polarization shows promise for treating CNS diseases, its clinical application remains a significant challenge. The effects of many inhibitors or inducers of M1 or M2 polarization phenotypes are not simply all-or-nothing, but are dependent on time and dose. Moreover, it should be noted that M1-mediated inflammatory responses also have potential benefits that should not be simply suppressed or eliminated. It is also important to consider that maintaining a high level of M2-type polarization for a long time may have negative consequences, such as increased susceptibility to tumor growth, as M2-type macrophages have been shown to promote tumor growth.
Recent studies have been attempted to manipulate the anti-inflammatory polarization of microglia at the site of stroke, with promising results (Li Y. et al., 2021). Li Y. et al. (2021) designed ultrasound controlled, platelet-decorated microglia targeting therapy for ischemic stroke. Using a platelet-hybridized microglia platform, microglia can adhere strongly to injured cerebral vessels through platelet membrane infusion and achieve on-demand anti-inflammatory polarization by decorating with ultrasound-responsive IL-4 liposomes. This microglia platform demonstrates anti-inflammatory polarization at the stroke site after i.v. injection, promoting endogenous M2-type polarization of microglia and aiding in long-term stroke recovery. These results provide inspiration for the future applications of microglia polarization in the targeted regulation of CNS ischemia injury.
Author contributions
HW and QC wrote the initial draft. JL, HZ, MW, LX, and YW contributed to reviewing the literature. QC designed the manuscript and prepared the final version. All authors read and approved the final manuscript.
Funding
This research was supported by grants from the National Natural Science Foundation of China, Nos. 81501058 and 82071355; the Natural Science Foundation of Jiangsu Higher Education Institutions of China, No. 20KJA310011; and a grant from the Priority Academic Program Development of Jiangsu Higher Education Institutions (PAPD).
Conflict of interest
The authors declare that the research was conducted in the absence of any commercial or financial relationships that could be construed as a potential conflict of interest.
Publisher’s note
All claims expressed in this article are solely those of the authors and do not necessarily represent those of their affiliated organizations, or those of the publisher, the editors and the reviewers. Any product that may be evaluated in this article, or claim that may be made by its manufacturer, is not guaranteed or endorsed by the publisher.
Abbreviations
3D, three-dimension; 5-LOX, 5-lipoxygenase; AD, Alzheimer’s disease; AIS, acute ischemic stroke; AMPK, AMP-activated protein kinase; ANXA1, Annexin-A1; ASK1, apoptotic signal-regulated kinase 1; ATF4, activating transcription factor 4; ATF6, activating transcription factor 6; Bcl-2, B-lymphoma-2; BNIP3, Bcl2/adenovirus E1B 19 kDa interacting protein-3; CAMs, CNS-associated macrophages; CHOP, CCAAT/enhancer-binding protein; CNS, central nervous system; CREB, cAMP-response element binding protein; CX3CR1, CX3 chemokine receptor 1; CysLTRs, Cysteinyl leukotriene receptors; CysLTs, Cysteinyl leukotrienes; DAMPs, damage-associated molecular patterns; DBZ, Tanshinol borneoleste; EIF2α, eukaryotic translation initiation factor 2α; ER, endoplasmic reticulum; EVT, endovascular therapy; FIZZ1, found in inflammatory zone 1; GRP78, glucose-regulated protein 78; GSK3β, glycogen synthase kinase-3; HIF-1, hypoxia-inducible factor-1; HO-1, heme oxygenase-1; HREs, Hypoxia response elements; i.c.v., intracerebroventricular; i.g., intragastrical; i.p., intraperitoneal; i.v., intravenous; ICH, intracerebral hemorrhage; IFN-γ, interferon-γ; IGF-1, insulin-like growth factor-1; IL, interleukin; iNOS, inducible nitric oxide synthase; IR, ischemia-reperfusion; IRE1, inositol requiring enzyme 1; IRF, interferon regulatory factor; IκBα, NF-κB inhibitor α; Iκk-α, IκB kinase-α; JAK, Janus kinase; JNK, c-Jun N-terminal kinase; KO, knock out; LC3, microtubule-associated protein light chain 3; LKB1, live kinase B1; LPS, lipopolysaccharide; MAPK, mitogen-activated protein kinases; MCAO, middle cerebral artery occlusion; MHC-II, major histocompatibility complex II; MIC-MAC, microglia and immune cells morphlogies analyzer and classifier; MIF, migration inhibitory factor; MS, multiple sclerosis; MTK, Montelukast; mTOR, mammalian target of rapamycin; mTORCs, mTOR complexes; MyD88, myeloid differentiation primary response 88; NADPH, nicotinamide adenine dinucleotide phosphate; NEAT1, nuclear enriched transcript 1; NF-κB, nuclear factor-κB; NLRP3, NACHT, LRR, and PYD domains-containing protein 3; NLRs, NOD-like receptors; NMDA, N-Methyl-D-Aspartate; nmFGF1, non-mitogenic fibroblast growth factor 1; NODs, nucleotide-biding oligomerzation domains; Nrf2, Nuclear factor erythroid 2-related factor 2; OGD, oxygen and glucose deprivation; p38 MAPK, p38 mitogen activated protein kinase; PD, Parkinson’s disease; PDE1, phosphodiesterase enzyme 1; PERK, protein kinase-like endoplasmic reticulum kinase; PI3K, phosphoinositide 3-kinase; pMCAO, permanent MCAO; PPARγ, peroxisome proliferation -activated receptor γ; rGal-3, Recombinant Galectin-3; RhFGF21, recombinant human fibroblast growth factor 21; ROS, reactive oxygen species; RSK1, Ras/Erk/p90 ribosome S6 kinase 1; RTKs, receptor tyrosine kinases; S1P, sphingosine 1 phosphate; SAH, subarachnoid hemorrhage; SRs, scavenger receptors; STAT, signal transducer and activator of transcription; TFEB, phosphorylation transcription factor EB; TGF-β, transforming growth factor-β; TLRs, toll-like receptors; tMCAO, transient MCAO; TNF-α, tumor necrosis factor-α; TNF-α, tumor necrosis factor α; TRAF2, tumor necrosis factor receptor-associated factor 2; TRAIL, tumor necrosis factor-associated apoptosis inducing ligand; TREM2, triggering receptor expressed on myeloid cells-2; TSC1/2, tuberous sclerosis complex 1/2; ULK1/2, unc-51-like kinase 1/2; UPR, unfolded protein response; WHO, World Health Organization; XBP1, X-box-binding protein 1; XCI, X chromosome inactivation.
References
Aalinkeel, R., and Mahajan, S. (2016). Neuroprotective role of galectin-1 in central nervous system pathophysiology. Neural Regen Res. 11, 896–897. doi: 10.4103/1673-5374.184455
Aarum, J., Sandberg, K., Haeberlein, S., and Persson, M. (2003). Migration and differentiation of neural precursor cells can be directed by microglia. Proc Natl Acad Sci U S A. 100, 15983–15988. doi: 10.1073/pnas.2237050100
Abo El-Ella, D. M. (2022). Autophagy/apoptosis induced by geraniol through HIF-1α/BNIP3/Beclin-1 signaling pathway in A549 CoCl2 treated cells. Adv. Pharm. Bull. 12, 155–162. doi: 10.34172/apb.2022.016
Ahmed, A., Wang, L., Abdelmaksoud, S., Aboelgheit, A., Saeed, S., and Zhang, C. (2017). Minocycline modulates microglia polarization in ischemia-reperfusion model of retinal degeneration and induces neuroprotection. Sci. Rep. 7:14065. doi: 10.1038/s41598-017-14450-5
Al Mamun, A., Chauhan, A., Qi, S., Ngwa, C., Xu, Y., Sharmeen, R., et al. (2020). Microglial IRF5-IRF4 regulatory axis regulates neuroinflammation after cerebral ischemia and impacts stroke outcomes. Proc. Natl. Acad. Sci. U S A. 117, 1742–1752. doi: 10.1073/pnas.1914742117
Al Mamun, A., Chauhan, A., Yu, H., Xu, Y., Sharmeen, R., and Liu, F. (2018). Interferon regulatory factor 4/5 signaling impacts on microglial activation after ischemic stroke in mice. Eur. J. Neurosci. 47, 140–149. doi: 10.1111/ejn.13778
Barthels, D., and Das, H. (2020). Current advances in ischemic stroke research and therapies. Biochim Biophys. Acta Mol. Basis Dis. 1866:165260. doi: 10.1016/j.bbadis.2018.09.012
Berglund, R., Guerreiro-Cacais, A., Adzemovic, M., Zeitelhofer, M., Lund, H., Ewing, E., et al. (2020). Microglial autophagy-associated phagocytosis is essential for recovery from neuroinflammation. Sci. Immunol. 5:eabb5077. doi: 10.1126/sciimmunol.abb5077
Bi, F., Zhang, Y., Liu, W., and Xie, K. (2021). Sinomenine activation of Nrf2 signaling prevents inflammation and cerebral injury in a mouse model of ischemic stroke. Exp. Ther. Med. 21:647. doi: 10.3892/etm.2021.10079
Biswas, S., and Lewis, C. E. (2010). NF-κB as a central regulator of macrophage function in tumors. J. Leukoc Biol. 88, 877–884. doi: 10.1189/jlb.0310153
Burguillos, M., Svensson, M., Schulte, T., Boza-Serrano, A., Garcia-Quintanilla, A., Kavanagh, E., et al. (2015). Microglia-Secreted Galectin-3 acts as a toll-like receptor 4 ligand and contributes to microglial activation. Cell Rep. 10, 1626–1638. doi: 10.1016/j.celrep.2015.02.012
Cai, M., Sun, S., Wang, J., Dong, B., Yang, Q., Tian, L., et al. (2021). Sevoflurane preconditioning protects experimental ischemic stroke by enhancing anti-inflammatory microglia/macrophages phenotype polarization through GSK-3β/Nrf2 pathway. CNS Neurosci. Ther. 27, 1348–1365. doi: 10.1111/cns.13715
Campden, R. I., and Zhang, Y. (2019). The role of lysosomal cysteine cathepsins in NLRP3 inflammasome activation. Arch. Biochem. Biophys. 670, 32–42. doi: 10.1016/j.abb.2019.02.015
Chamorro, Á, Dirnagl, U., Urra, X., and Planas, A. M. (2016). Neuroprotection in acute stroke: targeting excitotoxicity, oxidative and nitrosative stress, and inflammation. Lancet Neurol. 15, 869–881. doi: 10.1016/S1474-4422(16)00114-9
Chaudhari, N., Talwar, P., Parimisetty, A., Lefebvre d’Hellencourt, C., and Ravanan, P. (2014). A molecular web: endoplasmic reticulum stress, inflammation, and oxidative stress. Front. Cell Neurosci. 8:213. doi: 10.3389/fncel.2014.00213
Chen, C., Wu, C., Yang, T., Chang, Y., Sheu, M., and Liu, S. (2016). Green tea catechin prevents hypoxia/reperfusion-evoked oxidative stress-regulated autophagy-activated apoptosis and cell death in microglial cells. J. Agric Food Chem. 64, 4078–4085. doi: 10.1021/acs.jafc.6b01513
Chen, F., Ghosh, A., Wu, F., Tang, S., Hu, M., Sun, H., et al. (2017). Preventive effect of genetic knockdown and pharmacological blockade of CysLT. Brain Behav. Immun. 60, 255–269. doi: 10.1016/j.bbi.2016.10.021
Chen, S., Dong, Z., Cheng, M., Zhao, Y., Wang, M., Sai, N., et al. (2017). Homocysteine exaggerates microglia activation and neuroinflammation through microglia localized STAT3 overactivation following ischemic stroke. J. Neuroinflamm. 14:187. doi: 10.1186/s12974-017-0963-x
Chen, N., Ou, Z., Zhang, W., Zhu, X., Li, P., and Gong, J. (2018). Cathepsin B regulates non-canonical NLRP3 inflammasome pathway by modulating activation of caspase-11 in Kupffer cells. Cell Prolif. 51:e12487. doi: 10.1111/cpr.12487
Cheng, J., Liao, Y., Dong, Y., Hu, H., Yang, N., Kong, X., et al. (2020). Microglial autophagy defect causes parkinson disease-like symptoms by accelerating inflammasome activation in mice. Autophagy 16, 2193–2205. doi: 10.1080/15548627.2020.1719723
Choi, J., Kim, J., Park, J., Lee, W., and Lee, J. (2017). M2 phenotype microglia-derived cytokine stimulates proliferation and neuronal differentiation of endogenous stem cells in ischemic brain. Exp. Neurobiol. 26, 33–41. doi: 10.5607/en.2017.26.1.33
Colton, C. (2009). Heterogeneity of microglial activation in the innate immune response in the brain. J. Neuroimmune Pharmacol. 4, 399–418. doi: 10.1007/s11481-009-9164-4
Colton, C., and Wilcock, D. (2010). Assessing activation states in microglia. CNS Neurol. Disord Drug Targets 9, 174–191. doi: 10.2174/187152710791012053
Crain, J., Nikodemova, M., and Watters, J. (2013). Microglia express distinct M1 and M2 phenotypic markers in the postnatal and adult central nervous system in male and female mice. J. Neurosci. Res. 91, 1143–1151. doi: 10.1002/jnr.23242
Cuartero, M., Ballesteros, I., Moraga, A., Nombela, F., Vivancos, J., Hamilton, J., et al. (2013). N2 neutrophils, novel players in brain inflammation after stroke: modulation by the PPARγ agonist rosiglitazone. Stroke 44, 3498–3508.
da Rosa, M., de Aguiar Ferreira, M., de Oliveira Lima, C., Santos Mendonça, A., Silva, Y., Sharjeel, M., et al. (2021). Alzheimer’s disease: is there a role for galectins? Eur. J. Pharmacol. 909:174437. doi: 10.1016/j.ejphar.2021.174437
Dang, J., Brandenburg, L., Rosen, C., Fragoulis, A., Kipp, M., Pufe, T., et al. (2012). Nrf2 expression by neurons, astroglia, and microglia in the cerebral cortical penumbra of ischemic rats. J. Mol. Neurosci. 46, 578–584. doi: 10.1007/s12031-011-9645-9
Denton, D., and Kumar, S. (2019). Autophagy-dependent cell death. Cell Death Differ. 26, 605–616. doi: 10.1038/s41418-018-0252-y
Deszo, E. L., Brake, D. K., Kelley, K. W., and Freund, G. G. (2004). IL-4-dependent CD86 expression requires JAK/STAT6 activation and is negatively regulated by PKCdelta. Cell Signal. 16, 271–280. doi: 10.1016/s0898-6568(03)00137-2
Doorn, K., Goudriaan, A., Blits-Huizinga, C., Bol, J., Rozemuller, A., Hoogland, P., et al. (2014). Increased amoeboid microglial density in the olfactory bulb of Parkinson’s and Alzheimer’s patients. Brain Pathol. 24, 152–165. doi: 10.1111/bpa.12088
Dordoe, C., Wang, X., Lin, P., Wang, Z., Hu, J., Wang, D., et al. (2022). Non-mitogenic fibroblast growth factor 1 protects against ischemic stroke by regulating microglia/macrophage polarization through Nrf2 and NF-κB pathways. Neuropharmacology 212:109064. doi: 10.1016/j.neuropharm.2022.109064
Dorrington, M., and Fraser, I. D. C. (2019). NF-κB signaling in macrophages: dynamics, crosstalk, and signal integration. Front. Immunol. 10:705. doi: 10.3389/fimmu.2019.00705
Doverhag, C., Hedtjärn, M., Poirier, F., Mallard, C., Hagberg, H., Karlsson, A., et al. (2010). Galectin-3 contributes to neonatal hypoxic-ischemic brain injury. Neurobiol. Dis. 38, 36–46. doi: 10.1016/j.nbd.2009.12.024
Espinosa-Oliva, A., García-Miranda, P., Alonso-Bellido, I., Carvajal, A., González-Rodríguez, M., Carrillo-Jiménez, A., et al. (2021). Galectin-3 deletion reduces LPS and acute colitis-induced pro-inflammatory microglial activation in the ventral mesencephalon. Front. Pharmacol. 12:706439. doi: 10.3389/fphar.2021.706439
Fan, K., Li, D., Zhang, Y., Han, C., Liang, J., Hou, C., et al. (2015). The induction of neuronal death by up-regulated microglial cathepsin H in LPS-induced neuroinflammation. J. Neuroinflamm. 12:54. doi: 10.1186/s12974-015-0268-x
Fu, C., Zhang, X., Lu, Y., Wang, F., Xu, Z., Liu, S., et al. (2020). Geniposide inhibits NLRP3 inflammasome activation via autophagy in BV-2 microglial cells exposed to oxygen-glucose deprivation/reoxygenation. Int. Immunopharmacol. 84:106547. doi: 10.1016/j.intimp.2020.106547
Fu, Y., Liu, Q., Anrather, J., and Shi, F. (2015). Immune interventions in stroke. Nat. Rev. Neurol. 11, 524–535. doi: 10.1038/nrneurol.2015.144
Fukuta, T., Asai, T., Yanagida, Y., Namba, M., Koide, H., Shimizu, K., et al. (2017). Combination therapy with liposomal neuroprotectants and tissue plasminogen activator for treatment of ischemic stroke. FASEB J. 31, 1879–1890. doi: 10.1096/fj.201601209R
Gao, Y., Zhuang, Z., Lu, Y., Tao, T., Zhou, Y., Liu, G., et al. (2019). Curcumin mitigates neuro-inflammation by modulating microglia polarization through inhibiting TLR4 axis signaling pathway following experimental subarachnoid hemorrhage. Front. Neurosci. 13:1223. doi: 10.3389/fnins.2019.01223
Garcia-Cattaneo, A., Gobert, F., Müller, M., Toscano, F., Flores, M., Lescure, A., et al. (2012). Cleavage of Toll-like receptor 3 by cathepsins B and H is essential for signaling. Proc. Natl. Acad. Sci. U S A. 109, 9053–9058. doi: 10.1073/pnas.1115091109
Gelosa, P., Bonfanti, E., Castiglioni, L., Delgado-Garcia, J., Gruart, A., Fontana, L., et al. (2019). Improvement of fiber connectivity and functional recovery after stroke by montelukast, an available and safe anti-asthmatic drug. Pharmacol. Res. 142, 223–236. doi: 10.1016/j.phrs.2019.02.025
Gelosa, P., Colazzo, F., Tremoli, E., Sironi, L., and Castiglioni, L. (2017). Cysteinyl leukotrienes as potential pharmacological targets for cerebral diseases. Med. Inflamm. 2017:3454212. doi: 10.1155/2017/3454212
Gensel, J., and Zhang, B. (2015). Macrophage activation and its role in repair and pathology after spinal cord injury. Brain Res. 1619, 1–11. doi: 10.1016/j.brainres.2014.12.045
Ginhoux, F., Greter, M., Leboeuf, M., Nandi, S., See, P., Gokhan, S., et al. (2010). Fate mapping analysis reveals that adult microglia derive from primitive macrophages. Science 330, 841–845. doi: 10.1126/science.1194637
Gratuze, M., Leyns, C., Sauerbeck, A., St-Pierre, M., Xiong, M., Kim, N., et al. (2020). Impact of TREM2R47H variant on tau pathology-induced gliosis and neurodegeneration. J. Clin. Invest. 130, 4954–4968. doi: 10.1172/JCI138179
Gulyás, B., Tóth, M., Schain, M., Airaksinen, A., Vas, A., Kostulas, K., et al. (2012). Evolution of microglial activation in ischaemic core and peri-infarct regions after stroke: a PET study with the TSPO molecular imaging biomarker [((11))C]vinpocetine. J. Neurol. Sci. 320, 110–117. doi: 10.1016/j.jns.2012.06.026
Gyoneva, S., and Ransohoff, R. (2015). Inflammatory reaction after traumatic brain injury: therapeutic potential of targeting cell-cell communication by chemokines. Trends Pharmacol. Sci. 36, 471–480. doi: 10.1016/j.tips.2015.04.003
Han, H., Kim, T., Son, H., Park, W., and Han, P. (2013). Activation of autophagy pathway suppresses the expression of iNOS, IL6 and cell death of lps-stimulated microglia cells. Biomol. Ther. (Seoul). 21, 21–28. doi: 10.4062/biomolther.2012.089
Han, Y., Shen, X., Gao, Z., Ping Han, P., and Bi, X. (2022). Pre-ischaemic treatment with enriched environment alleviates acute neuronal injury by inhibiting endoplasmic reticulum stress-dependent autophagy and apoptosis. Neuroscience 513, 14–27. doi: 10.1016/j.neuroscience.2022.12.014
He, D., Fu, S., Zhou, A., Su, Y., Gao, X., Zhang, Y., et al. (2021). Camptothecin regulates microglia polarization and exerts neuroprotective effects via activating AKT/Nrf2/HO-1 and inhibiting NF-κB pathways in vivo and in vitro. Front. Immunol. 12:619761. doi: 10.3389/fimmu.2021.619761
He, H., Ren, L., Guo, T., and Deng, Y. (2019). Neuronal autophagy aggravates microglial inflammatory injury by downregulating CX3CL1/fractalkine after ischemic stroke. Neural Regen. Res. 14, 280–288. doi: 10.4103/1673-5374.244793
He, T., Li, W., Song, Y., Li, Z., Tang, Y., Zhang, Z., et al. (2020). Sestrin2 regulates microglia polarization through mTOR-mediated autophagic flux to attenuate inflammation during experimental brain ischemia. J. Neuroinflamm. 17:329. doi: 10.1186/s12974-020-01987-y
Henderson, S., Weitz, J., and Kim, P. (2018). Fibrinolysis: strategies to enhance the treatment of acute ischemic stroke. J. Thromb. Haemost. 16, 1932–1940. doi: 10.1111/jth.14215
Hernández-Jiménez, M., Abad-Santos, F., Cotgreave, I., Gallego, J., Jilma, B., Flores, A., et al. (2023). APRIL: a double-blind, placebo-controlled, randomized, Phase Ib/IIa clinical study of ApTOLL for the treatment of acute ischemic stroke. Front. Neurol. 14:1127585. doi: 10.3389/fneur.2023.1127585
Hernández-Jiménez, M., Martín-Vílchez, S., Ochoa, D., Mejía-Abril, G., Román, M., Camargo-Mamani, P., et al. (2022). First-in-human phase I clinical trial of a TLR4-binding DNA aptamer, ApTOLL: safety and pharmacokinetics in healthy volunteers. Mol. Ther. Nucleic Acids 28, 124–135. doi: 10.1016/j.omtn.2022.03.005
Hickman, S., Izzy, S., Sen, P., Morsett, L., and El Khoury, J. (2018). Microglia in neurodegeneration. Nat. Neurosci. 21, 1359–1369. doi: 10.1038/s41593-018-0242-x
Hickman, S., Kingery, N., Ohsumi, T., Borowsky, M., Wang, L., Means, T., et al. (2013). The microglial sensome revealed by direct RNA sequencing. Nat. Neurosci. 16, 1896–1905. doi: 10.1038/nn.3554
Houtman, J., Freitag, K., Gimber, N., Schmoranzer, J., Heppner, F., and Jendrach, M. (2019). Beclin1-driven autophagy modulates the inflammatory response of microglia via NLRP3. EMBO J. 38:e99430. doi: 10.15252/embj.201899430
Hsia, C., Jayakumar, T., Sheu, J., Hsia, C., Huang, W., Velusamy, M., et al. (2020). Synthetic ruthenium complex TQ-6 potently recovers cerebral ischemic stroke: attenuation of microglia and platelet activation. J. Clin. Med. 9:996. doi: 10.3390/jcm9040996
Hu, X., Li, P., Guo, Y., Wang, H., Leak, R., Chen, S., et al. (2012). Microglia/macrophage polarization dynamics reveal novel mechanism of injury expansion after focal cerebral ischemia. Stroke 43, 3063–3070. doi: 10.1161/STROKEAHA.112.659656
Hu, Z., Zhou, L., Yang, S., Chen, M., Yu, H., Tao, R., et al. (2021). FTY720 modulates microglia toward anti-inflammatory phenotype by suppressing autophagy via STAT1 pathway. Cell Mol. Neurobiol. 41, 353–364. doi: 10.1007/s10571-020-00856-9
Huang, Y., and Feng, Z. (2013). The good and bad of microglia/macrophages: new hope in stroke therapeutics. Acta Pharmacol. Sin. 34, 6–7. doi: 10.1038/aps.2012.178
Huang, Z., Zhou, X., Zhang, X., Huang, L., Sun, Y., Cheng, Z., et al. (2021). Pien-Tze-Huang, a Chinese patent formula, attenuates NLRP3 inflammasome-related neuroinflammation by enhancing autophagy via the AMPK/mTOR/ULK1 signaling pathway. Biomed. Pharmacother. 141:111814. doi: 10.1016/j.biopha.2021.111814
Ito, D., Imai, Y., Ohsawa, K., Nakajima, K., Fukuuchi, Y., and Kohsaka, S. (1998). Microglia-specific localisation of a novel calcium binding protein, Iba1. Brain Res. Mol. Brain Res. 57, 1–9. doi: 10.1016/s0169-328x(98)00040-0
Ji, J., Xue, T., Guo, X., Yang, J., Guo, R., Wang, J., et al. (2018). Antagonizing peroxisome proliferator-activated receptor γ facilitates M1-to-M2 shift of microglia by enhancing autophagy via the LKB1-AMPK signaling pathway. Aging Cell 17:e12774. doi: 10.1111/acel.12774
Jiang, G., Yang, X., Zhou, H., Long, J., Liu, B., Zhang, L., et al. (2021). cGAS knockdown promotes microglial M2 polarization to alleviate neuroinflammation by inhibiting cGAS-STING signaling pathway in cerebral ischemic stroke. Brain Res. Bull. 171, 183–195. doi: 10.1016/j.brainresbull.2021.03.010
Jiang, Y., Liu, N., Wang, Q., Yu, Z., Lin, L., Yuan, J., et al. (2018). Endocrine Regulator rFGF21 (Recombinant Human Fibroblast Growth Factor 21) improves neurological outcomes following focal ischemic stroke of type 2 diabetes mellitus male mice. Stroke 49, 3039–3049. doi: 10.1161/STROKEAHA.118.022119
Jin, M., Wang, F., Qi, D., Liu, W., Gu, C., Mao, C., et al. (2018). A critical role of autophagy in regulating microglia polarization in neurodegeneration. Front. Aging Neurosci. 10:378. doi: 10.3389/fnagi.2018.00378
Kalkman, H., and Feuerbach, D. (2016). Antidepressant therapies inhibit inflammation and microglial M1-polarization. Pharmacol. Ther. 163, 82–93. doi: 10.1016/j.pharmthera.2016.04.001
Kanazawa, M., Ninomiya, I., Hatakeyama, M., Takahashi, T., and Shimohata, T. (2017). Microglia and monocytes/macrophages polarization reveal novel therapeutic mechanism against stroke. Int. J. Mol. Sci. 18:2135. doi: 10.3390/ijms18102135
Karin, M. (2006). Nuclear factor-kappaB in cancer development and progression. Nature 441, 431–436. doi: 10.1038/nature04870
Kato, D., Ikegami, A., Horiuchi, H., Moorhouse, A., Nabekura, J., and Wake, H. (2019). In vivo two-photon imaging of microglial synapse contacts. Methods Mol. Biol. 2034, 281–286. doi: 10.1007/978-1-4939-9658-2_20
Kawabori, M., Hokari, M., Zheng, Z., Kim, J., Calosing, C., Hsieh, C., et al. (2013). Triggering receptor expressed on myeloid cells-2 correlates to hypothermic neuroprotection in ischemic stroke. Ther. Hypothermia Temp. Manag. 3, 189–198. doi: 10.1089/ther.2013.0020
Kawabori, M., Kacimi, R., Kauppinen, T., Calosing, C., Kim, J., Hsieh, C., et al. (2015). Triggering receptor expressed on myeloid cells 2 (TREM2) deficiency attenuates phagocytic activities of microglia and exacerbates ischemic damage in experimental stroke. J. Neurosci. 35, 3384–3396. doi: 10.1523/JNEUROSCI.2620-14.2015
Konishi, H., Kobayashi, M., Kunisawa, T., Imai, K., Sayo, A., Malissen, B., et al. (2017). Siglec-H is a microglia-specific marker that discriminates microglia from CNS-associated macrophages and CNS-infiltrating monocytes. Glia 65, 1927–1943. doi: 10.1002/glia.23204
Kurisu, K., Zheng, Z., Kim, J., Shi, J., Kanoke, A., Liu, J., et al. (2019). Triggering receptor expressed on myeloid cells-2 expression in the brain is required for maximal phagocytic activity and improved neurological outcomes following experimental stroke. J Cereb. Blood Flow Metab. 39, 1906–1918. doi: 10.1177/0271678X18817282
Kyriazis, A., Noroozizadeh, S., Refaee, A., Choi, W., Chu, L., Bashir, A., et al. (2019). An end-to-end system for automatic characterization of iba1 immunopositive microglia in whole slide imaging. Neuroinformatics 17, 373–389. doi: 10.1007/s12021-018-9405-x
Ladeby, R., Wirenfeldt, M., Garcia-Ovejero, D., Fenger, C., Dissing-Olesen, L., Dalmau, I., et al. (2005). Microglial cell population dynamics in the injured adult central nervous system. Brain Res. Brain Res. Rev. 48, 196–206. doi: 10.1016/j.brainresrev.2004.12.009
Lalancette-Hébert, M., Gowing, G., Simard, A., Weng, Y., and Kriz, J. (2007). Selective ablation of proliferating microglial cells exacerbates ischemic injury in the brain. J. Neurosci. 27, 2596–2605. doi: 10.1523/JNEUROSCI.5360-06.2007
Lalancette-Hébert, M., Swarup, V., Beaulieu, J., Bohacek, I., Abdelhamid, E., Weng, Y., et al. (2012). Galectin-3 is required for resident microglia activation and proliferation in response to ischemic injury. J. Neurosci. 32, 10383–10395. doi: 10.1523/JNEUROSCI.1498-12.2012
Laplante, M., and Sabatini, D. (2013). Regulation of mTORC1 and its impact on gene expression at a glance. J. Cell Sci. 126(Pt 8), 1713–1719. doi: 10.1242/jcs.125773
Lawson, L., Perry, V., Dri, P., and Gordon, S. (1990). Heterogeneity in the distribution and morphology of microglia in the normal adult mouse brain. Neuroscience 39, 151–170. doi: 10.1016/0306-4522(90)90229-w
Le, K., Song, Z., Deng, J., Peng, X., Zhang, J., Wang, L., et al. (2020). Quercetin alleviates neonatal hypoxic-ischemic brain injury by inhibiting microglia-derived oxidative stress and TLR4-mediated inflammation. Inflamm. Res. 69, 1201–1213. doi: 10.1007/s00011-020-01402-5
Leyh, J., Paeschke, S., Mages, B., Michalski, D., Nowicki, M., Bechmann, I., et al. (2021). Classification of microglial morphological phenotypes using machine learning. Front. Cell Neurosci. 15:701673. doi: 10.3389/fncel.2021.701673
Li, D., Wang, C., Yao, Y., Chen, L., Liu, G., Zhang, R., et al. (2016). mTORC1 pathway disruption ameliorates brain inflammation following stroke via a shift in microglia phenotype from M1 type to M2 type. FASEB J. 30, 3388–3399. doi: 10.1096/fj.201600495R
Li, F., Zhao, H., Han, Z., Wang, R., Tao, Z., Fan, Z., et al. (2019). Xuesaitong may protect against ischemic stroke by modulating microglial phenotypes and inhibiting neuronal cell apoptosis via the STAT3 signaling pathway. CNS Neurol Disord. Drug Targets 18, 115–123. doi: 10.2174/1871527317666181114140340
Li, L., Sun, L., Qiu, Y., Zhu, W., Hu, K., and Mao, J. (2020). Protective effect of stachydrine against cerebral ischemia-reperfusion injury by reducing inflammation and apoptosis through P65 and JAK2/STAT3 signaling pathway. Front. Pharmacol. 11:64. doi: 10.3389/fphar.2020.00064
Li, L., Gan, H., Jin, H., Fang, Y., Yang, Y., Zhang, J., et al. (2021). Astragaloside IV promotes microglia/macrophages M2 polarization and enhances neurogenesis and angiogenesis through PPARγ pathway after cerebral ischemia/reperfusion injury in rats. Int. Immunopharmacol. 92:107335. doi: 10.1016/j.intimp.2020.107335
Li, X., Xia, Q., Mao, M., Zhou, H., Zheng, L., Wang, Y., et al. (2021). Annexin-A1 SUMOylation regulates microglial polarization after cerebral ischemia by modulating IKKα stability via selective autophagy. Sci. Adv. 7:eabc5539. doi: 10.1126/sciadv.abc5539
Li, Y., Teng, X., Yang, C., Wang, Y., Wang, L., Dai, Y., et al. (2021). Ultrasound controlled anti-inflammatory polarization of platelet decorated microglia for targeted ischemic stroke therapy. Angew Chem. Int. Ed Engl. 60, 5083–5090. doi: 10.1002/anie.202010391
Li, R., Zhou, Y., Zhang, S., Li, J., Zheng, Y., and Fan, X. (2022). The natural (poly)phenols as modulators of microglia polarization via TLR4/NF-κB pathway exert anti-inflammatory activity in ischemic stroke. Eur. J. Pharmacol. 914:174660. doi: 10.1016/j.ejphar.2021.174660
Lian, L., Zhang, Y., Liu, L., Yang, L., Cai, Y., Zhang, J., et al. (2020). Neuroinflammation in ischemic stroke: focus on MicroRNA-mediated polarization of microglia. Front. Mol. Neurosci. 13:612439. doi: 10.3389/fnmol.2020.612439
Lian, X. W., and Luo, B. (2021). Knockdown of NEAT1 induced microglial M2 polarization via miR-374a-5p/NFAT5 axis to inhibit inflammatory response caused by OGD/R. Acta Neurobiol. Exp. (Wars) 81, 362–374.
Liao, S., Wu, J., Liu, R., Wang, S., Luo, J., Yang, Y., et al. (2020). A novel compound DBZ ameliorates neuroinflammation in LPS-stimulated microglia and ischemic stroke rats: role of Akt(Ser473)/GSK3β(Ser9)-mediated Nrf2 activation. Redox Biol. 36:101644. doi: 10.1016/j.redox.2020.101644
Liddelow, S., Guttenplan, K., Clarke, L., Bennett, F., Bohlen, C., Schirmer, L., et al. (2017). Neurotoxic reactive astrocytes are induced by activated microglia. Nature 541, 481–487. doi: 10.1038/nature21029
Lier, J., Streit, W., and Bechmann, I. (2021). Beyond activation: characterizing microglial functional phenotypes. Cells 10:2236. doi: 10.3390/cells10092236
Lin, J., Fang, S., Tang, S., Hu, M., Long, Y., Ghosh, A., et al. (2017). Hippocampal CysLT1R knockdowm or blockade represses LPS-induced depressive behaviors and neuroinflammatory response in mice. Acta Pharmacol. Sin. 38, 477–487. doi: 10.1038/aps.2016.145
Ling, Y., Jin, L., Ma, Q., Huang, Y., Yang, Q., Chen, M., et al. (2021). Salvianolic acid A alleviated inflammatory response mediated by microglia through inhibiting the activation of TLR2/4 in acute cerebral ischemia-reperfusion. Phytomedicine 87:153569. doi: 10.1016/j.phymed.2021.153569
Liu, J., Liao, H., Chen, Y., Zhu, H., Li, X., Liu, J., et al. (2022). Resveratrol inhibits oxidative stress and regulates M1/M2-Type polarization of microglia via mediation of the Nrf2/Shh signaling cascade after OGD/R injury in vitro. J. Pers. Med. 12:2087. doi: 10.3390/jpm12122087
Liu, L., Liu, X., Wang, R., Yan, F., Luo, Y., Chandra, A., et al. (2018). Mild focal hypothermia regulates the dynamic polarization of microglia after ischemic stroke in mice. Neurol Res. 40, 508–515. doi: 10.1080/01616412.2018.1454090
Liu, Q., Zhang, Y., Liu, S., Liu, Y., Yang, X., Liu, G., et al. (2019). Cathepsin C promotes microglia M1 polarization and aggravates neuroinflammation via activation of Ca. J. Neuroinflamm. 16:10. doi: 10.1186/s12974-019-1398-3
Liu, X., Liu, J., Zhao, S., Zhang, H., Cai, W., Cai, M., et al. (2016). Interleukin-4 is essential for microglia/macrophage M2 polarization and long-term recovery after cerebral ischemia. Stroke 47, 498–504. doi: 10.1161/STROKEAHA.115.012079
Liu, Z., Ran, Y., Huang, S., Wen, S., Zhang, W., Liu, X., et al. (2017). Curcumin protects against ischemic stroke by titrating microglia/macrophage polarization. Front. Aging Neurosci. 9:233. doi: 10.3389/fnagi.2017.00233
Lobo-Silva, D., Carriche, G., Castro, A., Roque, S., and Saraiva, M. (2017). Interferon-β regulates the production of IL-10 by toll-like receptor-activated microglia. Glia 65, 1439–1451. doi: 10.1002/glia.23172
Lu, X., Zhang, J., Ding, Y., Wu, J., and Chen, G. (2022). Novel therapeutic strategies for ischemic stroke: recent insights into autophagy. Oxid. Med. Cell Longev. 2022:3450207. doi: 10.1155/2022/3450207
Lu, Y., Zhou, M., Li, Y., Hua, Y., and Fan, Y. (2021). Minocycline promotes functional recovery in ischemic stroke by modulating microglia polarization through STAT1/STAT6 pathways. Biochem. Pharmacol. 186:114464. doi: 10.1016/j.bcp.2021.114464
Lucin, K., O’Brien, C., Bieri, G., Czirr, E., Mosher, K., Abbey, R., et al. (2013). Microglial beclin 1 regulates retromer trafficking and phagocytosis and is impaired in Alzheimer’s disease. Neuron 79, 873–886. doi: 10.1016/j.neuron.2013.06.046
Lugovaya, A., Emanuel, T., Kalinina, N., Mitreikin, V., Artemova, A., and Makienko, A. (2022). The role of autophagy in the regulation of neuroinflammation in acute ischemic stroke (review of literature). Klin Lab Diagn. 67, 391–398. doi: 10.51620/0869-2084-2022-67-7-391-398
Ma, D., Zhang, N., Zhang, Y., and Chen, H. (2021). Salvianolic Acids for Injection alleviates cerebral ischemia/reperfusion injury by switching M1/M2 phenotypes and inhibiting NLRP3 inflammasome/pyroptosis axis in microglia in vivo and in vitro. J. Ethnopharmacol. 270:113776. doi: 10.1016/j.jep.2021.113776
Ma, Y., Wang, J., Wang, Y., and Yang, G. (2017). The biphasic function of microglia in ischemic stroke. Prog. Neurobiol. 157, 247–272. doi: 10.1016/j.pneurobio.2016.01.005
Mabuchi, T., Kitagawa, K., Ohtsuki, T., Kuwabara, K., Yagita, Y., Yanagihara, T., et al. (2000). Contribution of microglia/macrophages to expansion of infarction and response of oligodendrocytes after focal cerebral ischemia in rats. Stroke 31, 1735–1743. doi: 10.1161/01.str.31.7.1735
Mansour, R., Ahmed, M., El-Sahar, A., and El Sayed, N. (2018). Montelukast attenuates rotenone-induced microglial activation/p38 MAPK expression in rats: possible role of its antioxidant, anti-inflammatory and antiapoptotic effects. Toxicol. Appl. Pharmacol. 358, 76–85. doi: 10.1016/j.taap.2018.09.012
Marschallinger, J., Schäffner, I., Klein, B., Gelfert, R., Rivera, F., Illes, S., et al. (2015). Structural and functional rejuvenation of the aged brain by an approved anti-asthmatic drug. Nat. Commun. 6:8466. doi: 10.1038/ncomms9466
Martinez, F. O., and Gordon, S. (2014). The M1 and M2 paradigm of macrophage activation: Time for reassessment. F1000Prime Rep. 6:13. doi: 10.12703/P6-13
Masuda, T., Amann, L., Sankowski, R., Staszewski, O., Lenz, M., and D Errico, P. (2020a). Author correction: novel hexb-based tools for studying microglia in the CNS. Nat. Immunol. 21, 802–815. doi: 10.1038/s41590-020-0707-4
Masuda, T., Amann, L., Sankowski, R., Staszewski, O., Lenz, M., D Errico, P., et al. (2020b). Author correction: novel hexb-based tools for studying microglia in the CNS. Nat. Immunol. 21:1302. doi: 10.1038/s41590-020-0774-6
McAlpine, F., Williamson, L., Tooze, S., and Chan, E. (2013). Regulation of nutrient-sensitive autophagy by uncoordinated 51-like kinases 1 and 2. Autophagy 9, 361–373. doi: 10.4161/auto.23066
Miao, H., Li, R., Han, C., Lu, X., and Zhang, H. (2018). Minocycline promotes posthemorrhagic neurogenesis via M2 microglia polarization via upregulation of the TrkB/BDNF pathway in rats. J. Neurophysiol. 120, 1307–1317. doi: 10.1152/jn.00234.2018
Miki, S., Suzuki, J., Takashima, M., Ishida, M., Kokubo, H., and Yoshizumi, M. (2021). S-1-Propenylcysteine promotes IL-10-induced M2c macrophage polarization through prolonged activation of IL-10R/STAT3 signaling. Sci. Rep. 11:22469. doi: 10.1038/s41598-021-01866-3
Morgan, S., Taylor, D., and Pocock, J. (2004). Microglia release activators of neuronal proliferation mediated by activation of mitogen-activated protein kinase, phosphatidylinositol-3-kinase/Akt and delta-Notch signalling cascades. J. Neurochem. 90, 89–101. doi: 10.1111/j.1471-4159.2004.02461.x
Morrison, H., and Filosa, J. (2016). Sex differences in astrocyte and microglia responses immediately following middle cerebral artery occlusion in adult mice. Neuroscience 339, 85–99. doi: 10.1016/j.neuroscience.2016.09.047
Mosser, D., and Edwards, J. (2008). Exploring the full spectrum of macrophage activation. Nat. Rev. Immunol. 8, 958–969. doi: 10.1038/nri2448
Mota, M., Porrini, V., Parrella, E., Benarese, M., Bellucci, A., Rhein, S., et al. (2020). Neuroprotective epi-drugs quench the inflammatory response and microglial/macrophage activation in a mouse model of permanent brain ischemia. J. Neuroinflamm. 17:361. doi: 10.1186/s12974-020-02028-4
Munson, M., Allen, G., Toth, R., Campbell, D., Lucocq, J., and Ganley, I. (2015). mTOR activates the VPS34-UVRAG complex to regulate autolysosomal tubulation and cell survival. EMBO J. 34, 2272–2290. doi: 10.15252/embj.201590992
Nakanishi, H. (2020). Cathepsin regulation on microglial function. Biochim Biophys. Acta Proteins Proteom. 1868:140465. doi: 10.1016/j.bbapap.2020.140465
Nakanishi, H., Tsukuba, T., Kondou, T., Tanaka, T., and Yamamoto, K. (1993). Transient forebrain ischemia induces increased expression and specific localization of cathepsins E and D in rat hippocampus and neostriatum. Exp. Neurol. 121, 215–223. doi: 10.1006/exnr.1993.1088
Napolitano, G., Esposito, A., Choi, H., Matarese, M., Benedetti, V., Di Malta, C., et al. (2018). mTOR-dependent phosphorylation controls TFEB nuclear export. Nat. Commun. 9:3312. doi: 10.1038/s41467-018-05862-6
Ni, J., Wu, Z., Peterts, C., Yamamoto, K., Qing, H., and Nakanishi, H. (2015). The critical role of proteolytic relay through cathepsins B and E in the phenotypic change of microglia/macrophage. J. Neurosci. 35, 12488–12501. doi: 10.1523/JNEUROSCI.1599-15.2015
Ni, J., Zhao, J., Zhang, X., Reinheckel, T., Turk, V., and Nakanishi, H. (2021). Cathepsin H deficiency decreases hypoxia-ischemia-induced hippocampal atrophy in neonatal mice through attenuated TLR3/IFN-β signaling. J. Neuroinflamm. 18:176. doi: 10.1186/s12974-021-02227-7
Ni, X., Su, Q., Xia, W., Zhang, Y., Jia, K., Su, Z., et al. (2020). Knockdown lncRNA NEAT1 regulates the activation of microglia and reduces AKT signaling and neuronal apoptosis after cerebral ischemic reperfusion. Sci. Rep. 10:19658. doi: 10.1038/s41598-020-71411-1
Nimmerjahn, A., Kirchhoff, F., and Helmchen, F. (2005). Resting microglial cells are highly dynamic surveillants of brain parenchyma in vivo. Science 308, 1314–1318. doi: 10.1126/science.1110647
Oh, W., Wu, C., Kim, S., Facchinetti, V., Julien, L., Finlan, M., et al. (2010). mTORC2 can associate with ribosomes to promote cotranslational phosphorylation and stability of nascent Akt polypeptide. EMBO J. 29, 3939–3951. doi: 10.1038/emboj.2010.271
Okada, R., Zhang, X., Harada, Y., Wu, Z., and Nakanishi, H. (2018). Cathepsin H deficiency in mice induces excess Th1 cell activation and early-onset of EAE though impairment of toll-like receptor 3 cascade. Inflamm. Res. 67, 371–374. doi: 10.1007/s00011-018-1136-9
Orihuela, R., McPherson, C., and Harry, G. (2016). Microglial M1/M2 polarization and metabolic states. Br. J. Pharmacol. 173, 649–665. doi: 10.1111/bph.13139
Parzych, K., and Klionsky, D. (2014). An overview of autophagy: morphology, mechanism, and regulation. Antioxid Redox Signal. 20, 460–473. doi: 10.1089/ars.2013.5371
Peferoen, L., Vogel, D., Ummenthum, K., Breur, M., Heijnen, P., Gerritsen, W., et al. (2015). Activation status of human microglia is dependent on lesion formation stage and remyelination in multiple sclerosis. J. Neuropathol. Exp. Neurol. 74, 48–63. doi: 10.1097/NEN.0000000000000149
Perego, C., Fumagalli, S., and De Simoni, M. (2011). Temporal pattern of expression and colocalization of microglia/macrophage phenotype markers following brain ischemic injury in mice. J. Neuroinflam. 8:174. doi: 10.1186/1742-2094-8-174
Perego, C., Fumagalli, S., and De Simoni, M. (2013). Three-dimensional confocal analysis of microglia/macrophage markers of polarization in experimental brain injury. J. Vis. Exp. 79: 50605. doi: 10.3791/50605
Perego, C., Fumagalli, S., and De Simoni, M. (2019b). Response by perego et al to letter regarding article, “Combined Genetic Deletion of IL (Interleukin)-4, IL-5, IL-9, and IL-13 Does Not Affect Ischemic Brain Injury in Mice”. Stroke 50:e330. doi: 10.1161/STROKEAHA.119.027169
Perego, C., Fumagalli, S., Miteva, K., Kallikourdis, M., and De Simoni, M. (2019a). Combined genetic deletion of IL (Interleukin)-4, IL-5, IL-9, and IL-13 does not affect ischemic brain injury in mice. Stroke 50, 2207–2215. doi: 10.1161/STROKEAHA.119.025196
Plaza-Zabala, A., Sierra-Torre, V., and Sierra, A. (2017). Autophagy and microglia: novel partners in neurodegeneration and aging. Int. J. Mol. Sci. 18:598. doi: 10.3390/ijms18030598
Qi, S., Al Mamun, A., Ngwa, C., Romana, S., Ritzel, R., Arnold, A., et al. (2021). X chromosome escapee genes are involved in ischemic sexual dimorphism through epigenetic modification of inflammatory signals. J. Neuroinflam. 18:70. doi: 10.1186/s12974-021-02120-3
Qin, C., Fan, W., Liu, Q., Shang, K., Murugan, M., Wu, L., et al. (2017). Fingolimod protects against ischemic white matter damage by modulating microglia toward m2 polarization via STAT3 pathway. Stroke 48, 3336–3346. doi: 10.1161/STROKEAHA.117.018505
Qin, C., Liu, Q., Hu, Z., Zhou, L., Shang, K., Bosco, D., et al. (2018). Microglial TLR4-dependent autophagy induces ischemic white matter damage. Theranostics 8, 5434–5451. doi: 10.7150/thno.27882
Rabinovich, G., and Toscano, M. (2009). Turning ‘sweet’ on immunity: galectin-glycan interactions in immune tolerance and inflammation. Nat. Rev. Immunol. 9, 338–352. doi: 10.1038/nri2536
Rahimian, R., Lively, S., Abdelhamid, E., Lalancette-Hebert, M., Schlichter, L., Sato, S., et al. (2019). Delayed Galectin-3-Mediated reprogramming of microglia after stroke is protective. Mol. Neurobiol. 56, 6371–6385. doi: 10.1007/s12035-019-1527-0
Ran, Y., Qie, S., Gao, F., Ding, Z., Yang, S., Tian, G., et al. (2021). Baicalein ameliorates ischemic brain damage through suppressing proinflammatory microglia polarization via inhibiting the TLR4/NF-κB and STAT1 pathway. Brain Res. 1770:147626. doi: 10.1016/j.brainres.2021.147626
Ransohoff, R., and Brown, M. (2012). Innate immunity in the central nervous system. J. Clin. Invest. 122, 1164–1171. doi: 10.1172/JCI58644
Ren, M., Guo, Y., Wei, X., Yan, S., Qin, Y., Zhang, X., et al. (2018). TREM2 overexpression attenuates neuroinflammation and protects dopaminergic neurons in experimental models of Parkinson’s disease. Exp. Neurol. 302, 205–213. doi: 10.1016/j.expneurol.2018.01.016
Rinaldi, M., Thomas, L., Mathieu, P., Carabias, P., Troncoso, M., Pasquini, J., et al. (2016). Galectin-1 circumvents lysolecithin-induced demyelination through the modulation of microglial polarization/phagocytosis and oligodendroglial differentiation. Neurobiol. Dis. 96, 127–143. doi: 10.1016/j.nbd.2016.09.003
Ruganzu, J., Zheng, Q., Wu, X., He, Y., Peng, X., Jin, H., et al. (2021). TREM2 overexpression rescues cognitive deficits in APP/PS1 transgenic mice by reducing neuroinflammation via the JAK/STAT/SOCS signaling pathway. Exp. Neurol. 336:113506. doi: 10.1016/j.expneurol.2020.113506
Salamanca, L., Mechawar, N., Murai, K., Balling, R., Bouvier, D., and Skupin, A. (2019). MIC-MAC: an automated pipeline for high-throughput characterization and classification of three-dimensional microglia morphologies in mouse and human postmortem brain samples. Glia 67, 1496–1509. doi: 10.1002/glia.23623
Sanjurjo, L., Aran, G., Téllez, É, Amézaga, N., Armengol, C., López, D., et al. (2018). CD5L promotes M2 macrophage polarization through autophagy-mediated upregulation of ID3. Front. Immunol. 9:480. doi: 10.3389/fimmu.2018.00480
Satriotomo, I., Bowen, K., and Vemuganti, R. (2006). JAK2 and STAT3 activation contributes to neuronal damage following transient focal cerebral ischemia. J. Neurochem. 98, 1353–1368. doi: 10.1111/j.1471-4159.2006.04051.x
Scheu, S., Ali, S., Mann-Nüttel, R., Richter, L., Arolt, V., Dannlowski, U., et al. (2019). Interferon β-Mediated protective functions of microglia in central nervous system autoimmunity. Int. J. Mol. Sci. 20:190. doi: 10.3390/ijms20010190
Schilling, M., Besselmann, M., Müller, M., Strecker, J., Ringelstein, E., and Kiefer, R. (2005). Predominant phagocytic activity of resident microglia over hematogenous macrophages following transient focal cerebral ischemia: an investigation using green fluorescent protein transgenic bone marrow chimeric mice. Exp. Neurol. 196, 290–297. doi: 10.1016/j.expneurol.2005.08.004
Shao, Z., and Liu, Z. (2015). Neuroinflammation and neuronal autophagic death were suppressed via Rosiglitazone treatment: new evidence on neuroprotection in a rat model of global cerebral ischemia. J. Neurol. Sci. 349, 65–71. doi: 10.1016/j.jns.2014.12.027
Shi, Q., Wang, H., Liu, Z., Fang, S., Song, X., Lu, Y., et al. (2015). HAMI 3379, a CysLT2R antagonist, dose- and time-dependently attenuates brain injury and inhibits microglial inflammation after focal cerebral ischemia in rats. Neuroscience 291, 53–69. doi: 10.1016/j.neuroscience.2015.02.002
Shi, W., Zhao, C., Zhao, B., Shi, Q., Zhang, L., Wang, Y., et al. (2012). Aggravated inflammation and increased expression of cysteinyl leukotriene receptors in the brain after focal cerebral ischemia in AQP4-deficient mice. Neurosci. Bull. 28, 680–692. doi: 10.1007/s12264-012-1281-z
Siddiqui, T., Lively, S., and Schlichter, L. (2016). Complex molecular and functional outcomes of single versus sequential cytokine stimulation of rat microglia. J. Neuroinflam. 13:66. doi: 10.1186/s12974-016-0531-9
Sierra, A., Encinas, J., Deudero, J., Chancey, J., Enikolopov, G., Overstreet-Wadiche, L., et al. (2010). Microglia shape adult hippocampal neurogenesis through apoptosis-coupled phagocytosis. Cell Stem Cell 7, 483–495. doi: 10.1016/j.stem.2010.08.014
Srimat Kandadai, K., Kotur, M., Dokalis, N., Amrein, I., Keller, C., Münz, C., et al. (2021). ATG5 in microglia does not contribute vitally to autoimmune neuroinflammation in mice. Autophagy 17, 3566–3576. doi: 10.1080/15548627.2021.1883880
Sudduth, T., Schmitt, F., Nelson, P., and Wilcock, D. (2013). Neuroinflammatory phenotype in early Alzheimer’s disease. Neurobiol. Aging 34, 1051–1059. doi: 10.1016/j.neurobiolaging.2012.09.012
Sun, L., Wu, Z., Hayashi, Y., Peters, C., Tsuda, M., Inoue, K., et al. (2012). Microglial cathepsin B contributes to the initiation of peripheral inflammation-induced chronic pain. J. Neurosci. 32, 11330–11342. doi: 10.1523/JNEUROSCI.0677-12.2012
Sundararajan, S., Gamboa, J. L., Victor, N. A., Wanderi, E. W., Lust, W. D., and Landreth, G. E. (2005). Peroxisome proliferator-activated receptor-gamma ligands reduce inflammation and infarction size in transient focal ischemia. Neuroscience 130, 685–696. doi: 10.1016/j.neuroscience.2004.10.021
Takagi, T., Kitashoji, A., Iwawaki, T., Tsuruma, K., Shimazawa, M., Yoshimura, S., et al. (2014). Temporal activation of Nrf2 in the penumbra and Nrf2 activator-mediated neuroprotection in ischemia-reperfusion injury. Free Radic Biol. Med. 72, 124–133. doi: 10.1016/j.freeradbiomed.2014.04.009
Takagi, Y., Harada, J., Chiarugi, A., and Moskowitz, M. (2002). STAT1 is activated in neurons after ischemia and contributes to ischemic brain injury. J. Cereb. Blood Flow Metab. 22, 1311–1318. doi: 10.1097/01.WCB.0000034148.72481.F4
Tanaka, M., Sackett, S., and Zhang, Y. (2020). Endocannabinoid modulation of microglial phenotypes in neuropathology. Front. Neurol. 11:87. doi: 10.3389/fneur.2020.00087
Tang, R., Qi, R., and Liu, H. (2019). Interleukin-4 affects microglial autophagic flux. Neural Regen. Res. 14, 1594–1602. doi: 10.4103/1673-5374.255975
Tao, W., Hu, Y., Chen, Z., Dai, Y., and Qi, M. (2021). Magnolol attenuates depressive-like behaviors by polarizing microglia towards the M2 phenotype through the regulation of Nrf2/HO-1/NLRP3 signaling pathway. Phytomedicine 91:153692. doi: 10.1016/j.phymed.2021.153692
Theron, A., Steel, H., Tintinger, G., Gravett, C., Anderson, R., and Feldman, C. (2014). Cysteinyl leukotriene receptor-1 antagonists as modulators of innate immune cell function. J. Immunol. Res. 2014:608930. doi: 10.1155/2014/608930
Tian, X., Liu, H., Xiang, F., Xu, L., and Dong, Z. (2019). β-Caryophyllene protects against ischemic stroke by promoting polarization of microglia toward M2 phenotype via the TLR4 pathway. Life Sci. 237:116915. doi: 10.1016/j.lfs.2019.116915
Ting, S., Zhao, X., Zheng, X., and Aronowski, J. (2020). Excitatory pathway engaging glutamate, calcineurin, and NFAT upregulates IL-4 in ischemic neurons to polarize microglia. J. Cereb. Blood Flow Metab. 40, 513–527. doi: 10.1177/0271678X19838189
Tsukahara, T., Haniu, H., Uemura, T., and Matsuda, Y. (2020). Therapeutic potential of porcine liver decomposition product: new insights and perspectives for microglia-mediated neuroinflammation in neurodegenerative diseases. Biomedicines 8:446. doi: 10.3390/biomedicines8110446
Venkat, P., Cui, C., Chopp, M., Zacharek, A., Wang, F., Landschoot-Ward, J., et al. (2019). MiR-126 mediates brain endothelial cell exosome treatment-induced neurorestorative effects after stroke in type 2 diabetes mellitus mice. Stroke 50, 2865–2874. doi: 10.1161/STROKEAHA.119.025371
Victor, N., Wanderi, E., Gamboa, J., Zhao, X., Aronowski, J., Deininger, K., et al. (2006). Altered PPARgamma expression and activation after transient focal ischemia in rats. Eur. J. Neurosci. 24, 1653–1663. doi: 10.1111/j.1460-9568.2006.05037.x
Walker, D., and Lue, L. (2015). Immune phenotypes of microglia in human neurodegenerative disease: challenges to detecting microglial polarization in human brains. Alzheimers Res. Ther. 7:56. doi: 10.1186/s13195-015-0139-9
Wang, D., Liu, F., Zhu, L., Lin, P., Han, F., Wang, X., et al. (2020). FGF21 alleviates neuroinflammation following ischemic stroke by modulating the temporal and spatial dynamics of microglia/macrophages. J. Neuroinflam. 17:257. doi: 10.1186/s12974-020-01921-2
Wang, N., Nie, H., Zhang, Y., Han, H., Wang, S., Liu, W., et al. (2022). Dexmedetomidine exerts cerebral protective effects against cerebral ischemic injury by promoting the polarization of M2 microglia via the Nrf2/HO-1/NLRP3 pathway. Inflamm. Res. 71, 93–106. doi: 10.1007/s00011-021-01515-5
Wang, P., Shao, B., Deng, Z., Chen, S., Yue, Z., and Miao, C. (2018). Autophagy in ischemic stroke. Prog. Neurobiol. 163-164, 98–117. doi: 10.1016/j.pneurobio.2018.01.001
Wang, X., Ma, J., Fu, Q., Zhu, L., Zhang, Z., Zhang, F., et al. (2017). Role of hypoxia-inducible factor-1α in autophagic cell death in microglial cells induced by hypoxia. Mol. Med. Rep. 15, 2097–2105. doi: 10.3892/mmr.2017.6277
Wang, Y., Huang, Y., Xu, Y., Ruan, W., Wang, H., Zhang, Y., et al. (2018). A dual AMPK/Nrf2 activator reduces brain inflammation after stroke by enhancing microglia M2 polarization. Antioxid. Redox Signal. 28, 141–163. doi: 10.1089/ars.2017.7003
Wang, Y., Yang, Y., Zhang, S., Li, C., and Zhang, L. (2020). Modulation of neuroinflammation by cysteinyl leukotriene 1 and 2 receptors: implications for cerebral ischemia and neurodegenerative diseases. Neurobiol. Aging 87, 1–10. doi: 10.1016/j.neurobiolaging.2019.12.013
Wimmer, I., Zrzavy, T., and Lassmann, H. (2018). Neuroinflammatory responses in experimental and human stroke lesions. J. Neuroimmunol. 323, 10–18. doi: 10.1016/j.jneuroim.2018.07.003
Wu, J., Wang, B., Li, M., Shi, Y., Wang, C., and Kang, Y. (2019). Network pharmacology identification of mechanisms of cerebral ischemia injury amelioration by Baicalin and Geniposide. Eur. J. Pharmacol. 859:172484. doi: 10.1016/j.ejphar.2019.172484
Wu, R., Li, X., Xu, P., Huang, L., Cheng, J., Huang, X., et al. (2017). TREM2 protects against cerebral ischemia/reperfusion injury. Mol. Brain 10:20. doi: 10.1186/s13041-017-0296-9
Xia, C., Zhang, S., Chu, S., Wang, Z., Song, X., Zuo, W., et al. (2016). Autophagic flux regulates microglial phenotype according to the time of oxygen-glucose deprivation/reperfusion. Int. Immunopharmacol. 39, 140–148. doi: 10.1016/j.intimp.2016.06.030
Xiong, X., Xu, L., Wei, L., White, R., Ouyang, Y., and Giffard, R. G. (2015). IL-4 is required for sex differences in vulnerability to focal ischemia in mice. Stroke 46, 2271–2276. doi: 10.1161/STROKEAHA.115.008897
Xu, S., Wu, D., and Ji, X. (2019). Letter by Xu et al regarding article, “combined genetic deletion of IL (Interleukin)-4, IL-5, IL-9, and IL-13 does not affect ischemic brain injury in mice”. Stroke 50:e329. doi: 10.1161/STROKEAHA.119.027144
Xu, Y., Propson, N., Du, S., Xiong, W., and Zheng, H. (2021). Autophagy deficiency modulates microglial lipid homeostasis and aggravates tau pathology and spreading. Proc. Natl. Acad. Sci. U S A. 118:e2023418118. doi: 10.1073/pnas.2023418118
Xu, Y., Shen, J., and Ran, Z. (2020). Emerging views of mitophagy in immunity and autoimmune diseases. Autophagy 16, 3–17. doi: 10.1080/15548627.2019.1603547
Xue, Y., Nie, D., Wang, L., Qiu, H., Ma, L., Dong, M., et al. (2021). Microglial polarization: novel therapeutic strategy against ischemic stroke. Aging Dis. 12, 466–479. doi: 10.14336/AD.2020.0701
Yang, C., Gong, S., Chen, X., Wang, M., Zhang, L., and Hu, C. (2021). Analgecine regulates microglia polarization in ischemic stroke by inhibiting NF-κB through the TLR4 MyD88 pathway. Int. Immunopharmacol. 99:107930. doi: 10.1016/j.intimp.2021.107930
Yang, M., Liu, J., Shao, J., Qin, Y., Ji, Q., Zhang, X., et al. (2014). Cathepsin S-mediated autophagic flux in tumor-associated macrophages accelerate tumor development by promoting M2 polarization. Mol. Cancer 13:43. doi: 10.1186/1476-4598-13-43
Yang, S., Wang, H., Yang, Y., Wang, R., Wang, Y., Wu, C., et al. (2019). Baicalein administered in the subacute phase ameliorates ischemia-reperfusion-induced brain injury by reducing neuroinflammation and neuronal damage. Biomed. Pharmacother. 117:109102. doi: 10.1016/j.biopha.2019.109102
Yang, Z., Zhang, N., Shen, H., Lin, C., Lin, L., and Yuan, B. (2014). Microglial activation with reduction in autophagy limits white matter lesions and improves cognitive defects during cerebral hypoperfusion. Curr. Neurovasc. Res. 11, 223–229. doi: 10.2174/1567202611666140520124407
Yao, X., Liu, S., Ding, W., Yue, P., Jiang, Q., Zhao, M., et al. (2017). TLR4 signal ablation attenuated neurological deficits by regulating microglial M1/M2 phenotype after traumatic brain injury in mice. J. Neuroimmunol. 310, 38–45. doi: 10.1016/j.jneuroim.2017.06.006
Ye, X., Zhu, M., Che, X., Wang, H., Liang, X., Wu, C., et al. (2020). Lipopolysaccharide induces neuroinflammation in microglia by activating the MTOR pathway and downregulating Vps34 to inhibit autophagosome formation. J. Neuroinflammation. 17:18. doi: 10.1186/s12974-019-1644-8
York, E., LeDue, J., Bernier, L., and MacVicar, B. (2018). 3DMorph automatic analysis of microglial morphology in three dimensions from ex vivo and in vivo imaging. eNeuro 5:ENEURO.0266-18.2018. doi: 10.1523/ENEURO.0266-18.2018
Yu, L., Su, X., Li, S., Zhao, F., Mu, D., and Qu, Y. (2020). Microglia and their promising role in ischemic brain injuries: an update. Front. Cell Neurosci. 14:211. doi: 10.3389/fncel.2020.00211
Yu, Z., Sun, D., Feng, J., Tan, W., Fang, X., Zhao, M., et al. (2015). MSX3 switches microglia polarization and protects from inflammation-induced demyelination. J. Neurosci. 35, 6350–6365. doi: 10.1523/JNEUROSCI.2468-14.2015
Zang, J., Wu, Y., Su, X., Zhang, T., Tang, X., Ma, D., et al. (2020). Inhibition of PDE1-B by vinpocetine regulates microglial exosomes and polarization through enhancing autophagic flux for neuroprotection against ischemic stroke. Front. Cell Dev. Biol. 8:616590. doi: 10.3389/fcell.2020.616590
Zanier, E., Fumagalli, S., Perego, C., Pischiutta, F., and De Simoni, M. (2015). Shape descriptors of the “never resting” microglia in three different acute brain injury models in mice. Intensive Care Med. Exp. 3:39. doi: 10.1186/s40635-015-0039-0
Zhai, Q., Li, F., Chen, X., Jia, J., Sun, S., Zhou, D., et al. (2017). Triggering receptor expressed on myeloid cells 2, a novel regulator of immunocyte phenotypes, confers neuroprotection by relieving neuroinflammation. Anesthesiology 127, 98–110. doi: 10.1097/ALN.0000000000001628
Zhang, G., Xia, F., Zhang, Y., Zhang, X., Cao, Y., Wang, L., et al. (2016). Ginsenoside Rd is efficacious against acute ischemic stroke by suppressing microglial proteasome-mediated inflammation. Mol. Neurobiol. 53, 2529–2540. doi: 10.1007/s12035-015-9261-8
Zhang, L., Liu, Y., Wang, S., Long, L., Zang, Q., Ma, J., et al. (2021). Vagus nerve stimulation mediates microglia M1/2 polarization via inhibition of TLR4 pathway after ischemic stroke. Biochem. Biophys. Res. Commun. 577, 71–79. doi: 10.1016/j.bbrc.2021.09.004
Zhang, X., Wang, X., Xu, D., Yu, S., Shi, Q., Zhang, L., et al. (2013). HAMI 3379, a CysLT2 receptor antagonist, attenuates ischemia-like neuronal injury by inhibiting microglial activation. J. Pharmacol. Exp. Ther. 346, 328–341. doi: 10.1124/jpet.113.203604
Zhang, X., Yan, F., Cui, J., Wu, Y., Luan, H., Yin, M., et al. (2017). Triggering receptor expressed on myeloid cells 2 overexpression inhibits proinflammatory cytokines in lipopolysaccharide-stimulated microglia. Med. Inflamm. 2017:9340610. doi: 10.1155/2017/9340610
Zhang, X., Zhu, X., Ji, B., Cao, X., Yu, L., Zhang, Y., et al. (2019). LncRNA-1810034E14Rik reduces microglia activation in experimental ischemic stroke. J. Neuroinflam. 16:75. doi: 10.1186/s12974-019-1464-x
Zhao, R., Ying, M., Gu, S., Yin, W., Li, Y., Yuan, H., et al. (2019). Cysteinyl leukotriene receptor 2 is involved in inflammation and neuronal damage by mediating microglia M1/M2 polarization through NF-κB pathway. Neuroscience 422, 99–118. doi: 10.1016/j.neuroscience.2019.10.048
Zhao, S., Wang, C., Xu, H., Wu, W., Chu, Z., Ma, L., et al. (2017). Age-related differences in interferon regulatory factor-4 and -5 signaling in ischemic brains of mice. Acta Pharmacol. Sin. 38, 1425–1434. doi: 10.1038/aps.2017.122
Zhao, X., Wang, H., Sun, G., Zhang, J., Edwards, N. J., and Aronowski, J. (2015). Neuronal Interleukin-4 as a modulator of microglial pathways and ischemic brain damage. J. Neurosci. 35, 11281–11291. doi: 10.1523/JNEUROSCI.1685-15.2015
Zhao, Y., Gan, Y., Xu, G., Hua, K., and Liu, D. (2020a). Exosomes from MSCs overexpressing microRNA-223-3p attenuate cerebral ischemia through inhibiting microglial M1 polarization mediated inflammation. Life Sci. 260:118403. doi: 10.1016/j.lfs.2020.118403
Zhao, Y., Gan, Y., Xu, G., Yin, G., and Liu, D. (2020b). MSCs-Derived exosomes attenuate acute brain injury and inhibit microglial inflammation by reversing CysLT2R-ERK1/2 mediated microglia M1 polarization. Neurochem. Res. 45, 1180–1190. doi: 10.1007/s11064-020-02998-0
Zhong, Y., Yin, B., Ye, Y., Dekhel, O., Xiong, X., Jian, Z., et al. (2021). The bidirectional role of the JAK2/STAT3 signaling pathway and related mechanisms in cerebral ischemia-reperfusion injury. Exp. Neurol. 341:113690. doi: 10.1016/j.expneurol.2021.113690
Zhou, D., Ji, L., and Chen, Y. (2020). TSPO modulates IL-4-Induced microglia/macrophage M2 polarization via PPAR-γ pathway. J. Mol. Neurosci. 70, 542–549. doi: 10.1007/s12031-019-01454-1
Zhu, J., Cao, D., Guo, C., Liu, M., Tao, Y., Zhou, J., et al. (2019). Berberine facilitates angiogenesis against ischemic stroke through modulating microglial polarization via AMPK signaling. Cell Mol. Neurobiol. 39, 751–768. doi: 10.1007/s10571-019-00675-7
Zhu, T., Meng, X., Dong, D., Zhao, L., Qu, M., Sun, G., et al. (2021). Xuesaitong injection (lyophilized) combined with aspirin and clopidogrel protect against focal cerebral ischemic/reperfusion injury in rats by suppressing oxidative stress and inflammation and regulating the NOX2/IL-6/STAT3 pathway. Ann. Palliat. Med. 10, 1650–1667. doi: 10.21037/apm-20-1681
Zhu, Y., Yu, J., Gong, J., Shen, J., Ye, D., Cheng, D., et al. (2021). PTP1B inhibitor alleviates deleterious microglial activation and neuronal injury after ischemic stroke by modulating the ER stress-autophagy axis via PERK signaling in microglia. Aging (Albany NY) 13, 3405–3427. doi: 10.18632/aging.202272
Keywords: microglia, polarization, neuroinflammation, autophagy, cerebral ischemia
Citation: Wang H, Li J, Zhang H, Wang M, Xiao L, Wang Y and Cheng Q (2023) Regulation of microglia polarization after cerebral ischemia. Front. Cell. Neurosci. 17:1182621. doi: 10.3389/fncel.2023.1182621
Received: 09 March 2023; Accepted: 22 May 2023;
Published: 08 June 2023.
Edited by:
Ertugrul Kilic, Istanbul Medipol University, TürkiyeCopyright © 2023 Wang, Li, Zhang, Wang, Xiao, Wang and Cheng. This is an open-access article distributed under the terms of the Creative Commons Attribution License (CC BY). The use, distribution or reproduction in other forums is permitted, provided the original author(s) and the copyright owner(s) are credited and that the original publication in this journal is cited, in accordance with accepted academic practice. No use, distribution or reproduction is permitted which does not comply with these terms.
*Correspondence: Qiong Cheng, cq1981@ntu.edu.cn