- National Brain Research Centre, Gurgaon, Haryana, India
Neurotropic viruses can cross the otherwise dynamically regulated blood-brain barrier (BBB) and affect the brain cells. Zika virus (ZIKV) is an enveloped neurotropic Flavivirus known to cause severe neurological complications, such as encephalitis and fetal microcephaly. In the present study, we employed human brain microvascular endothelial cells (hBMECs) and astrocytes derived from human progenitors to establish a physiologically relevant BBB model. We used this model to investigate the effects of ZIKV envelope (E) protein on properties of cells comprising the BBB. E protein is the principal viral protein involved in interaction with host cell surface receptors, facilitating the viral entry. Our findings show that the presence of ZIKV E protein leads to activation of both hBMECs and astrocytes. In hBMECs, we observed a decrease in the expression of crucial endothelial junction proteins such as ZO-1, Occludin and VE-Cadherin, which are vital in establishment and maintenance of the BBB. Consequently, the ZIKV E protein induced changes in BBB integrity and permeability. We also found upregulation of genes involved in leukocyte recruitment along with increased proinflammatory chemokines and cytokines upon exposure to E protein. Additionally, the E protein also led to astrogliosis, evident from the elevated expression of GFAP and Vimentin. Both cell types comprising the BBB exhibited inflammatory response upon exposure to E protein which may influence viral access into the central nervous system (CNS) and subsequent infection of other CNS cells. Overall, our study provides valuable insights into the transient changes that occur at the site of BBB upon ZIKV infection.
Introduction
Zika virus (ZIKV) is an enveloped, single stranded, positive sense RNA virus from the family Flaviviridae. It is an arthropod-borne virus spread through Aedes aegypti and Aedes albopictus mosquitoes and also transmitted through bodily fluids (Chimelli et al., 2017; Cordeiro et al., 2017; Miner and Diamond, 2017). It is the only Flavivirus known to cause teratogenic effects in humans, primarily resulting in abnormally small head circumference-microcephaly, intracranial calcification, and fetal death in some cases. ZIKV-associated clinical implications in adults predominantly include Guillain-Barré syndrome (GBS), and other neurological complications such as encephalitis, meningitis, encephalopathy (Muñoz et al., 2016, 2017; Carod-Artal, 2018; de Almeida Oliveira Evangelista et al., 2021). The genome of ZIKV is ∼10.8 kb and encodes for single polyprotein which is later processed into three structural- capsid (C), envelope (E), premembrane/membrane (PrM), and seven non-structural proteins- NS1, NS2A, NS2B, NS3, NS4A, NS4B, and NS5 (Kuno and Chang, 2007).
The blood-brain barrier (BBB) is a complex and well-regulated interface between the peripheral and the central nervous system (CNS). BBB plays a pivotal role in maintaining the homeostasis of the CNS which includes limiting passive diffusion of polar molecules from bloodstream into the brain, supply of nutrients and oxygen as well as efflux of harmful metabolites and xenobiotics, maintaining the water-electrolyte balance, and regulating the circulation of immune cells across the barrier (Luissint et al., 2012; Helms et al., 2015). BBB consists of unique microvascular endothelial cells which line the cerebral capillaries associated with other cell types such as pericytes and astrocytic-end feet processes, which together form the “neurovascular unit”. Brain microvascular endothelial cells (BMECs) are the primary component of BBB which are characterized by lack of fenestrations, scarce pinocytosis and presence of elaborate trans-membrane transport molecules (Joo, 2002; Naik and Cucullo, 2012; Helms et al., 2015). Presence of tight junctions (Occludin, claudin and zonula occludens-ZO) is the central feature of BMECs which are crucial in establishing and maintaining the BBB integrity. Inter-endothelial junctions also include adherens junctions (AJ), in which the primary component is vascular endothelium (VE)-Cadherin. The mutual interactions between the cells comprising the neurovascular unit are crucial for barrier formation and maintaining its integrity.
A characteristic feature of CNS viral infections is disruption of BBB which can occur due to viral replication or a consequence of neuroinflammation. Certain viral factors and host inflammatory responses can adversely affect the BBB integrity. Other flaviviruses, Japanese encephalitis virus (JEV) and West Nile virus (WNV) are known to disrupt BBB, whereas, the exact mechanisms underlying ZIKV neuroinvasion and encephalitis remain elusive. The compromised integrity of BBB in JEV and WNV infections occurs as a result of compromised tight junction complexes (Chaturvedi et al., 1991; Roe et al., 2012). ZIKV is detected in microcephalic brains of fetuses and is found to be neurotropic even in adults with an intact BBB. Previous studies have indicated that ZIKV is able to cross BBB using in vitro and animal BBB models (Alimonti et al., 2018; Clé et al., 2020). Characteristic BMECs of BBB are known to serve as reservoir for viral replication (Mladinich et al., 2017). However, a comprehensive understanding of the molecular mechanisms underlying the ZIKV-induced alterations in the properties of BBB cells remains an area that requires further investigation, as it represents a gap in the current knowledge of ZIKV pathophysiology.
Viral entry into host cells is facilitated by interaction of ZIKV envelope (E) protein with host surface receptors upon attachment. Evidences show the advent of ZIKV neuroinvasion and its virulence results from the genetic variation of 10 amino acids near the N-linked glycosylation site of E protein (Annamalai et al., 2017; Carbaugh et al., 2019). The N-linked glycosylation of E protein is a crucial determinant for its virulence. Asian ZIKV strains (H/PF/2013 and PRVABC59) are glycosylated at N154 of the E protein. This E glycosylation augments the transmission, viral attachment, and its pathogenesis (Faye et al., 2014; Carbaugh et al., 2019). In the present study, we investigated the effects of structural E protein of ZIKV on the properties of human brain microvascular endothelial cells (hBMECs) and human progenitor derived astrocytes (PDA) and how these interactions influence the BBB phenotype.
Materials and methods
Human primary progenitor derived astrocytes
Human fetal brain tissue samples (10–14 weeks old) were collected with informed consent of the mother(s) from elective abortions. Samples were processed as per approved protocols by Institutional Human Ethics Committee in compliance with recommendations of Indian Council of Medical Research, New Delhi, India. Cells isolated from the telencephalon region were used to derive human neural progenitor cells (hNPCs) by culturing and passaging under aseptic conditions. hNPCs were then cultured on Poly-D-lysine (Sigma-Aldrich, St. Louis, MO, USA, Cat# P7280) coated culture flasks in neurobasal media (Invitrogen, San Diego, CA, USA, Cat# 21103-049) containing growth factors- epidermal growth factor (EGF, 20 ng/ml) (Peprotech, Rocky Hill, NJ, USA, Cat#AF-100-15-500UG) and fibroblast growth factor (FGF, 25 ng/ml) (Peprotech, Rocky Hill, NJ, USA, Cat#100-18B-50UG) supplemented with neuronal survival factor-1 (NSF-1) (Lonza, Charles City, IA, USA, Cat# CC-4323), N2 supplement (Invitrogen, San Diego, CA, USA, Cat# 17505-048), bovine serum albumin (BSA) (Sigma-Aldrich, St. Louis, MO, USA, Cat# A9418), glutamine (Sigma-Aldrich, St. Louis, MO, USA, Cat# G7513) and antibiotics- penicillin-streptomycin (Invitrogen, San Diego, CA, USA, Cat# 15140122), and gentamycin (Sigma-Aldrich, St. Louis, MO, USA, Cat# G1522). hNPCs were characterized by expression of their respective functional markers where more than 99% cells were positive for Nestin and SOX-2 (Supplementary Figures 1A–F). These cells were further assessed for their ability to differentiate into neurons. More than 95% of differentiated cells were positive for neuronal markers MAP2 and Tuj1 (Supplementary Figures 1G–L).
Human neural progenitor cells (hNPCs) were differentiated into an astrocytic-lineage by replacing with astrocyte growth media- complete minimal essential media (CMEM) (Sigma-Aldrich, St. Louis, MO, USA, Cat# M0268-10 × 1L) supplemented with 10% fetal bovine serum (FBS) (Gibco, CA, USA, Cat# 10270-106). The differentiation was continued for 21 days with half media change and splitting. More than 95% cells were immunopositive for Vimentin and glial fibrillary acidic protein (GFAP) (Supplementary Figures 1M–R). The mature progenitor derived astrocytes (PDA) were used for further experiments. At least three different fetal tissues were used for the study.
Human primary brain microvascular endothelial cells
Primary human cerebral cortex microvascular endothelial cells (Passage 3, 12 CPD in vitro, ACBRI 376) were procured from Cell Systems (Kirkland, WA 98034, USA). Brain microvascular endothelial cells (BMECs) were cultured in Complete Classic Media (Kirkland, WA 98034, USA Cat#4Z0-500) supplemented with culture boost (Kirkland, WA 98034, USA Cat#4CB-500) and antibiotics- penicillin-streptomycin and gentamycin. Cells were grown in Attachment Factor (Kirkland, WA 98034, USA Cat#4Z0-210) coated culture-ware. Media was changed every 2 days and cells were split (> 80% confluent) with Passage Reagent Group (Kirkland, WA 98034, USA Cat#4Z0-800). Cultured hBMECs were found to be immunopositive for characteristic VE-Cadherin and ZO-1 (Supplementary Figures 2A–F). Cells were used at passage 8–12 for different assays.
Establishment of contact-based human blood-brain barrier model system using co-culture of primary progenitor derived astrocytes and primary brain microvascular endothelial cells
For BBB monoculture and/or co-culture, Polyester (PET) (3.0 μm pore, 12 mm diameter, collagen-coated, Corning Life Sciences, ME, USA Cat# 3462) Transwell inserts were used. For establishing co-culture and mimic the in vivo microenvironment, the astrocytes and hBMECs were seeded in close proximity. For that transwell inserts were inverted and a new external well was created using a small piece of sterile elastic silicon tubing. The luminal side was sealed with smaller enclosed silicon tubing to avoid leakage of media. A total of 35,000 PDAs were seeded onto the abluminal side of inserts and were allowed to adhere for 5–6 h in CMEM. The inserts were inverted back to its upright position after removing the tubing gently, and cells were allowed to grow for 24 h in CMEM. Next day, 75,000 transfected (with empty vector or ZIKV E-protein) BMECs were seeded onto the luminal side and both cell types were allowed to grow in ECs media for 24 h and were harvested for different assays (Supplementary Figure 3A). Cells grown on transwell membrane were found to be immunopositive for astrocytic marker (GFAP) and endothelial marker (VE-Cadherin) (Supplementary Figures 3B–E). In contact-based co-culture of BBB, astrocytes were able to extend their processes towards hBMECs grown on the luminal side as shown by the expression of GFAP in the total protein isolated from the luminal side of transwell (Supplementary Figures 3F–G).
Immunocytochemistry
Experiments were performed in eight-well and/or four-well Permanox chamber slides. Cells were seeded at 20,000 cells/well and 50,000 cells/well, respectively. Cells were fixed with 4% paraformaldehyde (PFA) for 20 min, followed by three washes with 1X PBS. Blocking and permeabilization was done with 4% BSA and 0.3% Triton-X-100. Cells were probed with respective primary antibodies overnight at 4°C: anti-ZO-1 (1:500, Invitrogen, San Diego, CA, USA, Cat# 61-7300), anti-VE-Cadherin (1:500, Abcam, Cambridge, UK, Cat# ab33168), anti- Occludin (1 μg/ml, Invitrogen, San Diego, CA, USA, Cat# 40-4700) anti- GFAP (1:2,000, Dako, USA, Cat# Z0334), anti-GFP (1:2,000, Abcam, Cambridge, UK, Cat# ab290) and anti-Vimentin (1:2,000, Santa Cruz, USA, Cat# sc-6260). Cells were washed three times with 1X PBS and incubated with suitable fluorophore tagged secondary antibodies for 1 h (1: 2,000, Invitrogen, San Diego, CA, USA). Cells were washed with 1X PBS before mounting, using hard set mounting media containing DAPI (Vector Labs, Burlingame, CA, USA). A total of 6–7 random images were taken using AxioImager.Z1 microscope (Zeiss, Germany) from each group by person blinded to the experimental groups The image analysis was done using ImageJ software (NIH, USA).
Immunofluorescence staining of hBMECs and astrocytes cultured on permeable PET membrane was performed as described (Agrawal et al., 2013). Transwell inserts were washed twice with cold 1X PBS. Cells on the membrane were fixed with cold methanol (−20°C) for 10 min followed by washing with cold 1X PBS thrice. Membrane was then released from the transwell apparatus using a scalpel blade into a 24-well plate. Cells were incubated with immunofluorescence (IMF) buffer (20 mM HEPES, pH7.5, 0.1% Triton-X-100, 150 mM NaCl, 5 mM EDTA and 0.02% sodium azide) for 5 min at room temperature (RT). Blocking was done with 2% normal goat serum (Vector Laboratories, Cat# S-1000) in IMF buffer for 10 min at RT. Cells were washed thrice with IMF buffer for 10 min and probed with respective primary antibodies: anti-VE-Cadherin (1:500, Abcam, Cambridge, UK, Cat# ab33168) and anti-GFAP (1:500, Millipore, USA, Cat# 636562) for 1 h at RT. Cells were then washed with IMF buffer thrice for 15 min and incubated with suitable fluorophore tagged secondary antibodies (1: 2,000, Invitrogen, San Diego, CA, USA). Cells were washed thrice with IMF buffer for 15 min and stained with Hoechst 33342 (Invitrogen, San Diego, CA, USA, Cat# R37605) in 1X PBS for a minute and rinsed with 1X PBS. Membranes were mounted on glass slides using aqueous mounting media (Biomeda Corp., Foster City, CA, USA, Cat# M01) and covered with coverslips. Images were acquired with Nikon confocal microscope model A1 HD25 at 100X objective.
Transient expression of ZIKV E protein
Full length ZIKV E protein cloned in pCAGIG-IRES-GFP expression vector was a kind gift from Dr. Shyamala Mani (IISc, Bangalore, India) also used in previous studies (Bhagat et al., 2018, 2021). Cells (80% confluent) were transfected using lipofectamine 3000 (Invitrogen, San Diego, CA, USA, Cat# L3000008) according to the manufacturer’s protocol. Cells were harvested after 24 h transfection for further experiments (Supplementary Figure 4). Empty vector was used as control.
Transendothelial electrical resistance (TEER) assay
Endothelial resistance was measured to assess BBB integrity of BMECs in monoculture as well as in co-cultured PDA and BMECs on transwell inserts. TEER was measured using Millicell ERS-2 electrical resistance instrument (Millipore, USA). After 24 h, co-culture was established and measurements were done. Final calculations were done by multiplying TEER values with the area of insert membrane (1.1 cm2). TEER values of E protein transfected BBB culture were compared with monoculture/co-culture transfected with empty vector.
Transendothelial permeability assay
Progenitor derived astrocytes (PDA) and BMECs grown on PET transwell inserts were used to assess endothelial permeability. Dextran-FITC (M. W. 3000-5000) (Sigma, USA, Cat# FD4) was added to the upper compartment of insert at a final concentration of 50 μg/ml. The inserts were incubated at 37°C in dark for 30 min. Samples were then removed from the lower compartment for measuring fluorescence intensity using microplate fluorometer (Ex 480 nm and Em 530 nm, Tecan, USA).
Western blotting
Protein extract was isolated using sodium dodecyl sulphate (SDS) lysis buffer [50 mM Tris Buffer (pH 7.5), 150 mM sodium chloride, 50 mM sodium fluoride, 1 mM EDTA (pH 8.0), 2% SDS, 10 mM sodium borate, 1 mM sodium orthovanadate, protease inhibitor tablets (Roche, Basel, Switzerland, Cat# 11836170001)]. Protein concentration was estimated using 4% copper sulfate and bicinchoninic acid (Sigma, USA, Cat#B9643). Protein samples were separated by 8–12% SDS-PAGE. Proteins were then transferred onto nitrocellulose membrane (MDI, India), followed by 2 h blocking with 5% skimmed milk at room temperature (RT). The blots were incubated with respective primary antibodies: anti-ZIKV-E protein (1:2000, GeneTex, Cat# GTX133314), anti-ZO-1 (1:2,000, Invitrogen, Cat# 61-7300), anti-VE-Cadherin (1:2,000, Abcam, Cat# ab33168), anti-Occludin (1:500, Invitrogen, Cat# OC-3F10), anti-GFAP (1:60,000, Dako, USA, Cat# Z0334), anti-Vimentin (1:10,000, Santa Cruz Biotechnology, USA, Cat# sc-6260), and anti-β-actin (1:40,000, Sigma Cat# A3854), overnight at 4°C. The blots were washed with 1X TBST thrice for 5 min and then incubated with HRP- conjugated secondary antibodies (1:4,000, Vector Labs, Burlingame, CA, USA) for 1–2 h at RT. The blots were then washed using 1X TBST five times for 5 min. Protein was detected using chemiluminescence reagent (Millipore, Bedford, MA, USA, Cat# WBKLS0500) and imaged using Nine Alliance mini-HD UVITEC (Cambridge, UK). Protein bands so obtained were densitometrically quantified using ImageJ software (NIH, USA).
Quantitative real-time PCR
Total RNA was extracted from transfected samples using TRIZOL (Ambion, TX, USA Cat# 15596018) according to the manufacturer’s protocol. cDNA was synthesized from RNA using high-capacity cDNA reverse transcription kit (Applied Biosystems, Austin, TX, USA, Cat# 4368814) as per the manufacturer’s instructions. RT-PCR was performed using SYBR Green master mix (Applied Biosystems, USA, Cat# 4367659) using specific primers for IL-6, IL-8, IL-1β, CCL2, CCL5, CXCL10, ICAM-1, VCAM-1, PTGS2, GFAP, Vimentin, and GAPDH, as mentioned in the Supplementary Table 1. The cycling conditions used were 95°C for 10 min (1 cycle), 95°C for 20 s, 58°C for 20 s, and 72°C for 30 s (40 cycles).
Cytokine bead array
Human brain microvascular endothelial cells (hBMECs) and astrocytes were transfected with ZIKV E-protein and respective empty vector (control) for 24 h, followed by collection of supernatants. To determine cytokine and chemokine levels, supernatant was incubated with cytokine beads (CBA; Multiplex magnetic bead-based antibody detection kits, BD Biosciences, CA, USA, Cat# 551811) as per the manufacturer’s protocol. Incubated complex was passed by flow cytometer, and represented data was analyzed using the BD FACSDiva software.
Monocyte chemoattractant protein-1 (MCP-1)/CCL2 ELISA
Cells were transfected with ZIKV E protein and empty vector, as control, for 24 h. Supernatant was collected to determine the levels of MCP-1/CCL2 using commercial kit (BD Biosciences, CA, USA, Cat# 555179) as per manufacturer’s protocol.
Extracellular glutamate release
Astrocytes were transfected with ZIKV E-protein and respective empty vector (control) for 24 h, followed by collection of supernatants. It was further processed for the detection of glutamate release using glutamate determination kit (Sigma Aldrich, USA, Cat# GLN1) as per manufacturer’s protocol.
Statistical analysis
Experimental results are represented as mean values ± standard error of the mean. Each experiment was performed at least three times to determine the significance of the means. Comparison between experimental and control group was analyzed using Student’s t-test. A level of p < 0.05 was considered statistically significant.
Results
ZIKV E protein results in modulation in BBB integrity and permeability
Human brain microvascular cells (hBMECs) have been reported to show productive ZIKV infection (Papa et al., 2017; Mutso et al., 2020; Zoladek et al., 2021). ZIKV infects and activates hBMECs, however, the effects of its surface protein- envelope are not explored. In the present study, we examined the effect of ZIKV E protein on human brain microvascular endothelial cells (hBMECs) and astrocytes, and their resulting effect on BBB integrity. hBMECs were subjected to 24 h transfection with ZIKV E protein and compared with cells transfected with empty vector (Supplementary Figure 4). To investigate the effect of ZIKV E -protein on the integrity of endothelial barrier, quantitative measurement of TEER was done in hBMECs monoculture (Figure 1A). E-protein resulted in significant effect on the integrity of BBB in monoculture of hBMECs, as shown by decreased TEER values (from 151.5 to 135.6 Ω.cm2 ± 2.082, p < 0.001) when compared with empty vector control (Figure 1B). We also assessed transendothelial permeability of BBB in monoculture toward dextran-FITC upon exposure to ZIKV E protein. Monoculture layer of BMECs showed small increased permeability to dextran-FITC (1.098 ± 0.016, p < 0.01) upon exposure to ZIKV E protein as compared to its control (Figure 1C). We further investigated the impact of astrocytes on the integrity of BBB in a contact-based co-culture (Figure 1D). Presence of astrocytes with hBMECs resulted in higher TEER values compared to monoculture, suggesting that contact-based BBB model gives greater stability. Albeit the transient expression of ZIKV E protein in hBMECs, decrease in TEER values (from 176.6 to 158.0 Ω.cm2 ± 3.602, p < 0.01) were observed in co-culture of BBB (Figure 1E). Co-culture model of BBB also exhibited increased permeability toward dextran-FITC (1.055 ± 0.006, p < 0.01) (Figure 1F). Permeability changes in BBB were more prominent in monoculture, as presence of astrocytes in the co-culture BBB model reflected greater barrier strength. These findings indicate perturbed BBB integrity as seen by decreased TEER values and increased permeability to dextran-FITC in both hBMECs monoculture and co-culture of hBMECs and astrocytes in response to E protein.
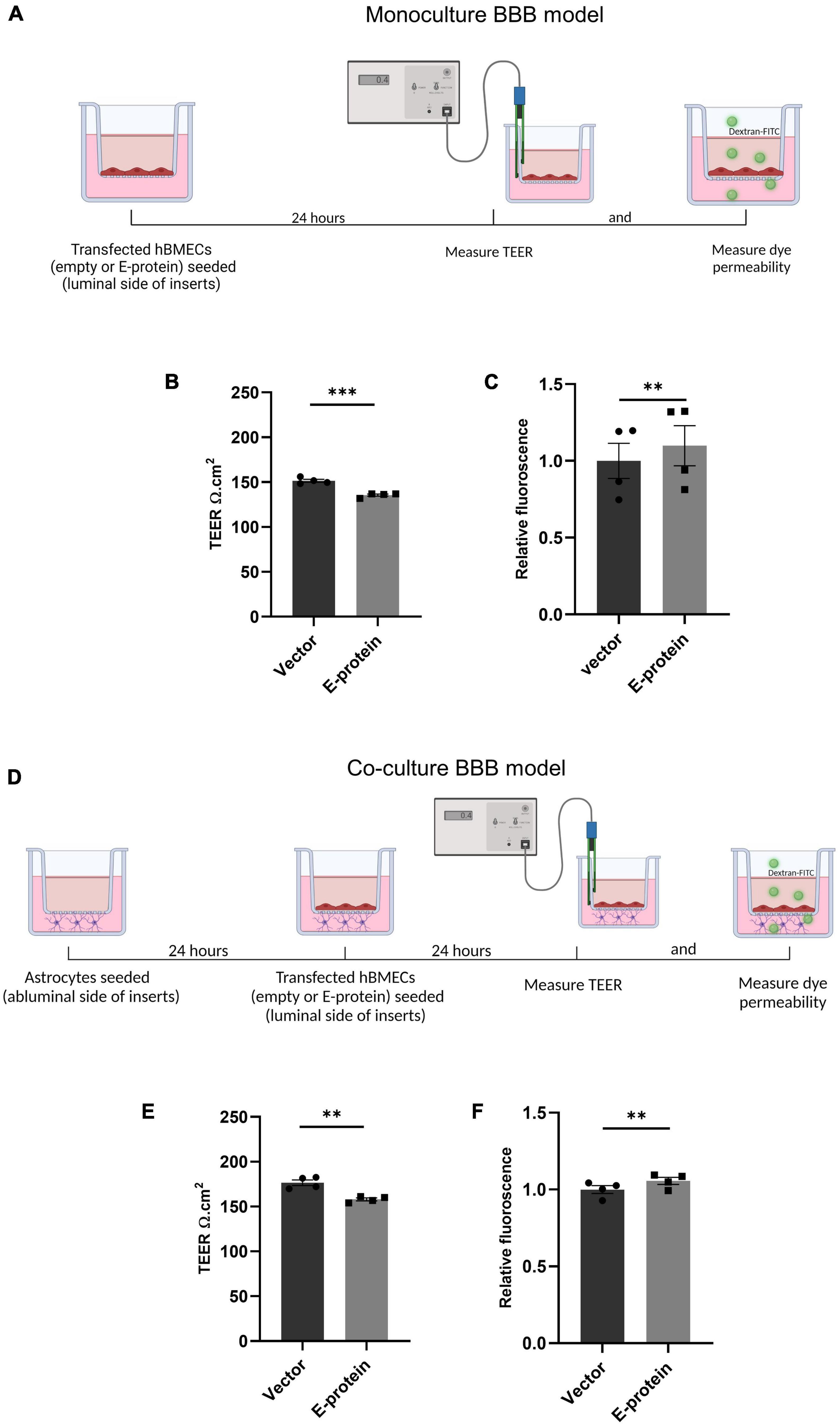
Figure 1. Effect of ZIKV E protein on BBB integrity. Schematic representation of monoculture BBB model establishment using hBMECs (A). hBMECs were transfected with empty vector or ZIKV E-protein. TEER was measured after 24 h. Bar graph shows TEER values given in ohm.cm2 (B). Dextran-FITC transendothelial permeability was assessed after 24 h of transfection. Bar graph shows relative fluorescence change (C). Schematic representation of coculture BBB model establishment using hBMECs and astrocytes (D). hBMECs transfected with empty vector or ZIKV E protein were grown in contact with astrocytes for 24 h followed by measurement of TEER values (E) and dextran-FITC transendothelial permeability (F). Data represents mean ± SEM for at least three independent experiments. **p < 0.01, ***p < 0.001 with respect to control.
ZIKV E protein dysregulate expression of tight junction and adherens junction proteins in hBMECs
To investigate the molecular drivers of disrupted BBB induced by ZIKV E protein, we investigated the effect of ZIKV E protein on expression of tight junction and adherens junction proteins which are crucial in establishment and maintenance of BBB integrity. hBMECs were transfected using ZIKV E protein expression vectors for 24 h and then harvested for further experimentation. We studied the expression of endothelial junction proteins, VE-Cadherin, ZO-1 and Occludin by Western blotting. Western blotting results showed reduced levels of endothelial ZO-1 (0.724 ± 0.080, p < 0.05), VE-Cadherin (0.630 ± 0.081, p < 0.01), and Occludin (0.806 ± 0.075, p < 0.05) proteins in ZIKV E-transfected hBMECs as compared to control cells which were transfected with empty vector (Figures 2A–D). We further investigated the effect of E protein on localization of endothelial junction proteins. We performed immunofluorescence analysis and observed radical changes in expression and perturbed localization of ZO-1 (0.859 ± 0.035, p < 0.05), VE-Cadherin (0.594 ± 0.066, p < 0.01), and Occludin (0.551 ± 0.145, p < 0.05) at the cell membrane in E-protein transfected cells compared to control (Figures 2E–M). These findings suggest that ZIKV E protein modulates the properties of hBMECs by altering expression and localization of endothelial junction proteins.
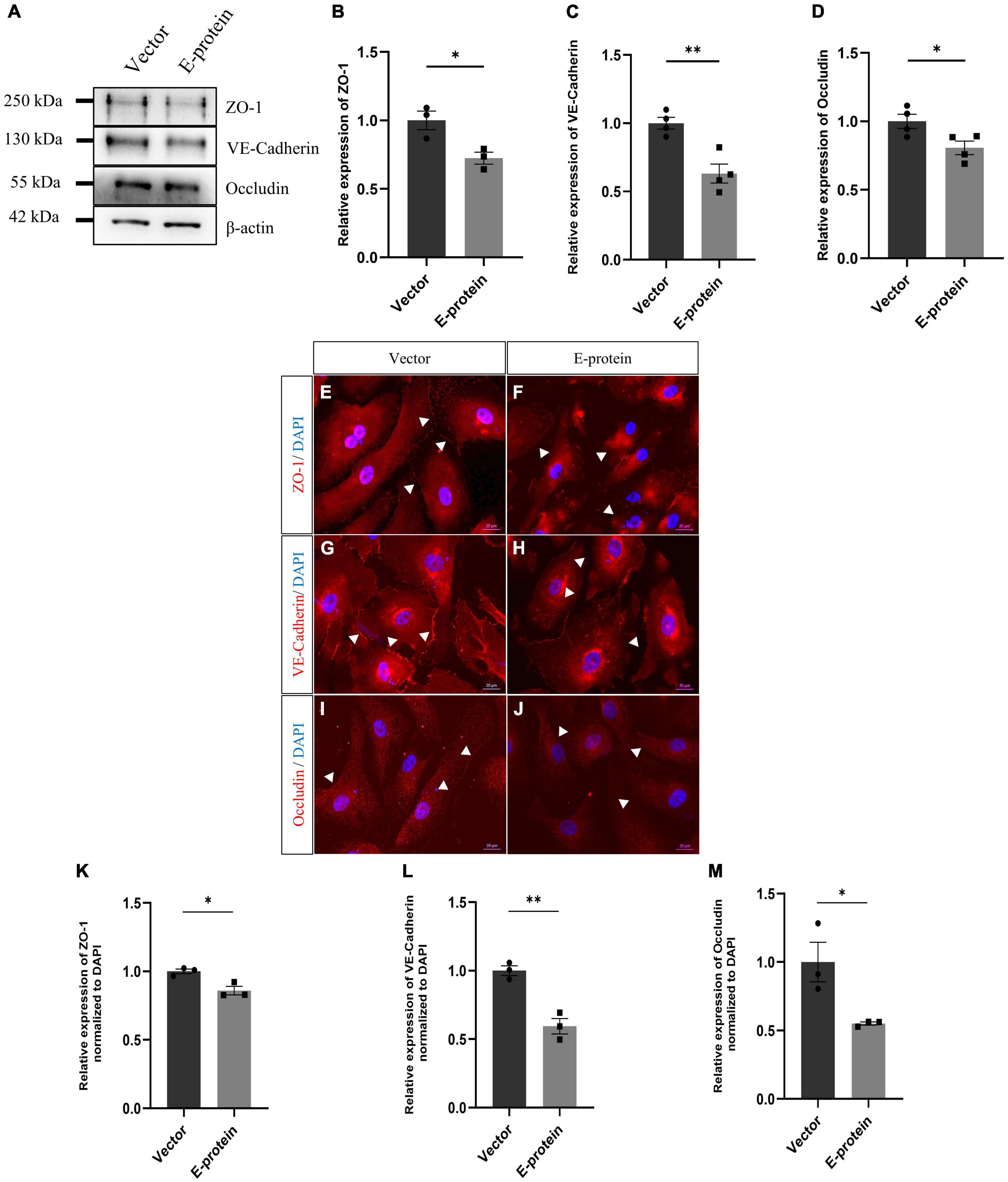
Figure 2. Effect of ZIKV E protein on endothelial junction protein expression. hBMECs were transfected with ZIKV E protein and empty vector as control for 24 h. (A) Total protein was isolated and subjected to Western blotting against ZO-1 (B), VE-Cadherin (C), Occludin (D) antibodies. β-actin was used as loading control. One representative blot is shown. Bar graphs shows relative protein expression in indicated groups. To assess localization of junction proteins, transfected hBMECs were immunostained with ZO-1 (E,F), VE-Cadherin (G,H) and Occludin (I,J). Scale bar 20 μm. Bar graph shows relative protein expression in indicated groups (K–M). Data represents mean ± SEM for at least three independent experiments. *p < 0.05, **p < 0.01 with respect to control.
ZIKV E protein induces inflammation in hBMECs
Blood-brain barrier (BBB) disruption enables inflammatory response at the injury site enabling the recruitment of immune cells, thereby, exacerbating the pro-inflammatory scenario and resulting in local inflammation (Clé et al., 2020; Huang et al., 2020). We investigated effect of E protein on the endothelial homeostasis, by studying the expression of IL-6, IL-8, IL-1β, CCL2, CCL5, CXCL10, ICAM-1, VCAM-1, and PTGS2 genes in E protein and vector control transfected cells. Upregulation of genes involved in inflammation such as IL-6 (1.393 ± 0.098, p < 0.05), IL-8 (1.307 ± 0.083, p < 0.05), IL-1β (1.868 ± 0.187, p < 0.01), CCL2 (1.937 ± 0.196, p < 0.01), CCL5 (1.207 ± 0.079, p < 0.05), and CXCL10 (1.504 ± 0.113, p < 0.01) was observed (Figures 3A–F). Interestingly, genes involved in immune cell recruitment, cell adhesion molecules (CAM) ICAM-1 (1.762 ± 0.254, p < 0.05), VCAM-1 (2.067 ± 0.197, p < 0.01), and angiogenesis, PTGS2 (1.720 ± 0.133, p < 0.001) were also significantly upregulated (Figures 3G–I). In addition to this, we examined the secretion of key proinflammatory cytokines IL-6 and IL-8 in the extracellular milieu of hBMECs transfected with E -protein. Increased secretion of IL-6 (1.417 ± 0.102, p < 0.01) and IL-8 (1.065 ± 0.019, p < 0.01) was observed as compared to control cells (Figures 4A–C). However, there was no difference in levels of IL-1β, IL-12p70 and TNF-α (data not shown). Our findings show perturbed endothelial homeostasis in response to E protein which can further modulate the expression and localization of tight junction and adherens junction proteins.
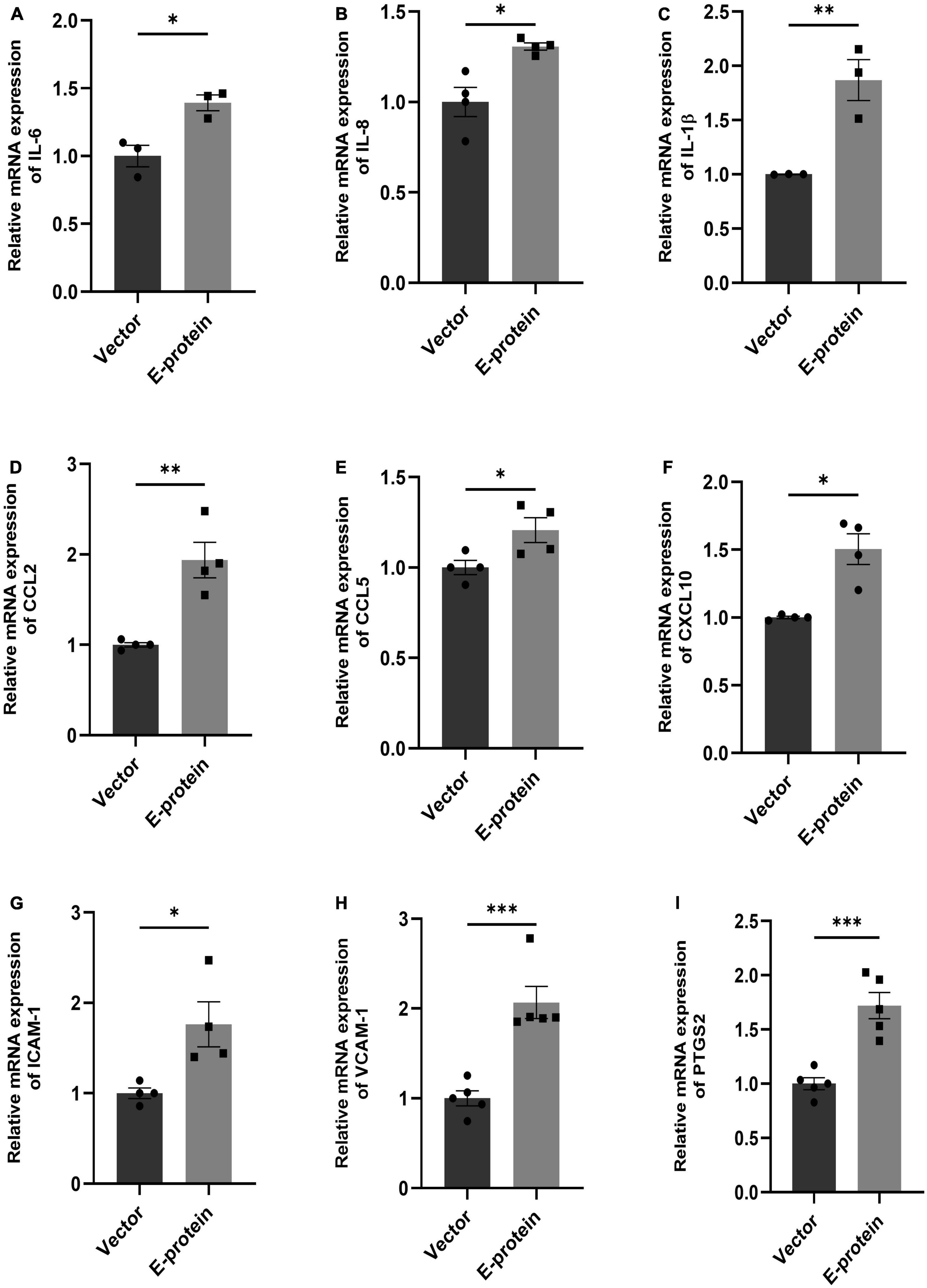
Figure 3. ZIKV E protein induces inflammation in hBMECs. Brain microvascular endothelial cells were transfected with empty vector and E protein for 24 h. Total RNA was isolated and transcript levels of inflammatory cytokines and chemokines were measured using quantitative RT-PCR for IL-6 (A), IL-8 (B), IL-1β (C), CCL2 (D), CCL5 (E) and CXCL10 (F). Transcript levels of genes involved in modulation of adhesion molecules and angiogenesis- ICAM-1 (G), VCAM-1 (H), and PTGS2 (I) were also measured. Bar graph shows relative transcript expression in indicated groups. Data represents mean ± SEM for at least three independent experiments. *p < 0.05, **p < 0.01, ***p < 0.001 with respect to control.
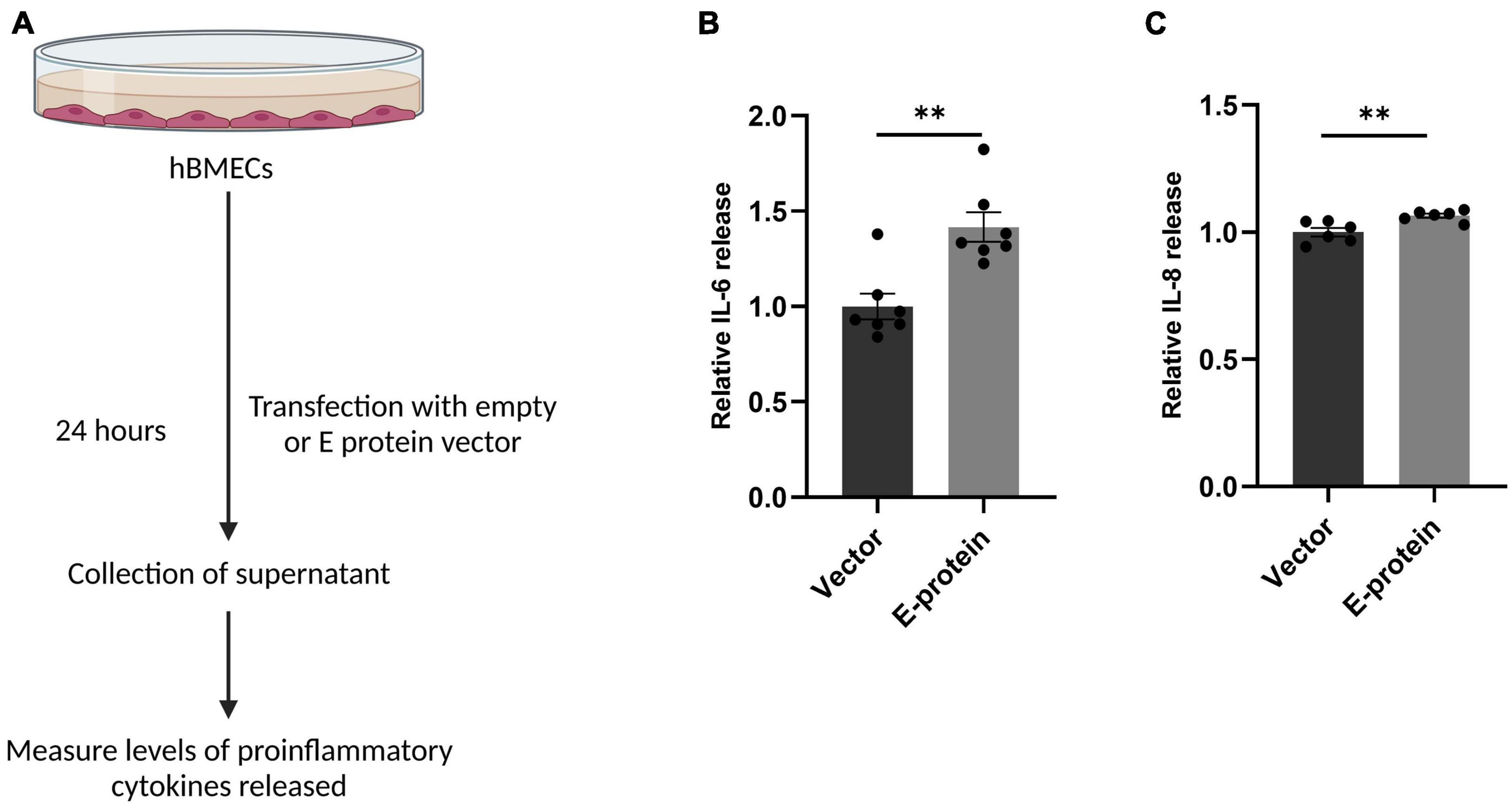
Figure 4. ZIKV E protein induces release of proinflammatory cytokines in hBMECs. Schematic representation of experimental setup (A). Brain microvascular endothelial cells were transfected with empty vector or E protein for 24 h. Supernatants collected from transfected cells were subjected to cytokine bead array for measurement of inflammatory cytokines IL-6 (B) and IL-8 (C). Data represents mean ± SEM for at least three independent experiments. **p < 0.01 with respect to control.
ZIKV E protein triggers inflammatory response in astrocytes
Astrocytes are the major brain cell population and are known to be highly permissible toward ZIKV infection. Because of their involvement in BBB formation and its maintenance, we investigated how ZIKV E protein impacts astrocytic activity. In response to CNS infections, astrocytes undergo morphological and functional changes pertaining to process known as “astrogliosis” or “reactive gliosis” (Escartin et al., 2021). Reactive astrocytes are characterized by increased levels of glial fibrillary acidic protein (GFAP) and Vimentin (Pekny et al., 2015). Dysregulated release of various soluble factor by reactive astrocytes also corroborates toward neuropathologies (Dong and Benveniste, 2001). We assessed the reactive state of astrocytes transfected with E protein and empty vector as control, for 24 h. A remarkable upregulation of GFAP (2.252 ± 0.432, p < 0.05) and Vimentin (1.990 ± 0.249, p < 0.05) was revealed in Western blotting (Figures 5A–D). Similar changes were also observed at the mRNA levels of GFAP (1.313 ± 0.135, p < 0.05) (Figure 5E) and Vimentin (1.919 ± 0.169, p < 0.01) (Figure 5F).
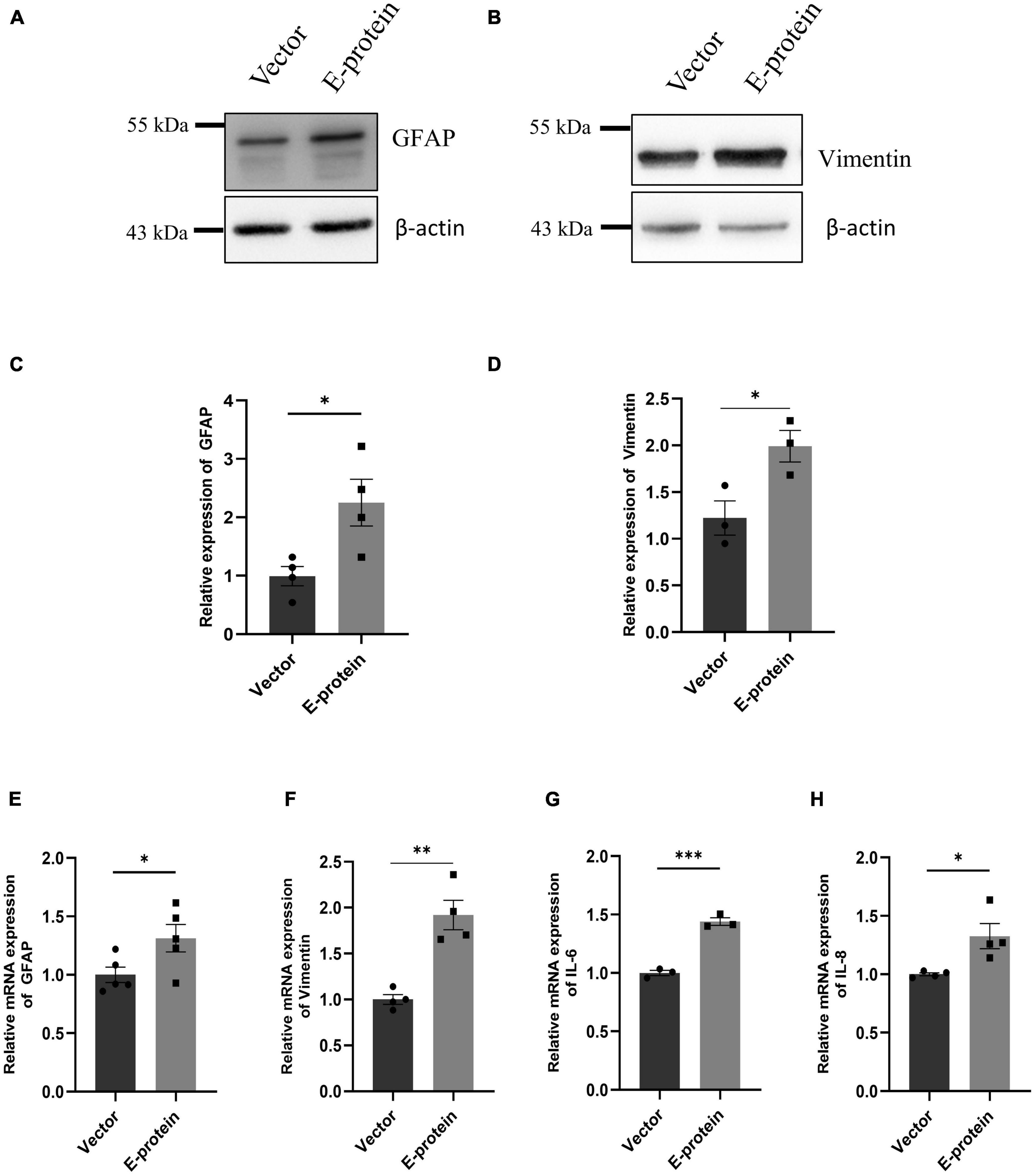
Figure 5. ZIKV E protein triggers astrogliosis. Progenitor derived astrocytes were transfected with empty vector or E protein for 24 h. Total protein was isolated and subjected to Western blotting against markers for astrocyte reactivity, GFAP (A) and Vimentin (B). β-actin was used as loading control. One representative blot is shown. Bar graph shows relative protein expression in indicated groups (C,D). To assess the transcript levels, total RNA was isolated and subjected to quantitative RT- PCR for GFAP (E), Vimentin (F), IL-6 (G), and IL-8 (H). Data represents mean ± SEM for at least three independent experiments. *p < 0.05, **p < 0.01, ***p < 0.001 with respect to control.
We further investigated the inflammatory response of astrocytes toward ZIKV E protein expression. RT-qPCR showed elevated levels of IL-6 (1.439 ± 0.039, p < 0.001) and IL-8 (1.326 ± 0.108, p < 0.05) after 24 h of ZIKV E protein expression (Figures 5G, H). In addition, we also examined the secretion of proinflammatory cytokines in the extracellular milieu of astrocytes transfected with E protein. Increased secretion of IL-6 (1.418 ± 0.065, p < 0.01) and IL-8 (1.038 ± 0.005, p < 0.05) was observed as compared to control cells (Figures 6A–C). These findings strongly suggests that astrocytes exacerbated the inflammation at the BBB site, in response to ZIKV E protein. Furthermore, astrocytes actively contribute in maintaining glutamate homeostasis in the brain which is perturbed in the reactive state of astrocytes, thereby pertaining to glutamate excitotoxicity (Mahmoud et al., 2019). Hence, we wanted to study secretion of glutamate from astrocytes after the ZIKV E protein transfection. E protein transfected astrocytes resulted in increased levels of glutamate in the extracellular milieu (1.337 ± 0.059, p < 0.01) (Figures 6A, D). Monocyte chemoattractant protein 1 (MCP-1) is one of the most commonly expressed chemokine expressed during CNS inflammation and is critical for monocyte recruitment and migration, BBB alteration, hence propagating inflammation (Sawyer et al., 2014). We checked the levels of MCP-1 release in supernatant collected from transfected astrocytes. Results showed elevated levels of MCP-1 (2.002 ± 0.354, p < 0.05) (Figures 6A, E). These findings indicate astrocytes being essential site of ZIKV infection potentiates inflammation in its reactive state when exposed to ZIKV E protein. These results suggest that astrocytes play role in altering integrity of endothelial barrier.
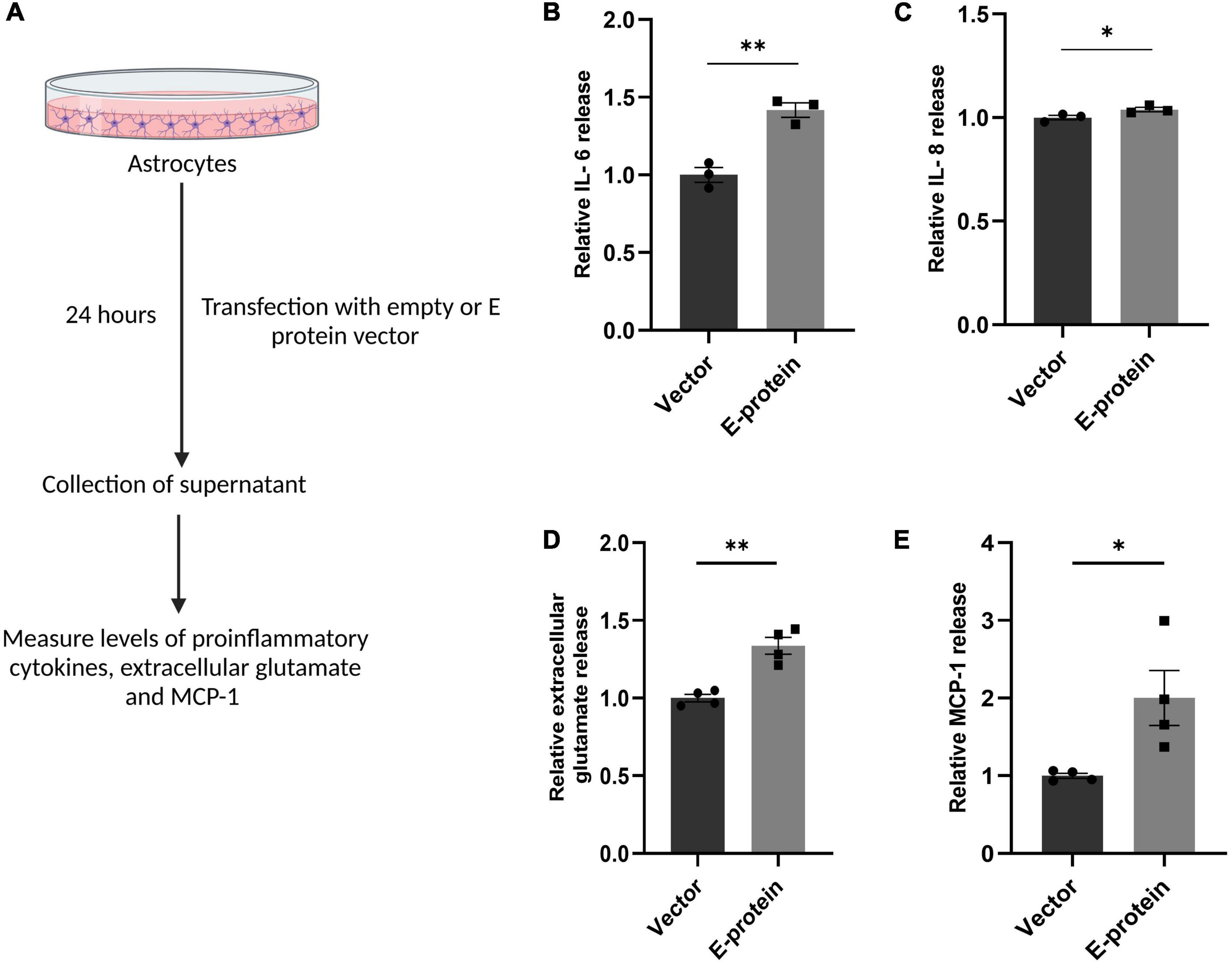
Figure 6. ZIKV E protein induced reactive astrocytes release proinflammatory cytokines, extracellular glutamate and MCP-1. Progenitor derived astrocytes were transfected with empty vector or E protein for 24 h. Schematic representation of experimental paradigm followed (A). Supernatant collected from transfected cells were subjected to cytokine bead array for measurement of inflammatory cytokines IL-6 (B) and IL-8 (C). Also, supernatant collected from transfected astrocytes was subjected to measurement of extracellular glutamate release (D) and also subjected to ELISA for measurement of MCP-1 (E). Data represents mean ± SEM for at least three independent experiments. *p < 0.05, **p < 0.01 with respect to control.
ZIKV induced reactive astrocytes alter expression of endothelial proteins
As astrocytes and BMECs mutually interact with each other via soluble factors and enhance barrier properties (Chang et al., 2015), we assessed whether E protein transfected “reactive astrocytes” influence the expression of endothelial junction proteins. To address this question, the supernatant from transfected astrocytes was collected after 24 h and mixed with equal proportion of fresh endothelial media. hBMECs were then treated with astrocyte-conditioned media (ACM)- empty vector and/or E- protein, respectively.
E protein exposure resulted in significant reduction in endothelial junction proteins- ZO-1 (0.641 ± 0.096, p < 0.01), VE-Cadherin (0.877 ± 0.196, p = 0.553), and Occludin (0.660 ± 0.075, p < 0.05) (Figures 7A–E). These results were further corroborated by our findings from contact-based co-culture model of BBB. Transfected hBMECs grown in direct contact with astrocytes were harvested after 24 h to check expression of endothelial junction proteins. Results showed prominent reduction in ZO-1 (0.554 ± 0.099, p < 0.01), VE-Cadherin (0.563 ± 0.102, p < 0.05), and Occludin (0.413 ± 0.262, p < 0.05) (Figures 8A–E). These results strongly emphasize how mutual interaction of astrocytes and BMECs play important role in maintaining BBB integrity, as both cell types were found to be vulnerable to ZIKV E protein exposure.
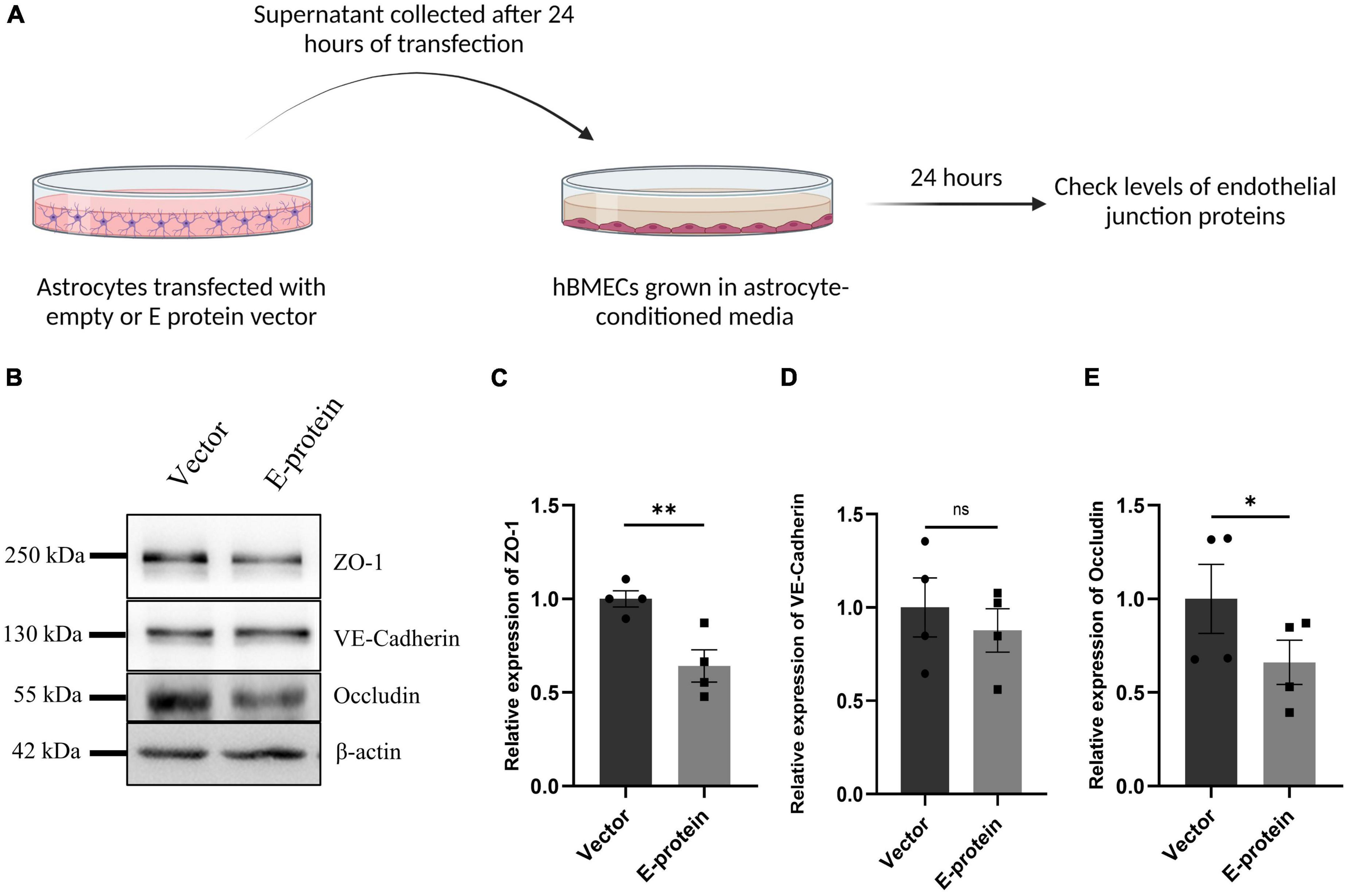
Figure 7. Reactive astrocytes affect endothelial junction proteins in hBMECs. Astrocytes were transfected with empty vector and E protein for 24 h. Schematic representation of experimental paradigm followed (A). Supernatants were collected and mixed with equal amount of fresh endothelial growth media. Brain microvascular endothelial cells were exposed to the astrocyte-conditioned media for 24 h. (B) Total protein was isolated and subjected to Western blotting against endothelial junction proteins- ZO-1 (C), VE-Cadherin (D), and Occludin (E). β-actin was used as loading control. One representative blot is shown. Bar graphs shows relative protein expression in indicated groups. Data represents mean ± SEM for at least three independent experiments. *p < 0.05, **p < 0.01, ns = not significant, with respect to control.
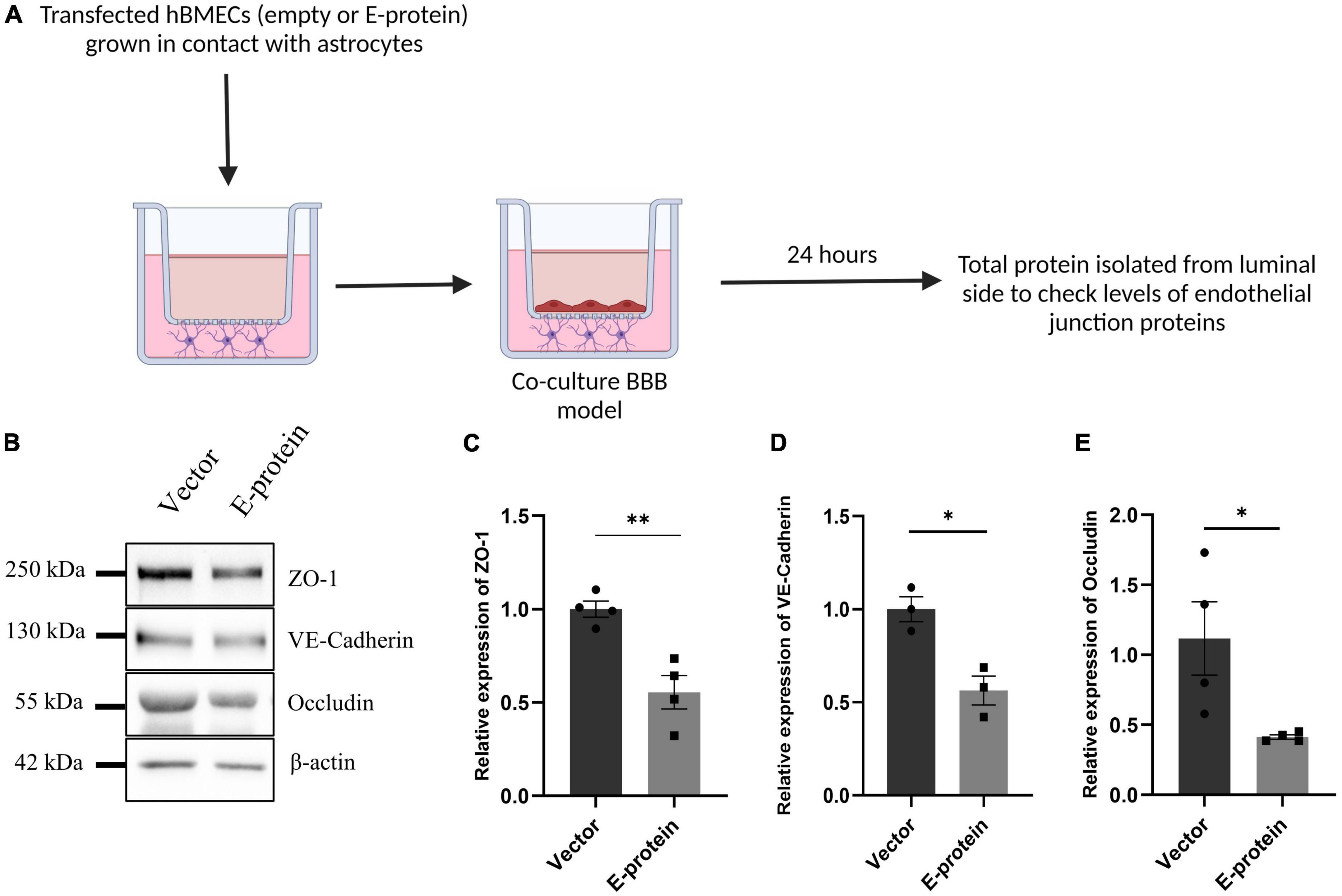
Figure 8. ZIKV E protein expression in hBMECs affects endothelial proteins in BBB of astrocytes and hBMECs. Transfected hBMECs were grown in contact with astrocytes for 24 h on transwell apparatus to form co-culture of BBB. Schematic representation of experimental paradigm followed (A). (B) Total protein was isolated from the luminal side of transwell and subjected to Western blotting against endothelial junction proteins- ZO-1 (C), VE-Cadherin (D), and Occludin (E). β-actin was used as loading control. One representative blot is shown. Bar graphs shows relative protein expression in indicated groups. Data represents mean ± SEM for at least three independent experiments. *p < 0.05, **p < 0.01 with respect to control.
Discussion
Our study aimed at the investigation of role of principal surface protein of Zika virus i.e., envelope (E) protein on the characteristic properties of cells comprising the BBB, and delineate the molecular mechanisms for the same. While the majority of work in the field has primarily focused on monoculture BBB model systems, we employed a two-dimensional model consisting of hBMECs and astrocytes to recapitulate the in vivo physiological conditions of BBB. This model allowed us to better mimic the complex interactions and dynamics between these cell types, providing a more comprehensive understanding of BBB. In our study we have employed two in vitro model systems of BBB- (1) monolayer of primary hBMECs cultured on transwell and (2) a contact-based co-culture of primary hBMECs and human neural stem cell derived astrocytes cultured on either side of transwell apparatus. Using both of our model systems we found that exposure of E protein to the BBB model resulted in significant modulation of BBB integrity and endothelial permeability. Although the changes in permeability of hBMECs were relatively small, these changes had notable effects on the activation state of hBMECs. In our study, we have employed dextran-FITC dye (4kDa) to assess the endothelial permeability, however, there are other low molecular weight dyes available which might provide additional insights into the permeability of small molecules across the BBB. Furthermore, previous studies have also shown that ZIKV does not induce significant disruptions on BBB integrity but rather affects activity and function of BMECs and astrocytes (Mladinich et al., 2017; Papa et al., 2017; Alimonti et al., 2018). These findings highlight the importance of investigating the cellular responses and interactions within the BBB during ZIKV infection, even in the absence of dramatic changes in barrier integrity. We further investigated the molecular drivers of BBB disruption induced by ZIKV E protein. We studied the effect on expression of tight and adherens junction proteins which are responsible for maintaining the junctional stability. Indeed, ZIKV E protein altered expression of endothelial junction proteins–ZO-1, Occludin and VE-Cadherin in both monoculture and co-culture model systems. Previous studies also showed a downregulation of tight junction protein expression in hBMECs infected with ZIKV Asian strain (PRVABC9, Puerto Rico), when compared to African strains (R103451 and MR 766) (Leda et al., 2019). Our study further fills the lacunae of other in vitro components of BBB model as seen by presence of astrocytes in contact with hBMECs. Our contact-based two-dimensional model system of human origin provides a more suitable and relevant in vitro platform for investigating the effects of ZIKV proteins, which has not been previously reported.
During viral invasion and BBB disruption BMECs function as source of pro-inflammatory chemokines and cytokines (Daniels and Klein, 2015). Hence, we tested the hypothesis if ZIKV E protein has any influence on BMECs activation. Our experiments describe activation of BMECs on E protein exposure, proinflammatory cytokines and chemokines (IL-6, IL-8, IL-1β, CCL2, CCL5, and CXCL10) which are known to alter BBB integrity upon CNS infection are increased (Daniels and Klein, 2015; Wolf et al., 2019; Alsaffar et al., 2020; Panganiban et al., 2020; Rodrigues de Sousa et al., 2021). Proinflammatory cytokines IL-6 and IL-8 were detected in the extracellular milieu of hBMECs transfected with E protein. Other cytokines were not present in detectable limits which could be attributed to their short half-lives and different time kinetics. Along with increase in inflammatory molecules, BMECs showed increased levels of other endothelial barrier disrupting molecules such as CAM, ICAM-1 and VCAM-1. Increased CAM levels are known to augment viral entry contributing in immune cell infiltration and elevate inflammatory response at the site of BBB (Al-Obaidi et al., 2018). Endothelium permeabilization is one mechanism by which neurotropic viruses access the CNS (Sips et al., 2012). Dysregulation of endothelial junction proteins, increased expression of cell adhesion molecules and inflammatory molecules can influence the viral access into the CNS without completely disrupting the BBB (Verma et al., 2009). These changes in molecular and cellular properties of BBB cells can create an environment that facilitates viral entry into the CNS while still maintaining some level of barrier function. Our findings are in agreement with the previous studies showing ZIKV persistence in hBMECs and potentially utilize the paracellular route to enter the privileged neuronal compartments (Mladinich et al., 2017). Our findings contribute to the growing body of evidence supporting the ability of ZIKV to establish CNS infection by exploiting the cellular mechanisms within the BBB.
Astrocytes are found to be major brain cell population susceptible to ZIKV infection during fetal brain development (Meertens et al., 2017). Their close contact with BMECs in maintaining the neurovasculature makes them eminent during viral infections (Zorec et al., 2019). Infected astrocytes are known to show cytopathogenic effects in response to ZIKV infection and act as reservoir for viral replication (Potokar et al., 2019). We investigated how astrocytes in BBB respond in presence of ZIKV E protein. Astrocytes transfected with E protein were found to be highly reactive as reflected by increase in GFAP and Vimentin expression. Astrocytes in their reactive state exhibited increased transcript levels of pro-inflammatory cytokines like IL-6 and IL-8 and extracellular release of IL-6 and IL-8. MCP-1 being an important chemokine in the inflammatory events in viral invasion was found to be increased when astrocytes were exposed to ZIKV E protein. MCP-1 is also known to regulate BBB permeability as brain endothelial cells express MCP-1 receptor, CCR2 (Stamatovic et al., 2003, 2005). Glutamate homeostasis was also perturbed resulting in increased levels of glutamate in the extracellular milieu of transfected astrocytes. These present findings indicate that activated astrocytes play crucial role in disruption of BBB integrity during the course of ZIKV infection. Furthermore, our co-culture BBB model system closely mimicked the physiological environment where both the cells were in mutual contact and their individual contributions to the BBB pathophysiology was studied in response to ZIKV E protein.
The attained neurotropic nature of ZIKV makes it capable to infect almost all types of brain cells−microglia, neural progenitor cells and astrocytes; resulting in severe consequences during fetal brain development (Bhagat et al., 2018, 2022; Quincozes-Santos et al., 2023). The associated neurological complications due to vertical transmission (from infected pregnant women to fetus) mainly involves miscarriages, impaired fetal growth or fetuses born with congenital Zika syndrome (CZS) (van der Linden et al., 2016). Studies have demonstrated incidence of ZIKV infection post-breaching the blood-placental barrier and blood-brain barrier (Chiu et al., 2020). Clinical studies indicated prevalence of cognitive abnormalities like seizure disorders and motor impairments in children born with CZS (Satterfield-Nash et al., 2017; van der Linden et al., 2018). As the membrane fusion and subsequent entry of the virus is facilitated by E protein, it proves to be a conducive target for drug therapy and vaccine development (Giraldo et al., 2020; Hu et al., 2021). Our previous lab studies have focused on how ZIKV E protein perturbed the miRNA circuitry of human fetal neural stem cells (fNSCs) pertaining to cell cycle arrest and inhibition of proliferation (Bhagat et al., 2018, 2021). This strongly highlights the fact that E protein plays eminent role in pathogenicity of the virus. In addition, endothelial dysfunction has been also reported due to ZIKV NS1 protein bystander effects. NS1 protein has been shown to destabilize VE-Cadherin complex and promoted disruption of claudin 5 (CLDN5) affecting the endothelial integrity (Rastogi and Singh, 2020; Bhardwaj and Singh, 2021). To further accentuate the findings resulting from single ZIKV structural protein, our study further requires the validation that can be performed in human ZIKV microcephalic brain tissues. Also, even though we tried to closely mimic the physiological environment by establishing a contact -based model of hBMECs and astrocytes, our present study will be benefitted from in vivo ZIKV animal models to better understand the corroborated effects of ZIKV E protein. Altogether, our study concludes that ZIKV E protein compromises the BBB maintenance. Our findings implicates that the envelope protein of ZIKV dysregulates the properties of hBMECs which may aid in release of viral particles into the parenchyma without causing complete disruption of BBB. The altered state of BBB results from the dysregulated state of endothelial junction proteins and activated state of BMECs, resulting in an inflammatory cascade. In addition to this, astrocytes were also found to be susceptible and reactive toward exposure of E protein, thereby influencing the BBB integrity suggesting a potential mechanism in ZIKV-mediated BBB dysregulation through direct contact with hBMECs.
Data availability statement
The raw data supporting the conclusions of this article will be made available by the authors, without undue reservation.
Ethics statement
The studies involving human participants were reviewed and approved by the Institutional Human Ethics Committee, National Brain Research Centre, Manesar, India. The patients/participants provided their written informed consent to participate in this study.
Author contributions
GK designed, performed experiments, analyzed, interpreted the data, and wrote the manuscript. PP performed the experiments. RB provided inputs and helped in writing the manuscript. PS designed the study and helped in analyzing data. All authors contributed to the article and approved the submitted version.
Acknowledgments
We greatly acknowledge the financial support from the core fund of NBRC, Manesar, India. We acknowledge the help of Ms. Vini Tiwari toward initial hBMECs culture work. The guidance of Mr. Hriday Shanker Pandey and Ms. Priyanka is greatly acknowledged. We thank Ms. Archana Mehta for her help in confocal imaging. ZIKV expression vectors were a kind gift from Dr. Shyamala Mani. Technical assistance from Mr. Naushad Alam, and Mr. Durga Lal Meena of NBRC, India is greatly appreciated. We also wish to acknowledge the support of the facilities provided under the Biotechnology Information System Network (BTISNET) grant, DBT India, and the Computing facility at NBRC, Manesar, India. Figure schematics were created with BioRender.com.
Conflict of interest
The authors declare that the research was conducted in the absence of any commercial or financial relationships that could be construed as a potential conflict of interest.
Publisher’s note
All claims expressed in this article are solely those of the authors and do not necessarily represent those of their affiliated organizations, or those of the publisher, the editors and the reviewers. Any product that may be evaluated in this article, or claim that may be made by its manufacturer, is not guaranteed or endorsed by the publisher.
Supplementary material
The Supplementary Material for this article can be found online at: https://www.frontiersin.org/articles/10.3389/fncel.2023.1173120/full#supplementary-material
References
Agrawal, T., Sharvani, V., Nair, D., and Medigeshi, G. (2013). Japanese encephalitis virus disrupts cell-cell junctions and affects the epithelial permeability barrier functions. PLoS One 8:e69465. doi: 10.1371/journal.pone.0069465
Alimonti, J., Ribecco-Lutkiewicz, M., Sodja, C., Jezierski, A., Stanimirovic, D., Liu, Q., et al. (2018). Zika virus crosses an in vitro human blood brain barrier model. Fluids Barriers CNS 15:15. doi: 10.1186/s12987-018-0100-y
Al-Obaidi, M., Bahadoran, A., Wang, S., Manikam, R., Raju, C., and Sekaran, S. (2018). Disruption of the blood brain barrier is vital property of neurotropic viral infection of the central nervous system. Acta Virol. 62, 16–27. doi: 10.4149/av_2018_102
Alsaffar, H., Martino, N., Garrett, J., and Adam, A. (2020). Interleukin-6 promotes a sustained loss of endothelial barrier function via Janus kinase-mediated STAT3 phosphorylation and de novo protein synthesis. Am. J. Physiol. Cell Physiol. 314, C589–C602. doi: 10.1152/ajpcell.00235.2017
Annamalai, A., Pattnaik, A., Sahoo, B., Muthukrishnan, E., Natarajan, S., Steffen, D., et al. (2017). Zika Virus Encoding Nonglycosylated Envelope Protein Is Attenuated and Defective in Neuroinvasion. J. Virol. 91, e1348–e1317. doi: 10.1128/JVI.01348-17
Bhagat, R., Prajapati, B., Narwal, S., Agnihotri, N., Adlakha, Y., Sen, J., et al. (2018). Zika virus E protein alters the properties of human fetal neural stem cells by modulating microRNA circuitry. Cell Death Differ. 25, 1837–1854. doi: 10.1038/s41418-018-0163-y
Bhagat, R., Rajpara, P., Kaur, G., Gupta, K., and Seth, P. (2021). Zika virus E protein dysregulate mir-204/WNT2 signalling in human fetal neural stem cells. Brain Res. Bull. 176, 93–102. doi: 10.1016/j.brainresbull.2021.08.009
Bhagat, R., Kaur, G., and Pankaj, S. (2022). Molecular Mechanisms of Zika Virus Pathogenesis: An Update. Indian J. Med. Res. 154, 433–45. doi: 10.4103/ijmr.IJMR_169_20
Bhardwaj, U., and Singh, S. (2021). Zika Virus NS1 Suppresses VE-Cadherin and Claudin-5 via hsa-miR-101-3p in Human Brain Microvascular Endothelial Cells. Mol. Neurobiol. 58, 6290–6303. doi: 10.1007/s12035-021-02548-x
Carbaugh, D., Baric, R., and Lazear, H. (2019). Envelope Protein Glycosylation Mediates Zika Virus Pathogenesis. J. Virol. 93, e113–e119. doi: 10.1128/JVI.00113-19
Carod-Artal, F. (2018). Neurological complications of Zika virus infection. Expert. Rev. Anti. Infect. Ther. 16, 399–410. doi: 10.1080/14787210.2018.1466702
Chang, C., Li, J., Chen, W., Ou, Y., Lai, C., Hu, Y., et al. (2015). Disruption of in vitro endothelial barrier integrity by Japanese encephalitis virus-Infected astrocytes. Glia 63, 1915–1932. doi: 10.1002/glia.22857
Chaturvedi, U., Dhawan, R., Khanna, M., and Mathur, A. (1991). Breakdown of the blood-brain barrier during dengue virus infection of mice. J. Gen. Virol. 72, 859–866. doi: 10.1099/0022-1317-72-4-859
Chimelli, L., Melo, A., Avvad-Portari, E., Wiley, C., Camacho, A., Lopes, V., et al. (2017). The spectrum of neuropathological changes associated with congenital Zika virus infection. Acta Neuropathol. 133, 983–999. doi: 10.1007/s00401-017-1699-5
Chiu, C., Chu, L., Liao, I., Simanjuntak, Y., Lin, Y., Juan, C., et al. (2020). The mechanism of the zika virus crossing the placental barrier and the blood-brain barrier. Front. Microbiol. 11:214. doi: 10.3389/fmicb.2020.00214
Clé, M., Desmetz, C., Barthelemy, J., Martin, M., Constant, O., Maarifi, G., et al. (2020). Zika Virus Infection Promotes Local Inflammation, Cell Adhesion Molecule Upregulation, and Leukocyte Recruitment at the Blood-Brain Barrier. mBio 11, e01183-20. doi: 10.1128/mBio.01183-20
Cordeiro, C., Bano, R., Washington Cross, C., and Segars, J. (2017). Zika virus and assisted reproduction. Curr. Opin. Obstet. Gynecol. 29, 175–179. doi: 10.1097/GCO.0000000000000366
Daniels, B., and Klein, R. (2015). Viral sensing at the blood-brain barrier: new roles for innate immunity at the CNS vasculature. Clin. Pharmacol. Ther. 97, 372–379. doi: 10.1002/cpt.75
de Almeida Oliveira Evangelista, G., Hughes Carvalho, R., Sant’Ana Menezes, G., Carvalho de Abreu, Y., Sardi, S., and Soares Campos, G. (2021). Meningoencephalitis Associated with Zika Virus and Chikungunya Virus Infection. Jpn. J. Infect. Dis. 74, 584–586. doi: 10.7883/yoken.JJID.2020.1000
Dong, Y., and Benveniste, E. (2001). Immune function of astrocytes. Glia 36, 180–190. doi: 10.1002/glia.1107
Escartin, C., Galea, E., Lakatos, A., O’Callaghan, J., Petzold, G., Serrano-Pozo, A., et al. (2021). Reactive astrocyte nomenclature, definitions, and future directions. Nat. Neurosci. 24, 312–325. doi: 10.1038/s41593-020-00783-4
Faye, O., Freire, C., Iamarino, A., Faye, O., de Oliveira, J., Diallo, M., et al. (2014). Molecular evolution of Zika virus during its emergence in the 20(th) century. PLoS Negl. Trop. Dis. 8:e2636. doi: 10.1371/journal.pntd.0002636
Giraldo, M., Xia, H., Aguilera-Aguirre, L., Hage, A., van Tol, S., Shan, C., et al. (2020). Envelope protein ubiquitination drives entry and pathogenesis of Zika virus. Nature 585, 414–419. doi: 10.1038/s41586-020-2457-8
Helms, H., Abbott, N., Burek, M., Cecchelli, R., Couraud, P., Deli, M., et al. (2015). In vitro models of the blood-brain barrier: An overview of commonly used brain endothelial cell culture models and guidelines for their use. J. Cereb. Blood Flow Metab. 36, 862–890. doi: 10.1177/0271678X16630991
Hu, T., Wu, Z., Wu, S., Chen, S., and Cheng, A. (2021). The key amino acids of E protein involved in early flavivirus infection: viral entry. Virol. J. 18, 136. doi: 10.1186/s12985-021-01611-2
Huang, Y., Chen, S., Luo, Y., and Han, Z. (2020). Crosstalk between Inflammation and the BBB in Stroke. Curr. Neuropharmacol. 18, 1227–1236. doi: 10.2174/1570159X18666200620230321
Joo, F. (2002). Endothelial Cells of the Brain and Other Organ Systems: Some Similarities and Differences. Prog. Neurobiol. 48, 255–273. doi: 10.1016/0301-0082(95)00046-1
Kuno, G., and Chang, G. (2007). Full-length sequencing and genomic characterization of Bagaza. Kedougou, and Zika viruses. Arch. Virol. 152, 687–696. doi: 10.1007/s00705-006-0903-z
Leda, A., Bertrand, L., Andras, I., El-Hage, N., Nair, M., and Toborek, M. (2019). Selective Disruption of the Blood-Brain Barrier by Zika Virus. Front. Microbiol. 10:2158. doi: 10.3389/fmicb.2019.02158
Luissint, A., Artus, C., Glacial, F., Ganeshamoorthy, K., and Couraud, P. (2012). Tight junctions at the blood brain barrier: physiological architecture and disease-associated dysregulation. Fluids Barriers CNS 9:23. doi: 10.1186/2045-8118-9-23
Mahmoud, S., Gharagozloo, M., Simard, C., and Gris, D. (2019). Astrocytes Maintain Glutamate Homeostasis in the CNS by Controlling the Balance between Glutamate Uptake and Release. Cells 8, 184. doi: 10.3390/cells8020184
Meertens, L., Labeau, A., Dejarnac, O., Cipriani, S., Sinigaglia, L., Bonnet-Madin, L., et al. (2017). Axl Mediates ZIKA Virus Entry in Human Glial Cells and Modulates Innate Immune Responses. Cell Rep. 18, 324–333. doi: 10.1016/j.celrep.2016.12.045
Miner, J., and Diamond, M. (2017). Zika Virus Pathogenesis and Tissue Tropism. Cell Host. Microbe 21, 134–142. doi: 10.1016/j.chom.2017.01.004
Mladinich, M., Schwedes, J., and Mackow, E. (2017). Zika Virus Persistently Infects and Is Basolaterally Released from Primary Human Brain Microvascular Endothelial Cells. mBio 8, e952–e917. doi: 10.1128/mBio.00952-17
Muñoz, L., Barreras, P., and Pardo, C. (2016). Zika Virus-Associated Neurological Disease in the Adult: Guillain-Barré Syndrome. Encephalitis, and Myelitis. Semin. Reprod. Med. 34, 273–279. doi: 10.1055/s-0036-1592066
Muñoz, L., Parra, B., and Pardo, C. (2017). Neurological Implications of Zika Virus Infection in Adults. J. Infect. Dis. 216, S897–S905. doi: 10.1093/infdis/jix511
Mutso, M., St John, J., Ling, Z., Burt, F., Poo, Y., Liu, X., et al. (2020). Basic insights into Zika virus infection of neuroglial and brain endothelial cells. J. Gen. Virol. 101, 622–634. doi: 10.1099/jgv.0.001416
Naik, P., and Cucullo, L. (2012). In vitro blood-brain barrier models: current and perspective technologies. J. Pharm. Sci. 101, 1337–1354. doi: 10.1002/jps.23022
Panganiban, A., Blair, R., Hattler, J., Bohannon, D., Bonaldo, M., Schouest, B., et al. (2020). A Zika virus primary isolate induces neuroinflammation, compromises the blood-brain barrier and upregulates CXCL12 in adult macaques. Brain Pathol. 30, 1017–1027. doi: 10.1111/bpa.12873
Papa, M., Meuren, L., Coelho, S., Lucas, C., Mustafá, Y., Lemos Matassoli, F., et al. (2017). Zika Virus Infects, Activates, and Crosses Brain Microvascular Endothelial Cells, without Barrier Disruption. Front. Microbiol. 8:2557. doi: 10.3389/fmicb.2017.02557
Pekny, M., Pekna, M., Messing, A., Steinhäuser, C., Lee, J., Parpura, V., et al. (2015). Astrocytes: a central element in neurological diseases. Acta Neuropathol. 131, 323–345. doi: 10.1007/s00401-015-1513-1
Potokar, M., Jorgačevski, J., and Zorec, R. (2019). Astrocytes in Flavivirus Infections. Int. J. Mol. Sci. 20, 691. doi: 10.3390/ijms20030691
Quincozes-Santos, A., Bobermin, L., Costa, N., Thomaz, N., Almeida, R., Beys-da-Silva, W., et al. (2023). The role of glial cells in Zika virus-induced neurodegeneration. Glia 71, 1791–1803. doi: 10.1002/glia.24353
Rastogi, M., and Singh, S. (2020). Zika virus NS1 affects the junctional integrity of human brain microvascular endothelial cells. Biochimie 176, 52–61. doi: 10.1016/j.biochi.2020.06.011
Rodrigues de Sousa, J., Azevedo, R., Quaresma, J., and Vasconcelos, P. (2021). The innate immune response in Zika virus infection. Rev. Med. Virol. 31, e2166. doi: 10.1002/rmv.2166
Roe, K., Kumar, M., Lum, S., Orillo, B., Nerurkar, V., and Verma, S. (2012). West Nile virus-induced disruption of the blood-brain barrier in mice is characterized by the degradation of the junctional complex proteins and increase in multiple matrix metalloproteinases. J. Gen. Virol. 93(Pt 6), 1193–1203. doi: 10.1099/vir.0.040899-0
Satterfield-Nash, A., Kotzky, K., Allen, J., Bertolli, J., Moore, C., Pereira, I., et al. (2017). Health and Development at Age 19-24 Months of 19 Children Who Were Born with Microcephaly and Laboratory Evidence of Congenital Zika Virus Infection During the 2015 Zika Virus Outbreak - Brazil, 2017. MMWR Morb. Mortal Wkly. Rep. 66, 1347–1351. doi: 10.15585/mmwr.mm6649a2
Sawyer, A., Tian, W., Saucier-Sawyer, J., Rizk, P., Saltzman, W., Bellamkonda, R., et al. (2014). The effect of inflammatory cell-derived MCP-1 loss on neuronal survival during chronic neuroinflammation. Biomaterials 35, 6698–6706. doi: 10.1016/j.biomaterials.2014.05.008
Sips, G., Wilschut, J., and Smit, J. (2012). Neuroinvasive flavivirus infections. Rev. Med. Virol. 22, 69–87. doi: 10.1002/rmv.712
Stamatovic, S., Keep, R., Kunkel, S., and Andjelkovic, A. (2003). Potential role of MCP-1 in endothelial cell tight junction ‘opening’: signaling via Rho and Rho kinase. J. Cell Sci. 116, 4615–4628. doi: 10.1242/jcs.00755
Stamatovic, S., Shakui, P., Keep, R., Moore, B., Kunkel, S., Van Rooijen, N., et al. (2005). Monocyte chemoattractant protein-1 regulation of blood-brain barrier permeability. J. Cereb. Blood Flow Metab. 25, 593–606. doi: 10.1038/sj.jcbfm.9600055
van der Linden, H., Carvalho, M., van der Linden, V., Lacerda, K., Pessoa, A., Carneiro, M., et al. (2018). Epilepsy Profile in Infants with Congenital Zika Virus Infection. N. Engl. J. Med. 379, 891–892. doi: 10.1056/NEJMc1716070
van der Linden, V., Pessoa, A., Dobyns, W., Barkovich, A., Júnior, H., Filho, E., et al. (2016). Description of 13 Infants Born During October 2015-January 2016 With Congenital Zika Virus Infection Without Microcephaly at Birth - Brazil. MMWR Morb. Mortal Wkly. Rep. 65, 1343–1348. doi: 10.15585/mmwr.mm6547e2
Verma, S., Lo, Y., Chapagain, M., Lum, S., Kumar, M., Gurjav, U., et al. (2009). West Nile virus infection modulates human brain microvascular endothelial cells tight junction proteins and cell adhesion molecules: Transmigration across the in vitro blood-brain barrier. Virology 385, 425–433. doi: 10.1016/j.virol.2008.11.047
Wolf, S., Taylor, A., Zaid, A., Freitas, J., Herrero, L., Rao, S., et al. (2019). Inhibition of Interleukin-1β Signaling by Anakinra Demonstrates a Critical Role of Bone Loss in Experimental Arthritogenic Alphavirus Infections. Arthritis. Rheumatol. 71, 1185–1190. doi: 10.1002/art.40856
Zoladek, J., Legros, V., Jeannin, P., Chazal, M., Pardigon, N., Ceccaldi, P., et al. (2021). Zika Virus Requires the Expression of Claudin-7 for Optimal Replication in Human Endothelial Cells. Front. Microbiol. 12:746589. doi: 10.3389/fmicb.2021.746589
Keywords: Zika virus, E protein, blood-brain barrier, tight junction, brain microvascular endothelial cells, astrocytes
Citation: Kaur G, Pant P, Bhagat R and Seth P (2023) Zika virus E protein modulates functions of human brain microvascular endothelial cells and astrocytes: implications on blood-brain barrier properties. Front. Cell. Neurosci. 17:1173120. doi: 10.3389/fncel.2023.1173120
Received: 24 February 2023; Accepted: 04 July 2023;
Published: 20 July 2023.
Edited by:
Arumugam R. Jayakumar, University of Miami, United StatesReviewed by:
Asanish Kalyanasundaram, Yale University, United StatesMichal Toborek, University of Miami, United States
Copyright © 2023 Kaur, Pant, Bhagat and Seth. This is an open-access article distributed under the terms of the Creative Commons Attribution License (CC BY). The use, distribution or reproduction in other forums is permitted, provided the original author(s) and the copyright owner(s) are credited and that the original publication in this journal is cited, in accordance with accepted academic practice. No use, distribution or reproduction is permitted which does not comply with these terms.
*Correspondence: Pankaj Seth, cHNldGhAbmJyYy5hYy5pbg==