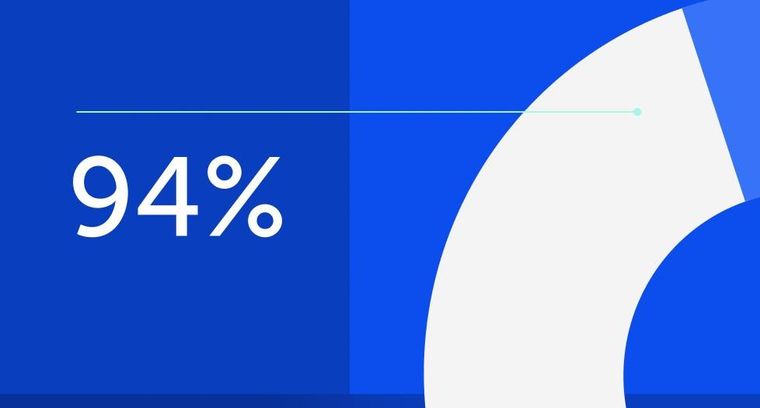
94% of researchers rate our articles as excellent or good
Learn more about the work of our research integrity team to safeguard the quality of each article we publish.
Find out more
ORIGINAL RESEARCH article
Front. Cell. Neurosci., 24 May 2023
Sec. Cellular Neurophysiology
Volume 17 - 2023 | https://doi.org/10.3389/fncel.2023.1159067
Carisbamate (CRS, RWJ-333369) is a new anti-seizure medication. It remains unclear whether and how CRS can perturb the magnitude and/or gating kinetics of membrane ionic currents, despite a few reports demonstrating its ability to suppress voltage-gated Na+ currents. In this study, we observed a set of whole-cell current recordings and found that CRS effectively suppressed the voltage-gated Na+ (INa) and hyperpolarization-activated cation currents (Ih) intrinsically in electrically excitable cells (GH3 cells). The effective IC50 values of CRS for the differential suppression of transient (INa(T)) and late INa (INa(L)) were 56.4 and 11.4 μM, respectively. However, CRS strongly decreased the strength (i.e., Δarea) of the nonlinear window component of INa (INa(W)), which was activated by a short ascending ramp voltage (Vramp); the subsequent addition of deltamethrin (DLT, 10 μM) counteracted the ability of CRS (100 μM, continuous exposure) to suppress INa(W). CRS strikingly decreased the decay time constant of INa(T) evoked during pulse train stimulation; however, the addition of telmisartan (10 μM) effectively attenuated the CRS (30 μM, continuous exposure)-mediated decrease in the decay time constant of the current. During continued exposure to deltamethrin (10 μM), known to be a pyrethroid insecticide, the addition of CRS resulted in differential suppression of the amplitudes of INa(T) and INa(L). The amplitude of Ih activated by a 2-s membrane hyperpolarization was diminished by CRS in a concentration-dependent manner, with an IC50 value of 38 μM. For Ih, CRS altered the steady-state I–V relationship and attenuated the strength of voltage-dependent hysteresis (Hys(V)) activated by an inverted isosceles-triangular Vramp. Moreover, the addition of oxaliplatin effectively reversed the CRS-mediated suppression of Hys(V). The predicted docking interaction between CRS and with a model of the hyperpolarization-activated cyclic nucleotide-gated (HCN) channel or between CRS and the hNaV1.7 channel reflects the ability of CRS to bind to amino acid residues in HCN or hNaV1.7 channel via hydrogen bonds and hydrophobic interactions. These findings reveal the propensity of CRS to modify INa(T) and INa(L) differentially and to effectively suppress the magnitude of Ih. INa and Ih are thus potential targets of the actions of CRS in terms of modulating cellular excitability.
Carisbamate (CRS, RWJ-333369), a bioactive, orally administered neuromodulator, has been demonstrated to be beneficial for the treatment of different types of convulsive disorders, including drug-resistant focal epilepsy and partial-onset seizure (Whalley et al., 2009; Sperling et al., 2010; Vohora et al., 2010; Ono et al., 2011; Faure et al., 2013, 2014; Fernandes et al., 2015; Marques-Carneiro et al., 2017; Steriade et al., 2020; Löscher et al., 2021; Lu et al., 2021; Strzelczyk and Schubert-Bast, 2021; Elkommos and Mula, 2022; Pong et al., 2022). This compound was also reported to be effective in the treatment of alcoholism (Rezvani et al., 2009, 2012). One study found that CRS prevented the development and production of epilepsy-like discharges and exerted a neuroprotective effect after epilepticus-like injury (Fernandes et al., 2015).
CRS was demonstrated to suppress glutamate transmission in granule cells of the dentate gyrus (Lee et al., 2011). It also was reported to effectively suppress action potential firing and exert neuroprotective effects in either cultured hippocampal neurons or a rat model of status epilepticus (Deshpande et al., 2008a,b, 2016a,b; Fernandes et al., 2015; Deshpande and DeLorenzo, 2020). Alternatively, CRS can inhibit voltage-gated Na+ channels (Liu Y. et al., 2009; Whalley et al., 2009; Vohora et al., 2010; Shim et al., 2013). One paper reported the ability of CRS to suppress T-type voltage-gated Ca2+ currents (Kim et al., 2017). Hence, it remains unclear whether and how CRS can perturb ionic currents.
The magnitude of the voltage-gated Na+ current (INa) through voltage-gated Na+ (NaV) channels is crucial to the generation and propagation of action potentials in excitable membranes (Catterall et al., 2020; Menezes et al., 2020). During abrupt depolarization, these channels become activated and can shift quickly from a resting (or closed) to an open state, thereby allowing the flow of Na+ ions from the extracellular solution to the cell interior under electrochemical driving forces. Na+ channels that have been opened in a voltage-dependent manner then shift to an inactive state, resulting in the transient enhancement of INa. In addition to the voltage dependence of slow INa inactivation, studies have reported the striking cumulative inhibition of the current activated during pulse train stimulation (Taddese and Bean, 2002; Huang et al., 2015; Navarro et al., 2020; Lin et al., 2022; Wu et al., 2022a). At present, it remains unclear whether and how CRS can interact with NaV channels to modify the magnitude, gating kinetics, and frequency-dependence of INa.
The hyperpolarization-activated cation current, Ih (i.e., the funny current [If]), is a key determinant of repetitive electrical activity in heart cells and various types of central neurons and endocrine or neuroendocrine cells (Belardinelli et al., 1988; Irisawa et al., 1993; Simasko and Sankaranarayanan, 1997; Stojilkovic et al., 2010; Kretschmannova et al., 2012; Wu and Huang, 2021; Choi et al., 2022; Chuang et al., 2022; Depuydt et al., 2022; Peters et al., 2022). This ionic current has unusually slow voltage-dependent activation kinetics and is a mixed, inwardly directed Na+/K+ current that is sensitive to blocking by CsCl or ivabradine (Cao et al., 2016; Hsiao et al., 2019). Activation of Ih may cause the resting potential to depolarize and, in turn, reach the threshold required to generate or elicit an action potential; as a result, Ih can affect pacemaker activity and impulse propagation (Anastasaki et al., 2022; Lei et al., 2022; Peters et al., 2022). Moreover, the slow kinetics of Ih in response to a long hyperpolarizing step can produce long-lasting, activity-dependent modification of membrane excitability in different excitable cell types (Kretschmannova et al., 2012; Peters et al., 2021; Mäki-Marttunen and Mäki-Marttunen, 2022). Ih is transmitted through channels encoded by members of the hyperpolarization-activated cyclic nucleotide-gated (HCN) gene family, and studies have shown that the activity of these channels is responsible for the ionic mechanisms of different convulsive disorders (Noam et al., 2011; Banarroch, 2013; Concepcion et al., 2021).
In this study, we extensively investigated the electrophysiological actions of CRS in excitable cells with consideration of the above-described information. We aimed to investigate the intrinsic effects of CRS on the magnitude and/or gating of INa or Ih in GH3 neuronal cells. Our findings prompt us to consider both INa and Ih in excitable cells as distinctive targets of CRS, through which it can act concertedly to influence the functional activities of the cells involved.
Carisbamate (CRS; also known as Comfyde™, RWJ-333369, YKP 509, [(2S)-2-(2-chlorophenyl)-2-hydroxyethyl] carbamate, (S)-2-O-carbamoyl-1-o-chlorophenyl-ethanol, C9H10ClNO3) and deltamethrin (DLT) were acquired from MedChemExpress (MCE®; Everything Biotech, New Taipei City, Taiwan). Telmisartan (Tel) was obtained from Tocris Cookson Ltd. (Bristol, UK). Oxaliplatin (OXAL) was obtained from Sanofi-Aventis (New York, NY, USA). Nimodipine, tetraethylammonium chloride (TEA), and tetrodotoxin (TTX) were obtained from Sigma-Aldrich (St. Louis, MO, USA). Chlorotoxin was a gift from Prof. Dr. Woei-Jer Chuang (Department of Biochemistry, National Cheng Kung University Medical College, Tainan, Taiwan). Other chemicals (e.g., CsCl, CsOH, and CdCl2) and reagents used in this work were obtained from commercial sources and of reagent grade. Reagent-grade water was de-ionized using a Milli-Q® water purification system (Millipore, Bedford, MA, USA).
HEPES-buffered normal Tyrode’s solution was used as the electrophysiological bath solution, with an ionic composition of (in mM): NaCl, 136.5; KCl, 5.4; MgCl2, 0.53; CaCl2, 1.8; glucose, 5.5; and HEPES-NaOH buffer, 5.5 (pH 7.4). The intracellular pipette solution used to measure the ions flowing through the whole-cell K+ current or hyperpolarization-activated inward current (Ih) had the following composition (in mM): Potassium aspartate, 130; KCl, 20; MgCl2, 1; Na2ATP, 3; Na2GTP, 0.1; EGTA, 0.1; and HEPES-KOH buffer, 5 (pH 7.2). To record different types of voltage-gated Na+ current (INa), we replaced K+ ions in the internal solution with equimolar Cs+ ions and titrated the pH value to 7.2 by adding CsOH; the studied cells were commonly bathed in Ca2+-free Tyrode’s solution containing 10 mM TEA. Generally, the bath and pipette solutions were filtered on the day of use through a syringe filter equipped with a 0.22-μm Supor® nylon membrane (#4612; Pall Corp.; Genechain, Kaohsiung, Taiwan).
The GH3 pituitary cell line (BCRC-60015) was obtained from the Bioresources Collection and Research Center (Hsinchu, Taiwan). The cells were maintained in 50-mL plastic culture flasks in Ham’s F-12 medium supplemented with 2.5% fetal calf serum (v/v), 15% horse serum (v/v), and 2 mM glutamine (Wu et al., 2017; Hung et al., 2021). The medium was replaced twice weekly, and the cells were carefully split into subcultures once weekly. The cells were subjected to electrophysiological measurements when they reached 60%–80% confluence (5 or 6 days after subculture).
Shortly before each measurement, the GH3 cells were carefully dispersed. A few drops of the cell suspension were rapidly transferred to a custom-built chamber, and the cells were allowed to settle on the bottom surface. The chamber used to record was tightly mounted on the stage of an inverted phase-contrast microscope (Diaphot-200; Nikon; Lin Trading Co., Taipei, Taiwan) coupled to a video camera system (magnification: 1500×) to monitor cell size during the experiments. The cells were kept at room temperature (20–25°C) in a bath of normal Tyrode’s solution containing 1.8 mM CaCl2. The patch electrodes were carefully drawn from Kimax-51 capillaries with an outer diameter of 1.5–1.8 mm (#34500; Kimble; Dogger, New Taipei City, Taiwan) using a two-stage PP-83 puller (Narishige; Taiwan Instrument, Tainan, Taiwan), and the electrode tips were fire-polished with an MF-83 microforge (Narishige). Once filled with different pipette solutions as described in the section “2.1. Chemicals, drugs, reagents, and solutions used in this work,” the tip resistance of the electrodes generally ranged from 3 to 5 MΩ. We used an RK-400 patch amplifier (Bio-Logic, Claix, France) to perform standard patch-clamp recordings in a modified whole-cell configuration (Lai et al., 2020, 2022). Junction potentials, which develop at the electrode tip when the composition of the internal solution differs from that in the bath, were nullified shortly before seal formation was made, and junction potential corrections were applied to the whole-cell data. While recording, the signal output data (i.e., potential or current tracings) were stored online at a frequency of ≥10 kHz using an ASUSPRO-BN401 LG laptop computer (ASUS; Yun-Dai, Tainan, Taiwan) equipped with a Digidata 1440A device (Molecular Devices; Advanced Biotech, New Taipei City, Taiwan) and controlled by pCLAMP 10.6 software (Molecular Devices).
We constructed and then analyzed the concentration–response curves of CRS-mediated inhibition on the peak (transient, INa(T)) and sustained (late, INa(L)) components of depolarization-activated INa and the hyperpolarization-activated inward current (Ih) present in GH3 cells. The INa was evoked by a 30-ms depolarizing pulse to −10 mV from a holding potential of −100 mV, and the current amplitudes were measured at the start (INa(T)) and end (INa(L)) of the depolarizing pulse in the presence or absence of exposure to different CRS concentrations. The Ih was activated slowly by a 2-s hyperpolarizing step from −40 to −110 mV, and the current amplitude was measured at the end of the hyperpolarizing command voltage. The concentration of CRS needed to inhibit 50% of the current amplitude (IC50) was approximated using the three-parameter logistic model, a modified form of the sigmoidal Hill equation, with goodness-of-fitness assessments as follows:
In this equation, [CRS] = the CRS concentration, nH = the Hill coefficient, and 1 – a represents the maximal inhibition.
Linear or nonlinear (e.g., exponential of sigmoidal function) regression was used to fit continuous curves to the experimental data, using pCLAMP 7 software (Molecular Devices), 64-bit OriginPro 2022b software (OriginLab®; Scientific Formosa, Kaohsiung, Taiwan), or Excel 2016 software (Microsoft, Redmond, WA, USA) with the “Solver” add-in function. The experimental data consist of different types of ionic currents and are presented as the mean ± standard error of the mean (SEM). The sample size (n) indicates the number of cells from which data were collected; and SEM error bars were plotted. The Kolmogorov Smirnov test for normality indicated that the data distribution obtained was satisfactory. To assess differences between more than two groups, we used an analysis of variance (ANOVA-1 or ANOVA-2) with or without repeated measures, followed by a post-hoc Fisher’s least significant difference test. Differences were considered statistically significant at a p-value < 0.05.
To explore the docking prediction, we first selected the structure from PDB (PDB: 5V4S) and entered it into the PyRx software. We also selected the chemical structure from PubChem (Compound CID: 6918474) and entered it into PyRx. After that, we performed appropriate docking actions in PyRx. Subsequently, we selected the results of this interaction and used LigPLOT+ software to generate an image that predicted the docking.
In our initial whole-cell experiments, we examined whether CRS exerted any effects on INa in pituitary GH3 cells. To avoid contamination by Ca2+ currents, the cells were bathed in Ca2+-free Tyrode’s solution containing 10 mM TEA and 0.5 mM CdCl2, and the recording electrode was filled with a Cs+-containing internal solution. In the recording pipette, Cl– ions were replaced with aspartate to eliminate any possible contamination by Cl– currents, because CRS was reported to alter the magnitude of Cl– currents (Whalley et al., 2009). As demonstrated in Figure 1A, after exposure of GH3 cells to CRS for 1 min at a concentration of 10 or 30 μM, the amplitudes of INa(T) and INa(L) decreased from the control values of 813 ± 15 pA (n = 7) and 9.7 ± 0.3 pA (n = 7), respectively, to 598 ± 11 pA (n = 7, p < 0.05) and 4.4 ± 0.2 pA (n = 7, p < 0.05), and to 485 ± 9 pA (n = 7, p < 0.05) and 2.5 ± 0.1 pA (n = 7, p < 0.05), respectively. After the removal of CRS, the amplitudes of INa(T) and INa(L) returned to 801 ± 14 (n = 7, p < 0.05) and 9.5 ± 0.2 pA (n = 7, p < 0.05), respectively. Continuous exposure of the cells to 1 μM TTX alone, but not to 1 μM nimodipine alone, nearly eliminated the INa(T) amplitude in response to the same voltage protocol, as demonstrated by a reduction from the control value of 814 ± 16 pA to 31 ± 5 pA (n = 7, p < 0.01). Concomitant with changes in the amplitude of INa(T) or INa(L), CRS (30 μM) decreased the time constant for the slow component of INa(T) inactivation (τinact(S)) from 9.5 ± 0.4 to 4.2 ± 0.4 ms (n = 7, p < 0.05); however, no clear change in the time constant for the fast component of current inactivation was observed.
Figure 1. Inhibitory effects of carisbamate (CRS) on the transient (peak, INa(T)) or late (sustained, INa(L)) component of the voltage-gated Na+ current (INa). (A) The exemplar current traces shown at left were acquired in the control period without CRS (a, black line) and during exposure to 10 μM (b, blue line) or 30 μM CRS (c, red line). The voltage-clamp protocol is illustrated at the top left, while the current traces shown at right represent expansions of the records from the dashed boxes in the left side of panel (A). (B) Concentration-dependent CRS-induced inhibition of INa(T) (filled blue circles) and INa(L) (open blue circles) in GH3 cells. Each data point indicates the mean ± standard error of the mean (n = 8). The red sigmoidal lines, on which the data points are overlaid, indicate the best fit to a modified Hill equation according to the averaged data obtained at each concentration of CRS, as elaborated in the section “2. Materials and methods”. The IC50 values required for CRS-mediated inhibition of INa(T) and INa(L) were 56.4 and 11.4 μM, respectively.
Figure 1B demonstrates that exposure to CRS can decrease the amplitudes of INa(T) and INa(L) evoked by a rapid depolarizing pulse in a concentration-dependent manner. According to the modified Hill equation detailed in the section “2.4. Data analyses,” the IC50 value needed for CRS-mediated suppression of INa(T) or INa(L) from GH3 cells was calculated as 56.4 or 11.4 μM, respectively, and these distinct values demonstrate the difference between the inhibitory effects of CRS on the magnitudes of INa(T) and INa(L) in these cells. Therefore, as observed during a rectangular depolarizing step, this drug exhibits a preferential inhibition of INa(L) over INa(T) when activated.
To further characterize the inhibitory effect of CRS, we explored whether this drug could alter the steady-state I–V relationship of INa(T) in GH3 cells. Figure 2 illustrates the average I–V relationship of INa(T) in the presence or absence of 30 μM CRS. Notably, the value of the threshold or maximal voltage required to elicit INa(T) did not differ between the absence and presence of CRS. Accordingly, we conclude that continuous exposure to CRS does not elicit an obvious change in the quasi-steady-state I–V relationship of INa(T), despite a significant reduction in INa(T) conductance.
Figure 2. Inhibitory effect of CRS on the steady-state current versus voltage (I–V) relationship of the transient peak component of the voltage-gated Na+ current (INa(T)). (A) Exemplar current traces acquired in pituitary GH3 cells in the control period without CRS (upper) and during exposure to 30 μM CRS (lower). The voltage-clamp protocol is illustrated at the top, and the potential traces shown in different colors correspond to the current traces evoked by the same levels of step commands. (B) Averaged I–V relationships of INa(T) acquired in the absence (blue filled circles) and presence (red open circles) of 30 μM CRS. Each point represents the mean ± standard error of the mean (n = 8). The current amplitude was obtained at the beginning of each depolarization step from a holding potential of –80 mV.
Instantaneous INa(W), which is activated in response to an upsloping (or ascending) ramp voltage (Vramp), has been demonstrated in different types of excitable cells (Morris et al., 2012; Yu et al., 2012; Wu et al., 2022a). We next examined whether CRS could alter the magnitude of INa(W) evoked by a rapidly ascending Vramp. In this set of experiments, we voltage-clamped the studied cell at −80 mV and applied an ascending Vramp from −100 to +40 mV for a duration of 50 ms (ramp speed = 2.8 mV/ms) to activate INa(W). Within 1 min of exposing the cells to CRS (30 or 100 μM), the magnitude (i.e., Δarea) was strikingly decreased due to the 50-ms ascending Vramp (Figure 3). For example, exposure to 30 or 100 μM CRS led to considerable attenuation of the Δarea of INa(W) from a control value of 4.43 ± 0.58 mV⋅nA (n = 7) to 2.91 ± 0.51 mV⋅nA (n = 7, p < 0.05) or 1.86 ± 0.47 mV⋅nA (n = 7, p < 0.05), respectively. Deltamethrin (DLT), a type II pyrethroid, was reported to activate INa (Bothe and Lampert, 2021). Under continuous exposure to 100 μM CRS, the exposure of cells to 10 μM DLT effectively increased the Δarea to 3.15 ± 0.54 mV⋅nA (n = 7, p < 0.05), indicating that CRS-mediated increase in INa(W) can be partially restored by further addition of DLT. Our results also confirm that CRS primarily inhibits INa(W) itself, rather than other types of voltage-gated ionic currents.
Figure 3. Modification by carisbamate (CRS) of a nonlinear window INa (INa(W)) in response to a short ascending ramp voltage (Vramp). (A) Exemplar current traces (i.e., the relationship of current amplitude versus non-linear membrane potential) obtained in the control period without CRS (a, black) and during exposure to 30 μM (b, red) or 100 μM CRS (c, blue). The voltage protocol is illustrated in the inset graph; the downward deflection denotes the instantaneous inward current (i.e., INa(W)) activated by a short ascending Vramp. (B) Bar graph summarizing the effect of CRS (30 or 100 μM) and CRS (100 μM) plus deltamethrin (DLT, 10 μM) on the Δarea of INa(W) (mean ± standard error of the mean; n = 7 for each bar). The Δarea was measured at voltages between –80 and +40 mV during the ascending Vramp. *Significantly different from the control group (p < 0.05), **significantly different from the CRS (30 μM) alone group (p < 0.05), and +significantly different from the CRS (100 μM) alone group (p < 0.05).
INa(T) inactivation was recently shown to accumulate during repetitive short pulses, and this accumulation is thought to be responsible for various electrical behaviors (Huang et al., 2015; Navarro et al., 2020). Therefore, an additional series of measurements was performed to ascertain whether CRS could perturb INa(T) inactivation following activation by a train of depolarizing stimuli. The studied cell was held at a voltage of −80 mV and subjected to the following stimulus protocol: repetitive depolarization from −10 mV (40 ms per pulse at a rate of 20 Hz for 1 s). INa(T) inactivation in GH3 cells was evoked by 1-s repetitive depolarization from −80 to −10 mV with a decay time constant of 56.4 ± 5.6 ms (n = 7), which was obtained during the control period (i.e., absence of CRS) (Figure 4). In other words, a rapid current decay occurred as a function of time in a single-exponential manner. In particular, while exposing cells to 10 or 30 μM CRS, the decay time constant of INa(T) activated by the same train of depolarizing stimuli decreased considerably to 48.3 ± 4.3 ms (n = 7, p < 0.05) or 31.2 ± 2.8 ms (n = 7, p < 0.05), respectively. Tel, an antagonist of the angiotensin II receptor, has been recognized as an effective activator of INa (Chang et al., 2018; Chang and Wu, 2018; Lai et al., 2020). The addition of Tel (10 μM) increased the decay time constant of the current to 40.4 ± 4.5 ms (n = 7, p < 0.05). Our results confirm that that CRS primarily inhibits Na+ current, which show cumulative inhibition, rather than other type of voltage-gated ionic currents.
Figure 4. Effect of carisbamate (CRS) on the transient peak component of the voltage-gated Na+ current (INa(T)) during a train of depolarizing command voltages. (A) Exemplar current traces during the control period without CRS (a, black color) or exposure to 30 μM CRS (b, red color) are shown at left. The upper left graph depicts the applied voltage-clamp protocol. The graph at right illustrates the expanded record from the purple dashed box at left. (B) The relationship between the INa(T) amplitude and pulse duration without CRS (a, black filled circles) and during exposure to 30 μM CRS (b, red open circles) (mean ± standard error of the mean [SEM]; n = 7 for each point). Each smooth gray line is well fitted to a single exponential. (C) Bar graph summarizing the effect of CRS (10 or 30 μM) and CRS (30 μM) plus telmisartan (Tel, 10 μM) on the decaying time constant (τ) of INa(T) elicited by repetitive depolarizing command voltages (mean ± SEM; n = 7 for each bar). *Significantly different from the control group (p < 0.05), **significantly different from the CRS (10 μM) alone group (p < 0.05), and +significantly different from the CRS (30 μM) alone group (p < 0.05).
CRS was shown to effectively modify the magnitude of INa (Whalley et al., 2009), while DLT was previously reported to be a pyrethroid insecticide that can activate INa (Bothe and Lampert, 2021; Lin et al., 2022). Therefore, we next investigated whether the presence of CRS could modify the DLT-enhanced INa evoked in GH3 cells by a rapid depolarizing pulse. In this set of experiments, when establishing whole-cell current recordings, we voltage-clamped the examined cell at −100 mV and applied a 30-ms depolarizing pulse to −10 mV. In keeping with previous observations (Liu Y. et al., 2009; Whalley et al., 2009; Vohora et al., 2010), we found that exposing cells to 10 μM DLT alone for 1 min enhanced the amplitude of INa(T) or INa(L) (Figure 5). However, the subsequent addition of CRS attenuated this effect of DLT on the depolarization-mediated activation of INa(T) or INa(L). For example, as the cells were depolarized from −100 to −10 mV to evoke INa, exposing the cells to 10 μM DLT resulted in a considerable increase in INa(T) or INa(L) from a control value of 412 ± 14 pA (n = 8) or 89 ± 9 pA (n = 8), respectively, to 607 ± 18 pA (n = 8, p < 0.05) or 178 ± 13 pA (n = 8, p < 0.05), respectively. Moreover, the further addition of 10 μM CRS to the cells, which remained under continuous exposure to DLT, greatly reduced INa(T) or INa(L) to 478 ± 15 pA (n = 8, p < 0.05) or 141 ± 11 pA (n = 8, p < 0.05), respectively. In this regard, the experimental data reflect that CRS could modify the DLT-mediated enhancement of the magnitude of INa(T) and INa(L) in GH3 cells.
Figure 5. Effects of deltamethrin (DLT) alone and plus carisbamate (CRS) on the voltage-gated Na+ current (INa). The cells were bathed in Ca2+-free Tyrode’s solution containing 10 mM tetraethylammonium chloride and 0.5 mM CdCl2, and the measuring electrode was filled with a Cs+-enriched solution. (A) Exemplar current traces obtained in the presence of DLT (10 μM) alone (a, black), DLT (10 μM) plus CRS (30 μM), or DLT (10 μM) plus CRS (100 μM). (B) Expanded records from the pink dashed box in panel (A). Of note, exposure to CRS effectively attenuated DLT-mediated stimulation of INa(T) and INa(L) in GH3 cells.
We further investigated the effects of CRS and other relevant compounds on Ih. In these experiments, we placed cells in Ca2+-free Tyrode’s solution and filled the recording electrode with a K+-enriched solution. We then applied long-lasting step hyperpolarization to activate an inwardly directed current with slowly activating and deactivating properties. Studies have identified this type of ionic current as the Ih or If (Figure 6A) (Belardinelli et al., 1988; Irisawa et al., 1993; Simasko and Sankaranarayanan, 1997; Liu Y. C. et al., 2009; Wu et al., 2022b; Chuang et al., 2022). Within 1 min of exposing the GH3 cells to CRS (10 or 30 μM), the Ih amplitude in response to such membrane hyperpolarization exhibited an evident decrease. For example, as the cells were hyperpolarized from −40 to −110 mV for a duration of 2 s, exposure to CRS at 10 or 30 μM noticeably reduced the amplitude of the late inward current at the end of hyperpolarization from a control value of 136 ± 18 pA (n = 8) to 103 ± 17 pA (n = 8, p < 0.05) or 73 ± 12 pA (n = 8, p < 0.05), respectively. Upon returning to −40 mV, the amplitude of the deactivating Ih decreased from a control value of 93 ± 13 pA (n = 8) to 73 ± 12 pA (n = 8, p < 0.05) or 51 ± 9 pA (n = 8, p < 0.05) under exposure to 10 or 30 μM CRS, respectively. As shown in Figure 6A, the activation time constant (τact) of Ih elicited during long membrane hyperpolarization also increased, as evidenced by a significant rise in the τact value from 212 ± 12 to 402 ± 19 m (n = 8, p < 0.05) in the presence of 30 μM CRS. This suppression of Ih in GH3 cells was readily reversed upon the removal of CRS.
Figure 6. Effect of carisbamate (CRS) on the hyperpolarization-activated cation current (Ih). This set of measurements was obtained from cells bathed in Ca2+-free Tyrode’s solution containing 1 μM tetrodotoxin, and the measuring electrode was filled with a K+-enriched solution. (A) Exemplar current traces (i.e., Ih) acquired in the control period without CRS (a, black line) and during exposure to 10 μM (b, blue line) or 30 μM CRS (c, red line). The upper graph depicts the applied voltage-clamp protocol. (B) Concentration-dependent CRS-mediated suppression of Ih activation via 2-s long-lasting membrane hyperpolarization (mean ± standard error of the mean, n = 8 for each point). The gray sigmoidal line, on which the data points are overlaid, indicates the best fit to a modified Hill equation as elaborated in the Materials and methods. The current amplitude was obtained at different CRS concentrations (3 μM–1 mM) at the end of a 2-s period of hyperpolarizing command voltage to –110 mV from a holding potential of –40 mV. The IC50 value required for CRS-mediated inhibition of Ih was 38 μM.
The relationship between the concentration of CRS and the relative amplitude of Ih is illustrated in Figure 6B. Exposure to CRS was found to reduce the amplitude of Ih during long membrane hyperpolarization in a concentration-dependent manner. Using the modified Hill equation, the IC50 value required for CRS to inhibit the Ih amplitude as seen in GH3 cells was estimated to be 38 μM; 1 mM CRS almost completely inhibited the current amplitude.
We further studied the average I–V relationship for Ih in the presence or absence of 30 μM CRS. As shown in Figures 7A, B, this I–V relationship exhibited robust, inwardly rectifying non-ohmic behavior. Under exposure to CRS, the Ih amplitude in the cells was depressed throughout the voltage clamp step. For example, CRS exposure markedly decreased the whole-cell Ih conductance, measured between −120 and −100 mV, from 6.9 ± 0.2 to 5.3 ± 0.2 nS (n = 8, p < 0.05); after washout of CRS, the Ih conductance was restored to 6.7 ± 0.2 nS (n = 7, p < 0.05).
Figure 7. Inhibitory effect of carisbamate (CRS) on the average steady-state current versus voltage (I–V) relationship of the hyperpolarization-activated cation current (Ih). The cells were bathed in Ca2+-free Tyrode’s solution, and a series of 2-s voltage steps between –120 and –40 mV (10 mV per step) was delivered to each studied cell. (A) Exemplar current traces obtained in the control period without CRS (upper) and during exposure to 30 μM CRS. The upper graph depicts the voltage-clamp protocol applied to the tested cell. Potential traces shown in different colors correspond to the ionic currents that were evoked by the same level of command voltage. (B) Average I–V relationship of Ih acquired in the absence (filled black squares) and presence (open red squares) of 30 μM CRS (mean ± standard error of the mean; n = 8 for each point). The current amplitude was measured at the end of each voltage step with a duration of 2 s.
The Hys(V) behavior of Ih has been shown to strongly influence electrical behaviors (i.e., action potential firing) in different types of excitable cells (Mãnnikkõ et al., 2005; Fürst and D’Alvanzo, 2015; Chan et al., 2020; Chuang et al., 2022; Wu et al., 2022b). For this reason, we explored whether CRS could perturb the Hys(V) behavior of Ih. In this stage of whole-cell experiments, we applied a long-lasting inverted triangular Vramp for a duration of 2 s (i.e., with a ramp pulse of ±40 mV/s) to measure the Hys(V) properties. As shown in Figure 8, the trajectory of Ih activation in response to the downsloping (i.e., hyperpolarization from −40 to −120 mV) and upsloping (depolarization from −120 to −40 mV) limbs of Vramp as a function of time was distinguishable. The current magnitude in response to the downsloping (descending) limb of the inverted triangular Vramp was much larger than that evoked by the upsloping (ascending) limb. In terms of the relationship between Ih amplitude and membrane potential, we clearly observed the Hys(V) behavior of the current amplitude in a clockwise direction. Interestingly, when the GH3 cells were continually exposed to CRS, the Hys(V) strength of Ih evoked by the inverted triangular Vramp progressively decreased (Figure 8). For example, the strength (i.e., Δarea) of Vramp-induced Ih in the control period (i.e., absence of CRS) was 4.16 ± 0.31 mV⋅nA (n = 8). However, after exposure to 10 or 30 μM CRS for 1 min, the value of Hys(V)’s Δarea in GH3 cells was reduced significantly to 3.24 ± 0.25 mV⋅nA (n = 8, p < 0.05) or 2.92 ± 0.22 mV⋅nA (n = 8, p < 0.05), respectively. OXAL, a chemotherapeutic drug, has been shown to activate Ih (Resta et al., 2018; Chang et al., 2020; Yongning et al., 2021). In cells exposed continuously to 30 μM CRS, the subsequent application of OXAL (10 μM) reversed the CRS-mediated decrease in the Δarea to 4.23 ± 0.28 mV⋅nA (n = 8, p < 0.05).
Figure 8. Attenuating effect of carisbamate (CRS) on the voltage-dependent hysteresis (Hys(V)) of the hyperpolarization-activated cation current (Ih). (A) Exemplar Hys(V) (i.e., current trace versus ramp voltage [Vramp]) acquired in the absence (upper graph, gray line) and presence (lower graph, red line) of 30 μM CRS. The inset in the upper graph depicts the applied Vramp protocol. Dashed arrows show the direction of Ih or the voltage trace in which time passes during the elicitation of an inverted triangular Vramp. (B) Bar graph summarizing the effects of CRS (10 or 30 μM) and CRS (30 μM) plus oxaliplatin (OXAL, 10 μM) on the Δarea of the Hys(V) of Ih (mean ± standard error of the mean; n = 8 for each yellow bar). The Δarea, indicated as a function of the strength of the window component of the voltage-gated Na+ current (INa(W)), was calculated under the encircled area, which was activated during the downsloping (descending) and upsloping (ascending) ends of the triangular Vramp. Note the Vramp-induced Hys(V) for Ih elicitation; the strength of this Hys(V) was attenuated by adding CRS. *Significantly different from the control group (p < 0.05), **significantly different from the CRS (10 μM) alone group (p < 0.05), and +significantly different from the CRS (30 μM) alone group (p < 0.05).
We wanted to study how CRS could interact with the HCN channel protein, but since it is not known so far the isoform(s) blocked by the CRS, we have used the structure of a closely related CNG (cyclic nucleotide-gated) channel. Information from the provider of the original structure data from PDB (James et al., 2017) revealed several close relationships between the cryostructure of CNG and HCN. The CNG channel protein structure was obtained from PDB (PDB ID: 5V4S); we used PyRx software to see how the CRS molecule could dock into the channel protein. Figure 9 presents the predicted docking sites of CRS for interaction with amino acid residues of the CNG channel. Specifically, the CRS molecule was predicted to form hydrophobic contacts with the CNG channel residues Ala232(A), Ala232(B), Ala232(C), Ser233(A) Ser233(B), Gly236(C), Gly236(D), and Ser237(D), as well as hydrogen bonds with residues Ala232(D) and Ser232(C), with estimated distances of 3.15 and 3.05 Å, respectively. It is likely that the CRS molecule interacts with the transmembrane region (position 211–238) or membrane segment (position 210–235 or 211–231) of the CNG channel, although details of these binding sites remain unclear. Moreover, as shown in Supplementary Figure 1, the docking interaction of the CRS molecule with the hNaV1.7 channel was generated in the Supplementary information.
Figure 9. Predicted docking between the CNG (cyclic nucleotide-gated) channel and the carisbamate (CRS) molecule. (Left panel) Depicts the CNG channel protein structure acquired from RCSB PDB (PDB ID: 5V4S) and the CRS molecular structure acquired from PubChem (Compound CID: 6918474). PyRx software (https://pyrx.sourceforge.io/) (accessed on 28 April 2023) was used to show that the HCN channel structure can be properly docked by the CRS molecule. (Right panel) Depicts an expansion of the red box in the left panel arrow. This diagram of the interaction between the CNG channel and the CRS molecule was generated using LigPlot+ (https://www.ebi.ac.uk/thornton-srv/software/LIGPLOT/) (accessed on 28 April 2023). The red arcs over which spokes radiate toward the ligand (i.e., CRS) represent hydrophobic contacts, while the green dotted lines indicate hydrogen bond formation. The graph in the right panel indicates an expanded display of red box with a curve arrow in the left side.
The current study has yielded several main findings. First, CRS can exert the inhibitory effect on INa which can vary depending on the concentration applied, time course of the current, and stimulus frequency used. Second, exposure to CRS led to differential suppression of INa(T) and INa(L) activation in response to a brief step depolarization, with effective IC50 values of 56.4 and 11.4 μM, respectively. Third, CRS failed to alter the steady-state I–V relationship of INa(T) in GH3 cells but decreased the decay time constant of cumulative INa(T) inactivation during pulse train stimulation. Fourth, DLT-mediated enhancement of INa was attenuated by subsequent exposure to CRS. Fifth, CRS elicited a concentration-dependent decrease in Ih amplitude, with an IC50 value of 38 μM. Sixth, CRS suppressed the Hys(V) strength of Ih activation in response to a long-lasting isosceles-triangular Vramp. Collectively, these results demonstrate that CRS-mediated changes in the magnitude, gating properties, and frequency dependence of INa and in the magnitude of Ih and Hys(V) can modify the functional activities of electrically excitable cells.
The IC50 value required for CRS-mediated inhibition of INa(T) or INa(L) was lower than the concentration used to suppress the evoked action potentials in previous studies of piriform cortical neurons or hippocampal neurons (Liu Y. et al., 2009; Whalley et al., 2009). Unlike a previous report showing inability of CRS to alter INa(L) measured from rat hippocampal neurons (Liu Y. et al., 2009), the IC50 value required for CRS-mediated inhibition of INa(T) in rat hippocampal neurons was estimated to be around 100 μM (Liu Y. et al., 2009), which is greater than the IC50 values that we estimated for the suppression of INa(T) or INa(L). Therefore, during cell exposure to CRS at pharmacologically achievable concentrations, it is plausible to assume that CRS-induced inhibition of INa is an important ionic mechanism underlying the perturbing effects of the drug on membrane excitability.
In this study, the exponential decline of INa(T) during a 20-Hz train of depolarizing pulses (i.e., 40-ms depolarizing pulses from −80 to −10 mV at 20 Hz for 1 s) became pronounced with exposure to CRS. The results reflect the frequency dependence of INa(T) during pulse train stimulation, consistent with recent studies (Huang et al., 2015; Navarro et al., 2020; Wu et al., 2022a). As a result, exposure to CRS would lead to a loss of function due to changes in the time-dependent current inactivation. Therefore, the CRS-mediated decrease in INa(T) is closely linked to the strong frequency-dependent decrease in INa(T) during pulse train stimulation (Navarro et al., 2020).
An earlier report showed that CRS could suppress the magnitude of a T-type Ca2+ current (Kim et al., 2017). Therefore, we postulated that in GH3 cells, a CRS-induced block of INa could be due, in part, to the inhibitory effect of the drug on the voltage-gated Ca2+ currents that are functionally expressed in excitable cells (Wu et al., 2003; Stojilkovic et al., 2010). However, under our experimental conditions, the voltage-gated inward currents were either sensitive to stimulation by Tel and DLT or were suppressed by TTX. Tel and DLT were previously shown to activate INa (Chang and Wu, 2018; Lai et al., 2020; Bothe and Lampert, 2021; Lin et al., 2022), while TTX is a potent inhibitor of INa. Under continuous exposure to CRS, exposure to DLT strikingly counteracted the CRS-induced decrease in the instantaneous INa(W) in GH3 cells, and exposure to Tef reversed the CRS-mediated reduction of the decaying time constant of INa(T) inactivation in response to PT depolarizing stimuli. Alternatively, neither CdCl2 nor nimodipine effectively inhibited the inward currents in GH3 cells. These results prompt us to reflect that, INa (INa(T), INa(L) and INa(W)) is susceptible to blockade by CRS, and that this drug exerts stronger suppressive effects on INa(L) than on INa(T). The action of CRS on excitable membranes cannot be explained solely by its ability to suppress the magnitude of a T-type Ca2+ current.
CRS was shown to increase Cl– conductance in piriform cortical neurons (Whalley et al., 2009). In our study, the Ih amplitude was not inhibited by chlorotoxin (1 μM) but was inhibited by ivabradine (10 μM) (data not shown). Similarly, chlorotoxin did not suppress the amplitude of INa(T) or INa(L) in tested cells. Chlorotoxin is known to suppress Cl– channels. It is therefore tempting to speculate that CRS-induced inhibition of INa or Ih is not associated with an increase in Cl– channel activity.
In this study, exposure to CRS led to a change in Ih in a concentration-dependent manner, with an IC50 value of 38 μM. There also was a marked retardation of the activation time course (i.e., an increase in the τact value) of Ih in response to a 2-s hyperpolarizing command voltage. Increasing evidence suggests that the strength of non-equilibrium Hys(V) in Vramp-evoked Ih plays an essential role in modifying electrical behavior (e.g., action potential firing) in various types of excitable cells (Mãnnikkõ et al., 2005; Fürst and D’Alvanzo, 2015; Peters et al., 2021, 2022; Wu et al., 2022b). In our study, exposure to CRS also suppressed the Hys(V) of Ih activation during the triangular Vramp, indicating that this drug is likely to interact with the voltage-sensing domains of the HCN channel.
There are four mammalian isoforms of HCN, namely HCN1, HCN2, HCN3, and HCN4, which constitute the macroscopic Ih (or If) (Fürst and D’Alvanzo, 2015; Peters et al., 2022). HCN2, HCN3, and mixed HCN2+HCN3 channels are abundantly expressed in GH3 cells and other types of endocrine and neuroendocrine cells (Kretschmannova et al., 2006, 2012). We emphasize that Ih has been demonstrated to be functionally present in heart cells (Belardinelli et al., 1988; Irisawa et al., 1993; Depuydt et al., 2022). Therefore, the CRS-mediated inhibition of Ih seen in excitable cells is very likely to be responsible for the drug’s ability to attenuate an increase in heart rate induced by exposure to organophosphate administration, as described previously (Deshpande et al., 2016a,b; Deshpande and DeLorenzo, 2020). Apart from the ability of CRS to inhibit INa, as detailed above, CRS-mediated changes in the magnitude, gating kinetics, and Hys(V) behavior of Ih also may be of pharmacological or therapeutic relevance.
In dendritic synapses, Ih has a shunt mechanism that paces the excitatory post-synaptic potential amplitude and duration and limits temporal summation (Magee, 1998, 1999). HCN2 is strongly expressed in the thalamus, where it is involved in the spontaneous firing of thalamocortical neurons in oscillation or in the 3-Hz spike and wave pattern (Poolos, 2004). A study of human subjects revealed a role for HCN2 in susceptibility to juvenile myoclonic epilepsy (Wu et al., 2018). HCN2-knockout animals have been reported to develop spontaneous absence seizures and cardiac sinus arrhythmia (Poolos, 2004; Heuermann et al., 2016). Dendritic HCN1 and HCN2 channels were found to be downregulated in a pilocarpine model of epilepsy, while in the chronic period, expression of these channels increased (Jung et al., 2007). The differential regulation of Ih in different seizure models suggests that a region-specific density of Ih may serve various purposes in epileptogenesis and may play a role in protection against or resistance to seizures. Furthermore, an increased Ih may co-exist with, and possibly contribute to, persistent dendritic hyperexcitability following febrile seizures in the hippocampus, in contrast to the exclusively anti-convulsive role often attributed to an increased Ih (Dyhrfjeld-Johnsen et al., 2008). Interestingly, the inhibitory action of Ih may be caused by its interaction with the delayed-rectifier M-type K+ current, which can enhance or inhibit spike firing in response to an excitatory post-synaptic potential when the spike threshold is low or high, respectively (George et al., 2009). Additionally, the coupling of Ih to A-type K+ channels was shown to regulate neuronal excitability (Mishra and Narayanan, 2015). Further studies are needed to clarify the concerted effects of CRS on Ih and INa in epileptogenesis.
Finally, the modulatory actions of CRS on ion channels demonstrated in this report are not limited to the interactions of this drug with NaV channels. The inhibitory effects of CRS on INa(T), INa(L), INa(W), and Ih are also expected to constitute important underlying mechanisms through which the drug exerts concerted effects on cellular function and excitability.
The original contributions presented in this study are included in the article/Supplementary material, further inquiries can be directed to the corresponding authors.
T-YH, S-NW, and C-WH designed the experiment, analyzed the data, and wrote the manuscript. S-NW and C-WH performed the experiments and created the figures. All authors contributed to the article and approved the submitted version.
This research described in this manuscript was partly supported by grants from the National Science and Technology Council (MOST-111-2320-B-006-028, MOST-110-2314-B-006-056, and 111-2314-B-006-103-MY2) and National Cheng Kung University Hospital (NCKUH-11201005). The funders of this research were not involved in the study design, data collection, analyses, or interpretation.
We gratefully recognize Meng-Cheng Yu for his technical assistance. Professional English language editing support provided by AsiaEdit (asiaedit.com).
The authors declare that the research was conducted in the absence of any commercial or financial relationships that could be construed as a potential conflict of interest.
All claims expressed in this article are solely those of the authors and do not necessarily represent those of their affiliated organizations, or those of the publisher, the editors and the reviewers. Any product that may be evaluated in this article, or claim that may be made by its manufacturer, is not guaranteed or endorsed by the publisher.
The Supplementary Material for this article can be found online at: https://www.frontiersin.org/articles/10.3389/fncel.2023.1159067/full#supplementary-material
Anastasaki, C., Mo, J., Cehn, J. K., Chatterjee, J., Pan, Y., Scheaffer, S. M., et al. (2022). Neuronal hyperexcitability drives central and peripheral nervous system tumor progression in models of neurofibromatosis-1. Nat. Commun. 13:2785. doi: 10.1038/s41467-022-30466-6
Banarroch, E. E. (2013). HCN channels: function and clinical implications. Neurology 80, 304–310. doi: 10.1212/WNL.0b013e31827dec42
Belardinelli, L., Giles, W. R., and West, A. (1988). Ionic mechanisms of adenosine actions in pacemaker cells from rabbit heart. J. Physiol. 405, 615–633. doi: 10.1113/jphysiol.1988.sp017352
Bothe, S. N., and Lampert, A. (2021). The insecticide deltamethrin enhances sodium channel slow inactivation of human Nav1.9, Nav1.8 and Nav1.7. Toxicol. Appl. Pharmacol. 428:115676. doi: 10.1016/j.taap.2021.115676
Cao, Y., Pang, J., and Zhou, P. (2016). HCN channel as therapeutic targets for heart failure and pain. Curr. Top. Med. Chem. 16, 1855–1861. doi: 10.2174/1568026616666151215104058
Catterall, W. A., Lenaeus, M. J., and Gamal El-Din, T. M. (2020). Structure and pharmacology of voltage-gated sodium and calcium channels. Annu. Rev. Pharmacol. Toxicol. 60, 133–154. doi: 10.1146/annurev-pharmtox-010818-021757
Chan, M. H., Chen, H. H., Lo, Y. C., and Wu, S. N. (2020). Effectiveness in the block by honokiol, a dimerized allylphenol from Magnolia officinalis, of hyperpolarization-activated cationic current and delayed-rectifier K+ current. Int. J. Mol. Sci. 21:4260. doi: 10.3390/ijms21124260
Chang, T. T., Yang, C. J., Lee, Y. C., and Wu, S. N. (2018). Stimulatory action of telmisartan, an antagonist of angiotensin II receptor, on voltage-gated Na+ current: experimental and theoretical studies. Chin. J. Physiol. 61, 1–13. doi: 10.4077/CJP.2018.BAG516
Chang, W. T., Gao, Z. H., Li, S. W., Liu, P. Y., Lo, Y. C., and Wu, S. N. (2020). Characterization in dual activation by oxaliplatin, a platinum-based chemotherapeutic agent of hyperpolarization-activated cation and electroporation-induced currents. Int. J. Mol. Sci. 21:396. doi: 10.3390/ijms21020396
Chang, W. T., and Wu, S. N. (2018). Activation of voltage-gated sodium current and inhibition of erg-mediated potassium current caused by telmisartan, an antagonist of angiotensin II type-1 receptor, in HL-1 atrial cardiomyocytes. Clin. Exp. Pharmacol. Physiol. 45, 797–807. doi: 10.1111/1440-1681.12943
Choi, K., Yi, J. H., Lee, C., Park, K., Kang, S. J., and Shin, K. S. (2022). Presynaptic HCN channel activity is required for the expression of long-term potentiation at lateral amygdala to basal amygdala synapses. Biochem. Biophys. Res. Commun. 637, 100–107. doi: 10.1016/j.bbrc.2022.11.020
Chuang, C. W., Chang, K. P., Cho, H. Y., Chuang, T. H., Yu, M. C., Wu, C. L., et al. (2022). Characterization of inhibitory capability on hyperpolarization-activated cation current caused by lutein (β,ε-carotene-3,3-diol), a dietary xanthophyll carotenoid. Int. J. Mol. Sci. 23:7186. doi: 10.3390/ijms23137186
Concepcion, F. A., Khan, M. N., Ju Wang, J. D., Wei, A. D., Ojemann, J. G., Ko, A. L., et al. (2021). HCN channel phosphorylation sites mapped by mass spectrometry in human epilepsy patients and in an animal model of temporal lobe epilepsy. Neuroscience 460, 13–30. doi: 10.1016/j.neuroscience.2021.01.038
Depuydt, A. S., Peigneur, S., and Tytgat, J. (2022). Review: HCN channels in the heart. Curr. Cardiol. Rev. 18:e040222200836.
Deshpande, L. S., Blair, R. E., Huang, B. A., Phillips, K. F., and DeLorenzo, R. J. (2016a). Pharmacological blockade of the calcium plateau provides neuroprotection following organophosphate paraoxon induced status epilepticus in rats. Neurotoxicol. Teratol. 56, 81–86. doi: 10.1016/j.ntt.2016.05.002
Deshpande, L. S., Blair, R. E., Phillips, K. F., and DeLorenzo, R. J. (2016b). Role of the calcium plateau in neuronal injury and behavioral morbidities following organophosphate intoxication. Ann. N. Y. Acad. Sci. 1374, 176–183. doi: 10.1111/nyas.13122
Deshpande, L. S., and DeLorenzo, R. J. (2020). Novel therapeutics for treating organophosphate-induced status epilepticus co-morbilities, based on changes in calcium homeostasis. Neurobiol. Dis. 133:104418. doi: 10.1016/j.nbd.2019.03.006
Deshpande, L. S., Nagarkatti, N., Sombati, S., and DeLorenzo, R. J. (2008a). The novel antiepileptic drug carisbamate (RWJ 333369) is effective in inhibiting spontaneous recurrent seizure discharges and blocking sustained repetitive firing in cultured hippocampal neurons. Epilepsy Res. 79, 158–165. doi: 10.1016/j.eplepsyres.2008.01.002
Deshpande, L. S., Nagarkatti, N., Ziobro, J. M., Sombati, S., and DeLorenzo, R. J. (2008b). Carisbamate prevents the development and expression of spontaneous recurrent epileptiform discharges and is neuroprotective in cultured hippocampal neurons. Epilepsia 49, 1795–1802. doi: 10.1111/j.1528-1167.2008.01667.x
Dyhrfjeld-Johnsen, J., Morgan, R. J., Földy, C., and Soltesz, I. (2008). Upregulated H-current in hyperexcitable CA1 dendrites after febrile seizures. Front. Cell. Neurosci. 2:2. doi: 10.3389/neuro.03.002.2008
Elkommos, S., and Mula, M. (2022). Current and future pharmacotherapy options for drug-resistant epilepsy. Expert Opin. Pharmacother. 23, 2023–2034. doi: 10.1080/14656566.2022.2128670
Faure, J. B., Akimana, G., Carneiro, J. E., Cosquer, B., Ferrandon, A., Geiger, K., et al. (2013). A comprehensive behavioral evaluation in the lithium-pilocarpine model in rats: effects of carisbamate administration during status epilepticus. Epilepsia 54, 1203–1213. doi: 10.1111/epi.12219
Faure, J. B., Marques-Carneiro, J. E., Akimana, G., Cosquer, B., Ferrandon, A., Herbeaux, K., et al. (2014). Attention and executive functions in a rat model of chronic epilepsy. Epilepsia 55, 644–653. doi: 10.1111/epi.12549
Fernandes, M. J., Carneiro, J. E., Amorim, R. P., Araujo, M. G., and Nehlig, A. (2015). Neuroprotective agents and modulation of temporal lobe epilepsy. Front. Biosci. 7, 79–93. doi: 10.2741/E719
Fürst, O., and D’Alvanzo, N. (2015). Isoform dependent regulation of human HCN channels by cholesterol. Sci. Rep. 5:14270. doi: 10.1038/srep14270
George, M. S., Abbott, L. F., and Siegelbaum, S. A. (2009). HCN hyperpolarization-activated cation channels inhibit EPSPs by interactions with M-type K(+) channels. Nat. Neurosci. 12, 577–584. doi: 10.1038/nn.2307
Heuermann, R. J., Jaramillo, T. C., Ying, S. W., Suter, B. A., Lyman, K. A., Han, Y., et al. (2016). Reduction of thalamic and cortical Ih by deletion of TRIP8b produces a mouse model of human absence epilepsy. Neurobiol. Dis. 85, 81–92. doi: 10.1016/j.nbd.2015.10.005
Hsiao, H. T., Liu, Y. C., Liu, P. Y., and Wu, S. N. (2019). Concerted suppression of Ih and activation of IK(M) by ivabradine, and HCN channel inhibitor, in pituitary cells and hippocampal neurons. Brain Res. Bull. 149, 11–20. doi: 10.1016/j.brainresbull.2019.03.016
Huang, C. W., Hung, T. Y., and Wu, S. N. (2015). The inhibitory actions by lacosamide, a functionalized amino acid, on voltage-gated Na+ currents. Neuroscience 287, 125–136. doi: 10.1016/j.neuroscience.2014.12.026
Hung, T. Y., Wu, S. N., and Huang, C. W. (2021). The integrated effects of brivaracetam, a selective analog of levetiracetam, on ionic currents and neuronal excitability. Biomedicines 9:369. doi: 10.3390/biomedicines9040369
Irisawa, H., Brown, H. F., and Giles, W. (1993). Cardiac pacemaking in the sinoatrial node. Physiol. Rev. 73, 197–227. doi: 10.1152/physrev
James, Z. M., Borst, A. J., Haitin, Y., Frenz, B., DiMaio, F., Zagotta, W. N., et al. (2017). CryoEM structure of a prokaryotic cyclic nucleotide-gated ion channel. Proc. Natl. Acad. Sci. U S A. 114, 4430–4435. doi: 10.1073/pnas.1700248114
Jung, S., Jones, T. D., Lugo, J. N. Jr., Sheerin, A. H., Miler, J. W., D’Ambrosio, R., et al. (2007). Progressive dendritic HCN channelopathy during epileptogenesis in the rat pilocarpine model of epilepsy. J. Neurosci. 27, 13012–13021. doi: 10.1523/JNEUROSCI.3605-07.2007
Kim, D. Y., Zhang, F. X., Nakanishi, S. T., Mettler, T., Cho, I. H., Ahn, Y., et al. (2017). Carisbamate blockade of T-type voltage-gated calcium channels. Epilepsia 58, 617–626. doi: 10.1111/epi.13710
Kretschmannova, K., Gonzalez-Iglesias, A. E., Tomić, M., and Stojilkovic, S. S. (2006). Dependence of hyperpolarisation-activated cyclic nucleotide-gated channel activity on basal cyclic adenosine monophosphate production in spontaneously firing GH3 cells. J. Neuroendocrinol. 18, 484–493. doi: 10.1111/j.1365-2826.2006.01438.x
Kretschmannova, K., Kucka, M., Gonzalez-Iglesias, A. E., and Stojilkovic, S. S. (2012). The expression and role of hyperpolarization-activate and cyclic nucleotide-gated channels in endocrine anterior pituitary cells. Mol. Endocrinol. 26, 153–164. doi: 10.1210/me.2011-1207
Lai, M. C., Wu, S. N., and Huang, C. W. (2020). Telmisartan, an antagonist of angiotensin II receptors, accentuates voltage-gated Na+ currents and hippocampal neuronal excitability. Front. Neurosci. 14:902. doi: 10.3389/fnins.2020.00902
Lai, M. C., Wu, S. N., and Huang, C. W. (2022). Zingerone modulates neuronal voltage-gated Na+ and L-type Ca2+ currents. Int. J. Mol. Sci. 23:3123. doi: 10.3390/ijms23063123
Lee, C. Y., Lee, M. L., Shih, C. C., and Liou, H. H. (2011). Carisbamate (RWJ-333369) inhibits glutamate transmission in the granule cell of the dentate gyrus. Neuropharmacology 61, 1239–1247. doi: 10.1016/j.neuropharm.2011.07.022
Lei, X., Zeng, J., Yan, Y., and Liu, X. (2022). Blockage of HCN channels inhibits the function of P2X receptors in rat dorsal root ganglion neurons. Neurochem. Res. 47, 1083–1096. doi: 10.1007/s11064-021-03509-5
Lin, M. H., Lin, J. F., Yu, M. C., Wu, S. N., Wu, C. L., and Cho, H. Y. (2022). Characterization in potent modulation on voltage-gated Na+ current exerted by deltamethrin, a pyrethroid insecticide. Int. J. Mol. Sci. 23:14733. doi: 10.3390/ijms232314733
Liu, Y., Yohrling, G. J., Wang, Y., Hutchinson, T. L., Brenneman, D. E., Flores, C. M., et al. (2009). Carisbamate, a novel neuromodulator, inhibits voltage-gated sodium channels and action potential firing of rat hippocampal neurons. Epilepsy Res. 83, 66–72. doi: 10.1016/j.eplepsyres.2008.09.006
Liu, Y. C., Wang, Y. J., Wu, P. Y., and Wu, S. N. (2009). Tramadol-induced block of hyperpolarization-activated cation current in rat pituitary lactotrophs. Naunyn-Schmiedeberg’s Arch. Pharmacol. 379, 127–135. doi: 10.1007/s00210-008-0353-0
Löscher, W., Sills, G. J., and White, H. S. (2021). The ups and downs of alkyl-carbamates in epilepsy therapy: how does cenobamate differ? Epilepsia 62, 596–614. doi: 10.1111/epi.16832
Lu, C., Zheng, J., Cao, Y., Bresnahan, R., and Martin-McGill, K. J. (2021). Carisbamate add-on therapy for drug-resistant focal epilepsy. Cochrane Database Syst. Rev. 12:CD012121. doi: 10.1002/14651858.CD012121.pub2
Magee, J. C. (1998). Dendritic hyperpolarization-activated currents modify the integrative properties of hippocampal CA1 pyramidal neurons. J. Neurosci. 18, 7613–7624.
Magee, J. C. (1999). Dendritic Ih normalizes temporal summation in hippocampal CA1 neurons. Nat. Neurosci. 2:848. doi: 10.1038/12229
Mäki-Marttunen, T., and Mäki-Marttunen, V. (2022). Excitatory and inhibitory effects of HCN channel modulation on excitability of layer V pyramidal cells. PLoS Comput. Biol. 18:e1010506. doi: 10.1371/journal.pcbi.1010506
Mãnnikkõ, R., Pandey, S., Larsson, H. P., and Elinder, F. (2005). Hysteresis in the voltage dependence of HCN channels: conversion between two modes affects pacemaker properties. J. Gen. Physiol. 125, 305–326. doi: 10.1085/jgp.200409130
Marques-Carneiro, J. E., Persike, D. S., Litzahn, J. J., Cassel, J. C., Nehlig, A., and Fernandes, M. J. D. S. (2017). Hippocampal proteome of rats subjected to the li-pilocarpine epilepsy model and the effect of carisbamate treatment. Pharmaceuticals (Basel) 10:67. doi: 10.3390/ph10030067
Menezes, L. F. S., Sabiá Júnior, E. F., Tibery, D. V., Carneiro, L. D. A., and Schwartz, E. F. (2020). Epilepsy-related voltage-gated sodium channelopathies: a review. Front. Pharmacol. 11:1276. doi: 10.3389/fphar.2020.01276
Mishra, P., and Narayanan, R. (2015). High-conductance states and A-type K+ channels are potential regulators of the conductance- current balance triggered by HCN channels. J. Neurophysiol. 113, 23–43. doi: 10.1152/jn.00601.2013
Morris, C. E., Boucher, P. A., and Joós, B. (2012). Left-shifted nav channels in injured bilayer. Primary targets for neuroprotective nav antagonist? Front. Pharmacol. 3:19. doi: 10.3389/fphar.2012.00019
Navarro, M. A., Salari, A., Lin, J. L., Cowan, L. M., Penington, N. J., Milescu, M., et al. (2020). Sodium channels implement a molecular leaky integrator that detects action potentials and regulates neuronal firing. eLife 9:e54940. doi: 10.7554/eLife.54940
Noam, Y., Bernard, C., and Baram, T. Z. (2011). Towards an integrated view of HCN channel role in epilepsy. Curr. Opin. Neurobiol. 21, 873–879. doi: 10.1016/j.conb.2011.06.013
Ono, T., Moshe, S. L., and Galanopoulou, A. S. (2011). Carisbamate acutely suppresses spasms in a rat model of symptomatic infantile spasms. Epilepsia 52, 1678–1684. doi: 10.1111/j.1528-1167.2011.03173.x
Peters, C. H., Liu, P. W., Morotti, S., Gantz, S. C., Brandi, E., Bean, B. P., et al. (2021). Bi-directional flow of the funny current (If) during the pacemaking cycle in murine sinoatrial node myocytes. Proc. Natl. Acad. Sci. U S A. 118:e2104668118. doi: 10.1073/pnas.2104668118
Peters, C. H., Singh, R. K., Bankston, J. R., and Proenza, C. (2022). Regulation of HCN channels by protein interactions. Front. Physiol. 13:928507. doi: 10.3389/fphys.2022.928507
Pong, A. W., Ross, J., Tyrlikova, I., Giermek, A. J., Kohli, M. P., Khan, Y. A., et al. (2022). Epilepsy: expert opinion on emerging drugs in phase 2/3 clinical trials. Expert Opin. Emerg. Drugs 27, 75–90. doi: 10.1080/14728214.2022.2059464
Poolos, N. P. (2004). The Yin and Yang of the H-Channel and Its Role in Epilepsy. Epilepsy Curr. 4, 3–6. doi: 10.1111/j.1535-7597.2004.04101.x
Resta, F., Micheli, L., Laurino, A., Spinelli, V., Mello, T., Sartiani, L., et al. (2018). Selective HCN block as a strategy to control oxaliplatin-induced neuropathy. Neuropharmacology 131, 403–413. doi: 10.1016/j.neuropharm.2018.01.014
Rezvani, A. H., Lawrence, A. J., Arolfo, M. P., Levin, E. D., and Overstreet, D. H. (2012). Novel medication targets for the treatment of alcoholism: preclinical studies. Recent Pat. CNS Drug Discov. 7, 151–162. doi: 10.2174/157488912800673182
Rezvani, A. H., Overstreet, D. H., Vaidya, A. H., Zhao, B., and Levin, E. D. (2009). Carisbamate, a novel antiepileptic candidate compound, attenuates alcohol intake in alcohol-preferring rats. Alcohol. Clin. Exp. Res. 33, 1366–1373. doi: 10.1111/j.1530-0277.2009.00966.x
Shim, S., El Mansari, M., and Blier, P. (2013). Modulation of the antidepressant-like effects of sustained administration of carisbamate and lamotrigine on monoaminergic systems: electrophysiological studies in the rat brain. J. Pharmacol. Exp. Ther. 347, 487–496. doi: 10.1124/jpet.113.203315
Simasko, S., and Sankaranarayanan, S. (1997). Characterization of a hyperpolarization-activated cation current in rat pituitary cells. Am. J. Physiol. 272 (3 Pt 1), E405–E414. doi: 10.1152/ajpendo.1997.272.3.E405
Sperling, M. R., Greenspan, A., Cramer, J. A., Kwan, P., Kälviäinen, R., Halford, J. J., et al. (2010). Carisbamate as adjunctive treatment of partial onset seizures in adults in two randomized, placebo-controlled trials. Epilepsia 51, 333–343. doi: 10.1111/j.1528-1167.2009.02318.x
Steriade, C., French, J., and Devinsky, O. (2020). Epilepsy: key experimental therapeutics in early clinical development. Expert Opin. Investig. Drugs 29, 373–383. doi: 10.1080/13543784.2020.1743678
Stojilkovic, S. S., Tabak, J., and Bertram, R. (2010). Ion channels and signaling in pituitary gland. Endocr. Rev. 31, 845–915. doi: 10.1210/er.2010-0005
Strzelczyk, A., and Schubert-Bast, S. (2021). Expanding the treatment landscape for Lennox-Gastaut syndrome: current and future strategies. CNS Drugs 35, 61–83. doi: 10.1007/s40263-020-00784-8
Taddese, A., and Bean, B. P. (2002). Subthreshold sodium current from rapidly inactivating sodium channels drives spontaneous firing of tuberomammillary neurons. Neuron 33, 587–600. doi: 10.1016/s0896-6273(02)00574-3
Vohora, D., Saraogi, P., Yazdani, M. A., Bhowmik, M., Khanam, R., and Pillai, K. K. (2010). Recent advances in adjunctive therapy for epilepsy: focus on sodium channel blockers as third-generation antiepileptic drugs. Drugs Today 46, 265–277. doi: 10.1358/dot.2010.46.4.1445795
Whalley, B. J., Strephens, G. J., and Constanti, A. (2009). Investigation of the effects of the novel anticonvulsant compound carisbamate (RWJ-333369) on rat piriform cortical neurons in vitro. Br. J. Pharmacol. 156, 994–1008. doi: 10.1111/j.1476-5381.2008.00110.x
Wu, P. M., Cho, H. Y., Chiang, C. W., Chuang, T. H., Wu, S. N., and Tu, Y. F. (2022a). Characterization in inhibitory effectiveness of carbamazepine in voltage-gated Na+ and erg-mediated K+ currents in a mouse neural crest-derived (neuro-2a) cell line. Int. J. Mol. Sci. 23:7892. doi: 10.3390/ijms23147892
Wu, S. N., Chern, J. H., Shen, S., Chen, H. H., Hsu, Y. T., Lee, C. C., et al. (2017). Stimulatory actions of a novel thiourea derivative on large-conductance, calcium-activated potassium channels. J. Cell. Physiol. 232, 3409–3421. doi: 10.1002/jcp.25788
Wu, S. N., Chiang, H. T., Shen, A. Y., and Lo, Y. K. (2003). Differential effects of quercetin, a natural polyphenolic flavonoid, on L-type calcium current in pituitary tumor (GH3) cells and neuronal NG108-15 cells. J. Cell. Physiol. 195, 298–308. doi: 10.1002/jcp.10244
Wu, S. N., and Huang, C. W. (2021). Editorial to the special issue “electrophysiology”. Int. J. Mol. Sci. 22:2956. doi: 10.3390/ijms22062956
Wu, S. N., Wu, C. L., Cho, H. Y., and Chiang, C. W. (2022b). Effective perturbations by small-molecule modulators on voltage-dependent hysteresis of transmembrane ionic currents. Int. J. Mol. Sci. 23:9453. doi: 10.3390/ijms23169453
Wu, S.-Z., Ye, H., Yang, Y.-G., Lu, Z.-L., Qu, Q., and Qu, J. (2018). Case-control pharmacologenetic study of HCN1/HCN2 variants and genetic generalized epilespsies. Clin. Exp. Pharmacol. Physiol. 45, 226–233. doi: 10.1111/1440-1681.12877
Yongning, Z., Xianguang, L., Hengling, C., Su, C., Fang, L., and Chenghong, L. (2021). The hyperpolarization-activated cyclic nucleotide-gated channel currents contribute to oxaliplatin-induced hyperexcitability of DRG neurons. Somatosens. Mot. Res. 38, 11–19. doi: 10.1080/08990220.2020.1834376
Keywords: carisbamate (RWJ-333369), voltage-gated Na+ current, late Na+ current, window Na+ current, hyperpolarization-activated cation current, current kinetics, antiepileptic drug
Citation: Hung T-Y, Wu S-N and Huang C-W (2023) Concerted suppressive effects of carisbamate, an anti-epileptic alkyl-carbamate drug, on voltage-gated Na+ and hyperpolarization-activated cation currents. Front. Cell. Neurosci. 17:1159067. doi: 10.3389/fncel.2023.1159067
Received: 07 February 2023; Accepted: 02 May 2023;
Published: 24 May 2023.
Edited by:
Maria Novella Romanelli, University of Florence, ItalyReviewed by:
Elisabetta Coppi, University of Florence, ItalyCopyright © 2023 Hung, Wu and Huang. This is an open-access article distributed under the terms of the Creative Commons Attribution License (CC BY). The use, distribution or reproduction in other forums is permitted, provided the original author(s) and the copyright owner(s) are credited and that the original publication in this journal is cited, in accordance with accepted academic practice. No use, distribution or reproduction is permitted which does not comply with these terms.
*Correspondence: Sheng-Nan Wu, c253dUBtYWlsLm5ja3UuZWR1LnR3; Chin-Wei Huang, aHVhbmdjd0BtYWlsLm5ja3UuZWR1LnR3
Disclaimer: All claims expressed in this article are solely those of the authors and do not necessarily represent those of their affiliated organizations, or those of the publisher, the editors and the reviewers. Any product that may be evaluated in this article or claim that may be made by its manufacturer is not guaranteed or endorsed by the publisher.
Research integrity at Frontiers
Learn more about the work of our research integrity team to safeguard the quality of each article we publish.