- Research Group Olfactory Coding, Max Planck Institute for Chemical Ecology, Jena, Germany
It is long known that the nervous system of vertebrates can be shaped by internal and external factors. On the other hand, the nervous system of insects was long assumed to be stereotypic, although evidence for plasticity effects accumulated for several decades. To cover the topic comprehensively, this review recapitulates the establishment of the term “plasticity” in neuroscience and introduces its original meaning. We describe the basic composition of the insect olfactory system using Drosophila melanogaster as a representative example and outline experience-dependent plasticity effects observed in this part of the brain in a variety of insects, including hymenopterans, lepidopterans, locusts, and flies. In particular, we highlight recent advances in the study of experience-dependent plasticity effects in the olfactory system of D. melanogaster, as it is the most accessible olfactory system of all insect species due to the genetic tools available. The partly contradictory results demonstrate that morphological, physiological and behavioral changes in response to long-term olfactory stimulation are more complex than previously thought. Different molecular mechanisms leading to these changes were unveiled in the past and are likely responsible for this complexity. We discuss common problems in the study of experience-dependent plasticity, ways to overcome them, and future directions in this area of research. In addition, we critically examine the transferability of laboratory data to natural systems to address the topic as holistically as possible. As a mechanism that allows organisms to adapt to new environmental conditions, experience-dependent plasticity contributes to an animal’s resilience and is therefore a crucial topic for future research, especially in an era of rapid environmental changes.
1. Introduction
The word “plasticity” is exceptionally popular nowadays and is used to describe various phenomena in different branches of science, particularly in neuroscience. Searching for this term in scientific texts yields about 3 million hits, a similar number to other commonly used words such as “neuron” or “Drosophila,” which yield 3.3 million and 2 million hits, respectively, and it generates even half as many hits as “climate” (via Google Scholar, as of 03.11.2022). Although the term is used so frequently, it is inadequately defined, probably largely due to ignorance of its original meaning.
In the 1860s, at the beginning of the industrial revolution, the concept of plasticity originated in physics and material sciences (Tresca, 1864). At this time, a hypothesis about a peculiar property of metals was published by Henri Tresca. According to his hypothesis, metals enter a flowing state when an applied force exceeds a certain threshold. This work formed the basis for our current comprehension of the term “plasticity” in the physical sense, according to which solid bodies deform in response to an applied force and retain this state even after the force is removed (Bruhns, 2018).
It only needed a few decades for this term to find its way into biology. William James used the term for the first time in a surprisingly modern way (Berlucchi and Buchtel, 2008) to link the morphological plasticity of organisms to their behaviors (James, 1890). He suggested that the observed behaviors of organisms depend on the plasticity of the organic matter that constitutes the organisms. He already emphasized that structural plasticity needs not necessarily to involve only the visible morphology of an organism, but can also take place at the molecular level. Moreover, James highlighted nervous tissue as an organic matter with an exceptional amount of plasticity. It was his adoption of the term “plasticity” that bridged the gap between biology and physics and that later became accepted in neuroscience.
Demoor (1896), Ramón y Cajal (1894), Lugaro (1906, 1909), and Minea (1909) were the first neuroscientists to demonstrably use the term “plasticity” (DeFelipe, 2006; Mateos-Aparicio and Rodríguez-Moreno, 2019). However, it took several decades for the term to become as popular as it is today. Shortly after 1970, the terms “plasticity” and “neuroplasticity” were used by an increasing number of neuroscientists. As early as 1976, it was noted that the term was being used more and more broadly without being properly defined (Paillard, 1976). Paillard proposed that plasticity describes the ability of a system to acquire new functions by transforming its internal connectivity or by changing the elements of which it consists in response to the internal or external environment (Berlucchi and Buchtel, 2008; Will et al., 2008). According to this definition, a phenomenon can be called plastic only if it combines a morphological and functional change. In addition, the changes must be lasting, even if the event that triggers the effect is only temporary. If morphological and functional changes are reversible, the term “elasticity” would be more appropriate. Since Paillard’s views were published, the term’s popularity has only grown, and has been paralleled by an intensified decline in the accuracy of its use (Jones, 2000; Will et al., 2008; Groh and Meinertzhagen, 2010), which today reached a state where “plasticity” is often just a synonym for “change” or “difference.”
Morphological and functional changes were observed in various parts of the nervous system of insects, including motor neurons and the visual system (Sugie et al., 2018). In this review, we focus on morphological and physiological changes in the insect olfactory system in response to environmental stimuli and on associated behavioral changes. To this end, we first present the composition of the olfactory circuitry of Drosophila melanogaster Meigen, 1830 as an example of a well-studied olfactory system and then show how plasticity affects this system in different insect species, emphasizing on recent discoveries in D. melanogaster. Various molecular mechanisms that give rise to plasticity effects are highlighted. We address challenges in this research area and suggest how they can be overcome. Furthermore, the transferability of plasticity effects observed in the laboratory to more natural conditions is discussed. Finally, we argue that neuronal plasticity may be a rapid and efficient way to enable organisms to adapt to new environmental conditions, a capability that is of paramount importance in the face of environmental degradation and climate change. With this review, we aim to shed light on this area of research by providing the most comprehensive overview to date on experience-dependent plasticity in the olfactory system of insects.
2. The olfactory system of Drosophila melanogaster
2.1. Olfactory organs, receptors, and sensory neurons
The olfactory system of D. melanogaster is one of the experimentally most accessible parts of any insect brain and therefore an ideal model system for the investigation of plasticity effects. Like other insects, the vinegar fly detects odorants with its antennae, more specifically the third antennal segment (the funiculus), and the maxillary palps (Figure 1; Hansson and Stensmyr, 2011). The cuticular surface of these structures is covered with various types of sensilla, some of which have an olfactory function while others are involved in the perception of non-olfactory cues. There are about 410–460 olfactory sensilla on the funiculus and 60 on the maxillary palp with slight differences between sexes. The olfactory sensilla can be morphologically categorized into four groups: club-shaped basiconic, spine-shaped trichoid, cone-shaped coeloconic, and morphologically less well-defined intermediate sensilla, which combine characteristics of basiconic and trichoid sensilla (Figure 2; Stocker, 1994; Shanbhag et al., 1999; Laissue and Vosshall, 2008; Galizia and Sachse, 2010). These different types are distributed in a stereotypic manner across the funicular surface. The cuticular surface of olfactory sensilla is covered with small pores, which allow volatile compounds to enter (Steinbrecht, 1997), but prevent the sensillar lymph from exiting. The transition from air to an aqueous medium poses a problem for volatile compounds, especially if they are hydrophobic. Odorant-binding proteins (OBPs) are present in high concentration in the aqueous sensillar lymph of sensilla and are thought to facilitate the transition, to transport compounds to the receptors of olfactory sensory neurons (OSNs) (Steinbrecht, 1998; Pelosi et al., 2006; Leal, 2013) and/or to contribute in the activation of receptors by forming a complex with the odorant (Laughlin et al., 2008). OBPs are also hypothesized to play a role in clearance of compounds from receptors to terminate responses (Vogt and Riddiford, 1981; Ziegelberger, 1995; Scheuermann and Smith, 2019). The chemoreceptors are anchored in the dendritic membrane of OSNs (Benton, 2006). OSNs express olfactory (ORs), gustatory (GRs), or ionotropic (IRs) receptors (Benton, 2006; Joseph and Carlson, 2015). Each sensillum houses dendrites of 1–4 OSNs in defined combinations (de Bruyne et al., 2001). Each OSN type expresses one or only very few chemoreceptors each (Fishilevich and Vosshall, 2005; Martin et al., 2013). The somata of OSNs are located directly at the bases of the sensilla. Additionally to OSN dendrites, sensilla also contain a thecogen cell, a trichogen cell and one or two tormogen cells (Shanbhag et al., 2000), which ensheath the OSNs and produce the sensillar lymph and OBPs (Park et al., 2000; Shanbhag et al., 2001; Larter et al., 2016; Gomez-Diaz et al., 2018).
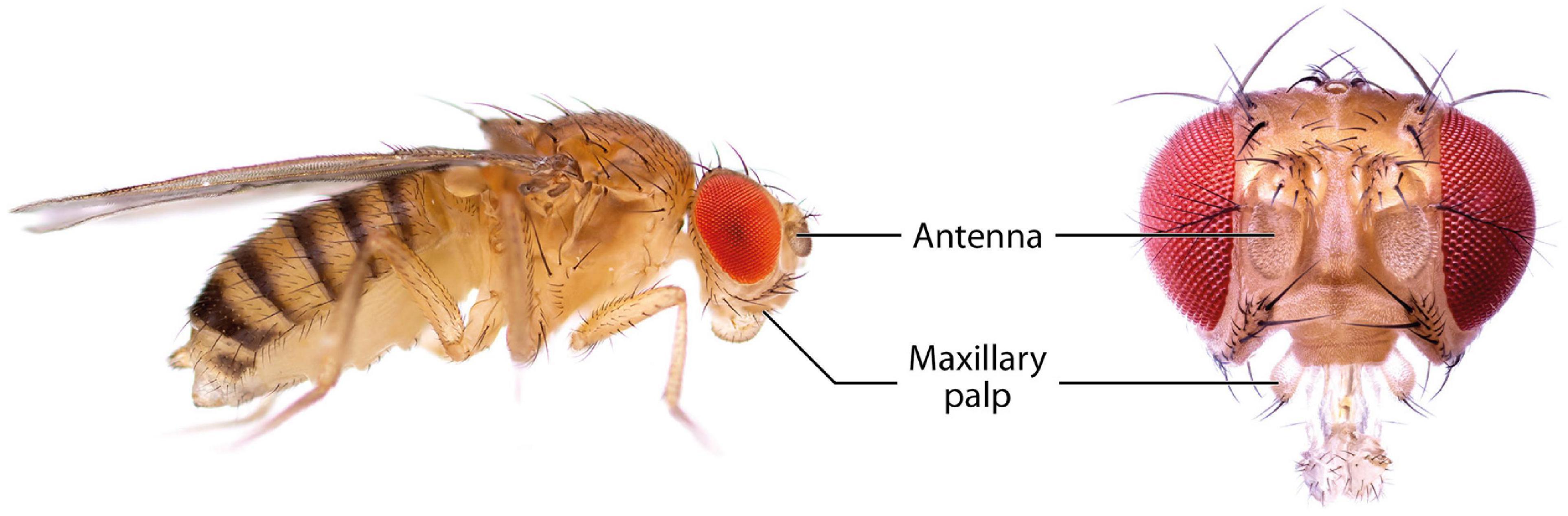
Figure 1. Photographs of D. melanogaster showing the main olfactory organs, the antennae and the maxillary palps.
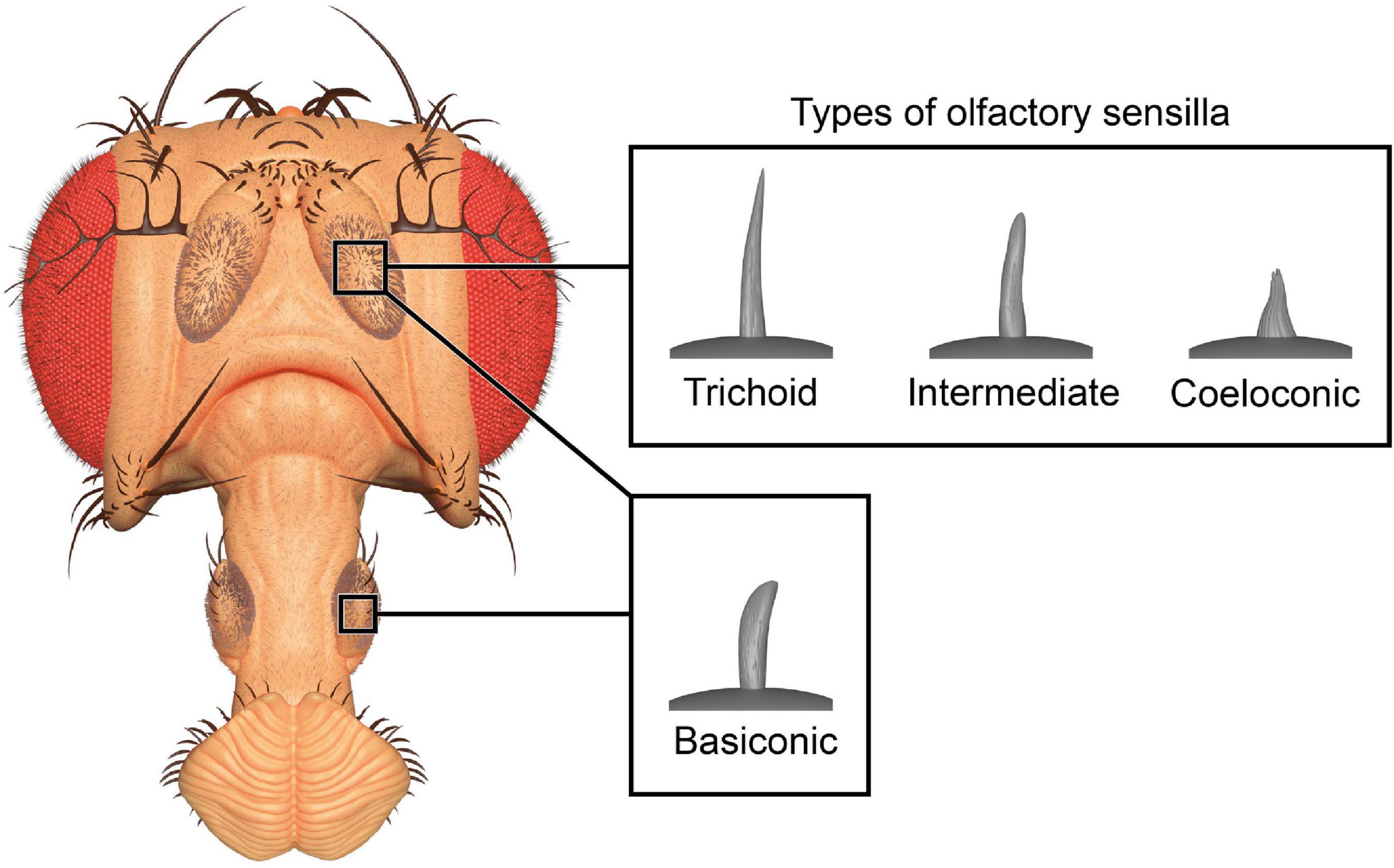
Figure 2. Three-dimensional render of olfactory sensilla types. Club-shaped basiconic sensilla are found on antennae and maxillary palps, while hair-like trichoid, cone-shaped coeloconic, and intermediate sensilla are only found on the antennae. The 3D model of the head is based on photographs and the models of sensilla are based on published micrographs (de Bruyne et al., 2001; Yao et al., 2005).
2.2. The first processing center – The antennal lobe
The first-order neurons in the olfactory system are the OSNs. OSNs that innervate the olfactory sensilla on the antenna and maxillary palps extend their axons to the first processing center, the antennal lobe (AL) (Figure 3), via the antennal nerve and labiomaxillary nerve, respectively (de Bruyne et al., 1999). These nerves bundle axons of ∼1,200 antennal and ∼120 maxillary OSNs (Singh and Nayak, 1985; Stocker et al., 1990). The deutocerebral AL forms a spherical structure in each brain hemisphere that is connected with the contralateral side via the antennal commissure (Stocker et al., 1983). Each AL consists of ∼54–58 small, mostly more or less spherical structures, the so-called glomeruli (Grabe et al., 2015; Schlegel et al., 2021). OSNs that express the same receptor type and which therefore are functionally equal were thought to converge in the same glomerulus (Gao et al., 2000; Vosshall et al., 2000). However, this simplistic model of segregated innervation was recently challenged by a study that showed evidence for a glomerular convergence of OSNs that co-express different receptor families (Task et al., 2022). Additionally, this observation was also made in the mosquito species Aedes aegypti (Linnaeus, 1762), indicating that the insect olfactory system might be more complex than was previously thought (Herre et al., 2022). Most OSN types innervate the same glomerulus on the ipsi- and contralateral brain hemisphere (Stocker et al., 1990; Vosshall et al., 2000; Scott et al., 2001). The size, form and location of each glomerulus are characteristic and form a topographic map of the AL that can be used to easily identify the same glomeruli across different individuals (Laissue et al., 1999; Couto et al., 2005; Fishilevich and Vosshall, 2005; Grabe et al., 2015). The relatively well established morphological segregation of glomeruli in the fly’s AL is accomplished by glia cells, which do not just wrap around the entire AL and around individual glomeruli, but also extend processes into glomeruli to ensheath large neuronal processes or bundles of smaller processes (Oland et al., 2008; Zwarts et al., 2015; Kremer et al., 2017).
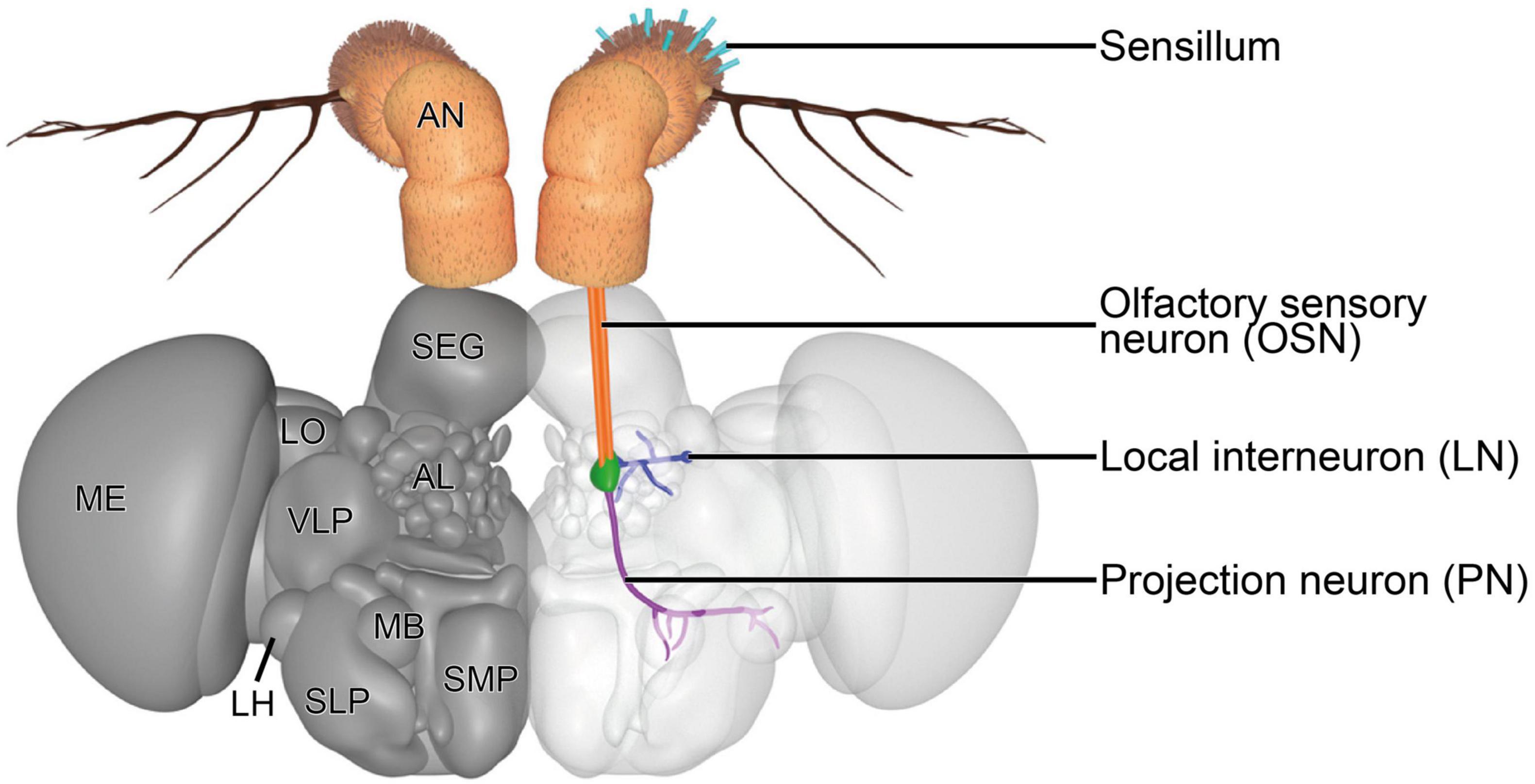
Figure 3. Three-dimensional render of the brain of D. melanogaster. A representative olfactory circuit is shown. It consists of OSNs (orange) that innervate a specific type of sensilla on the antenna (teal) with their dendrites and a specific glomerulus (green) with their axons. In the glomerulus, OSN axons synapse onto PNs (violet) and LNs (blue). PNs extend their axons to the MB and LH. LNs usually innervate a diverse set of glomeruli to contact OSNs, PNs, and other LNs, mainly to modulate signal transduction. AL, antennal lobe; AN, antenna; LH, lateral horn; LN, local interneuron; LO, lobula; MB, mushroom body; ME, medulla; OSN, olfactory sensory neuron; PN, projection neuron; SEG, subesophageal ganglion; SLP, superior lateral protocerebrum; SMP, superior medial protocerebrum; VLP, ventrolateral protocerebrum. The 3D model is based on published figures (Jenett et al., 2012; Scheffer et al., 2020) and the brain model of NeuroNLP FlyCircuits (Ukani et al., 2016).
Within the glomeruli, OSNs, projection neurons (PNs) and local interneurons (LNs) are the three main neuronal types that form a complex network by synapsing onto each other, among each other and in rare cases also onto themselves (autapses) (Rybak et al., 2016; Horne et al., 2018; Scheffer et al., 2020). OSN axons mainly form synaptic contacts with second-order PNs and LNs. The somata of PNs and LNs are located outside of the AL adjacent to its boundaries in the anterodorsal, lateral, and ventral periphery. These three clusters were previously thought to harbor in total ∼150 PN somata (Jefferis et al., 2001) and ∼200 LN somata (Chou et al., 2010; Seki et al., 2010) in a trophospongium formed by cortex glia cells (Zwarts et al., 2015). A recent and detailed connectome study suggests that there are ∼340 PNs that innervate the AL and supports the previously postulated LN number (Schlegel et al., 2021). LNs are a very diverse group of neurons, which extend a process into the center of the AL and branch from there to innervate different glomeruli. Several types of LNs can be distinguished according to their innervation patterns. There are globally innervating LNs that innervate all or almost all glomeruli, locally innervating LNs that innervate different glomeruli in a specific region of the AL, patchy innervating LNs that innervate approximately half of all glomeruli broadly distributed across the whole AL and LNs that only innervate very few glomeruli. Additionally, there are some LNs that also innervate glomeruli in the contralateral half of the AL and that differ in the innervation density within glomeruli (Chou et al., 2010; Seki et al., 2010). LNs form synapses with OSNs, PNs and other LNs. They are as physiologically diverse as they are morphologically, which is also reflected in their neurotransmitter repertoire. LNs release neuropeptides, glutamate, gamma-aminobutyric acid (GABA) and acetylcholine to either inhibit or excite their postsynaptic partners (Ng et al., 2002; Wilson and Laurent, 2005; Olsen et al., 2007; Shang et al., 2007; Olsen and Wilson, 2008; Root et al., 2008; Ignell et al., 2009; Chou et al., 2010; Seki et al., 2010; Das et al., 2011; Liu and Wilson, 2013). There also seems to be a high variability in LN wiring between different flies (Chou et al., 2010), which adds to the complexity of these neurons. Furthermore, some LNs release a combination of these neurotransmitters or form electrical synapses with PNs (Huang et al., 2010; Yaksi and Wilson, 2010). Their morphological and physiological complexity indicates that they fulfill a diverse set of functions that are still largely unknown. Functions that have previously been demonstrated for LNs include gain control by lateral inhibition (Wilson and Laurent, 2005; Olsen and Wilson, 2008), synergistic mixture interaction mediated by lateral excitation (Das et al., 2017) and a selective inhibitory cross-talk between specific glomeruli (Mohamed et al., 2019), which cause a modulation of the incoming signals from the periphery of the olfactory system.
Projection neurons receive the modulated signal and transfer it to the mushroom body (MB) and the lateral horn (LH) in the protocerebrum via three different tracts, the medial AL tract (mALT), mediolateral AL tract (mlALT), and lateral AL tract (lALT) (Stocker et al., 1990). Two groups of PNs can be distinguished according to their morphology. The first group consists of PNs that are excitatory and uniglomerular, meaning that they innervate only one glomerulus (uPNs). These acetylcholine expressing uPNs (Yasuyama and Salvaterra, 1999) have their somata in the anterodorsal and lateral clusters and extend their axons along the mALT or lALT to either the MB calyx and the LH or only the LH (Tanaka et al., 2012). The second group consists of PNs that are inhibitory and innervate multiple glomeruli (mPNs). The cell bodies of these GABA releasing mPNs are located in the ventral cluster (Lai et al., 2008). The mPNs extend their axons along the mlALT and bypass the MB to innervate the LH directly (Jefferis et al., 2007; Okada et al., 2009; Tanaka et al., 2012).
2.3. The higher brain centers – The mushroom body and lateral horn
The MB and LH harbor third-order neurons, which receive input from the AL via axonal terminals of PNs (Figure 3). The MB is a neuropil that is involved in higher cognitive tasks, such as olfactory learning and memory (Dubnau and Tully, 2001; Heisenberg, 2003; Davis, 2005; Fiala, 2007; Busto et al., 2010), context-dependent odor evaluation (Bräcker et al., 2013) and integration of different sensory modalities, such as olfaction (Heisenberg et al., 1985; Stocker et al., 1990), vision (Barth and Heisenberg, 1997; Vogt et al., 2016; Yagi et al., 2016; Sun et al., 2020), gustation (Kirkhart and Scott, 2015), thermo- (Frank et al., 2015), and hygrosensation (Frank et al., 2017). The input region of the MB is its calyx. The innervating PN axons form boutons that are contacted by dendrites of ∼2,500 MB intrinsic neurons, so-called Kenyon cells (KCs) (Technau and Heisenberg, 1982). KCs form characteristic claw-like structures at dendritic terminals. Each of these “claws” synapses onto one bouton, but each bouton is contacted by several KC “claws,” which forms a so-called microglomerulus (Yasuyama et al., 2002; Leiss et al., 2009; Butcher et al., 2012). This way, a given KC receives input from multiple PNs and each PN gives output to multiple KCs, creating a complex network of synaptic connectivity in the MB calyx (Tanaka et al., 2004; Lin et al., 2007; Murthy et al., 2008; Caron et al., 2013; Gruntman and Turner, 2013). KC axons form a dense tract, the pedunculus, which splits up terminally into three lobe-like structures. In the MB lobes, KCs synapse onto relatively few MB output neurons, the axons of which extend deeper into the brain (Tanaka et al., 2008; Strausfeld et al., 2009; Séjourné et al., 2011). Olfactory learning has the potential to affect the number of microglomeruli that respond to the learned odor, rendering the MB a dynamic neuropil that rearranges itself depending on prior experience (Baltruschat et al., 2021).
The LH is the second protocerebral region that receives input from PNs. It is a neuropil involved in innate behaviors that were thought to be independent of the influence of the MB (Wang et al., 2003; Min et al., 2013). Axonal terminals of excitatory PNs branch in a typical pattern. This innervation pattern is very similar, but not identical, between sister PNs that innervate the same glomerulus in a given fly but also between different animals (Marin et al., 2002; Wong et al., 2002; Jefferis et al., 2007). Additionally, PN morphology in the LH seems to be independent of sensory input, since PN axons develop before their dendrites establish a functional connectivity with OSNs and they develop normally even when sensory input is abolished by amputation of antennae and maxillary palps (Wong et al., 2002; Jefferis et al., 2004; Jefferis and Hummel, 2006). The stereotypy of PN axons allows dividing the LH into functional regions, which receive qualitatively different olfactory input (Jefferis et al., 2007; Strutz et al., 2014; Seki et al., 2017; Das Chakraborty and Sachse, 2021). Interestingly, there seems to be a tendency that axons of different PN classes, which innervate different glomeruli but transmit information with a similar valence, form a relatively high number of axo-axonic connections within the LH (Bates et al., 2020; Huoviala et al., 2020). However, higher brain centers such as the MB and LH do not only receive input from the AL, they also give feedback via centrifugal neurons (Stocker et al., 1990; Tanaka et al., 2012). This clear functional distinction between the MB as the learning and memory center and the LH as the center that is responsible for innate behavior was challenged in recent years. There is evidence that also the LH is involved in memory tasks (Dolan et al., 2018) and that the MB affects innate behavior (Bräcker et al., 2013; Lin et al., 2014; Lewis et al., 2015).
Olfactory sensory neurons that express a certain receptor and therefore converge onto the same glomerulus, and their associated PNs that also only innervate the same glomerulus, synapse onto these OSNs and extend their axons into the MB and LH, is what neuroscientists often refer to as a neuronal circuit. A given odorant can bind to several different receptors and activate different circuits at the same time. Furthermore, an odorant plume can consist of different odorant molecules that are also very likely to activate different circuits. The activity of different circuits creates an elaborate spatiotemporal map of odorant responses across the AL glomeruli, the so-called combinatorial code (Galizia et al., 1999; Sachse et al., 1999; Vosshall et al., 2000). Even though the amount of different circuits is limited in the fly’s brain, the amount of different odors that can be encoded this way is seemingly endless. It is the task of higher brain centers to decode this information so that the fly can behave adequately.
The connectivity of neurons that are further downstream of the MB and LH is much more complex than the connectivity of first- and second-order neurons in the olfactory system. It is therefore more difficult to define specific olfactory neurons downstream of the MB and the LH. The increasing integration of different sensory modalities in higher brain areas further adds to the complexity (Thiagarajan and Sachse, 2022). In recent years, however, technological advances allowed the acquisition of large-scale connectome data and detailed 3D reconstructions of neurons and their synaptic connections, specific circuits and even entire neuropils (Horne et al., 2018; Zheng et al., 2018; Dolan et al., 2019; Huoviala et al., 2020; Li et al., 2020; Marin et al., 2020; Scheffer et al., 2020; Schlegel et al., 2021). Such large-scale studies are necessary to understand how olfactory information is processed in higher brain areas and how it is transmitted across the brain to finally reach neuromuscular junctions that translate the information into motor activity.
3. Experience-dependent plasticity in the olfactory system of insects (excluding Drosophila)
Not surprisingly, much of our understanding of experience-dependent plasticity in insect brains is based on the honey bee olfactory system, as it is one of the most extensively studied insect olfactory systems. Early studies demonstrated that the behavioral changes that take place during the transition from hive workers to foragers were accompanied by structural changes in the brain, particularly volume changes of the AL, its glomeruli, and the MB (Withers et al., 1993; Durst et al., 1994; Winnington et al., 1996). Since this behavioral transition depends on the age of the bees, the morphological changes could be predetermined and not dependent on experience. Fahrbach et al. (1998) showed that the volume of MBs of isolated bees reared in darkness increased with age, supporting the age-dependency. However, foraging intensity was shown to be positively correlated with the volume of the lip and collar region of the MB and with the number of boutons in the lip region (Cabirol et al., 2018). The number of boutons in the lip region was further affected by the complexity of the environment in which the bees were reared (Cabirol et al., 2017). These morphological changes were shown to affect learning performance. Alterations in the social environment (Maleszka et al., 2009) and hive temperature (Groh et al., 2004, 2006) are other factors that influenced the morphological development of the MB, suggesting that its architecture and functionality depend not only on age but also experience. However, the MB is not the only brain region in honey bees that is shaped by experience-dependent plasticity. When young hive workers were artificially converted to precocious foragers, the whole AL and specific glomeruli enlarged, and the animals performed better in associative learning tasks of floral scents in comparison to hive workers of the same age (Sigg et al., 1997; Brown et al., 2004). Glomeruli stimulated to grow by this procedure harbored a greater number of synapses. In addition, several studies investigated the effects of long-term exposure to odorants on the AL functionality and morphology. Honey bees exposed to an odorant while having access to sucrose solution showed an odorant-specific increase in glomerular volume. The affected glomeruli also responded more strongly to the specific odorant, leading to increased appetitive behavior in proboscis extension response experiments. The behavioral and physiological changes were observed not only when honey bees were tested with the odor to which they were exposed, but also when they were tested with perceptually similar odorants (Arenas et al., 2009, 2012). Nevertheless, there is evidence that not all glomeruli in the AL are affected equally by odorant exposure and that the exposure procedure may have an influence. Andrione et al. (2017) found that long-term exposure to odorants caused glomeruli to shrink, and Hourcade et al. (2009) showed that Pavlovian conditioning of an odorant caused an odorant-specific enlargement of glomeruli, whereas their activity was unaffected.
In other eusocial hymenopterans, it was shown that experience can also modulate the morphology of the MB. These modulations were mostly attributed to social experience, which may involve olfactory stimulation. For example, Pseudomyrmex spinicola (Emery, 1890) is an acacia ant species with division of labor that is not associated with external morphological differences between individuals performing different tasks. There are individuals that forage mainly on leaves (“leaf-ants”) and individuals that stay mainly on tree trunks and defend the colony (“trunk-ants”). This division of labor is not as pronounced in small colonies, where individual ants frequently switch between these tasks. However, as the colony grows, task specialization of ants increases, affecting brain morphology. The MB volume of trunk-ants repeatedly involved in defensive tasks decreases, whereas an opposite effect was observed in leaf-ants, suggesting that memory and learning may play a greater role in specialized leaf-ants (Amador-Vargas et al., 2015). In another ant species, Camponotus floridanus (Buckley, 1866), it was shown that the size of the MB is age- and experience-dependent, similar to honey bees. Ants involved in brood care and foraging had more sensory experience and larger MBs than “idle” individuals that remained in the nest without being active (Gronenberg et al., 1996). In the facultative eusocial sweat bee Megalopta genalis Meade-Waldo, 1916, it was shown that the behavioral switch to eusociality was associated with division of labor, which affected MB volume depending on the task an individual was performing (Smith et al., 2010; Jaumann et al., 2019). It is hypothesized that eusociality presents animals with additional cognitive demands, such as recognizing nest mates, a task that often involves odor perception (Lorenzi and d’Ettorre, 2020). It is therefore not surprising that neuronal plasticity was observed in eusocial hymenopterans. However, experience-dependent plasticity was also observed in solitary alkali bees (Nomia melanderi Cockerell, 1906). Alkali bees kept under deprived laboratory conditions had smaller mushroom bodies than nesting individuals that moved around a complex environment while foraging. In addition, in alkali bees kept in isolation, the region of the MB that receives olfactory input, the lip, was smaller than in individuals paired with another conspecific, suggesting that even in solitary bees, olfaction has the potential to modulate the olfactory system in a social context (Hagadorn et al., 2021). In another solitary bee, the orchard mason bee Osmia lignaria Say, 1837, foraging experience was observed to correlate with increased MB size, which was also independent of age (Withers et al., 2008).
The olfactory system of moths and butterflies was shown to be influenced by experience as well. When males of Spodoptera littoralis (Boisduval, 1833) were briefly exposed to the female sex pheromone, they were more attracted to the pheromone for more than 24 h after the exposure (Anderson et al., 2003, 2007). In addition, neurons involved in the detection of the pheromone became sensitized, presumably by upregulating the expression of pheromone-binding proteins (Guerrieri et al., 2012). Interestingly, the same behavioral and physiological effects could be elicited by exposing male moths to the sounds of an insectivorous bat, demonstrating a unique case of experience-dependent plasticity across different sensory modalities (Anton et al., 2011). A subsequent study showed that the exposure also affected the morphology of the AL and MB calyx. Both olfactory and auditory stimuli caused volumetric growth of pheromone processing glomeruli in the macroglomerular complex, whereas exposure to the bat sound also caused growth of another glomerulus that processes plant odors (Anton et al., 2016). The AL of Polygonia c-album (Linnaeus, 1758) increased in size when these butterflies were exposed to an odor-rich environment with which they could not physically interact. When olfactory input was eliminated unilaterally by covering one antenna in beeswax, enlargement of the ipsilateral AL was prevented, while the contralateral AL showed reduced growth (Eriksson et al., 2019). Another study investigating P. c-album demonstrated that MB volume was positively affected when butterflies were exposed to a rich odorant environment while having the opportunity to physically interact with odor sources. However, this treatment did not result in volumetric changes in the AL (van Dijk et al., 2017). Interestingly, two other butterfly species, Aglais io (Linnaeus, 1758) and Aglais urticae (Linnaeus, 1758), tested in the same study, were demonstrated not to have their MB affected by the enriched environment. Work on the butterfly Heliconius hecale (Fabricius, 1775) showed that even insectary-reared butterflies having access to their host plants and additional pollen sources had smaller mushroom bodies than wild-caught individuals (Montgomery et al., 2016). These results suggest that an odorant-rich environment by itself is not always sufficient to cause morphological changes in the AL or MB, or that an odorant environment that is complex for one species might be an impoverished environment for another.
Morphological differences between swarm-forming locusts in the solitary and gregarious phases are an example for polyphenism, a special form of plasticity. It is known from several locust species that the population density of nymphs and also adults can trigger a behavioral and morphological shift from the solitary to the gregarious phase when a certain threshold is reached (e.g., Simpson et al., 1999; Pocco et al., 2019). Interestingly, this phase change could also be triggered in non-swarming locust species with similar behavioral and morphological consequences (Gotham and Song, 2013). Morphological changes associated with the phase shift are not restricted to the outer appearance of the animals, but are also reflected in their nervous system. For example, gregarious desert locusts [Schistocerca gregaria (Forsskål, 1775)] have a proportionally bigger MB calyx but proportionally smaller ALs compared to solitary individuals. This observation may indicate a greater need for primary sensory processing in solitary locusts and a greater need for information integration in higher brain centers in gregarious individuals (Ott and Rogers, 2010). Some studies demonstrated that learning of aversive food odors differs between phases (reviewed in Simões et al., 2016), which may argue for a greater need for information integration in the gregarious phase. However, the experience-dependent plasticity observed in locusts is a special case of plasticity compared to previously described examples. The reason is that the gregarization can be initiated in the nymph. Therefore, the observed changes could be partially predetermined in development rather than purely being experience-dependent. Because both nymphs and imagines can gregarize, the behavioral and morphological changes observed in adult individuals may also depend on the time at which gregarization was initiated. The switch from the solitary to the gregarious phenotype can be initiated within a few hours (Ellis, 1963; Roessingh et al., 1993), whereas the switch from the gregarious to the solitary phenotype is a slow process that requires several days, nymphal stages or even generations to complete (Simpson et al., 1999). Moreover, the gregarious phenotype can be inherited to subsequent generations without the need for them to experience an environment crowded with conspecifics (Miller et al., 2008), suggesting that some predetermined factors are indeed involved in this unique form of plasticity.
4. Experience-dependent plasticity in the olfactory system of D. melanogaster
The olfactory system of D. melanogaster is an ideal model for the investigation of experience-dependent plasticity because of its relative simplicity and the genetic tools that are available. Its composition of strongly segregated circuits and the knowledge about odorant tuning profiles for most olfactory receptors allows specific stimulation and precise investigation of certain parts of the system. Two decades ago (Table 1), the first study that documented a case of experience-dependent plasticity in the olfactory system of D. melanogaster was conducted by Devaud et al. (2001). From this early study it is known that exposure of flies to odorants for four subsequent days can have an impact on the morphology of the AL. It was shown that the exposure to benzaldehyde or to isoamyl acetate caused a glomerulus-specific volumetric decrease (benzaldehyde: DM2 and V glomerulus; isoamyl acetate: DM6 glomerulus). The volumetric effect after benzaldehyde exposure was shown to be persistent for at least 7 days after the exposure ended and it was accompanied by a slight trend toward a decrease in the number of synapses in the affected glomeruli. Additionally, flies were less repelled by the odorant they were previously exposed to Devaud et al. (2001). A subsequent study from the same authors demonstrated that the experience-dependent plasticity effects can only be evoked when flies are exposed to odorants during early adult life (Devaud et al., 2003). Since these early studies were published, only a few other publications focused on this topic, some of which reported contradictory results on morphological, physiological and behavioral effects.
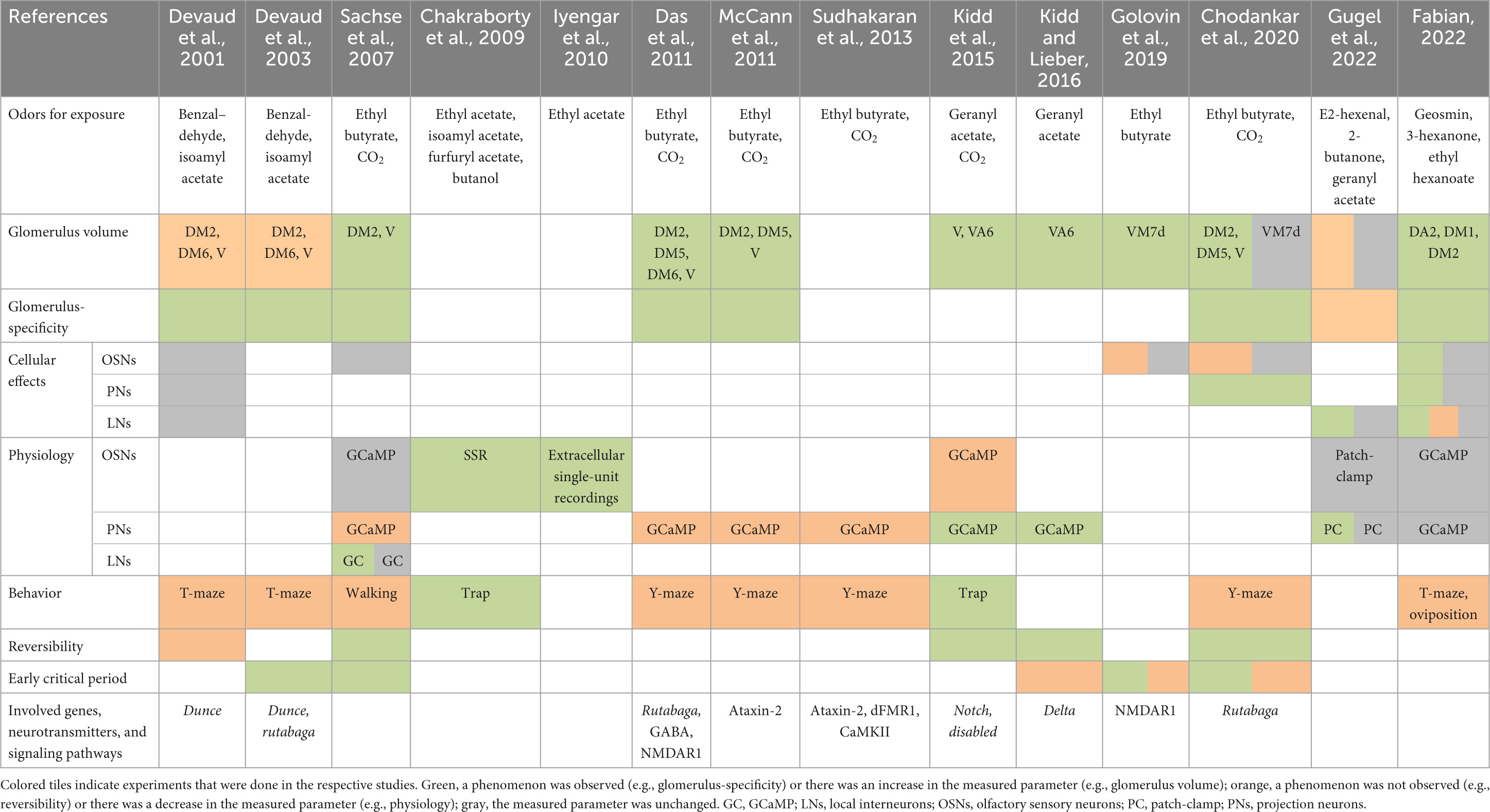
Table 1. Summary of the literature on experience-dependent plasticity in the olfactory system of Drosophila melanogaster using long-term odor exposure.
By focusing on other neuronal circuits, it was shown several years ago that the exposure to carbon dioxide or ethyl butyrate affects the associated V and DM2 glomerulus, respectively (Sachse et al., 2007). However, contrary to the mentioned previous studies, the glomeruli grew in size instead of shrinking due to the exposure. This finding was the first indicator that not every olfactory circuit is affected in the same way by the perception of odorants. Furthermore, it could be demonstrated that two subsequent days of odorant exposure are sufficient to induce the maximum volumetric effect in the V glomerulus. When flies were returned to ambient air for at least 2 days after the exposure period, the glomerulus size returned to normal. This observation of reversibility differs from the observations of Devaud et al. (2001). Nevertheless, the existence of an early critical period for the induction of experience-dependent plasticity effects was supported, as glomerular growth could not be induced when flies were exposed relatively late in their adult life (Sachse et al., 2007). Behavioral experiments demonstrated that carbon dioxide-exposed flies respond less to the odorant in walking assays, which is in line with Devaud’s findings as well. To elucidate the cellular processes that are involved in glomerulus volume changes, the OSNs that innervate the V glomerulus were investigated more precisely (Sachse et al., 2007). Quantifications of OSN cell somata in the antenna did not reveal any effects on OSN number due to odorant exposure. Moreover, detailed 3D reconstructions of OSN axonal terminals inside the V glomerulus and quantification of the number of their terminals, their length and volume showed that they were not affected by exposure to carbon dioxide either. These findings indicate that glomerular growth does not depend on changes in OSN number or morphology. Physiological experiments demonstrated that the signaling of OSNs in carbon dioxide-exposed flies was not changed. However, the output signaling of PNs in the LH was lower in exposed flies and this decrease could be explained by increased activity of inhibitory LNs (Sachse et al., 2007). Two follow-up studies by Das et al. (2011) and McCann et al. (2011) targeted the DM2, DM5, and V glomeruli and confirmed the morphological, physiological, and behavioral plasticity effects.
When flies are exposed to geranyl acetate, the associated VA6 glomerulus grows in size as well, which is an effect that was shown to be reversible (Kidd et al., 2015; Kidd and Lieber, 2016). The volumetric growth does not seem to rely on an early critical period, as it could also be evoked in flies that were exposed beginning on the eighth day after hatching from the pupa (Kidd and Lieber, 2016). Interestingly, exposure to geranyl acetate increases the attraction toward this odor in trap assays, which contradicts previous findings that showed that exposure to odors decreases the behavioral response toward them. The authors hypothesized that flies could form a positive association between the odorant and food during the exposure time, which could increase the attractiveness of the odor. Such a positive association might only occur when flies are exposed to neutral or attractive odorants such as geranyl acetate and not when they are exposed to repellent odors such as carbon dioxide or benzaldehyde. Additionally, exposure to geranyl acetate decreases responses of associated OSNs and increases PN responses (Kidd et al., 2015). Similarly, exposure to the neutral or attractive odors ethyl acetate, isoamyl acetate, furfuryl acetate or butanol (Knaden et al., 2012) was shown to cause an increased attraction toward the odor that was used during the exposure period (Chakraborty et al., 2009). This observation was correlated with an increased spike rate of different OSN classes. Moreover, OSNs of flies that were reared on a medium that contained ethyl acetate but was otherwise odorless showed an increased sensitivity to low ethyl acetate concentrations afterward (Iyengar et al., 2010).
Recent studies documented some additional interesting plasticity effects in the AL. Golovin et al. (2019), exposed flies to ethyl butyrate and investigated the consequences for the VM7d glomerulus (called “VM7” by the authors). The authors indicate to have observed a volumetric decrease of the VM7d glomerulus after flies were exposed to ethyl butyrate (Golovin et al., 2019; Figure 1). But what they actually measured according to the description of their methods was only GFP positive voxels of the OSN labeling, indicating that the OSNs retract their terminals from the area of the VM7d glomerulus. This finding is of particular interest because it is contradictory to the findings for OSNs of the V glomerulus, which were unchanged after flies were exposed to carbon dioxide (Sachse et al., 2007). The retraction of OSN axons was accompanied by a decrease of synaptic area and number of T-bars. Information about the actual glomerulus volume is lacking. Even though Golovin et al. (2019) emphasized on the presence of an early critical period for experience-dependent plasticity effects, they demonstrate that very high odorant concentrations can still induce plasticity effects relatively late in the adult life (7–9 days after flies hatched from the pupa). This observation may indicate that the ability to undergo plastic changes might be maintained throughout adulthood, or at least longer than previously thought, and that only the conditions to evoke them change. The reversibility of plasticity effects was also observed in the VM7d circuit, as was an unchanged number of OSNs, which is in line with previous observations (Sachse et al., 2007).
A subsequent study by Chodankar et al. (2020) followed up on Golovin et al. (2019). They support that the OSN axons that innervate the VM7d glomerulus (called “VM7” by the authors) seem to retract during long-term exposure to ethyl butyrate. The OSNs rapidly recover within 12 h when flies are supplied with ambient air after the exposure period. However, they demonstrate that the glomerular volume of VM7d is not affected by the exposure. In an experiment in which the authors exposed flies to ethyl butyrate or carbon dioxide and subsequently measured GFP positive pixels of PN labeling in associated glomeruli, they found that the PN dendrites increase in size in a glomerulus-specific manner. This finding reveals the growth of PN dendrites as one factor that contributes to the glomerular growth. Moreover, the authors support that plasticity effects in the VA6 glomerulus are independent of an early critical period, as this glomerulus also grows in size when flies are exposed to geranyl acetate relatively late in adulthood. Contrary to the VA6 glomerulus, the critical periods of the DM5 and V glomeruli close within 48 h after eclosion, after which the induction of glomerular growth is no longer possible (Chodankar et al., 2020).
For the publications mentioned so far, which dealt with experience-dependent plasticity in the olfactory system of D. melanogaster, very high odorant concentrations were used over an exposure period of usually four subsequent days (Golovin et al., 2019: only 2 days). In these studies, odorant supply was in most cases realized by a perforated centrifuge tube containing the odorant solution, which was placed inside a container that contained flies. Therefore, the odorant molecules were omnipresent throughout the exposure period, resulting in intense and unnatural stimulation of receptors (Gugel et al., 2022). At such high concentrations, the binding of odorant molecules to receptors is less specific (Hallem and Carlson, 2006; Hu et al., 2020), which could lead to combinatorial effects that are difficult to interpret and to detect as such. Moreover, due to these high concentrations and intense exposure procedures, observed exposure effects could be caused by excitotoxicity instead of experience-dependent plasticity. In order to prevent such unpredictable experimental influences, Gugel et al. (2022) used a pulsed exposure system with 1 s of odor at relatively low concentrations followed by 20 s ambient air. A similar pulsed exposure was already utilized previously (Sachse et al., 2007) but only for a single experiment. This exposure method allows flies to be exposed in a more subtle manner that also more closely resembles natural conditions, where a particular odor is usually not present all the time. Another positive side effect of a pulsed exposure system is that neuronal responses are less likely to adapt to the exposed odor (Gugel et al., 2022). Gugel and colleagues exposed flies to E2-hexenal, 2-butanone or geranyl acetate at concentrations that specifically stimulate OSNs that converge in the DL5, VM7d (called “VM7” by the authors), and VA6 glomerulus, respectively. With physiology experiments, they could demonstrate that OSN signaling is unaffected by the exposure procedure. However, the responses of DL5 and VM7d PNs to low odorant concentrations were increased when flies were exposed to either E2-hexenal or 2-butanone. The same effect was not observed in VA6 PNs when flies were exposed to geranyl acetate, demonstrating another odorant- or glomerulus-specific effect. Experiments showed that the excitability of PNs and the synaptic strength between OSNs and PNs does not explain the increased PN response for low odorant concentrations as both of these factors were unaffected by the exposure. Gugel et al. (2022) found that odor-evoked lateral excitation could be the reason for increased PN responses. Interesting observations in this study were glomerulus non-specific effects, which contradict previous publications that emphasized odor- and glomerulus-specificity of experience-dependent plasticity (Sachse et al., 2007; Das et al., 2011; Kidd et al., 2015; Kidd and Lieber, 2016; Chodankar et al., 2020). One of these non-specific effects was an increased spontaneous firing rate of VM7d PNs, although the flies were only exposed to E2-hexenal (which stimulates the DL5 glomerulus) and not 2-butanone. The exposure to E2-hexenal also caused a more prominent and prolonged hyperpolarization in VM7d PNs, which should not be affected by exposure to the odorant if the effects were purely glomerulus-specific. Morphological investigations revealed that exposure to E2-hexenal caused a variety of different glomeruli to be slightly smaller, an effect that was only significant when compared across all measured glomeruli. Additionally, the innervation density of NP3056 LNs in E2-hexenal-exposed flies was slightly higher in all measured glomeruli. This effect was also only significant when compared across different glomeruli. Those morphological effects were not observed when flies were exposed to 2-butanone.
In a recent study, we asked whether even a highly specific olfactory circuit, which is crucial for the survival of D. melanogaster because it conveys information about the presence of potentially toxic microorganisms (Stensmyr et al., 2012) is subject to plasticity as well (Fabian, 2022). For this, flies were exposed to geosmin in a pulsed way similarly to Gugel et al. (2022), so that flies perceived the odor for 1 min and subsequently ambient air for 5 min. As expected, the exposure caused a specific increase in volume of the geosmin-responsive DA2 glomerulus. We could reveal some of the first cellular effects that are associated with the glomerular growth. The dendrites of innervating PNs expanded in response to geosmin-exposure and clearly contributed to the volumetric growth of the glomerulus, which supports the observations of Chodankar et al. (2020). We also observed bigger PN somata in geosmin-exposed flies, possibly as a response to higher metabolic demands of larger dendrites. Single-cell photoactivation of different LN subpopulations revealed glomerulus-specific morphological changes at the level of boutons and terminals in globally innervating LNs, while patchy LNs were unaffected. These findings show that also LNs undergo major structural rearrangements in response to long-term odor exposure.
Notably, OSN axons and glia cell processes extending into the DA2 glomerulus were shown to not contribute to the glomerular growth (Fabian, 2022). However, the fission of mitochondria in OSNs innervating the DA2 glomerulus was the first evidence of morphological changes of subcellular structures caused by long-term odorant exposure. Despite structural rearrangements in PNs and LNs, there were no significant changes in the neuronal activity of OSNs and PNs innervating the DA2 glomerulus, which is in line with observations by Gugel et al. (2022). The observed reduced behavioral response to geosmin after flies were exposed to the odorant could be caused by physiological changes in higher brain centers (Gugel et al., 2022).
In contrast to work examining experience-dependent plasticity in the MB or LH in other insects, there are only few studies linking structural and functional changes in these higher olfactory brain centers of D. melanogaster. Disruption of signaling in PNs was shown to affect the physiology and morphology of microglomeruli in the MB (Kremer et al., 2010; Pech et al., 2015; Doll et al., 2017), suggesting that experience-dependent plasticity is also a feature of olfactory neuropils other than the AL. Similarly, silencing OSNs affects the morphology of axonal terminals of PNs in the MB (Figure 3 in Hayashi et al., 2022). However, not only the inability to experience odors evokes plasticity effects in the MB, but also the sensation of odorants during appetitive learning. It was shown that the formation of long-term appetitive memory for the pheromone 11-cis-vaccenyl acetate is associated with an increased number of functioning microglomeruli formed by PNs involved in the sensation of this compound (Baltruschat et al., 2021). Whether the LH is subject to experience-dependent plasticity is largely unknown. We performed the first experiments examining the influence of prolonged odor exposure on the branching patterns of PN axons in the LH and found that it remained unchanged (Fabian, 2022). Further studies are needed to uncover and understand experience-dependent plasticity effects in the MB and LH more broadly. These studies face the challenge of disentangling the influence of olfaction from other sensory modalities that converge in these neuropils.
5. Molecular underpinnings of experience-dependent plasticity in the antennal lobe of D. melanogaster
For many of the morphological, physiological and behavioral consequences of long-term exposure to odorants, molecular and genetic drivers were found (Table 1). Early studies discovered that flies with a mutation in the dunce gene (dnc1) do not exhibit changes in glomerular volumes or behavior in response to odorant exposure (Devaud et al., 2001, 2003). This gene encodes a phosphodiesterase that is responsible for the degradation of cyclic adenosine monophosphate (cAMP). Consequently, a mutation in this gene leads to increased levels of cAMP in the cell (Davis and Kiger, 1981; Scheunemann et al., 2018), which has the potential to detrimentally affect intracellular signaling (Lee, 2015). These findings indicate that the intracellular cAMP level seems to be responsible for glomerular volume changes and behavioral changes (Devaud et al., 2001). Similarly, glomerular volumes, neural physiology and behavior in rutabaga (rut2080) mutants are unaffected by long-term odor exposure (Devaud et al., 2003; Das et al., 2011). The rutabaga gene encodes a Ca2+/calmodulin-responsive adenylate cyclase, which is involved in the synthesis of cAMP (Livingstone et al., 1984; Levin et al., 1992). A mutation in this gene causes decreased levels of intracellular cAMP, further highlighting the importance of cAMP for experience-dependent plasticity effects. Das et al. (2011) could demonstrate that the activity of rutabaga is particularly important in NP1227-Gal4 (LN1) LNs for the formation of short- and long-term behavioral habituation caused by long-term odor exposure. Additionally, the enhanced branching of PN dendrites that coincides with glomerular growth during odorant exposure was inhibited in rut2080 mutants or when rutabaga was knocked down in NP1227-Gal4 LNs. The effect could be rescued in rut2080 mutants when functional rutabaga was specifically expressed in NP1227-Gal4 LNs (Chodankar et al., 2020). Das et al. (2011) found that artificial expression of an inhibitory form of the cAMP response element-binding protein (CREB) in NP1227-Gal4 LNs blocked experience-dependent behavioral and morphological changes as well, highlighting the importance of cAMP signaling for plasticity effects in the AL. Moreover, dFMR1 and Ataxin-2 (Atx2), RNA-binding proteins that have been found to be involved in the repression of translation by microRNAs, were shown to be important for the establishment of plasticity effects as well (McCann et al., 2011; Sudhakaran et al., 2013). For example, a knockdown of Atx2 in PNs abolishes morphological, behavioral and physiological effects that occur after long-term exposure of flies to carbon dioxide or ethyl butyrate.
Another gene that was reported to play a role in experience-dependent plasticity in the olfactory system of D. melanogaster is notch (Kidd et al., 2015; Kidd and Lieber, 2016). This gene encodes a transmembrane receptor protein (Wharton et al., 1985). It is involved in signal transduction between cells and fulfills various functions during developmental processes (e.g., Brückner et al., 2000; Ramain et al., 2001; Okajima et al., 2003; Wilkin et al., 2004). Knocking down notch in V or VA6 OSNs abrogates the glomerular growth that is induced by long-term exposure to carbon dioxide or geranyl acetate, respectively. The knockdown of notch in OSNs also abolishes the physiological effects of odorant exposure that the authors observed in OSNs and PNs. Furthermore, overexpression of the adaptor protein Disabled (Dab), which physically associates with transmembrane receptors, blocks the growth of the VA6 glomerulus in geranyl acetate-exposed flies (Kidd et al., 2015).
The gene delta encodes a ligand for the notch receptor (Rebay et al., 1991) and it is therefore not surprising that the manipulation of delta has an impact on odor-evoked plasticity effects as well. The increased neuronal response of VA6 PNs that were observed in geranyl acetate-exposed flies is abolished when delta is knocked down in these PNs (Kidd and Lieber, 2016). Surprisingly, this knockdown causes an amplified volumetric growth of the glomerulus in odor-exposed flies. These findings indicate that the relationship between physiological and morphological plasticity effects is not straightforward. The authors conclude that the notch pathway affects the glomerular plasticity by canonical and non-canonical mechanisms. Interestingly, the VA6 glomerulus still grows in size when vesicle release in the associated OSNs is blocked by tetanus toxin, indicating that the volume increase is independent of activation of downstream neurons. The authors hypothesize that the delta pathway could be activated by neuropeptide signals from OSNs to PNs. Moreover, the notch pathway was shown to be involved in the reversibility of the plasticity effect. When delta is knocked down, the volumetric increase of glomeruli that is caused by exposure to odorants is irreversible for 2 days after the exposure (Kidd and Lieber, 2016).
In the context of retracting OSN axons and the associated decrease of synaptic area and T-bars in the VM7d glomerulus, the Wnt signaling pathways were investigated in more detail (Golovin et al., 2019). Wnt signaling is involved in many processes, including the regulation of synaptic connections between neurons (Packard et al., 2002; Chiang et al., 2009; Korkut et al., 2009; Friedman et al., 2013; Kopke et al., 2017). Therefore, this kind of signaling could be involved in the morphological effects observed in this study after flies were exposed to ethyl butyrate. However, perturbations at different steps of the Wnt signaling pathway revealed that the morphological alterations are independent of this kind of signal transduction (Golovin et al., 2019).
Beside the experiments that aimed at different gene regulatory networks, some studies focused on synaptic transmitters in order to elucidate underlying mechanisms of experience-dependent plasticity. For example, Das et al. (2011) targeted the signaling between LNs and PNs. They demonstrated that a reduction of the synaptic output of GABAergic NP1227-Gal4 LNs inhibits the behavioral habituation and morphological effects of long-term odor exposure. In contrast, artificial activation of these LNs was sufficient to elicit the plasticity effects. The knockdown of GABAA receptors in PNs blocks the GABAergic signaling between LNs and PNs and likewise abolished behavioral but not morphological plasticity effects. Similarly, the inhibition of glutamate signaling from LNs to PNs by knockdown of the NMDAR1 receptor in PNs was shown to block the behavioral habituation and prevent the glomerular growth. These findings demonstrate that both glutamatergic and GABAergic signaling are involved in experience-dependent plasticity effects in the AL.
Golovin et al. (2019) knocked the GABAB receptor in VM7d OSNs down, which had no effect on the retraction of OSN axons that they observed after exposing flies to ethyl butyrate. However, when they knocked down the glutamatergic NMDAR1 receptor specifically in VM7d OSNs, they could not observe an experience-dependent effect on the OSN axons. Therefore, for experience-dependent plasticity effects, the glutamate signaling seems to play a role in PNs (Das et al., 2011) as well as OSNs (Golovin et al., 2019). Blocking the neurotransmission to downstream neurons by expressing tetanus toxin in VM7d OSNs, did not inhibit the retraction of their axons but even increased the effect that odorant exposure has on these neurons. Interestingly, when the authors abolished action potentials in VM7d OSNs by expressing the exogenous inward rectifying potassium channel 2.1 (Kir2.1) in these neurons, OSN axons did not just retract in odorant-exposed flies but also in flies that were only exposed to the vehicle control. The authors argue that neuronal activity does not just seem to be needed for the control of OSN refinements, but also for the initial innervation of the VM7d glomerulus (Golovin et al., 2019). A similar experiment by Chodankar et al. (2020) could show that the critical period for odor evoked plasticity is extended in flies in which OSNs were silenced with Kir2.1 for the first 2 days after eclosion.
All these genetic and molecular findings demonstrate that experience-dependent plasticity in the AL underlies various signaling pathways with complex interactions (reviewed in Golovin and Broadie, 2016). Moreover, the aforementioned molecular mechanisms are not only involved in the generation of experience-dependent plasticity effects, but also in other cellular processes, adding to the complexity. From the limited data available, it is impossible to conclude whether some of these molecular mechanisms occur in a general manner across different glomeruli and circuits. Future studies need to uncover glomerulus-specific and more general principles to understand how plasticity effects arise in different olfactory circuits of the AL.
6. Limiting factors for the investigation of experience-dependent plasticity effects
The investigation of long-term plasticity effects in the olfactory system of insects poses a challenge. We have to assume that some individuals are strongly affected by the exposure procedure while others might not be affected at all, without any evidence to indicate which of the exposed flies were not affected. For example, in our recent study, we observed that the standard deviation of the volume of the DA2 glomerulus was in most cases higher in the group of animals that perceived geosmin (Fabian, 2022) compared to the control group receiving the solvent control mineral oil, indicating an increased variability in the experimental group. This could, of course, conceal very subtle morphological effects. The difficulty of detecting activity-dependent effects in neuronal circuits within inter-animal variability is a serious issue that was also mentioned in a study on plasticity effects of MB input synapses (Kremer et al., 2010). Golovin et al. (2019) suggest that the onset of odor exposure very early, at the late pupal stage, leads to more robust phenotypes, which could lower inter-animal variability. This approach should be considered for future experience-dependent plasticity studies to increase the chances of detecting very subtle plasticity effects. In addition, the introduction of methods to study morphological parameters in the olfactory system before and after exposure would allow us to document subtle changes within individuals. But, of course, such experiments are challenging to perform and involve additional difficulties concerning the ethical treatment of animals.
The measurement of morphological characters only at specific time points during the development also poses a problem. Just because no morphological changes were observed in the neurites of neurons after the exposure period it does not necessarily mean that there were no changes at all. During development, OSNs axons, for example, frequently extend and retract their axons (Li et al., 2021). In the developing larval visual system, these extensions and retractions of neurite terminals were shown to occur at the same rate, so that the overall length did not change (Sheng et al., 2018), although intense dynamic processes were at work. However, such dynamic plasticity effects could only be detected in adult flies if glomerular growth could be observed in real time. Moreover, the glomerular growth was shown to be reversible in some olfactory circuits (Sachse et al., 2007; Das et al., 2011; Kidd et al., 2015), emphasizing the importance of the time point at which experiments are conducted. Kidd et al. (2015) suggested that differences in the exposure paradigm, e.g., the time window between the end of the exposure and the beginning of the experiment, could explain the discrepancy in the results of some studies. Morphological results show that at least PNs and LNs undergo extensive structural changes (Chodankar et al., 2020; Fabian, 2022) during the exposure period and that in one olfactory circuit also OSN axons were affected (Golovin et al., 2019; Chodankar et al., 2020). It is likely that synapses are not yet properly established in the grown glomerulus, which could lead to different responses at different time points after the end of the exposure period because the measurements are taken in the middle of an ongoing developmental process.
There is evidence that the observed volumetric effects could also be influenced by methodological procedures. Specific changes in glomerular volume were consistently observed in all of our in vivo experiments but, surprisingly, not in in vitro experiments (Fabian, 2022). In addition, the DA2 glomerulus was strikingly smaller in the in vitro experiments than in the in vivo experiments. The dissection and fixation of brain tissue adversely affects its volumetric properties (Ma et al., 2008; Grabe et al., 2015), which could conceal subtle morphological differences in some cases.
7. Discussion
All of the mentioned observations in the olfactory system of D. melanogaster and other insect species draw a clear picture – plasticity effects in this part of the nervous system are common in insects. In general, neuronal plasticity is also known from other arthropods, such as crustaceans (Harrison et al., 2002; Sandeman and Sandeman, 2003) and jumping spiders (Steinhoff et al., 2018), but also in other invertebrates like cephalopods (Dickel et al., 2013) and gastropods (Bailey and Chen, 1991; Bailey and Kandel, 2008). It is therefore likely that neuronal plasticity is a widespread feature of centralized nervous systems.
Most studies that focused on plasticity effects in the olfactory system of insect species observed volumetric changes in entire neuropils like the MB and AL. Only a few studies managed to resolve plasticity effects on the scale of individual glomeruli. These morphological changes may be triggered, for example, by foraging, social, or olfactory experiences. In species other than D. melanogaster it is more difficult to link morphological changes to functional changes. However, as genetic techniques for generating genetically modified strains advance, we are likely to obtain more detailed data in the future for comparison with observations in D. melanogaster.
The knowledge we already have today about experience-dependent plasticity in the olfactory system of D. melanogaster indicates that exposure to odorants can cause a variety of opposing morphological, physiological and behavioral effects. These effects are likely to depend on the odorants that were used and the glomeruli that were investigated. Although only very few odorants and glomeruli were investigated in the context of experience-dependent plasticity so far, there was no general effect found that applies to all tested combinations of glomeruli and odorants (Table 1). Some studies report decreased, increased, or unchanged glomerular volumes after flies were exposed to certain odorants. If glomerular sizes are affected, these effects can either be long-lasting or reversible, glomerulus-specific or general, and dependent or independent of an early critical period. The physiological response of associated neurons is also either higher, lower, or unaffected. Similarly, the behavioral response to the exposed odorant can also be either enhanced or weakened dependent on which odorant flies were exposed to during the exposure period. But why are such opposing effects observed across different studies? An obvious answer to this question could be that various circuits in the olfactory system underlie some different molecular mechanisms that cause these circuit-specific effects. Additionally, the impact of the odorant and the resulting changes in the olfactory system could depend on its hedonic valence, which might be specifically important for the behavioral effects of long-term exposure. However, methodological issues cannot be excluded. Although the exposure method is similar in a few studies, it still varies at least in some fine details, which could influence the outcome of the experiments. Standardized procedures in future investigations would certainly help to gather data that is more comparable between studies.
Most mentioned studies that investigated plasticity effects caused by long-term odorant exposure in D. melanogaster used extremely artificial exposure procedures. A continuous exposure period of 2–4 days with odorant concentrations between 0.1% and 25% (Devaud et al., 2001, 2003; Sachse et al., 2007; Das et al., 2011; Kidd et al., 2015; Kidd and Lieber, 2016; Golovin et al., 2019; Chodankar et al., 2020) is very intense and unlikely to occur under natural conditions. At high odorant concentrations, it is more likely that many OSN classes are stimulated, making interpretations of the results difficult (Gugel et al., 2022). The first study to attempt to mimic natural conditions by using much lower odorant concentrations and a periodic exposure method was conducted by Gugel et al. (2022). Such less intense exposure procedures resemble natural conditions more closely. Nevertheless, because glomerular growth was observed in the laboratory under admittedly high odorant concentrations, it is conceivable that it must also occur under natural conditions, since such structural changes are costly and the underlying mechanisms that induce these changes should not become established over evolutionary time periods if they were meaningless. Most olfactory circuits are broadly tuned and activated by many different odorants (Hallem and Carlson, 2006). Therefore, it is conceivable that it is not necessary to expose a fly to high concentrations of a single odorant over a long period of time to induce changes in broadly tuned circuits. Exposure to varying concentrations of different odorants that bind to the same receptor, thereby activating the same underlying neural circuit, might be sufficient to elicit the same plasticity effects that were observed after exposure to a single highly concentrated odorant. Such complex and dynamic stimulation is more likely to occur under natural conditions (Gorur-Shandilya et al., 2019). It would be interesting to see if future studies could replicate the same plasticity effects in broadly tuned circuits using different odors at fluctuating concentrations during the exposure period. To better understand how the observed plasticity effects might occur under natural conditions, it would also be worthwhile to investigate which is the least intense exposure procedure (exposure duration, odor concentration, and stimulus frequency) that still elicits the effects.
Highly specific receptors such as Or56a, GR21a, GR63a or pheromone receptors are crucial for an animal’s behavior. At first glance, it seems puzzling that circuits with a high specificity like the geosmin circuit, which conveys information about the presence of toxic threats (Stensmyr et al., 2012), change based on past experience. One reason for observing experience-dependency in such highly specific olfactory circuits could be their evolutionary past. It is likely that these circuits did not emerge de novo, but evolved from a more broadly tuned circuit and became more specific over time. For example, the emergence of new olfactory circuits could be caused by gene duplications (Prieto-Godino et al., 2017) or by suppression of apoptotic events during development (Prieto-Godino et al., 2020). Therefore, it is credible that narrowly tuned and broadly tuned circuits share at least some similar molecular mechanisms that cause experience-dependent plasticity effects. However, the ability to exhibit plastic changes in highly specific circuits may be more than just a relic of their evolutionary past. Plastic effects in neuronal tissue are an efficient way to adjust the energetically expensive nervous system in response to changing environmental conditions (Eriksson et al., 2019) to optimize the cost-benefit ratio (Niven and Laughlin, 2008).
Moreover, plasticity effects could also play a role in speciation processes. The data show that a female fly exposed to an aversive odorant such as geosmin for an extended period of time subsequently behaves indifferently to the odorant. It is likely to lay more eggs on a medium exposed to a geosmin source, and thus the offspring is exposed to the odorant throughout its development. Golovin et al. (2019) suggest that under such circumstances, the exposure-related phenotype is more robust. If several subsequent generations are exposed to the odor, it could become meaningless for that subpopulation. Under such conditions, random mutations in genes involved in the development of a particular olfactory circuit could accumulate because there would be a lack of positive selection pressure acting upon the integrity of the associated gene-regulatory network. Eventually, the olfactory circuit might cease to function, leading to distinctive behaviors in a genetically segregated subpopulation – a potential starting point for speciation processes. However, the consequences do not necessarily have to be so drastic that an olfactory circuit becomes useless. There could also be much more subtle effects, such as changes in the specificity and sensitivity of OSNs. For example, shifts in host plant use by distinct subpopulations of Drosophila mojavensis Patterson and Crow, 1940 have been shown to be associated with changes in their olfactory systems (Crowley-Gall et al., 2016), which are also involved in reproductive isolation between subpopulations (Khallaf et al., 2020).
Interesting observations were made in studies in which D. melanogaster cohabitated with the parasitoid wasp Leptopilina heterotoma (Thomson, 1862). Flies exposed to these wasps developed a preference for ethanol-containing substrate for oviposition, as an ethanol-rich diet protected larvae from parasitoids (Kacsoh et al., 2013). Surprisingly, this behavioral change was observed in subsequent generations, although the offspring never experienced the presence of wasps (Bozler et al., 2019). Furthermore, exposure of subsequent fly generations to wasps enhanced the effect. To date, it is not known which stimulus triggers the transgenerational effect. However, it is likely that the sense of smell is involved, since L. heterotoma produces iridomyrmecin, a compound that activates a dedicated, highly specific olfactory circuit in D. melanogaster (Ebrahim et al., 2015). Elucidating plasticity effects in this olfactory circuit might be worthwhile to investigate in future studies. Whether exposure to other odorants also has the potential to elicit transgenerational plasticity effects that may be enhanced by exposure of multiple subsequent generations is another intriguing question.
Experience-dependent plasticity in the insect olfactory system as a consequence of an altered chemical environment has the potential to change odorant perception and associated behavior over relatively short time scales. Similar to associative learning (Cook et al., 2020), it may contribute to insect resilience to the effects of climate change and environmental pollution and therefore remains an important area of research for the future.
Author contributions
BF and SS conceived and wrote the manuscript. Both authors contributed to the article and approved the submitted version.
Conflict of interest
The authors declare that the research was conducted in the absence of any commercial or financial relationships that could be construed as a potential conflict of interest.
Publisher’s note
All claims expressed in this article are solely those of the authors and do not necessarily represent those of their affiliated organizations, or those of the publisher, the editors and the reviewers. Any product that may be evaluated in this article, or claim that may be made by its manufacturer, is not guaranteed or endorsed by the publisher.
References
Amador-Vargas, S., Gronenberg, W., Wcislo, W. T., and Mueller, U. (2015). Specialization and group size: brain and behavioural correlates of colony size in ants lacking morphological castes. Proc. R. Soc. B Biol. Sci. 282:20142502. doi: 10.1098/rspb.2014.2502
Anderson, P., Hansson, B. S., Nilsson, U., Han, Q., Sjöholm, M., Skals, N., et al. (2007). Increased behavioral and neuronal sensitivity to sex pheromone after brief odor experience in a moth. Chem. Senses 32, 483–491. doi: 10.1093/chemse/bjm017
Anderson, P., Sadek, M. M., and Hansson, B. S. (2003). Pre-exposure modulates attraction to sex pheromone in a moth. Chem. Senses 28, 285–291. doi: 10.1093/chemse/28.4.285
Andrione, M., Timberlake, B. F., Vallortigara, G., Antolini, R., and Haase, A. (2017). Morphofunctional experience-dependent plasticity in the honeybee brain. Learn. Mem. 24, 622–629. doi: 10.1101/lm.046243.117
Anton, S., Chabaud, M.-A., Schmidt-Büsser, D., Gadenne, B., Iqbal, J., Juchaux, M., et al. (2016). Brief sensory experience differentially affects the volume of olfactory brain centres in a moth. Cell Tissue Res. 364, 59–65. doi: 10.1007/s00441-015-2299-0
Anton, S., Evengaard, K., Barrozo, R. B., Anderson, P., and Skals, N. (2011). Brief predator sound exposure elicits behavioral and neuronal long-term sensitization in the olfactory system of an insect. Proc. Natl. Acad. Sci. U.S.A. 108, 3401–3405. doi: 10.1073/pnas.1008840108
Arenas, A., Giurfa, M., Farina, W. M., and Sandoz, J. C. (2009). Early olfactory experience modifies neural activity in the antennal lobe of a social insect at the adult stage. Eur. J. Neurosci. 30, 1498–1508. doi: 10.1111/j.1460-9568.2009.06940.x
Arenas, A., Giurfa, M., Sandoz, J. C., Hourcade, B., Devaud, J. M., and Farina, W. M. (2012). Early olfactory experience induces structural changes in the primary olfactory center of an insect brain. Eur. J. Neurosci. 35, 682–690. doi: 10.1111/j.1460-9568.2012.07999.x
Bailey, C. H., and Chen, M. (1991). Morphological aspects of synaptic plasticity in Aplysia. An anatomical substrate for long-term memory. Ann. N.Y. Acad. Sci. 627, 181–196. doi: 10.1111/j.1749-6632.1991.tb25924.x
Bailey, C. H., and Kandel, E. R. (2008). “Chapter 10 Synaptic remodeling, synaptic growth and the storage of long-term memory in Aplysia,” in Progress in brain research, Vol. 169, eds W. S. Sossin, J.-C. Lacaille, V. F. Castellucci, and S. Belleville (Amsterdam: Elsevier), 179–198. doi: 10.1016/S0079-6123(07)00010-6
Baltruschat, L., Prisco, L., Ranft, P., Lauritzen, J. S., Fiala, A., Bock, D. D., et al. (2021). Circuit reorganization in the Drosophila mushroom body calyx accompanies memory consolidation. Cell Rep. 34:108871. doi: 10.1016/j.celrep.2021.108871
Barth, M., and Heisenberg, M. (1997). Vision affects mushroom bodies and central complex in Drosophila melanogaster. Learn. Mem. 4, 219–229. doi: 10.1101/lm.4.2.219
Bates, A. S., Schlegel, P., Roberts, R. J. V., Drummond, N., Tamimi, I. F. M., Turnbull, R., et al. (2020). Complete connectomic reconstruction of olfactory projection neurons in the fly brain. Curr. Biol. 30, 3183–3199.e6. doi: 10.1016/j.cub.2020.06.042
Benton, R. (2006). On the ORigin of smell: odorant receptors in insects. Cell. Mol. Life Sci. 63, 1579–1585. doi: 10.1007/s00018-006-6130-7
Berlucchi, G., and Buchtel, H. A. (2008). Neuronal plasticity: historical roots and evolution of meaning. Exp. Brain Res. 192, 307–319. doi: 10.1007/s00221-008-1611-6
Boisduval, J. A. (1833). Faune entomologique de Madagascar, Bourbon et Maurice. Lépidoptères, pl. 1-16. Paris: Roret, 1–122.
Bozler, J., Kacsoh, B. Z., and Bosco, G. (2019). Transgenerational inheritance of ethanol preference is caused by maternal NPF repression. eLife 8:e45391. doi: 10.7554/eLife.45391
Bräcker, Lasse, B., Siju, K. P., Varela, N., Aso, Y., Zhang, M., et al. (2013). Essential role of the mushroom body in context-dependent CO2 avoidance in Drosophila. Curr. Biol. 23, 1228–1234. doi: 10.1016/j.cub.2013.05.029
Brown, S. M., Napper, R. M., and Mercer, A. R. (2004). Foraging experience, glomerulus volume, and synapse number: a stereological study of the honey bee antennal lobe. J. Neurobiol. 60, 40–50. doi: 10.1002/neu.20002
Brückner, K., Perez, L., Clausen, H., and Cohen, S. (2000). Glycosyltransferase activity of fringe modulates notch–delta interactions. Nature 406, 411–415. doi: 10.1038/35019075
Bruhns, O. T. (2018). “History of plasticity,” in Encyclopedia of continuum mechanics, eds H. Altenbach and A. Öchsner (Berlin: Springer), 1–61.
Buckley, S. B. (1866). Descriptions of new species of North American Formicidae. Proc. Entomol. Soc. Philadelphia 6, 152–172.
Busto, G. U., Cervantes-Sandoval, I., and Davis, R. L. (2010). Olfactory learning in Drosophila. Physiology 25, 338–346. doi: 10.1152/physiol.00026.2010
Butcher, N. J., Friedrich, A. B., Lu, Z., Tanimoto, H., and Meinertzhagen, I. A. (2012). Different classes of input and output neurons reveal new features in microglomeruli of the adult Drosophila mushroom body calyx. J. Comp. Neurol. 520, 2185–2201. doi: 10.1002/cne.23037
Cabirol, A., Brooks, R., Groh, C., Barron, A. B., and Devaud, J.-M. (2017). Experience during early adulthood shapes the learning capacities and the number of synaptic boutons in the mushroom bodies of honey bees (Apis mellifera). Learn. Mem. 24, 557–562. doi: 10.1101/lm.045492.117
Cabirol, A., Cope, A. J., Barron, A. B., and Devaud, J. M. (2018). Relationship between brain plasticity, learning and foraging performance in honey bees. PLoS One 13:e0196749. doi: 10.1371/journal.pone.0196749
Caron, S. J., Ruta, V., Abbott, L. F., and Axel, R. (2013). Random convergence of olfactory inputs in the Drosophila mushroom body. Nature 497, 113–117. doi: 10.1038/nature12063
Chakraborty, T. S., Goswami, S. P., and Siddiqi, O. (2009). Sensory correlates of imaginal conditioning in Drosophila melanogaster. J. Neurogenet. 23, 210–219. doi: 10.1080/01677060802491559
Chiang, A., Priya, R., Ramaswami, M., VijayRaghavan, K., and Rodrigues, V. (2009). Neuronal activity and Wnt signaling act through Gsk3-β to regulate axonal integrity in mature Drosophila olfactory sensory neurons. Development 136, 1273–1282. doi: 10.1242/dev.031377
Chodankar, A., Sadanandappa, M. K., VijayRaghavan, K., and Ramaswami, M. (2020). Glomerulus-selective regulation of a critical period for interneuron plasticity in the Drosophila antennal lobe. J. Neurosci. 40, 5549–5560. doi: 10.1523/jneurosci.2192-19.2020
Chou, Y. H., Spletter, M. L., Yaksi, E., Leong, J. C., Wilson, R. I., and Luo, L. (2010). Diversity and wiring variability of olfactory local interneurons in the Drosophila antennal lobe. Nat. Neurosci. 13, 439–449. doi: 10.1038/nn.2489
Cockerell, T. D. A. (1906). Some bees from Washington State. Can. Entomol. 38, 277–282. doi: 10.4039/Ent38277-8
Cook, B., Haverkamp, A., Hansson, B. S., Roulston, T. A., Lerdau, M., and Knaden, M. (2020). Pollination in the anthropocene: a moth can learn ozone-altered floral blends. J. Chem. Ecol. 46, 987–996. doi: 10.1007/s10886-020-01211-4
Couto, A., Alenius, M., and Dickson, B. J. (2005). Molecular, anatomical, and functional organization of the Drosophila olfactory system. Curr. Biol. 15, 1535–1547. doi: 10.1016/j.cub.2005.07.034
Crowley-Gall, A., Date, P., Han, C., Rhodes, N., Andolfatto, P., Layne, J. E., et al. (2016). Population differences in olfaction accompany host shift in Drosophila mojavensis. Proc. R. Soc. B Biol. Sci. 283, 20161562. doi: 10.1098/rspb.2016.1562
Das Chakraborty, S., and Sachse, S. (2021). Olfactory processing in the lateral horn of Drosophila. Cell Tissue Res. 383, 113–123. doi: 10.1007/s00441-020-03392-6
Das, S., Sadanandappa, M. K., Dervan, A., Larkin, A., Lee, J. A., Sudhakaran, I. P., et al. (2011). Plasticity of local GABAergic interneurons drives olfactory habituation. Proc. Natl. Acad. Sci. U.S.A. 108, E646–E654. doi: 10.1073/pnas.1106411108
Das, S., Trona, F., Khallaf, M. A., Schuh, E., Knaden, M., Hansson, B. S., et al. (2017). Electrical synapses mediate synergism between pheromone and food odors in Drosophila melanogaster. Proc. Natl. Acad. Sci. U.S.A. 114, E9962–E9971. doi: 10.1073/pnas.1712706114
Davis, R. L. (2005). Olfactory memory formation in Drosophila: from molecular to systems neuroscience. Annu. Rev. Neurosci. 28, 275–302. doi: 10.1146/annurev.neuro.28.061604.135651
Davis, R. L., and Kiger, J. A. Jr. (1981). Dunce mutants of Drosophila melanogaster: mutants defective in the cyclic AMP phosphodiesterase enzyme system. J. Cell Biol. 90, 101–107. doi: 10.1083/jcb.90.1.101
de Bruyne, M., Clyne, P. J., and Carlson, J. R. (1999). Odor coding in a model olfactory organ: the Drosophila maxillary palp. J. Neurosci. 19, 4520–4532. doi: 10.1523/jneurosci.19-11-04520.1999
de Bruyne, M., Foster, K., and Carlson, J. R. (2001). Odor coding in the Drosophila antenna. Neuron 30, 537–552. doi: 10.1016/S0896-6273(01)00289-6
DeFelipe, J. (2006). Brain plasticity and mental processes: cajal again. Nat. Rev. Neurosci. 7, 811–817. doi: 10.1038/nrn2005
Devaud, J.-M., Acebes, A., and Ferrús, A. (2001). Odor exposure causes central adaptation and morphological changes in selected olfactory glomeruli in Drosophila. J. Neurosci. 21, 6274–6282. doi: 10.1523/JNEUROSCI.21-16-06274.2001
Devaud, J.-M., Acebes, A., Ramaswami, M., and Ferrús, A. (2003). Structural and functional changes in the olfactory pathway of adult Drosophila take place at a critical age. J. Neurobiol. 56, 13–23. doi: 10.1002/neu.10215
Dickel, L., Darmaillacq, A.-S., Jozet-Alves, C., and Bellanger, C. (2013). “Chapter 25 - Learning, memory, and brain plasticity in cuttlefish (Sepia officinalis),” in Handbook of behavioral neuroscience, Vol. 22, eds R. Menzel and P. R. Benjamin (Amsterdam: Elsevier), 318–333.
Dolan, M.-J., Belliart-Guérin, G., Bates, A. S., Frechter, S., Lampin-Saint-Amaux, A., Aso, Y., et al. (2018). Communication from learned to innate olfactory processing centers is required for memory retrieval in Drosophila. Neuron 100, 651–668.e8. doi: 10.1016/j.neuron.2018.08.037
Dolan, M.-J., Frechter, S., Bates, A. S., Dan, C., Huoviala, P., Roberts, R. J. V., et al. (2019). Neurogenetic dissection of the Drosophila lateral horn reveals major outputs, diverse behavioural functions, and interactions with the mushroom body. eLife. 8:e43079. doi: 10.7554/eLife.43079
Doll, C. A., Vita, D. J., and Broadie, K. (2017). Fragile X mental retardation protein requirements in activity-dependent critical period neural circuit refinement. Curr. Biol. 27, 2318–2330.e3. doi: 10.1016/j.cub.2017.06.046
Dubnau, J., and Tully, T. (2001). Functional anatomy: from molecule to memory. Curr. Biol. 11, R240–R243. doi: 10.1016/S0960-9822(01)00115-4
Durst, C., Eichmüller, S., and Menzel, R. (1994). Development and experience lead to increased volume of subcompartments of the honeybee mushroom body. Behav. Neural Biol. 62, 259–263. doi: 10.1016/S0163-1047(05)80025-1
Ebrahim, S. A. M., Dweck, H. K. M., Stökl, J., Hofferberth, J. E., Trona, F., Weniger, K., et al. (2015). Drosophila avoids parasitoids by sensing their semiochemicals via a dedicated olfactory circuit. PLoS Biol. 13:e1002318. doi: 10.1371/journal.pbio.1002318
Ellis, P. E. (1963). Changes in the social aggregation of locust hoppers with changes in rearing conditions. Anim. Behav. 11, 152–160. doi: 10.1016/0003-3472(63)90023-X
Emery, C. (1890). Studii sulle formiche della fauna neotropica. Bull. della Soc. Entomol. Ital. 22, 38–80.
Eriksson, M., Nylin, S., and Carlsson, M. A. (2019). Insect brain plasticity: effects of olfactory input on neuropil size. R. Soc. Open Sci. 6:190875. doi: 10.1098/rsos.190875
Fabian, B. (2022). Experience-dependent plasticity of olfactory circuits (PhD Thesis). Jena: Friedrich Schiller University.
Fabricius, J. C. (1775). Systema entomologiae, sistens insectorum classes, ordines, genera, species, adiectis synonymis, locis, descriptionibus, observationibus. Library kortii: Flensburgi et lipsiae [=flensburg and leipzig], Germany, p. 832.
Fahrbach, S. E., Moore, D., Capaldi, E. A., Farris, S. M., and Robinson, G. E. (1998). Experience-expectant plasticity in the mushroom bodies of the honeybee. Learn. Mem. 5, 115–123.
Fiala, A. (2007). Olfaction and olfactory learning in Drosophila: recent progress. Curr. Opin. Neurobiol. 17, 720–726. doi: 10.1016/j.conb.2007.11.009
Fishilevich, E., and Vosshall, L. B. (2005). Genetic and functional subdivision of the Drosophila antennal lobe. Curr. Biol. 15, 1548–1553. doi: 10.1016/j.cub.2005.07.066
Forsskål, P. (1775). Descriptiones animalium, avium, amphibiorum, piscium, insectorum, vermium. Hauniæ: ex officina Mölleri.
Frank, D. D., Enjin, A., Jouandet, G. C., Zaharieva, E. E., Para, A., Stensmyr, M. C., et al. (2017). Early integration of temperature and humidity stimuli in the Drosophila brain. Curr. Biol. 27, 2381–2388.e4. doi: 10.1016/j.cub.2017.06.077
Frank, D. D., Jouandet, G. C., Kearney, P. J., Macpherson, L. J., and Gallio, M. (2015). Temperature representation in the Drosophila brain. Nature 519, 358–361. doi: 10.1038/nature14284
Friedman, S. H., Dani, N., Rushton, E., and Broadie, K. (2013). Fragile X mental retardation protein regulates trans-synaptic signaling in Drosophila. Dis. Models Mech. 6, 1400–1413. doi: 10.1242/dmm.012229
Galizia, C. G., Sachse, S., Rappert, A., and Menzel, R. (1999). The glomerular code for odor representation is species specific in the honeybee Apis mellifera. Nat. Neurosci. 2, 473–478. doi: 10.1038/8144
Gao, Q., Yuan, B., and Chess, A. (2000). Convergent projections of Drosophila olfactory neurons to specific glomeruli in the antennal lobe. Nat. Neurosci. 3, 780–785. doi: 10.1038/77680
Golovin, R. M., and Broadie, K. (2016). Developmental experience-dependent plasticity in the first synapse of the Drosophila olfactory circuit. J. Neurophysiol. 116, 2730–2738. doi: 10.1152/jn.00616.2016
Golovin, R. M., Vest, J., Vita, D. J., and Broadie, K. (2019). Activity-dependent remodeling of Drosophila olfactory sensory neuron brain innervation during an early-life critical period. J. Neurosci. 39, 2995–3012. doi: 10.1523/JNEUROSCI.2223-18.2019
Gomez-Diaz, C., Martin, F., Garcia-Fernandez, J. M., and Alcorta, E. (2018). The two main olfactory receptor families in Drosophila, ORs and IRs: a comparative approach. Front. Cell. Neurosci. 12:253. doi: 10.3389/fncel.2018.00253
Gorur-Shandilya, S., Martelli, C., Demir, M., and Emonet, T. (2019). Controlling and measuring dynamic odorant stimuli in the laboratory. J. Exp. Biol. 222(Pt. 23):jeb207787. doi: 10.1242/jeb.207787
Gotham, S., and Song, H. (2013). Non-swarming grasshoppers exhibit density-dependent phenotypic plasticity reminiscent of swarming locusts. J. Insect Physiol. 59, 1151–1159. doi: 10.1016/j.jinsphys.2013.08.017
Grabe, V., Strutz, A., Baschwitz, A., Hansson, B. S., and Sachse, S. (2015). Digital in vivo 3D atlas of the antennal lobe of Drosophila melanogaster. J. Comp. Neurol. 523, 530–544. doi: 10.1002/cne.23697
Groh, C., Ahrens, D., and Rössler, W. (2006). Environment- and age-dependent plasticity of synaptic complexes in the mushroom bodies of honeybee queens. Brain Behav. Evol. 68, 1–14. doi: 10.1159/000092309
Groh, C., and Meinertzhagen, I. A. (2010). Brain plasticity in Diptera and Hymenoptera. Front. Biosci. 2:268–288. doi: 10.2741/s63
Groh, C., Tautz, J., and Rossler, W. (2004). Synaptic organization in the adult honey bee brain is influenced by brood-temperature control during pupal development. Proc. Natl. Acad. Sci. U.S.A. 101, 4268–4273. doi: 10.1073/pnas.0400773101
Gronenberg, W., Heeren, S., and Hölldobler, B. (1996). Age-dependent and task-related morphological changes in the brain and the mushroom bodies of the ant Camponotus floridanus. J. Exp. Biol. 199, 2011–2019. doi: 10.1242/jeb.199.9.2011
Gruntman, E., and Turner, G. C. (2013). Integration of the olfactory code across dendritic claws of single mushroom body neurons. Nat. Neurosci. 16, 1821–1829. doi: 10.1038/nn.3547
Guerrieri, F., Gemeno, C., Monsempes, C., Anton, S., Jacquin-Joly, E., Lucas, P., et al. (2012). Experience-dependent modulation of antennal sensitivity and input to antennal lobes in male moths (Spodoptera littoralis) pre-exposed to sex pheromone. J. Exp. Biol. 215, 2334–2341. doi: 10.1242/jeb.060988
Gugel, Z. V., Maurais, E., and Hong, E. J. (2022). Chronic exposure to odors at naturally occurring concentrations triggers limited plasticity in early stages of Drosophila olfactory processing. bioRxiv [Preprint]. doi: 10.1101/2021.09.03.458834
Hagadorn, M. A., Johnson, M. M., Smith, A. R., Seid, M. A., and Kapheim, K. M. (2021). Experience, but not age, is associated with volumetric mushroom body expansion in solitary alkali bees. J. Exp. Biol. 224:jeb238899. doi: 10.1242/jeb.238899
Hallem, E. A., and Carlson, J. R. (2006). Coding of odors by a receptor repertoire. Cell 125, 143–160. doi: 10.1016/j.cell.2006.01.050
Hansson, B. S., and Stensmyr, M. C. (2011). Evolution of insect olfaction. Neuron 72, 698–711. doi: 10.1016/j.neuron.2011.11.003
Harrison, P. J. H., Cate, H. S., Steullet, P., and Derby, C. D. (2002). Structural plasticity in the olfactory system of adult spiny lobsters: postembryonic development permits life-long growth, turnover, and regeneration. Mar. Freshw. Res. 52, 1357–1365. doi: 10.1071/MF01103
Hayashi, T. T., MacKenzie, A. J., Ganguly, I., Ellis, K. E., Smihula, H. M., Jacob, M. S., et al. (2022). Mushroom body input connections form independently of sensory activity in Drosophila melanogaster. Curr. Biol. 32, 4000–4012.e5. doi: 10.1016/j.cub.2022.07.055
Heisenberg, M. (2003). Mushroom body memoir: from maps to models. Nat. Rev. Neurosci. 4, 266–275. doi: 10.1038/nrn1074
Heisenberg, M., Borst, A., Wagner, S., and Byers, D. (1985). Drosophila mushroom body mutants are deficient in olfactory learning. J. Neurogenet. 2, 1–30. doi: 10.3109/01677068509100140
Herre, M., Goldman, O. V., Lu, T.-C., Caballero-Vidal, G., Qi, Y., Gilbert, Z. N., et al. (2022). Non-canonical odor coding in the mosquito. Cell 185, 3104–3123.e28. doi: 10.1016/j.cell.2022.07.024
Horne, J. A., Langille, C., McLin, S., Wiederman, M., Lu, Z., Xu, C. S., et al. (2018). A resource for the Drosophila antennal lobe provided by the connectome of glomerulus VA1v. eLife 7:e37550. doi: 10.7554/eLife.37550
Hourcade, B., Perisse, E., Devaud, J. M., and Sandoz, J. C. (2009). Long-term memory shapes the primary olfactory center of an insect brain. Learn. Mem. 16, 607–615. doi: 10.1101/lm.1445609
Hu, X. S., Ikegami, K., Vihani, A., Zhu, K. W., Zapata, M., de March, C. A., et al. (2020). Concentration-dependent recruitment of mammalian odorant receptors. eneuro 7, ENEURO.0103–ENEURO.0119. doi: 10.1523/eneuro.0103-19.2019
Huang, J., Zhang, W., Qiao, W., Hu, A., and Wang, Z. (2010). Functional connectivity and selective odor responses of excitatory local interneurons in Drosophila antennal lobe. Neuron 67, 1021–1033. doi: 10.1016/j.neuron.2010.08.025
Huoviala, P., Dolan, M.-J., Love, F. M., Myers, P., Frechter, S., Namiki, S., et al. (2020). Neural circuit basis of aversive odour processing in Drosophila from sensory input to descending output. bioRxiv [Preprint]. doi: 10.1101/394403
Ignell, R., Root, C. M., Birse, R. T., Wang, J. W., Nässel, D. R., and Winther, ÅM. E. (2009). Presynaptic peptidergic modulation of olfactory receptor neurons in Drosophila. Proc. Natl. Acad. Sci. U.S.A. 106, 13070–13075. doi: 10.1073/pnas.0813004106
Iyengar, A., Chakraborty, T. S., Goswami, S. P., Wu, C. F., and Siddiqi, O. (2010). Post-eclosion odor experience modifies olfactory receptor neuron coding in Drosophila. Proc. Natl. Acad. Sci. U.S.A. 107, 9855–9860. doi: 10.1073/pnas.1003856107
James, W. (1890). “Chapter IV-habit,” in The principles of psychology, Vol. I. (New York, NY: H. Holt and Company), 104–127.
Jaumann, S., Seid, M. A., Simons, M., and Smith, A. R. (2019). Queen dominance may reduce worker mushroom body size in a social bee. Dev. Neurobiol. 79, 596–607. doi: 10.1002/dneu.22705
Jefferis, G. S. X. E., Marin, E. C., Stocker, R. F., and Luo, L. (2001). Target neuron prespecification in the olfactory map of Drosophila. Nature 414, 204–208. doi: 10.1038/35102574
Jefferis, G. S., and Hummel, T. (2006). Wiring specificity in the olfactory system. Semin. Cell Dev. Biol. 17, 50–65. doi: 10.1016/j.semcdb.2005.12.002
Jefferis, G. S., Potter, C. J., Chan, A. M., Marin, E. C., Rohlfing, T., and Maurer, C. R. Jr., et al. (2007). Comprehensive maps of Drosophila higher olfactory centers: spatially segregated fruit and pheromone representation. Cell 128, 1187–1203. doi: 10.1016/j.cell.2007.01.040
Jefferis, G. S., Vyas, R. M., Berdnik, D., Ramaekers, A., Stocker, R. F., Tanaka, N. K., et al. (2004). Developmental origin of wiring specificity in the olfactory system of Drosophila. Development 131, 117–130. doi: 10.1242/dev.00896
Jenett, A., Rubin, Gerald, M., Ngo, T.-T. B., Shepherd, D., Murphy, C., et al. (2012). A GAL4-driver line resource for Drosophila neurobiology. Cell Rep. 2, 991–1001. doi: 10.1016/j.celrep.2012.09.011
Jones, E. G. (2000). NEUROwords 8 plasticity and neuroplasticity. J. Hist. Neurosci. 9, 37–39. doi: 10.1076/0964-704x(200004)9:1;1-2;ft037
Joseph, R. M., and Carlson, J. R. (2015). Drosophila chemoreceptors: a molecular interface between the chemical world and the brain. Trends Genet. 31, 683–695. doi: 10.1016/j.tig.2015.09.005
Kacsoh, B. Z., Lynch, Z. R., Mortimer, N. T., and Schlenke, T. A. (2013). Fruit flies medicate offspring after seeing parasites. Science 339, 947–950.
Khallaf, M., Auer, T. O., Grabe, V., Depetris-Chauvin, A., Ammagarahalli, B., Zhang, D.-D., et al. (2020). Mate discrimination among subspecies through a conserved olfactory pathway. Sci. Adv. 6:eaba5279. doi: 10.1126/sciadv.aba5279
Kidd, S., and Lieber, T. (2016). Mechanism of notch pathway activation and its role in the regulation of olfactory plasticity in Drosophila melanogaster. PLoS One 11:e0151279. doi: 10.1371/journal.pone.0151279
Kidd, S., Struhl, G., and Lieber, T. (2015). Notch is required in adult Drosophila sensory neurons for morphological and functional plasticity of the olfactory circuit. PLoS Genet. 11:e1005244. doi: 10.1371/journal.pgen.1005244
Kirkhart, C., and Scott, K. (2015). Gustatory learning and processing in the Drosophila mushroom bodies. J. Neurosci. 35, 5950–5958. doi: 10.1523/jneurosci.3930-14.2015
Knaden, M., Strutz, A., Ahsan, J., Sachse, S., Hansson, and Bill, S. (2012). Spatial representation of odorant valence in an insect brain. Cell Rep. 1, 392–399.
Kopke, D. L., Lima, S. C., Alexandre, C., and Broadie, K. (2017). Notum coordinates synapse development via extracellular regulation of Wnt Wingless trans-synaptic signaling. Development 144, 3499–3510. doi: 10.1242/dev.148130
Korkut, C., Ataman, B., Ramachandran, P., Ashley, J., Barria, R., Gherbesi, N., et al. (2009). Trans-synaptic transmission of vesicular Wnt signals through Evi/Wntless. Cell 139, 393–404. doi: 10.1016/j.cell.2009.07.051
Kremer, M. C., Christiansen, F., Leiss, F., Paehler, M., Knapek, S., Andlauer, T. F., et al. (2010). Structural long-term changes at mushroom body input synapses. Curr. Biol. 20, 1938–1944. doi: 10.1016/j.cub.2010.09.060
Kremer, M. C., Jung, C., Batelli, S., Rubin, G. M., and Gaul, U. (2017). The glia of the adult Drosophila nervous system. Glia 65, 606–638. doi: 10.1002/glia.23115
Lai, S. L., Awasaki, T., Ito, K., and Lee, T. (2008). Clonal analysis of Drosophila antennal lobe neurons: diverse neuronal architectures in the lateral neuroblast lineage. Development 135, 2883–2893. doi: 10.1242/dev.024380
Laissue, P. P., and Vosshall, L. B. (2008). “The olfactory sensory map in Drosophila,” in Brain development in Drosophila melanogaster, ed. G. M. Technau (New York, NY: Springer), 102–114.
Laissue, P. P., Reiter, C., Hiesinger, P. R., Halter, S., Fischbach, K. F., and Stocker, R. F. (1999). Three-dimensional reconstruction of the antennal lobe in Drosophila melanogaster. J. Comp. Neurol. 405, 543–552. doi: 10.1002/(SICI)1096-9861(19990322)405:4<543::AID-CNE7<3.0.CO;2-A
Larter, N. K., Sun, J. S., and Carlson, J. R. (2016). Organization and function of Drosophila odorant binding proteins. eLife 5:e20242. doi: 10.7554/eLife.20242
Laughlin, J. D., Ha, T. S., Jones, D. N. M., and Smith, D. P. (2008). Activation of pheromone-sensitive neurons is mediated by conformational activation of pheromone-binding protein. Cell 133, 1255–1265. doi: 10.1016/j.cell.2008.04.046
Leal, W. S. (2013). Odorant reception in insects: roles of receptors, binding proteins, and degrading enzymes. Annu. Rev. Entomol. 58, 373–391. doi: 10.1146/annurev-ento-120811-153635
Lee, D. (2015). Global and local missions of cAMP signaling in neural plasticity, learning, and memory. Front. Pharmacol. 6:161. doi: 10.3389/fphar.2015.00161
Leiss, F., Groh, C., Butcher, N. J., Meinertzhagen, I. A., and Tavosanis, G. (2009). Synaptic organization in the adult Drosophila mushroom body calyx. J. Comp. Neurol. 517, 808–824. doi: 10.1002/cne.22184
Levin, L. R., Han, P.-L., Hwang, P. M., Feinstein, P. G., Davis, R. L., and Reed, R. R. (1992). The Drosophila learning and memory gene rutabaga encodes a Ca2+calmodulin-responsive adenylyl cyclase. Cell 68, 479–489. doi: 10.1016/0092-8674(92)90185-F
Lewis, Laurence, P. C., Siju, K. P., Aso, Y., Friedrich, Anja, B., et al. (2015). A higher brain circuit for immediate integration of conflicting sensory information in Drosophila. Curr. Biol. 25, 2203–2214. doi: 10.1016/j.cub.2015.07.015
Li, F., Lindsey, J. W., Marin, E. C., Otto, N., Dreher, M., Dempsey, G., et al. (2020). The connectome of the adult Drosophila mushroom body provides insights into function. eLife 9:62576. doi: 10.7554/eLife.62576
Li, T., Fu, T.-M., Wong, K. K. L., Li, H., Xie, Q., Luginbuhl, D. J., et al. (2021). Cellular bases of olfactory circuit assembly revealed by systematic time-lapse imaging. Cell 184, 5107–5121.e14. doi: 10.1016/j.cell.2021.08.030
Lin, H.-H., Lai, J. S.-Y., Chin, A.-L., Chen, Y.-C., and Chiang, A.-S. (2007). A map of olfactory representation in the Drosophila mushroom body. Cell 128, 1205–1217. doi: 10.1016/j.cell.2007.03.006
Lin, S., Owald, D., Chandra, V., Talbot, C., Huetteroth, W., and Waddell, S. (2014). Neural correlates of water reward in thirsty Drosophila. Nat. Neurosci. 17, 1536–1542. doi: 10.1038/nn.3827
Linnaeus, C. (1758). Systema naturæper regna tria naturæ, secundum classes, ordines, genera, species, cum characteribus, differentiis, synonymis, locis, 10th Edn, Vol. 1. Holmiæ: Laurentii Salvii.
Linnaeus, C. (1762). “Zweyter Theil, enthalt Beschreibungen verschiedener wichtiger Naturalien,” in Reise nach Palästina in den Jahren von 1749 bis 1752, ed. T. H. Gadebusch (Germany: Rostock), 267–606.
Liu, W. W., and Wilson, R. I. (2013). Glutamate is an inhibitory neurotransmitter in the Drosophila olfactory system. Proc. Natl. Acad. Sci. U.S.A. 110, 10294–10299. doi: 10.1073/pnas.1220560110
Livingstone, M. S., Sziber, P. P., and Quinn, W. G. (1984). Loss of calcium/calmodulin responsiveness in adenylate cyclase of rutabaga, a Drosophila learning mutant. Cell 37, 205–215. doi: 10.1016/0092-8674(84)90316-7
Lorenzi, M. C., and d’Ettorre, P. (2020). Nestmate recognition in social insects: what does it mean to be chemically insignificant? Front. Ecol. Evol. 7:488. doi: 10.3389/fevo.2019.00488
Lugaro, E. (1909). Modern problems in psychiatry. translated by D. Orr and R. G. Rows. Manchester: The University Press.
Ma, Y., Smith, D., Hof, P., Foerster, B., Hamilton, S., Blackband, S., et al. (2008). In vivo 3D digital atlas database of the adult C57BL/6J mouse brain by magnetic resonance microscopy. Front. Neuroanat. 2:1. doi: 10.3389/neuro.05.001.2008
Maleszka, J., Barron, A. B., Helliwell, P. G., and Maleszka, R. (2009). Effect of age, behaviour and social environment on honey bee brain plasticity. J. Comp. Physiol. A 195, 733–740. doi: 10.1007/s00359-009-0449-0
Marin, E. C., Büld, L., Theiss, M., Sarkissian, T., Roberts, R. J. V., Turnbull, R., et al. (2020). Connectomics analysis reveals first-, second-, and third-order thermosensory and hygrosensory neurons in the adult Drosophila brain. Curr. Biol. 30, 3167–3182.e4. doi: 10.1016/j.cub.2020.06.028
Marin, E. C., Jefferis, G. S. X. E., Komiyama, T., Zhu, H., and Luo, L. (2002). Representation of the glomerular olfactory map in the Drosophila brain. Cell 109, 243–255. doi: 10.1016/S0092-8674(02)00700-6
Martin, F., Boto, T., Gomez-Diaz, C., and Alcorta, E. (2013). Elements of olfactory reception in adult Drosophila melanogaster. Anat. Record 296, 1477–1488. doi: 10.1002/ar.22747
Mateos-Aparicio, P., and Rodríguez-Moreno, A. (2019). The impact of studying brain plasticity. Front. Cell. Neurosci. 13:66. doi: 10.3389/fncel.2019.00066
McCann, C., Holohan, E. E., Das, S., Dervan, A., Larkin, A., Lee, J. A., et al. (2011). The Ataxin-2 protein is required for microRNA function and synapse-specific long-term olfactory habituation. Proc. Natl. Acad. Sci. U.S.A. 108, E655–E662. doi: 10.1073/pnas.1107198108
Meade-Waldo, G. (1916). LIII.—Notes on the Apidæ (Hymenoptera) in the collection of the British Museum, with descriptions of new species. Ann. Magaz. Natural Hist. 17, 448–470. doi: 10.1080/00222931608693811
Meigen, J. W. (1830). Systematische beschreibung der bekannten europäischen zweiflügeligen insekten, Vol. 6. Tirschenreuth: Hamm, 11–401.
Miller, G. A., Islam, M. S., Claridge, T. D. W., Dodgson, T., and Simpson, S. J. (2008). Swarm formation in the desert locust Schistocerca gregaria: isolation and NMR analysis of the primary maternal gregarizing agent. J. Exp. Biol. 211, 370–376. doi: 10.1242/jeb.013458
Min, S., Ai, M., Shin, S. A., and Suh, G. S. B. (2013). Dedicated olfactory neurons mediating attraction behavior to ammonia and amines in Drosophila. Proc. Natl. Acad. Sci. U.S.A. 110, E1321–E1329. doi: 10.1073/pnas.1215680110
Minea, I. (1909). Cercetäri experimentale asupra variatiunilor morfologice ale neuronului sensitiv (studiul ‘reactiunii plastice’). Bucharest: Brozer and Parzer.
Mohamed, A. A. M., Retzke, T., Das Chakraborty, S., Fabian, B., Hansson, B. S., Knaden, M., et al. (2019). Odor mixtures of opposing valence unveil inter-glomerular crosstalk in the Drosophila antennal lobe. Nat. Commun. 10:1201. doi: 10.1038/s41467-019-09069-1
Montgomery, S. H., Merrill, R. M., and Ott, S. R. (2016). Brain composition in Heliconius butterflies, posteclosion growth and experience-dependent neuropil plasticity. J. Comp. Neurol. 524, 1747–1769. doi: 10.1002/cne.23993
Murthy, M., Fiete, I., and Laurent, G. (2008). Testing odor response stereotypy in the Drosophila mushroom body. Neuron 59, 1009–1023. doi: 10.1016/j.neuron.2008.07.040
Ng, M., Roorda, R. D., Lima, S. Q., Zemelman, B. V., Morcillo, P., and Miesenböck, G. (2002). Transmission of olfactory information between three populations of neurons in the antennal lobe of the fly. Neuron 36, 463–474. doi: 10.1016/S0896-6273(02)00975-3
Niven, J. E., and Laughlin, S. B. (2008). Energy limitation as a selective pressure on the evolution of sensory systems. J. Exp. Biol. 211, 1792–1804. doi: 10.1242/jeb.017574
Okada, R., Awasaki, T., and Ito, K. (2009). Gamma-aminobutyric acid (GABA)-mediated neural connections in the Drosophila antennal lobe. J. Comp. Neurol. 514, 74–91. doi: 10.1002/cne.21971
Okajima, T., Xu, A., and Irvine, K. D. (2003). Modulation of Notch-Ligand Binding by Protein O-Fucosyltransferase 1 and Fringe*. J. Biol. Chem. 278, 42340–42345. doi: 10.1074/jbc.M308687200
Oland, L. A., Biebelhausen, J. P., and Tolbert, L. P. (2008). Glial investment of the adult and developing antennal lobe of Drosophila. J. Comp. Neurol. 509, 526–550. doi: 10.1002/cne.21762
Olsen, S. R., and Wilson, R. I. (2008). Lateral presynaptic inhibition mediates gain control in an olfactory circuit. Nature 452, 956–960. doi: 10.1038/nature06864
Olsen, S. R., Bhandawat, V., and Wilson, R. I. (2007). Excitatory interactions between olfactory processing channels in the Drosophila antennal lobe. Neuron 54, 89–103. doi: 10.1016/j.neuron.2007.03.010
Ott, S. R., and Rogers, S. M. (2010). Gregarious desert locusts have substantially larger brains with altered proportions compared with the solitarious phase. Proc. R. Soc. B Biol. Sci. 277, 3087–3096. doi: 10.1098/rspb.2010.0694
Packard, M., Koo, E. S., Gorczyca, M., Sharpe, J., Cumberledge, S., and Budnik, V. (2002). The Drosophila Wnt, wingless, provides an essential signal for pre- and postsynaptic differentiation. Cell 111, 319–330. doi: 10.1016/S0092-8674(02)01047-4’
Paillard, J. (1976). Réflexions sur l’usage du concept de plasticité en neurobiologie. J. Psychol. Normale Pathologique 1, 33–47.
Park, S. K., Shanbhag, S. R., Wang, Q., Hasan, G., Steinbrecht, R. A., and Pikielny, C. W. (2000). Expression patterns of two putative odorant-binding proteins in the olfactory organs of Drosophila melanogaster have different implications for their functions. Cell Tissue Res. 300, 181–192. doi: 10.1007/s004410000187
Patterson, J., and Crow, J. (1940). XII. Hybridization in the mulleri group of Drosophila. Univ. Texas Public. 4032, 251–256.
Pech, U., Revelo, N. H., Seitz, K. J., Rizzoli, S. O., and Fiala, A. (2015). Optical dissection of experience-dependent pre- and postsynaptic plasticity in the Drosophila brain. Cell Rep. 10, 2083–2095. doi: 10.1016/j.celrep.2015.02.065
Pelosi, P., Zhou, J. J., Ban, L. P., and Calvello, M. (2006). Soluble proteins in insect chemical communication. Cell. Mol. Life Sci. 63, 1658–1676. doi: 10.1007/s00018-005-5607-0
Pocco, M. E., Cigliano, M. M., Foquet, B., Lange, C. E., Nieves, E. L., and Song, H. (2019). Density-dependent phenotypic plasticity in the South American Locust, Schistocerca cancellata (Orthoptera: Acrididae). Ann. Entomol. Soc. Am. 112, 458–472. doi: 10.1093/aesa/saz032
Prieto-Godino, L. L., Rytz, R., Cruchet, S., Bargeton, B., Abuin, L., Silbering, A. F., et al. (2017). Evolution of acid-sensing olfactory circuits in drosophilids. Neuron 93, 661–676.e6. doi: 10.1016/j.neuron.2016.12.024
Prieto-Godino, L. L., Silbering, A. F., Khallaf, M. A., Cruchet, S., Bojkowska, K., Pradervand, S., et al. (2020). Functional integration of “undead” neurons in the olfactory system. Sci. Adv. 6:eaaz7238. doi: 10.1126/sciadv.aaz7238
Ramain, P., Khechumian, K., Seugnet, L., Arbogast, N., Ackermann, C., and Heitzler, P. (2001). Novel Notch alleles reveal a Deltex-dependent pathway repressing neural fate. Curr. Biol. 11, 1729–1738. doi: 10.1016/S0960-9822(01)00562-0
Ramón y Cajal, S. (1894). Consideraciones generales sobre la morfología de la célula nerviosa. La Veterinaria Española 37, 257–260, 273–275, 289–291.
Rebay, I., Fleming, R. J., Fehon, R. G., Cherbas, L., Cherbas, P., and Artavanis-Tsakonas, S. (1991). Specific EGF repeats of Notch mediate interactions with delta and serrate: implications for notch as a multifunctional receptor. Cell 67, 687–699. doi: 10.1016/0092-8674(91)90064-6
Roessingh, P., Simpson, S. J., and James, S. (1993). Analysis of phase-related changes in behaviour of desert locust nymphs. Proc. R. Soc. London Ser. B Biol. Sci. 252, 43–49. doi: 10.1098/rspb.1993.0044
Root, C. M., Masuyama, K., Green, D. S., Enell, L. E., Nässel, D. R., Lee, C.-H., et al. (2008). A presynaptic gain control mechanism fine-tunes olfactory behavior. Neuron 59, 311–321. doi: 10.1016/j.neuron.2008.07.003
Rybak, J., Talarico, G., Ruiz, S., Arnold, C., Cantera, R., and Hansson, B. S. (2016). Synaptic circuitry of identified neurons in the antennal lobe of Drosophila melanogaster. J. Comp. Neurol. 524, 1920–1956. doi: 10.1002/cne.23966
Sachse, S., Rappert, A., and Galizia, C. G. (1999). The spatial representation of chemical structures in the antennal lobe of honeybees: steps towards the olfactory code. Eur. J. Neurosci. 11, 3970–3982. doi: 10.1046/j.1460-9568.1999.00826.x
Sachse, S., Rueckert, E., Keller, A., Okada, R., Tanaka, N. K., Ito, K., et al. (2007). Activity-dependent plasticity in an olfactory circuit. Neuron 56, 838–850. doi: 10.1016/j.neuron.2007.10.035
Sandeman, R., and Sandeman, D. (2003). Development, growth, and plasticity in the crayfish olfactory system. Microsc. Res. Technique 60, 266–277. doi: 10.1002/jemt.10266
Say, T. (1837). Descriptions of new species of North American Hymenoptera, and observations on some already described. Boston J. Nat. Hist. 1, 361–416.
Scheffer, L. K., Xu, C. S., Januszewski, M., Lu, Z., Takemura, S.-Y., Hayworth, K. J., et al. (2020). A connectome and analysis of the adult Drosophila central brain. eLife 9:e57443. doi: 10.7554/eLife.57443
Scheuermann, E. A., and Smith, D. P. (2019). Odor-specific deactivation defects in a Drosophila odorant-binding protein mutant. Genetics 213, 897–909. doi: 10.1534/genetics.119.302629
Scheunemann, L., Plaçais, P.-Y., Dromard, Y., Schwärzel, M., and Preat, T. (2018). Dunce phosphodiesterase acts as a checkpoint for Drosophila long-term memory in a pair of serotonergic neurons. Neuron 98, 350–365.e5. doi: 10.1016/j.neuron.2018.03.032
Schlegel, P., Bates, A. S., Stürner, T., Jagannathan, S. R., Drummond, N., Hsu, J., et al. (2021). Information flow, cell types and stereotypy in a full olfactory connectome. eLife 10:e66018. doi: 10.7554/eLife.66018
Scott, K., Brady, R., Cravchik, A., Morozov, P., Rzhetsky, A., Zuker, C., et al. (2001). A chemosensory gene family encoding candidate gustatory and olfactory receptors in Drosophila. Cell 104, 661–673. doi: 10.1016/S0092-8674(01)00263-X
Séjourné, J., Plaçais, P.-Y., Aso, Y., Siwanowicz, I., Trannoy, S., Thoma, V., et al. (2011). Mushroom body efferent neurons responsible for aversive olfactory memory retrieval in Drosophila. Nat. Neurosci. 14, 903–910. doi: 10.1038/nn.2846
Seki, Y., Dweck, H. K. M., Rybak, J., Wicher, D., Sachse, S., and Hansson, B. S. (2017). Olfactory coding from the periphery to higher brain centers in the Drosophila brain. BMC Biol. 15:56. doi: 10.1186/s12915-017-0389-z
Seki, Y., Rybak, J., Wicher, D., Sachse, S., and Hansson, B. S. (2010). Physiological and morphological characterization of local interneurons in the Drosophila antennal lobe. J. Neurophysiol. 104, 1007–1019. doi: 10.1152/jn.00249.2010
Shanbhag, S. R., Hekmat-Scafe, D., Kim, M. S., Park, S. K., Carlson, J. R., Pikielny, C., et al. (2001). Expression mosaic of odorant-binding proteins in Drosophila olfactory organs. Microsc. Res. Technique 55, 297–306. doi: 10.1002/jemt.1179
Shanbhag, S. R., Müller, B., and Steinbrecht, R. A. (1999). Atlas of olfactory organs of Drosophila melanogaster: 1. Types, external organization, innervation and distribution of olfactory sensilla. Int. J. Insect Morphol. Embryol. 28, 377–397. doi: 10.1016/S0020-7322(99)00039-2
Shanbhag, S. R., Müller, B., and Steinbrecht, R. A. (2000). Atlas of olfactory organs of Drosophila melanogaster: 2. Internal organization and cellular architecture of olfactory sensilla. Arthropod Struct. Dev. 29, 211–229. doi: 10.1016/S1467-8039(00)00028-1
Shang, Y., Claridge-Chang, A., Sjulson, L., Pypaert, M., and Miesenböck, G. (2007). Excitatory local circuits and their implications for olfactory processing in the fly antennal lobe. Cell 128, 601–612. doi: 10.1016/j.cell.2006.12.034
Sheng, C., Javed, U., Gibbs, M., Long, C., Yin, J., Qin, B., et al. (2018). Experience-dependent structural plasticity targets dynamic filopodia in regulating dendrite maturation and synaptogenesis. Nat. Commun. 9:3362. doi: 10.1038/s41467-018-05871-5
Sigg, D., Thompson, C. M., and Mercer, A. R. (1997). Activity-dependent changes to the brain and behavior of the honey bee, Apis mellifera (L.). J. Neurosci. 17, 7148–7156. doi: 10.1523/jneurosci.17-18-07148.1997
Simões, P. M. V., Ott, S. R., and Niven, J. E. (2016). Environmental adaptation, phenotypic plasticity, and associative learning in insects: the desert locust as a case study. Integr. Comp. Biol. 56, 914–924. doi: 10.1093/icb/icw100
Simpson, S. J., McCaffery, A. R., and Hägele, B. F. (1999). A behavioural analysis of phase change in the desert locust. Biol. Rev. 74, 461–480. doi: 10.1017/S000632319900540X
Singh, N. R., and Nayak, S. V. (1985). Fine structure and primary sensory projections of sensilla on the maxillary palp of Drosophila melanogaster Meigen (Diptera : Drosophilidae). Int. J. Insect Morphol. Embryol. 14, 291–306. doi: 10.1016/0020-7322(85)90044-3
Smith, A. R., Seid, M. A., Jiménez, L. C., and Wcislo, W. T. (2010). Socially induced brain development in a facultatively eusocial sweat bee Megalopta genalis (Halictidae). Proc. R. Soc. B Biol. Sci. 277, 2157–2163. doi: 10.1098/rspb.2010.0269
Steinbrecht, R. A. (1997). Pore structures in insect olfactory sensilla: a review of data and concepts. Int. J. Insect Morphol. Embryol. 26, 229–245. doi: 10.1016/S0020-7322(97)00024-X
Steinbrecht, R. A. (1998). Odorant-binding proteins: expression and function. Ann. N.Y. Acad. Sci. 855, 323–332. doi: 10.1111/j.1749-6632.1998.tb10591.x
Steinhoff, P. O. M., Liedtke, J., Sombke, A., Schneider, J. M., and Uhl, G. (2018). Early environmental conditions affect the volume of higher-order brain centers in a jumping spider. J. Zool. 304, 182–192. doi: 10.1111/jzo.12512
Stensmyr, M. C., Dweck, H. K., Farhan, A., Ibba, I., Strutz, A., Mukunda, L., et al. (2012). A conserved dedicated olfactory circuit for detecting harmful microbes in Drosophila. Cell 151, 1345–1357. doi: 10.1016/j.cell.2012.09.046
Stocker, R. F. (1994). The organization of the chemosensory system in Drosophila melanogaster: a rewiew. Cell Tissue Res. 275, 3–26. doi: 10.1007/BF00305372
Stocker, R. F., Lienhard, M. C., Borst, A., and Fischbach, K. F. (1990). Neuronal architecture of the antennal lobe in Drosophila melanogaster. Cell Tissue Res. 262, 9–34. doi: 10.1007/BF00327741
Stocker, R. F., Singh, R. N., Schorderet, M., and Siddiqi, O. (1983). Projection patterns of different types of antennal sensilla in the antennal glomeruli of Drosophila melanogaster. Cell Tissue Res. 232, 237–248. doi: 10.1007/BF00213783
Strausfeld, N. J., Sinakevitch, I., Brown, S. M., and Farris, S. M. (2009). Ground plan of the insect mushroom body: functional and evolutionary implications. J. Comp. Neurol. 513, 265–291. doi: 10.1002/cne.21948
Strutz, A., Soelter, J., Baschwitz, A., Farhan, A., Grabe, V., Rybak, J., et al. (2014). Decoding odor quality and intensity in the Drosophila brain. eLife 3:e04147. doi: 10.7554/eLife.04147
Sudhakaran, I. P., Hillebrand, J., Dervan, A., Das, S., Holohan, E. E., Hulsmeier, J., et al. (2013). FMRP and Ataxin-2 function together in long-term olfactory habituation and neuronal translational control. Proc. Natl. Acad. Sci. U.S.A. 111, E99–E108. doi: 10.1073/pnas.1309543111
Sugie, A., Marchetti, G., and Tavosanis, G. (2018). Structural aspects of plasticity in the nervous system of Drosophila. Neural Dev. 13:14. doi: 10.1186/s13064-018-0111-z
Sun, Y., Qiu, R., Li, X., Cheng, Y., Gao, S., Kong, F., et al. (2020). Social attraction in Drosophila is regulated by the mushroom body and serotonergic system. Nat. Commun. 11:5350. doi: 10.1038/s41467-020-19102-3
Tanaka, N. K., Awasaki, T., Shimada, T., and Ito, K. (2004). Integration of chemosensory pathways in the Drosophila second-order olfactory centers. Curr. Biol. 14, 449–457. doi: 10.1016/j.cub.2004.03.006
Tanaka, N. K., Endo, K., and Ito, K. (2012). Organization of antennal lobe-associated neurons in adult Drosophila melanogaster brain. J. Comp. Neurol. 520, 4067–4130. doi: 10.1002/cne.23142
Tanaka, N. K., Tanimoto, H., and Ito, K. (2008). Neuronal assemblies of the Drosophila mushroom body. J. Comp. Neurol. 508, 711–755. doi: 10.1002/cne.21692
Task, D., Lin, C.-C., Vulpe, A., Afify, A., Ballou, S., Brbic, M., et al. (2022). Chemoreceptor co-expression in Drosophila melanogaster olfactory neurons. eLife 11:e72599. doi: 10.7554/eLife.72599
Technau, G., and Heisenberg, M. (1982). Neural reorganization during metamorphosis of the corpora pedunculata in Drosophila melanogaster. Nature 295, 405–407. doi: 10.1038/295405a0
Thiagarajan, D., and Sachse, S. (2022). Multimodal information processing and associative learning in the insect brain. Insects 13:332.
Thomson, C. G. (1862). Försök till uppställning och beskrifning av Sveriges Figiter. Öfversigt af Konglelige Vetenskaps-Akademiens Förhandlingar 18, 395–420.
Tresca, H. E. (1864). Mémoire sur l’écoulement des corps solides soumis á de fortes pressions. C. R. Acad. Sci. Paris 59, 754–758.
Ukani, N. H., Tomkins, A., Yeh, C.-H., Bruning, W., Fenichel, A. L., Zhou, Y., et al. (2016). NeuroNLP: a natural language portal for aggregated fruit fly brain data. bioRxiv [Preprint]. doi: 10.1101/092429
van Dijk, L. J. A., Janz, N., Schäpers, A., Gamberale-Stille, G., and Carlsson, M. A. (2017). Experience-dependent mushroom body plasticity in butterflies: consequences of search complexity and host range. Proc. R. Soc. B Biol. Sci. 284:20171594. doi: 10.1098/rspb.2017.1594
Vogt, K., Aso, Y., Hige, T., Knapek, S., Ichinose, T., Friedrich, A. B., et al. (2016). Direct neural pathways convey distinct visual information to Drosophila mushroom bodies. eLife 5:e14009. doi: 10.7554/eLife.14009
Vogt, R. G., and Riddiford, L. M. (1981). Pheromone binding and inactivation by moth antennae. Nature 293, 161–163. doi: 10.1038/293161a0
Vosshall, L. B., Wong, A. M., and Axel, R. (2000). An olfactory sensory map in the fly brain. Cell 102, 147–159. doi: 10.1016/S0092-8674(00)00021-0
Wang, Y., Chiang, A.-S., Xia, S., Kitamoto, T., Tully, T., and Zhong, Y. (2003). Blockade of neurotransmission in Drosophila mushroom bodies impairs odor attraction, but not repulsion. Curr. Biol. 13, 1900–1904. doi: 10.1016/j.cub.2003.10.003
Wharton, K. A., Johansen, K. M., Xu, T., and Artavanis-Tsakonas, S. (1985). Nucleotide sequence from the neurogenic locus Notch implies a gene product that shares homology with proteins containing EGF-like repeats. Cell 43(3, Part 2), 567–581. doi: 10.1016/0092-8674(85)90229-6
Wilkin, M. B., Carbery, A.-M., Fostier, M., Aslam, H., Mazaleyrat, S. L., Higgs, J., et al. (2004). Regulation of notch endosomal sorting and signaling by Drosophila Nedd4 family proteins. Curr. Biol. 14, 2237–2244. doi: 10.1016/j.cub.2004.11.030
Will, B., Dalrymple-Alford, J., Wolff, M., and Cassel, J.-C. (2008). The concept of brain plasticity—Paillard’s systemic analysis and emphasis on structure and function (followed by the translation of a seminal paper by Paillard on plasticity). Behav. Brain Res. 192, 2–7. doi: 10.1016/j.bbr.2007.11.030
Wilson, R. I., and Laurent, G. (2005). Role of GABAergic inhibition in shaping odor-evoked spatiotemporal patterns in the Drosophila antennal lobe. J. Neurosci. 25, 9069–9079. doi: 10.1523/jneurosci.2070-05.2005
Winnington, A. P., Napper, R. M., and Mercer, A. R. (1996). Structural plasticity of identified glomeruli in the antennal lobes of the adult worker honey bee. J. Comp. Neurol. 365, 479–490. doi: 10.1002/(sici)1096-9861(19960212)365:3<479::aid-cne10<3.0.co;2-m
Withers, G. S., Day, N. F., Talbot, E. F., Dobson, H. E. M., and Wallace, C. S. (2008). Experience-dependent plasticity in the mushroom bodies of the solitary bee Osmia lignaria (Megachilidae). Dev. Neurobiol. 68, 73–82. doi: 10.1002/dneu.20574
Withers, G. S., Fahrbach, S. E., and Robinson, G. E. (1993). Selective neuroanatomical plasticity and division of labour in the honeybee. Nature 364:238. doi: 10.1038/364238a0
Wong, A. M., Wang, J. W., and Axel, R. (2002). Spatial representation of the glomerular map in the Drosophila protocerebrum. Cell 109, 229–241. doi: 10.1016/S0092-8674(02)00707-9
Yagi, R., Mabuchi, Y., Mizunami, M., and Tanaka, N. K. (2016). Convergence of multimodal sensory pathways to the mushroom body calyx in Drosophila melanogaster. Sci. Rep. 6:29481. doi: 10.1038/srep29481
Yaksi, E., and Wilson, R. I. (2010). Electrical coupling between olfactory glomeruli. Neuron 67, 1034–1047. doi: 10.1016/j.neuron.2010.08.041
Yao, C. A., Ignell, R., and Carlson, J. R. (2005). Chemosensory coding by neurons in the coeloconic sensilla of the Drosophila antenna. J. Neurosci. 25, 8359–8367. doi: 10.1523/jneurosci.2432-05.2005
Yasuyama, K., and Salvaterra, P. M. (1999). Localization of choline acetyltransferase-expressing neurons in Drosophila nervous system. Microsc. Res. Technique 45, 65–79. doi: 10.1002/(SICI)1097-0029(19990415)45:2<65::AID-JEMT2<3.0.CO;2-0
Yasuyama, K., Meinertzhagen, I. A., and Schürmann, F.-W. (2002). Synaptic organization of the mushroom body calyx in Drosophila melanogaster. J. Comp. Neurol. 445, 211–226. doi: 10.1002/cne.10155
Zheng, Z., Lauritzen, J. S., Perlman, E., Robinson, C. G., Nichols, M., Milkie, D., et al. (2018). A complete electron microscopy volume of the brain of adult Drosophila melanogaster. Cell 174, 730–743.e22. doi: 10.1016/j.cell.2018.06.019
Ziegelberger, G. (1995). Redox-shift of the pheromone-binding protein in the silkmoth Antheraea polyphemus. Eur. J. Biochem. 232, 706–711. doi: 10.1111/j.1432-1033.1995.706zz.x
Keywords: olfaction, plasticity, odor experience, Drosophila, insects, antennal lobe
Citation: Fabian B and Sachse S (2023) Experience-dependent plasticity in the olfactory system of Drosophila melanogaster and other insects. Front. Cell. Neurosci. 17:1130091. doi: 10.3389/fncel.2023.1130091
Received: 22 December 2022; Accepted: 07 February 2023;
Published: 22 February 2023.
Edited by:
Bente Gunnveig Berg, Norwegian University of Science and Technology, NorwayReviewed by:
Moshe Parnas, Tel Aviv University, IsraelPeter Kloppenburg, University of Cologne, Germany
Copyright © 2023 Fabian and Sachse. This is an open-access article distributed under the terms of the Creative Commons Attribution License (CC BY). The use, distribution or reproduction in other forums is permitted, provided the original author(s) and the copyright owner(s) are credited and that the original publication in this journal is cited, in accordance with accepted academic practice. No use, distribution or reproduction is permitted which does not comply with these terms.
*Correspondence: Silke Sachse, c3NhY2hzZUBpY2UubXBnLmRl