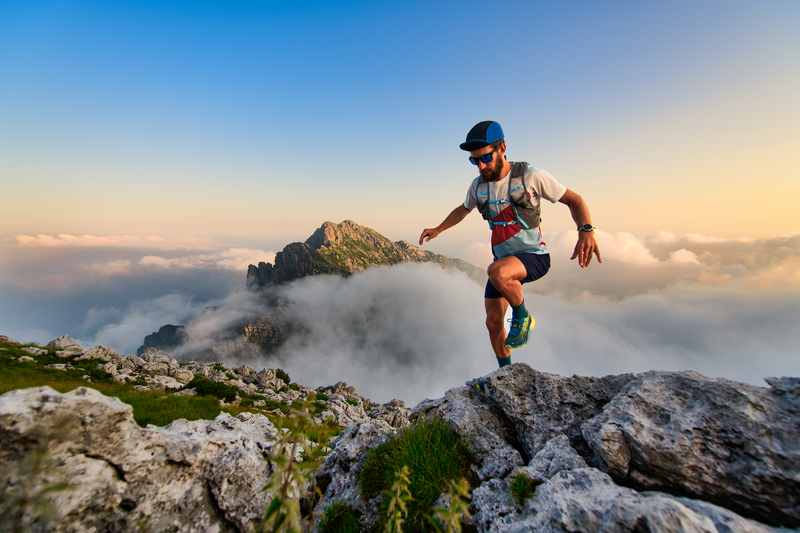
95% of researchers rate our articles as excellent or good
Learn more about the work of our research integrity team to safeguard the quality of each article we publish.
Find out more
REVIEW article
Front. Cell. Neurosci. , 15 February 2023
Sec. Non-Neuronal Cells
Volume 17 - 2023 | https://doi.org/10.3389/fncel.2023.1101379
This article is part of the Research Topic The Brain Barriers in Diseases of the Nervous System View all 7 articles
The blood–brain barrier (BBB) and the blood-cerebrospinal fluid barrier (BCSFB) represent two complex structures protecting the central nervous system (CNS) against potentially harmful agents and circulating immune cells. The immunosurveillance of the CNS is governed by immune cells that constantly patrol the BCSFB, whereas during neuroinflammatory disorders, both BBB and BCSFB undergo morphological and functional alterations, promoting leukocyte intravascular adhesion and transmigration from the blood circulation into the CNS. Multiple sclerosis (MS) is the prototype of neuroinflammatory disorders in which peripheral T helper (Th) lymphocytes, particularly Th1 and Th17 cells, infiltrate the CNS and contribute to demyelination and neurodegeneration. Th1 and Th17 cells are considered key players in the pathogenesis of MS and its animal model, experimental autoimmune encephalomyelitis. They can actively interact with CNS borders by complex adhesion mechanisms and secretion of a variety of molecules contributing to barrier dysfunction. In this review, we describe the molecular basis involved in the interactions between Th cells and CNS barriers and discuss the emerging roles of dura mater and arachnoid layer as neuroimmune interfaces contributing to the development of CNS inflammatory diseases.
The central nervous system (CNS) is protected against potentially harmful molecules and circulating immune cells by two distinct biological barriers localized at its borders: the blood–brain barrier (BBB) and the blood-cerebrospinal fluid barrier (BCSFB) (Mastorakos and McGavern, 2019). Under physiological conditions, CNS immunosurveillance is mediated by a low and strictly controlled number of immune cells that constantly patrols the BCSFB by migrating through pial vessels or crossing the choroid plexus (Prinz and Priller, 2017). However, during neuroinflammatory diseases, both BBB and BCSFB undergo morphological and functional alterations, promoting leukocyte migration from the blood stream into the CNS parenchyma and CSF and consequent glial and neuronal dysfunction (Solár et al., 2020; Takata et al., 2021).
Multiple sclerosis (MS) represents the most common chronic inflammatory disorder affecting the CNS, and it is characterized by multifocal perivascular inflammatory infiltrates, gliosis, progressive myelin loss, and axon degeneration (Compston and Coles, 2008). It is one of the most widespread causes of neurological disability in young adults, arising usually between 20 and 40 years of age and affecting women about twice as often as men (Compston and Coles, 2008). Although the etiopathology is still unknown, MS is a complex multifactorial disorder, in which genetic susceptibility and environmental factors, such as infectious agents, play a key role in disease development (Sospedra and Martin, 2005). In most clinical cases (85–90%), the disease starts as a relapsing-remitting disorder (RRMS), characterized by transient episodes of neurologic dysfunction and acute lesions in which inflammation is the main component. Chronic forms of the disease are mainly defined by neurodegeneration and brain atrophy with a minor inflammatory component, and can result from a gradual worsening of the RRMS course [secondary progressive MS (SPMS)] or start from the disease onset as primary progressive MS (PPMS) (Kaskow and Baecher-Allan, 2018; Tillery et al., 2018).
Multiple sclerosis has long been supposed to be mediated by an autoimmune process (Rivers et al., 1933; Kabat et al., 1947; Sospedra and Martin, 2005). A crucial role for CD4+ lymphocytes was suggested by pioneering studies in experimental autoimmune encephalomyelitis (EAE), an animal model of MS (Ben-Nun et al., 1981; Zamvil et al., 1985). The contribution of CD4+ T cells has been further dissected by using the 2D2 transgenic mouse strain, whose T cell receptor repertoire biased toward the myelin oligodendrocyte glycoprotein (MOG) 35–55 antigen spontaneously determines the onset of CNS autoimmunity (Bettelli et al., 2003). The genetic risk related to the major histocompatibility complex (MHC) class II locus (Barcellos et al., 2003; Sawcer et al., 2005), along with the local inflammatory response orchestrated particularly by T helper (Th) 1 and Th17 cells (Kunkl et al., 2020), prompted the scientific community to investigate the specific contribution of activated T cell subpopulations in the pathogenesis of MS. A higher number of different populations of activated T cells was found in the CNS parenchyma, CSF and blood of MS patients compared to control subjects indicating that these cells have the capacity to cross brain borders (Wallström et al., 2000; Arbour et al., 2003; Brucklacher-Waldert et al., 2009; El-Behi et al., 2011). These observations opened numerous research lines using the EAE model to elucidate the mechanisms controlling T cell trafficking into the inflamed CNS.
Pathogenic features, clinical symptoms and disease course are highly variable in MS patients, suggesting that multiple mechanisms can contribute to disease development. Two main hypotheses on the role of immune cells in the etiopathogenesis of MS have been proposed to date. One is the “outside-in” hypothesis supporting the idea that autoreactive CD4+ T lymphocytes activate in peripheral lymphoid organs during infections or other inflammatory reactions, supposedly due to molecular mimicry or bystander activation, and then reach the “naïve” CNS starting local autoimmune responses and neuroinflammation. On the other hand, the “inside-out” hypothesis suggests that the pathological process begins within the CNS, leading to the release of highly antigenic constituents that secondarily promote an autoimmune and neuroinflammatory response in predisposed individuals (Stys et al., 2012; Sen et al., 2020). Dysfunctional brain barriers may represent critical contributors to both scenarios, either promoting the onset of the peripheral immune attack according to the “outside-in” hypothesis or sustaining the “inside-out” pathway by facilitating peripheral recruitment of ancillary proinflammatory and autoimmune cells. Moreover, in both circumstances, the pathological outcome of BBB and BCSFB breakdown contributes to myelin and axonal loss and, consequently, to neurodegeneration and neurological impairment (Engelhardt, 2010).
The BBB represents a specialized layer of endothelial cells with tight junctions sealing cell-to-cell contacts and regulating the passage of cells and molecules between the blood and the CNS. Together, pericytes, astrocytes, microglial cells, neurons, and endothelial cells contribute to the neurovascular unit, a key anatomical and functional structure for the maintenance of CNS homeostasis (Zenaro et al., 2017; Saint-Pol et al., 2020). BBB dysfunction is considered a pathological hallmark in several inflammatory and neurodegenerative disorders, including MS, since this barrier is one of the gateways to the CNS for circulating leukocytes (Prinz and Priller, 2017). BBB perturbation upon neuroinflammatory disorders is associated with two main processes: (i) alteration of junctional molecules leading to BBB breakdown and vascular leakage, and (ii) endothelial activation with upregulation of adhesion molecules and chemokines driving leukocyte recruitment into the brain parenchyma and thus favoring their subsequent local reactivation. While migration of autoreactive T cells into the CNS is required to mount an autoimmune response, other activated T lymphocytes can infiltrate the CNS regardless of antigen specificity, contributing to the inflammation process (Hickey et al., 1991). In support of these data, it was recently shown that in a passive EAE model most invading CD4+ T cells were not myelin-specific. Particularly, these lymphocytes displayed an antigen-independent, bystander-activated, memory phenotype and contributed to disease pathology by expanding the local production of pro-inflammatory cytokines (Lee et al., 2019).
Magnetic resonance imaging (MRI), using gadolinium (Gd) as a marker of cerebral vascular leakage, is a diagnostic tool and a prognostic evaluator for MS (Filippi, 2000; Steinman, 2001; Leray et al., 2010; Polman et al., 2011). Previous studies have shown that BBB alterations may appear at very early disease stages, preceding active lesion formation, and clinical manifestation (Filippi et al., 1998; Plumb et al., 2002; Soon et al., 2007; Alvarez et al., 2015; Barkauskas et al., 2015). A recent report based on elegant in vitro experiments even speculates that an intrinsic BBB malfunctioning could represent an additional pathogenetic mechanism for the development of MS (Nishihara et al., 2022). Further, in RRMS patients, MRI clearly indicates how areas of BBB disruption are topologically heterogenous and coincide with perivascular inflammation and demyelinating lesions during relapses (Miller et al., 1998; Treabă et al., 2014). This suggests that BBB dysfunction is an early feature of MS and may favor T cell transendothelial migration and subsequent immune attack contributing to clinical worsening (Ortiz et al., 2014; Spencer et al., 2018). Moreover, as previously shown in animal models, leukocyte-BBB adhesive interactions during the process of leukocyte extravasation in the CNS may lead to vascular inflammation and further BBB impairment, amplifying neuroinflammation and promoting neuronal damage (Siffrin et al., 2010; Rossi et al., 2011, 2021; Zenaro et al., 2013; Rossi and Constantin, 2016; Spencer et al., 2018).
Multiple sclerosis evolution to neurodegeneration and brain atrophy during chronic forms seems to be less associated to BBB alterations and more to brain-compartmentalized self-sustaining pathological processes (Steinman, 2001; Leray et al., 2010). Indeed, the absence of Gd-enhancing lesions and the paucity of therapeutical responses in the progressive stages of MS suggested that the BBB could regain integrity during disease progression (Coles et al., 1999; Molyneux et al., 2000; Filippi and Rocca, 2005; Anderson et al., 2006; Waxman, 2008; Young et al., 2008), whereas brain-compartmentalized inflammation could be fostered by meningeal lymphoid follicles (Serafini et al., 2004; Meinl et al., 2008; Choi et al., 2012). Nevertheless, several observations, such as cortical deposition of fibrinogen and diffuse tight junction aberrations, argue that BBB dysfunction can still be present in the progressive forms of MS (Leech et al., 2007; Yates et al., 2017).
Lymphocyte trafficking into the inflamed tissues represents a critical event in the pathogenesis of autoimmune diseases. Based on in vitro and in vivo evidence, leukocyte migration through the vascular wall is described as a sequential process including distinct adhesive events: tethering, rolling, chemoattractant-dependent activation of integrins, slow rolling, firm adhesion (also called arrest or sticking), crawling, and diapedesis (Ley et al., 2007; Vestweber, 2015). Specificity and diversity in leukocyte-endothelial cell interactions are generated by different combinations of interchangeable ligand-receptor pairs for each step of the adhesion cascade. The molecular specificity directing leukocytes to the site of inflammation is regulated by adhesion molecules such as selectins, integrins, and members of the immunoglobulin superfamily. A critical step in the transition from leukocyte rolling to arrest is the induction of firm adhesion by chemokines expressed on activated endothelium. Chemokine receptors on the surface of rolling immune cells bind to their cognate ligand, triggering a G protein-dependent signaling that leads to the activation of membrane-expressed β1 and β2 integrins (Montresor et al., 2012). At the BBB level, leukocyte extravasation follows the standard paradigm of cell migration, and the inhibition of adhesion mechanisms using different therapeutical approaches proved to be beneficial in several models of brain inflammatory conditions (Yednock et al., 1992; Piccio et al., 2002; Constantin, 2008; Rossi et al., 2011; Fabene et al., 2013; Zenaro et al., 2015; Farinazzo et al., 2018).
Adhesion molecules expressed by brain endothelial cells have been studied for three decades for their role in lymphocyte trafficking during EAE and MS. P-selectin is upregulated on CNS vasculature during EAE (Piccio et al., 2002, 2005; Döring et al., 2007), whereas E-selectin was found expressed on autoptic cerebral micro-vessels of MS patients (Washington et al., 1994). In support of these data, in vitro studies have shown that P-selectin glycoprotein ligand (PSGL)-1, the major P-selectin ligand, is involved in rolling of CD8+ T cells isolated from MS patients and contributes to the transendothelial migration of MS-derived CD4+ T cells (Battistini et al., 2003; Bahbouhi et al., 2009). Moreover, MRI targeting of P-selectin with iron oxide-conjugated antibodies, to evaluate the modulation of its expression on the endothelium during inflammation, was found useful as a predictive method of EAE activity (Fournier et al., 2017). However, blockade of P- and E-selectin had no therapeutic effect on EAE models (Engelhardt et al., 2005; Döring et al., 2007). Intriguingly, combined blockade of P-selectin and α4 integrins resulted in significantly better clinical outcome than anti-α4 integrin alone in EAE mice, suggesting that selectin blockade may have some therapeutic effect in MS as well (Kerfoot et al., 2006). Together, these data suggest that further studies are needed to better understand the potential pathological role of endothelial selectins in MS.
Cellular adhesion molecules (CAM) belonging to the immunoglobulin superfamily such as vascular CAM-1 (VCAM-1), intercellular CAM-1 (ICAM-1), activated leukocyte CAM (ALCAM), and platelet endothelial CAM-1 (PECAM-1) are upregulated on the CNS vasculature, enhancing leukocyte adhesion and migration to the brain in both EAE and MS (Steffen et al., 1994; Cayrol et al., 2008; Steiner et al., 2010; Greenwood et al., 2011; Wimmer et al., 2019). The involvement of VCAM-1 and ICAM-1 is confirmed by the high expression of very late antigen (VLA)-4 and lymphocyte function-activated antigen (LFA)-1, their main ligands, respectively, on the immune infiltrates populating MS lesions (Cannella and Raine, 1995; Bö et al., 1996). ICAM-1 expression on neuro-vasculature was found to strongly correlate with relapses in RRMS patients, while the blood levels of its soluble form is associated with the degree of BBB impairment and disease activity in MS subjects (Cannella et al., 1990; Hartung et al., 1995). Although VLA-4 can also bind fibronectin with low affinity, its high affinity ligand VCAM-1 is believed to have a central role in encephalitogenic T cell migration into the CNS, as suggested by the strong blocking effect of anti-α4 integrin and anti-VCAM-1 antibodies on EAE development, corroborated by the therapeutic success of Natalizumab in MS patients (Yednock et al., 1992; Baron et al., 1993; Chan and Aruffo, 1993; Steffen et al., 1994; Miller et al., 2003; Belachew et al., 2011). Moreover, ALCAM and its counter-ligand CD6 were correlated to an increased risk of MS development (Wagner et al., 2014), further emphasizing the relevance of CAMs in MS.
Chemokines are fundamental molecules for integrin activation and leukocyte migration into the CNS (Constantin, 2008). C-C motif chemokine ligand 19 (CCL19) and CCL21, two chemokines able to induce integrin activation, were found to be expressed on CNS endothelial cells of post-capillary venules in both healthy and EAE mice, and these data correlated to C-C chemokine receptor type 7 (CCR7) expression on perivascular T cells (Alt et al., 2002). However, cerebral expression of CCL19 and CCL21 was not found in MS subjects, suggesting that different chemokines may be responsible for triggering intravascular integrin activation during human disease (Kivisäkk et al., 2004). Several studies have instead identified a constitutive expression of C-X-C motif chemokine ligand 12 (CXCL12) on BBB endothelial cells in EAE mice and MS patients (Krumbholz et al., 2006; McCandless et al., 2006, 2008). Particularly, a CXCL12 expression gradient was detected along the abluminal BBB surface in healthy animals and during early EAE, as well as in uninflamed regions of the MS brain and in control subjects. However, a preferential luminal localization of CXCL12 was found at EAE peak and in active MS lesions, suggesting a role for this chemokine in integrin activation and intravascular leukocyte adhesion (McCandless et al., 2006, 2008). Indeed, in both MS and EAE, the intravascular expression of CXCL12 was associated to the presence of intraluminal CXCR4+ adhering leukocytes as well as to CXCR4 expression on perivascular infiltrating leukocytes (McCandless et al., 2008). Previous studies have also suggested an involvement of CXCR4 in the confinement of infiltrated T cells at the perivascular level, promoting their compartmentalization around blood vessels and preventing widespread parenchymal infiltration (McCandless et al., 2006; Siffrin et al., 2009). Moreover, CXCR7, an alternative receptor for CXCL12, is present on endothelial cells within the CNS and its expression is increased in EAE at sites of inflammatory infiltration (Cruz-Orengo et al., 2011). Notably, CXCR7 was shown to be critical in mediating CXCL12 internalization and redistribution at CNS endothelial barriers and CXCR7 inhibition ameliorated EAE and reduced leukocyte infiltration into the CNS parenchyma (Cruz-Orengo et al., 2011). CCL2, CCL4, and CCL5 were also found to be expressed on cultured brain endothelial cell suggesting that multiple chemokines may contribute to integrin activation and subsequent arrest in inflamed CNS vessels, even if their expression on the BBB and potential role during in vivo CNS inflammation remains to be elucidated (Andjelkovic et al., 1999; Quandt and Dorovini-Zis, 2004).
During inflammatory conditions, leukocyte adhesion on vascular wall per se may lead to increased endothelial permeability (Figure 1). Accordingly, the overexpression of endothelial CAMs during neuroinflammation was shown to promote not only leukocyte adhesion, but also BBB dysfunction and increased permeability. Indeed, ICAM-1 cross-linking leads to reorganization of endothelial cytoskeleton and tight junctions phosphorylation and destabilization, favoring leukocyte adhesion and increasing BBB leakage (Durieu-Trautmann et al., 1994; Adamson et al., 1999). In this context, Rho/ROCK and Rac downstream signals are central modulators of endothelial alterations induced by ICAM-1 and VCAM-1 crosslinking during leukocyte adhesive contacts. Particularly, ICAM-1 engagement elicits RhoA activation and intracellular calcium increase with subsequent reactive oxygen species (ROS) production, actomyosin contraction, and formation of stress fibers (Etienne et al., 1998; Etienne-Manneville et al., 2000; Wang and Doerschuk, 2000). On the other hand, VCAM-1 clustering triggers Rac1 activation and intracellular calcium release, allowing ROS generation, transient disruption of adherent junctions and focal adhesion formation (Lorenzon et al., 1998; Matheny et al., 2000; van Wetering et al., 2003). The relevance of RhoA signaling was confirmed in vivo, since its blockade attenuated EAE severity by reducing leukocyte migration into the CNS (Greenwood et al., 2003). This activating signaling within the cerebral endothelium deserves further investigation as a potential molecular target to prevent leukocyte CNS invasion and safeguard BBB integrity in MS. In addition to ICAM-1 and VCAM-1, ALCAM was also found to be present on CNS endothelium during MS and EAE and its expression was associated to T cell migration (Cayrol et al., 2008). However, other studies performed in a chronic EAE model demonstrated that ALCAM maintains BBB integrity by controlling tight junction stability (Lécuyer et al., 2017), suggesting a dual function of ALCAM in neuroinflammation as well as BBB homeostasis (Cayrol et al., 2008; Lyck et al., 2017). Similarly, it was shown that PECAM-1 favors T cell diapedesis across the BBB during neuroinflammation, but also stabilizes BBB integrity (Graesser et al., 2002; Wimmer et al., 2019). Together, these findings suggest that, in addition to the inhibition of leukocyte trafficking into the CNS, BBB stabilization and tight junctions recovery may also represent a therapeutic strategy in brain inflammatory and demyelinating diseases (Spencer et al., 2018).
Figure 1. Leukocyte intravascular adhesion contributes to vascular leakage during EAE. In vivo two photon laser scanning microscopy was performed on exposed spinal cord of an EAE mouse at disease peak. Pial vessels were visualized through the intravenous injection of 70,000 kDa molecular weight FITC-dextran. White arrows indicate leakage of FITC-dextran into the extravascular space at the point where circulating leukocytes (detected by the imaging system as black dots) perform intravascular adhesion. Representative sequential images at T0 (A), after 40 s (B), and 80 s (C). Adapted and modified from Dusi et al., 2019, (supplementary videos 1 and 2).
Multiple sclerosis has been described as a T cell-mediated autoimmune disease and its widely used model, EAE, is characterized by a Th1 and Th17-driven autoimmune and neuroinflammatory process (Segal, 2019). The study of recruitment kinetics of Th1 and Th17 cells into the CNS during EAE led to the publication of some contrasting reports, probably due to the in vivo phenotype plasticity of these cells (Geginat et al., 2014). Whereas some EAE studies indicated Th1 lymphocytes as the earliest CD4+ T cells infiltrating the CNS, followed by secondary Th17 migration (O’Connor et al., 2008), more recent findings suggested that Th17 are the pioneer lymphocytes with a later accumulation of Th1 cells during EAE development (Murphy et al., 2010). Accordingly, in vitro migration studies support a preferential capacity of Th17 cells to migrate across the BBB when compared to Th1 lymphocytes (Kebir et al., 2007), but the precise kinetics of Th cell infiltration into the CNS in MS and EAE still remains a matter of debate.
To date, Natalizumab, a monoclonal antibody that blocks activated T cell trafficking targeting the α4 chain of VLA-4 integrin, is one of the most efficient treatments for MS (Polman et al., 2006), demonstrating the involvement of VLA-4 in leukocyte migration into the CNS (Schneider-Hohendorf et al., 2014). However, while the blockade of α4 integrin completely abolishes Th1 recruitment and partially reduces Th17 cell accumulation in the spinal cord of EAE mice, it fails to prevent Th17 lymphocyte entrance into the cerebrum in an experimental EAE model with atypical brain manifestations (Rothhammer et al., 2011). Under flow adhesion assays using an in vitro BBB model confirmed that VCAM-1, the main VLA-4 ligand, is required for the arrest of murine Th1 cells (Steiner et al., 2010). β2 integrins have also a role in firm adhesion and crawling of Th1 lymphocytes on the BBB in in vitro models, whereas LFA-1 expression on human Th17 cells has a critical contribution to transmigration across interferon (IFN)-γ activated BBB, suggesting that both VLA-4 and LFA-1 integrins control Th lymphocyte trafficking into the brain in MS (Kebir et al., 2009; Steiner et al., 2010). Intriguingly, in MS patients treated with Natalizumab, Th cells overcome α4 blockade by upregulating alternative adhesion receptors, such as PSGL-1 and melanoma CAM (MCAM) (Schneider-Hohendorf et al., 2014). MCAM binds to laminin 411, a component of the endothelial basement membrane, facilitating T lymphocyte penetration into the parenchyma during EAE (Sixt et al., 2001; Wu et al., 2009). Recently, the dual immunoglobulin domain containing CAM (DICAM) was shown to be preferentially expressed on Th17 cells contributing to their migration and pathogenic capacity during EAE. Interestingly, DICAM is particularly expressed on circulating CD4+ T lymphocytes of MS patients and, together with its ligand αVβ3, is upregulated on lesion-associated BBB, further suggesting a role for this molecules in Th cell migration during EAE and MS (Du et al., 2016; Charabati et al., 2022).
Interferon-γ, the main Th1-related cytokine, is massively produced during neuroinflammatory conditions. The analysis of blood, CSF, and brain tissue samples from MS patients clearly supported the pathogenic role of Th1 cells and their signature cytokine in disease development. Particularly, increased frequency of IFN-γ-producing myelin-reactive T lymphocytes in the blood of MS subjects correlated with the active phase of disease and the worsening of neurological symptoms (Arbour et al., 2003; Moldovan et al., 2003). IFN-γ+ T cells were also found to be enriched in the CSF of MS patients compared to healthy controls (Wallström et al., 2000). Accordingly, IFN-γ levels were significantly elevated in the blood, CSF and CNS lesions of subjects with MS (Mycko et al., 2003; Arellano et al., 2017). The pathogenic effect of IFN-γ is corroborated by an early pilot study evaluating the efficacy of recombinant IFN-γ administration to RRMS patients, who showed a significantly higher exacerbation rate with respect to pre- and post-treatment rates (Panitch et al., 1987).
The overall picture emerging from in vitro and in vivo observations is that IFN-γ alone is able to regulate the expression of several surface molecules on brain endothelium including MHCI, MHCII, programmed death-ligand (PD-L)1 VCAM-1, mucosal vascular addressin (Mad)CAM-1 and ICAM-1 (Brown et al., 2007; Sonar et al., 2017; Figure 2 and Table 1). Interestingly, time-lapse confocal microscopy experiments showed that IFN-γ, rather than inducing ICAM-1 overexpression, determined a rapid re-localization of this molecule from the basal to the apical side of endothelial cells, potentially promoting leukocyte adhesion (Sonar et al., 2017). Notably, in vitro and in vivo data suggested that IFN-γ promote BBB leakage by inducing STAT-1 expression and cytoskeleton remodeling affecting tight junction protein organization (Sonar et al., 2017; Bonney et al., 2019). Also, exposure to IFN-γ promotes endothelial permeability through zonula occludens (ZO)-1 and claudin (CLDN) 5 delocalization and adherent junction molecule VE-cadherin perturbation in an in vitro system using bEnd3.1 cell line, and these changes were associated to a relevant increase of actin stress fibers and cytoskeletal contraction (Sonar et al., 2017; Liu et al., 2018). In vitro inhibition of Rho kinase (ROCK) was sufficient to restore the integrity of brain endothelial cells during IFN-γ treatment by blocking the junctional localization of phosphorylated myosin light chain (Bonney et al., 2019), a phenomenon linked to weakened cell-cell interactions (Hirano and Hirano, 2016). In line with this, in vivo ROCK inhibition induced the upregulation of occludin and ZO-1 tight junction proteins on cerebral vessels, making this drug a valuable candidate to tackle BBB breakdown in MS (Yan et al., 2019).
Figure 2. Modulation of BBB permeability and function by T helper cell cytokines. Encephalitogenic T cells communicate with brain vasculature in a paracrine fashion secreting cytokines. During neuroinflammation, IL-17, and IL-22 exert a detrimental effect on brain endothelium functioning. The exposure to these cytokines triggers the loosening of the vascular barrier along with increased expression of adhesion molecules and leukocyte recruitment. Also, although it has been described as an anti-inflammatory cytokine, IL-4 triggers a loss of BBB integrity coupled with changes in endothelial cell morphology. Conversely, IL-26 induces a downregulation of pro-inflammatory and oxidative pathways in endothelial cells, concomitantly stimulating the upregulation of tight junction protein transcripts. IFN-γ can contribute to both BBB breakdown and sealing. Created with BioRender.com.
However, the detrimental effect of IFN-γ on BBB integrity was challenged by Ni et al. (2014), whose findings suggested a role for IFN-γ in inducing the clinching of brain endothelial junctions. In particular, these authors showed that IFN-γ treatment of bEnd3.1 cells enhances their paracellular tightness in a dose-dependent manner and dramatically reduces splenocyte transmigration, with CLDN 5 being essential to link cytokine-dependent signaling to junctional strengthening (Ni et al., 2014). Additionally, exposure of murine brain endothelial cells to IFN-γ decreased CXCR7 expression and led to a reduction of CXCL12 internalization (Cruz-Orengo et al., 2011). Interestingly, blocking CXCL12 sequestration may allow CXCL12 to tether T cells to the perivascular space, preventing infiltration and having a beneficial effect on EAE (Cruz-Orengo et al., 2011). In support of these results, EAE induction in mice expressing IFN-γ receptor exclusively on endothelial cells was sufficient to significantly mitigate brain inflammation, resulting in a lower incidence of atypical symptoms, compared to IFN-γ receptor deficient animals (Ni et al., 2014). Notably, studies performed almost three decades ago showed that IFN-γ signaling plays a protective role during neuroinflammation, as proved by exacerbation of EAE symptoms in IFN-γ–/– mice (Ferber et al., 1996; Willenborg et al., 1996) or disease alleviation upon IFN-γ administration, supporting the idea that IFN-γ promotes BBB stability (Voorthuis et al., 1990; Naves et al., 2013). However, more recent studies showed that IFN-γ signaling is required to trigger spinal cord inflammation and classic EAE, whereas IFN-γ receptor deficiency promotes the development of atypical EAE symptoms, associated to Th17 cell migration in the brainstem and cerebellum (Lees et al., 2008; Stromnes et al., 2008; Pierson et al., 2012). Together, these data suggest that regional CNS responses to IFN-γ, including BBB inflammation and breakdown, determine lesion localization patterns during EAE development.
IL-17A, IL-17F, IL-22, and IL-26 represent a group of cytokines produced by Th17 cells and are classically associated to the CNS inflammatory milieu during MS (Figure 2 and Table 1). Indeed, increased levels of IL-17 mRNA and IL-17 secreting CD4+ T cells were detected in the blood, CSF, and brain lesions of MS patients (Matusevicius et al., 1999; Lock et al., 2002; Tzartos et al., 2008; Durelli et al., 2009). Particularly, IL-17A is elevated in the CSF of RRMS subjects and correlates with CSF/serum albumin quotient, an index of BBB permeability. In this regard, treating RRMS patients with secukinumab, an anti-IL-17A antibody, reduced MRI lesion activity compared to placebo, supporting a role for IL-17 in disease development (Havrdová et al., 2016).
Cytokines produced by Th17 cells may directly affect BBB integrity and function. Indeed, in vitro stimulation of human brain endothelial cells (HBEC) with IL-17A diminishes the expression of tight junction protein-encoding genes Pecam1, Cdh5, and Tjp1 and leads to the disassembly of ZO-1 and reduction of occludin and CLDN 5 protein expression (Rahman et al., 2018; Setiadi et al., 2019). Similarly, in vitro data obtained using the mouse bEnd.3 cell line showed that IL-17A activates endothelial contractile machinery through a ROS-dependent pathway, inducing occludin downregulation, ZO-1 disorganization, and ICAM-1 upregulation (Huppert et al., 2010) (Figure 2 and Table 1). In line with this, inhibition of IL-17A in a model of EAE led to a reduced leukocyte infiltration of the CNS, lower CNS oxidative stress and milder clinical disease, further demonstrating a role for IL-17 in BBB breakdown (Huppert et al., 2010; Setiadi et al., 2019). Moreover, IL-17, conversely to IFN-γ, induces upregulation of CXCR7 on primary mouse brain endothelial cells (Cruz-Orengo et al., 2011) potentially promoting leukocyte migration, as also suggested by in vivo data demonstrating that activation of CXCR7 reduces abluminal CXCL12 concentration and leads to an increased leukocyte entry in the inflamed CNS during EAE (Cruz-Orengo et al., 2011). IL-22 produced by Th17 cells can also affect BBB permeability, as shown by studies performed with primary endothelial cells from human CNS tissue specimens (Kebir et al., 2007; Figure 2 and Table 1). Remarkably, the receptors for IL-17 and IL-22 are absent in CNS samples from healthy human subjects, but they are strongly expressed on CNS endothelial cells within MS lesions, suggesting that the human BBB is highly responsive to the detrimental effect of these two cytokines (Kebir et al., 2007). In agreement with these data, both IL-17 and IL-22 can boost Th cell transmigration across brain endothelium in vitro, although IL-17 is more effective in inducing the production of endothelial pro-inflammatory molecules (Kebir et al., 2007; Wojkowska et al., 2017). However, differently from IL-17, which downregulates tight junction proteins, the mechanisms underlying IL-22 effect on the BBB remain uncertain.
Unlike IL-17 and IL-22, IL-26 showed a protective effect on BBB integrity (Broux et al., 2020; Figure 2 and Table 1). Brain endothelial cells treated with IL-26 showed a general downregulation of pro-inflammatory pathways and upregulation of Tjp1, Ocln, and Cldn18 gene transcripts. Additionally, IL-26 treatment of EAE mice resulted in a reduced infiltration of pathogenic T lymphocytes and disease attenuation, clearly demonstrating a protective effect of IL-26 at the BBB level (Broux et al., 2020). Overall, the existing literature on MS and EAE point to Th17 cytokines as key players in modulating BBB permeability and leukocyte trafficking during CNS autoimmune inflammatory conditions.
Whereas the BBB is considered a major gateway for T cell migration from the blood into the CNS, the BCSFB represents a weir tightly regulating the passage of solutes and migrating cells form the blood directly into the CSF. The BCSFB includes two main structures: the leptomeningeal compartment, in which the pial vessels have a central role, and the choroid plexus. The leptomeninges have proven to be an important entry site for peripheral leukocytes (Bartholomäus et al., 2009; Kivisäkk et al., 2009). Notably, a growing body of experimental evidence identifies the leptomeninges as a pivotal anatomical site for T cell reactivation by local antigen presenting cells, development of inflammatory reactions and subsequent CNS invasion by immune cells (Bartholomäus et al., 2009; Kivisäkk et al., 2009; Lodygin et al., 2013; Schläger et al., 2016). On the other hand, the choroid plexus is a highly specialized structure lining the walls of cerebral ventricles and populated by patrolling leukocytes under physiological conditions (de Graaf et al., 2011; Kunis et al., 2013). Interestingly, the selectivity of the choroid plexus barrier is not due to its endothelial cells, which are fenestrated and leaky, but to its innermost epithelial cell layer, which has tight junctions and modulates the trafficking of immune cells into the CSF in response to environmental cues (Maxwell and Pease, 1956; Engelhardt et al., 2001; Ayub et al., 2021).
The discovery of lymphoid follicles in the leptomeninges of MS patients and EAE mice redirected the attention from the BBB dysfunction to meningeal inflammation and its potential role in disease pathogenesis (Magliozzi et al., 2004; Serafini et al., 2004). In RRMS and PPMS, the leptomeningeal area seems to be predominantly characterized by a chaotic accumulation of inflammatory cells, whereas in SPMS, immune cells were found in highly organized tertiary lymphoid structures, pointing to leptomeningeal inflammation as a pathogenic driver for disease development (Choi et al., 2012; Howell et al., 2015; Magliozzi et al., 2018). As observed in EAE, MRI investigations corroborated by histopathology studies highlighted a direct correlation between leptomeningeal contrast enhancement, subarachnoid space (SAS)-localized inflammation and subpial cortical pathology in MS patients (Lucchinetti et al., 2011; Choi et al., 2012; Howell et al., 2015; Harrison et al., 2017; Magliozzi et al., 2018; Bergsland et al., 2019; Zurawski et al., 2020). At early stages of human disease, meningeal inflammation is mainly detected in the proximity of areas with altered BBB, cortical demyelination, and gray matter lesions and seems to develop before the emergence of white matter plaques (Lucchinetti et al., 2011). Similarly, inflammatory features appear in the cerebral and spinal cord meninges before EAE onset (Brown and Sawchenko, 2007; Shrestha et al., 2017). Finally, a recent ultra-high field MRI study demonstrated that the cerebral leptomeningeal contrast enhancement magnitude, which reaches a peak of intensity during the acute phase of EAE, is associated to the clinical signs and high inflammatory cell density in EAE mice (Pol et al., 2019). Together, these data underline the importance of the leptomeningeal BCSFB as one of the earliest routes of entry for peripheral immune cells in both MS and EAE.
The SAS is a surgically easily accessible area, and several in vivo imaging studies have described the central role of pial vessels during autoreactive T cell recruitment into the CNS (Zenaro et al., 2013; Rossi and Constantin, 2016). Given their permissive endothelial layer and the lack of astrocyte sheathes typical of the BBB, leptomeningeal pial vessels represent a preferential CNS gateway for pioneer circulating autoreactive CD4+ T cells during the preclinical phase of EAE (Bartholomäus et al., 2009). Th cells reach the maximum accumulation in the leptomeninges at EAE peak and migrate in the SAS attracted by the CXCL9-11 and CCL5 chemokines (Bartholomäus et al., 2009; Lodygin et al., 2013; Schläger et al., 2016). The endothelium of inflamed pial vessels expresses P- and E-selectins (Kerfoot and Kubes, 2002; Piccio et al., 2002; Kivisäkk et al., 2003). Particularly, P-selectin was found to be a key molecule involved in leukocyte adhesive interactions in brain pial vessels during the early stages of EAE (Kerfoot and Kubes, 2002; Kerfoot et al., 2006). In agreement with these data, intravital microscopy studies performed in the leptomeningeal microcirculation have shown that a properly glycosylated PSGL-1 is the major P-selectin ligand for efficient tethering and rolling of activated T cells (Piccio et al., 2002, 2005). Likewise, CD8+ T cells from MS patients preferentially exploit PSGL-1 for their rolling on inflamed pial vessels endothelium, further indicating a role for PSGL-1 in activated T cell adhesion in brain pial vessels (Battistini et al., 2003). Interestingly, T cell immunoglobulin and mucin domain 1 (TIM-1) was shown to cooperate with PSGL-1 in the mediation of Th1 and Th17 tethering and rolling on inflamed pial vasculature, contributing to the CNS infiltration of autoreactive T cells and to the development of EAE (Angiari and Constantin, 2014; Angiari et al., 2014). Moreover, integrins VLA-4 and LFA-1 were found to be involved in rolling and G protein-mediated firm adhesion of peripheral leukocytes on inflamed endothelium of cerebral pial vessels, potentially leading to vascular disfunction and leakage (Kerfoot and Kubes, 2002; Piccio et al., 2002; Kerfoot et al., 2006; Figure 1).
Once migrated in the leptomeninges, autoreactive CD4+ T cells are reactivated by local antigen presenting cells and directed to the underlying CNS parenchyma (Bartholomäus et al., 2009; Lodygin et al., 2013; Schläger et al., 2016). However, how autoreactive T cells access the parenchyma from the SAS is still unclear. In this respect, it was suggested that, similar to the blood circulation, CSF dynamics could operate as a physical transport mechanism facilitating cell migration into the inflamed areas of the CNS (Schläger et al., 2016). A potential molecular mechanism controlling T cell migration into the parenchyma could be mediated by laminin 111 (α1β1γ1 or laminin 1), which is preferentially expressed by the leptomeningeal cells and in close contact with astrocyte end feet (Sixt et al., 2001; Agrawal et al., 2006). Also, glia limitans breakdown and microglia activation, both potentially due to meningeal inflammation, may promote autoreactive T cell entry in the cortex and spinal cord during MS and EAE (Gardner et al., 2013; Magliozzi et al., 2018, 2019; James et al., 2020). Furthermore, astrocytes can also contribute to T cell trafficking during EAE. In this context, Th1 and Th17 cytokines induce region-specific astrocyte expression of VCAM-1 and CXCR7, modulating local astrocyte-dependent immune cell trafficking into the CNS during EAE (Williams et al., 2020).
Due to its location within the cerebral ventricles and the presence of fenestrated capillaries, the choroid plexus is another important gateway involved in CNS immunosurveillance (Nathanson and Chun, 1989). However, the poor vascular selectivity is compensated by the expression of apical tight junctions and basolateral adherens junctions in the epithelial layer of ependymal cells that strictly controls immune cell trafficking from the choroid plexus stroma into the CSF (Maxwell and Pease, 1956; van Deurs and Koehler, 1979; Lippoldt et al., 2000; Ayub et al., 2021). Indeed, the murine choroid plexus epithelium constitutively expresses ICAM-1, VCAM-1, MadCAM, P-selectin, and CCL20, which may explain, at least in part, why memory CD4+ T cells are the most represented leukocytes within the CSF under physiological conditions (Steffen et al., 1996; Wolburg et al., 1999; Kivisäkk et al., 2003; Reboldi et al., 2009; de Graaf et al., 2011; Figure 3). Additionally, recent in vitro studies suggest that, once migrated in the choroid plexus stroma, human CD4+ T cells could further migrate into the CSF by binding ICAM-1 expressed at the luminal side of epithelial cells (Nishihara et al., 2020).
Figure 3. T helper cells regulate choroid plexus function through cytokine secretion. T helper (Th) cells contribute to fine-tune immune processes at the level of the choroid plexus during both homeostasis and neuroinflammation. In the steady state, IFN-γ released by local effector memory CD4+ T lymphocytes is required for an efficient CNS immune surveillance, since it regulates the expression of key trafficking molecules expressed on ependymal cells. Under neuroinflammatory conditions, IFN-γ signaling in the choroid plexus promotes the transmigration of pro-resolving macrophages, which can exert their protective function in the CNS. IL-17 is relevant for EAE development through the induction of CCL20 secretion by choroid plexus epithelium, leading to subsequent invasion of CCR6+ encephalitogenic T lymphocytes. Created with BioRender.com.
The onset of CNS-targeted autoimmune responses during EAE induces a prominent upregulation of ICAM-1 and VCAM-1 on choroid plexus epithelium, further favoring leukocytes trafficking through this anatomical route (Steffen et al., 1996; Figure 3). When exposed to IFN-γ, the murine choroid plexus epithelium becomes activated, producing chemokines (CCL2, CCL5, CXCL9, CXCL10, CX3CL1, and M-CSF) and upregulating cell adhesion receptors (ICAM-1 and VCAM-1) and MHC-II molecules (Figure 3; Kunis et al., 2013). On the other hand, exposing choroid plexus epithelial cells to IL-17 results in further upregulation of CCL20, a chemokine involved in the recruitment of CCR6+ encephalitogenic Th17 cells during early EAE (Reboldi et al., 2009; Kunis et al., 2013; Figure 3). Notably, CCR6+ CD25- CD4+ pro-inflammatory lymphocytes are present in the CSF of MS patients starting from the earliest disease episode, supporting the pivotal involvement of the evolutionarily conserved CCL20-CCR6 axis in the establishment of a pathological loop amplifying local CNS immune responses (Reboldi et al., 2009; Kara et al., 2015). Interestingly, CD4+ memory T cells expressing CXCR3, a receptor highly present on Th1 cells, were found enriched in the CSF compared to circulating levels (Kivisäkk et al., 2002). However, no differences in CXCR3 expression were detected between MS and control subjects, suggesting a potential role for this chemokine receptor in T cell intrathecal residency (Kivisäkk et al., 2002).
The analysis of postmortem MS brain samples revealed an impairment of epithelial CLDN 3 at the level of the choroid plexus. Accordingly, mice lacking CLDN 3 displayed an earlier EAE onset due to higher levels of infiltrated leukocytes in the CSF (Kooij et al., 2014). The early accumulation of activated T cells in the choroid plexus during EAE suggests a crucial role for this CNS compartment in disease pathogenesis (Brown and Sawchenko, 2007; Murugesan et al., 2012). Throughout the EAE course, the choroid plexus appears enlarged, morphologically altered, and expresses immune-related genes including proinflammatory cytokines, co-stimulatory molecules for T cells and adhesion molecules, suggesting its potential capacity to recall and activate migrating autoreactive T cells (Murugesan et al., 2012). Also, high-throughput studies showed that the majority of T cell receptor repertoire inside the choroid plexus is specific for CNS antigens in mice that were immunized with spinal cord homogenates (Baruch et al., 2013). In addition, recent data demonstrated that the choroid plexus is characterized by the constitutive presence of CNS antigens and antigen presenting cells, that can rapidly react to inflammatory signals, promoting T cell proliferation and a second wave of leukocyte migration into the CNS (Strominger et al., 2018). In agreement with studies performed in mouse models, results obtained on post-mortem choroid plexus samples found increased T cell migration and HLA-DR expression on the choroid plexus stroma of patients with MS compared to control subjects, suggesting that choroid plexus may represent a site for lymphocyte entry in the CSF and antigen presentation also during human disease (Vercellino et al., 2008).
Recent MRI data showed a significant increase of choroid plexus volume in EAE mice compared to untreated animals at baseline, as well as in RRMS patients compared to healthy controls, correlating to acute disease episodes and disability worsening (Fleischer et al., 2021). Notably, Natalizumab treatment proved effective in reducing choroid plexus enlargement in MS patients at follow-up, while dimethyl fumarate, which does not interfere with leukocyte recruitment into the CNS, had no effect (Fleischer et al., 2021). Altogether, these data suggest a role for leukocyte migration through the choroid plexus in promoting MS pathology.
Recent studies showed that the dura mater also represents a bona fide immune interface, opening new scenarios on the role of meningeal immunity during homeostasis and pathological conditions (Rustenhoven et al., 2021). The rediscovery of a full-fledged CSF-draining lymphatic system located in the dura mater sparked interest in the immune properties of this overlooked meningeal structure (Louveau et al., 2015). Interestingly, the ablation of the meningeal lymphatics impairs the drainage of immune cells and macromolecules from the CSF to deep cervical lymph nodes, dampening the capacity of encephalitogenic T cells to mount an efficient autoimmune response during EAE (Louveau et al., 2018). Furthermore, dural sinuses are characterized by VCAM-1 expression and are surrounded by gradients of CXCL12, representing an ideal spot for T cell extravasation (Rustenhoven et al., 2021). The preferential distribution of antigen presenting cells and patrolling T lymphocytes at the peri-sinusal level is also of strategic importance for local immune surveillance, further pointing to this meningeal layer as a true neuro-immune interface (Rustenhoven et al., 2021). Moreover, the abundance of dural dendritic cells with a migratory phenotype suggests an active transport of CNS antigens to the draining lymph nodes (Van Hove et al., 2019). Recent studies have shown that, following EAE induction, the number of dural antigen presenting cells is not altered, but there is a significant peri-sinusal accumulation of peripherally activated MOG-reactive T cells that, once migrated into the dura, acquire a tissue resident memory phenotype (Rustenhoven et al., 2021). However, the role of dural interface during CNS autoimmunity has been recently challenged by data showing that meningeal inflammatory processes underlying MS and EAE pathogenesis take place predominantly in the leptomeningeal compartment, whereas the dura mater is only marginally and passively involved in CNS autoimmunity (Merlini et al., 2022). Interestingly, the pool of dural leukocytes seems to be constantly replenished by the skull and vertebral bone marrow through direct channels under normal conditions, with a massive cell mobilization to the dura upon inflammation (Cugurra et al., 2021; Mazzitelli et al., 2022). However, these newly described immune mechanisms mainly regard myeloid cells, while T lymphocytes seem to migrate preferentially through the blood vessels (Rustenhoven et al., 2021). Overall, the role of dural interface in T cell migration and immune responses during MS and EAE is still unclear and requires further investigation.
T helper 2 cells were first described as a distinct subset of IL-4-secreting CD4+ T lymphocytes, laying the foundation for the classical Th1/Th2 dualism (Mosmann et al., 1986). Later, the array of Th2-related cytokines was broadened to also include IL-5, IL-10, and IL-13 (Fiorentino et al., 1989; Fort et al., 2001). Th2 cells and their cytokines are mainly considered neuroprotective in EAE and MS (Weiner et al., 1994; Sospedra and Martin, 2005; Fernando et al., 2014). However, contrasting evidence highlighted that CD4+ T lymphocytes with a Th2-like phenotype clonally expanded and actively fueled the B cell response in MS lesions characterized by antibody and complement deposition (Planas et al., 2015), pointing to a multifaceted role for Th2 cells in CNS autoimmune conditions.
Contrary to Th1 and Th17 cells, whose ability to cross brain barriers has been widely investigated, little is known about Th2 lymphocyte migration into the CNS during neuroinflammatory conditions. Intravital microscopy experiments demonstrated that both murine and human Th2 cells show a reduced capability to adhere in inflamed brain pial vessels compared to Th1 lymphocytes, due to their low expression of a specific glycosylation epitope on PSGL-1 molecule (Wagers et al., 1998; Colantonio et al., 2004; Piccio et al., 2005). However, in vitro Th2 cell migration through a layer of HBEC was more efficient when compared to Th1 cells and was dependent on the CCR2-CCL2 axis and ICAM-1 (Biernacki et al., 2001). These in vitro data were recently challenged by other studies showing that Th2 lymphocytes display the lowest ability to migrate using in vitro models of human BBB and BCSFB, when compared to other Th subsets (Wimmer et al., 2019; Nishihara et al., 2020). These discrepancies suggest that different experimental settings of brain barriers and Th cell preparations may be responsible for the contrasting results obtained using Th2 lymphocytes in vitro. Recent findings confirmed a key role for ICAM-1 and also showed a role for CD99 in Th2 cell migration using models of human BBB and BCSFB, suggesting this may also be the case during human neuroinflammatory diseases (Wimmer et al., 2019; Nishihara et al., 2020). Interestingly, recent in vitro data showed that ICAM-1 mediate the reverse migration of CSF-derived Th2 and other Th cells through the epithelial layer of the choroid plexus, suggesting that these cells may leave the CNS by crossing the BCSFB (Nishihara et al., 2020).
Despite the seemingly protective role played by Th2 lymphocytes and their signature cytokine, treating in vitro cultured HBEC with IL-4 triggers a loss of barrier integrity together with changes in cellular morphology (Smyth et al., 2018; Table 1 and Figure 2). These surprising data indicate that IL-4 and Th2 cell-dependent anti-inflammatory functions are not necessarily linked to protective effects on the BBB, and more studies are needed to clarify the impact of Th2 lymphocytes on brain barriers.
Whereas T cell migration at the BBB and leptomeningeal level has been more extensively studied, the interplay between T lymphocytes and other CNS borders such as the choroid plexus and dura mater is still largely unexplored. Also, the anatomy of the choroid plexus is still not fully characterized, adding further difficulty to the understanding of this CNS border and its relationship with immune cells (Wolburg and Paulus, 2010). Hopefully, cutting-edge in vivo imaging approaches will help to better determine how T cells traffic through the choroid plexus into the CSF (Shipley et al., 2020). A deeper knowledge of the inflammatory events at the level of the choroid plexus may also be relevant from a therapeutic point of view, as suggested by recent studies which propose the targeting of this interface between the systemic circulation and the ventricular system as a novel therapeutic approach for CNS diseases (Bryniarski et al., 2020).
Dura mater has also emerged as an additional neuroimmune interface due to its functional organization and specific localization. However, its involvement in the immune responses and interplay with Th lymphocytes during EAE and MS deserves further investigation. Whether T cells can directly migrate through the meningeal layers is unclear. In support of this possibility, it was shown that CLDN 11, a tight junction protein enriched in the arachnoid mater, is downregulated at later stages of EAE, potentially allowing the migration of T lymphocytes through an impaired arachnoid layer (Uchida et al., 2019). The pathological changes of the arachnoid barrier during CNS autoimmune and inflammatory diseases have been poorly dissected and can constitute a future field of investigation.
Finally, the scientific community has long considered the BBB as a weir, but, at the same time, a major route of leukocyte migration into the brain during neuroinflammation, dedicating significant efforts to the identification of therapeutic targets interfering with Th1 and Th17 cell migration into the brain. Nevertheless, the restoration of BBB morphological integrity and functionality following neuroinflammatory insults may represent a promising therapeutic strategy to accelerate CNS recovery during MS (Spencer et al., 2018).
All authors listed have made a substantial, direct, and intellectual contribution to the work, and approved it for publication.
This work was supported in part by the European Research Council (ERC) advanced grant number 695714 IMMUNOALZHEIMER and the ERC Proof of Concept grant numbers 693606 IMPEDE and 101069397 NeutrAD, the National Recovery and Resilience Plan (PNRR), National Centers Program (National Biodiversity Future Center – NBFC), PNRR “Partenariati estesi” Program, grant MNESYS (to GC), and Fondazione Italiana Sclerosi Multipla (FISM), Genoa, Italy Project cod.2013/R/21 (to BR).
The authors declare that the research was conducted in the absence of any commercial or financial relationships that could be construed as a potential conflict of interest.
All claims expressed in this article are solely those of the authors and do not necessarily represent those of their affiliated organizations, or those of the publisher, the editors and the reviewers. Any product that may be evaluated in this article, or claim that may be made by its manufacturer, is not guaranteed or endorsed by the publisher.
Adamson, P., Etienne, S., Couraud, P., Calder, V., and Greenwood, J. (1999). Lymphocyte migration through brain endothelial cell monolayers involves signaling through endothelial ICAM-1 via a rho-dependent pathway. J. Immunol. 162, 2964–2973. doi: 10.4049/jimmunol.162.5.2964
Agrawal, S., Anderson, P., Durbeej, M., van Rooijen, N., Ivars, F., Opdenakker, G., et al. (2006). Dystroglycan is selectively cleaved at the parenchymal basement membrane at sites of leukocyte extravasation in experimental autoimmune encephalomyelitis. J. Exp. Med. 203, 1007–1019. doi: 10.1084/jem.20051342
Alt, C., Laschinger, M., and Engelhardt, B. (2002). Functional expression of the lymphoid chemokines CCL19 (ELC) and CCL 21 (SLC) at the blood-brain barrier suggests their involvement in G-protein-dependent lymphocyte recruitment into the central nervous system during experimental autoimmune encephalomyelitis. Eur. J. Immunol. 32, 2133–2144.
Alvarez, J., Saint-Laurent, O., Godschalk, A., Terouz, S., Briels, C., Larouche, S., et al. (2015). Focal disturbances in the blood-brain barrier are associated with formation of neuroinflammatory lesions. Neurobiol. Dis. 74, 14–24. doi: 10.1016/j.nbd.2014.09.016
Anderson, V., Fox, N., and Miller, D. (2006). Magnetic resonance imaging measures of brain atrophy in multiple sclerosis. J. Magn. Reson. Imaging. 23, 605–618. doi: 10.1002/jmri.20550
Andjelkovic, A., Spencer, D., and Pachter, J. (1999). Visualization of chemokine binding sites on human brain microvessels. .J Cell Biol. 145, 403–412. doi: 10.1083/jcb.145.2.403
Angiari, S., and Constantin, G. (2014). Regulation of T cell trafficking by the T cell immunoglobulin and mucin domain 1 glycoprotein. Trends Mol. Med. 20, 675–684. doi: 10.1016/j.molmed.2014.10.003
Angiari, S., Donnarumma, T., Rossi, B., Dusi, S., Pietronigro, E., Zenaro, E., et al. (2014). TIM-1 glycoprotein binds the adhesion receptor P-selectin and mediates T cell trafficking during inflammation and autoimmunity. Immunity 40, 542–553. doi: 10.1016/j.immuni.2014.03.004
Arbour, N., Holz, A., Sipe, J., Naniche, D., Romine, J., Zyroff, J., et al. (2003). A new approach for evaluating antigen-specific T cell responses to myelin antigens during the course of multiple sclerosis. J. Neuroimmunol. 137, 197–209. doi: 10.1016/s0165-5728(03)00080-8
Arellano, G., Acuña, E., Reyes, L., Ottum, P., De Sarno, P., Villarroel, L., et al. (2017). Th1 and Th17 cells and associated cytokines discriminate among clinically isolated syndrome and multiple sclerosis phenotypes. Front. Immunol. 8:753. doi: 10.3389/fimmu.2017.00753
Ayub, M., Jin, H., and Bae, J. (2021). The blood cerebrospinal fluid barrier orchestrates immunosurveillance, immunoprotection, and immunopathology in the central nervous system. BMB Rep. 54, 196–202. doi: 10.5483/BMBRep.2021.54.4.205
Bahbouhi, B., Berthelot, L., Pettré, S., Michel, L., Wiertlewski, S., Weksler, B., et al. (2009). Peripheral blood CD4+ T lymphocytes from multiple sclerosis patients are characterized by higher PSGL-1 expression and transmigration capacity across a human blood-brain barrier-derived endothelial cell line. J. Leukoc. Biol. 86, 1049–1063. doi: 10.1189/jlb.1008666
Barcellos, L., Oksenberg, J., Begovich, A., Martin, E., Schmidt, S., Vittinghoff, E., et al. (2003). HLA-DR2 dose effect on susceptibility to multiple sclerosis and influence on disease course. Am. J. Hum. Genet. 72, 710–716. doi: 10.1086/367781
Barkauskas, D., Dixon Dorand, R., Myers, J., Evans, T., Barkauskas, K., Askew, D., et al. (2015). Focal transient CNS vessel leak provides a tissue niche for sequential immune cell accumulation during the asymptomatic phase of EAE induction. Exp. Neurol. 266, 74–85. doi: 10.1016/j.expneurol.2015.02.018
Baron, J., Madri, J., Ruddle, N., Hashim, G., and Janeway, C. (1993). Surface expression of alpha 4 integrin by CD4 T cells is required for their entry into brain parenchyma. J. Exp. Med. 177, 57–68. doi: 10.1084/jem.177.1.57
Bartholomäus, I., Kawakami, N., Odoardi, F., Schläger, C., Miljkovic, D., Ellwart, J., et al. (2009). Effector T cell interactions with meningeal vascular structures in nascent autoimmune CNS lesions. Nature 462, 94–98. doi: 10.1038/nature08478
Baruch, K., Ron-Harel, N., Gal, H., Deczkowska, A., Shifrut, E., Ndifon, W., et al. (2013). CNS-specific immunity at the choroid plexus shifts toward destructive Th2 inflammation in brain aging. Proc. Natl. Acad. Sci. U.S.A. 110, 2264–2269. doi: 10.1073/pnas.1211270110
Battistini, L., Piccio, L., Rossi, B., Bach, S., Galgani, S., Gasperini, C., et al. (2003). CD8+ T cells from patients with acute multiple sclerosis display selective increase of adhesiveness in brain venules: A critical role for P-selectin glycoprotein ligand-1. Blood 101, 4775–4782. doi: 10.1182/blood-2002-10-3309
Belachew, S., Phan-Ba, R., Bartholomé, E., Delvaux, V., Hansen, I., Calay, P., et al. (2011). Natalizumab induces a rapid improvement of disability status and ambulation after failure of previous therapy in relapsing-remitting multiple sclerosis. Eur. J. Neurol. 18, 240–245. doi: 10.1111/j.1468-1331.2010.03112.x
Ben-Nun, A., Wekerle, H., and Cohen, I. (1981). The rapid isolation of clonable antigen-specific T lymphocyte lines capable of mediating autoimmune encephalomyelitis. Eur. J. Immunol. 11, 195–199. doi: 10.1002/eji.1830110307
Bergsland, N., Ramasamy, D., Tavazzi, E., Hojnacki, D., Weinstock-Guttman, B., and Zivadinov, R. (2019). Leptomeningeal contrast enhancement is related to focal cortical thinning in relapsing-remitting multiple sclerosis: A cross-sectional MRI study. AJNR Am. J. Neuroradiol. 40, 620–625. doi: 10.3174/ajnr.A6011
Bettelli, E., Pagany, M., Weiner, H., Linington, C., Sobel, R., and Kuchroo, V. (2003). Myelin oligodendrocyte glycoprotein-specific T cell receptor transgenic mice develop spontaneous autoimmune optic neuritis. J. Exp. Med. 197, 1073–1081. doi: 10.1084/jem.20021603
Biernacki, K., Prat, A., Blain, M., and Antel, J. (2001). Regulation of Th1 and Th2 lymphocyte migration by human adult brain endothelial cells. J. Neuropathol. Exp. Neurol. 60, 1127–1136. doi: 10.1093/jnen/60.12.1127
Bö, L., Peterson, J., Mørk, S., Hoffman, P., Gallatin, W., Ransohoff, R., et al. (1996). Distribution of immunoglobulin superfamily members ICAM-1, –2, –3, and the beta 2 integrin LFA-1 in multiple sclerosis lesions. J. Neuropathol. Exp. Neurol. 55, 1060–1072.
Bonney, S., Seitz, S., Ryan, C., Jones, K., Clarke, P., Tyler, K., et al. (2019). Gamma interferon alters junctional integrity via rho kinase, resulting in blood-brain barrier leakage in experimental viral encephalitis. mBio. 10, e1675–e1619. doi: 10.1128/mBio.01675-19
Broux, B., Zandee, S., Gowing, E., Charabati, M., Lécuyer, M., Tastet, O., et al. (2020). Interleukin-26, preferentially produced by TH17 lymphocytes, regulates CNS barrier function. Neurol. Neuroimmunol. Neuroinflamm. 7:e870. doi: 10.1212/NXI.0000000000000870
Brown, D., and Sawchenko, P. (2007). Time course and distribution of inflammatory and neurodegenerative events suggest structural bases for the pathogenesis of experimental autoimmune encephalomyelitis. J. Comp. Neurol. 502, 236–260. doi: 10.1002/cne.21307
Brown, R., Morris, A., and O’Neil, R. (2007). Tight junction protein expression and barrier properties of immortalized mouse brain microvessel endothelial cells. Brain Res. 1130, 17–30. doi: 10.1016/j.brainres.2006.10.083
Brucklacher-Waldert, V., Stuerner, K., Kolster, M., Wolthausen, J., and Tolosa, E. (2009). Phenotypical and functional characterization of T helper 17 cells in multiple sclerosis. Brain 132 (Pt 12), 3329–3341. doi: 10.1093/brain/awp289
Bryniarski, M., Ren, T., Rizvi, A., Snyder, A., and Morris, M. (2020). Targeting the choroid plexuses for protein drug delivery. Pharmaceutics 12:963. doi: 10.3390/pharmaceutics12100963
Cannella, B., and Raine, C. (1995). The adhesion molecule and cytokine profile of multiple sclerosis lesions. Ann. Neurol. 37, 424–435. doi: 10.1002/ana.410370404
Cannella, B., Cross, A., and Raine, C. (1990). Upregulation and coexpression of adhesion molecules correlate with relapsing autoimmune demyelination in the central nervous system. J. Exp. Med. 172, 1521–1524. doi: 10.1084/jem.172.5.1521
Cayrol, R., Wosik, K., Berard, J., Dodelet-Devillers, A., Ifergan, I., Kebir, H., et al. (2008). Activated leukocyte cell adhesion molecule promotes leukocyte trafficking into the central nervous system. Nat. Immunol. 9, 137–145. doi: 10.1038/ni1551
Chan, P., and Aruffo, A. (1993). VLA-4 integrin mediates lymphocyte migration on the inducible endothelial cell ligand VCAM-1 and the extracellular matrix ligand fibronectin. J. Biol. Chem. 268, 24655–24664.
Charabati, M., Grasmuck, C., Ghannam, S., Bourbonnière, L., Fournier, A., Lécuyer, M., et al. (2022). DICAM promotes TH17 lymphocyte trafficking across the blood-brain barrier during autoimmune neuroinflammation. Sci. Transl. Med. 14:eabj0473. doi: 10.1126/scitranslmed.abj0473
Choi, S., Howell, O., Carassiti, D., Magliozzi, R., Gveric, D., Muraro, P., et al. (2012). Meningeal inflammation plays a role in the pathology of primary progressive multiple sclerosis. Brain 135 (Pt 10), 2925–2937. doi: 10.1093/brain/aws189
Colantonio, L., Rossi, B., Constantin, G., and D’Ambrosio, D. (2004). Integration and independent acquisition of specialized skin- versus gut-homing and Th1 versus Th2 cytokine synthesis phenotypes in human CD4+ T cells. Eur. J. Immunol. 34, 2419–2429. doi: 10.1002/eji.200425159
Coles, A., Wing, M., Molyneux, P., Paolillo, A., Davie, C., Hale, G., et al. (1999). Monoclonal antibody treatment exposes three mechanisms underlying the clinical course of multiple sclerosis. Ann. Neurol. 46, 296–304.
Compston, A., and Coles, A. (2008). Multiple sclerosis. Lancet 372, 1502–1517. doi: 10.1016/S0140-6736(08)61620-7
Constantin, G. (2008). Chemokine signaling and integrin activation in lymphocyte migration into the inflamed brain. J. Neuroimmunol. 198, 20–26. doi: 10.1016/j.jneuroim.2008.04.023
Cruz-Orengo, L., Holman, D., Dorsey, D., Zhou, L., Zhang, P., Wright, M., et al. (2011). CXCR7 influences leukocyte entry into the CNS parenchyma by controlling abluminal CXCL12 abundance during autoimmunity. J. Exp. Med. 208, 327–339. doi: 10.1084/jem.20102010
Cugurra, A., Mamuladze, T., Rustenhoven, J., Dykstra, T., Beroshvili, G., Greenberg, Z., et al. (2021). Skull and vertebral bone marrow are myeloid cell reservoirs for the meninges and CNS parenchyma. Science 373:eabf7844. doi: 10.1126/science.abf7844
de Graaf, M., Smitt, P., Luitwieler, R., van Velzen, C., van den Broek, P., Kraan, J., et al. (2011). Central memory CD4+ T cells dominate the normal cerebrospinal fluid. Cytometry B Clin. Cytom. 80, 43–50. doi: 10.1002/cyto.b.20542
Döring, A., Wild, M., Vestweber, D., Deutsch, U., and Engelhardt, B. (2007). E- and P-selectin are not required for the development of experimental autoimmune encephalomyelitis in C57BL/6 and SJL mice. J. Immunol. 179, 8470–8479. doi: 10.4049/jimmunol.179.12.8470
Du, F., Garg, A., Kosar, K., Majumder, S., Kugler, D., Mir, G., et al. (2016). Inflammatory Th17 Cells Express Integrin αvβ3 for Pathogenic Function. Cell Rep. 16, 1339–1351. doi: 10.1016/j.celrep.2016.06.065
Durelli, L., Conti, L., Clerico, M., Boselli, D., Contessa, G., Ripellino, P., et al. (2009). T-helper 17 cells expand in multiple sclerosis and are inhibited by interferon-beta. Ann. Neurol. 65, 499–509. doi: 10.1002/ana.21652
Durieu-Trautmann, O., Chaverot, N., Cazaubon, S., Strosberg, A., and Couraud, P. (1994). Intercellular adhesion molecule 1 activation induces tyrosine phosphorylation of the cytoskeleton-associated protein cortactin in brain microvessel endothelial cells. J Biol Chem 269, 12536–12540.
Dusi, S., Angiari, S., Pietronigro, E., Lopez, N., Angelini, G., Zenaro, E., et al. (2019). LFA-1 Controls Th1 and Th17 Motility behavior in the inflamed central nervous system. Front. Immunol. 10:2436. doi: 10.3389/fimmu.2019.02436
El-Behi, M., Ciric, B., Dai, H., Yan, Y., Cullimore, M., Safavi, F., et al. (2011). The encephalitogenicity of T(H)17 cells is dependent on IL-1- and IL-23-induced production of the cytokine GM-CSF. Nat. Immunol. 12, 568–575. doi: 10.1038/ni.2031
Engelhardt, B. (2010). T cell migration into the central nervous system during health and disease: Different molecular keys allow access to different central nervous system compartments. Clin. Exp. Neuroimmunol. 1, 79–93. doi: 10.1111/j.1759-1961.2010.009.x
Engelhardt, B., Kempe, B., Merfeld-Clauss, S., Laschinger, M., Furie, B., Wild, M., et al. (2005). P-selectin glycoprotein ligand 1 is not required for the development of experimental autoimmune encephalomyelitis in SJL and C57BL/6 mice. J. Immunol. 175, 1267–1275. doi: 10.4049/jimmunol.175.2.1267
Engelhardt, B., Wolburg-Buchholz, K., and Wolburg, H. (2001). Involvement of the choroid plexus in central nervous system inflammation. Microsc. Res. Tech. 52, 112–129.
Etienne, S., Adamson, P., Greenwood, J., Strosberg, A., Cazaubon, S., and Couraud, P. (1998). ICAM-1 signaling pathways associated with Rho activation in microvascular brain endothelial cells. J. Immunol. 161, 5755–5761.
Etienne-Manneville, S., Manneville, J., Adamson, P., Wilbourn, B., Greenwood, J., and Couraud, P. O. (2000). ICAM-1-coupled cytoskeletal rearrangements and transendothelial lymphocyte migration involve intracellular calcium signaling in brain endothelial cell lines. J. Immunol. 165, 3375–3383. doi: 10.4049/jimmunol.165.6.3375
Fabene, P., Laudanna, C., and Constantin, G. (2013). Leukocyte trafficking mechanisms in epilepsy. Mol. Immunol. 55, 100–104. doi: 10.1016/j.molimm.2012.12.009
Farinazzo, A., Angiari, S., Turano, E., Bistaffa, E., Dusi, S., Ruggieri, S., et al. (2018). Nanovesicles from adipose-derived mesenchymal stem cells inhibit T lymphocyte trafficking and ameliorate chronic experimental autoimmune encephalomyelitis. Sci. Rep. 8:7473. doi: 10.1038/s41598-018-25676-2
Ferber, I., Brocke, S., Taylor-Edwards, C., Ridgway, W., Dinisco, C., Steinman, L., et al. (1996). Mice with a disrupted IFN-gamma gene are susceptible to the induction of experimental autoimmune encephalomyelitis (EAE). J. Immunol. 156, 5–7. doi: 10.4049/jimmunol.156.1.5
Fernando, V., Omura, S., Sato, F., Kawai, E., Martinez, N., Elliott, S., et al. (2014). Regulation of an autoimmune model for multiple sclerosis in Th2-biased GATA3 transgenic mice. Int. J. Mol. Sci. 15, 1700–1718. doi: 10.3390/ijms15021700
Filippi, M. (2000). Enhanced magnetic resonance imaging in multiple sclerosis. Mult. Scler. 6, 320–326. doi: 10.1177/135245850000600505
Filippi, M., and Rocca, M. A. (2005). MRI evidence for multiple sclerosis as a diffuse disease of the central nervous system. J. Neurol. 252(Suppl. 5) v16–v24. doi: 10.1007/s00415-005-5004-5
Filippi, M., Rocca, M., Martino, G., Horsfield, M., and Comi, G. (1998). Magnetization transfer changes in the normal appearing white matter precede the appearance of enhancing lesions in patients with multiple sclerosis. Ann. Neurol. 43, 809–814. doi: 10.1002/ana.410430616
Fiorentino, D., Bond, M., and Mosmann, T. (1989). Two types of mouse T helper cell. IV. Th2 clones secrete a factor that inhibits cytokine production by Th1 clones. J. Exp. Med. 170, 2081–2095. doi: 10.1084/jem.170.6.2081
Fleischer, V., Gonzalez-Escamilla, G., Ciolac, D., Albrecht, P., Küry, P., Gruchot, J., et al. (2021). Translational value of choroid plexus imaging for tracking neuroinflammation in mice and humans. Proc. Natl. Acad. Sci. U.S.A. 118, e2025000118. doi: 10.1073/pnas.2025000118
Fort, M., Cheung, J., Yen, D., Li, J., Zurawski, S., Lo, S., et al. (2001). IL-25 induces IL-4, IL-5, and IL-13 and Th2-associated pathologies in vivo. Immunity 15, 985–995. doi: 10.1016/s1074-7613(01)00243-6
Fournier, A., Quenault, A., Martinez de Lizarrondo, S., Gauberti, M., Defer, G., Vivien, D., et al. (2017). Prediction of disease activity in models of multiple sclerosis by molecular magnetic resonance imaging of P-selectin. Proc. Natl. Acad. Sci. U.S.A. 114, 6116–6121. doi: 10.1073/pnas.1619424114
Gardner, C., Magliozzi, R., Durrenberger, P., Howell, O., Rundle, J., and Reynolds, R. (2013). Cortical grey matter demyelination can be induced by elevated pro-inflammatory cytokines in the subarachnoid space of MOG-immunized rats. Brain 136 (Pt 12), 3596–3608. doi: 10.1093/brain/awt279
Geginat, J., Paroni, M., Maglie, S., Alfen, J., Kastirr, I., Gruarin, P., et al. (2014). Plasticity of human CD4 T cell subsets. Front. Immunol. 5:630. doi: 10.3389/fimmu.2014.00630
Graesser, D., Solowiej, A., Bruckner, M., Osterweil, E., Juedes, A., Davis, S., et al. (2002). Altered vascular permeability and early onset of experimental autoimmune encephalomyelitis in PECAM-1-deficient mice. J. Clin. Invest. 109, 383–392. doi: 10.1172/JCI13595
Greenwood, J., Heasman, S., Alvarez, J., Prat, A., Lyck, R., and Engelhardt, B. (2011). Review: Leucocyte-endothelial cell crosstalk at the blood-brain barrier: A prerequisite for successful immune cell entry to the brain. Neuropathol. Appl. Neurobiol. 37, 24–39. doi: 10.1111/j.1365-2990.2010.01140.x
Greenwood, J., Walters, C., Pryce, G., Kanuga, N., Beraud, E., Baker, D., et al. (2003). Lovastatin inhibits brain endothelial cell Rho-mediated lymphocyte migration and attenuates experimental autoimmune encephalomyelitis. FASEB J. 17, 905–907. doi: 10.1096/fj.02-1014fje
Harrison, D., Wang, K., Fiol, J., Naunton, K., Royal, W., Hua, J., et al. (2017). Leptomeningeal enhancement at 7T in multiple sclerosis: Frequency, morphology, and relationship to cortical volume. J. Neuroimaging 27, 461–468. doi: 10.1111/jon.12444
Hartung, H., Reiners, K., Archelos, J., Michels, M., Seeldrayers, P., Heidenreich, F., et al. (1995). Circulating adhesion molecules and tumor necrosis factor receptor in multiple sclerosis: Correlation with magnetic resonance imaging. Ann. Neurol. 38, 186–193. doi: 10.1002/ana.410380210
Havrdová, E., Belova, A., Goloborodko, A., Tisserant, A., Wright, A., Wallstroem, E., et al. (2016). Activity of secukinumab, an anti-IL-17A antibody, on brain lesions in RRMS: Results from a randomized, proof-of-concept study. J. Neurol. 263, 1287–1295. doi: 10.1007/s00415-016-8128-x
Hickey, W., Hsu, B., and Kimura, H. (1991). T-lymphocyte entry into the central nervous system. J. Neurosci. Res. 28, 254–260. doi: 10.1002/jnr.490280213
Hirano, M., and Hirano, K. (2016). Myosin di-phosphorylation and peripheral actin bundle formation as initial events during endothelial barrier disruption. Sci. Rep. 6:20989. doi: 10.1038/srep20989
Howell, O., Schulz-Trieglaff, E., Carassiti, D., Gentleman, S., Nicholas, R., Roncaroli, F., et al. (2015). Extensive grey matter pathology in the cerebellum in multiple sclerosis is linked to inflammation in the subarachnoid space. Neuropathol. Appl. Neurobiol. 41, 798–813. doi: 10.1111/nan.12199
Huppert, J., Closhen, D., Croxford, A., White, R., Kulig, P., Pietrowski, E., et al. (2010). Cellular mechanisms of IL-17-induced blood-brain barrier disruption. FASEB J. 24, 1023–1034. doi: 10.1096/fj.09-141978
James, R., Schalks, R., Browne, E., Eleftheriadou, I., Munoz, C., Mazarakis, N., et al. (2020). Persistent elevation of intrathecal pro-inflammatory cytokines leads to multiple sclerosis-like cortical demyelination and neurodegeneration. Acta Neuropathol. Commun. 8:66. doi: 10.1186/s40478-020-00938-1
Kabat, E. A., Wolf, A., and Bezer, A. E. (1947). The rapid production of acute disseminated encephalomyelitis in rhesus monkeys by injection of heterologous and homologous brain tissue with adjuvants. J. Exp. Med. 85, 117–130. doi: 10.1084/jem.85.1.117
Kara, E., McKenzie, D., Bastow, C., Gregor, C., Fenix, K., Ogunniyi, A., et al. (2015). CCR2 defines in vivo development and homing of IL-23-driven GM-CSF-producing Th17 cells. Nat. Commun. 6:8644. doi: 10.1038/ncomms9644
Kaskow, B., and Baecher-Allan, C. (2018). Effector T cells in multiple sclerosis. Cold Spring Harb. Perspect. Med. 8, a029025. doi: 10.1101/cshperspect.a029025
Kebir, H., Ifergan, I., Alvarez, J., Bernard, M., Poirier, J., Arbour, N., et al. (2009). Preferential recruitment of interferon-gamma-expressing TH17 cells in multiple sclerosis. Ann. Neurol. 66, 390–402. doi: 10.1002/ana.21748
Kebir, H., Kreymborg, K., Ifergan, I., Dodelet-Devillers, A., Cayrol, R., Bernard, M., et al. (2007). Human TH17 lymphocytes promote blood-brain barrier disruption and central nervous system inflammation. Nat. Med. 13, 1173–1175. doi: 10.1038/nm1651
Kerfoot, S., and Kubes, P. (2002). Overlapping roles of P-selectin and alpha 4 integrin to recruit leukocytes to the central nervous system in experimental autoimmune encephalomyelitis. J. Immunol. 169, 1000–1006. doi: 10.4049/jimmunol.169.2.1000
Kerfoot, S., Norman, M., Lapointe, B., Bonder, C., Zbytnuik, L., and Kubes, P. (2006). Reevaluation of P-selectin and alpha 4 integrin as targets for the treatment of experimental autoimmune encephalomyelitis. J. Immunol. 176, 6225–6234. doi: 10.4049/jimmunol.176.10.6225
Kivisäkk, P., Imitola, J., Rasmussen, S., Elyaman, W., Zhu, B., Ransohoff, R., et al. (2009). Localizing central nervous system immune surveillance: Meningeal antigen-presenting cells activate T cells during experimental autoimmune encephalomyelitis. Ann. Neurol. 65, 457–469. doi: 10.1002/ana.21379
Kivisäkk, P., Mahad, D., Callahan, M., Sikora, K., Trebst, C., Tucky, B., et al. (2004). Expression of CCR7 in multiple sclerosis: Implications for CNS immunity. Ann. Neurol. 55, 627–638. doi: 10.1002/ana.20049
Kivisäkk, P., Mahad, D., Callahan, M., Trebst, C., Tucky, B., Wei, T., et al. (2003). Human cerebrospinal fluid central memory CD4+ T cells: Evidence for trafficking through choroid plexus and meninges via P-selectin. Proc. Natl. Acad. Sci. U.S.A. 100, 8389–8394. doi: 10.1073/pnas.1433000100
Kivisäkk, P., Trebst, C., Liu, Z., Tucky, B., Sørensen, T., Rudick, R., et al. (2002). T-cells in the cerebrospinal fluid express a similar repertoire of inflammatory chemokine receptors in the absence or presence of CNS inflammation: Implications for CNS trafficking. Clin. Exp. Immunol. 129, 510–518. doi: 10.1046/j.1365-2249.2002.01947.x
Kooij, G., Kopplin, K., Blasig, R., Stuiver, M., Koning, N., Goverse, G., et al. (2014). Disturbed function of the blood-cerebrospinal fluid barrier aggravates neuro-inflammation. Acta Neuropathol. 128, 267–277. doi: 10.1007/s00401-013-1227-1
Krumbholz, M., Theil, D., Cepok, S., Hemmer, B., Kivisäkk, P., Ransohoff, R., et al. (2006). Chemokines in multiple sclerosis: CXCL12 and CXCL13 up-regulation is differentially linked to CNS immune cell recruitment. Brain 129 (Pt 1), 200–211. doi: 10.1093/brain/awh680
Kunis, G., Baruch, K., Rosenzweig, N., Kertser, A., Miller, O., Berkutzki, T., et al. (2013). IFN-γ-dependent activation of the brain’s choroid plexus for CNS immune surveillance and repair. Brain 136 (Pt 11), 3427–3440. doi: 10.1093/brain/awt259
Kunkl, M., Frascolla, S., Amormino, C., Volpe, E., and Tuosto, L. T. (2020). Helper cells: The modulators of inflammation in multiple sclerosis. Cells 9:482. doi: 10.3390/cells9020482
Lécuyer, M., Saint-Laurent, O., Bourbonnière, L., Larouche, S., Larochelle, C., Michel, L., et al. (2017). Dual role of ALCAM in neuroinflammation and blood-brain barrier homeostasis. Proc. Natl. Acad. Sci. U.S.A. 114, E524–E533. doi: 10.1073/pnas.1614336114
Lee, H., Lee, J., Kim, D., Lim, S., Kang, I., and Choi, J. (2019). Pathogenic function of bystander-activated memory-like CD4+ T cells in autoimmune encephalomyelitis. Nat. Commun. 10:709. doi: 10.1038/s41467-019-08482-w
Leech, S., Kirk, J., Plumb, J., and McQuaid, S. (2007). Persistent endothelial abnormalities and blood-brain barrier leak in primary and secondary progressive multiple sclerosis. Neuropathol. Appl. Neurobiol. 33, 86–98. doi: 10.1111/j.1365-2990.2006.00781.x
Lees, J., Golumbek, P., Sim, J., Dorsey, D., and Russell, J. (2008). Regional CNS responses to IFN-gamma determine lesion localization patterns during EAE pathogenesis. J. Exp. Med. 205, 2633–2642. doi: 10.1084/jem.20080155
Leray, E., Yaouanq, J., Le Page, E., Coustans, M., Laplaud, D., Oger, J., et al. (2010). Evidence for a two-stage disability progression in multiple sclerosis. Brain 133 (Pt 7), 1900–1913. doi: 10.1093/brain/awq076
Ley, K., Laudanna, C., Cybulsky, M., and Nourshargh, S. (2007). Getting to the site of inflammation: The leukocyte adhesion cascade updated. Nat. Rev. Immunol. 7, 678–689. doi: 10.1038/nri2156
Lippoldt, A., Jansson, A., Kniesel, U., Andbjer, B., Andersson, A., Wolburg, H., et al. (2000). Phorbol ester induced changes in tight and adherens junctions in the choroid plexus epithelium and in the ependyma. Brain Res. 854, 197–206. doi: 10.1016/s0006-8993(99)02355-0
Liu, Y., Alahiri, M., Ulloa, B., Xie, B., and Sadiq, S. (2018). Adenosine A2A receptor agonist ameliorates EAE and correlates with Th1 cytokine-induced blood brain barrier dysfunction via suppression of MLCK signaling pathway. Immun. Inflamm. Dis. 6, 72–80. doi: 10.1002/iid3.187
Lock, C., Hermans, G., Pedotti, R., Brendolan, A., Schadt, E., Garren, H., et al. (2002). Gene-microarray analysis of multiple sclerosis lesions yields new targets validated in autoimmune encephalomyelitis. Nat. Med. 8, 500–508. doi: 10.1038/nm0502-500
Lodygin, D., Odoardi, F., Schläger, C., Körner, H., Kitz, A., Nosov, M., et al. (2013). A combination of fluorescent NFAT and H2B sensors uncovers dynamics of T cell activation in real time during CNS autoimmunity. Nat. Med. 19, 784–790. doi: 10.1038/nm.3182
Lorenzon, P., Vecile, E., Nardon, E., Ferrero, E., Harlan, J., Tedesco, F., et al. (1998). Endothelial cell E- and P-selectin and vascular cell adhesion molecule-1 function as signaling receptors. J. Cell Biol. 142, 1381–1391. doi: 10.1083/jcb.142.5.1381
Louveau, A., Herz, J., Alme, M., Salvador, A., Dong, M., Viar, K., et al. (2018). CNS lymphatic drainage and neuroinflammation are regulated by meningeal lymphatic vasculature. Nat. Neurosci. 21, 1380–1391. doi: 10.1038/s41593-018-0227-9
Louveau, A., Smirnov, I., Keyes, T., Eccles, J., Rouhani, S., Peske, J., et al. (2015). Structural and functional features of central nervous system lymphatic vessels. Nature 523, 337–341. doi: 10.1038/nature14432
Lucchinetti, C., Popescu, B., Bunyan, R., Moll, N., Roemer, S., Lassmann, H., et al. (2011). Inflammatory cortical demyelination in early multiple sclerosis. N. Engl. J. Med. 365, 2188–2197. doi: 10.1056/NEJMoa1100648
Lyck, R., Lécuyer, M., Abadier, M., Wyss, C., Matti, C., Rosito, M., et al. (2017). ALCAM (CD166) is involved in extravasation of monocytes rather than T cells across the blood-brain barrier. J. Cereb. Blood Flow Metab. 37, 2894–2909. doi: 10.1177/0271678X16678639
Magliozzi, R., Columba-Cabezas, S., Serafini, B., and Aloisi, F. (2004). Intracerebral expression of CXCL13 and BAFF is accompanied by formation of lymphoid follicle-like structures in the meninges of mice with relapsing experimental autoimmune encephalomyelitis. J. Neuroimmunol. 148, 11–23. doi: 10.1016/j.jneuroim.2003.10.056
Magliozzi, R., Howell, O., Durrenberger, P., Aricò, E., James, R., Cruciani, C., et al. (2019). Meningeal inflammation changes the balance of TNF signalling in cortical grey matter in multiple sclerosis. J. Neuroinflammation 16:259. doi: 10.1186/s12974-019-1650-x
Magliozzi, R., Howell, O., Nicholas, R., Cruciani, C., Castellaro, M., Romualdi, C., et al. (2018). Inflammatory intrathecal profiles and cortical damage in multiple sclerosis. Ann. Neurol. 83, 739–755. doi: 10.1002/ana.25197
Mastorakos, P., and McGavern, D. (2019). The anatomy and immunology of vasculature in the central nervous system. Sci. Immunol. 4:eaav0492. doi: 10.1126/sciimmunol.aav0492
Matheny, H., Deem, T., and Cook-Mills, J. (2000). Lymphocyte migration through monolayers of endothelial cell lines involves VCAM-1 signaling via endothelial cell NADPH oxidase. J. Immunol. 164, 6550–6559. doi: 10.4049/jimmunol.164.12.6550
Matusevicius, D., Kivisäkk, P., He, B., Kostulas, N., Ozenci, V., Fredrikson, S., et al. (1999). Interleukin-17 mRNA expression in blood and CSF mononuclear cells is augmented in multiple sclerosis. Mult. Scler. 5, 101–104. doi: 10.1177/135245859900500206
Maxwell, D., and Pease, D. (1956). The electron microscopy of the choroid plexus. J. Biophys. Biochem. Cytol. 2, 467–474. doi: 10.1083/jcb.2.4.467
Mazzitelli, J., Smyth, L., Cross, K., Dykstra, T., Sun, J., Du, S., et al. (2022). Cerebrospinal fluid regulates skull bone marrow niches via direct access through dural channels. Nat. Neurosci. 25, 555–560. doi: 10.1038/s41593-022-01029-1
McCandless, E., Piccio, L., Woerner, B., Schmidt, R., Rubin, J., Cross, A., et al. (2008). Pathological expression of CXCL12 at the blood-brain barrier correlates with severity of multiple sclerosis. Am. J. Pathol. 172, 799–808. doi: 10.2353/ajpath.2008.070918
McCandless, E., Wang, Q., Woerner, B., Harper, J., and Klein, R. (2006). CXCL12 limits inflammation by localizing mononuclear infiltrates to the perivascular space during experimental autoimmune encephalomyelitis. J. Immunol. 177, 8053–8064. doi: 10.4049/jimmunol.177.11.8053
Meinl, E., Krumbholz, M., Derfuss, T., Junker, A., and Hohlfeld, R. (2008). Compartmentalization of inflammation in the CNS: A major mechanism driving progressive multiple sclerosis. J. Neurol. Sci. 274, 42–44. doi: 10.1016/j.jns.2008.06.032
Merlini, A., Haberl, M., Strauß, J., Hildebrand, L., Genc, N., Franz, J., et al. (2022). Distinct roles of the meningeal layers in CNS autoimmunity. Nat. Neurosci. 25, 887–899. doi: 10.1038/s41593-022-01108-3
Miller, D., Grossman, R., Reingold, S., and McFarland, H. (1998). The role of magnetic resonance techniques in understanding and managing multiple sclerosis. Brain 121 (Pt 1), 3–24. doi: 10.1093/brain/121.1.3
Miller, D., Khan, O., Sheremata, W., Blumhardt, L., Rice, G., Libonati, M., et al. (2003). A controlled trial of natalizumab for relapsing multiple sclerosis. N. Engl. J. Med. 348, 15–23. doi: 10.1056/NEJMoa020696
Moldovan, I., Rudick, R., Cotleur, A., Born, S., Lee, J., Karafa, M., et al. (2003). Interferon gamma responses to myelin peptides in multiple sclerosis correlate with a new clinical measure of disease progression. J. Neuroimmunol. 141, 132–140. doi: 10.1016/s0165-5728(03)00221-2
Molyneux, P., Kappos, L., Polman, C., Pozzilli, C., Barkhof, F., Filippi, M., et al. (2000). The effect of interferon beta-1b treatment on MRI measures of cerebral atrophy in secondary progressive multiple sclerosis. European study group on interferon beta-1b in secondary progressive multiple sclerosis. Brain 123 (Pt 11), 2256–2263. doi: 10.1093/brain/123.11.2256
Montresor, A., Toffali, L., Constantin, G., and Laudanna, C. (2012). Chemokines and the signaling modules regulating integrin affinity. Front. Immunol. 3:127. doi: 10.3389/fimmu.2012.00127
Mosmann, T., Cherwinski, H., Bond, M., Giedlin, M., and Coffman, R. (1986). Two types of murine helper T cell clone. I. Definition according to profiles of lymphokine activities and secreted proteins. J. Immunol 136, 2348–2357.
Murphy, A., Lalor, S., Lynch, M., and Mills, K. (2010). Infiltration of Th1 and Th17 cells and activation of microglia in the CNS during the course of experimental autoimmune encephalomyelitis. Brain. Behav. Immun. 24, 641–651. doi: 10.1016/j.bbi.2010.01.014
Murugesan, N., Paul, D., Lemire, Y., Shrestha, B., Ge, S., and Pachter, J. (2012). Active induction of experimental autoimmune encephalomyelitis by MOG35-55 peptide immunization is associated with differential responses in separate compartments of the choroid plexus. Fluids Barriers CNS 9:15. doi: 10.1186/2045-8118-9-15
Mycko, M., Papoian, R., Boschert, U., Raine, C., and Selmaj, K. (2003). cDNA microarray analysis in multiple sclerosis lesions: Detection of genes associated with disease activity. Brain 126 (Pt 5), 1048–1057. doi: 10.1093/brain/awg107
Nathanson, J., and Chun, L. (1989). Immunological function of the blood-cerebrospinal fluid barrier. Proc. Natl. Acad. Sci. U.S.A. 86, 1684–1688. doi: 10.1073/pnas.86.5.1684
Naves, R., Singh, S., Cashman, K., Rowse, A., Axtell, R., Steinman, L., et al. (2013). The interdependent, overlapping, and differential roles of type I and II IFNs in the pathogenesis of experimental autoimmune encephalomyelitis. J. Immunol. 191, 2967–2977. doi: 10.4049/jimmunol.1300419
Ni, C., Wang, C., Zhang, J., Qu, L., Liu, X., Lu, Y., et al. (2014). Interferon-γ safeguards blood-brain barrier during experimental autoimmune encephalomyelitis. Am. J. Pathol. 184, 3308–3320. doi: 10.1016/j.ajpath.2014.08.019
Nishihara, H., Perriot, S., Gastfriend, B., Steinfort, M., Cibien, C., Soldati, S., et al. (2022). Intrinsic blood-brain barrier dysfunction contributes to multiple sclerosis pathogenesis. Brain 145, 4334–4348. doi: 10.1093/brain/awac019
Nishihara, H., Soldati, S., Mossu, A., Rosito, M., Rudolph, H., Muller, W., et al. (2020). Human CD4+ T cell subsets differ in their abilities to cross endothelial and epithelial brain barriers in vitro. Fluids Barriers CNS. 17:3. doi: 10.1186/s12987-019-0165-2
O’Connor, R., Prendergast, C., Sabatos, C., Lau, C., Leech, M., Wraith, D., et al. (2008). Cutting edge: Th1 cells facilitate the entry of Th17 cells to the central nervous system during experimental autoimmune encephalomyelitis. J. Immunol. 181, 3750–3754. doi: 10.4049/jimmunol.181.6.3750
Ortiz, G., Pacheco-Moisés, F., Macías-Islas, M., Flores-Alvarado, L., Mireles-Ramírez, M., González-Renovato, E., et al. (2014). Role of the blood-brain barrier in multiple sclerosis. Arch. Med. Res. 45, 687–697. doi: 10.1016/j.arcmed.2014.11.013
Panitch, H., Hirsch, R., Haley, A., and Johnson, K. (1987). Exacerbations of multiple sclerosis in patients treated with gamma interferon. Lancet 1, 893–895. doi: 10.1016/s0140-6736(87)92863-7
Piccio, L., Rossi, B., Colantonio, L., Grenningloh, R., Gho, A., Ottoboni, L., et al. (2005). Efficient recruitment of lymphocytes in inflamed brain venules requires expression of cutaneous lymphocyte antigen and fucosyltransferase-VII. J. Immunol. 174, 5805–5813. doi: 10.4049/jimmunol.174.9.5805
Piccio, L., Rossi, B., Scarpini, E., Laudanna, C., Giagulli, C., Issekutz, A., et al. (2002). Molecular mechanisms involved in lymphocyte recruitment in inflamed brain microvessels: Critical roles for P-selectin glycoprotein ligand-1 and heterotrimeric G(i)-linked receptors. J. Immunol. 168, 1940–1949. doi: 10.4049/jimmunol.168.4.1940
Pierson, E., Simmons, S., Castelli, L., and Goverman, J. (2012). Mechanisms regulating regional localization of inflammation during CNS autoimmunity. Immunol. Rev. 248, 205–215. doi: 10.1111/j.1600-065X.2012.01126.x
Planas, R., Metz, I., Ortiz, Y., Vilarrasa, N., Jelčić, I., Salinas-Riester, G., et al. (2015). Central role of Th2/Tc2 lymphocytes in pattern II multiple sclerosis lesions. Ann. Clin. Transl. Neurol. 2, 875–893. doi: 10.1002/acn3.218
Plumb, J., McQuaid, S., Mirakhur, M., and Kirk, J. (2002). Abnormal endothelial tight junctions in active lesions and normal-appearing white matter in multiple sclerosis. Brain Pathol. 12, 154–169. doi: 10.1111/j.1750-3639.2002.tb00430.x
Pol, S., Schweser, F., Bertolino, N., Preda, M., Sveinsson, M., Sudyn, M., et al. (2019). Characterization of leptomeningeal inflammation in rodent experimental autoimmune encephalomyelitis (EAE) model of multiple sclerosis. Exp. Neurol. 314, 82–90. doi: 10.1016/j.expneurol.2019.01.013
Polman, C., O’Connor, P., Havrdova, E., Hutchinson, M., Kappos, L., Miller, D., et al. (2006). A randomized, placebo-controlled trial of natalizumab for relapsing multiple sclerosis. N. Engl. J. Med. 354, 899–910. doi: 10.1056/NEJMoa044397
Polman, C., Reingold, S., Banwell, B., Clanet, M., Cohen, J., Filippi, M., et al. (2011). Diagnostic criteria for multiple sclerosis: 2010 revisions to the McDonald criteria. Ann. Neurol. 69, 292–302. doi: 10.1002/ana.22366
Prinz, M., and Priller, J. (2017). The role of peripheral immune cells in the CNS in steady state and disease. Nat. Neurosci. 20, 136–144. doi: 10.1038/nn.4475
Quandt, J., and Dorovini-Zis, K. (2004). The beta chemokines CCL4 and CCL5 enhance adhesion of specific CD4+ T cell subsets to human brain endothelial cells. J. Neuropathol. Exp. Neurol. 63, 350–362. doi: 10.1093/jnen/63.4.350
Rahman, M., Ghosh, C., Hossain, M., Linfield, D., Rezaee, F., Janigro, D., et al. (2018). IFN-γ, IL-17A, or zonulin rapidly increase the permeability of the blood-brain and small intestinal epithelial barriers: Relevance for neuro-inflammatory diseases. Biochem. Biophys. Res. Commun. 507, 274–279. doi: 10.1016/j.bbrc.2018.11.021
Reboldi, A., Coisne, C., Baumjohann, D., Benvenuto, F., Bottinelli, D., Lira, S., et al. (2009). C-C chemokine receptor 6-regulated entry of TH-17 cells into the CNS through the choroid plexus is required for the initiation of EAE. Nat. Immunol. 10, 514–523. doi: 10.1038/ni.1716
Rivers, T., Sprunt, D., and Berry, G. (1933). Observations on attempts to produce acute disseminated encephalomyelitis in monkeys. J. Exp. Med. 58, 39–53.
Rossi, B., and Constantin, G. (2016). Live imaging of immune responses in experimental models of multiple sclerosis. Front. Immunol. 7:506. doi: 10.3389/fimmu.2016.00506
Rossi, B., Angiari, S., Zenaro, E., Budui, S., and Constantin, G. (2011). Vascular inflammation in central nervous system diseases: Adhesion receptors controlling leukocyte-endothelial interactions. J. Leukoc. Biol. 89, 539–556. doi: 10.1189/jlb.0710432
Rossi, B., Santos-Lima, B., Terrabuio, E., Zenaro, E., and Constantin, G. (2021). Common peripheral immunity mechanisms in multiple sclerosis and Alzheimer’s disease. Front. Immunol. 12:639369. doi: 10.3389/fimmu.2021.639369
Rothhammer, V., Heink, S., Petermann, F., Srivastava, R., Claussen, M., Hemmer, B., et al. (2011). Th17 lymphocytes traffic to the central nervous system independently ofα4 integrin expression during EAE. J. Exp. Med. 208, 2465–2476. doi: 10.1084/jem.20110434
Rustenhoven, J., Drieu, A., Mamuladze, T., de Lima, K., Dykstra, T., Wall, M., et al. (2021). Functional characterization of the dural sinuses as a neuroimmune interface. Cell 184, 1000.e–1016.e. doi: 10.1016/j.cell.2020.12.040
Saint-Pol, J., Gosselet, F., Duban-Deweer, S., Pottiez, G., and Karamanos, Y. (2020). Targeting and crossing the blood-brain barrier with extracellular vesicles. Cells 9:851. doi: 10.3390/cells9040851
Sawcer, S., Ban, M., Maranian, M., Yeo, T., Compston, A., Kirby, A., et al. (2005). A high-density screen for linkage in multiple sclerosis. Am. J. Hum. Genet. 77, 454–467. doi: 10.1086/444547
Schläger, C., Körner, H., Krueger, M., Vidoli, S., Haberl, M., Mielke, D., et al. (2016). Effector T-cell trafficking between the leptomeninges and the cerebrospinal fluid. Nature 530, 349–353. doi: 10.1038/nature16939
Schneider-Hohendorf, T., Rossaint, J., Mohan, H., Böning, D., Breuer, J., Kuhlmann, T., et al. (2014). VLA-4 blockade promotes differential routes into human CNS involving PSGL-1 rolling of T cells and MCAM-adhesion of TH17 cells. J. Exp. Med. 211, 1833–1846. doi: 10.1084/jem.20140540
Segal, B. (2019). The diversity of encephalitogenic CD4+ T cells in multiple sclerosis and its animal models. J. Clin. Med. 8:120. doi: 10.3390/jcm8010120
Sen, M., Almuslehi, M., Shortland, P., Coorssen, J., and Mahns, D. (2020). Revisiting the pathoetiology of multiple sclerosis: Has the tail been wagging the mouse? Front. Immunol. 11:572186. doi: 10.3389/fimmu.2020.572186
Serafini, B., Rosicarelli, B., Magliozzi, R., Stigliano, E., and Aloisi, F. (2004). Detection of ectopic B-cell follicles with germinal centers in the meninges of patients with secondary progressive multiple sclerosis. Brain Pathol. 14, 164–174. doi: 10.1111/j.1750-3639.2004.tb00049.x
Setiadi, A., Abbas, A., Jeet, S., Wong, K., Bischof, A., Peng, I., et al. (2019). IL-17A is associated with the breakdown of the blood-brain barrier in relapsing-remitting multiple sclerosis. J. Neuroimmunol. 332, 147–154. doi: 10.1016/j.jneuroim.2019.04.011
Shipley, F., Dani, N., Xu, H., Deister, C., Cui, J., Head, J., et al. (2020). Tracking Calcium dynamics and immune surveillance at the choroid plexus blood-cerebrospinal fluid interface. Neuron 108, 623.e–639.e. doi: 10.1016/j.neuron.2020.08.024
Shrestha, B., Jiang, X., Ge, S., Paul, D., Chianchiano, P., and Pachter, J. (2017). Spatiotemporal resolution of spinal meningeal and parenchymal inflammation during experimental autoimmune encephalomyelitis. Neurobiol. Dis. 108, 159–172. doi: 10.1016/j.nbd.2017.08.010
Siffrin, V., Brandt, A., Radbruch, H., Herz, J., Boldakowa, N., Leuenberger, T., et al. (2009). Differential immune cell dynamics in the CNS cause CD4+ T cell compartmentalization. Brain 132(Pt 5), 1247–1258. doi: 10.1093/brain/awn354
Siffrin, V., Radbruch, H., Glumm, R., Niesner, R., Paterka, M., Herz, J., et al. (2010). In vivo imaging of partially reversible th17 cell-induced neuronal dysfunction in the course of encephalomyelitis. Immunity 33, 424–436. doi: 10.1016/j.immuni.2010.08.018
Sixt, M., Engelhardt, B., Pausch, F., Hallmann, R., Wendler, O., and Sorokin, L. (2001). Endothelial cell laminin isoforms, laminins 8 and 10, play decisive roles in T cell recruitment across the blood-brain barrier in experimental autoimmune encephalomyelitis. J. Cell Biol. 153, 933–946. doi: 10.1083/jcb.153.5.933
Smyth, L., Rustenhoven, J., Park, T., Schweder, P., Jansson, D., Heppner, P., et al. (2018). Unique and shared inflammatory profiles of human brain endothelia and pericytes. J. Neuroinflammation 15:138. doi: 10.1186/s12974-018-1167-8
Solár, P., Zamani, A., Kubíčková, L., Dubový, P., and Joukal, M. (2020). Choroid plexus and the blood-cerebrospinal fluid barrier in disease. Fluids Barriers CNS 17:35. doi: 10.1186/s12987-020-00196-2
Sonar, S., Shaikh, S., Joshi, N., Atre, A., and Lal, G. (2017). IFN-γ promotes transendothelial migration of CD4+ T cells across the blood-brain barrier. Immunol. Cell Biol. 95, 843–853. doi: 10.1038/icb.2017.56
Soon, D., Tozer, D., Altmann, D., Tofts, P., and Miller, D. (2007). Quantification of subtle blood-brain barrier disruption in non-enhancing lesions in multiple sclerosis: A study of disease and lesion subtypes. Mult. Scler. 13, 884–894. doi: 10.1177/1352458507076970
Sospedra, M., and Martin, R. (2005). Immunology of multiple sclerosis. Annu. Rev. Immunol. 23, 683–747. doi: 10.1146/annurev.immunol.23.021704.115707
Spencer, J., Bell, J., and DeLuca, G. (2018). Vascular pathology in multiple sclerosis: Reframing pathogenesis around the blood-brain barrier. J. Neurol. Neurosurg. Psychiatry 89, 42–52. doi: 10.1136/jnnp-2017-316011
Steffen, B., Breier, G., Butcher, E., Schulz, M., and Engelhardt, B. (1996). ICAM-1, VCAM-1, and MAdCAM-1 are expressed on choroid plexus epithelium but not endothelium and mediate binding of lymphocytes in vitro. Am. J. Pathol. 148, 1819–1838.
Steffen, B., Butcher, E., and Engelhardt, B. (1994). Evidence for involvement of ICAM-1 and VCAM-1 in lymphocyte interaction with endothelium in experimental autoimmune encephalomyelitis in the central nervous system in the SJL/J mouse. Am. J. Pathol. 145, 189–201.
Steiner, O., Coisne, C., Cecchelli, R., Boscacci, R., Deutsch, U., Engelhardt, B., et al. (2010). Differential roles for endothelial ICAM-1, ICAM-2, and VCAM-1 in shear-resistant T cell arrest, polarization, and directed crawling on blood-brain barrier endothelium. J. Immunol. 185, 4846–4855. doi: 10.4049/jimmunol.0903732
Steinman, L. (2001). Multiple sclerosis: A two-stage disease. Nat. Immunol. 2, 762–764. doi: 10.1038/ni0901-762
Strominger, I., Elyahu, Y., Berner, O., Reckhow, J., Mittal, K., Nemirovsky, A., et al. (2018). The Choroid plexus functions as a niche for T-cell stimulation within the central nervous system. Front. Immunol. 9:1066. doi: 10.3389/fimmu.2018.01066
Stromnes, I., Cerretti, L., Liggitt, D., Harris, R., and Goverman, J. (2008). Differential regulation of central nervous system autoimmunity by T(H)1 and T(H)17 cells. Nat. Med. 14, 337–342. doi: 10.1038/nm1715
Stys, P., Zamponi, G., van Minnen, J., and Geurts, J. (2012). Will the real multiple sclerosis please stand up? Nat. Rev. Neurosci. 13, 507–514. doi: 10.1038/nrn3275
Takata, F., Nakagawa, S., Matsumoto, J., and Dohgu, S. (2021). Blood-brain barrier dysfunction amplifies the development of neuroinflammation: Understanding of cellular events in brain microvascular endothelial cells for prevention and treatment of BBB dysfunction. Front. Cell Neurosci. 15:661838. doi: 10.3389/fncel.2021.661838
Tillery, E., Clements, J., and Howard, Z. (2018). What’s new in multiple sclerosis? Ment. Health Clin. 7, 213–220. doi: 10.9740/mhc.2017.09.213
Treabă, C., Bălaşa, R., Podeanu, D., Simu, I., and Buruian, M. (2014). Cerebral lesions of multiple sclerosis: Is gadolinium always irreplaceable in assessing lesion activity? Diagn. Interv. Radiol. 20, 178–184. doi: 10.5152/dir.2013.13313
Tzartos, J., Friese, M., Craner, M., Palace, J., Newcombe, J., Esiri, M., et al. (2008). Interleukin-17 production in central nervous system-infiltrating T cells and glial cells is associated with active disease in multiple sclerosis. Am. J. Pathol. 172, 146–155. doi: 10.2353/ajpath.2008.070690
Uchida, Y., Sumiya, T., Tachikawa, M., Yamakawa, T., Murata, S., Yagi, Y., et al. (2019). Involvement of Claudin-11 in disruption of blood-brain, -spinal cord, and -arachnoid barriers in multiple sclerosis. Mol. Neurobiol. 56, 2039–2056. doi: 10.1007/s12035-018-1207-5
van Deurs, B., and Koehler, J. (1979). Tight junctions in the choroid plexus epithelium. A freeze-fracture study including complementary replicas. J. Cell Biol. 80, 662–673. doi: 10.1083/jcb.80.3.662
Van Hove, H., Martens, L., Scheyltjens, I., De Vlaminck, K., Pombo Antunes, A., De Prijck, S., et al. (2019). A single-cell atlas of mouse brain macrophages reveals unique transcriptional identities shaped by ontogeny and tissue environment. Nat. Neurosci. 22, 1021–1035. doi: 10.1038/s41593-019-0393-4
van Wetering, S., van den Berk, N., van Buul, J., Mul, F., Lommerse, I., Mous, R., et al. (2003). VCAM-1-mediated Rac signaling controls endothelial cell-cell contacts and leukocyte transmigration. Am. J. Physiol. Cell Physiol. 285, C343–C352. doi: 10.1152/ajpcell.00048.2003
Vercellino, M., Votta, B., Condello, C., Piacentino, C., Romagnolo, A., Merola, A., et al. (2008). Involvement of the choroid plexus in multiple sclerosis autoimmune inflammation: A neuropathological study. J. Neuroimmunol. 199, 133–141. doi: 10.1016/j.jneuroim.2008.04.035
Vestweber, D. (2015). How leukocytes cross the vascular endothelium. Nat. Rev. Immunol. 15, 692–704. doi: 10.1038/nri3908
Voorthuis, J., Uitdehaag, B., De Groot, C., Goede, P., van der Meide, P., and Dijkstra, C. (1990). Suppression of experimental allergic encephalomyelitis by intraventricular administration of interferon-gamma in Lewis rats. Clin. Exp. Immunol. 81, 183–188. doi: 10.1111/j.1365-2249.1990.tb03315.x
Wagers, A., Waters, C., Stoolman, L., and Kansas, G. (1998). Interleukin 12 and interleukin 4 control T cell adhesion to endothelial selectins through opposite effects on alpha1, 3-fucosyltransferase VII gene expression. J. Exp. Med. 188, 2225–2231. doi: 10.1084/jem.188.12.2225
Wagner, M., Bilinska, M., Pokryszko-Dragan, A., Sobczynski, M., Cyrul, M., Kusnierczyk, P., et al. (2014). ALCAM and CD6–multiple sclerosis risk factors. J. Neuroimmunol. 276, 98–103. doi: 10.1016/j.jneuroim.2014.08.621
Wallström, E., Khademi, M., Andersson, M., and Olsson, T. (2000). Increased numbers of mononuclear cells from blood and CSF expressing interferon-gamma mRNA in multiple sclerosis are from both the CD4+ and the CD8+ subsets. Eur. J. Neurol. 7, 71–76. doi: 10.1046/j.1468-1331.2000.00027.x
Wang, Q., and Doerschuk, C. (2000). Neutrophil-induced changes in the biomechanical properties of endothelial cells: Roles of ICAM-1 and reactive oxygen species. J. Immunol. 164, 6487–6494. doi: 10.4049/jimmunol.164.12.6487
Washington, R., Burton, J., Todd, R., Newman, W., Dragovic, L., and Dore-Duffy, P. (1994). Expression of immunologically relevant endothelial cell activation antigens on isolated central nervous system microvessels from patients with multiple sclerosis. Ann. Neurol. 35, 89–97. doi: 10.1002/ana.410350114
Waxman, S. (2008). Axonal dysfunction in chronic multiple sclerosis: Meltdown in the membrane. Ann. Neurol. 63, 411–413. doi: 10.1002/ana.21361
Weiner, H., Friedman, A., Miller, A., Khoury, S., al-Sabbagh, A., Santos, L., et al. (1994). Oral tolerance: Immunologic mechanisms and treatment of animal and human organ-specific autoimmune diseases by oral administration of autoantigens. Annu. Rev. Immunol. 12, 809–837. doi: 10.1146/annurev.iy.12.040194.004113
Willenborg, D., Fordham, S., Bernard, C., Cowden, W., and Ramshaw, I. (1996). IFN-gamma plays a critical down-regulatory role in the induction and effector phase of myelin oligodendrocyte glycoprotein-induced autoimmune encephalomyelitis. J. Immunol. 157, 3223–3227.
Williams, J., Manivasagam, S., Smith, B., Sim, J., Vollmer, L., Daniels, B., et al. (2020). Astrocyte-T cell crosstalk regulates region-specific neuroinflammation. Glia 68, 1361–1374. doi: 10.1002/glia.23783
Wimmer, I., Tietz, S., Nishihara, H., Deutsch, U., Sallusto, F., Gosselet, F., et al. (2019). PECAM-1 stabilizes blood-brain barrier integrity and favors paracellular T-cell diapedesis across the blood-brain barrier during neuroinflammation. Front. Immunol. 10:711. doi: 10.3389/fimmu.2019.00711
Wojkowska, D., Szpakowski, P., and Glabinski, A. (2017). Interleukin 17A promotes lymphocytes adhesion and induces CCL2 and CXCL1 release from brain endothelial cells. Int. J. Mol. Sci. 18:1000. doi: 10.3390/ijms18051000
Wolburg, H., and Paulus, W. (2010). Choroid plexus: Biology and pathology. Acta Neuropathol. 119, 75–88. doi: 10.1007/s00401-009-0627-8
Wolburg, K., Gerhardt, H., Schulz, M., Wolburg, H., and Engelhardt, B. (1999). Ultrastructural localization of adhesion molecules in the healthy and inflamed choroid plexus of the mouse. Cell Tissue Res. 296, 259–269. doi: 10.1007/s004410051287
Wu, C., Ivars, F., Anderson, P., Hallmann, R., Vestweber, D., Nilsson, P., et al. (2009). Endothelial basement membrane laminin alpha5 selectively inhibits T lymphocyte extravasation into the brain. Nat. Med. 15, 519–527. doi: 10.1038/nm.1957
Yan, Y., Yu, J., Gao, Y., Kumar, G., Guo, M., Zhao, Y., et al. (2019). Therapeutic potentials of the Rho kinase inhibitor Fasudil in experimental autoimmune encephalomyelitis and the related mechanisms. Metab. Brain Dis. 34, 377–384. doi: 10.1007/s11011-018-0355-7
Yates, R., Esiri, M., Palace, J., Jacobs, B., Perera, R., and DeLuca, G. (2017). Fibrin(ogen) and neurodegeneration in the progressive multiple sclerosis cortex. Ann. Neurol. 82, 259–270. doi: 10.1002/ana.24997
Yednock, T., Cannon, C., Fritz, L., Sanchez-Madrid, F., Steinman, L., and Karin, N. (1992). Prevention of experimental autoimmune encephalomyelitis by antibodies against alpha 4 beta 1 integrin. Nature 356, 63–66. doi: 10.1038/356063a0
Young, N., Weinshenker, B., and Lucchinetti, C. (2008). Acute disseminated encephalomyelitis: Current understanding and controversies. Semin. Neurol. 28, 84–94. doi: 10.1055/s-2007-1019130
Zamvil, S., Nelson, P., Trotter, J., Mitchell, D., Knobler, R., Fritz, R., et al. (1985). T-cell clones specific for myelin basic protein induce chronic relapsing paralysis and demyelination. Nature 317, 355–358. doi: 10.1038/317355a0
Zenaro, E., Piacentino, G., and Constantin, G. (2017). The blood-brain barrier in Alzheimer’s disease. Neurobiol. Dis. 107, 41–56. doi: 10.1016/j.nbd.2016.07.007
Zenaro, E., Pietronigro, E., Della Bianca, V., Piacentino, G., Marongiu, L., Budui, S., et al. (2015). Neutrophils promote Alzheimer’s disease-like pathology and cognitive decline via LFA-1 integrin. Nat. Med. 21, 880–886. doi: 10.1038/nm.3913
Zenaro, E., Rossi, B., Angiari, S., and Constantin, G. (2013). Use of imaging to study leukocyte trafficking in the central nervous system. Immunol. Cell Biol. 91, 271–280. doi: 10.1038/icb.2012.81
Keywords: blood–brain barrier, multiple sclerosis, choroid plexus, T helper cells, neuroinflammation, adhesion molecules, meningeal inflammation
Citation: Angelini G, Bani A, Constantin G and Rossi B (2023) The interplay between T helper cells and brain barriers in the pathogenesis of multiple sclerosis. Front. Cell. Neurosci. 17:1101379. doi: 10.3389/fncel.2023.1101379
Received: 17 November 2022; Accepted: 31 January 2023;
Published: 15 February 2023.
Edited by:
Marek Joukal, Masaryk University, CzechiaReviewed by:
Jelena Skuljec, Essen University Hospital, GermanyCopyright © 2023 Angelini, Bani, Constantin and Rossi. This is an open-access article distributed under the terms of the Creative Commons Attribution License (CC BY). The use, distribution or reproduction in other forums is permitted, provided the original author(s) and the copyright owner(s) are credited and that the original publication in this journal is cited, in accordance with accepted academic practice. No use, distribution or reproduction is permitted which does not comply with these terms.
*Correspondence: Gabriela Constantin, Z2FicmllbGEuY29uc3RhbnRpbkB1bml2ci5pdA==; Barbara Rossi,
YmFyYmFyYS5yb3NzaUB1bml2ci5pdA==
†These authors share first authorship
Disclaimer: All claims expressed in this article are solely those of the authors and do not necessarily represent those of their affiliated organizations, or those of the publisher, the editors and the reviewers. Any product that may be evaluated in this article or claim that may be made by its manufacturer is not guaranteed or endorsed by the publisher.
Research integrity at Frontiers
Learn more about the work of our research integrity team to safeguard the quality of each article we publish.