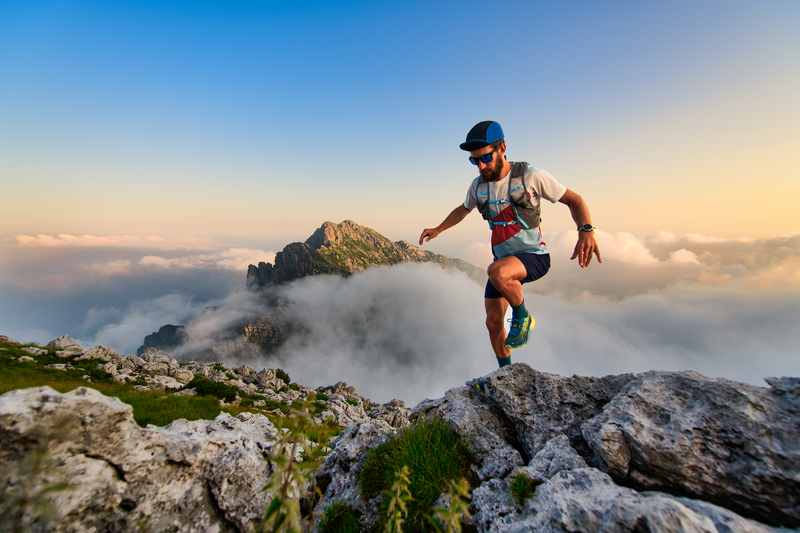
95% of researchers rate our articles as excellent or good
Learn more about the work of our research integrity team to safeguard the quality of each article we publish.
Find out more
REVIEW article
Front. Cell. Neurosci. , 30 September 2022
Sec. Cellular Neuropathology
Volume 16 - 2022 | https://doi.org/10.3389/fncel.2022.966202
This article is part of the Research Topic Endolysosomal and Autophagic Dysfunctions as Early Drivers of Neurodegeneration View all 8 articles
Sensorineural hearing loss (SNHL) is currently a major health issue. As one of the most common neurodegenerative diseases, SNHL is associated with the degradation of hair cells (HCs), spiral ganglion neurons (SGNs), the stria vascularis, supporting cells and central auditory system cells. Autophagy is a highly integrated cellular system that eliminates impaired components and replenishes energy to benefit cellular homeostasis. Etiological links between autophagy alterations and neurodegenerative diseases, such as SNHL, have been established. The hearing pathway is complex and depends on the comprehensive functions of many types of tissues and cells in auditory system. In this review, we discuss the roles of autophagy in promoting and inhibiting hearing, paying particular attention to specific cells in the auditory system, as discerned through research. Hence, our review provides enlightening ideas for the role of autophagy in hearing development and impairment.
As a key sensory basis for communication, hearing plays an essential role in the development of language and mental functions, and therefore, hearing loss can lead to a battery of economic difficulties and psychosocial problems (Shan et al., 2020; Yigider et al., 2020). For example, in individuals over 65 years old, hearing loss is a leading contributor to disability, which have important implications in the loneliness, social isolation, and cognitive decline (Lin et al., 2013; Shukla et al., 2020; Ge et al., 2021). Data from the WHO show that approximately one-half billion people worldwide have disabling hearing loss, and the incidence is expected to rise to 1 in 10 people by 2050 (Chadha et al., 2021). Therefore, hearing loss has come to be a major global health concern (Wilson et al., 2017; Campos and Launer, 2020).
Hearing loss can be categorized as conductive or sensorineural, which difference lies in the impairment of sound transmission or perception, and when both forms are evident, the condition is categorized as mixed (Cunningham and Tucci, 2017; Anastasiadou and Al, 2020). Caused by degenerative processes associated with aging, ototoxic drugs, noise exposure, and genetic mutations, SNHL is the most common type of hearing loss, and it is associated with a decrease in hearing sensitivity and an increase in hearing thresholds. Age-related hearing loss (AHL) is mainly caused by age-related degeneration at various auditory sites, which involves a gradually reduced hearing capacity and poor speech discernibility that is initially perceived in noisy environments. Many chemicals and clinical medications, such as aminoglycoside antibiotics and cisplatin, are known to induce deleterious ototoxic side effects. Loud sounds are ubiquitous in modern life and can damage hearing acuity, and loud noise exposure can result in temporary threshold shifts (TTSs) or permanent threshold shifts (PTSs) through direct mechanical stress and stress-induced molecular changes. Furthermore, researchers have indicated that certain genetic defects including mutations can lead to SNHL. Although SNHL is a common disease, SNHL pathogenetic mechanisms and interventions remain to be elucidated.
In the past decade, the field of autophagy research has grown exponentially (Mizushima, 2018). Since the breakthrough discovery of the molecular mechanism of autophagy by Yoshinori Ohsumi, who was awarded the Nobel Prize in 2016, considerable attention has been directed toward the role of physiopathological autophagy in various diseases (Levine and Kroemer, 2019). To maintain homeostasis, cells have evolved a self-regulating quality control system that enables adaptation to nutrient deprivation, metabolic stress, damaging challenges, and development or differentiation processes (Pohl and Dikic, 2019; Gross and Graef, 2020; Xiong et al., 2020; Yun et al., 2020). Given the prominent role of autophagy in organisms, it is important to investigate the extent to which autophagy contributes to hearing loss. Because autophagy is a programmed cell death process (Wu et al., 2020), several studies have been performed to determine whether interfering with autophagy may be potentially useful as a therapeutic strategy in SNHL. The results of these studies have increasingly shown that autophagy is connected to SNHL caused by ototoxic drugs, noise exposure, aging factors, and other causes.
In general, among the types of autophagy, the three most common forms are macroautophagy, microautophagy, and chaperone-mediated autophagy. Macroautophagy, hereafter referred to as autophagy, is the major type of autophagy and is regarded as a classical degradation pathway. Degradation pathway in cells under stress is vital for preventing several clinical conditions among which cancer, neurodegeneration, and SNHL (Levy et al., 2017; Fu and Chai, 2019; Djajadikerta et al., 2020; Yang and Klionsky, 2020). Significant progress has been made in understanding the features of autophagy, and a series of genes named autophagy-related genes (ATG) have been identified in this process (Figure 1A). In response to the aforementioned cell stress, the ATG1/ULK kinase complex activates autophagy. After the assembly of multiprotein complexes (including Beclin1 and VPS34), phosphatidylinositol 3-phosphate (PI3P) is generated and is involved in phagophore formation. Organelle membrane sources and PI3P production promote membrane elongation through the recruitment of the ATG2–WIPI (ATG18 in yeast) complex. In addition, ATG9 vesicles, consisting of transmembrane core ATG protein, contribute to the expanding phagophore membrane. Underscoring the importance of the ATG complex, ATG12 is conjugated to ATG5 and ATL16L1, and LC3 subfamily proteins are bound to phosphatidylethanolamine (PE), resulting in an expanding phagophore that engulfs autophagic cargo via autophagy receptors and the completion of double-membrane autophagosomes. Eventually, autophagosomes fuse with lysosomes, and thus mature into autophagolysosomes, in which the autophagy cargo is degraded (Glick et al., 2010; Morishita and Mizushima, 2019; Mizushima, 2020).
Figure 1. Autophagy in the cells of the auditory system. (A) In response to cellular stress, autophagy is promoted by AMPK or suppressed by mTORC1 and initiated by the activation of the ATG1/ULK kinase complex. After the assembly of multiprotein complexes (including Beclin1 and VPS34), the class III phosphatidylinositol 3-phosphate complex (PI3KC3) is generated to act in phagophore formation. Membrane sources from organelles and the production of PI3KC3 promote membrane elongation by recruitment of the ATG2–WIPI (ATG18 in yeast) complex. In addition, ATG9 vesicles, the transmembrane core ATG protein, contribute to the membrane supply. Underscoring the importance of the ATG complex, ATG12 conjugates with ATG5 and ATL16L1, and the LC3 subfamily conjugates with PE, resulting in an expanded phagophore engulfing autophagic cargo via autophagy receptors and the completion of double-membrane autophagosomes. Eventually, it gives rise to the maturation of autophagolysosomes and degradation following fusion with lysosomes. (B) Structure of the cochlea, especially meticulous cells in the scala media, filled with endolymph. The crucial part of auditory perception, hair cells (including IHC and OHC), are located on the basilar membrane and surrounded by epithelia-supporting cells in the Organ of Corti. Sensory HCs make synaptic connections with SGNs whose dendrites form connections with the cochlear nucleus. The stria vascularis lies on the lateral wall, and work with supporting cells plays an important role in cochlear homeostasis and acoustic function.
To reduce cell stress, reactive oxygen species (ROS) and damaged organelles, can be removed through the autophagy mechanism described. As expected, previous reviews have suggested that in SNHL of various etiologies, autophagy leads to a certain antioxidative effect (He et al., 2018; Ye et al., 2018). However, these reviews mainly discussed different categories of hearing loss, not diverse structures or cells involved in hearing loss. Autophagy in auditory system diseases is cell-specific and difficult to generalize on the basis of type of deafness. Therefore, increasing attention has been directed to the significance of cell specificity in hearing loss (Takeda et al., 2018; McGovern et al., 2019; Roccio et al., 2020; van der Valk et al., 2021). Herein, we update the progress in understanding autophagy in auditory development and hearing loss, uniquely focusing on cellular components. Cognition of cellular state not only can lead to a better understanding of the specific role of autophagy in physiological and pathological auditory systems but can also indicate future directions in the work of this field.
The cochlea, a mammalian sensor stimulated by environmental sounds, is a sophisticated and minute structure (Raphael and Altschuler, 2003; Stephenson, 2012; Iyer et al., 2016; Wright and Horn, 2016; Fettiplace, 2017). Figure 1B shows that the membranous cochlea comprises three ducts filled with endo/perilymph. The membranous cochlear duct, also called scala media, is the middle duct, and plays the most important role in sound sensing. The scala media is bounded by the vestibular membrane (on the superior side) and basilar membrane (on the basal side). The spiral ligament and stria vascularis comprise the lateral wall. Located on the basilar membrane, the spiral organ, also known as the organ of Corti, includes inner/outer hair cells (IHC/OHC), supporting cells, and the tectorial membrane. Overall, cells in the cochlea and surrounding structures are crucial for auditory perception and transmission. A full understanding of autophagy in specific cell types has been the basis for the physiological and pathological studies described in detail below.
Autophagy is required during the development of multiple organisms (Allen and Baehrecke, 2020). Otocysts, also named otic vesicles, are the origins of the developing sensory organs in the early embryonic inner ear. Otic vesicle ventral cells give rise to the auditory organ consisting of HCs, several types of supporting cells and neurons, which together comprise the cochlea in mammals (Magariños et al., 2014). Interestingly, an excessive number of otic cells are generated during development and are later exquisitely cleared by regulated mechanisms such as apoptosis and senescence. Developmental senescence seems to control the balance of specific cell populations in endolymphatic sac formation and modulate the otic vesicle morphology. Autophagy provides the energy for the elimination of apoptotic cells and migration of otic neuroblasts (Aburto et al., 2012; Varela-Nieto et al., 2019). In contrast, deficient autophagy leads to aberrant inner ear development (Magariños et al., 2017).
Recently, reports cited certain deafness genes (DFNA5, DFNA59, DFNA67, and connexin26) linked to autophagy and described their roles in genetic hearing loss (Hayashi et al., 2020; Koh et al., 2022). It is conceivable that autophagy is a significant process in both antenatal and postnatal cochlear cell development. A study showed that mice deficient in the Atg5 gene presented with severe congenital hearing loss and HC degeneration, revealing that autophagy is requisite for HC morphogenesis and hearing acuity stabilization (Fujimoto et al., 2017). Similarly, a newly published study described time-dependent stereocilia damage, somatic electromotility disturbances, and synaptic ribbon degeneration in mouse OHCs due to the genetic ablation of Atg7, reconfirming the role of ATG-dependent autophagy in HC preservation and hearing (Zhou et al., 2020). Another study has proven that disruptions to autophagy are involved in impaired development of cochlear ribbon synapses between IHCs and SGNs, with reduced exocytosis by IHCs in postnatal mice before hearing onset (Xiong et al., 2020). In addition, after sevoflurane exposure in utero, offspring mice exhibited poor hearing, as measured by the auditory brainstem response (ABR) test, with degenerated ribbon synapses despite unchanged HCs. Remarkably, impaired autophagy has been observed in cochlear explant cultures (Yuan et al., 2020). The ATG, Becn1, Atg4, Atg5, and Atg9 genes have been reported to be expressed during mouse cochlear development and their upregulated expression has been associated with concerted inner ear functional maturity. In particular, LC3B is abundant in SGNs since the first month of life, which may signify the primary association of autophagy with SGN activity (de Iriarte Rodríguez et al., 2015). In contrast to apoptosis, autophagy is gradually increased during SGN development (Hou et al., 2020).
Although no single illustration of the role of autophagy in non-sensory cell development has been described, autophagy undoubtedly has an effect on hearing. However, more evidence is needed on cochlear cell development and autophagy in pathological auditory cells.
HCs are crucial to auditory perception and they exhibit limited regenerative capacity in mammals. Specifically, IHCs specialize in the transformation of mechanical force into an electrical signal, while OHCs enhance the quality and sensitivity of the transduced signal. Damage to HCs is the major cause of SNHL. Figure 2 shows the involvement of autophagy in HC dysfunction. Some groups have found that C57BL/6 mice are susceptible to early onset hearing loss and HC loss upon upregulation of miR-34a expression; indeed, in these mice, autophagic flux is impaired (Pang et al., 2017). Specifically, ATG9A was significantly decreased after miR-34a overexpression (Yang et al., 2013) and when Sirtuin 1 (SIRT1) expression was deficient. SIRT1 is a conserved NAD-dependent deacetylase that has been confirmed as a miR-34a target (Yamakuchi et al., 2008; Imai and Guarente, 2014). A study suggested that SIRT1 deacetylates ATG9A to induce autophagy, protecting HCs and delaying AHL (Pang et al., 2019). When exposed to noise, adult CBA/J mice undergo a TTS, PTS, or sPTS (severe permanent threshold shift), with elevated lipid oxidation and protein nitration (4-hydroxynonenal and 3-nitrotyrosine, respectively, leading increased oxidative stress), mainly in OHCs. Interestingly, potentiated autophagy has been observed in OHCs exposed to moderate noise levels (such as those associated with TTSs), but autophagy was unaltered in OHCs exposed to noise associated with sPTSs, while suppressed autophagy (inhibited by 3-MA or LC3B siRNA) converted TTS to PTS and exacerbated OHC death. Additionally, activation of autophagy by rapamycin attenuated oxidative stress and thus promoted OHC survival, suggesting that autophagy modulates HC death in hearing loss and that excessive oxidative stress eliminates the benefits of autophagy (Yuan et al., 2015). Pexophagy (Germain and Kim, 2020), a selective form of autophagy, has been recently linked to pejvakin-mediated protection of HCs against noise-induced oxidative damage (Defourny et al., 2019).
Figure 2. The delicate balance of autophagy in cell survival and death. Autophagy is involved in the auditory pathway, which is reviewed exactly from the peripheral to the central auditory system. Autophagy is activated by Fox family members, BNIP3L, Urolithin A, PINK1, AMPK, DRP-1, rapamycin, SIRT1, and PRDX1 but inhibited by miR-34a, 3-MA, GSK3β, and mTORC1 signaling in cells suffering from aging, noise and ototoxic drugs. Some common stresses usually exist, such as ROS and LPS. Accumulated lipofuscin and disrupted nuclear translocation of TFEB are shown in degenerated SGNs with impaired autophagy; in contrast, SGN degeneration is ameliorated after restoring autophagy and promoting TFEB nuclear translocation. Furthermore, autophagy is thought to defend against damage to the auditory cortex caused by aging or ototoxicity. Autophagy can alleviate these conditions and protect cells, although controversy remains. Activation of autophagy by Pou4f3 mutation and NLRX1 mediation can accelerate the ototoxic potential of cisplatin in hair cells and HEI-OC1 cells. Most importantly, appropriate autophagy plays a vital role in maintaining the balance of cell homeostasis.
As studies have progressed, increasing evidence has supported the idea that autophagy is closely associated with the alleviation of drug-induced ototoxicity of inner ear cells. Ototoxicity is an attention-attracting adverse effect of cisplatin (Yu et al., 2020), and cisplatin exposure as well as noise induce apoptosis and autophagy in HCs (Xu et al., 2018). However, the effect of autophagy on cisplatin-induced ototoxicity in HCs remains ambiguous and is debated. A study revealed an otoprotective effect of rapamycin, which reduces HC loss after cisplatin treatment by inducing autophagy (Fang and Xiao, 2014). The protective effect of autophagy induced by SIRT1 has also been described in age-dependent HC loss (Xiong et al., 2015; Pang et al., 2019). Pang et al. (2018) proposed that SIRT1-induced autophagy activation attenuates cisplatin-induced HC death in the mouse cochlea and zebrafish lateral line. Moreover, promoted autophagy in HCs accompanied by glycogen synthase kinase 3β (GSK3β) inhibition or PTEN-induced putative kinase 1 (PINK1) activation has been reported to alleviate cisplatin-induced ototoxicity. PINK1, known for its role in mitophagy, inhibits JNK pathway-related apoptosis induced in response to cisplatin injury of C57BL/6 murine cochlear explants (Yang et al., 2018a; Liu T. et al., 2019). Intriguingly, autophagy may accelerate cisplatin-induced cytotoxicity, but debate on this possibility continues (Yin et al., 2018). Recent research has indicated that mutation of the transcription factor Pou4f3 promotes apoptotic HC death by inducing autophagy in cisplatin-treated murine models of deafness (Xu et al., 2020). It is difficult to elucidate the reasons for the discrepancies in different conclusions, but they might be related to various experimental subjects. Alternatively, diversities in the timing and extent of autophagy activation may explain the differences. For example, early and moderate upregulation of autophagy has been shown to exert a protective function, but later and excessive autophagy leads to a deleterious outcome (Maiuri et al., 2007). Aminoglycoside antibiotics constitute a class of noted ototoxic medicines that includes neomycin and gentamicin, which seem to enter HCs by endocytosis through the apical membrane, basolateral membrane or mechanoelectrical transducer (MET) channel located atop stereocilia (Huth et al., 2011). A study revealed that autophagy was increased in HC explants after 2 mM neomycin treatment, and stimulation of autophagy by rapamycin rescued HCs from ROS injury and death (He et al., 2017). Other studies have shown that in gentamicin-treated cochlear HCs, multiple autophagic vacuole formed, lysosomal fusion was decreased and autophagic flux was impaired, which caused delayed onset ototoxicity (Kim et al., 2017, 2019). These studies suggest that enhancing autophagic flux might prevent aminoglycoside-induced SNHL, although we need be aware of the proapoptotic effects of autophagy overactivation.
The stria vascularis consists of three layers of cells, including marginal, intermediate, and basal cells. It is critical for maintaining endocochlear potential (EP) and endolymph ion homeostasis and is constantly exposed to cell stress because of its high metabolic activity. It is generally assumed that the K + cycle is one of the most important mechanisms in cochlear cells; indeed, a normal stria is essential for HC mechanosensory function (Liu et al., 2016). Aberrant stria vascularis is involved in multiple types of hearing loss (Shi, 2016; Tawfik et al., 2019) and is associated with heavy oxidative burden, microvascular insults, and depletion of ion transport channels and pumps, all of which contribute to stria vascularis atrophy and, eventually, to hearing loss. Pillar, Deiters’, and Hensen’s cells are all portrayed as supporting cells with distinct morphology, location, and function, providing structural and molecular support for HCs. Furthermore, supporting cells are thought to be involved in the development and survival of HCs and SGNs and show remarkable potential for promoting HC regeneration, analogous to glia in the central nervous system (Monzack and Cunningham, 2013). Emerging evidence has suggested that the earliest signs of ototoxic drug-induced death appear in supporting cells (Ding et al., 2020); however, supporting cells are not as well characterized as sensory cells, and they deserve further investigation in efforts to prevent hearing loss.
Unfortunately, few studies have focused on autophagy related to dysfunctional non-sensory cells in hearing loss, and this aspect remains to be addressed. Poly (ADP-ribose) polymerase-1 (PARP-1) is activated under oxidative stress in marginal cells of the stria vascularis, particularly the epithelium-derived cells lining the endolymphatic surface, and subsequently induces PARP-1-dependent cell death (parthanatos). Synchronously, autophagy has been observed in these marginal cells, and autophagy suppression by 3-MA has led to aggravated parthanatos, demonstrating a prosurvival role played by autophagy in marginal cells (Jiang et al., 2018). Outer sulcus cells (OSCs) are non-sensory cells in the cochlear lateral wall, and abnormal protein aggregations in OSCs are caused by a genetic disorder with hearing loss (Pendred syndrome). It has been reported that, even at low doses, rapamycin activates autophagy in OSCs in vivo, thereby ameliorating anomalous protein aggregation and reducing the susceptibility of cells to disease (Saegusa et al., 2020). Additionally, as previously described (Lee et al., 2017), clusterin (CLU) has been found to be expressed in basal cells of the stria vascularis, pillar cells and Deiters’ cells in developing and mature mouse cochlea, and it has been identified as a stress-activated signaling chaperone molecule that exerts a cytoprotective effects by clearing misfolded or aggregated proteins. Studies have proven that high CLU expression in tumor cells promotes autophagy, thereby regulating the prosurvival pathway in cancer (Zhang et al., 2014; Fu et al., 2020); therefore, determination of whether CLU-related autophagy has a potential effect on the stria vascularis and supporting cells is a valid research consideration.
Due to the elaborate organization of components and movements of stereocilia that protrude from the apical domain of HCs, sound impulses are transduced to the auditory nerve. There are two types of auditory nerves that arise from the differentiation of SGNs (Petitpré et al., 2018; Liu W. et al., 2019), and their peripheral processes contact HCs. Type I SGNs receive 95% afferent innervation with IHCs through ribbon synapsis development (Coate et al., 2019), and OHCs make synaptic connections with Type II SGNs. Interestingly, OHCs receive a major efferent innervation, which contributes to an efferent feedback loop with medial olivocochlear system (Guinan, 2018). With the intervention of primary sensory neurons of SGN, acoustic information is transmitted from the cochlea to the central nervous system. SGN lesions lead to SNHL due to impaired neurotrophic signaling, neurotransmitter excitotoxicity, gene deficiency and oxidative imbalances caused by aging, noise and ototoxic drugs (Nayagam et al., 2011; Liu W. et al., 2019). Herein, we explain the involvement of autophagy in SGN physiology and injury-associated hearing loss.
SGNs gradually deteriorate with HC loss. In a recently published study (Ye et al., 2019), impaired autophagic flux was found during the incipient stage of SGN degeneration. Lipofuscin and autophagic vacuoles accumulated in the cytoplasm of the degenerated SGNs in mice, and the nuclear translocation of TFEB, a transcription factor that regulates lysosomal and autophagic function, was disrupted. After intervention to promote TFEB nuclear translocation, autophagy-lysosomal function was restored, attenuating SGN degeneration. It has been demonstrated in latest reports on TFEB-mediated autophagy against aging or cisplatin-induced HC damage as well (Li et al., 2022; Xiong et al., 2022). As mentioned above, rapamycin, a specific mTORC1 inhibitor, also enhances autophagy in SGNs and ameliorates AHL in C57BL/6J mice (Liu et al., 2022). In SAMP8 mice, which are used in gerontological research, the organ of Corti and SGNs were observed to exhibit abnormalities similar to those in AHL. Specifically, a high level of LC3-II expression in SGNs was sustained from the first to the twelfth month. Notably, triggered autophagy is widely considered to play a dual role in the survival of young individuals and the death of old individuals due to cell stress (Menardo et al., 2012).
SGNs are also the targets of ototoxic drug injury. For instance, treatment with 30 μM cisplatin caused ROS activation and apoptosis of SGNs from C57BL/6 murine cochlea in vitro and, moreover, activation of PINK, which elicits autophagy generally. Furthermore, PINK1 silencing resulted in weakened autophagy but intensified mortality, implying that PINK1-induced autophagy might confer a protective effect in cells stimulated by cisplatin (Yang et al., 2018a). Peroxiredoxin 1 (PRDX1) is a multifunctional antioxidant and is universally expressed in the cochlea as well as in SGNs (Le et al., 2017). A recent study investigated autophagy involvement in SGN apoptosis and hearing loss induced by cisplatin and showed that PRDX1 regulated PTEN-AKT signaling to activate autophagy, thus promoting SGN survival (Liu W. et al., 2021). Another study identified prominent downregulated expression of a gene in the SGNs of rat cochlear organotypic cultures after gentamicin treatment. Ubiquitin carboxyl-terminal hydrolase isozyme L1 (Uchl1), the deficit of which leads to SGN loss and impaired autophagic flux, showed the potential to salvage autophagy in SGNs dying because of gentamicin ototoxicity (Kim et al., 2019). Furthermore, hyperactivation of the mTORC1 signaling pathway was found to be involved in the drastic gentamicin-induced reduction of SGN density and neurite outgrowth, which was restrained by rapamycin, the well-known autophagy activator (Guo et al., 2019). In addition to medicines, industrial pollution is thought to cause auditory dysfunction in humans and animals. Zhang et al. (2019) demonstrated that adult guinea pigs subjected to 60 days of chronic exposure to the heavy metal lead showed SGN loss and corresponding hearing impairment, and increased expression of ATG5, ATG6, and LC3B was found in the guinea pig brainstem after 30 days of lead exposure. Therefore, it has been suggested that autophagy possibly confers a protective effect in the early stage of lead exposure.
House Ear Institute-organ of Corti 1 (HEI-OC1) is one of the rare mouse auditory cell lines. An epithelial cell line derived from a conditionally immortalized organ of Corti, the HEI-OC1 cell line expresses markers of HCs, including prestin, myosin-VIIa (MYOSIN 7a), BDNF, Atoh1, calbindin, and calmodulin (Kalinec et al., 2003). HEI-OC1 cells were originally used to investigate the potential ototoxicity or otoprotective properties of drugs in systems in vitro (Kalinec et al., 2016), and they are now widely used to study evaluate apoptotic pathways, inflammatory responses, gene regulation, autophagy and senescence, and oxidative and endoplasmic reticulum (ER) stress in various types of SNHL.
Autophagy correlated with AMPK has been demonstrated to regulate oxidative stress-induced premature senescence of HEI-OC1 cells, and knockdown of Atg7 has been shown to induce premature senescence by impairing autophagy (Tsuchihashi et al., 2015). Based on in vivo findings (Pang et al., 2017, 2019), further exploration of HEI-OC1 cells has validated that overexpression of miR-34a impairs autophagic flux by repressing ATG9A expression, whereas SIRT1 activation deacetylates ATG9A to protect against HC loss and delay AHL through autophagy recovery. As a selective autophagy subtype, mitophagy has been observed to be decreased in aged cochlea of C57BL/6 mice, which might be a result of cell damage (Oh et al., 2020; Yu et al., 2021). In other words, activation of SIRT1 or inhibition of miR-34a equally protects HEI-OC1 cells against oxidative stress and delays AHL by maintaining a balance between mitophagy and mitochondrial biogenesis (Xiong et al., 2019). Urolithin A is reported as a mitophagy activator in various mammalian cells, as well as in auditory cells. Declined mitophagy is counteracted upon Urolithin A pre-treatment in H2O2-induced senescent HEI-OC1 cells, thus maintaining mitochondrial function and preventing age-related damage (Cho et al., 2022). Dynamin-related protein-1 (DRP-1) is a GTPase that contributes to mitochondrial fission and plays a core role in mitophagy (Reddy et al., 2011; Breitzig et al., 2018). Oxidative damage induces mitochondrial dysfunction in senescent HEI-OC1 cells, while DRP-1 overexpression rescues aged cochlea from AHL by initiating mitophagy (Lin et al., 2019). Similarly, BNIP3L/NIX, a member of the BCL2 family, has been suggested to regulate mitophagy against premature senescence (Kim et al., 2021). Forkhead box (FOX) family transcription factors are influential in mammalian development and disease and are molecular sensors that regulate the interactions between the genome and signaling responses to internal or external cues (Lam et al., 2013; Tia et al., 2018; Herman et al., 2021). FoxG1 has been implicated in promoted HC survival and development in postnatal cochlea of mice (He et al., 2019). Recently, a study has demonstrated reduced autophagy levels after downregulation of FoxG1 expression in aging HEI-OC1 cells. We now have a better understanding of the role of FoxG1 in activating autophagy, which may contribute to aging cell survival (He et al., 2020, 2021). Similarly, mutation of FoxO3 (a member of a FOX family subgroup) has been reported to cause adult-onset auditory neuropathy in mice (Gilels et al., 2013). When observing external damage to cells, Liang et al. (2021) found that the FoxO3 pathway-mediated autophagy machinery possibly exerted antagonistic effects against ototoxicity. Under ER stress-induced cell damage induced by tunicamycin, a FoxO family member mediated increased autophagic levels, reducing the HEI-OC1 cell death rate (Kishino et al., 2017).
Autophagy clearly acts as a survival mechanism that protects against HEI-OC1 cell death under oxidative stress (Hayashi et al., 2015; Tsuchihashi et al., 2015); similarly, this protective effect has been effectively shown against hearing loss induced by ototoxic drugs, while impaired mitophagy aggravates cytotoxicity (Cho et al., 2021). As models used for screening the pharmacological effects of ototoxicity, studies of autophagy mechanism in HEI-OC1 cells treated with drugs have proceed apace recently (Zhao et al., 2021). It has been reported that induction of autophagy and inhibition of the p53 signaling pathway by PINK can protect HEI-OC1 cells against gentamicin-induced damage (Yang et al., 2018b), although dissention among scholars remains (Setz et al., 2018). Similar to the results with HCs, the results of in vitro experiments with HEI-OC1 cells have shown beneficial (Pang et al., 2018; Yang et al., 2018a; Liu T. et al., 2019) and harmful (Youn et al., 2015; Li et al., 2018; Yin et al., 2018) impacts of autophagy on cisplatin-induced ototoxicity. The latest research has indicated that autophagy-dependent ferroptosis contributes to the ototoxicity induced by cisplatin. In this study, autophagic flux was blocked by chloroquine, attenuating cisplatin injury (Jian et al., 2021). Ferroptosis is a ROS- and iron-dependent cell death. Excessive release of redox-active iron has been hypothesized to result in ferroptosis, which depends on a specific form of autophagy called ferritinophagy (Biasiotto et al., 2016; Latunde-Dada, 2017; Tang et al., 2018). After cisplatin treatment, ferritinophagy was activated and augmented iron availability, which led to ferroptotic HEI-OC1 cell death. We analyzed and discussed autophagy duality in the previous section. Remarkably, HEI-OC1 cells were used to verify the results found with in HCs in vivo and to explain the specific mechanism of action. Consistent with the results obtained with in vivo and organotypic cochlear cultures, autophagy activity, including autophagosome-lysosome fusion, was activated in HEI-OC1 cells in response to neomycin or gentamicin treatment (He et al., 2017); however, a difference was observed in other studies showing increased autophagosome formation but not lysosome fusion (Kim et al., 2017, 2019). Surprisingly, administration of the autophagic flux activator rapamycin has been universally shown to reduce ROS levels and HC death, while treatment with the autophagy inhibitor 3-MA or deletion of an autophagy gene leads to cell apoptosis.
Sound information is converted from mechanical to bioelectrical signals by cochlear HCs, and then neural processing is initiated with nerve fibers consisting of SGNs. The central auditory system analyzes and deciphers sonic information, which proceeds sequentially through the cochlear nuclei, trapezoid body, superior olivary complex, lateral lemniscus, inferior colliculus, medial geniculate nucleus, and finally the auditory cortex. Due to the complexity of acoustic perception, pathological changes in the central auditory pathway, as shown in Figure 2 are worth examining. In particular, we focus on the few studies on autophagy.
Mammalian cochlear nuclei integrate acoustic and somatosensory information to localize sound sources. A study on hearing impairment in rats with diabetes reported that cochlear nucleus neurons were damaged by hyperglycemia and that the level of autophagy stimulus was increased (Xueqin et al., 2017). Another study (Fan et al., 2013) investigated the neurotoxic course of kanamycin (an aminoglycoside) in the rat dorsal cochlear nucleus, revealing aggravated injury to neurons while gradually recovered with early increases in autophagy. From the reversible damage process, we can deduce the potential protective role of promoted autophagy in kanamycin-induced neurotoxicity, but further elucidation is needed to determine the exact effect of promoted autophagy. The primary auditory cortex is the first integrative cortical area, where an elaborate network through which conscious perception and comprehension of sound and utterances are performed, and its degeneration is the major contributor to hearing loss, especially presbycusis (equally AHL). Mitophagy aids in removing ROS and maintaining mitochondrial homeostasis; however, these processes appear to be disrupted in the auditory cortex with aging. This finding indicated that mitochondrial autophagy is a promising mechanism for protecting the central auditory system against AHL (Youn et al., 2020). Using a D-galactose-induced rat model of aging, a study found that LC3 and Beclin1 were increased from young to adult in the auditory cortex because of AMPK-mTOR-ULK1 signaling, which maintained neuronal ultrastructural morphology. In contrast, these proteins were reduced at old, and the apoptosis rate and substantial neuron degeneration were increased. These results suggested antiapoptotic and antiaging functions of autophagy in the degeneration of the auditory cortex (Yuan et al., 2018). Additionally, SIRT1 has been found to play a protective role in initiating autophagy in the peripheral auditory system. A decrease in SIRT1 expression in the auditory cortex with aging has been reported, and this decrease may contribute to presbycusis (Xiong et al., 2014). Since research data have revealed that SIRT1 induces autophagy in response to survival stress, this result suggests the possibility of autophagy function in the auditory cortex. Altogether, evidence indicates that autophagy plays a role in the central auditory system, but more studies need to be performed to discern the relationship between autophagy and hearing loss in this field.
Hearing loss is a major cause of disability that continues to be invisible and is therefore, a silent epidemic. As shown in Table 1, we mainly reviewed the pathological factors, genesis, and molecular mechanism of hearing loss to shed light on the effects of autophagy in specific cells of the auditory system. Notably, an increasing number of studies have been published on aural impairment; nonetheless, studies on the autophagic role have been rare to date. Mitochondrion is vital for cellular function and mitophagy has gained increasing importance in response to stress. Studies on mitophagy in SNHL are promising, note also that there is no literature reported regarding other forms of autophagy including microautophagy and chaperone-mediated autophagy, which need more exploration in auditory system. Research on hearing loss therapy is flourishing and focuses on the cell biology of the auditory system (Nishio et al., 2017; Takeda et al., 2018; Furness, 2019; Ranum et al., 2019; Hoa et al., 2020). To better understand the function of autophagy in different cell-enriched regions of the cochlea, we collated and summarized advances in autophagic knowledge from the perspective of different cells. Regardless of the disease model, research should be directed to specific cellular targets. Moreover, researchers and clinical practitioners are showing considerable interest in cellular targets of SNHL that are modulated by autophagy. Autophagy might perform diverse biological functions in different SNHL cells, even those derived from the same etiological factor. Certainly, taking a cell perspective rather than an etiological view can improve our understanding of the exact position of SNHL and development of appropriate treatments.
Unsurprisingly, autophagy-related studies mainly focus on auditory sensory cells, and greater attention needs to be directed to non-sensory cells. Of these cells, autophagy in HCs has been the most widely researched in various forms of SNHL, and HEI-OC1 cells have been concurrently used for in vitro confirmation and supplementation of experimental results (Figure 2). Unexpectedly, we found less concrete evidence linking autophagy with hearing loss in stria vascularis and supporting cells, that is, in non-sensory cells; however, hints of a connection were found. Moreover, to fully understand hearing loss caused by aberrations in the auditory pathway (Figure 2), we briefly reviewed the function of autophagy in the central auditory system. Recognizing interrelations among unexplored systems may provide a comprehensive view of autophagy and hearing loss, and possibly other neurodegenerative diseases.
The literature has confirmed the importance of intact autophagy in the development of cells in the inner ear (Fujimoto et al., 2017; Magariños et al., 2017). In addition, autophagy may not have been given attention in studies on hearing in healthy adult mice, but under abnormal conditions caused by aging or exposure to noise or ototoxic drugs, appropriate autophagy has been shown to be particularly important in hearing conservation (Fujimoto et al., 2017; Magariños et al., 2017; Fu and Chai, 2019). For each cell type studied, including HCs, SGNs, non-sensory cells and central auditory cells, activated autophagy signaling through common or similar regulatory pathways has been shown to be beneficial under most conditions. The antioxidant effect seems to be a major role played by autophagy to protect against hearing impairment, as indicated by ROS clearance and cell survival observed in autophagy-activated cells. In addition, ameliorating organelle degradation, relieving the inflammatory response and attenuating aberrant molecular aggregation contribute to the cytoprotective effect conferred by autophagy. Given the favorable role of autophagy in most cases, many researchers agree that autophagy promotes the survival of auditory cells under stress. However, autophagy-related apoptosis remains disputed territory. Pou4f3 mutation and NLRX1-mediated autophagy or ferritinophagy can accelerate the damage to HCs treated with ototoxic drugs. Overactivation of autophagy may be associated with apoptotic effects; however, the complex relationship between autophagy and apoptosis remains unclear (Gordy and He, 2012; Doherty and Baehrecke, 2018; Yan et al., 2019). Therefore, several topics worth exploring for subsequent studies include (1) How does autophagy affect the developing auditory system? (2) Is the role of autophagy related to prosurvival or proapoptosis in pathological auditory cells? (3) Is autophagy consistent in different cells and regions of the auditory system? (4) How can we detect and modulate the dual roles of autophagy?
Overall, research on the role played by autophagy in hearing loss is in an emergent phase (He et al., 2018; Ye et al., 2018; Fu and Chai, 2019; Guo et al., 2021; Liu C. et al., 2021). Certainly, more investigation into various auditory cells, including non-sensory cells, is needed to determine the autophagic function in specific cells involved hearing loss. In summary, our review suggests cellular autophagy as a promising target and strategy for future research and clinical therapeutics in hearing loss.
HW collected the data, wrote original draft, and performed data analysis. YZ performed data analysis, reviewed and edited, resourced, and supervised. QH conceived and designed, reviewed and edited, resourced, and supervised. All authors read and approved the final manuscript, contributed to the study conception and design.
This work was supported by the National Natural Science Foundation of China (grant no. 81700916), the Natural Science Foundation of Hubei Province (grant no. 2017CFB242) and the National Award cultivation project (grant no. 2020GJPYC13).
The authors declare that the research was conducted in the absence of any commercial or financial relationships that could be construed as a potential conflict of interest.
All claims expressed in this article are solely those of the authors and do not necessarily represent those of their affiliated organizations, or those of the publisher, the editors and the reviewers. Any product that may be evaluated in this article, or claim that may be made by its manufacturer, is not guaranteed or endorsed by the publisher.
ABR, auditory brainstem response; AHL, Age-related hearing loss; ATG, autophagy-related genes; CLU, clusterin; DRP-1, Dynamin-related protein-1; EP, endocochlear potential; ER, endoplasmic reticulum; GSK3 β, glycogen synthase kinase 3 β; HCs, hair cells; HEI-OC1, House Ear Institute-organ of Corti 1; IHC/OHC, inner/outer hair cells; OSCs, Outer sulcus cells; PARP-1, Poly (ADP-ribose) polymerase-1; PE, phosphatidylethanolamine; PI3P, phosphatidylinositol 3-phosphate; PINK1, PTEN-induced putative kinase 1; PRDX1, Peroxiredoxin 1; PTSs, permanent threshold shifts; ROS, reactive oxygen species; SGNs, spiral ganglion neurons; SIRT1, Sirtuin 1; SNHL, Sensorineural hearing loss; sPTS, severe permanent threshold shift; TTSs, temporary threshold shifts; Uchl1, Ubiquitin carboxyl-terminal hydrolase isozyme L1.
Aburto, M. R., Sánchez-Calderón, H., Hurlé, J. M., Varela-Nieto, I., and Magariños, M. (2012). Early otic development depends on autophagy for apoptotic cell clearance and neural differentiation. Cell Death Dis. 3:e394. doi: 10.1038/cddis.2012.132
Allen, E. A., and Baehrecke, E. H. (2020). Autophagy in animal development. Cell Death Differ. 27, 903–918. doi: 10.1038/s41418-020-0497-0
Biasiotto, G., Di Lorenzo, D., Archetti, S., and Zanella, I. (2016). Iron and neurodegeneration: Is ferritinophagy the link? Mol. Neurobiol. 53, 5542–5574. doi: 10.1007/s12035-015-9473-y
Breitzig, M. T., Alleyn, M. D., Lockey, R. F., and Kolliputi, N. (2018). A mitochondrial delicacy: Dynamin-related protein 1 and mitochondrial dynamics. Am. J. Physiol. Cell Ph. 315, C80–C90. doi: 10.1152/ajpcell.00042.2018
Campos, J. L., and Launer, S. (2020). From healthy hearing to healthy living: A holistic approach. Ear Hear. 41:931. doi: 10.1097/AUD.0000000000000931
Chadha, S., Kamenov, K., and Cieza, A. (2021). The world report on hearing, 2021. Bull. World Health Organ. 99:242. doi: 10.2471/BLT.21.285643
Cho, S. I., Jo, E., and Song, H. (2021). Mitophagy impairment aggravates Cisplatin-Induced ototoxicity. Biomed. Res. Int. 2021:5590973. doi: 10.1155/2021/5590973
Cho, S. I., Jo, E., and Song, H. (2022). Urolithin A attenuates auditory cell senescence by activating mitophagy. Sci. Rep. UK 12, 1–10. doi: 10.1038/s41598-022-11894-2
Coate, T. M., Scott, M. K., and Gurjar, M. (2019). Current concepts in cochlear ribbon synapse formation. Synapse 73:e22087. doi: 10.1002/syn.22087
Cunningham, L. L., and Tucci, D. L. (2017). Hearing loss in adults. N. Engl. J. Med. 377, 2465–2473. doi: 10.1056/NEJMra1616601
de Iriarte Rodríguez, R., Pulido, S., Rodríguez-de La Rosa, L., Magariños, M., and Varela-Nieto, I. (2015). Age-regulated function of autophagy in the mouse inner ear. Hear. Res. 330, 39–50. doi: 10.1016/j.heares.2015.07.020
Defourny, J., Aghaie, A., Perfettini, I., Avan, P., Delmaghani, S., and Petit, C. (2019). Pejvakin-mediated pexophagy protects auditory hair cells against noise-induced damage. Proc. Natl. Acad. Sci. U.S.A. 116, 8010–8017. doi: 10.1073/pnas.1821844116
Ding, D., Zhang, J., Jiang, H., Xuan, W., Qi, W., and Salvi, R. (2020). Some ototoxic drugs destroy cochlear support cells before damaging sensory hair cells. Neurotox. Res. 37, 743–752. doi: 10.1007/s12640-020-00170-8
Djajadikerta, A., Keshri, S., Pavel, M., Prestil, R., Ryan, L., and Rubinsztein, D. C. (2020). Autophagy induction as a therapeutic strategy for neurodegenerative diseases. J. Mol. Biol. 432, 2799–2821. doi: 10.1016/j.jmb.2019.12.035
Doherty, J., and Baehrecke, E. H. (2018). Life, death and autophagy. Nat. Cell Biol. 20, 1110–1117. doi: 10.1038/s41556-018-0201-5
Fan, G., Yin, Z., Sun, Y., Chen, S., Zhang, W., Huang, X., et al. (2013). Reversible neurotoxicity of kanamycin on dorsal cochlear nucleus. Brain Res. 1502, 30–46. doi: 10.1016/j.brainres.2012.12.049
Fang, B., and Xiao, H. (2014). Rapamycin alleviates cisplatin-induced ototoxicity in vivo. Biochem. Biophys. Res. Commun. 448, 443–447. doi: 10.1016/j.bbrc.2014.04.123
Fettiplace, R. (2017). Hair cell transduction, tuning, and synaptic transmission in the mammalian cochlea. Compr. Physiol. 7, 1197–1227. doi: 10.1002/cphy.c160049
Fu, N., Du, H., Li, D., Lu, Y., Li, W., Wang, Y., et al. (2020). Clusterin contributes to hepatitis C virus-related hepatocellular carcinoma by regulating autophagy. Life Sci. 256:117911. doi: 10.1016/j.lfs.2020.117911
Fu, X., and Chai, R. (2019). Regulation of autophagy: A promising therapeutic target for the treatment of hearing loss. J. Bio-X Res. 2, 57–61. doi: 10.1097/JBR.0000000000000031
Fujimoto, C., Iwasaki, S., Urata, S., Morishita, H., Sakamaki, Y., Fujioka, M., et al. (2017). Autophagy is essential for hearing in mice. Cell Death Dis. 8:e2780. doi: 10.1038/cddis.2017.194
Furness, D. N. (2019). Forgotten fibrocytes: A neglected, supporting cell type of the cochlea with the potential to be an alternative therapeutic target in hearing loss. Front. Cell Neurosci. 13:532. doi: 10.3389/fncel.2019.00532
Ge, S., McConnell, E. S., Wu, B., Pan, W., Dong, X., and Plassman, B. L. (2021). Longitudinal association between hearing loss, vision loss, dual sensory loss, and cognitive decline. J. Am. Geriatr. Soc. 69, 644–650. doi: 10.1111/jgs.16933
Germain, K., and Kim, P. K. (2020). Pexophagy: A model for selective autophagy. Int. J. Mol. Sci. 21:578. doi: 10.3390/ijms21020578
Gilels, F., Paquette, S. T., Zhang, J., Rahman, I., and White, P. M. (2013). Mutation of Foxo3 causes adult onset auditory neuropathy and alters cochlear synapse architecture in mice. J. Neurosci. 33, 18409–18424. doi: 10.1523/JNEUROSCI.2529-13.2013
Glick, D., Barth, S., and Macleod, K. F. (2010). Autophagy: Cellular and molecular mechanisms. J. Pathol. 221, 3–12. doi: 10.1002/path.2697
Gordy, C., and He, Y. (2012). The crosstalk between autophagy and apoptosis: Where does this lead? Protein Cell 3, 17–27. doi: 10.1007/s13238-011-1127-x
Gross, A. S., and Graef, M. (2020). Mechanisms of autophagy in metabolic stress response. J. Mol. Biol. 432, 28–52. doi: 10.1016/j.jmb.2019.09.005
Guinan, J. J. J. (2018). Olivocochlear efferents: Their action, effects, measurement and uses, and the impact of the new conception of cochlear mechanical responses. Hear. Res. 362, 38–47. doi: 10.1016/j.heares.2017.12.012
Guo, L., Cao, W., Niu, Y., He, S., Chai, R., and Yang, J. (2021). Autophagy regulates the survival of hair cells and spiral ganglion neurons in cases of noise, ototoxic drug, and Age-Induced sensorineural hearing loss. Front. Cell Neurosci. 15:760422. doi: 10.3389/fncel.2021.760422
Guo, S., Xu, N., Chen, P., Liu, Y., Qi, X., Liu, S., et al. (2019). Rapamycin protects spiral ganglion neurons from gentamicin-induced degeneration in vitro. J. Assoc. Res. Otolaryngol. 20, 475–487. doi: 10.1007/s10162-019-00717-3
Hayashi, K., Dan, K., Goto, F., Tshuchihashi, N., Nomura, Y., Fujioka, M., et al. (2015). The autophagy pathway maintained signaling crosstalk with the Keap1-Nrf2 system through p62 in auditory cells under oxidative stress. Cell Signal. 27, 382–393. doi: 10.1016/j.cellsig.2014.11.024
Hayashi, K., Suzuki, Y., Fujimoto, C., and Kanzaki, S. (2020). Molecular mechanisms and biological functions of autophagy for genetics of hearing impairment. Genes 11:1331. doi: 10.3390/genes11111331
He, Z., Fang, Q., Li, H., Shao, B., Zhang, Y., Zhang, Y., et al. (2019). The role of FOXG1 in the postnatal development and survival of mouse cochlear hair cells. Neuropharmacology 144, 43–57. doi: 10.1016/j.neuropharm.2018.10.021
He, Z., Fang, Q., Waqas, M., Wu, X., Cheng, C., He, L., et al. (2018). Role of autophagy in auditory system development and survival. J. Otorhinolaryngol. Hear. Bal. Med. 1:7. doi: 10.3390/ohbm1010007
He, Z., Guo, L., Shu, Y., Fang, Q., Zhou, H., Liu, Y., et al. (2017). Autophagy protects auditory hair cells against neomycin-induced damage. Autophagy 13, 1884–1904. doi: 10.1080/15548627.2017.1359449
He, Z., Li, M., Fang, Q., Liao, F., Zou, S., Wu, X., et al. (2021). FOXG1 promotes aging inner ear hair cell survival through activation of the autophagy pathway. Autophagy 17, 4341–4362. doi: 10.1080/15548627.2021.1916194
He, Z. H., Zou, S. Y., Li, M., Liao, F. L., Wu, X., Sun, H. Y., et al. (2020). The nuclear transcription factor FoxG1 affects the sensitivity of mimetic aging hair cells to inflammation by regulating autophagy pathways. Redox Biol. 28:101364. doi: 10.1016/j.redox.2019.101364
Herman, L., Todeschini, A., and Veitia, R. A. (2021). Forkhead transcription factors in health and disease. Trends Genet. 37, 460–475. doi: 10.1016/j.tig.2020.11.003
Hoa, M., Olszewski, R., Li, X., Taukulis, I., Gu, S., DeTorres, A., et al. (2020). Characterizing adult cochlear supporting cell transcriptional diversity using Single-Cell RNA-Seq: Validation in the adult mouse and translational implications for the adult human cochlea. Front. Mol. Neurosci. 13:13. doi: 10.3389/fnmol.2020.00013
Hou, S., Chen, P., Chen, J., Chen, J., Sun, L., Chen, J., et al. (2020). Distinct expression patterns of apoptosis and Autophagy-Associated proteins and genes during postnatal development of spiral ganglion neurons in rat. Neural Plast. 2020:9387560. doi: 10.1155/2020/9387560
Huth, M. E., Ricci, A. J., and Cheng, A. G. (2011). Mechanisms of aminoglycoside ototoxicity and targets of hair cell protection. Int. J. Otolaryngol. 2011:937861. doi: 10.1155/2011/937861
Imai, S., and Guarente, L. (2014). NAD+ and sirtuins in aging and disease. Trends Cell Biol. 24, 464–471. doi: 10.1016/j.tcb.2014.04.002
Iyer, J. S., Batts, S. A., Chu, K. K., Sahin, M. I., Leung, H. M., Tearney, G. J., et al. (2016). Micro-optical coherence tomography of the mammalian cochlea. Sci. Rep. UK 6:33288. doi: 10.1038/srep33288
Jian, B., Pang, J., Xiong, H., Zhang, W., Zhan, T., Su, Z., et al. (2021). Autophagy-dependent ferroptosis contributes to cisplatin-induced hearing loss. Toxicol. Lett. 350, 249–260. doi: 10.1016/j.toxlet.2021.07.010
Jiang, H., Yang, Y., Zhang, Y., Xie, Z., Zhao, X., Sun, Y., et al. (2018). The dual role of poly(ADP-ribose) polymerase-1 in modulating parthanatos and autophagy under oxidative stress in rat cochlear marginal cells of the stria vascularis. Redox Biol. 14, 361–370. doi: 10.1016/j.redox.2017.10.002
Kalinec, G., Thein, P., Park, C., and Kalinec, F. (2016). HEI-OC1 cells as a model for investigating drug cytotoxicity. Hear. Res. 335, 105–117. doi: 10.1016/j.heares.2016.02.019
Kalinec, G. M., Webster, P., Lim, D. J., and Kalinec, F. (2003). A cochlear cell line as an in vitro system for drug ototoxicity screening. Audiol. Neurootol. 8, 177–189. doi: 10.1159/000071059
Kim, Y. J., Choo, O., Lee, J., Jang, J. H., Woo, H. G., and Choung, Y. (2021). BCL2 interacting protein 3-like/NIX-mediated mitophagy plays an important role in the process of age-related hearing loss. Neuroscience 455, 39–51. doi: 10.1016/j.neuroscience.2020.12.005
Kim, Y. J., Kim, K., Lee, Y. Y., Choo, O. S., Jang, J. H., and Choung, Y. H. (2019). Downregulated UCHL1 accelerates gentamicin-induced auditory cell death via autophagy. Mol. Neurobiol. 56, 7433–7447. doi: 10.1007/s12035-019-1598-y
Kim, Y. J., Tian, C., Kim, J., Shin, B., Choo, O. S., Kim, Y. S., et al. (2017). Autophagic flux, a possible mechanism for delayed gentamicin-induced ototoxicity. Sci. Rep. 7:41356. doi: 10.1038/srep41356
Kishino, A., Hayashi, K., Hidai, C., Masuda, T., Nomura, Y., and Oshima, T. (2017). XBP1-FoxO1 interaction regulates ER stress-induced autophagy in auditory cells. Sci. Rep. UK 7:4442. doi: 10.1038/s41598-017-02960-1
Koh, Y. I., Oh, K. S., Kim, J. A., Noh, B., Choi, H. J., Joo, S. Y., et al. (2022). OSBPL2 mutations impair autophagy and lead to hearing loss, potentially remedied by rapamycin. Autophagy 2022:2040891. doi: 10.1080/15548627.2022.2040891
Lam, E. W. F., Brosens, J. J., Gomes, A. R., and Koo, C. (2013). Forkhead box proteins: Tuning forks for transcriptional harmony. Nat. Rev. Cancer 13, 482–495. doi: 10.1038/nrc3539
Latunde-Dada, G. O. (2017). Ferroptosis: Role of lipid peroxidation, iron and ferritinophagy. Biochim. Biophys. Acta 1861, 1893–1900. doi: 10.1016/j.bbagen.2017.05.019
Le, Q., Tabuchi, K., Warabi, E., and Hara, A. (2017). The role of peroxiredoxin I in cisplatin-induced ototoxicity. Auris Nasus Larynx 44, 205–212. doi: 10.1016/j.anl.2016.06.001
Lee, S., Shin, J., Sagong, B., Kim, U., and Bok, J. (2017). Spatiotemporal expression patterns of clusterin in the mouse inner ear. Cell Tissue Res. 370, 89–97. doi: 10.1007/s00441-017-2650-8
Levine, B., and Kroemer, G. (2019). Biological functions of autophagy genes: A disease perspective. Cell 176, 11–42. doi: 10.1016/j.cell.2018.09.048
Levy, J. M. M., Towers, C. G., and Thorburn, A. (2017). Targeting autophagy in cancer. Nat. Rev. Cancer 17, 528–542. doi: 10.1038/nrc.2017.53
Li, H., Song, Y., He, Z., Chen, X., Wu, X., Li, X., et al. (2018). Meclofenamic acid reduces reactive oxygen species accumulation and apoptosis, inhibits excessive autophagy, and protects hair cell-like HEI-OC1 cells from cisplatin-induced damage. Front. Cell Neurosci. 12:139. doi: 10.3389/fncel.2018.00139
Li, Z., Yao, Q., Tian, Y., Jiang, Y., Xu, M., Wang, H., et al. (2022). Trehalose protects against cisplatin-induced cochlear hair cell damage by activating TFEB-mediated autophagy. Biochem. Pharmacol. 197:114904. doi: 10.1016/j.bcp.2021.114904
Liang, Z., Zhang, T., Zhan, T., Cheng, G., Zhang, W., Jia, H., et al. (2021). Metformin alleviates cisplatin-induced ototoxicity by autophagy induction possibly via the AMPK/FOXO3a pathway. J. Neurophysiol. 125, 1202–1212. doi: 10.1152/jn.00417.2020
Lin, F. R., Yaffe, K., Xia, J., Xue, Q. L., Harris, T. B., Purchase-Helzner, E., et al. (2013). Hearing loss and cognitive decline in older adults. JAMA Intern. Med. 173, 293–299. doi: 10.1001/jamainternmed.2013.1868
Lin, H., Xiong, H., Su, Z., Pang, J., Lai, L., Zhang, H., et al. (2019). Inhibition of DRP-1-Dependent mitophagy promotes cochlea hair cell senescence and exacerbates Age-Related hearing loss. Front. Cell Neurosci. 13:550. doi: 10.3389/fncel.2019.00550
Liu, C., Zheng, Z., Wang, P., He, S., He, Y., and Wang, J. (2021). Autophagy: A novel horizon for hair cell protection. Neural Plast. 2021:5511010. doi: 10.1155/2021/5511010
Liu, H., Li, F., Li, X., Wu, Q., and Dai, C. (2022). Rapamycin ameliorates age-related hearing loss in C57BL/6J mice by enhancing autophagy in the SGNs. Neurosci. Lett. 772:136493. doi: 10.1016/j.neulet.2022.136493
Liu, H., Li, Y., Chen, L., Zhang, Q., Pan, N., Nichols, D. H., et al. (2016). Organ of corti and stria vascularis: Is there an interdependence for survival? PLoS One 11:e168953. doi: 10.1371/journal.pone.0168953
Liu, T., Zong, S., Luo, P., Qu, Y., Wen, Y., Du, P., et al. (2019). Enhancing autophagy by down-regulating GSK-3β alleviates cisplatin-induced ototoxicity in vivo and in vitro. Toxicol. Lett. 313, 11–18. doi: 10.1016/j.toxlet.2019.05.025
Liu, W., Wang, X., Wang, M., and Wang, H. (2019). Protection of spiral ganglion neurons and prevention of auditory neuropathy. Adv. Exp. Med. Biol. 1130, 93–107. doi: 10.1007/978-981-13-6123-4_6
Liu, W., Xu, L., Wang, X., Zhang, D., Sun, G., Wang, M., et al. (2021). PRDX1 activates autophagy via the PTEN-AKT signaling pathway to protect against cisplatin-induced spiral ganglion neuron damage. Autophagy 17, 4159–4181. doi: 10.1080/15548627.2021.1905466
Magariños, M., Contreras, J., and Varela-Nieto, I. (2014). “Chapter 1 - early development of the vertebrate inner ear,” in Development of Auditory and Vestibular Systems, eds R. Romand and I. Varela-Nieto (San Diego: Academic Press), 1–30. doi: 10.1016/B978-0-12-408088-1.00001-4
Magariños, M., Pulido, S., Aburto, M. R., de Iriarte, R. R., and Varela-Nieto, I. (2017). Autophagy in the vertebrate inner ear. Front. Cell Dev. Biol. 5:56. doi: 10.3389/fcell.2017.00056
Maiuri, M. C., Zalckvar, E., Kimchi, A., and Kroemer, G. (2007). Self-eating and self-killing: Crosstalk between autophagy and apoptosis. Nat. Rev. Mol. Cell Biol. 8, 741–752. doi: 10.1038/nrm2239
McGovern, M. M., Randle, M. R., Cuppini, C. L., Graves, K. A., and Cox, B. C. (2019). Multiple supporting cell subtypes are capable of spontaneous hair cell regeneration in the neonatal mouse cochlea. Development 146:v171009. doi: 10.1242/dev.171009
Menardo, J., Tang, Y., Ladrech, S., Lenoir, M., Casas, F., Michel, C., et al. (2012). Oxidative stress, inflammation, and autophagic stress as the key mechanisms of premature age-related hearing loss in SAMP8 mouse Cochlea. Antioxid. Redox Signal. 16, 263–274. doi: 10.1089/ars.2011.4037
Mizushima, N. (2018). A brief history of autophagy from cell biology to physiology and disease. Nat. Cell Biol. 20, 521–527. doi: 10.1038/s41556-018-0092-5
Mizushima, N. (2020). The ATG conjugation systems in autophagy. Curr. Opin. Cell Biol. 63, 1–10. doi: 10.1016/j.ceb.2019.12.001
Monzack, E. L., and Cunningham, L. L. (2013). Lead roles for supporting actors: Critical functions of inner ear supporting cells. Hear. Res. 303, 20–29. doi: 10.1016/j.heares.2013.01.008
Morishita, H., and Mizushima, N. (2019). Diverse cellular roles of autophagy. Annu. Rev. Cell Dev. Biol. 35, 453–475. doi: 10.1146/annurev-cellbio-100818-125300
Nayagam, B. A., Muniak, M. A., and Ryugo, D. K. (2011). The spiral ganglion: Connecting the peripheral and central auditory systems. Hear. Res. 278, 2–20. doi: 10.1016/j.heares.2011.04.003
Nishio, S., Takumi, Y., and Usami, S. (2017). Laser-capture micro dissection combined with next-generation sequencing analysis of cell type-specific deafness gene expression in the mouse cochlea. Hear. Res. 348, 87–97. doi: 10.1016/j.heares.2017.02.017
Oh, J., Youn, C. K., Jun, Y., Jo, E., and Cho, S. I. (2020). Reduced mitophagy in the cochlea of aged C57BL/6J mice. Exp. Gerontol. 137:110946. doi: 10.1016/j.exger.2020.110946
Pang, J., Xiong, H., Lin, P., Lai, L., Yang, H., Liu, Y., et al. (2017). Activation of miR-34a impairs autophagic flux and promotes cochlear cell death via repressing ATG9A: Implications for age-related hearing loss. Cell Death Dis. 8:e3079. doi: 10.1038/cddis.2017.462
Pang, J., Xiong, H., Ou, Y., Yang, H., Xu, Y., Chen, S., et al. (2019). SIRT1 protects cochlear hair cell and delays age-related hearing loss via autophagy. Neurobiol. Aging 80, 127–137. doi: 10.1016/j.neurobiolaging.2019.04.003
Pang, J., Xiong, H., Zhan, T., Cheng, G., Jia, H., Ye, Y., et al. (2018). Sirtuin 1 and autophagy attenuate Cisplatin-Induced hair cell death in the mouse cochlea and zebrafish lateral line. Front. Cell Neurosci. 12:515. doi: 10.3389/fncel.2018.00515
Petitpré, C., Wu, H., Sharma, A., Tokarska, A., Fontanet, P., Wang, Y., et al. (2018). Neuronal heterogeneity and stereotyped connectivity in the auditory afferent system. Nat. Commun. 9:3691. doi: 10.1038/s41467-018-06033-3
Pohl, C., and Dikic, I. (2019). Cellular quality control by the ubiquitin-proteasome system and autophagy. Science 366:818.
Ranum, P. T., Goodwin, A. T., Yoshimura, H., Kolbe, D. L., Walls, W. D., Koh, J., et al. (2019). Insights into the biology of hearing and deafness revealed by single-cell RNA sequencing. Cell Rep. 26, 3160–3171. doi: 10.1016/j.celrep.2019.02.053
Raphael, Y., and Altschuler, R. A. (2003). Structure and innervation of the cochlea. Brain Res. Bull. 60, 397–422. doi: 10.1016/S0361-9230(03)00047-9
Reddy, P. H., Reddy, T. P., Manczak, M., Calkins, M. J., Shirendeb, U., and Mao, P. (2011). Dynamin-related protein 1 and mitochondrial fragmentation in neurodegenerative diseases. Brain Res. Rev. 67, 103–118. doi: 10.1016/j.brainresrev.2010.11.004
Roccio, M., Senn, P., and Heller, S. (2020). Novel insights into inner ear development and regeneration for targeted hearing loss therapies. Hear. Res. 397:107859. doi: 10.1016/j.heares.2019.107859
Saegusa, C., Hosoya, M., Nishiyama, T., Saeki, T., Fujimoto, C., Okano, H., et al. (2020). Low-dose rapamycin-induced autophagy in cochlear outer sulcus cells. Laryngosc. Invest. Otolaryngol. 5, 520–528. doi: 10.1002/lio2.392
Setz, C., Benischke, A., Pinho Ferreira Bento, A. C., Brand, Y., Levano, S., Paech, F., et al. (2018). Induction of mitophagy in the HEI-OC1 auditory cell line and activation of the Atg12/LC3 pathway in the organ of Corti. Hear. Res. 361, 52–65. doi: 10.1016/j.heares.2018.01.003
Shan, A., Ting, J. S., Price, C., Goman, A. M., Willink, A., Reed, N. S., et al. (2020). Hearing loss and employment: A systematic review of the association between hearing loss and employment among adults. J. Laryngol. Otol. 134, 387–397. doi: 10.1017/S0022215120001012
Shi, X. (2016). Pathophysiology of the cochlear intrastrial fluid-blood barrier (review). Hear. Res. 338, 52–63. doi: 10.1016/j.heares.2016.01.010
Shukla, A., Harper, M., Pedersen, E., Goman, A., Suen, J. J., Price, C., et al. (2020). Hearing loss, loneliness, and social isolation: A systematic review. Otolaryngol. Head. Neck. Surg. 162, 622–633. doi: 10.1177/0194599820910377
Stephenson, L. (2012). Journal of visual communication in medicine. J. Vis. Commun. Med. 35:159. doi: 10.3109/08039488.2012.747176
Takeda, H., Dondzillo, A., Randall, J. A., and Gubbels, S. P. (2018). Challenges in Cell-Based therapies for the treatment of hearing loss. Trends Neurosci. 41, 823–837. doi: 10.1016/j.tins.2018.06.008
Tang, M., Chen, Z., Wu, D., and Chen, L. (2018). Ferritinophagy/ferroptosis: Iron-related newcomers in human diseases. J. Cell Physiol. 233, 9179–9190. doi: 10.1002/jcp.26954
Tawfik, K. O., Klepper, K., Saliba, J., and Friedman, R. A. (2019). Advances in understanding of presbycusis. J. Neurosci. Res. 98, 1685–1697. doi: 10.1002/jnr.24426
Tia, N., Singh, A. K., Pandey, P., Azad, C. S., Chaudhary, P., and Gambhir, I. S. (2018). Role of Forkhead Box O (FOXO) transcription factor in aging and diseases. Gene 648, 97–105. doi: 10.1016/j.gene.2018.01.051
Tsuchihashi, N. A., Hayashi, K., Dan, K., Goto, F., Nomura, Y., Fujioka, M., et al. (2015). Autophagy through 4EBP1 and AMPK regulates oxidative stress-induced premature senescence in auditory cells. Oncotarget 6, 3644–3655. doi: 10.18632/oncotarget.2874
van der Valk, W. H., Steinhart, M. R., Zhang, J., and Koehler, K. R. (2021). Building inner ears: Recent advances and future challenges for in vitro organoid systems. Cell Death Differ. 28, 24–34. doi: 10.1038/s41418-020-00678-8
Varela-Nieto, I., Palmero, I., and Magariños, M. (2019). Complementary and distinct roles of autophagy, apoptosis and senescence during early inner ear development. Hear. Res. 376, 86–96. doi: 10.1016/j.heares.2019.01.014
Wilson, B. S., Tucci, D. L., Merson, M. H., and O’Donoghue, G. M. (2017). Global hearing health care: New findings and perspectives. Lancet 390, 2503–2515. doi: 10.1016/S0140-6736(17)31073-5
Wright, G. D., and Horn, H. F. (2016). Three-dimensional image analysis of the mouse cochlea. Differentiation 91, 104–108. doi: 10.1016/j.diff.2016.01.002
Wu, J., Ye, J., Kong, W., Zhang, S., and Zheng, Y. (2020). Programmed cell death pathways in hearing loss: A review of apoptosis, autophagy and programmed necrosis. Cell Proliferat. 53:e12915. doi: 10.1111/cpr.12915
Xiong, H., Chen, S., Lai, L., Yang, H., Xu, Y., Pang, J., et al. (2019). Modulation of miR-34a/SIRT1 signaling protects cochlear hair cells against oxidative stress and delays age-related hearing loss through coordinated regulation of mitophagy and mitochondrial biogenesis. Neurobiol. Aging 79, 30–42. doi: 10.1016/j.neurobiolaging.2019.03.013
Xiong, H., Dai, M., Ou, Y., Pang, J., Yang, H., Huang, Q., et al. (2014). SIRT1 expression in the cochlea and auditory cortex of a mouse model of age-related hearing loss. Exp. Gerontol. 51, 8–14. doi: 10.1016/j.exger.2013.12.006
Xiong, H., Pang, J., Min, X., Ye, Y., Lai, L., and Zheng, Y. (2022). MiR-34a/ATG9A/TFEB signaling modulates autophagy in cochlear hair cells and correlates with age-related hearing loss. Neuroscience 491, 98–109. doi: 10.1016/j.neuroscience.2022.03.033
Xiong, H., Pang, J., Yang, H., Dai, M., Liu, Y., Ou, Y., et al. (2015). Activation of miR-34a/SIRT1/p53 signaling contributes to cochlear hair cell apoptosis: Implications for age-related hearing loss. Neurobiol. Aging 36, 1692–1701. doi: 10.1016/j.neurobiolaging.2014.12.034
Xiong, W., Wei, W., Qi, Y., Du, Z., Qu, T., Liu, K., et al. (2020). Autophagy is required for remodeling in postnatal developing ribbon synapses of cochlear inner hair cells. Neuroscience 431, 1–16. doi: 10.1016/j.neuroscience.2020.01.032
Xu, F., Cheng, Y., and Yan, W. (2018). Up-regulation of autophagy and apoptosis of cochlear hair cells in mouse models for deafness. Arch. Med. Sci. 17, 535–541. doi: 10.5114/aoms.2018.75348
Xu, F., Yan, W., and Cheng, Y. (2020). Pou4f3 gene mutation promotes autophagy and apoptosis of cochlear hair cells in cisplatin-induced deafness mice. Arch. Biochem. Biophys. 680:108224. doi: 10.1016/j.abb.2019.108224
Xueqin, H., Wei, Z., Yixin, Y., Xiaolong, L., Chen, Y., Qi, L., et al. (2017). Implication of autophagy and apoptosis in spiral ganglion cells and cochlear nucleus nuerons in diabetes-induced hearing impairment in rats. Biomed. Res. Tokyo 28, 2164–2169.
Yamakuchi, M., Ferlito, M., and Lowenstein, C. J. (2008). MiR-34a repression of SIRT1 regulates apoptosis. Proc. Natl. Acad. Sci. 105:13421. doi: 10.1073/pnas.0801613105
Yan, X., Zhou, R., and Ma, Z. (2019). Autophagy-Cell survival and death. Adv. Exp. Med. Biol. 1206, 667–696. doi: 10.1007/978-981-15-0602-4_29
Yang, J., Chen, D., He, Y., Meléndez, A., Feng, Z., Hong, Q., et al. (2013). MiR-34 modulates Caenorhabditis elegans lifespan via repressing the autophagy gene atg9. Age 35, 11–22. doi: 10.1007/s11357-011-9324-3
Yang, Q., Sun, G., Yin, H., Li, H., Cao, Z., Wang, J., et al. (2018a). PINK1 protects auditory hair cells and spiral ganglion neurons from cisplatin-induced ototoxicity via inducing autophagy and inhibiting JNK signaling pathway. Free Radical. Biol. Med. 120, 342–355. doi: 10.1016/j.freeradbiomed.2018.02.025
Yang, Q., Zhou, Y., Yin, H., Li, H., Zhou, M., Sun, G., et al. (2018b). PINK1 protects against gentamicin-induced sensory hair cell damage: Possible relation to induction of autophagy and inhibition of p53 signal pathway. Front. Mol. Neurosci. 11:403. doi: 10.3389/fnmol.2018.00403
Yang, Y., and Klionsky, D. J. (2020). Autophagy and disease: Unanswered questions. Cell Death Differ. 27, 858–871. doi: 10.1038/s41418-019-0480-9
Ye, B., Fan, C., Shen, Y., Wang, Q., Hu, H., and Xiang, M. (2018). The antioxidative role of autophagy in hearing loss. Front. Neurosci. 12:1010. doi: 10.3389/fnins.2018.01010
Ye, B., Wang, Q., Hu, H., Shen, Y., Fan, C., Chen, P., et al. (2019). Restoring autophagic flux attenuates cochlear spiral ganglion neuron degeneration by promoting TFEB nuclear translocation via inhibiting MTOR. Autophagy 15, 998–1016. doi: 10.1080/15548627.2019.1569926
Yigider, A. P., Yilmaz, S., Ulusoy, H., Kara, T., Kufeciler, L., and Kaya, K. H. (2020). Emotional and behavioral problems in children and adolescents with hearing loss and their effects on quality of life. Int. J. Pediatr. Otorhinolaryngol. 137:110245. doi: 10.1016/j.ijporl.2020.110245
Yin, H., Yang, Q., Cao, Z., Li, H., Yu, Z., Zhang, G., et al. (2018). Activation of NLRX1-mediated autophagy accelerates the ototoxic potential of cisplatin in auditory cells. Toxicol. Appl. Pharm. 343, 16–28. doi: 10.1016/j.taap.2018.02.007
Youn, C. K., Jun, Y., Jo, E., and Cho, S. I. (2020). Age-Related hearing loss in C57BL/6J mice is associated with mitophagy impairment in the central auditory system. Int. J. Mol. Sci. 21:7202. doi: 10.3390/ijms21197202
Youn, C. K., Kim, J., Park, J., Do, N. Y., and Cho, S. I. (2015). Role of autophagy in cisplatin-induced ototoxicity. Int. J. Pediatr. Otorhinolaryngol. 79, 1814–1819. doi: 10.1016/j.ijporl.2015.08.012
Yu, D., Gu, J., Chen, Y., Kang, W., Wang, X., and Wu, H. (2020). Current strategies to combat Cisplatin-Induced ototoxicity. Front. Pharmacol. 11:999. doi: 10.3389/fphar.2020.00999
Yu, X., Guan, M., Shang, H., Teng, Y., Gao, Y., Wang, B., et al. (2021). The expression of PHB2 in the cochlea: Possible relation to age-related hearing loss. Cell Biol. Int. 45, 2490–2498. doi: 10.1002/cbin.11693
Yuan, H., Wang, X., Hill, K., Chen, J., Lemasters, J., Yang, S. M., et al. (2015). Autophagy attenuates noise-induced hearing loss by reducing oxidative stress. Antioxid. Redox Signal. 22, 1308–1324. doi: 10.1089/ars.2014.6004
Yuan, J., Zhao, X., Hu, Y., Sun, H., Gong, G., Huang, X., et al. (2018). Autophagy regulates the degeneration of the auditory cortex through the AMPK-mTOR-ULK1 signaling pathway. Int. J. Mol. Med. 41, 2086–2098. doi: 10.3892/ijmm.2018.3393
Yuan, X., Liu, H., Li, Y., Li, W., Yu, H., and Shen, X. (2020). Ribbon synapses and hearing impairment in mice after in utero sevoflurane exposure. Drug Des. Devel. Ther. 14, 2685–2693. doi: 10.2147/DDDT.S253031
Yun, H. R., Jo, Y. H., Kim, J., Shin, Y., Kim, S. S., and Choi, T. G. (2020). Roles of autophagy in oxidative stress. Int. J. Mol. Sci. 21:3289. doi: 10.3390/ijms21093289
Zhang, F., Kumano, M., Beraldi, E., Fazli, L., Du, C., Moore, S., et al. (2014). Clusterin facilitates stress-induced lipidation of LC3 and autophagosome biogenesis to enhance cancer cell survival. Nat. Commun. 5:5775. doi: 10.1038/ncomms6775
Zhang, Y., Jiang, Q., Xie, S., Wu, X., Zhou, J., and Sun, H. (2019). Lead induced ototoxicity and neurotoxicity in adult guinea pig. Biomed. Res. Int. 2019:3626032. doi: 10.1155/2019/3626032
Zhao, T., Zheng, T., Yu, H., Hu, B. H., Hu, B., Ma, P., et al. (2021). Autophagy impairment as a key feature for acetaminophen-induced ototoxicity. Cell Death Dis. 12:3. doi: 10.1038/s41419-020-03328-6
Keywords: autophagy, auditory cells, cochlea, sensorineural hearing loss, auditory pathway
Citation: Wan H, Zhang Y and Hua Q (2022) Cellular autophagy, the compelling roles in hearing function and dysfunction. Front. Cell. Neurosci. 16:966202. doi: 10.3389/fncel.2022.966202
Received: 10 June 2022; Accepted: 14 September 2022;
Published: 30 September 2022.
Edited by:
Evandro Fei Fang, University of Oslo, NorwayReviewed by:
Hyeong-Geug Kim, Indiana University–Purdue University Indianapolis, United StatesCopyright © 2022 Wan, Zhang and Hua. This is an open-access article distributed under the terms of the Creative Commons Attribution License (CC BY). The use, distribution or reproduction in other forums is permitted, provided the original author(s) and the copyright owner(s) are credited and that the original publication in this journal is cited, in accordance with accepted academic practice. No use, distribution or reproduction is permitted which does not comply with these terms.
*Correspondence: Yuanyuan Zhang, enl5LWVudEB3aHUuZWR1LmNu; Qingquan Hua, aHFxMTIxNEB3aHUuZWR1LmNu
Disclaimer: All claims expressed in this article are solely those of the authors and do not necessarily represent those of their affiliated organizations, or those of the publisher, the editors and the reviewers. Any product that may be evaluated in this article or claim that may be made by its manufacturer is not guaranteed or endorsed by the publisher.
Research integrity at Frontiers
Learn more about the work of our research integrity team to safeguard the quality of each article we publish.