- 1Ningxia Key Laboratory of Cerebrocranial Diseases, College of Traditional Chinese Medicine, Ningxia Medical University, Yinchuan, China
- 2Ningxia Key Laboratory of Cerebrocranial Diseases, Ningxia Medical University, Yinchuan, China
- 3School of Clinical Medicine, Ningxia Medical University, Yinchuan, China
- 4Ningxia Key Laboratory of Cerebrocranial Diseases, Department of Neurosurgery, General Hospital of Ningxia Medical University, Ningxia Medical University, Yinchuan, China
- 5College of Traditional Chinese Medicine, Ningxia Medical University, Yinchuan, China
Seizures in rodent models that are induced by lithium-pilocarpine mimic human seizures in a highly isomorphic manner. The hippocampus is a brain region that generates and spreads seizures. In order to understand the early phases of seizure events occurring in the hippocampus, global protein expression levels in the hippocampus on day 1 and day 3 were analyzed in lithium-pilocarpine induced acute epileptic rat models using a tandem mass tag-based proteomic approach. Our results showed that differentially expressed proteins were likely to be enhanced rather than prohibited in modulating seizure activity on days 1 and 3 in lithium-pilocarpine induced seizure rats. The differentially regulated proteins differed on days 1 and 3 in the seizure rats, indicating that different molecules and pathways are involved in seizure events occurring from day 1 to day 3 following lithium-pilocarpine administration. In regard to subcellular distribution, the results suggest that post-seizure cellular function in the hippocampus is possibly regulated in a differential manner on seizure progression. Gene ontology annotation results showed that, on day 1 following lithium-pilocarpine administration, it is likely necessary to regulate macromolecular complex assembly, and cell death, while on day 3, it may be necessary to modulate protein metabolic process, cytoplasm, and protein binding. Protein metabolic process rather than macromolecular complex assembly and cell death were affected on day 3 following lithium-pilocarpine administration. The extracellular matrix, receptors, and the constitution of plasma membranes were altered most strongly in the development of seizure events. In a KEGG pathway enrichment cluster analysis, the signaling pathways identified were relevant to sustained angiogenesis and evading apoptosis, and complement and coagulation cascades. On day 3, pathways relevant to Huntington’s disease, and tumor necrosis factor signaling were most prevalent. These results suggest that seizure events occurring in day 1 modulate macromolecular complex assembly and cell death, and in day 3 modulate biological protein metabolic process. In summary, our study found limited evidence for ongoing seizure events in the hippocampus of lithium-pilocarpine induced animal models; nevertheless, evaluating the global differential expression of proteins and their impacts on bio-function may offer new perspectives for studying epileptogenesis in the future.
Introduction
Epilepsy manifests as repeated transient seizures with longer interictal periods between seizures. The primary goal of epilepsy research is to understand the mechanisms of epileptogenesis and ictogenesis. In epilepsy disorders, the brain tends to generate seizures (Fisher et al., 2005). The pilocarpine-induced animal model is commonly used as an epileptic seizure model that mimics the human disease in a highly isomorphic manner (Turski et al., 1983a,b).
Seizures induced by pilocarpine possibly exert their effects through the muscarinic receptor to cause an imbalance between excitatory and inhibitory transmission (Hamilton et al., 1997; Priel and Albuquerque, 2002). The vital characteristics of the pilocarpine model include rapid induction of acute status epilepticus (SE), the presence of a latent period and spontaneous recurrent seizures (SRSs, chronic phase) (Leite et al., 1990; Cavalheiro et al., 1991), the occurrence of widespread lesions, and seizures that are poorly controlled by antiepileptic drugs (Glien et al., 2002; Chakir et al., 2006; André et al., 2007). In a modification of the pilocarpine model, pilocarpine has also been combined with lithium to achieve a reduction dose and increased sensitivity to pilocarpine for inducing seizures; this model is similar to the pilocarpine model behaviorally, metabolically, electrographically, and neuropathologically (Honchar et al., 1983; Clifford et al., 1987).
After injecting pilocarpine, ictal, and interictal epileptic events are evoked and a clear pattern of theta rhythms is evident in the hippocampus (Turski et al., 1983a,b). Along with seizure event development, electrographic seizures are originated in the hippocampus and are propagated from the hippocampus to the amygdala and neocortex (Turski et al., 1983a,b). However, these hippocampal alterations appear to intensify progressively until 80 days after SE. In view of the important role of the hippocampus in generating and spreading seizures in epilepsy, it is important to understand the mechanisms and molecule alterations during early seizure events in animal models and patients with epilepsy. Biochemical changes reflect critical alterations in integral processes during the development of seizure events, yet they have received limited attention. The proteome studies of the human hippocampus in patients with Alzheimer’s disease (Edgar et al., 1999b; Sultana et al., 2007; Begcevic et al., 2013; Hondius et al., 2016), non-CNS malignancies (Yang et al., 2004), and refractory temporal lobe epilepsy has been reported (Persike et al., 2012, 2018). The proteome studies of epileptic animal models in the chronic phase induced by the kindling and pilocarpine models have also studied (Sadeghi et al., 2021). However, studies of biochemical changes during seizures in the acute phase of epileptic animal models have been quite limited to date.
Given the known role of the hippocampus in seizure development, we examined molecules and signaling pathways that may plausibly regulate seizures in the hippocampus using tandem mass tag (TMT)-labeled quantitative proteomic analysis in a lithium-pilocarpine induced epileptic rat model. Our results show that differentially expressed proteins are likely to be enhanced rather than prohibited in modulating seizures in a lithium-pilocarpine induced rat model. On day 1 following lithium-pilocarpine administration, macromolecular complex assembly, RNA binding, the extracellular regulation, and cell death were mainly regulated in the hippocampus. On day 3 following lithium-pilocarpine administration, protein metabolic process, cytoplasm, and protein binding were generally modulated. Moreover, on day 1 following lithium-pilocarpine administration (compared with controls), the majority of regulated signaling pathways comprised pathways relevant to cancer (regulating sustained angiogenesis and evading apoptosis), and complement and coagulation cascades. On day 3 following lithium-pilocarpine administration (compared with controls), the majority of regulated signaling pathways were as follows: Huntington’s disease, tumor necrosis factor (TNF) signaling, tight junction, and nuclear factor (NF)-kappa B pathways. Our study may offer potential indicators for seizure development in the acute phase in epilepsy. Although our study found limited evidence for ongoing seizure events in the hippocampus of lithium-pilocarpine induced animal models, evaluating the global differential expression of proteins and their impacts on biological function is critical to understanding the features of seizure events and may offer new perspectives for studying epileptogenesis in the future.
Materials and methods
Lithium-pilocarpine induced status epilepticus rat
Epileptic seizure rats (male Sprague Dawley rats; weight, approximately 220 g, n = 3 in each experimental group) were induced by intraperitoneal (IP) injection of lithium (130 mg/kg in 0.9% saline)-pilocarpine hydrochloride (30 mg/kg in 0.9% saline, Sigma), as previously described (with minor modifications) (Wang et al., 2021). In the present study, only those animals whose convulsion activity reached scale IV and scale V activity levels (Racine, 1972) were utilized; convulsions were allowed to last for 30 min. Finally, convulsion activity was terminated using chloral hydrate (400 mg/kg, Damao, Tianjin, China). The mortality of epileptic seizure rat was 10%. In experiment, three animals were divided in control group, three survival epileptic seizure rats were terminated after 1 day; three survival epileptic seizure rats were terminated after 1 day.
Animals were housed with free access to food and water at 25°C for 1 and 3 days after lithium-pilocarpine administration. At the end of the study, the both hippocampus of each rat was collected for TMT-labeled quantitative proteomic analysis (Jingjie, Hangzhou, China). All protocols and procedures were approved by the National Institutes of Health and the ethics committee of Ningxia Medial University (Ningxia, China). We followed all relevant national and international guidelines for animal care and welfare (e.g., the ARRIVE guidelines) in conducting this study. Research involving animals and all protocols and procedures were approved by the National Institutes of Health and the animal welfare committee of Ningxia Medical University (Ethics Approval Number: 2019-151, Ningxia, China).
Tandem mass tag-labeled quantitative proteomic analysis
The hippocampi obtained from epileptic rats from all experimental groups were analyzed by quantitative proteomic analysis. All collected samples were ground into cell powder using liquid nitrogen. Four volumes of lysis buffer (8 M urea, 1% Protease Inhibitor Cocktail) were added and the samples were sonicated three times on ice using a high intensity ultrasonic processor (Scientz, Ningbo, China). The supernatant was collected after centrifugation at 12,000 g at 4°C for 10 min, and protein concentrations were measured using a bicinchoninic acid assay kit according to the manufacturer’s instructions. After that, the supernatant was incubated with trypsin in order to digest the protein to a peptide product. The peptide was desalted using a Strata X C18 SPE column (Phenomenex, Torrance, CA, USA) and was vacuum-dried. The peptide was reconstituted in 0.5 M triethylammonium bicarbonate (TEAB) and labeled with a TMT kit according to the manufacturer’s protocol.
Database search
The MaxQuant search engine (v.1.5.2.81) was used to analyze the resulting tandem mass spectrometry (MS/MS) data, and the Human UniProt Database2 was concatenated with a reverse decoy database search for the tandem mass spectra. Trypsin/P was used as the cleavage enzyme, allowing for up to four missing cleavages. The set of mass tolerance for precursor ions in the first search was 20 ppm; this value was set to 5 ppm in the main search (0.02 da, mass tolerance for fragment ions). Carbamidomethyl on Cys was specified as a fixed modification, whereas acetylation and oxidation on Met were specified as variable modifications. The false discovery rate (FDR) was adjusted to <1% and the minimum score for modified peptides was set at >40.
Gene ontology annotation
The UniProt-GOA database3 was used to perform Gene Ontology (GO) annotation of the proteome. Proteins were classified by Gene Ontology annotation based on three categories: biological processes, cellular components, and molecular function.
Enrichment of gene ontology analysis
A two-tailed Fisher’s exact test was used to test the enrichment of the differentially expressed proteins against all identified proteins in each category of the GO annotation. A corrected p-value of <0.05 was considered statistically significant.
Enrichment of pathway analysis
The Encyclopedia of Genes and Genomes (KEGG) database was used to identify enriched pathways using a two-tailed Fisher’s exact test for enrichment of differentially expressed proteins among all identified proteins. Pathways with a corrected p-value of <0.05 were considered statistically significant. These pathways were classified into hierarchical categories according to criteria applied within the KEGG website.
Statistics
All data were reported as means ± standard errors of the mean (SEM) for the three independent experiments. Statistical analysis was performed with one-way analysis of variance (ANOVA), followed by Benjamini and Hochberg (BH) with FDR correction in code of R followed by a Tukey’s post-test (Jingjie, Hangzhou, China). Two-tailed Fisher’s exact tests were used to calculate the statistical significance of the values of the conditions in each comparison for each independent condition in GO analysis (UniProt-GOA database, see footnote 3, and the InterProScan soft) and KEGG (KEGG Orthology database, and KAAS). In all cases, the threshold for statistical significance was set at p < 0.05.
Results
Identification of differentially expressed proteins in the hippocampus
As shown in Figure 1, proteins in the hippocampus were analyzed in three biological replicates. To understand the early phase of seizure events occurring in the hippocampus, global protein expression levels in the hippocampus on day 1 and day 3 in lithium-pilocarpine induced acute epileptic rat models were analyzed using a TMT-based proteomic approach. In total, 6,157 proteins were identified, and 5,593 proteins were quantified. Therefore, the fold-change threshold was set to 1.2, and statistically significant values were defined as those with corrected p-values of <0.05.
On day 1 following lithium-pilocarpine administration, the expression of 89 proteins was upregulated, whereas the expression of 28 proteins was downregulated compared with controls (Table 1). On day 3 following lithium-pilocarpine administration, the expression of 34 proteins was promoted whereas that of 25 proteins was inhibited as compared with controls (Table 2).
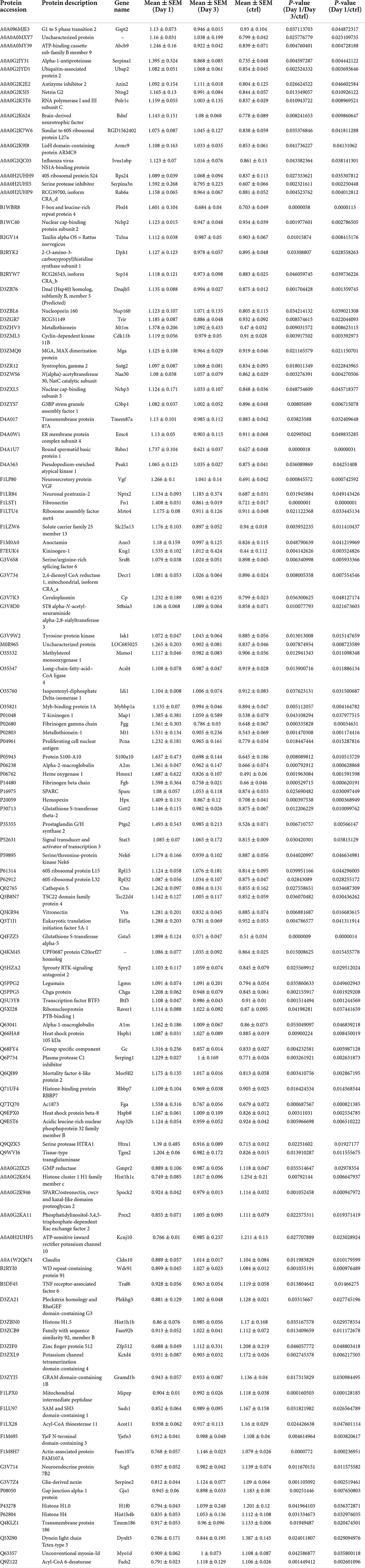
Table 1. Differentially expression proteins on Day 1 comparing with control (ctrl) in hippocampus post ANOVA analysis.
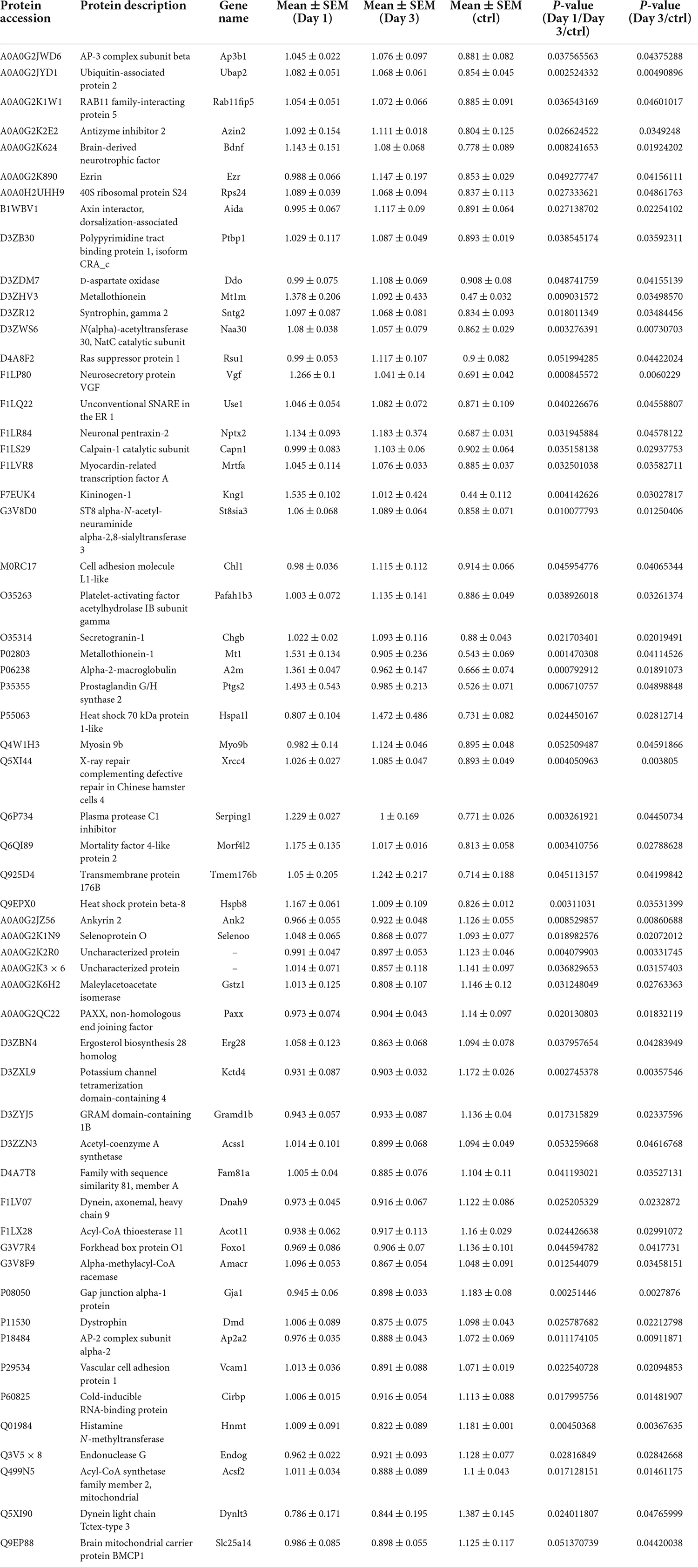
Table 2. Differentially expression proteins on Day 3 comparing with control (ctrl) in hippocampus post ANOVA analysis.
These results indicate that protein expression is likely to be enhanced rather than prohibited in modulating seizures. Moreover, the expression levels of only 16 proteins were upregulated on both day 1 and day 3, while only five proteins were downregulated on both day 1 and day 3, suggesting that different molecules and pathways are involved in seizure events occurring from day 1 to day 3 following lithium-pilocarpine administration.
These results also suggest that the decreased number of upregulated proteins from day 1 to day 3 following lithium-pilocarpine administration may illustrate the possibility that the early phase of seizure events requires more molecules and activated pathways than are necessary in the late phase. Indeed, comparing day 3 to day 1 following lithium-pilocarpine administration, we found that 14 proteins were promoted and 37 proteins were impeded. Thus, only two upregulated proteins were the same on days 1 and 3 following lithium-pilocarpine administration (Table 3). These results suggest that epileptic events on day 1 and day 3 following lithium-pilocarpine administration require different molecules and different pathways for effective facilitation.
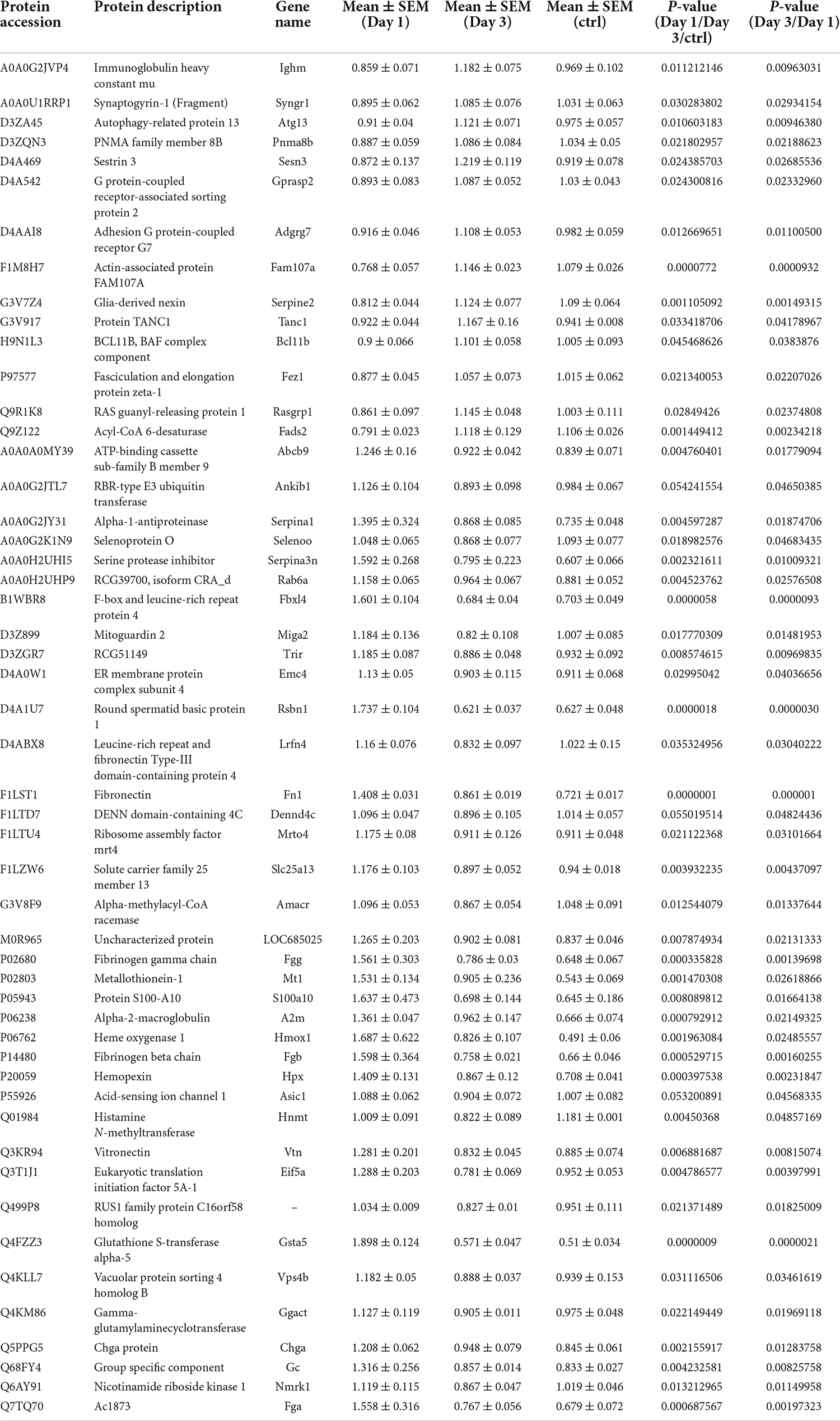
Table 3. Differentially expression proteins on Day 3 comparing with Day 1 in hippocampus post ANOVA analysis.
Subcellular distribution of differentially expressed proteins in the hippocampus
To predict the cellular functions of differentially expressed proteins, the locations of these proteins were analyzed in the current study. The total number of differentially expressed protein in the hippocampus was 117 on day 1 following lithium-pilocarpine administration (compared with controls). Using subcellular location analysis, the distribution was as follows: nucleus (26.5%, 31 proteins), extracellular (25.64%, 30 proteins), cytoplasm (24.79%, 29 proteins), mitochondria (7.69%, 9 proteins), plasma membrane (5.98%, 7 proteins), cytoplasm, nucleus (5.98%, 7 proteins), peroxisome (1.71%, two proteins, heme oxygenase 1, HMOX1; methylsterol monooxygenase 1, MSMO1), endoplasmic reticulum (0.85%, one protein, serine protease inhibitor, SERPINA3N), and cytoskeleton (0.85%, one protein, LisH domain-containing protein ARMC9, ARMC9) (Table 4). On day 3 following lithium-pilocarpine administration (as compared with controls), 59 proteins were distributed in the hippocampus as follows (as determined by subcellular location analysis): extracellular (29%, 17 proteins), the nucleus (22%, 13 proteins), cytoplasm (22%, 13 proteins), mitochondria (10%, six proteins), plasma membrane (8%, 5 proteins), cytoplasm and nucleus (7%, 4 proteins), and endoplasmic reticulum (2%, one protein, cell adhesion molecule L1-like, CHL1) (Table 5). The subcellular location of 51 differentially expressed proteins on day 3 following lithium-pilocarpine administration, compared with day 1, was as follows: the extracellular (25%, 13 proteins), nucleus (25%, 13 proteins), cytoplasm (20%, 10 proteins), plasma membrane (18%, 9 proteins), mitochondria (8%, 4 proteins), peroxisome (2%, 1 protein: HMOX1), and endoplasmic reticulum (2%, 1 protein, SERPINA3N) (Table 6).
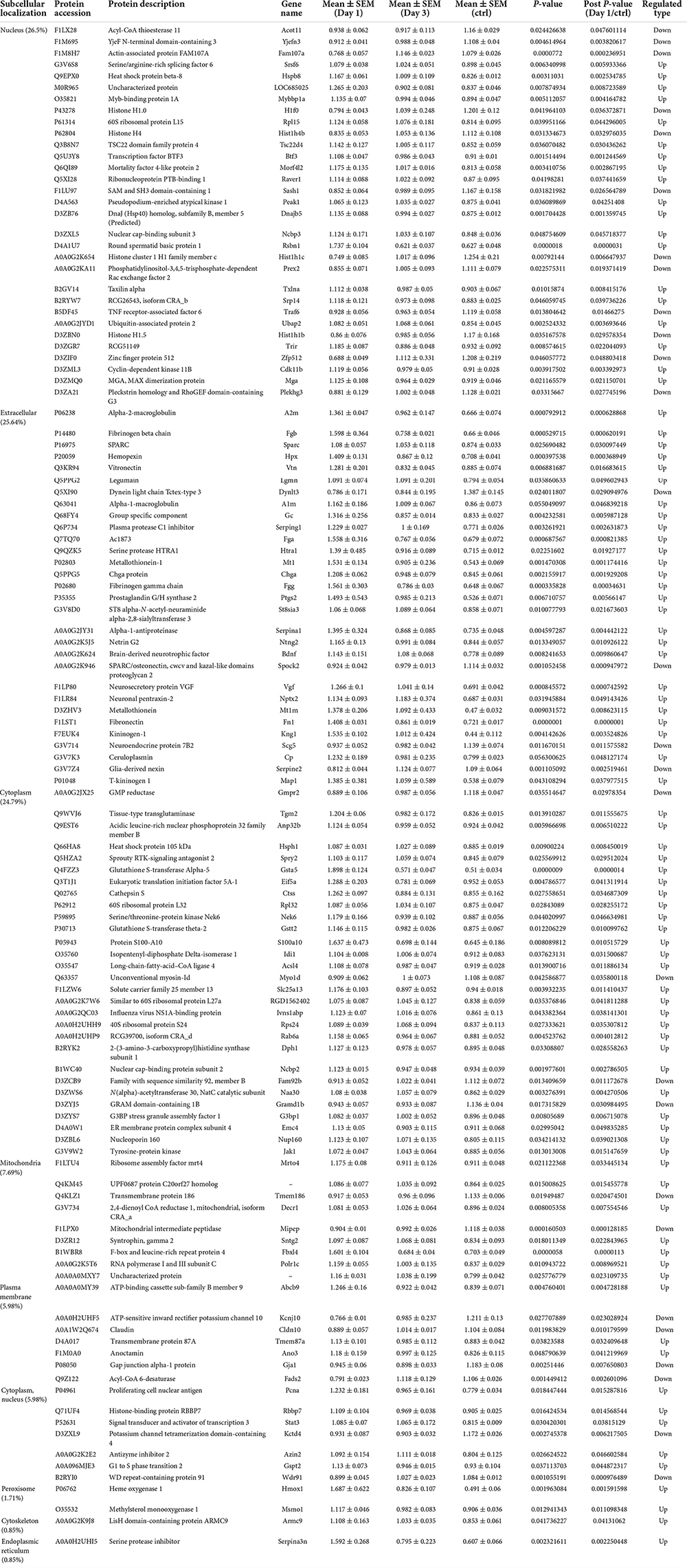
Table 4. Cellular distribution of differentially expression proteins on Day 1 comparing with control (ctrl) by ANOVA analysis in hippocampus.
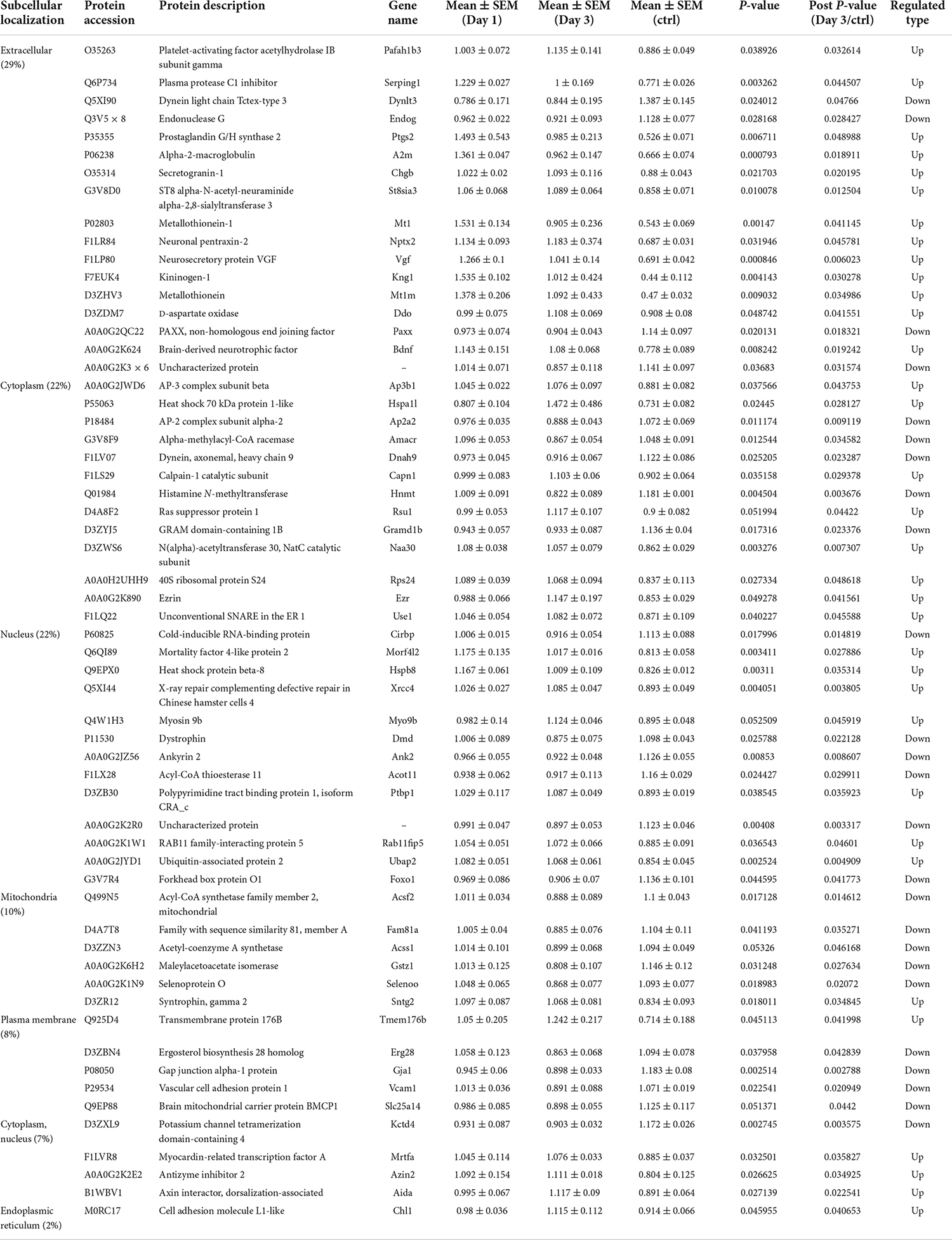
Table 5. Cellular distribution of differentially expression proteins on Day 3 comparing with control (ctrl) by ANOVA analysis in hippocampus.
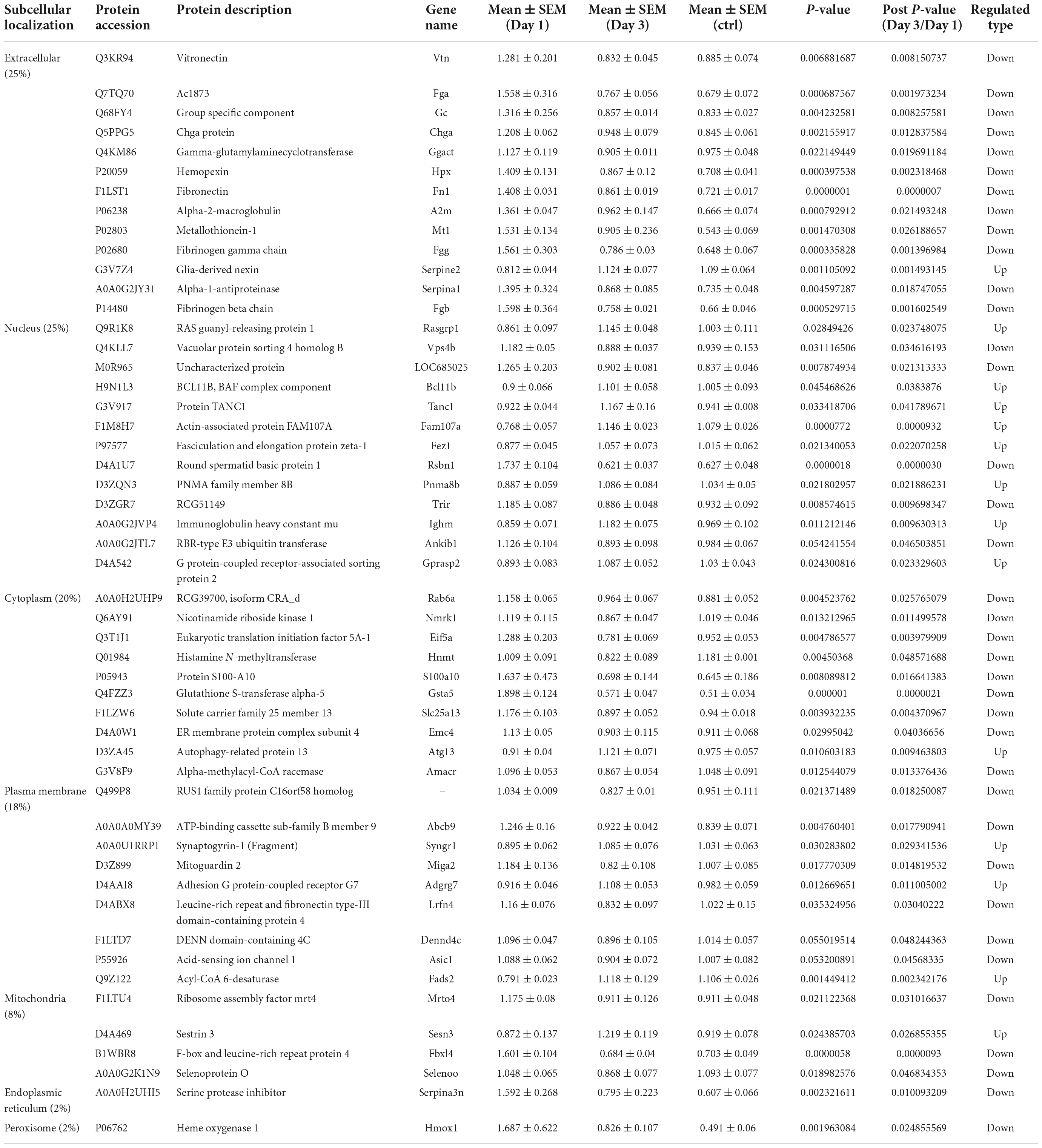
Table 6. Cellular distribution of differentially expression proteins on Day 3 comparing with Day 1 by ANOVA analysis in hippocampus.
These results show that the number of subcellularly distributed proteins decreased by more than half on day 3 compared with that found on day 1 following lithium-pilocarpine administration, indicating that different cellular functions are required during seizure progression. Indeed, SERPINA3N was the only protein to be regulated in the endoplasmic reticulum, ARMC9 was the only proteins to be modulated in the cytoskeleton on day 1 following lithium-pilocarpine administration compared with controls (Table 4), and CHL1 was the only proteins to be modulated in the endoplasmic reticulum on day 3 following lithium-pilocarpine administration compared with controls (Table 5).
All of these results suggest that cellular function in the hippocampus following seizures is possibly regulated in a differential manner. On day 3 following lithium-pilocarpine administration (compared with day 1), shared 21 proteins among differentially regulated proteins distributed in subcellular locations, representing a small portion of regulated proteins (Tables 4, 5). On day 3 following lithium-pilocarpine administration (compared with day 1), 22 proteins were the same within day 1 following lithium-pilocarpine administration compared with controls (in evaluations conducted via subcellular analysis, Tables 4, 6), and only four proteins were same within day 3 following lithium-pilocarpine administration compared with controls (Tables 5, 6), suggesting that cells are recruited on a large scale in the mediation of early versus late seizure activity in the hippocampus. Moreover, alpha-2-macroglobulin (A2M), and Metallothionein-1 (MT1) were observed to be regulated on both day 1 and day 3 following lithium-pilocarpine administration (as compared with controls). Specifically, A2M, and MT1 were upregulated on both days, but the increases on day 3 were lower than that on day 1. To better understand the functionality of differentially expressed proteins, GO and KEGG pathway-based enrichment analyses were performed, as described below.
Gene ontology annotation and analysis of differentially expressed proteins
The number of differentially expressed proteins was calculated using level 2 GO terms according to GO annotation information, which contributed to characterization of their bio-functions. On day 1 following lithium-pilocarpine administration (compared with controls), 18 proteins were mapped within macromolecular complex assembly, 17 proteins were mapped within regulation of programmed cell death or regulation of apoptotic process, 20 proteins were mapped within negative regulation of programmed cell death, and five proteins were mapped within positive regulation of blood circulation in the “Biological Process” category (Figure 2A). A total of 18 proteins were clustered within RNA binding, six proteins were classified as peptidase inhibitor activity, endopeptidase regulator activity or endopeptidase inhibitor activity in the “Molecular Function” category (Figure 2B). A total of 101 proteins participated in extracellular exosome, vesicle, organelle, and region in the “Cellular Component” category (Figure 2C).
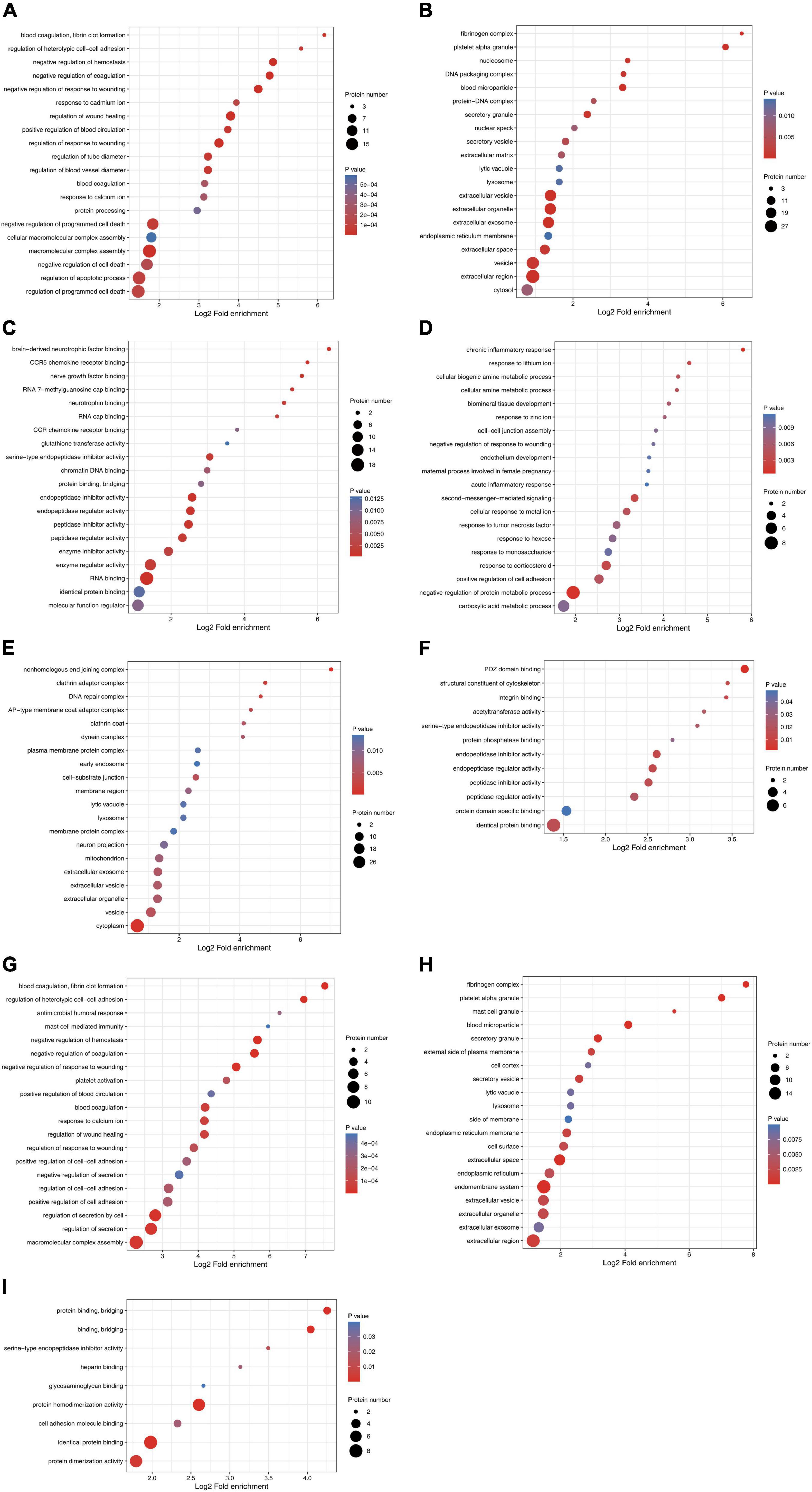
Figure 2. Functional enrichment and cluster analysis of differentially expressed proteins in hippocampus in lithium-pilocarpine induced acute epileptic rat. (A) GO “biological process” enrichment of differentially expressed proteins on Day 1 comparing with control (ctrl) in hippocampus. (B) GO “cellular component” enrichment of differentially expressed proteins on Day 1 comparing with control (ctrl) in hippocampus. (C) GO “molecular function” enrichment of differentially expressed proteins on Day 1 comparing with control (ctrl) in hippocampus. (D) GO “biological process” enrichment of differentially expressed proteins on Day 3 comparing with control (ctrl) in hippocampus. (E) GO “cellular component” enrichment of differentially expressed proteins on Day 3 comparing with control (ctrl) in hippocampus. (F) GO “molecular function” enrichment of differentially expressed proteins on Day 3 comparing with control (ctrl) in hippocampus. (G) GO “biological process” enrichment of differentially expressed proteins on Day 3 comparing with Day 1 in hippocampus. (H) GO “cellular component” enrichment of differentially expressed proteins on Day 3 comparing with Day 1 in hippocampus. (I) GO “molecular function” enrichment of differentially expressed proteins on Day 3 comparing with Day 1 in hippocampus.
All these results indicate that, on day 1 following lithium-pilocarpine administration, macromolecular complex assembly, cell death and apoptotic process, blood circulation, RNA binding, and the extracellular regulation, were the main regulation targets in the hippocampus. On day 3 following lithium-pilocarpine administration (compared with controls), eight proteins were mapped within the negative regulation of protein metabolic process, four proteins were clustered within positive regulation of cell adhesion, or response to corticosteroid in the “Biological Process” category (Figure 2D). Thirty-three proteins were mapped to cytoplasm, 14 proteins were predicted within vesicle, and 4 proteins were mapped within cell-substrate junction in the “Cellular Component” category (Figure 2E). Moreover, seven proteins were mapped to identical protein binding, and three proteins were clustered within PDZ domain binding, endopeptidase inhibitor activity, endopeptidase regulator activity, or peptidase inhibitor activity in the “Molecular Function” category (Figure 2F).
In addition, all these results suggest that, on day 3 following lithium-pilocarpine administration, protein metabolic process rather than macromolecular complex assembly and cell death were affected in the hippocampus. In addition, on day 3 following the induced seizures (compared with day 1 following seizures), 10 proteins were clustered in macromolecular complex assembly, 8 proteins were mapped in the regulation of secretion by the cell, and 5 proteins were classified in the regulation of cell–cell adhesion in the “Biological Process” category (Figure 2G). Moreover, 17 proteins were mapped to the endomembrane system, 16 proteins were mapped to the extracellular region, 11 proteins were clustered within extracellular space, and 5 proteins were mapped to the secretory granule, or blood microparticle in the “Cellular Component” category (Figure 2H). Eight proteins were predicted in identical protein binding, seven proteins were mapped to protein homodimerization or dimerization activity, and three proteins were predicted in cell adhesion molecule binding or protein binding and bridging in the “Molecular Function” category (Figure 2I).
These results show that, on day 3 following lithium-pilocarpine administration (compared with day 1), the ECM, the constitution of plasma membranes, cell contact and secretion, and protein complexes in the hippocampus were altered in the development of seizure events.
Distribution and KEGG function analysis of differentially expressed proteins
KEGG pathway enrichment cluster analysis was performed to assess the possible involvement of signaling pathways in seizure events. On day 1 following lithium-pilocarpine administration, as compared with controls, 10 proteins were found to be clustered in the signaling pathway in cancer (regulating sustained angiogenesis and evading apoptosis); nine proteins were upregulated, indicating the cell death processes for further seizure events. This is in line with the findings of the GO analysis presented above.
Moreover, nine upregulated proteins were predicted in complement and coagulation cascades, which participate in inflammation response, cell lysis, and phagocytosis, and four proteins were mapped to pathways relevant to MicroRNAs in cancer. Three proteins (Fibronectin, FN; Kininogen 1, KNG1; T-kininogen 1, MAP1), all increased, represent pivotal pathways for modulating seizures; namely, regulatory processes for filopodia and lamellipodia of the actin cytoskeleton, the PI3K-Akt signaling pathway, platelet activation, cell adhesion molecules (CAMs) pathway, the nucleotide-binding oligomerization domain (NOD)-like receptor signaling pathway, the sphingolipid signaling pathway, the hypoxia-inducible factor 1 (HIF-1) signaling pathway, lysosomes, ECM-receptor interaction, and inflammatory mediator regulation of transient receptor potential (TRP) channels were all regulated (Figure 3A).
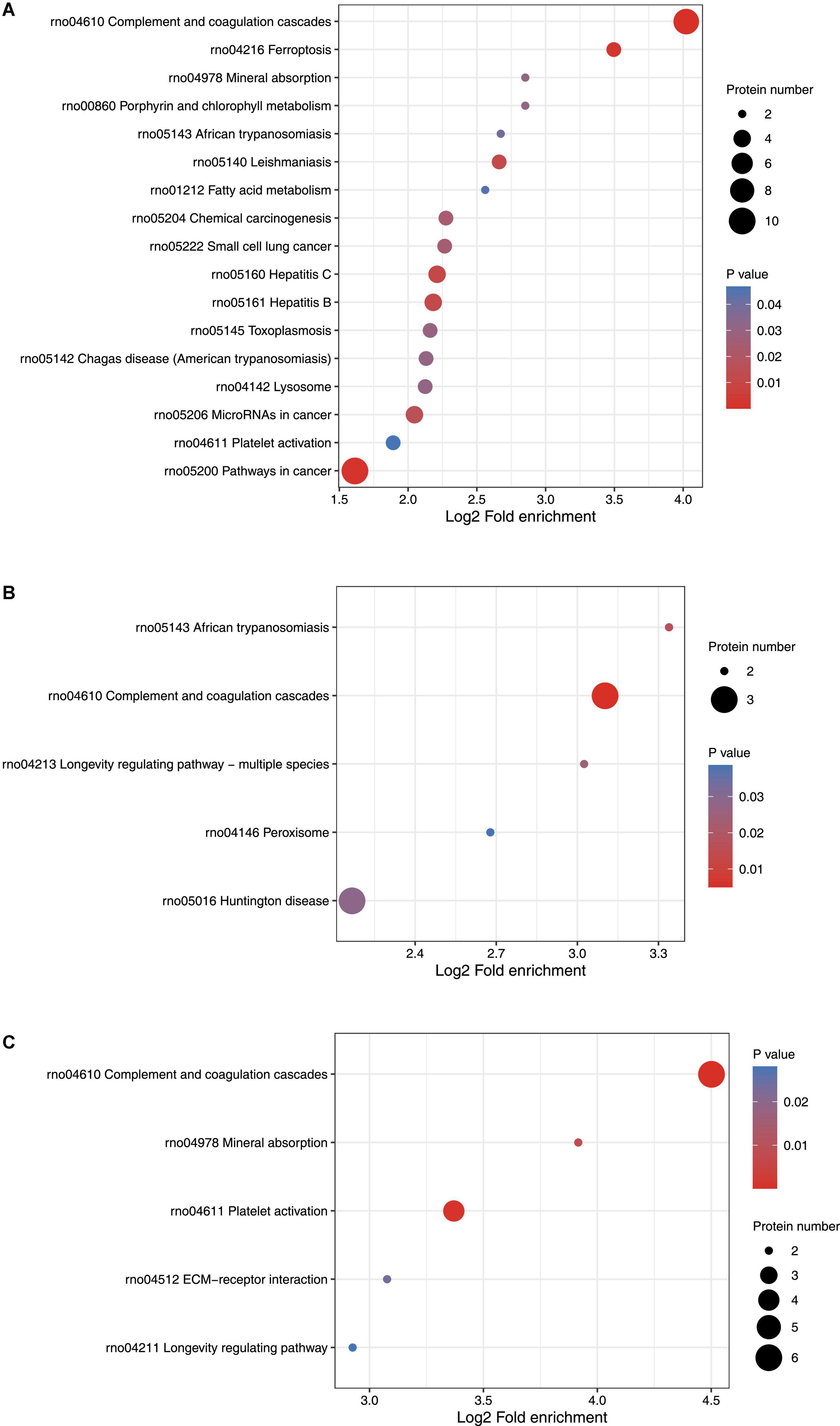
Figure 3. KEGG function analysis of differentially expressed proteins. (A) KEGG function analysis of differentially expressed proteins on Day 1 comparing with control (ctrl) in hippocampus. (B) KEGG function analysis of differentially expressed proteins on Day 3 comparing with control (ctrl) in hippocampus. (C) KEGG function analysis of differentially expressed proteins on Day 3 comparing with Day 1 in hippocampus.
On day 3 following lithium-pilocarpine administration (compared with controls), the only indicated pathways were those relevant to Huntington’s disease, TNF signaling, NF-kappa B signaling, complement and coagulation cascades, MAPK signaling, PI3K-Akt signaling, apoptosis, regulation of the actin cytoskeleton, and protein processing in the endoplasmic reticulum. However, there were no more than four proteins in each pathway, indicating that the involved pathways were possibly less active on day 3 than on day 1 following lithium-pilocarpine administration (as compared with controls) (Figure 3B).
On day 3 following lithium-pilocarpine administration (as compared with on day 1), six proteins were found to participate in complement and coagulation cascades, four proteins were mapped in the pathway of cancer, or platelet activation. The minority of proteins were predicted to regulate were the PI3K-Akt signaling pathway, mineral absorption, proteoglycans (mediators of cancer tissue mechanics), and ECM-receptor interaction (in which only two proteins were involved) (Figure 3C).
Discussion
Epilepsy in an umbrella term that describes varieties of convulsive disorders. Studies on epileptogenesis have been conducted in animal models of SE, such as lithium-pilocarpine induced rodent animal models. The hippocampus is the primary site of epileptic activity. Therefore, we conducted a study to detect global protein expression in the hippocampus in SE induced by lithium-pilocarpine in order to understand seizure events in regard to late phase epileptogenesis. In our study, we identified 6,157 proteins in total and quantified 5,593 proteins. Most of the differentially expressed proteins were predicted to be upregulated in the hippocampus on days 1 and 3 following lithium-pilocarpine administration, indicating that protein expression was likely to be enhanced rather than prohibited in the modulation of seizures within SE. Moreover, the number of enhanced proteins in the hippocampus decreased by more than half from day 1 to day 3, and only a small portion of proteins were the same when comparing these timepoints, suggesting that different molecules and pathways are involved in epilepsy events occurring from day 1 to day 3 following lithium-pilocarpine administration.
In our study, several differential expression proteins involved in the phasing of seizure events, such as EIF5A. Previous work has demonstrated that reduced hypusinated EIF5A causes neurological impairment, including seizures (Ganapathi et al., 2019). Further, a prior study demonstrated that EIF5A regulated neuronal survival and growth (Huang et al., 2007), indicating that EIF5A and other molecules are upregulated in protecting neurons against the damage caused by seizures. Several roles of EIF5A have been reported; for example, neuronal apoptosis is regulated by EIF5A/p53 (Li et al., 2004), axonal growth of dorsal root ganglion (DRG) neurons is stimulated by brain-derived neurotrophic factor (BDNF)/arginase I/EIF5A/cAMP (Cai et al., 2002; Huang et al., 2007), and EIF5A variants have been found to cause several disorders, such as developmental delay, intellectual disability, facial dysmorphisms, and microcephaly (Park et al., 2022). Of note, EIF5A stabilizes ribosome components and promotes mRNA translation termination and elongation (Chen et al., 1996; Schuller et al., 2017). In our study, the levels of several ribosome components increased on day 1 following lithium-pilocarpine administration, suggesting the potential function of EIF5A in stabilizing ribosomes for mRNA transcription. However, on day 3 following lithium-pilocarpine administration, the levels of EIF5A and ribosome components were decreased and were similar to those of controls, suggesting neuron loss, apoptosis, and degeneration in the hippocampus.
In intractable epilepsy (IE), the neural network is reorganized to properly transduce signals; this is a prominent pathological change that leads to the transient expression of several molecules, such as netrin G2, fibronectin (Fn), and vitronectin. Netrin G2 has been shown to modulate synapse formation and neurite outgrowth (Lin et al., 2003; Kim et al., 2006). Moreover, the overexpression of netrin G2 within excitatory neurons in patients with IE and in the hippocampus of lithium-pilocarpine induced rat models is assumed to be commensurate with abnormal synapse development and neuron migration (Woo et al., 2009; Pan et al., 2010); this is in line with our findings reported here, as epileptic discharges and spreading are supported by abnormal synapses (Buckmaster et al., 2002). Moreover, synaptic reorganization promotes the development of the excitatory loop, and Fn and its integrin receptor are known to participate in the pathophysiology of epilepsy (Gall and Lynch, 2004; Dityatev and Fellin, 2008; Wu and Reddy, 2012; Pitkanen et al., 2014). Other studies in addition to our own have shown that Fn expression is increased in the hippocampus after a first behavioral seizure (Hoffman et al., 1998; Wu et al., 2017). Moreover, epileptogenesis is modulated by Fn by modulating neuronal cell plasticity and mechanical properties in the hippocampus in epilepsy via its integrin receptor (Wu et al., 2017). Fn is rapidly synthesized, which was assumed by proliferated astrocytes in the hippocampus of epileptic rats (Niquet et al., 1994; Hoffman et al., 1998), and Fn and integrin interactions modulate cell adhesion and membrane elasticity in epilepsy model mice (Wu et al., 2016). Some proliferated astrocytes produce vitronectin in the hippocampus, which is related with neuronal degeneration in rat models of kainic acid (KA)-induced seizures (Niquet et al., 1996). Taken together, these findings suggest that several molecules, especially ECM molecules, contribute to reorganizing the neural network for modulating excitotoxicity in seizures in the hippocampus in lithium-pilocarpine induced SE animal models.
Lack of mitochondrial intermediate peptidase (MIP) causes seizures (Eldomery et al., 2016). Tumor necrosis factor-α receptor-associated factor 6 (TRAF6) is a key element of the transforming growth factor beta (TGFβ)-associated inflammation pathway and activates TGFβ-activated kinase 1 (TAK1) (Takaesu et al., 2000); this further leads to promoting the expression of proinflammatory cytokines and to the aggravation of inflammation (Onodera et al., 2015). Moreover, molecule causing demyelination/hypomyelination, such as gap junction alpha-1 protein (GJA1), decreases in the hippocampus on day 1 in the epileptic rat; this shows that demyelination is present in the early phases of seizure development, which is in line with the findings of our previously published study (Hobson and Garbern, 2012; Yalcinkaya et al., 2012; Basu and Sarma, 2018; Li T. et al., 2020; Wang et al., 2021). The deficit of adenosine triphosphate (ATP)-sensitive inward rectifier potassium channel 10 (KCNJ10) causes seizures and myelin vacuolization (Phani et al., 2014; Larson et al., 2018; Zhu et al., 2020). Metallothionein-1 (MT1), a zinc binding protein, exerts neuroprotection by reducing proinflammatory responses, increasing neurotrophins, and delaying neuron degeneration (Penkowa et al., 2005). In MT1-deficient mice, seizures are enhanced and neurons in hippocampus are injured, leading to apoptosis (Carrasco et al., 2000). Moreover, higher hemopexin levels are detected in the serum of schizophrenic patients than in normal subjects (Clarke et al., 1970).
Signal transducer and activator of transcription 3 (STAT3) is highly expressed in children with epilepsy (Li Y. Z. et al., 2020). Moreover, SE induced by pilocarpine activates the Janus kinase-signal transducer and activator of transcription (JAK/STAT) pathway and the STAT3-mediated signaling pathway and promotes neuronal cell death and glia activation by producing interleukin 1 beta (IL-1β) in mice with induced SE (Tian et al., 2017; Han et al., 2020). Further, inhibition of STAT3 decreases spontaneous seizure frequency and the severity of chronic epilepsy (Grabenstatter et al., 2014). DnaJ heat shock protein family member B5 (DNAJB5) and heat shock proteins (HSPs) (HSPH1 and HSPB8) are upregulated in the hippocampus of epileptic mice to protect neurons (Lee et al., 2021).
HMOX1 is upregulated in epileptic rats (Wang et al., 2013; Prakash et al., 2019), and previous studies have found that tissue-type transglutaminase (TGM2) is mostly produced by neurons in the mammalian nervous system and is elevated in neurodegenerative diseases as well as in response to acute CNS injury, which possibly induces neuronal cell death (Tucholski et al., 2006). Cathepsin S (CTSS) is mainly produced by microglia in the hippocampi of kainate-injected mice (Akahoshi et al., 2007), and prohibition of its function resulted in reducing inflammation and alleviating brain edema in a mouse model of traumatic brain injury (Xu et al., 2013). Proliferation cell nuclear antigen (PCNA) is highly expressed in epileptic animal models and in the human brain (Zhang et al., 2005; Liu et al., 2008). BDNF and the tropomyosin kinase receptor B (TRKB) pathway are predicted to function in the prevention or suppression of epilepsy targets (Lin et al., 2020; Sullivan and Kadam, 2021). The neurosecretory vascular growth factor (VGF) protein plays a critical role in the control of energy homeostasis, and the high expression of VGF in the CNS in seizure animal models is in line with a high requirement for energy (Salton et al., 2000). In addition, dystrophin is a component of gamma-aminobutyric acid (GABA)ergic synapses and plays a role in normal cognitive (i.e., episodic memory) processes (Knuesel et al., 2001; Hoogland et al., 2019); the absence of dystrophin is associated with epilepsy (Hoogland et al., 2019). Taken together, these findings provide a comprehensive picture of relevant pathways occurring during seizure development.
In conclusion, to the best of our knowledge, this study is to investigate global protein expression in the acute phase of epileptic seizures from lithium-pilocarpine induced rats using a tandem mass tag (TMT)-based proteomic approach and identified 6,157 differentially expressed proteins in total and 5,593 proteins quantified in the experimental and control groups. Of note, the majority of the differentially expressed proteins were predicted to be upregulated in the hippocampus on days 1 and 3 following lithium-pilocarpine administration, indicating that protein expression was likely to be enhanced rather than prohibited in the modulation of seizures within SE. Moreover, the number of enhanced proteins in the hippocampus decreased by more than half from day 1 to day 3, and only a small portion of proteins were the same when comparing day 1 to day 3, suggesting that different molecules and pathways are involved in epilepsy events occurring from day 1 to day 3 following lithium-pilocarpine administration. On day 1 following lithium-pilocarpine administration, as compared with controls, 10 proteins were found to be clustered in the signaling pathway in cancer (regulating sustained angiogenesis and evading apoptosis); nine proteins were upregulated, indicating the cell death processes for further seizure events. Moreover, nine upregulated proteins were predicted in complement and coagulation cascades, which participate in inflammation response, cell lysis, and phagocytosis, and four proteins were mapped to pathways relevant to MicroRNAs in cancer. On day 3 following lithium-pilocarpine administration (compared with controls), the only indicated pathways were those relevant to Huntington’s disease, TNF signaling, NF-kappa B signaling, etc., however, there were no more than four proteins in each pathway. On day 3 following lithium-pilocarpine administration (as compared with day 1), the majority of proteins were found to participate in complement and coagulation cascades, pathways relevant to cancer, and platelet activation. Our results suggest that the different molecules and pathways are involved in seizure events occurring from day 1 to day 3 following lithium-pilocarpine administration. These proteins may serve as candidate proteins for the development of seizure events and need to be studied further. Meanwhile, it is necessary to point out that the present study is a preliminary investigation. These differentially expressed proteins need to be further validated using other analyses, and a large-scale validation and a long-term strategy for proteomics analysis in the chronic phase of epileptic animals are also required.
Data availability statement
The datasets presented in this study can be found in online repositories. The data presented in the study are deposited in FTP repository, the website is ftp://115.238.71.26, the account number is: ptm_ftp_0935 and the accession number is: B4y171.
Ethics statement
This animal study was reviewed and approved by the National Institutes of Health and the Animal Welfare Committee of Ningxia Medical University (Ethics Approval Number: 2019-151, Ningxia, China).
Author contributions
PW and LY conceived and designed the experiments. LY, ZC, XR, and FW performed the experiments. RY, YJ, YD, and FY analyzed the data and collected the references. PW wrote the first draft. HM, TS, and PW checked and revised the draft. All authors approved the submission of this manuscript to be published.
Funding
This work was supported by grants from the National Natural Science Foundation of China (81960232 and 82001282), the Key R&D Plan Project of Ningxia (2019BEB04041), Overseas Students’ Innovation and Entrepreneurship Individual Project of Ningxia (2021), Youth Talents Supporting Program of Ningxia Medical University and Ningxia (XT2019018 and TJGC2019081), and College Students’ Innovation and Entrepreneurship Training Program (X202210752038). These funders had no role in the design, conducts, or reporting of this work.
Conflict of interest
The authors declare that the research was conducted in the absence of any commercial or financial relationships that could be construed as a potential conflict of interest.
Publisher’s note
All claims expressed in this article are solely those of the authors and do not necessarily represent those of their affiliated organizations, or those of the publisher, the editors and the reviewers. Any product that may be evaluated in this article, or claim that may be made by its manufacturer, is not guaranteed or endorsed by the publisher.
Footnotes
References
Akahoshi, N., Murashima, Y. L., Himi, T., Ishizaki, Y., and Ishii, I. (2007). Increased expression of the lysosomal protease cathepsin S in hippocampal microglia following kainate-induced seizures. Neurosci. Lett. 429, 136–141. doi: 10.1016/j.neulet.2007.10.007
André, V., Dubé, C., Francóis, J., Leroy, C., Rigoulot, M. A., Roch, C., et al. (2007). Pathogenesis and pharmacology of epilepsy in the lithium–pilocarpine model. Epilepsia 48, 41–47. doi: 10.1111/j.1528-1167.2007.01288.x
Basu, R., and Sarma, J. D. (2018). Connexin 43/47 channels are important for astrocyte/oligodendrocyte cross-talk in myelination and demyelination. J. Biosci. 43, 1055–1068. doi: 10.1007/s12038-018-9811-0
Begcevic, I., Kosanam, H., Martínez-Morillo, E., Dimitromanolakis, A., Diamandis, P., Kuzmanov, U., et al. (2013). Semiquantitative proteomic analysis of human hippocampal tissues from Alzheimer’s disease and age-matched control brains. Clin. Proteomics 10:5. doi: 10.1186/1559-0275-10-5
Buckmaster, P. S., Zhang, G. F., and Yamawaki, R. (2002). Axon sprouting in a model of temporal lobe epilepsy creates a predominantly excitatory feedback circuit. J. Neurosci. 22, 6650–6658. doi: 10.1523/JNEUROSCI.22-15-06650.2002
Cai, D., Deng, K., Mellado, W., Lee, J., Ratan, R. R., and Filbin, M. T. (2002). Arginase I and polyamines act downstream from cyclic AMP in overcoming inhibition of axonal growth MAG and myelin in vitro. Neuron 35, 711–719. doi: 10.1016/s0896-6273(02)00826-7
Carrasco, J., Penkowa, M., Hadberg, H., Molinero, A., and Hidalgo, J. (2000). Enhanced seizures and hippocampal neurodegeneration following kainic acid-induced seizures in metallothionein-I + II-deficient mice. Eur. J. Neurosci. 12, 2311–2322. doi: 10.1046/j.1460-9568.2000.00128.x
Cavalheiro, E. A., Leite, J. P., Bortolotto, Z. A., Turski, W. A., Ikonomidou, C., and Turski, L. (1991). Long-term effects of pilocarpine in rats: Structural damage of the brain triggers kindling and spontaneous recurrent seizures. Epilepsia 32, 778–782.
Chakir, A., Fabene, P. F., Ouazzani, R., and Bentivoglio, M. (2006). Drug resistance and hippocampal damage after delayed treatment of pilocarpine-induced epilepsy in the rat. Brain Res. Bull. 71, 127–138.
Chen, Z. P., Yan, Y. P., Ding, Q. J., Knapp, S., Potenza, J. A., Schugar, H. J., et al. (1996). Effects of inhibitors of deoxyhypusine synthase on the differentiation of mouse neuroblastoma and erythroleukemia cells. Cancer Lett. 105, 233–239. doi: 10.1016/0304-3835(96)04287-5
Clarke, H. G., Freeman, T., and Pryse-Phillips, W. (1970). Quantitative immunoelectrophoresis of serum from hospitalized chronic schizophrenic and epileptic patients. J. Neurol. Neurosurg. Psychiatry 33, 694–697. doi: 10.1136/jnnp.33.5.694
Clifford, D. B., Olney, J. W., Maniotis, A., Collins, R. C., and Zorumski, C. F. (1987). The functional anatomy and pathology of lithium-pilocarpine and high-dose pilocarpine seizures. Neuroscience 23, 953–968.
Dityatev, A., and Fellin, T. (2008). Extracellular matrix in plasticity and epileptogenesis. Neuron Glia Biol. 4, 235–247.
Edgar, P. F., Schonberger, S. J., Dean, B., Faull, R. L., Kydd, R., and Cooper, G. J. (1999b). A comparative proteome analysis of hippocampal tissue from schizophrenic and Alzheimer’s disease individuals. Mol. Psychiatry 4, 173–178. doi: 10.1038/sj.mp.4000463
Eldomery, M. K., Akdemir, Z. C., Vögtle, F. N., Charng, W. L., Mulica, P., Rosenfeld, J. A., et al. (2016). MIPEP recessive variants cause a syndrome of left ventricular non-compaction, hypotonia, and infantile death. Genome Med. 8:106. doi: 10.1186/s13073-016-0360-6
Fisher, R. S., van Emde Boas, W., Blume, W., Elger, C., Genton, P., Lee, P., et al. (2005). Epileptic seizures and epilepsy: Definitions proposed by the international league against epilepsy (ILAE) and the international bureau for epilepsy (IBE). Epilepsia 46, 470–472.
Gall, C. M., and Lynch, G. (2004). Integrins, synaptic plasticity and epileptogenesis. Adv. Exp. Med. Biol. 548, 12–33.
Ganapathi, M., Padgett, L. R., Yamada, K., Devinsky, O., Willaert, R., Person, R., et al. (2019). Recessive rare variants in deoxyhypusine synthase, an enzyme involved in the synthesis of hypusine, are associated with a neurodevelopmental disorder. Am. J. Hum. Genet. 104, 287–298. doi: 10.1016/j.ajhg.2018.12.017
Glien, M., Brandt, C., Potschka, H., and Löscher, W. (2002). Effects of the novel antiepileptic drug levetiracetam on spontaneous recurrent seizures in the rat pilocarpine model of temporal lobe epilepsy. Epilepsia 43, 350–357.
Grabenstatter, H. L., Del Angel, Y. C., Carlsen, J., Wempe, M. F., White, A. M., Cogswell, M., et al. (2014). The effect of STAT3 inhibition on status epilepticus and subsequent spontaneous seizures in the pilocarpine model of acquired epilepsy. Neurobiol. Dis. 62, 73–85. doi: 10.1016/j.nbd.2013.09.003
Hamilton, S. E., Loose, M. D., Qi, M., Levey, A. I., Hille, B., McKnight, G. S., et al. (1997). Disruption of the m1 receptor gene ablates muscarinic receptor-dependent M current regulation and seizure activity in mice. Proc. Natl. Acad. Sci. U.S.A. 94, 13311–13316.
Han, C. L., Liu, Y. P., Guo, C. J., Du, T. T., Jiang, Y., Wang, K. L., et al. (2020). The lncRNA H19 binding to let-7b promotes hippocampal glial cell activation and epileptic seizures by targeting Stat3 in a rat model of temporal lobe epilepsy. Cell Prolif. 53:e12856. doi: 10.1111/cpr.12856
Hobson, G. M., and Garbern, J. Y. (2012). Pelizaeus-Merzbacher disease. Pelizaeus-merzbacher-like disease 1, and related hypomyelinating disorders. Semin. Neurol. 32, 62–67. doi: 10.1055/s-0032-1306388
Hoffman, K. B., Pinkstaff, J. K., Gall, C. M., and Lynch, G. (1998). Seizure induced synthesis of fibronectin is rapid and age dependent: Implications for long-term potentiation and sprouting. Brain Res. 812, 209–215.
Honchar, M. P., Olney, J. W., and Sherman, W. R. (1983). Systemic cholinergic agents induce seizures and brain damage in lithium-treated rats. Science 220, 323–325. doi: 10.1126/science.6301005.
Hondius, D. C., van Nierop, P., Li, K. W., Hoozemans, J. J. M., van der Schors, R. C., van Haastert, E. S., et al. (2016). Profiling the human hippocampal proteome at all pathologic stages of Alzheimer’s disease. Alzheimers Dement. 12, 654–668. doi: 10.1016/j.jalz.2015.11.002
Hoogland, G., Hendriksen, R. G. F., Slegers, R. J., Hendriks, M. P. H., Schijns, O. E. M. G., Aalbers, M. W., et al. (2019). The expression of the distal dystrophin isoforms Dp140 and Dp71 in the human epileptic hippocampus in relation to cognitive functioning. Hippocampus 29, 102–110. doi: 10.1002/hipo.23015
Huang, Y., Higginson, D. S., Hester, L., Park, M. H., and Snyder, S. H. (2007). Neuronal growth and survival mediated by eIF5A, a polyamine-modified translation initiation factor. Proc. Natl. Acad. Sci. U.S.A. 104, 4194–4199. doi: 10.1073/pnas.0611609104
Kim, S., Burette, A., Chung, H. S., Kwon, S. K., Woo, J., Lee, H. W., et al. (2006). NGL family PSD-95-interacting adhesion molecules regulate excitatory synapse formation. Nat. Neurosci. 9, 1294–1301.
Knuesel, I., Zuellig, R. A., Schaub, M. C., and Fritschy, J. M. (2001). Alterations in dystrophin and utrophin expression parallel the reorganization of GABAergic synapses in a mouse model of temporal lobe epilepsy. Eur. J. Neurosci. 13, 1113–1124. doi: 10.1046/j.0953-816x.2001.01476.x
Larson, V. A., Mironova, Y., Vanderpool, K. G., Waisman, A., Rash, J. E., Agarwal, A., et al. (2018). Oligodendrocytes control potassium accumulation in white matter and seizure susceptibility. eLife. 7:e34829. doi: 10.7554/eLife.34829
Lee, T. S., Li, A. Y., Rapuano, A., Mantis, J., Eid, T., Seyfried, T. N., et al. (2021). Gene expression in the epileptic (EL) mouse hippocampus. Neurobiol. Dis. 147:105152. doi: 10.1016/j.nbd.2020.105152
Leite, J. P., Bortolotto, Z. A., and Cavalheiro, E. A. (1990). Spontaneous recurrent seizures in rats: An experimental model of partial epilepsy. Neurosci. Biobehav. Rev. 14, 511–517.
Li, A. L., Li, H. Y., Jin, B. F., Ye, Q. N., Zhou, T., Yu, X. D., et al. (2004). A novel eIF5A complex functions as a regulator of p53 and p53-dependent apoptosis. J. Biol. Chem. 279, 49251–49258. doi: 10.1074/jbc.M407165200
Li, T., Niu, J., Yu, G., Ezan, P., Yi, C., Wang, X., et al. (2020). Connexin 43 deletion in astrocytes promotes CNS remyelination by modulating local inflammation. Glia 68, 1201–1212. doi: 10.1002/glia.23770
Li, Y. Z., Zhang, L., Liu, Q., Bian, H. T., and Cheng, W. J. (2020). The effect of single nucleotide polymorphisms of STAT3 on epilepsy in children. Eur. Rev. Med. Pharmacol. Sci. 24, 837–842. doi: 10.26355/eurrev_202001_20067
Lin, J. C., Ho, W. H., Gurney, A., and Rosenthal, A. (2003). The netrin-G1 ligand NGL-1 promotes the outgrowth of thalamocortical axons. Nat. Neurosci. 6, 1270–1276.
Lin, T. W., Harward, S. C., Huang, Y. Z., and McNamara, J. O. (2020). Targeting BDNF/TrkB pathways for preventing or suppressing epilepsy. Neuropharmacology 167:107734. doi: 10.1016/j.neuropharm.2019.107734
Liu, Y. W., Curtis, M. A., Gibbons, H. M., Mee, E. W., Bergin, P. S., Teoh, H. H., et al. (2008). Doublecortin expression in the normal and epileptic adult human brain. Eur. J. Neurosci. 28, 2254–2265. doi: 10.1111/j.1460-9568.2008.06518.x
Niquet, J., Gillian, A., Ben-Ari, Y., and Represa, A. (1996). Reactive glial cells express a vitronectin-like protein in the hippocampus of epileptic rats. Glia 16, 359–367. doi: 10.1002/(SICI)1098-1136(199604)16:4<359:AID-GLIA8<3.0.CO;2-V
Niquet, J., Jorquera, I., Ben-Ari, Y., and Represa, A. (1994). Proliferative astrocytes may express fibronectin-like protein in the hippocampus of epileptic rats. Neurosci. Lett. 180, 13–16. doi: 10.1016/0304-3940(94)90902-4
Onodera, Y., Teramura, T., Takehara, T., Shigi, K., and Fukuda, K. (2015). Reactive oxygen species induce Cox-2 expression via TAK1 activation in synovial fibroblast cells. FEBS Open Bio. 5, 492–501. doi: 10.1016/j.fob.2015.06.001
Pan, Y., Liu, G., Fang, M., Shen, L., Wang, L., Han, Y., et al. (2010). Abnormal expression of netrin-G2 in temporal lobe epilepsy neurons in humans and a rat model. Exp. Neurol. 224, 340–346. doi: 10.1016/j.expneurol.2010.04.001
Park, M. H., Kar, R. K., Banka, S., Ziegler, A., and Chung, W. K. (2022). Post-translational formation of hypusine in eIF5A: Implications in human neurodevelopment. Amino Acids 54, 485–499. doi: 10.1007/s00726-021-03023-6
Penkowa, M., Florit, S., Giralt, M., Quintana, A., Molinero, A., Carrasco, J., et al. (2005). Metallothionein reduces central nervous system inflammation, neurodegeneration, and cell death following kainic acid-induced epileptic seizures. J. Neurosci. Res. 79, 522–534. doi: 10.1002/jnr.20387
Persike, D. S., Marques-Carneiro, J. E., Stein, M. L. D. L., Yacubian, E. M. T., Centeno, R., Canzian, M., et al. (2018). Altered proteins in the hippocampus of patients with mesial temporal lobe epilepsy. Pharmaceuticals (Basel). 11:E95. doi: 10.3390/ph11040095
Persike, D., Lima, M., Amorim, R., Cavalheiro, E., Yacubian, E., Centeno, R., et al. (2012). Hippocampal proteomic profile in temporal lobe epilepsy. J. Epilepsy Clin. Neurophysiol. 18, 53–56. doi: 10.1590/S1676-26492012000200007
Phani, N. M., Acharya, S., Xavy, S., Bhaskaranand, N., Bhat, M. K., Jain, A., et al. (2014). Genetic association of KCNJ10 rs1130183 with seizure susceptibility and computational analysis of deleterious non-synonymous SNPs of KCNJ10 gene. Gene 536, 247–253. doi: 10.1016/j.gene.2013.12.026
Pitkanen, A., Ndode-Ekane, X. E., Lukasiuk, K., Wilczynski, G. M., Dityatev, A., Walker, M. C., et al. (2014). Neural ECM and epilepsy. Prog. Brain Res. 214, 229–262.
Prakash, C., Mishra, M., Kumar, P., Kumar, V., and Sharma, D. (2019). Dehydroepiandrosterone alleviates oxidative stress and apoptosis in iron-induced epilepsy via activation of Nrf2/ARE signal pathway. Brain Res. Bull. 153, 181–190. doi: 10.1016/j.brainresbull.2019.08.019
Priel, M. R., and Albuquerque, E. X. (2002). Short-term effects of pilocarpine on rat hippocampal neurons in culture. Epilepsia 43, 40–46.
Racine, R. J. (1972). Modification of seizure activity by electrical stimulation. II. Motor seizure. Electroencephalogr. Clin. Neurophysiol. 32, 281–294. doi: 10.1016/0013-4694(72)90177-0
Sadeghi, L., Rizvanov, A. A., Dabirmanesh, B., Salafutdinov, I. I., Sayyah, M., Shojaei, A., et al. (2021). Proteomic profiling of the rat hippocampus from the kindling and pilocarpine models of epilepsy: Potential targets in calcium regulatory network. Sci. Rep. 11:8252. doi: 10.1038/s41598-021-87555-7
Salton, S. R., Ferri, G. L., Hahm, S., Snyder, S. E., Wilson, A. J., Possenti, R., et al. (2000). VGF: A novel role for this neuronal and neuroendocrine polypeptide in the regulation of energy balance. Front. Neuroendocrinol. 21:199–219. doi: 10.1006/frne.2000.0199
Schuller, A. P., Wu, C. C., Dever, T. E., Buskirk, A. R., and Green, R. (2017). eIF5A functions globally in translation elongation and termination. Mol. Cell 66, 194.e–205.e.
Sullivan, B. J., and Kadam, S. D. (2021). Brain-derived neurotrophic factor in neonatal seizures. Pediatr. Neurol. 118, 35–39. doi: 10.1016/j.pediatrneurol.2021.01.011
Sultana, R., Boyd-Kimball, D., Cai, J., Pierce, W. M., Klein, J. B., Merchant, M., et al. (2007). Proteomics analysis of the Alzheimer’s disease hippocampal proteome. J. Alzheimers Dis. 11, 153–164. doi: 10.3233/jad-2007-11203
Takaesu, G., Kishida, S., Hiyama, A., Yamaguchi, K., Shibuya, H., Irie, K., et al. (2000). TAB2, a novel adaptor protein, mediates activation of TAK1 MAPKKK by linking TAK1 to TRAF6 in the IL-1 signal transduction pathway. Mol. Cell 5, 649–658. doi: 10.1016/s1097-2765(00)80244-0
Tian, D. S., Peng, J., Murugan, M., Feng, L. J., Liu, J. L., Eyo, U. B., et al. (2017). Chemokine CCL2-CCR2 signaling induces neuronal cell death via STAT3 activation and IL-1β production after status epilepticus. J. Neurosci. 37, 7878–7892. doi: 10.1523/JNEUROSCI.0315-17.2017
Tucholski, J., Roth, K. A., and Johnson, G. V. (2006). Tissue transglutaminase overexpression in the brain potentiates calcium-induced hippocampal damage. J. Neurochem. 97, 582–594. doi: 10.1111/j.1471-4159.2006.03780.x
Turski, W. A., Cavalheiro, E. A., Schwarz, M., Czuczwar, S. L. J., Kleinrok, Z., and Turski, L. (1983a). Limbic seizures produced by pilocarpine in rats: Behavioural, electroencephalographic and neuropathological study. Behav. Brain Res. 9, 315–335.
Turski, W. A., Czuczwar, S. J., Kleinrok, Z., and Turski, L. (1983b). Cholinomimetics produce seizures and brain damage in rats. Experientia 39, 1408–1411.
Wang, P., Ma, K., Yang, L., Zhang, G., Ye, M., Wang, S., et al. (2021). Predicting signaling pathways regulating demyelination in a rat model of lithium-pilocarpine-induced acute epilepsy: A proteomics study. Int. J. Biol. Macromol. 193, 1457–1470. doi: 10.1016/j.ijbiomac.2021.10.209
Wang, W., Wang, W. P., Zhang, G. L., Wu, Y. F., Xie, T., Kan, M. C., et al. (2013). Activation of Nrf2-ARE signal pathway in hippocampus of amygdala kindling rats. Neurosci. Lett. 543, 58–63. doi: 10.1016/j.neulet.2013.03.038
Woo, J., Kwon, S. K., and Kim, E. (2009). The NGL family of leucine-rich repeat-containing synaptic adhesion molecules. Mol. Cell. Neurosci. 42, 1–10.
Wu, X., and Reddy, D. S. (2012). Integrins as receptor targets for neurological disorders. Pharmacol. Ther. 134, 68–81.
Wu, X., Muthuchamy, M., and Reddy, D. S. (2016). Atomic force microscopy protocol for measurement of membrane plasticity and extracellular interactions in single neurons in epilepsy. Front. Aging Neurosci. 8:88. doi: 10.3389/fnagi.2016.00088
Wu, X., Muthuchamy, M., and Reddy, D. S. (2017). Atomic force microscopy investigations of fibronectin and α5β1-integrin signaling in neuroplasticity and seizure susceptibility in experimental epilepsy. Epilepsy Res. 138, 71–80. doi: 10.1016/j.eplepsyres.2017.10.013
Xu, J., Wang, H., Ding, K., Lu, X., Li, T., Wang, J., et al. (2013). Inhibition of cathepsin S produces neuroprotective effects after traumatic brain injury in mice. Mediators Inflamm. 2013:187873. doi: 10.1155/2013/187873
Yalcinkaya, C., Erturk, O., Tuysuz, B., Yesil, G., Verbeke, J. I., Keyser, B., et al. (2012). A novel GJC2 mutation associated with hypomyelination and Müllerian agenesis syndrome: Coincidence or a new entity? Neuropediatrics 43, 159–161. doi: 10.1055/s-0032-1313912
Yang, J. W., Czech, T., and Lubec, G. (2004). Proteomic profiling of human hippocampus. Electrophoresis 25, 1169–1174. doi: 10.1002/elps.200305809
Zhang, S., Zhu, C., Liu, Q., and Wang, W. (2005). Effects of chloroquine on GFAP. PCNA and cyclin D1 in hippocampus and cerebral cortex of rats with seizures induced by pentylenetetrazole. J. Huazhong Univ. Sci. Technolog. Med. Sci. 25, 625–628. doi: 10.1007/BF02896153
Keywords: acute epilepsy, hippocampus, proteomics study, seizures, signaling pathways
Citation: Wang P, Yang L, Yang R, Chen Z, Ren X, Wang F, Jiao Y, Ding Y, Yang F, Sun T and Ma H (2022) Predicted molecules and signaling pathways for regulating seizures in the hippocampus in lithium-pilocarpine induced acute epileptic rats: A proteomics study. Front. Cell. Neurosci. 16:947732. doi: 10.3389/fncel.2022.947732
Received: 19 May 2022; Accepted: 14 November 2022;
Published: 01 December 2022.
Edited by:
Marian Christoph Neidert, Kantonsspital St. Gallen, SwitzerlandReviewed by:
Imran Imran, Bahauddin Zakariya University, PakistanMaryam Ardalan, University of Gothenburg, Sweden
Copyright © 2022 Wang, Yang, Yang, Chen, Ren, Wang, Jiao, Ding, Yang, Sun and Ma. This is an open-access article distributed under the terms of the Creative Commons Attribution License (CC BY). The use, distribution or reproduction in other forums is permitted, provided the original author(s) and the copyright owner(s) are credited and that the original publication in this journal is cited, in accordance with accepted academic practice. No use, distribution or reproduction is permitted which does not comply with these terms.
*Correspondence: Peng Wang, cGVuZ3dhbmdAbnhtdS5lZHUuY24=; Huisheng Ma, bWFkb2N0b3JAMTYzLmNvbQ==