- Department of Rehabilitation Medicine, Tongji Hospital, Tongji Medical College, Huazhong University of Science and Technology, Wuhan, China
Excitatory projection neurons and inhibitory interneurons primarily accomplish the neural activity of the cerebral cortex, and an imbalance of excitatory-inhibitory neural networks may lead to neuropsychiatric diseases. Gamma-aminobutyric acid (GABA)ergic interneurons mediate inhibition, and the embryonic medial ganglionic eminence (MGE) is a source of GABAergic interneurons. After transplantation, MGE cells migrate to different brain regions, differentiate into multiple subtypes of GABAergic interneurons, integrate into host neural circuits, enhance synaptic inhibition, and have tremendous application value in diseases associated with interneuron disorders. In the current review, we describe the fate of MGE cells derived into specific interneurons and the related diseases caused by interneuron loss or dysfunction and explore the potential of MGE cell transplantation as a cell-based therapy for a variety of interneuron disorder-related diseases, such as epilepsy, schizophrenia, autism spectrum disorder, and Alzheimer’s disease.
Introduction
Located in the ventral telencephalon of the embryo, the medial ganglionic eminence (MGE), the primordium of the globus pallidus, is a vital source of gamma-aminobutyric acid (GABA)ergic interneuron progenitors (Qi et al., 2018). During embryonic development, these cells migrate tangentially to the cortex, striatum, and hippocampal region of the brain to form inhibitory circuits (Marín and Rubenstein, 2001). The normal function of the cerebral cortex, including the neocortex, and hippocampus, requires a neural network consisting of glutamate projection neurons and GABAergic interneurons (Xu et al., 2004; Wonders and Anderson, 2006). GABAergic interneurons form inhibitory synapses in one or more parts of the brain and participate in the regulation of the firing patterns of projection neurons in the cerebral cortex. The balance between excitation and inhibition in the cortical neural circuits is maintained by GABAergic interneurons (Wang et al., 2016). In animals and humans, interneuron loss or malfunctioning can result in mood disorders, working memory disorders, or cognitive decline (Casalia et al., 2021; Williams and Riedemann, 2021), leading to epilepsy (Knopp et al., 2008), Alzheimer’s Disease (AD) (Fu et al., 2017; Najm et al., 2020), schizophrenia (Dienel and Lewis, 2019; Van Derveer et al., 2021), autism spectrum disorder (ASD) (Shin et al., 2021; Deemyad et al., 2022) and other neurological disorders. Researches have revealed that MGE-derived interneuron transplantation offers hope for treating these diseases (De la Cruz et al., 2011; Perez and Lodge, 2013; Upadhya et al., 2019; Lu et al., 2020; Southwell et al., 2020). MGE is a crucial source of inhibitory interneurons during development. The transplanted MGE-derived cells survive and migrate in the cerebral cortex, producing parvalbumin (PV), somatostatin (SST/SOM), and other GABAergic interneuron subtypes, which are integrated into the host circuit, increase inhibitory tension, and regulate neural excitability (Zipancic et al., 2010). Therefore, MGE cells, an excellent source of GABAergic interneurons, play a vital role in the treatment of interneuron disorder-related diseases. In the current review, we discuss the link between interneuron loss or dysfunction and disorders such as epilepsy, schizophrenia, ASD, and AD, and the potential of MGE cells to treat these diseases. The aim is to guide the subsequent transfer of MGE cell transplantation from animal models to clinical application.
Medial Ganglionic Eminence-Derived Interneurons
The ganglionic eminence (GE) is a temporary brain structure that appears during the middle of embryonic development and is the core region of the ventral brain. The GE includes the MGE, lateral ganglionic eminence (LGE), and caudal ganglionic eminence (CGE). In rodents, the GE gives rise to various neuron types, including projective neurons and interneurons, which distribute widely in the brain (Bandler et al., 2017). The MGE can produce GABAergic interneurons migrating to the striatum and cerebral cortex, GABAergic projection neurons migrating to the globus pallidus, and cholinergic neurons remaining in the basal telencephalon (Marín and Rubenstein, 2001). However, the LGE produces striatal medium spiny neurons and olfactory bulb interneurons. The CGE produces GABAergic projection neurons in the amygdala and other nuclei of the limbic system, and multiple GABAergic interneurons in the cerebral cortex (Wichterle et al., 1999; Eriksson et al., 2003; Zhao et al., 2003; Shi et al., 2021). Most cortical and hippocampal interneurons in mammals are derived from three different regions in the basal telencephalon: the MGE, CGE, and preoptic area (Christodoulou et al., 2021). Approximately 60% of cortical interneurons are derived from the MGE, 30% from the CGE, and the remaining 10% from the preoptic area (Williams and Riedemann, 2021). Inhibitory interneurons play a dominant role in maintaining the functional balance of neural circuits (as elaborated in the next section). Thus, MGE, which provides the most GABAergic interneurons, is critical for nervous system development.
The MGE migrates tangentially from the subpallium to the pallium, producing different GABAergic interneurons in the hippocampus and striatum (Chang et al., 2020). Based on their neurochemical expression profile, GABAergic interneurons can be divided into three categories: PV-positive (PV+), SOM-positive (SOM+)/SST-positive (SST+), and 5-hydroxytryptamine 3A receptor-positive interneurons (Lee et al., 2010; Rudy et al., 2011; Wamsley and Fishell, 2017). The MGE primarily produces cortical interneurons that express PV and SST (Wonders and Anderson, 2006; Bandler et al., 2017; Asgarian et al., 2019). The expression of the transcription factor NKX2.1, in the MGE, is required to specify cortical interneurons expressing PV or SST (Du et al., 2008). Mice with NKX2.1 deletion cannot produce normal MGE tissues (Sussel et al., 1999), or cortical interneurons expressing PV or SST (Xu et al., 2004). PV+ and SST+ interneurons are major contributors to oscillatory activity in the early postnatal hippocampus (Akgul and McBain, 2020). They play an important role in memory function and their defects can lead to related diseases, as described below.
Interneuron Dysfunction Leads to Related Diseases
The neural network in the vertebrate brain is composed of excitatory neurons and inhibitory interneurons. Although excitatory neurons account for most neural circuits, they only generate a large amount of excitement and cannot perform effective computations. Therefore, the primary inhibitory interneurons play a dominant role in regulating neuronal discharge and maintaining the functional balance of the neural circuits (DeFelipe et al., 2013; Wamsley and Fishell, 2017). Furthermore, interneurons coordinate nervous system activity by producing and stabilizing network oscillations (Benes and Berretta, 2001; Huang et al., 2007). PV+ interneurons rapidly spike and target the initial segment of axons and cell bodies to regulate the discharge of pyramidal cells; interneurons containing SST exhibit slow-firing patterns and synaptic formation in the distal dendrites of pyramidal cells (Perez et al., 2019). Hippocampal PV+ interneurons play a crucial role in generating and maintaining network oscillations associated with learning and episodic memory (Cardin et al., 2009; Sohal et al., 2009). The coordination of these neurons facilitates the synchronization of the activity of complex networks of neurons, which is crucial for maintaining brain function (Benes and Berretta, 2001). The primary task of the interneuron is to provide inhibitory GABAergic synaptic input, a physiological effect of intracellular chloride influx or potassium outflow via homologous GABAA or GABAB receptor activation, respectively, to shunt the membrane away from the action potential threshold and enable instantaneous hyperpolarization (Pelkey et al., 2017). Dysfunction of GABAergic signal transduction, developmental defects, and loss of GABAergic interneurons are related to neurodevelopmental disorders.
Knopp et al. (2008) observed a significant loss of PV− and calmodulin-immunoreactive neurons in pilocarpine-treated mice, resulting in impaired GABAergic inhibition and temporal lobe epilepsy. In mice and patients with AD, tau protein significantly accumulates in GABAergic neurons in the dentate gyrus (DG) of the hippocampus, which inhibits GABAergic signal transmission, impairs hippocampal neurogenesis, and aggravates cognitive impairment in AD (Zheng et al., 2020). Patients and animal models of schizophrenia (Lodge et al., 2009; Kaar et al., 2019) and ASD (Ajram et al., 2017; Paterno et al., 2021) have impaired cortical or hippocampal interneurons and reduced GABAergic inhibition. For example, in patients with schizophrenia, PV expression is reduced in the cortical region, PV and SST expression is reduced in the hippocampus as well (Konradi et al., 2011; Wang et al., 2011; Gonzalez-Burgos et al., 2015). The mechanism by which interneuron dysfunction contributes to these disorders remains unclear. However, interneuron dysfunction, such as GABAergic interneurons, has been linked to several neurological diseases including epilepsy, schizophrenia, ASD, and AD. Supplementing these cells with transplantation strategies is a feasible and effective method to treat related diseases. Since interneuron development is conserved between humans and mice, studying murine interneurons is of interest to understand human biology and disease.
The Medial Ganglionic Eminence Cell Is a Reliable Source of Cell Therapy
Medial ganglionic eminence cells can survive transplantation into the host, even in harsh environments (Tong et al., 2014; Lu et al., 2020). The study revealed that 1 year after transplantation, the cell survival rate was approximately 20% (Zipancic et al., 2010). Moreover, MGE cells have a strong migration potential. When transplanted into the postnatal brain, embryonic cells from the LGE and dorsal forebrain mostly cluster at the injection site. MGE-derived interneurons are widely dispersed across developmental regions of the central nervous system (CNS), including the striatum, hippocampus, neocortex, amygdala, thalamus, and spinal cord in adults and neonates (Southwell et al., 2014). MGE cells can migrate over long distances within the brain parenchyma, integrate into the host tissue, and differentiate into neurons, including GABAergic neurons (Wichterle et al., 1999). When embryonic MGE cells are transplanted into the neocortex of postnatal mice, MGE cells still follow their inherent migration routes (tangential and radial) and cell fate, survive and integrate into their host circuits (Alvarez-Dolado et al., 2006).
The transplanted MGE-derived cells differentiate into mature cortical interneurons, most of which express GABA, including SST, neuropeptide Y (NPY), PV, and calmodulin subtypes (Alvarez-Dolado et al., 2006). Transplanted MGE cells can modify neural circuits and selectively enhance local inhibition (Alvarez-Dolado et al., 2006). MGE-derived interneurons retain the ability to disperse and integrate functions, receive excitatory and inhibitory inputs, and form inhibitory synapses with host neurons when transplanted into adolescent and adult brains (Wichterle et al., 1999). Thus, MGE cells can replace lost cells and rebuild damaged neural network connections. MGE-derived cell transplantation alters synaptic inhibition and offers a unique opportunity to treat disorders associated with interneuron dysfunction or circuit over-excitation.
Compared to other stem cells, the advantages of MGE cells are as follows: (1) in the adult brain, some neural stem cells are limited in their ability to migrate (Gioia et al., 2020), while MGE cells can migrate widely after transplantation (Tong et al., 2014; Perez et al., 2019; Upadhya et al., 2019); (2) MGE cell transplantation is relatively safe, and teratoma formation has not been observed in related studies of MGE cell transplantation (De la Cruz et al., 2011; Upadhya et al., 2019), while pluripotent stem cells (PSCs) have the risk of tumor formation (Sugaya and Vaidya, 2018); (3) more importantly, most cortical interneurons are generated in the MGE region during development. MGE cells were transplanted into the brain and differentiated into interneurons, expressing GABA and specific interneuron markers, such as PV, SST, NPY, and calretinin (CR). Their ratios were similar to those typically produced by the MGE during development and conform to their intrinsically defined differentiation program (Rodriguez-Martinez et al., 2017). All of these properties make MGE cells the most promising neuronal progenitor cells for cell-based therapies for interneuron disorder-related diseases.
Potential of Medial Ganglionic Eminence Cell Transplantation for Interneuron Disorder-Related Diseases
Epilepsy
Epilepsy is a chronic disease in which neurons in the brain fire abnormally, resulting in temporary brain dysfunction. Many studies have revealed that the loss or dysfunction of inhibitory interneurons in the cortex and hippocampus leads to persistent hyperexcitability of neural circuits, resulting in epilepsy (During et al., 1995; Arellano et al., 2004; Knopp et al., 2008). Current anti-epileptic drugs primarily target glutamate receptors or GABA receptors, thereby inhibiting seizures. However, long-term drug therapy may produce severe side effects, and some patients still develop drug resistance (Ghosh et al., 2021). Approximately 30–40% of people with epilepsy are resistant to traditional anti-epileptic drugs (Li et al., 2022). Some of these patients might require surgery to remove epileptic lesions, but even after surgery, some patients have recurrent seizures and a range of neurological deficits (Sinha et al., 2021). Therefore, a safe and effective therapeutic strategy must be developed. In the CNS, GABA-mediated inhibition is crucial for controlling synaptic circuits and inhibiting neuronal circuitry overexcitation, preventing the spread of epileptic discharges (Freund and Buzsáki, 1996). Several subpopulations of interneurons, including dendritic cells expressing PV (Gallo et al., 2020), basket cells expressing SST (Baraban, 1998), and NPY (Baraban, 1998) have been associated with the suppression of seizures. Therefore, inhibitory interneuron transplantation is a promising therapeutic option for epilepsy.
The Efficiency of Medial Ganglionic Eminence Cell Transplantation as a Prophylactic Treatment
Initial investigations on the efficacy of MGE progenitor cells for alleviating seizures were mostly prophylactic. Before induction of an animal model of epilepsy, MGE cells were transplanted into the cerebral cortex, hippocampus, and other regions. In these studies, spontaneous seizures were induced by maximal electroshock (Calcagnotto et al., 2010), local injection of pentylenetetrazol (Zipancic et al., 2010), or 4-aminopyridine (4-AP) (De la Cruz et al., 2011). Shetty and Upadhya (2016) reviewed partially relevant studies before 2011, which are not described here. In a subsequent study, cells from the MGE region of embryonic day (E) 13.5 mice were injected into the sensorimotor cortex of adult CD1 mice aged 6–8 weeks (De la Cruz et al., 2011, Table 1). Acute focal epileptic discharge was induced by injecting 4-AP, 2 mm from the graft site at 1, 2.5, and 6–8 weeks after cell transplantation. One day after transplantation, De la Cruz et al. (2011) observed that MGE-derived progenitor cells into layers 2–6 of the mouse cortex, and a lot of cells near the injection site displayed bipolar morphology typical of migrating interneurons. Immunofluorescence detected the expression of several markers of cortical interneuron 2 months after transplantation. MGE cells significantly reduced the 4-AP-induced discharge power 2.5 or 6–8 weeks after transplantation compared to the dead MGE cells of the control group. This study demonstrated that MGE cell transplantation alleviated seizures independent of the number of transplanted cells. Additionally, 1 day after transplantation dual immunofluorescence for green fluorescent protein (GFP) and Ki-67 was rare, indicating that only a small proportion of MGE cells were still undergoing cell division in the adult brain 24 h after transplantation. No tumors or cysts were observed in this study by De la Cruz et al. (2011). Prophylactic studies have shown preliminary promise for treating epilepsy using MGE cells.
The Influence of Medial Ganglionic Eminence Cell Transplantation After the Occurrence of Epilepsy
Studies have revealed that injecting MGE cells into the brains of epileptic animal models, such as voltage-gated potassium channel 1.1 -/- animal (Baraban et al., 2009) and Stargazer mouse model of absence epilepsy (Hammad et al., 2015), significantly reduced the number of seizures and the mortality of epilepsy models. Transplantation of MGE cells is a potential clinical treatment for epilepsy.
New progress has been made in the treatment of epilepsy using MGE cells. One study (Romariz et al., 2017, Table 1) compared the anticonvulsant effects and cell differentiation patterns of neurosphere−grown MGE cells and freshly isolated MGE cells. MGE region of E14.5 Sprague Dawley rats was dissected to harvest cells. Cells were transplanted into the hippocampus 7–10 days after the development of status epilepticus (SE). Transplantation of fresh MGE cells and neurosphere−cultivated MGE cells in the presence of growth factors reduced the number of seizures. Their mechanisms are slightly different: fresh MGE cells preferentially differentiate into inhibitory neurons to regulate neural activity and alleviate seizures, while MGE cells cultivated as neurospheres under growth factor conditions may modulate the frequency of epileptic seizures through glia-related mechanisms, such as secretion of nutrient factors and regulation of inflammation. However, transplantation of fresh MGE progenitor cells exhibited more potent anticonvulsant effects than transplantation of neurosphere−grown MGE cells. The possible reasons may be as follows: (1) fresh MGE cells have a regional molecular memory and can differentiate into inhibitory interneurons in vivo after transplantation; (2) culture medium molecules influence the fate and differentiation of MGE cells as nerve spheres. Therefore, freshly obtained MGE cells may be more suitable for transplantation. Another possible structural mechanism for the anti-epileptic effect of MGE cells is increased GABAergic innervation by transplanted MGE progenitor cells, which may limit dendritic growth in adult hippocampal dentate granule cells (Gupta et al., 2019, Table 1). MGE progenitor cells from E13.5 GFP mice were transplanted into the hippocampus of mice 2 months after SE (Casalia et al., 2017, Table 1). At 210 days after transplantation, the grafts differentiated into multiple GABAergic interneuron subtypes, including PV+ and SST+ interneuron. Similar expression patterns were still detected 12 months after transplantation, confirming the long-term integration of MGE-derived interneurons in the mouse brain.
Fetal MGE cells have a potential positive value in epilepsy applications. Nevertheless, to a certain extent, ethical issues and xenograft rejection limit its transformation into the clinic. A more appropriate method is needed, and human-induced PSC (hiPSC)-derived MGE neural progenitor cells are an excellent choice. A 2019 study explored this option (Upadhya et al., 2019, Table 1). MGE-like neural progenitor cells derived from hiPSCs were transplanted into the hippocampus of rats 7 days after SE. Histological results showed that the transplanted cells survived well, and most differentiated into GABAergic interneurons (76%), including PV+ (27%) and NPY-positive (NPY+) (11%) interneuron subtypes. More importantly, synapses formed between graft-derived axons and excitatory neurons of the host. Continuous video electroencephalograph (EEG) recordings showed that the frequency and severity of spontaneous recurrent seizures in rats receiving bilateral hiPSC-MGE cell transplantation were significantly reduced. In addition, MGE cell transplantation can improve cognitive and pattern separation dysfunction in epileptic rats to a certain extent. MGE cells were transplanted into the hippocampus, migrated to the cell layer of the hippocampus, including DG, CA1 subfield, and CA3 subfield, and differentiated into GABAergic interneurons, which then enhanced functional synaptic inhibition, decreased nerve excitability, and reduced abnormal mossy fiber germination and abnormal neurogenesis in the DG, which provided evidence for the amelioration of behavioral defects in epileptic rats. Excitingly, no teratoma formation was observed 5 months after transplantation.
As an essential source of GABAergic interneurons in the cerebral cortex and hippocampus, MGE cells are ideal for cell transplantation. The transplanted MGE cells not only differentiate into GABAergic interneurons but also form inhibitory synapses and increase the transmission of GABAergic synapses to adjacent pyramidal cells (Alvarez-Dolado et al., 2006). Cell replacement therapy restores the excitatory-inhibitory imbalance, which may be central to treating many forms of epilepsy. Over the past decade, MGE cell transplantation has shown remarkable success in the prevention and treatment of epilepsy.
Schizophrenia
Schizophrenia is an unknown etiology of heterogenous psychosis, which can manifest with personal sensory abnormalities along with emotional and behavioral abnormalities. The core characteristics are positive symptoms (delusions and hallucinations; so-called psychotic symptoms of loss of connection with reality), negative symptoms (impaired motivation, spontaneous speech loss, and social withdrawal), and further cognitive impairment (Jauhar et al., 2022). The exact pathophysiological mechanism has not yet been elucidated, and the dopamine hypothesis (that the mesolimbic dopamine system is overactive) is widely supported (Jauhar et al., 2022). However, there is no direct evidence of primary changes in dopamine neurons (Harrison, 1999). The main antipsychotic drugs, which function by blocking dopamine receptors, reduce symptoms and cause severe side effects, such as Parkinson’s extrapyramidal symptoms and tardive dyskinesia (Jauhar et al., 2022). Several other treatments, including psychotherapy, cognitive-behavioral therapy, and electric shocks, have been unsuccessful. It has been hypothesized that GABA dysfunction plays a central role in the pathophysiology of schizophrenia (Roberts, 1972). Selective deletions of PV and SST expression in the hippocampus and cortex have been found in post-mortem brain tissue (Perez et al., 2019). PV+ and SST+ interneurons are essential for the stability of neural network activity and the coordination of brain oscillations (Medrano-Fernandez et al., 2019). In particular, PV+ interneurons play an important role in the inhibitory control of pyramidal cell activity (Lodge et al., 2009). Hyperactivity in the resting hippocampus has been observed in patients and rodent models with schizophrenia (Lodge and Grace, 2007; Schobel et al., 2009). Symptoms associated with schizophrenia are caused by abnormalities in the function of the downstream dopaminergic system when hippocampal activity increases (Donegan et al., 2017). Therefore, restoring GABAergic inhibitory interneurons may be a promising strategy for the treatment of schizophrenia.
In a study conducted in 2013, cells from the MGE region of the gestational day 14.5 GFP-positive (GFP+) transgenic rats were transplanted into the hippocampus of methylazoxymethanol acetate (MAM)-treated rats (40–45 days old) (Perez and Lodge, 2013, Table 2). Two months after transplantation, the cells migrated to the entire hippocampus and the adjacent areas. Immunostaining showed that 18.1% of GFP+ cells were PV+. Normalization of the enhanced ventral hippocampus discharge rate of pyramidal neurons was observed in MAM-treated rats after MGE cell transplantation. Furthermore, the improved motor response to low amphetamine doses was reversed after transplantation. This study demonstrated that MGE cells, transplanted into the ventral hippocampus in MAM-treated rats, normalized abnormal pyramidal neuronal activity and downstream dopamine system function and had a potential therapeutic effect on the positive symptoms of schizophrenia. A subsequent study transplanted cells from the MGE region of E15.5 mice into the hippocampus of adult cyclin dopamine receptor 2 knockout (Ccnd2 KO) mice (6–8 weeks old) and yielded equivalent results (Gilani et al., 2014, Table 2). Immunolabeling indicated that 97% of the GFP+ cells were GABAergic, 56% expressed PV, and 35% expressed SST. In addition to normalizing hippocampal metabolic activity and dopaminergic neural activity, such transplantation also improves cognitive function in mice. A recent study of the lysophosphatidic acid receptor 1 deficient mice led to hippocampal and behavioral abnormalities that mimic the core features observed in common neuropsychiatric disorders (Rosell-Valle et al., 2021). MGE cell transplantation improved behavioral defects in mice and compensated for the loss of interneurons.
These studies illustrate the potential value of MGE cells, which produce many GABAergic interneurons, for the treatment of schizophrenia. In the future, we can further explore the exact mechanism of MGE cell transplantation in the treatment of schizophrenia, including whether the secretion of neurotrophic factors and hippocampal neurogenesis is involved.
Autism Spectrum Disorder
Autism spectrum disorder is an increasingly common neurodevelopmental disorder characterized by problems with social interaction, communication, and repetitive and stereotypical behaviors (Bremer et al., 2016). The etiology is still not fully understood, and it is generally believed that it may be related to genetics, infection, immunity, and physicochemical factors during pregnancy (Mandy and Lai, 2016; Martinez-Cerdeno et al., 2016). Currently, there is no specific drug for the core symptoms of ASD, only for symptomatic treatment (Ajram et al., 2017). An imbalance between excitation and inhibition may be the neurobiological basis of ASD (Bozzi et al., 2018). There is evidence that the disruption of GABAergic signaling may be the underlying mechanism leading to the development of autism (LeBlanc and Fagiolini, 2011). Studies in several mouse models of ASD and patients with ASD further support the hypothesis of GABAergic interneuron disorders, particularly PV+ interneuron loss (Ajram et al., 2017; Hashemi et al., 2017; Vogt et al., 2018; Di et al., 2020; Paterno et al., 2021). PV+ interneurons are fast spike cells that synchronize the activity of pyramidal cells through peri-somatic and axo-axonic inhibition. Reduced numbers of PV+ interneurons may disrupt the excitatory-inhibitory balance and alter gamma wave oscillations in the cortex of autistic patients (Hashemi et al., 2017). Therefore, restoring the interneurons in specific areas may be an effective therapeutic approach.
A previous study of embryonic stem cell (ESC)-derived interneurons transplanted into GABA-deficient ASD models demonstrated that PV+ interneuron transplantation reduced pyramidal neuron firing in the prefrontal cortex (PFC) and ameliorated deficits in social interaction and cognitive flexibility (Donegan et al., 2018). This study suggested that enhanced GABA inhibition was associated with improved behavioral deficits in ASD. Does interneuron transplantation also improve the related behavioral deficits in mouse models of ASD with excessive synaptic inhibition? Phosphatase Pten mutant mice exhibited deficits in social behavior, increased synaptic inhibition in the PFC, altered composition of cortical interneuron subtypes, and abnormal EEG baseline (Vogt et al., 2015; Southwell et al., 2020). MGE cells from E13.5 mice expressing GFP were injected into the bilateral PFC of neonatal Pten conditional knock-out (cKO) recipient mice in research by Southwell et al. (2020, Table 3). Seven weeks after transplantation, the behavioral analysis revealed a significant increase in social activity in mice, while patch-clamp recordings indicated increased spontaneous inhibitory postsynaptic current frequency in pyramid-based neurons. Eight weeks after transplantation, MGE-derived interneurons expressed PV, SST, and Reelin markers. The following results were observed: (1) MGE cell therapy could salvage the behavioral deficits of ASD even in the context of over-inhibition rather than a lack of inhibition; (2) MGE cell therapy could improve behavioral deficits without normalizing synaptic inhibition levels or EEG baseline. Therefore, transplantation may rescue autistic behaviors not only by simply normalizing pathological neural circuits but also by inducing new physiological states to produce therapeutic effects, which requires further exploration.
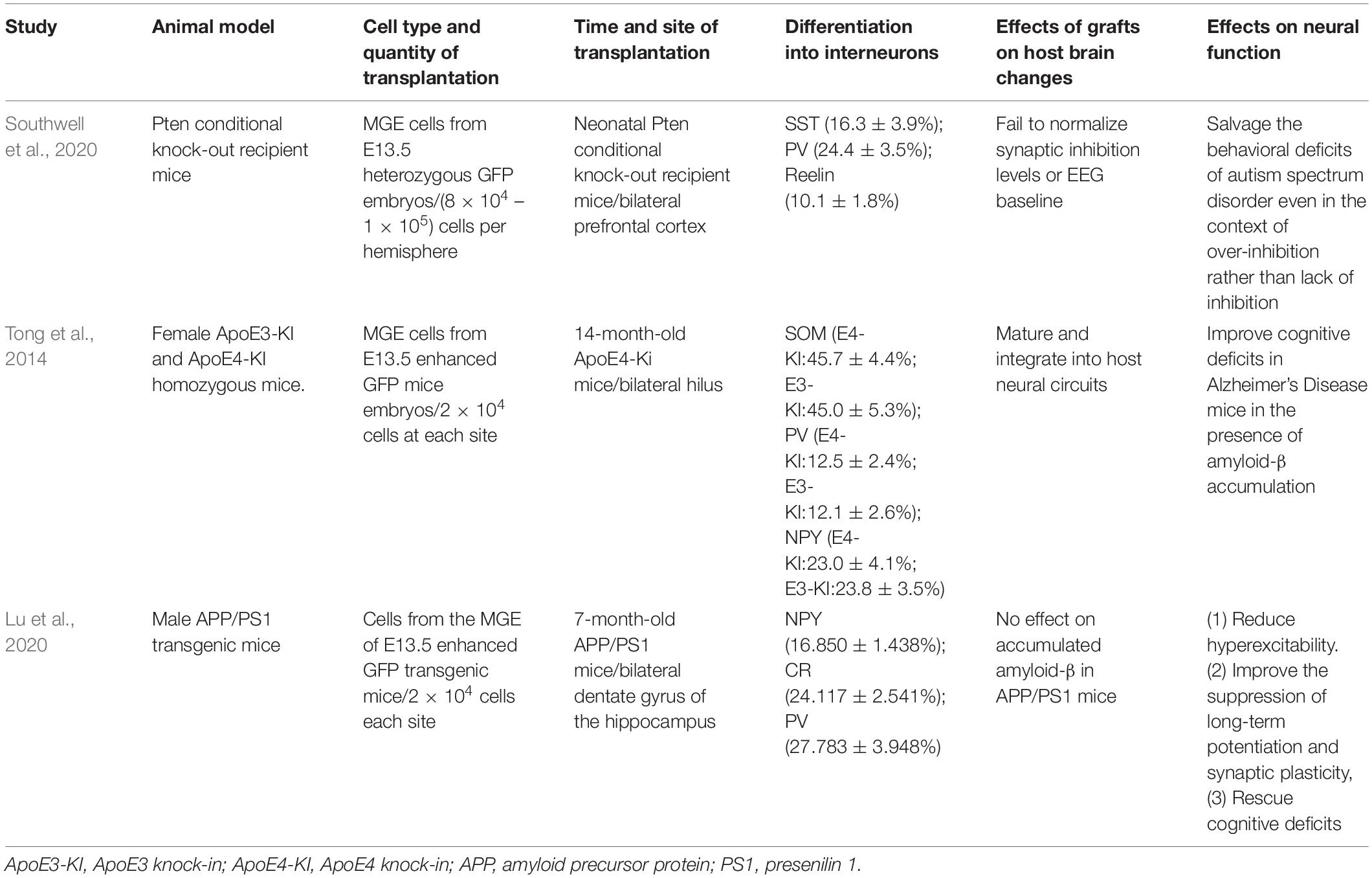
Table 3. Medial ganglionic eminence cell transplantation for autism spectrum disorder and Alzheimer’s Disease.
Alzheimer’s Disease
The balance between excitatory and inhibitory neural networks is essential for maintaining normal brain function, especially cognitive function (Vico Varela et al., 2019). An excitatory-inhibitory imbalance can lead to neurological disorders such as AD. AD is a central neurodegenerative disease characterized by progressive cognitive dysfunction, and its pathogenesis is complex. The amyloid precursor protein (APP) and presenilin (PS) 1 or PS2 are thought to be involved in the development of early onset AD. They promote the aggregation and accumulation of amyloid-β (Aβ) in the brain, and the accumulation of oligomeric Aβ leads to tau hyperphosphorylation (Huang and Mucke, 2012). The loss of GABAergic neurons in mice with early Aβ deposition begins at 6 months. Additionally, glutamic acid decarboxylase (GAD), SOM, and PV are significantly reduced in the hippocampus of mice with high expression of human tau protein. Therefore, the pathological markers of AD may be related to impaired GABA signal transmission (Jimenez-Balado and Eich, 2021). Apolipoprotein E4 (ApoE4) may induce GABAergic interneuron injury through both Aβ-dependent and -independent effects, resulting in reduced neurogenesis in the hippocampus and impaired learning and memory (Huang and Mucke, 2012). The loss of GABA may be the trigger factor leading to the over-excitation of neural network activity in AD, and over-excitation of the neural network is one of the reasons leading to cognitive impairment in AD patients. AD is difficult to be identified in an early stage. Most cases are identified in the middle and late stages of development. Therefore, the treatment of middle- and late-stage AD is critical. However, therapies targeting specific AD-related pathways have failed in human trials of advanced AD (Huang and Mucke, 2012). Patients with advanced AD are no longer treated with existing drugs, due to the loss of inhibitory interneurons in the hippocampus. Inhibition of hyperexcitability has been shown to ameliorate cognitive deficits in AD (Haberman et al., 2017). Cortical GABAergic interneurons are produced in embryonic MGE. MGE cells can survive, migrate, and differentiate into a variety of inhibitory interneurons when transplanted into rodent hippocampi. MGE cells are widely used in the study of neurological diseases, such as epilepsy (Casalia et al., 2017; Romariz et al., 2017; Backofen-Wehrhahn et al., 2018; Gupta et al., 2019; Upadhya et al., 2019), and schizophrenia (Perez and Lodge, 2013; Gilani et al., 2014). Therefore, transplanting MGE neural progenitor cells into the hippocampus of patients with AD may offer new insights.
The hippocampal hilus of 14-month-old female ApoE4 knock-in mice was injected with a suspension of fresh cells from the MGE region of E13.5 mice (Tong et al., 2014, Table 3). The transplanted cells migrated throughout the hilus, with the neurites extending to the molecular layer of the DG, survived (>3 months), and differentiated into mature GABA-inhibitory interneurons (96.1 ± 2.1%), including SOM+, NPY+, and PV+ interneurons. MGE cell transplantation increased the frequency of spontaneous inhibitory currents and increased inhibitory charge transfer in the dentate granule neurons. Electrophysiology verified that MGE-derived GABAergic interneurons were functionally integrated into the host hippocampal circuitry. MGE cell transplantation also improved cognitive deficits in AD mice in the presence of Aβ accumulation. In a 2020 study, similar changes were observed in the bilateral hippocampal DG of 7-month-old APP/PS1 mice transplanted with MGE cells from E13.5 enhanced GFP transgenic mice (Lu et al., 2020, Table 3). APP/PSL mice, a mature and widely used AD model, overexpress the human APP and PS1, leading to elevated levels of Aβ42 (Hollnagel et al., 2019). APP/PS1 mice showed reduced hippocampal CR positive (CR+) and NPY+ interneurons at both 7 and 12 months, and reduced PV+ interneurons at 12 months (Lu et al., 2020, Table 3). These results indicate an age-dependent loss of GABAergic interneurons associated with AD pathology and cognitive deficits. On the 30 days after transplantation, the transplanted cells have migrated to the DG subregion, and a minority of cells have migrated outside the DG subregion. On the 60 days after transplantation, MGE cells showed good migration in the hippocampus and differentiated into GABA interneurons and their subtypes, including NPY+, CR+, and PV+ interneurons. EEG monitoring suggested that MGE cell transplantation reduced hyperexcitability in the APP/PS1 transgenic mice. Additionally, improved learning and memory function in APP/PS1 mice were further verified by behavioral tests. MGE cell transplantation also improved long-term potentiation and synaptic plasticity. However, no effect on Aβ was observed in these two studies by Tong et al. (2014) and Lu et al. (2020), which may be related to the time point of cell transplantation. In conclusion, the above studies indicate that MGE cells can still differentiate into neurons and restore the normal cognitive function of AD even in harsh environments, which has immense potential in treating AD. The mechanism may be related to the excitatory-inhibitory regulation of neural network activity, but whether other mechanisms are involved requires further investigation.
Conclusion
Medial ganglionic eminence cells are a valuable source for the treatment of various neurological diseases. In addition to these diseases mentioned above, MGE cell transplantation can improve memory impairment after traumatic brain injury (Zhu et al., 2019), neuropathic pain after spinal cord injury (Fandel et al., 2016), and motor function in rats with Parkinson’s disease (Martínez-Cerdeño et al., 2010). MGE cells are a viable potential treatment for diseases associated with interneuron disorders based on the following points: (1) MGE cells are transplanted into the host brain for long-distance migration and differentiation into mature interneurons; (2) interneurons derived from transplanted cells establish synaptic connections with the host neurons and selectively modify neural circuits to enhance inhibition; (3) MGE cell therapy can reduce mortality in animals with partial genetic defects; (4) MGE cell therapy improves cognitive and mood disorders.
Genetic variation is an important factor that leads to the occurrence of diseases associated with interneuron disorders. With an in-depth understanding of the pathogenesis of diseases, other cutting-edge approaches, including gene therapy and re-programming, have emerged. This provides a new direction for the treatment of CNS diseases by upregulating or knocking down specific pathogenic genes or inducing human non-neuron cells to transform into neurons to replace damaged neurons. Compared with them, the advantages of MGE cell transplantation are as follows: (1) safety: cells are directly injected into the target area for cell transplantation without vectors; however, gene therapy or re-programming may require the use of viral vectors, which may cause the body to initiate an immune response, activate macrophages (Zhang and Wang, 2021), and there is a risk of off-target (Pattanayak et al., 2013; Turner et al., 2021); (2) influence of intracerebral environment on differentiation pattern: MGE cells are transplanted to the specific damaged brain area, follow their inherent migration routes and cell fate, differentiate into interneurons, form functional synapses, and reconstruct neural circuits (Zipancic et al., 2010); the efficiency and subtype specifications of in vivo re-programming may be influenced by environmental signals after brain injury (Vignoles et al., 2019); (3) stable internal environment: Grafts secrete neuroprotective factors and release factors that repair and fight inflammation and scars to create a homeostasis environment (Chohan and Moore, 2016).
However, some problems remain to be solved before MGE cell transplantation can be safely used clinically. First, there is the problem of cell origin. Currently, most MGE cells used in animal experiments are derived from the MGE region of fetal mice. Due to ethical problems, it is impossible to obtain MGE cells from human fetuses for clinical application. Therefore, it is important to generate similar MGE cells through directed differentiation of human PSCs (hPSCs) (including hiPSCs and human ESCs). There is no lack of research on the generation of MGE-like neural progenitor cells from hPSCs, which then differentiate into GABAergic interneurons and mature in vitro (Liu et al., 2013; Noakes et al., 2019; Li et al., 2021). Ground-breaking researches have shown that MGE cells derived from hPSCs can be used for the treatment of epilepsy (Upadhya et al., 2019), neuropathic pain (Fandel et al., 2016), and stroke (Li et al., 2021), opening the possibility of developing suitable human therapies. If the efficiency of inducing differentiation can be improved and large-scale amplification of functional MGE progenitor cells can be achieved, this will greatly improve the accessibility of these cells (Ma et al., 2019). In this process, attention must also be paid to the safety of hPSC-derived progenitor cells to avoid the formation of tumors. Second, further studies are needed to explore the underlying mechanisms of interneuron disorder-related diseases, to promote the differentiation of these transplanted cells into appropriate interneuron subtypes and their integration into local circuits for long-term efficacy. Third, transplantation is an invasive intervention, and the choice of transplantation route requires more consideration. Transplantation of stem cells intravenously was thought to reduce invasiveness (Chu et al., 2004). However, most of the stem cells remained in the lungs as they circulated and were cleared by the body (Pendharkar et al., 2010). Even when they reached the brain, only 10% of the cells differentiated into neurons (Chu et al., 2004). Therefore, transplantation of cells intravenously may not be the ideal route for MGE cells. A large number of studies on MGE cell transplantation were performed by intracerebral injection (Calcagnotto et al., 2010; Gupta et al., 2019; Upadhya et al., 2019). Intracerebral injection of MGE cells can directly target the desired graft region, reduce cell loss during long-term migration, and more cells can differentiate into neurons compared to transplantation of cells intravenously. Finally, although intracerebral transplantation can transport cells directly to the target area, it is an invasive intervention and therefore strict use conditions should be set, which may limit its clinical application. In cases of drug resistance, severe drug side effects, recurrent attacks, or ineffective surgical treatment, options can be made after weighing the pro and cons in the future. There is still a long way to go before MGE cell therapy for neuropsychiatric diseases can be translated into clinical practice. However, there are reasons for optimism, given the growing research into the mechanisms of interneuron disorder-related diseases and the rapid development of cell differentiation techniques.
Author Contributions
XH conceived and revised the manuscript. DL designed and drafted the manuscript. QW collected literatures. All authors contributed to the article and approved the submitted version.
Funding
This study was supported by National Natural Science Foundation of China (Grant No. 81774404).
Conflict of Interest
The authors declare that the research was conducted in the absence of any commercial or financial relationships that could be construed as a potential conflict of interest.
Publisher’s Note
All claims expressed in this article are solely those of the authors and do not necessarily represent those of their affiliated organizations, or those of the publisher, the editors and the reviewers. Any product that may be evaluated in this article, or claim that may be made by its manufacturer, is not guaranteed or endorsed by the publisher.
Acknowledgments
We would like to thank Editage (www.editage.cn) for English language editing.
References
Ajram, L. A., Horder, J., Mendez, M. A., Galanopoulos, A., Brennan, L. P., Wichers, R. H., et al. (2017). Shifting brain inhibitory balance and connectivity of the prefrontal cortex of adults with autism spectrum disorder. Transl. Psychiatry 7:e1137. doi: 10.1038/tp.2017.104
Akgul, G., and McBain, C. J. (2020). AMPA receptor deletion in developing MGE-derived hippocampal interneurons causes a redistribution of excitatory synapses and attenuates postnatal network oscillatory activity. Sci. Rep. 10:1333. doi: 10.1038/s41598-020-58068-6
Alvarez-Dolado, M., Calcagnotto, M. E., Karkar, K. M., Southwell, D. G., Jones-Davis, D. M., Estrada, R. C., et al. (2006). Cortical inhibition modified by embryonic neural precursors grafted into the postnatal brain. J. Neurosci. 26, 7380–7389. doi: 10.1523/JNEUROSCI.1540-06.2006
Arellano, J. I., Munoz, A., Ballesteros-Yanez, I., Sola, R. G., and DeFelipe, J. (2004). Histopathology and reorganization of chandelier cells in the human epileptic sclerotic hippocampus. Brain 127, 45–64. doi: 10.1093/brain/awh004
Asgarian, Z., Magno, L., Ktena, N., Harris, K. D., and Kessaris, N. (2019). Hippocampal CA1 somatostatin interneurons originate in the embryonic MGE/POA. Stem Cell Rep. 13, 793–802. doi: 10.1016/j.stemcr.2019.09.008
Backofen-Wehrhahn, B., Gey, L., Broer, S., Petersen, B., Schiff, M., Handreck, A., et al. (2018). Anticonvulsant effects after grafting of rat, porcine, and human mesencephalic neural progenitor cells into the rat subthalamic nucleus. Exp. Neurol. 310, 70–83. doi: 10.1016/j.expneurol.2018.09.004
Bandler, R. C., Mayer, C., and Fishell, G. (2017). Cortical interneuron specification: the juncture of genes, time and geometry. Curr. Opin. Neurobiol. 42, 17–24. doi: 10.1016/j.conb.2016.10.003
Baraban, S. C. (1998). Neuropeptide Y and limbic seizures. Rev. Neurosci. 9, 117–128. doi: 10.1515/REVNEURO.1998.9.2.117
Baraban, S. C., Southwell, D. G., Estrada, R. C., Jones, D. L., Sebe, J. Y., Alfaro-Cervello, C., et al. (2009). Reduction of seizures by transplantation of cortical GABAergic interneuron precursors into Kv11 mutant mice. PNAS 106, 1547–1552. doi: 10.1073/pnas.0900141106
Benes, F. M., and Berretta, S. (2001). GABAergic interneurons: implications for understanding schizophrenia and bipolar disorder. Neuropsychopharmacology 25, 1–27. doi: 10.1016/s0893-133x(01)00225-1
Bozzi, Y., Provenzano, G., and Casarosa, S. (2018). Neurobiological bases of autism-epilepsy comorbidity: a focus on excitation/inhibition imbalance. Eur. J. Neurosci. 47, 534–548. doi: 10.1111/ejn.13595
Bremer, E., Crozier, M., and Lloyd, M. (2016). A systematic review of the behavioural outcomes following exercise interventions for children and youth with autism spectrum disorder. Autism 20, 899–915. doi: 10.1177/1362361315616002
Calcagnotto, M. E., Ruiz, L. P., Blanco, M. M., Santos-Junior, J. G., Valente, M. F., Patti, C., et al. (2010). Effect of neuronal precursor cells derived from medial ganglionic eminence in an acute epileptic seizure model. Epilepsia 51, 71–75. doi: 10.1111/j.1528-1167.2010.02614.x
Cardin, J. A., Carlen, M., Meletis, K., Knoblich, U., Zhang, F., Deisseroth, K., et al. (2009). Driving fast-spiking cells induces gamma rhythm and controls sensory responses. Nature 459, 663–667. doi: 10.1038/nature08002
Casalia, M. L., Howard, M. A., and Baraban, S. C. (2017). Persistent seizure control in epileptic mice transplanted with gamma-aminobutyric acid progenitors. Ann. Neurol. 82, 530–542. doi: 10.1002/ana.25021
Casalia, M. L., Li, T., Ramsay, H., Ross, P. J., Paredes, M. F., and Baraban, S. C. (2021). Interneuron origins in the embryonic porcine medial ganglionic eminence. J. Neurosci. 41, 3105–3119. doi: 10.1523/JNEUROSCI.2738-20.2021
Chang, C. C., Kuo, H. Y., Chen, S. Y., Lin, W. T., Lu, K. M., Saito, T., et al. (2020). Developmental characterization of Zswim5 expression in the progenitor domains and tangential migration pathways of cortical interneurons in the mouse forebrain. J. Comp. Neurol. 528, 2404–2419. doi: 10.1002/cne.24900
Chohan, M. O., and Moore, H. (2016). Interneuron Progenitor Transplantation to Treat CNS Dysfunction. Front. Neural Circ. 10:64. doi: 10.3389/fncir.2016.00064
Christodoulou, O., Maragkos, I., Antonakou, V., and Denaxa, M. (2021). The development of MGE-derived cortical interneurons: an Lhx6 tale. Int. J. Dev. Biol. 66, 43–49. doi: 10.1387/ijdb.210185md
Chu, K., Kim, M., Chae, S.-H., Jeong, S.-W., Kang, K.-S., Jung, K.-H., et al. (2004). Distribution and in situ proliferation patterns of intravenously injected immortalized human neural stem-like cells in rats with focal cerebral ischemia. Neurosci. Res. 50, 459–465. doi: 10.1016/j.neures.2004.08.015
De la Cruz, E., Zhao, M., Guo, L., Ma, H., Anderson, S. A., and Schwartz, T. H. (2011). Interneuron progenitors attenuate the power of acute focal ictal discharges. Neurotherapeutics 8, 763–773. doi: 10.1007/s13311-011-0058-9
Deemyad, T., Puig, S., Papale, A. E., Qi, H., LaRocca, G. M., Aravind, D., et al. (2022). Lateralized decrease of parvalbumin+ cells in the somatosensory cortex of ASD models is correlated with unilateral tactile hypersensitivity. Cereb. Cortex 32, 554–568. doi: 10.1093/cercor/bhab233
DeFelipe, J., Lopez-Cruz, P. L., Benavides-Piccione, R., Bielza, C., Larranaga, P., Anderson, S., et al. (2013). New insights into the classification and nomenclature of cortical GABAergic interneurons. Nat. Rev. Neurosci. 14, 202–216. doi: 10.1038/nrn3444
Di, J., Li, J., O’Hara, B., Alberts, I., Xiong, L., Li, J., et al. (2020). The role of GABAergic neural circuits in the pathogenesis of autism spectrum disorder. Int. J. Dev. Neurosci. 80, 73–85. doi: 10.1002/jdn.10005
Dienel, S. J., and Lewis, D. A. (2019). Alterations in cortical interneurons and cognitive function in schizophrenia. Neurobiol. Dis. 131:104208. doi: 10.1016/j.nbd.2018.06.020
Donegan, J. J., Boley, A. M., and Lodge, D. J. (2018). Embryonic stem cell transplants as a therapeutic strategy in a rodent model of autism. Neuropsychopharmacology 43, 1789–1798. doi: 10.1038/s41386-018-0021-0
Donegan, J. J., Tyson, J. A., Branch, S. Y., Beckstead, M. J., Anderson, S. A., and Lodge, D. J. (2017). Stem cell-derived interneuron transplants as a treatment for schizophrenia: preclinical validation in a rodent model. Mol. Psychiatry 22, 1492–1501. doi: 10.1038/mp.2016.121
Du, T., Xu, Q., Ocbina, P. J., and Anderson, S. A. (2008). NKX2.1 specifies cortical interneuron fate by activating Lhx6. Development 135, 1559–1567. doi: 10.1242/dev.015123
During, M., Ryder, K., and Spencer, D. (1995). Hippocampal GABA transporter function in temporal-lobe epilepsy. Nature 376, 174–177. doi: 10.1038/376174a0
Eriksson, C., Björklund, A., and Wictorin, K. (2003). Neuronal differentiation following transplantation of expanded mouse neurosphere cultures derived from different embryonic forebrain regions. Exp. Neurol. 184, 615–635. doi: 10.1016/s0014-4886(03)00271-1
Fandel, T. M., Alpa Trivedi Nicholas Cory, R., Zhang, H., Chen, J., et al. (2016). Transplanted human stem cell-derived interneuron precursors mitigate mouse bladder dysfunction and central neuropathic pain after spinal cord injury. Cell. Stem Cell 19, 544–557. doi: 10.1016/j.stem.2016.08.020
Freund, T. F., and Buzsáki, G. (1996). Interneurons of the hippocampus. Hippocampus 6, 347–470. doi: 10.1002/(SICI)1098-106319966:4<347::AID-HIPO1<3.0.CO;2-I
Fu, H., Rodriguez, G. A., Herman, M., Emrani, S., Nahmani, E., Barrett, G., et al. (2017). Tau pathology induces excitatory neuron loss, grid cell dysfunction, and spatial memory deficits reminiscent of early Alzheimer’ disease. Neuron 93, 533–541:e5. doi: 10.1016/j.neuron.2016.12.023
Gallo, N. B., Paul, A., and Van Aelst, L. (2020). Shedding light on chandelier cell development, connectivity, and contribution to neural disorders. Trends Neurosci 43, 565–580. doi: 10.1016/j.tins.2020.05.003
Ghosh, S., Sinha, J. K., Khan, T., Devaraju, K. S., Singh, P., Vaibhav, K., et al. (2021). Pharmacological and therapeutic approaches in the treatment of epilepsy. Biomedicines 9:470. 1–14. doi: 10.3390/biomedicines9050470
Gilani, A. I., Chohan, M. O., Inan, M., Schobel, S. A., Chaudhury, N. H., Paskewitz, S., et al. (2014). Interneuron precursor transplants in adult hippocampus reverse psychosis-relevant features in a mouse model of hippocampal disinhibition. Proc. Natl Acad Sci U.S.A. 111, 7450–7455. doi: 10.1073/pnas.1316488111
Gioia, R. D., Biella, F., Nizzardo, M., Citterio, G., Rizzo, F., Abati, E., et al. (2020). Neural stem cell transplantation for neurodegenerative diseases. Int. J. Mol. Sci. 21:3103. doi: 10.3390/ijms21093103
Gonzalez-Burgos, G., Cho, R. Y., and Lewis, D. A. (2015). Alterations in cortical network oscillations and parvalbumin neurons in schizophrenia. Biol. Psychiatry 77, 1031–1040. doi: 10.1016/j.biopsych.2015.03.010
Gupta, J., Bromwich, M., Radell, J., Arshad, M. N., Gonzalez, S., Luikart, B. W., et al. (2019). Restrained dendritic growth of adult-born granule cells innervated by transplanted fetal GABAergic interneurons in mice with temporal lobe epilepsy. eNeuro 6, ENEURO.0110–18. doi: 10.1523/ENEURO.0110-18.2019
Haberman, R. P., Branch, A., and Gallagher, M. (2017). Targeting neural hyperactivity as a treatment to stem progression of late-onset Alzheimer’s Disease. Neurotherapeutics 14, 662–676. doi: 10.1007/s13311-017-0541-z
Hammad, M., Schmidt, S. L., Zhang, X., Bray, R., Frohlich, F., and Ghashghaei, H. T. (2015). Transplantation of GABAergic interneurons into the neonatal primary visual cortex reduces absence seizures in stargazer mice. Cereb. Cortex 25, 2970–2979. doi: 10.1093/cercor/bhu094
Harrison, P. (1999). The neuropathology of schizophrenia. A critical review of the data and their interpretation. Brain 122, 593–624. doi: 10.1093/brain/122.4.593
Hashemi, E., Ariza, J., Rogers, H., Noctor, S. C., and Martinez-Cerdeno, V. (2017). The number of parvalbumin-expressing interneurons is decreased in the prefrontal cortex in autism. Cereb. Cortex 27, 1931–1943. doi: 10.1093/cercor/bhw021
Hollnagel, J. O., Elzoheiry, S., Gorgas, K., Kins, S., Beretta, C. A., Kirsch, J., et al. (2019). Early alterations in hippocampal perisomatic GABAergic synapses and network oscillations in a mouse model of Alzheimer’s disease amyloidosis. PLoS One 14:e0209228. doi: 10.1371/journal.pone.0209228
Huang, Y., and Mucke, L. (2012). Alzheimer mechanisms and therapeutic strategies. Cell 148, 1204–1222. doi: 10.1016/j.cell.2012.02.040
Huang, Z. J., Di Cristo, G., and Ango, F. (2007). Development of GABA innervation in the cerebral and cerebellar cortices. Nat. Rev. Neurosci. 8, 673–686. doi: 10.1038/nrn2188
Jauhar, S., Johnstone, M., and McKenna, P. J. (2022). Schizophrenia. Lancet 399, 473–486. doi: 10.1016/s0140-6736(21)01730-x
Jimenez-Balado, J., and Eich, T. S. (2021). GABAergic dysfunction, neural network hyperactivity and memory impairments in human aging and Alzheimer’s disease. Semin. Cell Dev. Biol. 116, 146–159. doi: 10.1016/j.semcdb.2021.01.005
Kaar, S. J., Angelescu, I., Marques, T. R., and Howes, O. D. (2019). Pre-frontal parvalbumin interneurons in schizophrenia: a meta-analysis of post-mortem studies. J. Neural Transm. (Vienna) 126, 1637–1651. doi: 10.1007/s00702-019-02080-2
Knopp, A., Frahm, C., Fidzinski, P., Witte, O. W., and Behr, J. (2008). Loss of GABAergic neurons in the subiculum and its functional implications in temporal lobe epilepsy. Brain 131, 1516–1527. doi: 10.1093/brain/awn095
Konradi, C., Yang, C. K., Zimmerman, E. I., Lohmann, K. M., Gresch, P., Pantazopoulos, H., et al. (2011). Hippocampal interneurons are abnormal in schizophrenia. Schizophr. Res. 131, 165–173. doi: 10.1016/j.schres.2011.06.007
LeBlanc, J. J., and Fagiolini, M. (2011). Autism: a “critical period” disorder? Neural Plast. 2011:921680. doi: 10.1155/2011/921680
Lee, S., Hjerling-Leffler, J., Zagha, E., Fishell, G., and Rudy, B. (2010). The largest group of superficial neocortical GABAergic interneurons expresses ionotropic serotonin receptors. J. Neurosci. 30, 16796–16808. doi: 10.1523/JNEUROSCI.1869-10.2010
Li, J., Chen, L., Li, D., Lu, M., Huang, X., Han, X., et al. (2021). Electroacupuncture promotes the survival of the grafted human MGE neural progenitors in rats with cerebral ischemia by promoting angiogenesis and inhibiting inflammation. Neural Plast. 2021:4894881. doi: 10.1155/2021/4894881
Li, L., Lu, J., Xu, Y., and Zhao, Y. (2022). Changes in pre– and postsurgery for drug resistant epilepsy: cognition and sleep. Biomed. Res. Int. 2022:9971780. doi: 10.1155/2022/9971780
Liu, Y., Weick, J. P., Liu, H., Krencik, R., Zhang, X., Ma, L., et al. (2013). Medial ganglionic eminence–like cells derived from human embryonic stem cells correct learning and memory deficits. Nat. Biotechnol. 31, 440–447. doi: 10.1038/nbt.2565
Lodge, D. J., Behrens, M. M., and Grace, A. A. (2009). A loss of parvalbumin-containing interneurons is associated with diminished oscillatory activity in an animal model of schizophrenia. J. Neurosci. 29, 2344–2354. doi: 10.1523/JNEUROSCI.5419-08.2009
Lodge, D. J., and Grace, A. A. (2007). Aberrant hippocampal activity underlies the dopamine dysregulation in an animal model of schizophrenia. J. Neurosci. 27, 11424–11430. doi: 10.1523/JNEUROSCI.2847-07.2007
Lu, M.-H., Zhao, X.-Y., Xu, D.-E., Chen, J.-B., Ji, W.-L., Huang, Z.-P., et al. (2020). Transplantation of GABAergic interneuron progenitor attenuates cognitive deficits of Alzheimer’s disease model mice. J. Alzheimers Dis. 75, 245–260. doi: 10.3233/jad-200010
Ma, L., Wang, Y., Hui, Y., Du, Y., Chen, Z., Feng, H., et al. (2019). WNT/NOTCH pathway is essential for the maintenance and expansion of human MGE progenitors. Stem Cell Rep. 12, 934–949. doi: 10.1016/j.stemcr.2019.04.007
Mandy, W., and Lai, M. C. (2016). Annual research review: the role of the environment in the developmental psychopathology of autism spectrum condition. J. Child Psychol. Psychiatry 57, 271–292. doi: 10.1111/jcpp.12501
Marín, O., and Rubenstein, J. L. R. (2001). A long, remarkable journey: tangential migration in the telencephalon. Nat. Rev. Neurosci. 2, 780–790. doi: 10.1038/35097509
Martinez-Cerdeno, V., Camacho, J., Fox, E., Miller, E., Ariza, J., Kienzle, D., et al. (2016). Prenatal exposure to autism-specific maternal autoantibodies alters proliferation of cortical neural precursor cells, enlarges brain, and increases neuronal size in adult animals. Cereb. Cortex 26, 374–383. doi: 10.1093/cercor/bhu291
Martínez-Cerdeño, V., Noctor, S. C., Espinosa, A., Ariza, J., Parker, P., Orasji, S., et al. (2010). Embryonic MGE precursor cells grafted into adult rat striatum integrate and ameliorate motor symptoms in 6-OHDA-lesioned rats. Cell. Stem Cell 6, 238–250. doi: 10.1016/j.stem.2010.01.004
Medrano-Fernandez, A., Delgado-Garcia, J. M., Del Blanco, B., Llinares, M., Sanchez-Campusano, R., Olivares, R., et al. (2019). The epigenetic factor CBP is required for the differentiation and function of medial ganglionic eminence-derived interneurons. Mol. Neurobiol. 56, 4440–4454. doi: 10.1007/s12035-018-1382-4
Najm, R., Rao, A., and Huang, Y. (2020). Too much tau in interneurons impairs adult hippocampal neurogenesis in Alzheimer’s Disease. Cell. Stem Cell 26, 297–299. doi: 10.1016/j.stem.2020.02.004
Noakes, Z., Keefe, F., Tamburini, C., Kelly, C. M., Santos, M. C., Dunnett, S. B., et al. (2019). Human pluripotent stem cell-derived striatal interneurons: differentiation and maturation in vitro and in the rat brain. Stem Cell Rep. 12, 191–200. doi: 10.1016/j.stemcr.2018.12.014
Paterno, R., Marafiga, J. R., Ramsay, H., Li, T., Salvati, K. A., and Baraban, S. C. (2021). Hippocampal gamma and sharp-wave ripple oscillations are altered in a Cntnap2 mouse model of autism spectrum disorder. Cell Rep. 37:109970. doi: 10.1016/j.celrep.2021.109970
Pattanayak, V., Lin, S., Guilinger, J. P., Ma, E., Doudna, J. A., and Liu, D. R. (2013). High-throughput profiling of off-target DNA cleavage reveals RNA-programmed Cas9 nuclease specificity. Nat. Biotechnol. 31, 839–843. doi: 10.1038/nbt.2673
Pelkey, K. A., Chittajallu, R., Craig, M. T., Tricoire, L., Wester, J. C., and McBain, C. J. (2017). Hippocampal GABAergic inhibitory interneurons. Physiol. Rev. 97, 1619–1747. doi: 10.1152/physrev.00007.2017
Pendharkar, A. V., Chua, J. Y., Andres, R. H., Wang, N., Gaeta, X., Wang, H., et al. (2010). Biodistribution of neural stem cells after intravascular therapy for hypoxic–ischemia. Stroke 41, 2064–2070. doi: 10.1161/STROKEAHA.109.575993
Perez, S. M., Boley, A., and Lodge, D. J. (2019). Region specific knockdown of parvalbumin or somatostatin produces neuronal and behavioral deficits consistent with those observed in schizophrenia. Transl. Psychiatry 9:264. doi: 10.1038/s41398-019-0603-6
Perez, S. M., and Lodge, D. J. (2013). Hippocampal interneuron transplants reverse aberrant dopamine system function and behavior in a rodent model of schizophrenia. Mol. Psychiatry 18, 1193–1198. doi: 10.1038/mp.2013.111
Qi, J., Kim, M., Sanchez, R., Ziaee, S. M., Kohtz, J. D., and Koh, S. (2018). Enhanced susceptibility to stress and seizures in GAD65 deficient mice. PLoS One 13:e0191794. doi: 10.1371/journal.pone.0191794
Roberts, E. (1972). A hypothesis suggesting that there is a defect in the GABA system in schizophrenia. Neurosci. Res. Progr. Bull. 10, 468–482.
Rodriguez-Martinez, D., Martinez-Losa, M. A. M., and Alvarez-Dolado, M. (2017). Cryopreservation of GABAergic neuronal precursors for cell-based therapy. PLoS One 12:e0170776. doi: 10.1371/journal.pone.0170776
Romariz, S. A., Paiva, D. S., Galindo, L. T., Barnabe, G. F., Guedes, V. A., Borlongan, C. V., et al. (2017). Medial ganglionic eminence cells freshly obtained or expanded as neurospheres show distinct cellular and molecular properties in reducing epileptic seizures. CNS Neurosci. Ther. 23, 127–134. doi: 10.1111/cns.12650
Rosell-Valle, C., Martinez-Losa, M., Matas-Rico, E., Castilla-Ortega, E., Zambrana-Infantes, E., Gomez-Conde, A. I., et al. (2021). GABAergic deficits in absence of LPA1 receptor, associated anxiety-like and coping behaviors, and amelioration by interneuron precursor transplants into the dorsal hippocampus. Brain Struct. Funct. 226, 1479–1495. doi: 10.1007/s00429-021-02261-4
Rudy, B., Fishell, G., Lee, S., and Hjerling-Leffler, J. (2011). Three groups of interneurons account for nearly 100% of neocortical GABAergic neurons. Dev. Neurobiol. 71, 45–61. doi: 10.1002/dneu.20853
Schobel, S. A., Lewandowski, N. M., Corcoran, C. M., Moore, H., Brown, T., Malaspina, D., et al. (2009). Differential targeting of the CA1 subfield of the hippocampal formation by schizophrenia and related psychotic disorders. Arch. Gen. Psychiatry 66, 938–946. doi: 10.1001/archgenpsychiatry.2009.115
Shetty, A. K., and Upadhya, D. (2016). GABA-ergic cell therapy for epilepsy: advances, limitations and challenges. Neurosci. Biobehav. Rev. 62, 35–47. doi: 10.1016/j.neubiorev.2015.12.014
Shi, Y., Wang, M., Mi, D., Lu, T., Wang, B., Dong, H., et al. (2021). Mouse and human share conserved transcriptional programs for interneuron development. Science 374::eabj6641. doi: 10.1126/science.abj6641
Shin, S., Santi, A., and Huang, S. (2021). Conditional Pten knockout in parvalbumin– or somatostatin-positive neurons sufficiently leads to autism-related behavioral phenotypes. Mol. Brain 14:24. doi: 10.1186/s13041-021-00731-8
Sinha, N., Wang, Y., da Moreira Silva, N., Miserocchi, A., McEvoy, A. W., de Tisi, J., et al. (2021). Structural brain network abnormalities and the probability of seizure recurrence after epilepsy surgery. Neurology 96, e758–e771. doi: 10.1212/WNL.0000000000011315
Sohal, V. S., Zhang, F., Yizhar, O., and Deisseroth, K. (2009). Parvalbumin neurons and gamma rhythms enhance cortical circuit performance. Nature 459, 698–702. doi: 10.1038/nature07991
Southwell, D. G., Nicholas, C. R., Basbaum, A. I., Stryker, M. P., Kriegstein, A. R., Rubenstein, J. L., et al. (2014). Interneurons from embryonic development to cell-based therapy. Science 344:1240622. doi: 10.1126/science.1240622
Southwell, D. G., Seifikar, H., Malik, R., Lavi, K., Vogt, D., Rubenstein, J. L., et al. (2020). Interneuron transplantation rescues social behavior deficits without restoring wild-type physiology in a mouse model of autism with excessive synaptic inhibition. J. Neurosci. 40, 2215–2227. doi: 10.1523/JNEUROSCI.1063-19.2019
Sugaya, K., and Vaidya, M. (2018). Stem Cell Therapies for Neurodegenerative Diseases. Adv. Exp. Med. Biol. 1056, 61–84. doi: 10.1007/978-3-319-74470-4_5
Sussel, L., Marin, O., Kimura, S., and Rubenstein, J. L. R. (1999). Loss of Nkx2.1 homeobox gene function results in a ventral to dorsal molecular respecification within the basal telencephalon evidence for a transformation of the pallidum into the striatum. Development 126, 3359–3370. doi: 10.1242/dev.126.15.3359
Tong, L. M., Djukic, B., Arnold, C., Gillespie, A. K., Yoon, S. Y., Wang, M. M., et al. (2014). Inhibitory interneuron progenitor transplantation restores normal learning and memory in ApoE4 knock-in mice without or with Abeta accumulation. J. Neurosci. 34, 9506–9515. doi: 10.1523/JNEUROSCI.0693-14.2014
Turner, T. J., Zourray, C., Schorge, S., and Lignani, G. (2021). Recent advances in gene therapy for neurodevelopmental disorders with epilepsy. J. Neurochem. 157, 229–262. doi: 10.1111/jnc.15168
Upadhya, D., Hattiangady, B., Castro, O. W., Shuai, B., Kodali, M., Attaluri, S., et al. (2019). Human induced pluripotent stem cell-derived MGE cell grafting after status epilepticus attenuates chronic epilepsy and comorbidities via synaptic integration. Proc. Natl. Acad. Sci. U.S.A. 116, 287–296. doi: 10.1073/pnas.1814185115
Van Derveer, A. B., Bastos, G., Ferrell, A. D., Gallimore, C. G., Greene, M. L., Holmes, J. T., et al. (2021). A role for somatostatin-positive interneurons in neuro-oscillatory and information processing deficits in schizophrenia. Schizophr. Bull. 47, 1385–1398. doi: 10.1093/schbul/sbaa184
Vico Varela, E., Etter, G., and Williams, S. (2019). Excitatory-inhibitory imbalance in Alzheimer’s Disease and therapeutic significance. Neurobiol. Dis. 127, 605–615. doi: 10.1016/j.nbd.2019.04.010
Vignoles, R., Lentini, C., d’Orange, M., and Heinrich, C. (2019). Direct lineage reprogramming for brain repair: breakthroughs and challenges. Trends Mol. Med. 25, 897–914. doi: 10.1016/j.molmed.2019.06.006
Vogt, D., Cho, K. K. A., Lee, A. T., Sohal, V. S., and Rubenstein, J. L. R. (2015). The parvalbumin/somatostatin ratio is increased in Pten mutant mice and by human PTEN ASD alleles. Cell Rep. 11, 944–956. doi: 10.1016/j.celrep.2015.04.019
Vogt, D., Cho, K. K. A., Shelton, S. M., Paul, A., Huang, Z. J., Sohal, V. S., et al. (2018). Mouse Cntnap2 and human CNTNAP2 ASD alleles cell autonomously regulate PV+ cortical interneurons. Cereb. Cortex 28, 3868–3879. doi: 10.1093/cercor/bhx248
Wamsley, B., and Fishell, G. (2017). Genetic and activity-dependent mechanisms underlying interneuron diversity. Nat. Rev. Neurosci. 18, 299–309. doi: 10.1038/nrn.2017.30
Wang, A. Y., Lohmann, K. M., Yang, C. K., Zimmerman, E. I., Pantazopoulos, H., Herring, N., et al. (2011). Bipolar disorder type 1 and schizophrenia are accompanied by decreased density of parvalbumin- and somatostatin-positive interneurons in the parahippocampal region. Acta Neuropathol. 122, 615–626. doi: 10.1007/s00401-011-0881-4
Wang, Q., Hong, P., Gao, H., Chen, Y., Yang, Q., Jiang, M., et al. (2016). An interneuron progenitor maintains neurogenic potential in vivo and differentiates into GABAergic interneurons after transplantation in the postnatal rat brain. Sci. Rep. 6:19003. doi: 10.1038/srep19003
Wichterle, H., Garcia-Verdugo, J. M., Herrera, D. G., and Alvarez-Buylla, A. (1999). Young neurons from medial ganglionic eminence disperse in adult and embryonic brain. Nat. Neurosci. 2, 461–466. doi: 10.1038/8131
Williams, R. H., and Riedemann, T. (2021). Development, diversity, and death of MGE-derived cortical interneurons. Int. J. Mol. Sci. 22:9297. doi: 10.3390/ijms22179297
Wonders, C. P., and Anderson, S. A. (2006). The origin and specification of cortical interneurons. Nat. Rev. Neurosci. 7, 687–696. doi: 10.1038/nrn1954
Xu, Q., Cobos, I., De La Cruz, E., Rubenstein, J. L., and Anderson, S. A. (2004). Origins of cortical interneuron subtypes. J. Neurosci. 24, 2612–2622. doi: 10.1523/JNEUROSCI.5667-03.2004
Zhang, L., and Wang, Y. (2021). Gene therapy in epilepsy. Biomed. Pharmacother. 143:112075. doi: 10.1016/j.biopha.2021.112075
Zhao, Y., Marin, O., Hermesz, E., Powell, A., Flames, N., Palkovits, M. S., et al. (2003). The LIM-homeobox gene Lhx8 is required for the development of many cholinergic neurons in the mouse forebrain. Proc. Natl. Acad. Sci. U.S.A. 100, 9005–9010. doi: 10.1073/pnas.1537759100
Zheng, J., Li, H. L., Tian, N., Liu, F., Wang, L., Yin, Y., et al. (2020). Interneuron accumulation of phosphorylated tau impairs adult hippocampal neurogenesis by suppressing GABAergic transmission. Cell. Stem Cell 26, 331–345 e6. doi: 10.1016/j.stem.2019.12.015
Zhu, B., Eom, J., and Hunt, R. F. (2019). Transplanted interneurons improve memory precision after traumatic brain injury. Nat. Commun. 10:5156. doi: 10.1038/s41467-019-13170-w
Keywords: MGE cell, transplantation, GABAergic interneuron, epilepsy, schizophrenia, Alzheimer’s Disease, interneuron disorders/dysfunction, autism spectrum disorder
Citation: Li D, Wu Q and Han X (2022) Application of Medial Ganglionic Eminence Cell Transplantation in Diseases Associated With Interneuron Disorders. Front. Cell. Neurosci. 16:939294. doi: 10.3389/fncel.2022.939294
Received: 09 May 2022; Accepted: 20 June 2022;
Published: 05 July 2022.
Edited by:
Claudia Saraiva, University of Luxembourg, LuxembourgReviewed by:
Takashi Tominaga, Tokushima Bunri University, JapanLudovic Telley, University of Lausanne, Switzerland
Copyright © 2022 Li, Wu and Han. This is an open-access article distributed under the terms of the Creative Commons Attribution License (CC BY). The use, distribution or reproduction in other forums is permitted, provided the original author(s) and the copyright owner(s) are credited and that the original publication in this journal is cited, in accordance with accepted academic practice. No use, distribution or reproduction is permitted which does not comply with these terms.
*Correspondence: Xiaohua Han, aGFueGlhbzE0NzBAaHVzdC5lZHUuY24=