- 1Department of Mental Diseases, Shanghai Municipal Hospital of Traditional Chinese Medicine, Shanghai University of Traditional Chinese Medicine, Shanghai, China
- 2School of Rehabilitation Science, Shanghai University of Traditional Chinese Medicine, Shanghai, China
- 3Institute of Rehabilitation Science, Shanghai University of Traditional Chinese Medicine, Shanghai, China
- 4Department of Traditional Treatment, Longhua Hospital, Shanghai University of Traditional Chinese Medicine, Shanghai, China
- 5School of Basic Medicine, Shanghai University of Traditional Chinese Medicine, Shanghai, China
- 6Shanghai Geriatric Institute of Chinese Medicine, Shanghai University of Traditional Chinese Medicine, Shanghai, China
- 7Department of Physical Therapy, Graduate Institute of Rehabilitation Science, China Medical University, Taichung, Taiwan
- 8Department of Physical Therapy, Asia University, Taichung, Taiwan
Sleep disturbances not only deteriorate Alzheimer’s disease (AD) progress by affecting cognitive states but also accelerate the neuropathological changes of AD. Astrocytes and microglia are the principal players in the regulation of both sleep and AD. We proposed that possible astrocyte-mediated and microglia-mediated neuropathological changes of sleep disturbances linked to AD, such as astrocytic adenosinergic A1, A2, and A3 regulation; astrocytic dopamine and serotonin; astrocyte-mediated proinflammatory status (TNFα); sleep disturbance-attenuated microglial CX3CR1 and P2Y12; microglial Iba-1 and astrocytic glial fibrillary acidic protein (GFAP); and microglia-mediated proinflammatory status (IL-1b, IL-6, IL-10, and TNFα). Furthermore, astrocytic and microglial amyloid beta (Aβ) and tau in AD were reviewed, such as astrocytic Aβ interaction in AD; astrocyte-mediated proinflammation in AD; astrocytic interaction with Aβ in the central nervous system (CNS); astrocytic apolipoprotein E (ApoE)-induced Aβ clearance in AD, as well as microglial Aβ clearance and aggregation in AD; proinflammation-induced microglial Aβ aggregation in AD; microglial-accumulated tau in AD; and microglial ApoE and TREM2 in AD. We reviewed astrocytic and microglial roles in AD and sleep, such as astrocyte/microglial-mediated proinflammation in AD and sleep; astrocytic ApoE in sleep and AD; and accumulated Aβ-triggered synaptic abnormalities in sleep disturbance. This review will provide a possible astrocytic and microglial mechanism of sleep disturbance linked to AD.
Introduction to Sleep Disturbance and Alzheimer’s Disease
Sleep plays an important role in maintaining normal biological and physiological functions. Disturbed sleeping affects not only the health condition but also the life quality of individuals. Chronic sleep loss is linked to a wide range of unhealthy conditions, such as altered food intake, weight loss or gain, skin lesions, compromised thermoregulation, and even death, causing mental and economic burdens to the family (Rechtschaffen et al., 1989; Siegel, 2008). Sleep states can be characterized by electroencephalogram (EEG) activity, non-rapid eye movement (NREM), and rapid eye movement (REM) sleep. NREM sleep is featured with slow-wave EEG, while the REM sleep stage shows higher frequency brain activity with low amplitude but high-frequency EEG (Scammell et al., 2017). The timing for sleep is controlled by sleep and circadian rhythm. The former is regulated by homeostasis, which determines the sleep duration and amount, and the latter relies on the function of the suprachiasmatic nucleus of the lateral hypothalamus (LH) (Partch et al., 2014; Borbély et al., 2016).
Sleeping disturbance can be triggered by the disturbance of environmental and physiological factors (Donlea and Shaw, 2009) including senescence, genetic mutation, and disease states (Potter et al., 2016; Charrier et al., 2017; Veatch et al., 2017). Sleep gene mutation is seen in the disruption of synaptic activity and neuronal homeostasis, which may contribute to the sleeping disturbance in multiple psychiatric disorders and diseases (Potter et al., 2016; Mulas et al., 2019). Specifically, sleep disturbance in neurodegenerative diseases, for example, Alzheimer’s disease (AD), has gained increasingly wide attention worldwide. Patients with AD have been suffering from different magnitudes of sleeping difficulties, which is also a classical symptom of AD (McCleery and Sharpley, 2020; Matsumoto and Tsunematsu, 2021).
Sleep disturbance and AD have huge impacts on individuals and society, therefore growing to be global health concerns. Loss of sleep accelerates AD progression not only by emotionally affecting the mental states of patients but also through the pathological changes of AD (Irwin and Vitiello, 2019; Van Egroo et al., 2019; Wang and Holtzman, 2020). Sleep disorders and AD partially share pathological mechanisms and induce similar cognitive deficits (Uddin et al., 2020; Lucey et al., 2021). Therefore, it is important to further characterize the possible neuropathological changes of sleep disturbance linked to AD. AD is well acknowledged that genetic disturbance is an important pathological driving force of AD (Lane et al., 2018). Poor sleep seems to exacerbate neurodegeneration (Grimmer et al., 2020; Baril et al., 2022; Blackman et al., 2022). Genome-wide studies identified more than 20 high-risk genes associated with AD from thousands of patients. Mutation of a few typical genes, including amyloid precursor protein and apolipoprotein (APOE), accounts for a majority of AD development (Bateman et al., 2011; Lane et al., 2018). It is known that the fibrillar conformation of amyloids’ oligomeric forms interacts with the innate immune system to initiate a transcriptional inflammatory response (Lee et al., 2020). Mutation of these genes leads to amyloid beta (Aβ) abnormal protein functions, which can trigger a series of immune reactions in the central nervous system (CNS). In patients without risk alleles, chronic sleep restriction induces frontal cortical mitochondrial dysfunction and mitochondria-related Aβ accumulation, which is considered to be a risk factor for the pathophysiology of sporadic Alzheimer’s disease (Zhao et al., 2016, 2019; Liu et al., 2020). Misfolded Aβ and tau trigger glial cell vigilance and then induce a series of complex immune responses with neuroinflammation and neurodegeneration (Wu et al., 2021). Thus, neuro-immune interaction has surged as a critical convergent point to study the pathological mechanism of AD.
Sleep is essential for the recharging of immune system, and sleep disturbance-induced immune dysfunction could promote AD psychosis (Wu et al., 2019). It has been reported that sleep disturbance can trigger the proinflammatory process of the body to protect itself from harm during daytime.
Astrocytic and Microglial Roles in Sleep or Sleep Disturbance
Central nervous system infections gained more and more attention in the exploration of the pathology of sleep homeostasis (Tesoriero et al., 2019). Accumulating body of research indicates the principal role of astrocytes and microglia in the regulation of both sleep and AD (Garofalo et al., 2020; Gentry et al., 2022). Chronic sleep deprivation was found to increase the activity of both astrocyte and microglia (Bellesi et al., 2017), which are distributed widely across the CNS, are in charge of controlling neuroinflammation, and have surged to be the major players in the regulation of sleep-awake cycle.
Astrocyte in Sleep or Sleep Disturbance
Circadian oscillation and sleep homeostasis determine the sleep cycle (Fuller et al., 2006). Astrocytes have been found to participate in the regulation of sleep cycle and homeostasis (Brown et al., 2012; Czeisler et al., 2019; Lazarus et al., 2019; Bojarskaite et al., 2020). Studies monitoring astrocytic Ca2+ dynamics in vivo showed that astrocytic Ca2+ activity was characterized by a spatial-temporal feature corresponding to sleep-wake cycles (Bojarskaite et al., 2020; Peng et al., 2020). Astrocytes show lower astrocytic Ca2+ activity during the slow-wave sleep state compared with that in the awake and NREM state. While increased astrocytic Ca2+ signaling had been detected before the transition from slow-wave sleep to wakefulness (Bojarskaite et al., 2020; Vaidyanathan et al., 2021). It is speculated that the downregulation of astrocytic activity is accommodated by the relative inactive state of neural activity during sleep (Bojarskaite et al., 2020). Interestingly, this study also discovers that the astrocytic process presents more frequent Ca2+ signals compared with the glial cell body during a slow-wave sleep state (Bojarskaite et al., 2020). Astrocytes use the process to scan the perturbation of synaptic activity. Therefore, although the general activity of astrocytes is low, Ca2+ activity in the process could preserve their ability in maintaining the homeostasis of CNS during sleep.
Astrocytic A1, A2, and A3 Regulation in Sleep
How do astrocytes or astrocytic processes accomplish this process? Astrocyte exerts its actions mainly through the release of adenosine (Porkka-Heiskanen et al., 1997; Halassa et al., 2009), which is a metabolizing production of adenosine triphosphate (ATP). Once released into the extracellular space, it binds with A1, A2 (A2A and A2B subtypes), and A3 receptors (A3Rs), which are all G-protein-coupled receptors on the neural membrane (Fredholm et al., 1994). A1 and A3A Rs mainly inhibit the concentration of cyclic adenosine monophosphate (cAMP) and downregulate neural activity. In contrast, A2A and A2B Rs activate the cAMP intracellular signaling pathway to upregulate neural activity. Imbalanced activation of these two groups of receptors could cause perturbation in the homeostasis of the neural network associated with sleep disorder and cognitive deficits (Portas et al., 1997; Pereira et al., 2005). Few reviews have summarized the specific role of adenosine, A1 and A2Rs, in the regulation of sleep and wakefulness (Huang et al., 2014; Lazarus et al., 2019). In the model proposed by Lazarus and his colleagues, these two types of receptors are phase-locked with a certain period of sleep (Lazarus et al., 2019). A1Rs are mostly located on presynapses and are responsible for maintaining the slow-wave oscillation during the sleep state by reducing the presynaptic release of neurotransmitters. It has been found that the administration of A1R antagonist in a variety of brain regions facilitates the sleep process (Rainnie et al., 1994; Liu and Gao, 2007; Oishi et al., 2008), while A2ARs allows the brain to enter a sleep state. The activation of A2aR in these brain regions promotes REM (Satoh et al., 1996; Urade et al., 2003) and the transition from sleep to awake state. However, A2aR activation-induced waking is often accompanied by higher-order cognitive deficits resembling the consequence of sleep loss (Urry and Landolt, 2015). Therefore, hyperactivation of A2aR might underlie the stimulant-induced impairment of attention.
Astrocytic Dopamine and Serotonin in Sleep
Astrocytes also mediate sleep through dopamine and serotonin. Neural circuits transmitting monoamine signals help maintain the sleep homeostasis. Glial cells are known to metabolize monoamine (Nall and Sehgal, 2014). In turn, norepinephrine can control the network formation between astrocyte and neurons (Bar El et al., 2019). Studies in drosophila revealed that dopamine promoted awake state and facilitated memory formation during sleep. Another monoamine family member, serotonin, also enhanced the sleeping process through different signaling pathways though (for review see Nall and Sehgal (2014)). AANAT1, an astrocyte gene that acetylates and inactivates monoamine, is found critical for the regulation of serotonin and dopamine levels in the brain. Studies have shown that AANAT1 can acetylate dopamine and affect melatonin production to regulate sleep (Cheng et al., 2012; Kulczykowska et al., 2017; Fagan et al., 2021), and it can limit the accumulation of serotonin and dopamine in the brain after sleep deprivation (Nall and Sehgal, 2014; Davla et al., 2020). Sleep deprivation leads to a significant increase in serotonin and dopamine, and this effect is reversed by astrocyte, but not neuron-specific AANAT1 mutation flies (Davla et al., 2020), suggesting that astrocyte-mediated monoamine metabolism is important for sleep homeostasis.
Astrocyte-Mediated Proinflammatory Status in Sleep
Apart from the astrocyte-neuron interaction through Ca2+ signal modulation, the astrocyte-mediated inflammatory pathway is another leading player in the regulation of sleep. One of the important regulatory substances of sleep associated with astrocyte is tumor necrosis factor alpha (TNFα), a proinflammatory factor. TNF-α not only promotes non-REM but also contributes to the establishment of sleep homeostasis following sleep deprivation (Yamasu et al., 1992). This function is mainly accomplished through the regulation of neural activity and synaptic plasticity of sleep circuits (Turrin and Rivest, 2004; Kaneko et al., 2008). A recent study discovered that knockdown Drosophila TNF-α homolog, Eiger, specifically in astrocyte could largely reduce the sleep duration (Vanderheyden et al., 2018a), indicating a waking-favor role the TNF-α plays in sleep homeostasis. In fact, human studies found that TNF-α G308A polymorphism, which caused a reduction of TNF-α function, could predict a subject’s resilience to sleep deprivation (Satterfield et al., 2015). This evidence together makes astrocyte an essential player in the normal sleeping process and a mediator of sleep homeostasis.
Sleep Disturbance-Attenuated Microglial CX3C Chemokine Receptor 1 and P2Y12
Microglia serve as tissue-resident macrophages in the CNS and account for 5–12% of total brain cells (Lawson et al., 1990). They respond to disturbance of neural homeostasis caused by pathological challenges in the neural environment and are involved in the regulation of aging, neuropathic pain, and neurodegenerative disease (for review, Salter and Stevens (2017), Inoue and Tsuda (2018), Schwabe et al. (2020)). Although glial cells appear to be strong candidates of sleep homeostasis, the role microglia play in the process is still underestimated. Deurveilher et al. (2021) summarized the cell population, morphology, microglia genes, and physiology feature changes during daytime and sleep. They claimed that microglia cell intensity (number) was not changed after sleep loss. Recent research also showed the circadian rhythm of microglia is accompanied by morphological and molecular phenotypic changes (Deurveilher et al., 2021).
The fractalkine (CX3CL1) and its receptor CX3C chemokine receptor 1 (CX3CR1) and P2Y12, a chemoreceptor for adenosine diphosphate (ADP) that belongs to the Gi class of a group of G protein-coupled (GPCR) purinergic receptors, were found to be associated with sleep disturbance and were subjected to alteration following sleep deprivation. Microglial mRNA levels of CX3CR1 and P2Y12, two widely expressed microglial receptors in the CNS, were significantly reduced 72 h later following sleep deprivation in the hippocampus (Tuan and Lee, 2019). The increase in these mRNA was specifically in the brain region and highly relevant with age. In the medial prefrontal cortex, sleep deprivation resulted in less reduction of microglia gene expression in old mice compared with young mice (Guo et al., 2019). However, it is hard to conclude from this evidence that how microglia participate in the regulation of the normal sleeping state or the transition between sleep and awake states.
Sleep Disturbance-Induced Microglial Ionized Calcium-Binding Adaptor Molecule 1 and Astrocytic Glial Fibrillary Acidic Protein
Microglia also participate in the adenosine signaling pathway regulating the sleep process. Chronic sleep deprivation increases the permeability of BBB and the expression of A2a receptor in multiple brain regions including hippocampus, basal nuclei, and cerebral cortex (Hurtado-Alvarado et al., 2016). Ionized calcium-binding adaptor molecule 1 (Iba-1), also known as Aif-1 (allograft inflammatory factor 1), and glial fibrillary acidic protein (GFAP) in microglia and astrocytes were induced by sleep deprivation. While the application of A2a-specific antagonist can attenuate the increase of iba-1 and GFAP induced by sleep deprivation (Hurtado-Alvarado et al., 2016). A2a receptor was shown to regulate the average capillary cerebral blood flow (CBF) of multiple cortical regions during rapid eye movement (REM) sleep, while there is no difference in capillary CBF between active awake and NREM sleep (van Calker et al., 2019; Tsai et al., 2021). These findings suggest that the microglia-adenosine interaction has a crucial role in sleep loss. However, it is still unclear whether microglia are directly involved in A2a receptor-mediated synaptic function in the regulation of sleep homeostasis. It is known that one of the major functions of microglia is phagocytosis. Relying on this function, microglia not only control synaptic punning (Tuan and Lee, 2019) but also clean up synaptic elements following overexcitation caused by sleep loss in the cortex and hippocampus (Bellesi et al., 2017; Tuan and Lee, 2019). Importantly, research conducted by Gentry et al. (2022) showed that microglia is essential for synaptic homeostasis and the protection of memories potentially through the upregulation of synaptic-homeostasis-related genes and further protection of nascent dendritic spines that may be removed during recovery sleep. Therefore, microglia are responsible for synaptic pruning not only during normal development but also when against pathological stimulation in the CNS (Ho, 2019; Sellgren et al., 2019). Lack of microglia-mediated synaptic pruning and clearance may result in sleep disturbance and further impairment of synaptic-plasticity-dependent higher-order cognitive function (Tuan and Lee, 2019; Deurveilher et al., 2021). Microglial CX3C-chemokine receptor 1 (CX3CR1) deficiency-attenuated neuroinflammation and the related synaptic pruning lead to cognition decline during sleep deprivation (Xin et al., 2021). Cytokine is another regulatory substance mediating the function of microglia in the sleep process (Qiu et al., 2021). It has been discovered that chronic deprivation of sleep drastically increases the expression of Iba-1 and glial fibrillary acidic protein (GFAP) levels which are receptively microglial- and astrocytic-specific markers (Bellesi et al., 2017).
Microglial Proinflammatory Cytokine in Sleep Disturbance
Systematic proinflammatory cytokines including IL-6 and TNF-α could facilitate the NREM sleep, while anti-proinflammatory cytokines including IL-4 and IL-10 could generate the opposite effect (Rico-Rosillo and Vega-Robledo, 2018). However, the meta-analysis revealed the covariation of cytokines and sleep duration. Comparing short and long sleep duration together, sleep disturbance is associated with a significantly higher level of systematic IL-6 (Irwin et al., 2016). Although TNF-α is not remarkably associated with sleep duration (Irwin et al., 2016), chronic sleep disturbance induced by circadian misalignment could rise up the TNF-α and IL-10 levels in plasma (Wright et al., 2015). Short-term sleep loss in zebra finch remarkably increased the proinflammatory [interleukin (IL)-1b and IL-6] cytokine gene expression but reduced the anti-inflammatory (IL-10) cytokine gene expression in the CNS, specifically the hippocampus (Cooper et al., 2019). Sleep disturbances can increase inflammatory responses through the release of a series of inflammatory factors such as IL-1β, IL-6, and TNF-α, which further exacerbates symptoms or the risk of neurodegenerative diseases (Marshall and Born, 2002; Weil et al., 2009; Besedovsky et al., 2019; Green et al., 2020). Observation and findings concerning microglia function implicate an indispensable role of microglial immune response in sleep regulation. Interestingly, as elaborated in the above section, TNF-α in microglia plays an awaking-favor role suggesting that microglia could regulate the sleeping loss through shared signaling pathways.
Astrocytic and Microglial Roles in Alzheimer’s Disease
Astrocytic and Microglial Aβ and Tau in Alzheimer’s Disease
Astrocytes and microglia are the major players of innate immune response but they contribute to the pathology of AD in different manners. Aβ and tau are two major substances of AD; astrocytes and microglia contribute to their clearance and halt their spreading (Fagan and Holtzman, 2000; Gratuze et al., 2021; Mahan et al., 2022). Astrocytes in the healthy brain remain in resting state. Accumulation of Aβ and NFT in the CNS triggers the activation of astrocytes before the onset of AD psychosis, which will further induce the release of a series of proinflammatory factors and ultimately neuroinflammation (Berridge, 2014). In turn, the neuroinflammation further accelerates the progress of AD. Astrocytes are one of the important resources of adenosine in the CNS. Astrocytes dysfunction in LH could thus be responsible for the sleep disturbance.
Astrocytic Aβ Interaction in Alzheimer’s Disease
At the early stage of AD, astrocytes serve as a protector of the neuro system to digest accumulated Aβ and transport it out of the BBB with the facilitation of chaperones, one type of heat shock proteins (Ries and Sastre, 2016). While along with the surge of Aβ and formation of NFT, overactivated astrocytes introduce perturbation to the CNS and cause damage to the surrounding neurons which results in the imbalance of immune-neuron homeostasis (Agostinho et al., 2010; Avila-Muñoz and Arias, 2014; Ahmad et al., 2019) and Aβ accumulation in both neurons and astrocytes. A previous study revealed that the entorhinal cortex of patients with AD exhibited an increased level of Aβ, suggesting that astrocyte was also a victim of Aβ accumulation in AD generation.
Astrocytic Interaction With Aβ in Central Nervous System
How do astrocytes interact with Aβ in CNS during the onset and progression of AD? Conventionally, it is believed that as CNS immune cells, astrocytes eliminate and degrade Aβ through proteolysis, which involves a variety of proteases including neprilysin, endothelin-converting enzyme, insulin-degrading enzyme, and matrix metalloproteases (Apelt et al., 2003; Ahmad et al., 2019). Deficiency in these proteins directly leads to abnormal degradation and accumulation of Aβ. Disruption of the sleep-awake cycle and decrease in sleep duration directly impair the ability of astrocytes in Aβ clearance (Sunkaria and Bhardwaj, 2022).
Astrocytic Apolipoprotein E-Induced Aβ Clearance in Alzheimer’s Disease
ApoE, a lipid-loaded protein that facilitates the transportation of Aβ by binding with ApoE receptors on the surface of the cell membrane, also mediates the astrocyte and Aβ interaction (Liao et al., 2017; Zhao et al., 2018; Uddin et al., 2019). Among the ApoE family, ApoE2 is considered as a neuroprotector against AD pathology, while ApoE4 promotes the Aβ accumulation and AD symptoms (Fleisher et al., 2013; Serrano-Pozo et al., 2015). The major source of ApoE is reported to be glial cells (Mahley, 2016). To form ApoE particles, they will be lapidated by ATP-binding cassette A1 or G1 transporters. These particles are responsible for the transportation of lipids in the CNS. Besides, ApoE is essential for the homeostasis of lipid metabolism (Farmer et al., 2019) and endocytic clearance of Aβ in astrocytes (Prasad and Rao, 2018). Acidification of endosome in ApoE4 astrocytes increases the expression of low-density lipoprotein receptor-related protein in intracellular compartments, which is responsible for the deficit of Aβ clearance through endocytosis (Verghese et al., 2013; Prasad and Rao, 2018). Therefore, astrocytic ApoE might serve as the bridge in the interaction between astrocyte and Aβ in the exploration of AD pathology.
Microglial Aβ Clearance and Aggregation in Alzheimer’s Disease
The function of microglia in AD is partially overlapped with astrocytes carrying distinct features. Microglia also gather around Aβ plaques in the CNS of patients with AD (Perlmutter et al., 1992; Rozemuller et al., 1992). Microglia-mediated Aβ clearance relies on the stimulation of Aβ itself. TREM2, expressed in the microglia membrane and its exosome membrane, combines with Aβ and then changes its surrounding inflammatory microenvironment further promoting microglia phagocytosis of Aβ (Huang et al., 2022). The complex pathological changes of AD, including pTau, Aβ, and pSyn, also affect the microglia phenotype in turn (Fixemer et al., 2022). Aβ causes dysregulation of mitochondria in microglia, which further activates them to trigger phagocytosis activity and, in the meanwhile, stimulates the release of proinflammatory cytokines to remove extracellular Aβ plaques (Chiozzi et al., 2019). Recently, Oualid Sbai’s team showed that RAGE-TXNIP axis inhibition in microglia could reduce Aβ transport from the cell surface to mitochondria and restore mitochondrial function and Aβ toxicity, which in turn inhibit NLRP3 inflammasome activation (Sbai et al., 2022). Disruption of the sleep-awake cycle and decrease in sleep duration directly impair the ability of microglia in Aβ clearance (as shown in Figure 1).
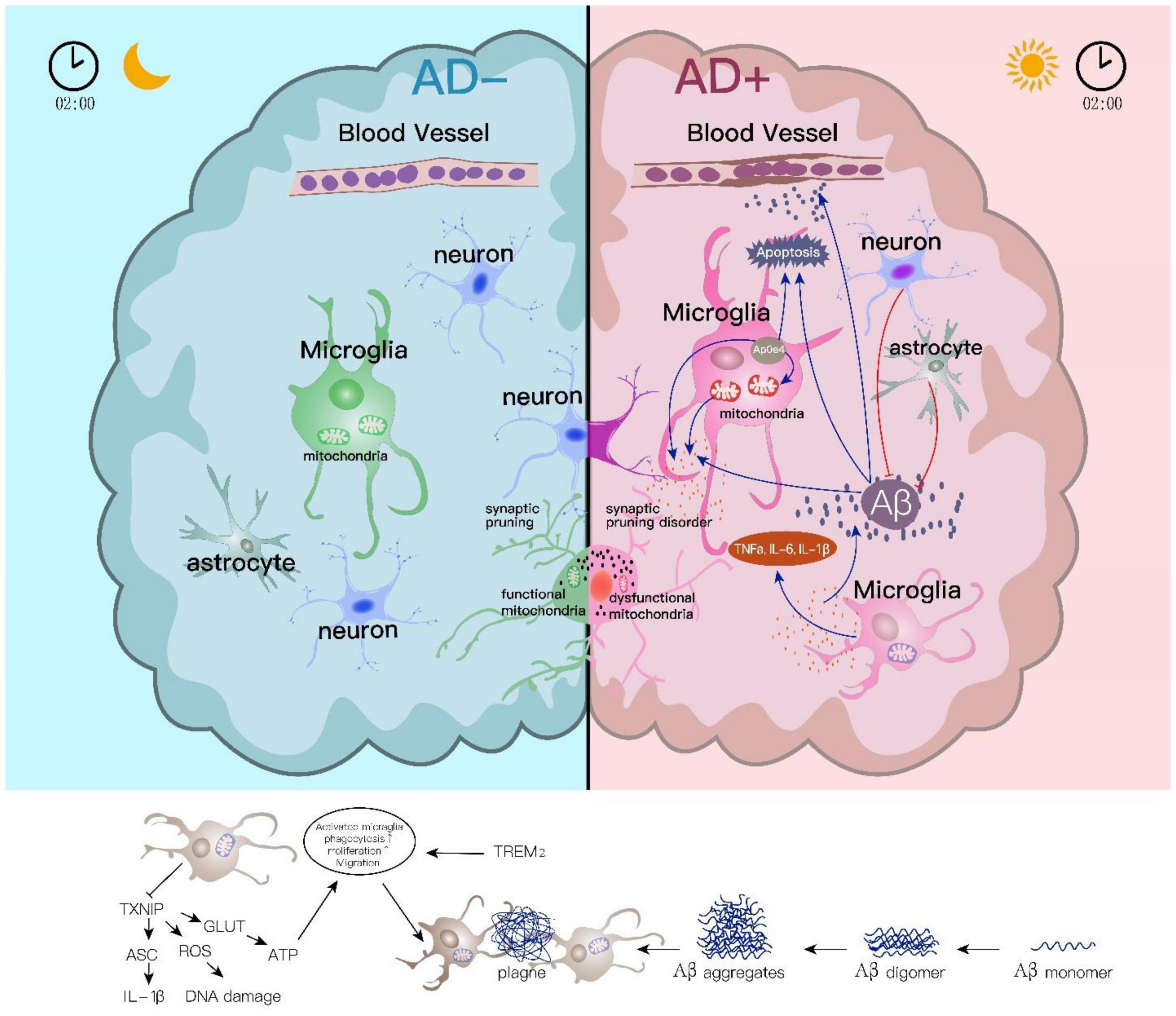
Figure 1. Astrocyte and microglial roles in sleep disturbance linked Alzheimer’s disease. Sleep and CNS immune influence each other. Microglial responses in the sleep/wake are essential for Aβ clearance and inflammatory activation. Aβ accumulation induces abnormal mitochondrial function in microglia which further activates the release of inflammatory cytokines, while Aβ clearance by microglia phagocytosis relies on the stimulation of Aβ itself. TREM2 in the microglia membrane combines with Aβ and then enhances its ability on Aβ phagocytosis. The downregulated TXNIP induces ROS inhibition and further causes DNA damage during sleep disorders. On the contrary, lower TXNIP under sleep disturbances could increase NLRP3 inflammasome activation and IL-1β-initiated inflammatory response. Apart from the role in Aβ clearance and Aβ involved inflammation, microglia directly participate in synapse removal or “synaptic stripping,” and this is regulated by normal sleep/wake rhythm.
Proinflammation-Induced Microglial Aβ Aggregation in Alzheimer’s Disease
Proinflammatory cytokines, including IL-1b, IL6, and TNF-alpha, are elevated in cultured neurons derived from patients with AD (Murphy et al., 1998; Liu and Hong, 2003; Ye et al., 2013; Wood et al., 2015; Barroeta-Espar et al., 2019). These proinflammatory cytokines could derive from microglia upon being stimulated by extracellular accumulated Aβ. Microglia participate in soluble Aβ (sAβ) macrophages in vitro and in vivo, which have been shown to be trafficked into the late lysosomal compartment and degraded (Prokop et al., 2013; John and Reddy, 2021). In contrast, some of the proinflammatory and anti-inflammatory cytokines that mediate Aβ clearance by astrocytes are also the major ones that microglia secrete to promote Aβ aggregation (Decourt et al., 2017; Ng et al., 2018; Taipa et al., 2019), suggesting a reciprocal interaction between Aβ aggregation and microglia dominating clearance.
Microglial Accumulated Tau in Alzheimer’s Disease
Although Aβ is considered as the driving force of tau pathology, downstream biological pathways mediated by Aβ and tau in AD could be unrelated to each other (van der Kant et al., 2020). Tau accumulates in the entorhinal cortex during the onset of AD and propagates to the neocortex along with the development of the disease (van der Kant et al., 2020). Microglia facilitate the propagation of tau among neurons through exosome secretion from the entorhinal cortex to the hippocampus (Asai et al., 2015; Hopp et al., 2018).
Microglial Apolipoprotein E and Triggering Receptor Expressed on Myeloid Cells 2 in Alzheimer’s Disease
Although the leading position between tauopathy and microglia activation is undefined, there is no doubt that tauopathy is not only the cause but also the consequence of microglia activation in AD (for review, Vogels et al., 2019). Among those AD risk genes, ApoE and triggering receptor expressed on myeloid cells 2 (TREM2) are the main regulators of lipid metabolism by glial cells. ApoE is predominantly expressed in astrocytes, while its low expression in microglia under normal stage increases significantly close to astrocyte-level when reactive (Wang et al., 2021; Parhizkar and Holtzman, 2022). TREM2 is expressed in microglia with higher specificity (Pfrieger, 2003; Kim et al., 2009). Those microglia expressing AD risk genes including ApoE and TREM2 are classified as disease-associated microglia (Kfoury et al., 2012), which are the main players among the microglia family in the participation of AD. Alteration of TREM2 changes the homeostasis of microglia. For example, overexpression of TREM2 upregulates the homeostatic genes in microglia (Jiang et al., 2016). In contrast, downregulating TREM2 results in failed activation of microglia and release of proinflammatory factors that usually favor the formation of NFT (Jiang et al., 2018). Therefore, ApoE and TREM2 might be more important in controlling the progress of AD pathology, rather than timing the onset of it.
Astrocytic and Microglial Roles in Alzheimer’s Disease and Sleep
Sleep Disturbance in Alzheimer’s Disease
The major pathological features of AD include the accumulation of Aβ plaques and the neurofibrillary tangles (NFTs), which resulted from hyperphosphorylated tau protein. These substances cause oxidative stress, cell death, and destabilizing microtubules in the CNS, which triggers inflammatory cascades and causes physiological and cognitive deficits (McNaull et al., 2010; Heneka et al., 2015; Bronzuoli et al., 2016; Calsolaro and Edison, 2016; Guo et al., 2016). Poor sleep widely occurs during the normal aging process. However, sleep disturbance in AD is very common which adds extra burden and stress on patients. A previous study assessed the sleep quality of 215 patients with AD and found that 24.5% of them showed sleep disturbance with different magnitudes varying from mild to medium level (Moran et al., 2005). AD and sleep disturbance mutually affect each other. Neurodegeneration and immune reaction of AD may disrupt the sleep-wake cycle (Vanderheyden et al., 2018b). In the meanwhile, sleep disturbance further accelerates the pathological progress and symptoms of AD including impairment of cognitive function (Rauchs et al., 2008; Ooms et al., 2014). Therefore, characterizing the pathological mechanism linking sleep disturbance and AD will provide new insights on developing more potent therapeutic treatments.
Aβ and Tau in Alzheimer’s Disease and Sleep Disturbance
It is thus reasonable to speculate that Aβ and tau triggering CNS immune reaction may play a noticeable role in the sleeping disturbance seen in AD. Accumulated Aβ would further trigger more severe synaptic inhibition and cause more synapse loss in the sleep-associated neural circuit to deteriorate the sleeping disturbance in AD (Spinedi and Cardinali, 2019). This evidence implicates that a direct interaction between Aβ and synapse might link sleep regulation and AD, which provide a novel target for treating sleeping disturbance in AD. In fact, loss of sleep is sufficient to promote the accumulation of Aβ in the CNS in drosophila, rodents, and human (Kang et al., 2009; Tabuchi et al., 2015; Shokri-Kojori et al., 2018). Moreover, Aβ-induced synaptic deficits are major reasons driving AD pathogenesis (Hardy and Selkoe, 2002; Forloni and Balducci, 2018; Torres et al., 2021). Direct application of Aβ oligomer could also lead to long-term depression in synapses and cognitive deficits (Shankar et al., 2008; Marcello et al., 2019; Yu et al., 2021). Therefore, it is highly possible that Aβ accumulation in AD could impact synaptic function in neural circuits associated with sleep, followed by sleep disturbance and poor performance of cognition. However, how direct application of adenosine may regulate AB accumulation in AD and how it may change the sleep-awake cycle of patients with AD still need further clarification.
Astrocytic Apolipoprotein E in Sleep and Alzheimer’s Disease
Astrocytic gene ApoE might be one of the factors causing sleep disturbance in AD. The increase of ApoE expression has been seen in the CNS of AD, which is considered to enhance the amyloid pathology (Muñoz et al., 2019). Patients with AD carrying homozygous ApoEε4 gene are found to coexist with sleep disorders at a higher ratio (Koo et al., 2019; Pyun et al., 2019). However, ApoE facilitates the transportation of Aβ by binding with ApoE receptors on the surface of the cell membrane (Liao et al., 2017; Zhao et al., 2018; Uddin et al., 2019). The increased Aβ may promote the degradation of CNS and the change of circadian gene expression, thus interfering with the behavioral regulatory circuit and leading to Sundown syndrome. This also resulted in a higher proportion of patients with AD with homozygous ApoEε4 gene coexisting with sleep disorders (Koo et al., 2019; Pyun et al., 2019). We speculate that increased ApoE in AD patients with sundown syndrome was a protective mechanism against Aβ accumulation. In fact, delirium occurrence or even exacerbation during the evening in AD is consistent with “sundowning” (Vitiello et al., 1992; Volicer et al., 2001), and the effectiveness of circadian alignment by bright light therapy and melatonin contradicts the sundowning and other sleep-wake disorders in patients with AD (Cardinali et al., 2010; Sharma et al., 2021). The fluctuation curve of ApoE in astrocytes across different sleep stages remains unclear.
Microglial Proinflammatory Status in Alzheimer’s Disease and Sleep Disturbance
Microglia are the major source of cytokine in the CNS. Microglia also participate in sleep loss by enhancing the TNF-α signal. Interestingly, in patients with AD, proinflammatory cytokines, including IL-1b, IL6, and TNF-α, are particularly elevated through Aβ stimulating microglia (Murphy et al., 1998; Liu and Hong, 2003; Ye et al., 2013; Wood et al., 2015; Barroeta-Espar et al., 2019) and disrupt the homeostasis of immune system in the CNS, which may cause direct result in sleep disturbance seen in patients with AD. Therefore, microglia-mediated proinflammatory cytokines including TNF-α may underly the sleep disturbance seen in AD.
Conclusion and Future Directions
Loss of sleep not only deteriorates AD progress by emotionally affecting the mental states of patients but also accelerates the pathological changes of AD. Sleep disorders and AD partially share the common pathomechanisms with similar cognitive dysfunction. Therefore, it is important to fully characterize these pathological changes for better therapeutic intervention. In this review, we navigated through the mechanism associated with astrocytes and microglia in both sleep and AD (as shown in Figure 2). We particularly summarized substrates shared by astrocytes and microglia, including proinflammatory and anti-inflammatory factors (IL6, TNFα, IL-1β, and IL10). In the meanwhile, the discrepancy was presented between microglia and astrocytes in AD, for example, the specificity of ApoE and TREM. Aβ appears to be a strong candidate in linking sleep disturbance through the above-mentioned cytokines and genes with AD risk involving the engagement of both astrocytes and microglia. In future, certain questions still remain to be addressed in the field of exploring joint mechanisms underlying sleep disturbance and AD. (1) The fluctuation curve of ApoE in astrocytes across different sleep stages remains unclear. (2) How the direct application of adenosine may regulate AB accumulation in AD and how it may change the sleep-awake cycle of patients with AD still await further clarification. Comprehensive studies are needed to better answer these questions and potentially inspire better ideas for therapeutic treatments of sleep disturbance and AD. (3) The interaction between Aβ and synapse in simultaneously accelerating sleep disorder and AD provides a novel point to study the joint mechanism underlying these two disorders. However, the details of neural mechanisms at synaptic, cellular, and circuitry levels require more exploration in the future.
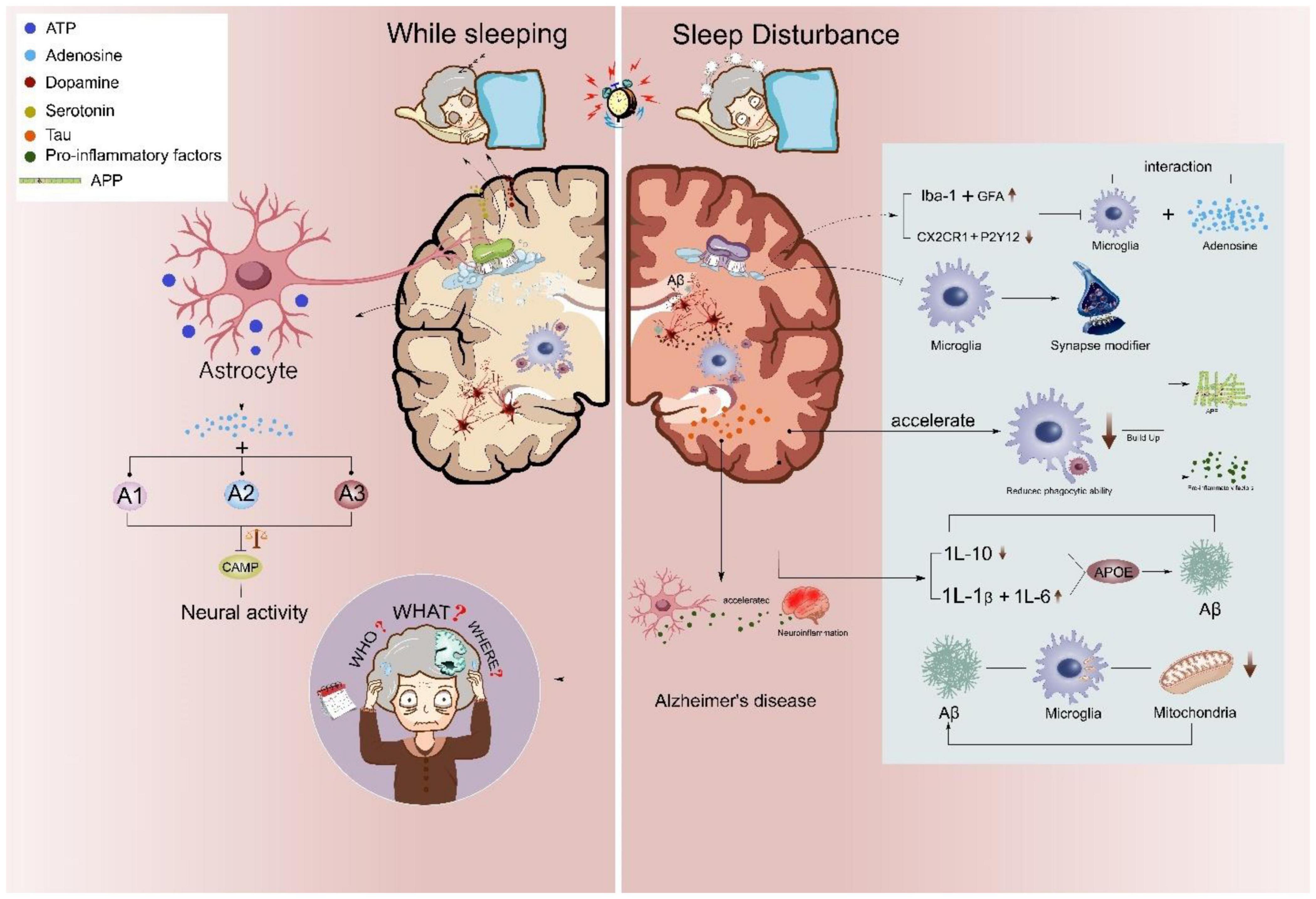
Figure 2. Astrocyte and microglial roles in sleep disturbance linked Alzheimer’s disease. Sleep disturbances accelerate the neuropathological changes of AD. During normal sleep/wake rhythm, astrocytic adenosinergic A1, A2, and A3 inhibit neural overactivation, while sleep disturbance attenuates microglial CX3CR1 and P2Y12 further inhibiting the phagocytic capacity of microglia. Abnormal sleep rhythms also promote microglial Iba-1 and astrocytic glial fibrillary acidic protein (GFAP) and increase microglia-mediated proinflammatory releases, such as IL-1b, IL-6, IL-10, and TNFα. Activated inflammatory status further induces microglial Aβ aggregation and microglial-accumulated tau in AD.
Author Contributions
S-YX and Y-JL wrote the main body of the manuscript. WL, CX, and S-DL did the proofreading and grammar checking. WL and Z-WS contributed to the graph abstract drawing. S-YX, Z-HY, and S-DL designed the study and guided the writing. Y-JL, Z-HY, and S-DL contributed to the manuscript revision. All authors contributed to the article and approved the submitted version.
Funding
We gratefully acknowledge the support provided by the Shanghai Municipal Key Clinical Specialty (Grant no. shslczdzk04901) and Three-Year Initiative Plan for Strengthening Public Health System Construction in Shanghai (GWV-10.1-XK20).
Conflict of Interest
The authors declare that the research was conducted in the absence of any commercial or financial relationships that could be construed as a potential conflict of interest.
Publisher’s Note
All claims expressed in this article are solely those of the authors and do not necessarily represent those of their affiliated organizations, or those of the publisher, the editors and the reviewers. Any product that may be evaluated in this article, or claim that may be made by its manufacturer, is not guaranteed or endorsed by the publisher.
Abbreviations
EEG, electroencephalogram; NREM, non-rapid eye movement; LH, lateral hypothalamus; BBB, blood–brain barrier; AD, Alzheimer’s disease; ApoE, apolipoprotein; PSEN1, presenilin 1; PSEN2, presenilin 2; A β, amyloid beta; ATP, adenosine triphosphate; cAMP, cyclic adenosine monophosphate; TNF α, tumor necrosis factor alpha; GFAP, glial fibrillary acidic protein; IL, interleukin; NFTs, neurofibrillary tangles; TREM2, triggering receptor expressed on myeloid cells 2.
References
Agostinho, P., Cunha, R. A., and Oliveira, C. (2010). Neuroinflammation, oxidative stress and the pathogenesis of Alzheimer’s disease. Curr. Pharm. Des. 16, 2766–2778. doi: 10.2174/138161210793176572
Ahmad, M. H., Fatima, M., and Mondal, A. C. (2019). Influence of microglia and astrocyte activation in the neuroinflammatory pathogenesis of Alzheimer’s disease: rational insights for the therapeutic approaches. J. Clin. Neurosci. 59, 6–11. doi: 10.1016/j.jocn.2018.10.034
Apelt, J., Ach, K., and Schliebs, R. (2003). Aging-related down-regulation of neprilysin, a putative β-amyloid-degrading enzyme, in transgenic Tg2576 Alzheimer-like mouse brain is accompanied by an astroglial upregulation in the vicinity of β-amyloid plaques. Neurosci. Lett. 339, 183–186. doi: 10.1016/S0304-3940(03)00030-2
Asai, H., Ikezu, S., Tsunoda, S., Medalla, M., Luebke, J., Haydar, T., et al. (2015). Depletion of microglia and inhibition of exosome synthesis halt tau propagation. Nat. Neurosci. 18, 1584–1593. doi: 10.1038/nn.4132
Avila-Muñoz, E., and Arias, C. (2014). When astrocytes become harmful: functional and inflammatory responses that contribute to Alzheimer’s disease. Ageing Res. Rev. 18, 29–40. doi: 10.1016/j.arr.2014.07.004
Bar El, Y., Kanner, S., Barzilai, A., and Hanein, Y. (2019). Calcium imaging, MEA recordings, and immunostaining images dataset of neuron-astrocyte networks in culture under the effect of norepinephrine. GigaScience 8:giy161. doi: 10.1093/gigascience/giy161
Baril, A. A., Beiser, A. S., Sanchez, E., Mysliwiec, V., Redline, S., Gottlieb, D. J., et al. (2022). Insomnia symptom severity and cognitive performance: moderating role of APOE genotype. Alzheimers Dement. 18, 408–421. doi: 10.1002/alz.12405
Barroeta-Espar, I., Weinstock, L. D., Perez-Nievas, B. G., Meltzer, A. C., Siao Tick Chong, M., Amaral, A. C., et al. (2019). Distinct cytokine profiles in human brains resilient to Alzheimer’s pathology. Neurobiol. Dis. 121, 327–337. doi: 10.1016/j.nbd.2018.10.009
Bateman, R. J., Aisen, P. S., De Strooper, B., Fox, N. C., Lemere, C. A., Ringman, J. M., et al. (2011). Autosomal-dominant Alzheimer’s disease: a review and proposal for the prevention of Alzheimer’s disease. Alzheimers Res. Ther. 3:1. doi: 10.1186/alzrt59
Bellesi, M., de Vivo, L., Chini, M., Gilli, F., Tononi, G., and Cirelli, C. (2017). Sleep loss promotes astrocytic phagocytosis and microglial activation in mouse cerebral cortex. J. Neurosci. 37, 5263–5273. doi: 10.1523/jneurosci.3981-16.2017
Berridge, M. J. (2014). Calcium regulation of neural rhythms, memory and Alzheimer’s disease. J. Physiol. 592, 281–293. doi: 10.1113/jphysiol.2013.257527
Besedovsky, L., Lange, T., and Haack, M. (2019). The sleep-immune crosstalk in health and disease. Physiol. Rev. 99, 1325–1380. doi: 10.1152/physrev.00010.2018
Blackman, J., Love, S., Sinclair, L., Cain, R., and Coulthard, E. (2022). APOE ε4, Alzheimer’s disease neuropathology and sleep disturbance, in individuals with and without dementia. Alzheimers Res. Ther. 14:47. doi: 10.1186/s13195-022-00992-y
Bojarskaite, L., Bjørnstad, D. M., Pettersen, K. H., Cunen, C., Hermansen, G. H., Åbjørsbråten, K. S., et al. (2020). Astrocytic Ca2+ signaling is reduced during sleep and is involved in the regulation of slow wave sleep. Nat. Commun. 11:3240. doi: 10.1038/s41467-020-17062-2
Borbély, A. A., Daan, S., Wirz-Justice, A., and Deboer, T. (2016). The two-process model of sleep regulation: a reappraisal. J. Sleep Res. 25, 131–143. doi: 10.1111/jsr.12371
Bronzuoli, M. R., Iacomino, A., Steardo, L., and Scuderi, C. (2016). Targeting neuroinflammation in Alzheimer’s disease. J. Inflamm. Res. 9, 199–208. doi: 10.2147/JIR.S86958
Brown, R. E., Basheer, R., McKenna, J. T., Strecker, R. E., and McCarley, R. W. (2012). Control of sleep and wakefulness. Physiol. Rev. 92, 1087–1187. doi: 10.1152/physrev.00032.2011
Calsolaro, V., and Edison, P. (2016). Neuroinflammation in Alzheimer’s disease: current evidence and future directions. Alzheimers Dement. 12, 719–732. doi: 10.1016/j.jalz.2016.02.010
Cardinali, D. P., Furio, A. M., and Brusco, L. I. (2010). Clinical aspects of melatonin intervention in Alzheimer’s disease progression. Curr. Neuropharmacol. 8, 218–227. doi: 10.2174/157015910792246209
Charrier, A., Olliac, B., Roubertoux, P., and Tordjman, S. (2017). Clock genes and altered sleep-wake rhythms: their role in the development of psychiatric disorders. Int. J. Mol. Sci. 18:938. doi: 10.3390/ijms18050938
Cheng, K. C., Liao, J. N., and Lyu, P. C. (2012). Crystal structure of the dopamine N-acetyltransferase-acetyl-CoA complex provides insights into the catalytic mechanism. Biochem. J. 446, 395–404. doi: 10.1042/bj20120520
Chiozzi, P., Sarti, A. C., Sanz, J. M., Giuliani, A. L., Adinolfi, E., Vultaggio-Poma, V., et al. (2019). Amyloid β-dependent mitochondrial toxicity in mouse microglia requires P2X7 receptor expression and is prevented by nimodipine. Sci. Rep. 9:6475. doi: 10.1038/s41598-019-42931-2
Cooper, L. N., Mishra, I., and Ashley, N. T. (2019). Short-term sleep loss alters cytokine gene expression in brain and peripheral tissues and increases plasma corticosterone of zebra finch (Taeniopygia guttata). Physiol. Biochem. Zool. 92, 80–91. doi: 10.1086/701170
Czeisler, C. M., Silva, T. M., Fair, S. R., Liu, J., Tupal, S., Kaya, B., et al. (2019). The role of PHOX2B-derived astrocytes in chemosensory control of breathing and sleep homeostasis. J. Physiol. 597, 2225–2251. doi: 10.1113/jp277082
Davla, S., Artiushin, G., Li, Y., Chitsaz, D., Li, S., Sehgal, A., et al. (2020). AANAT1 functions in astrocytes to regulate sleep homeostasis. eLife 9:e53994. doi: 10.7554/eLife.53994
Decourt, B., Lahiri, D. K., and Sabbagh, M. N. (2017). Targeting tumor necrosis factor alpha for Alzheimer’s disease. Curr. Alzheimer Res. 14, 412–425. doi: 10.2174/1567205013666160930110551
Deurveilher, S., Golovin, T., Hall, S., and Semba, K. (2021). Microglia dynamics in sleep/wake states and in response to sleep loss. Neurochem. Int. 143:104944. doi: 10.1016/j.neuint.2020.104944
Donlea, J. M., and Shaw, P. J. (2009). Sleeping together using social interactions to understand the role of sleep in plasticity. Adv. Genet. 68, 57–81. doi: 10.1016/s0065-2660(09)68003-2
Fagan, A. M., and Holtzman, D. M. (2000). Astrocyte lipoproteins, effects of apoE on neuronal function, and role of apoE in amyloid-beta deposition in vivo. Microsc. Res. Tech. 50, 297–304. doi: 10.1002/1097-0029(20000815)50:4<297::AID-JEMT9>3.0.CO;2-C
Fagan, R. R., Kearney, P. J., Luethi, D., Bolden, N. C., Sitte, H. H., Emery, P., et al. (2021). Dopaminergic Ric GTPase activity impacts amphetamine sensitivity and sleep quality in a dopamine transporter-dependent manner in Drosophila melanogaster. Mol. Psychiatry 26, 7793–7802. doi: 10.1038/s41380-021-01275-y
Farmer, B. C., Kluemper, J., and Johnson, L. A. (2019). Apolipoprotein E4 alters astrocyte fatty acid metabolism and lipid droplet formation. Cells 8:182. doi: 10.3390/cells8020182
Fixemer, S., Ameli, C., Hammer, G., Salamanca, L., Uriarte Huarte, O., Schwartz, C., et al. (2022). Microglia phenotypes are associated with subregional patterns of concomitant tau, amyloid-β and α-synuclein pathologies in the hippocampus of patients with Alzheimer’s disease and dementia with Lewy bodies. Acta Neuropathol. Commun. 10:36. doi: 10.1186/s40478-022-01342-7
Fleisher, A. S., Chen, K., Liu, X., Ayutyanont, N., Roontiva, A., Thiyyagura, P., et al. (2013). Apolipoprotein E ε4 and age effects on florbetapir positron emission tomography in healthy aging and Alzheimer disease. Neurobiol. Aging 34, 1–12. doi: 10.1016/j.neurobiolaging.2012.04.017
Forloni, G., and Balducci, C. (2018). Alzheimer’s disease, oligomers, and inflammation. J. Alzheimers Dis. 62, 1261–1276. doi: 10.3233/jad-170819
Fredholm, B. B., Abbracchio, M. P., Burnstock, G., Daly, J. W., Harden, T. K., Jacobson, K. A., et al. (1994). Nomenclature and classification of purinoceptors. Pharmacol. Rev. 46, 143–156.
Fuller, P. M., Gooley, J. J., and Saper, C. B. (2006). Neurobiology of the sleep-wake cycle: sleep architecture, circadian regulation, and regulatory feedback. J. Biol. Rhythms 21, 482–493. doi: 10.1177/0748730406294627
Garofalo, S., Picard, K., Limatola, C., Nadjar, A., Pascual, O., and Tremblay, M. (2020). Role of glia in the regulation of sleep in health and disease. Compr. Physiol. 10, 687–712. doi: 10.1002/cphy.c190022
Gentry, N. W., McMahon, T., Yamazaki, M., Webb, J., Arnold, T. D., Rosi, S., et al. (2022). Microglia are involved in the protection of memories formed during sleep deprivation. Neurobiol. Sleep Circadian Rhythms 12:100073. doi: 10.1016/j.nbscr.2021.100073
Gratuze, M., Chen, Y., Parhizkar, S., Jain, N., Strickland, M. R., Serrano, J. R., et al. (2021). Activated microglia mitigate Aβ-associated tau seeding and spreading. J. Exp. Med. 218:e20210542. doi: 10.1084/jem.20210542
Green, T. R. F., Ortiz, J. B., Wonnacott, S., Williams, R. J., and Rowe, R. K. (2020). The bidirectional relationship between sleep and inflammation links traumatic brain injury and Alzheimer’s disease. Front. Neurosci. 14:894. doi: 10.3389/fnins.2020.00894
Grimmer, T., Laub, T., Hapfelmeier, A., Eisele, T., Fatke, B., Hölzle, P., et al. (2020). The overnight reduction of amyloid β 1-42 plasma levels is diminished by the extent of sleep fragmentation, sAPP-β, and APOE ε4 in psychiatrists on call. Alzheimers Dement. 16, 759–769. doi: 10.1002/alz.12072
Guo, H. D., Zhu, J., Tian, J. X., Shao, S. J., Xu, Y. W., Mou, F. F., et al. (2016). Electroacupuncture improves memory and protects neurons by regulation of the autophagy pathway in a rat model of Alzheimer’s disease. Acupunct. Med. 34, 449–456. doi: 10.1136/acupmed-2015-010894
Guo, X., Keenan, B. T., Sarantopoulou, D., Lim, D. C., Lian, J., Grant, G. R., et al. (2019). Age attenuates the transcriptional changes that occur with sleep in the medial prefrontal cortex. Aging Cell 18:e13021. doi: 10.1111/acel.13021
Halassa, M. M., Florian, C., Fellin, T., Munoz, J. R., Lee, S. Y., Abel, T., et al. (2009). Astrocytic modulation of sleep homeostasis and cognitive consequences of sleep loss. Neuron 61, 213–219. doi: 10.1016/j.neuron.2008.11.024
Hardy, J., and Selkoe, D. J. (2002). The amyloid hypothesis of Alzheimer’s disease: progress and problems on the road to therapeutics. Science 297, 353–356. doi: 10.1126/science.1072994
Heneka, M. T., Carson, M. J., Khoury, J. E., Landreth, G. E., Brosseron, F., Feinstein, D. L., et al. (2015). Neuroinflammation in Alzheimer’s disease. Lancet Neurol. 14, 388–405. doi: 10.1016/S1474-4422(15)70016-5
Ho, M. S. (2019). Microglia in Parkinson’s disease. Adv. Exp. Med. Biol. 1175, 335–353. doi: 10.1007/978-981-13-9913-8_13
Hopp, S. C., Lin, Y., Oakley, D., Roe, A. D., DeVos, S. L., Hanlon, D., et al. (2018). The role of microglia in processing and spreading of bioactive tau seeds in Alzheimer’s disease. J. Neuroinflammation 15:269. doi: 10.1186/s12974-018-1309-z
Huang, S., Liao, X., Wu, J., Zhang, X., Li, Y., Xiang, D., et al. (2022). The Microglial membrane receptor TREM2 mediates exosome secretion to promote phagocytosis of amyloid-β by microglia. FEBS Lett. 596, 1059–1071. doi: 10.1002/1873-3468.14336
Huang, Z. L., Zhang, Z., and Qu, W. M. (2014). Roles of adenosine and its receptors in sleep-wake regulation. Int. Rev. Neurobiol. 119, 349–371. doi: 10.1016/b978-0-12-801022-8.00014-3
Hurtado-Alvarado, G., Domínguez-Salazar, E., Velázquez-Moctezuma, J., and Gómez-González, B. (2016). A2A adenosine receptor antagonism reverts the blood-brain barrier dysfunction induced by sleep restriction. PLoS One 11:e0167236. doi: 10.1371/journal.pone.0167236
Inoue, K., and Tsuda, M. (2018). Microglia in neuropathic pain: cellular and molecular mechanisms and therapeutic potential. Nat. Rev. Neurosci. 19, 138–152. doi: 10.1038/nrn.2018.2
Irwin, M. R., Olmstead, R., and Carroll, J. E. (2016). Sleep disturbance, sleep duration, and inflammation: a systematic review and meta-analysis of cohort studies and experimental sleep deprivation. Biol. Psychiatry 80, 40–52. doi: 10.1016/j.biopsych.2015.05.014
Irwin, M. R., and Vitiello, M. V. (2019). Implications of sleep disturbance and inflammation for Alzheimer’s disease dementia. Lancet Neurol. 18, 296–306. doi: 10.1016/s1474-4422(18)30450-2
Jiang, T., Zhang, Y. D., Chen, Q., Gao, Q., Zhu, X. C., Zhou, J. S., et al. (2016). TREM2 modifies microglial phenotype and provides neuroprotection in P301S tau transgenic mice. Neuropharmacology 105, 196–206. doi: 10.1016/j.neuropharm.2016.01.028
Jiang, T., Zhang, Y. D., Gao, Q., Ou, Z., Gong, P. Y., Shi, J. Q., et al. (2018). TREM2 ameliorates neuronal tau pathology through suppression of microglial inflammatory response. Inflammation 41, 811–823. doi: 10.1007/s10753-018-0735-5
John, A., and Reddy, P. H. (2021). Synaptic basis of Alzheimer’s disease: focus on synaptic amyloid beta, P-tau and mitochondria. Ageing Res. Rev. 65, 101208. doi: 10.1016/j.arr.2020.101208
Kaneko, M., Stellwagen, D., Malenka, R. C., and Stryker, M. P. (2008). Tumor necrosis factor-alpha mediates one component of competitive, experience-dependent plasticity in developing visual cortex. Neuron 58, 673–680. doi: 10.1016/j.neuron.2008.04.023
Kang, J. E., Lim, M. M., Bateman, R. J., Lee, J. J., Smyth, L. P., Cirrito, J. R., et al. (2009). Amyloid-beta dynamics are regulated by orexin and the sleep-wake cycle. Science 326, 1005–1007. doi: 10.1126/science.1180962
Kfoury, N., Holmes, B. B., Jiang, H., Holtzman, D. M., and Diamond, M. I. (2012). Trans-cellular propagation of Tau aggregation by fibrillar species. J. Biol. Chem. 287, 19440–19451. doi: 10.1074/jbc.M112.346072
Kim, J., Basak, J. M., and Holtzman, D. M. (2009). The role of apolipoprotein E in Alzheimer’s disease. Neuron 63, 287–303. doi: 10.1016/j.neuron.2009.06.026
Koo, K. Y. G., Schweizer, T. A., Fischer, C. E., and Munoz, D. G. (2019). Abnormal sleep behaviours across the spectrum of Alzheimer’s disease severity: influence of APOE genotypes and lewy bodies. Curr. Alzheimer Res. 16, 243–250. doi: 10.2174/1567205016666190103161034
Kulczykowska, E., Kleszczyńska, A., Gozdowska, M., and Sokołowska, E. (2017). The time enzyme in melatonin biosynthesis in fish: day/night expressions of three aralkylamine N-acetyltransferase genes in three-spined stickleback. Comp. Biochem. Physiol. A Mol. Integr. Physiol. 208, 46–53. doi: 10.1016/j.cbpa.2017.03.005
Lane, C. A., Hardy, J., and Schott, J. M. (2018). Alzheimer’s disease. Eur. J. Neurol. 25, 59–70. doi: 10.1111/ene.13439
Lawson, L. J., Perry, V. H., Dri, P., and Gordon, S. (1990). Heterogeneity in the distribution and morphology of microglia in the normal adult mouse brain. Neuroscience 39, 151–170. doi: 10.1016/0306-4522(90)90229-w
Lazarus, M., Chen, J. F., Huang, Z. L., Urade, Y., and Fredholm, B. B. (2019). Adenosine and sleep. Handb. Exp. Pharmacol. 253, 359–381. doi: 10.1007/164_2017_36
Lee, E. Y., Srinivasan, Y., de Anda, J., Nicastro, L. K., Tükel, Ç, and Wong, G. C. L. (2020). Functional reciprocity of amyloids and antimicrobial peptides: rethinking the role of supramolecular assembly in host defense, immune activation, and inflammation. Front. Immunol. 11:1629. doi: 10.3389/fimmu.2020.01629
Liao, F., Yoon, H., and Kim, J. (2017). Apolipoprotein E metabolism and functions in brain and its role in Alzheimer’s disease. Curr. Opin. Lipidol. 28, 60–67. doi: 10.1097/mol.0000000000000383
Liu, B., and Hong, J.-S. (2003). Role of microglia in inflammation-mediated neurodegenerative diseases: mechanisms and strategies for therapeutic intervention. J. Pharmacol. Exp. Ther. 304, 1–7. doi: 10.1124/jpet.102.035048
Liu, P., Zhao, B., Wei, M., Li, Y., Liu, J., Ma, L., et al. (2020). Activation of inflammation is associated with amyloid-β accumulation induced by chronic sleep restriction in rats. J. Alzheimers Dis. 74, 759–773. doi: 10.3233/jad-191317
Liu, Z. W., and Gao, X. B. (2007). Adenosine inhibits activity of hypocretin/orexin neurons by the A1 receptor in the lateral hypothalamus: a possible sleep-promoting effect. J. Neurophysiol. 97, 837–848. doi: 10.1152/jn.00873.2006
Lucey, B. P., Wisch, J., Boerwinkle, A. H., Landsness, E. C., Toedebusch, C. D., McLeland, J. S., et al. (2021). Sleep and longitudinal cognitive performance in preclinical and early symptomatic Alzheimer’s disease. Brain 144, 2852–2862. doi: 10.1093/brain/awab272
Mahan, T. E., Wang, C., Bao, X., Choudhury, A., Ulrich, J. D., and Holtzman, D. M. (2022). Selective reduction of astrocyte apoE3 and apoE4 strongly reduces Aβ accumulation and plaque-related pathology in a mouse model of amyloidosis. Mol. Neurodegener. 17:13. doi: 10.1186/s13024-022-00516-0
Mahley, R. W. (2016). Central nervous system lipoproteins: ApoE and regulation of cholesterol metabolism. Arterioscler. Thromb. Vasc. Biol. 36, 1305–1315. doi: 10.1161/atvbaha.116.307023
Marcello, E., Musardo, S., Vandermeulen, L., Pelucchi, S., Gardoni, F., Santo, N., et al. (2019). Amyloid-β oligomers regulate ADAM10 synaptic localization through aberrant plasticity phenomena. Mol. Neurobiol. 56, 7136–7143. doi: 10.1007/s12035-019-1583-5
Marshall, L., and Born, J. (2002). Brain-immune interactions in sleep. Int. Rev. Neurobiol. 52, 93–131. doi: 10.1016/s0074-7742(02)52007-9
Matsumoto, S., and Tsunematsu, T. (2021). Association between Sleep, Alzheimer’s, and Parkinson’s disease. Biology 10:1127. doi: 10.3390/biology10111127
McCleery, J., and Sharpley, A. L. (2020). Pharmacotherapies for sleep disturbances in dementia. Cochrane Database Syst. Rev. 11:Cd009178. doi: 10.1002/14651858.CD009178.pub4
McNaull, B. B., Todd, S., McGuinness, B., and Passmore, A. P. (2010). Inflammation and anti-inflammatory strategies for Alzheimer’s disease–a mini-review. Gerontology 56, 3–14. doi: 10.1159/000237873
Moran, M., Lynch, C. A., Walsh, C., Coen, R., Coakley, D., and Lawlor, B. A. (2005). Sleep disturbance in mild to moderate Alzheimer’s disease. Sleep Med. 6, 347–352. doi: 10.1016/j.sleep.2004.12.005
Mulas, F., Rojas, M., and Gandía, R. (2019). [Sleep in neurodevelopmental disorders]. Medicina 79, (Suppl. 3), 33–36.
Muñoz, S. S., Garner, B., and Ooi, L. (2019). Understanding the role of ApoE fragments in Alzheimer’s disease. Neurochem. Res. 44, 1297–1305. doi: 10.1007/s11064-018-2629-1
Murphy, G. M., Yang, L., and Cordell, B. (1998). Macrophage colony-stimulating factor augments β-amyloid-induced interleukin-1, interleukin-6, and nitric oxide production by microglial cells. J. Biol. Chem. 273, 20967–20971. doi: 10.1074/jbc.273.33.20967
Nall, A., and Sehgal, A. (2014). Monoamines and sleep in Drosophila. Behav. Neurosci. 128, 264–272. doi: 10.1037/a0036209
Ng, A., Tam, W. W., Zhang, M. W., Ho, C. S., Husain, S. F., McIntyre, R. S., et al. (2018). IL-1β, IL-6, TNF- α and CRP in elderly patients with depression or Alzheimer’s disease: systematic review and meta-analysis. Sci. Rep. 8:12050. doi: 10.1038/s41598-018-30487-6
Oishi, Y., Huang, Z. L., Fredholm, B. B., Urade, Y., and Hayaishi, O. (2008). Adenosine in the tuberomammillary nucleus inhibits the histaminergic system via A1 receptors and promotes non-rapid eye movement sleep. Proc. Natl. Acad. Sci. U.S.A. 105, 19992–19997. doi: 10.1073/pnas.0810926105
Ooms, S., Overeem, S., Besse, K., Rikkert, M. O., Verbeek, M., and Claassen, J. A. (2014). Effect of 1 night of total sleep deprivation on cerebrospinal fluid β-amyloid 42 in healthy middle-aged men: a randomized clinical trial. JAMA Neurol. 71, 971–977. doi: 10.1001/jamaneurol.2014.1173
Parhizkar, S., and Holtzman, D. M. (2022). APOE mediated neuroinflammation and neurodegeneration in Alzheimer’s disease. Semin. Immunol. [Epub ahead of print]. doi: 10.1016/j.smim.2022.101594
Partch, C. L., Green, C. B., and Takahashi, J. S. (2014). Molecular architecture of the mammalian circadian clock. Trends Cell Biol. 24, 90–99. doi: 10.1016/j.tcb.2013.07.002
Peng, W., Wu, Z., Song, K., Zhang, S., Li, Y., and Xu, M. (2020). Regulation of sleep homeostasis mediator adenosine by basal forebrain glutamatergic neurons. Science 369:eabb0556. doi: 10.1126/science.abb0556
Pereira, G. S., Rossato, J. I., Sarkis, J. J. F., Cammarota, M., Bonan, C. D., and Izquierdo, I. (2005). Activation of adenosine receptors in the posterior cingulate cortex impairs memory retrieval in the rat. Neurobiol. Learn. Mem. 83, 217–223. doi: 10.1016/j.nlm.2004.12.002
Perlmutter, L. S., Scott, S. A., Barrón, E., and Chui, H. C. (1992). MHC class II-positive microglia in human brain: association with Alzheimer lesions. J. Neurosci. Res. 33, 549–558. doi: 10.1002/jnr.490330407
Pfrieger, F. W. (2003). Cholesterol homeostasis and function in neurons of the central nervous system. Cell. Mol. Life Sci. 60, 1158–1171. doi: 10.1007/s00018-003-3018-7
Porkka-Heiskanen, T., Strecker, R. E., Thakkar, M., Bjorkum, A. A., Greene, R. W., and McCarley, R. W. (1997). Adenosine: a mediator of the sleep-inducing effects of prolonged wakefulness. Science 276, 1265–1268. doi: 10.1126/science.276.5316.1265
Portas, C. M., Thakkar, M., Rainnie, D. G., Greene, R. W., and McCarley, R. W. (1997). Role of adenosine in behavioral state modulation: a microdialysis study in the freely moving cat. Neuroscience 79, 225–235. doi: 10.1016/S0306-4522(96)00640-9
Potter, G. D. M., Skene, D. J., Arendt, J., Cade, J. E., Grant, P. J., and Hardie, L. J. (2016). Circadian rhythm and sleep disruption: causes, metabolic consequences, and countermeasures. Endocr. Rev. 37, 584–608. doi: 10.1210/er.2016-1083
Prasad, H., and Rao, R. (2018). Amyloid clearance defect in ApoE4 astrocytes is reversed by epigenetic correction of endosomal pH. Proc. Natl. Acad. Sci. U.S.A. 115, E6640–E6649. doi: 10.1073/pnas.1801612115
Prokop, S., Miller, K. R., and Heppner, F. L. (2013). Microglia actions in Alzheimer’s disease. Acta Neuropathol. 126, 461–477. doi: 10.1007/s00401-013-1182-x
Pyun, J. M., Kang, M. J., Yun, Y., Park, Y. H., and Kim, S. (2019). APOE ε4 and REM sleep behavior disorder as risk factors for sundown syndrome in Alzheimer’s disease. J. Alzheimers Dis. 69, 521–528. doi: 10.3233/jad-190032
Qiu, C., Wang, M., Yu, W., Rong, Z., Zheng, H. S., Sun, T., et al. (2021). Activation of the hippocampal LXRβ improves sleep-deprived cognitive impairment by inhibiting neuroinflammation. Mol. Neurobiol. 58, 5272–5288. doi: 10.1007/s12035-021-02446-2
Rainnie, D. G., Grunze, H. C., McCarley, R. W., and Greene, R. W. (1994). Adenosine inhibition of mesopontine cholinergic neurons: implications for EEG arousal. Science 263, 689–692. doi: 10.1126/science.8303279
Rauchs, G., Schabus, M., Parapatics, S., Bertran, F., Clochon, P., Hot, P., et al. (2008). Is there a link between sleep changes and memory in Alzheimer’s disease? Neuroreport 19, 1159–1162. doi: 10.1097/wnr.0b013e32830867c4
Rechtschaffen, A., Bergmann, B. M., Everson, C. A., Kushida, C. A., and Gilliland, M. A. (1989). Sleep deprivation in the rat: X. Integration and discussion of the findings. Sleep 12, 68–87.
Rico-Rosillo, M. G., and Vega-Robledo, G. B. (2018). [Sleep and immune system]. Rev. Alerg. Mex. 65, 160–170. doi: 10.29262/ram.v65i2.359
Ries, M., and Sastre, M. (2016). Mechanisms of Aβ clearance and degradation by glial cells. Front. Aging Neurosci. 8:160. doi: 10.3389/fnagi.2016.00160
Rozemuller, J. M., der Valk, P. V., and Eikelenboom, P. (1992). Activated microglia and cerebral amyloid deposits in alzheimer’s disease. Res. Immunol. 143, 646–649. doi: 10.1016/0923-2494(92)80050-U
Salter, M. W., and Stevens, B. (2017). Microglia emerge as central players in brain disease. Nat. Med. 23, 1018–1027. doi: 10.1038/nm.4397
Satoh, S., Matsumura, H., Suzuki, F., and Hayaishi, O. (1996). Promotion of sleep mediated by the A2a-adenosine receptor and possible involvement of this receptor in the sleep induced by prostaglandin D2 in rats. Proc. Natl. Acad. Sci. U.S.A. 93, 5980–5984. doi: 10.1073/pnas.93.12.5980
Satterfield, B. C., Wisor, J. P., Field, S. A., Schmidt, M. A., and Van Dongen, H. P. (2015). TNFα G308A polymorphism is associated with resilience to sleep deprivation-induced psychomotor vigilance performance impairment in healthy young adults. Brain Behav. Immun. 47, 66–74. doi: 10.1016/j.bbi.2014.12.009
Sbai, O., Djelloul, M., Auletta, A., Ieraci, A., Vascotto, C., and Perrone, L. (2022). AGE-TXNIP axis drives inflammation in Alzheimer’s by targeting Aβ to mitochondria in microglia. Cell Death Dis. 13:302. doi: 10.1038/s41419-022-04758-0
Scammell, T. E., Arrigoni, E., and Lipton, J. O. (2017). Neural circuitry of wakefulness and sleep. Neuron 93, 747–765. doi: 10.1016/j.neuron.2017.01.014
Schwabe, T., Srinivasan, K., and Rhinn, H. (2020). Shifting paradigms: the central role of microglia in Alzheimer’s disease. Neurobiol. Dis. 143:104962. doi: 10.1016/j.nbd.2020.104962
Sellgren, C. M., Gracias, J., Watmuff, B., Biag, J. D., Thanos, J. M., Whittredge, P. B., et al. (2019). Increased synapse elimination by microglia in schizophrenia patient-derived models of synaptic pruning. Nat. Neurosci. 22, 374–385. doi: 10.1038/s41593-018-0334-7
Serrano-Pozo, A., Qian, J., Monsell, S. E., Betensky, R. A., and Hyman, B. T. (2015). APOEε2 is associated with milder clinical and pathological Alzheimer disease. Ann. Neurol. 77, 917–929. doi: 10.1002/ana.24369
Shankar, G. M., Li, S., Mehta, T. H., Garcia-Munoz, A., Shepardson, N. E., Smith, I., et al. (2008). Amyloid-beta protein dimers isolated directly from Alzheimer’s brains impair synaptic plasticity and memory. Nat. Med. 14, 837–842. doi: 10.1038/nm1782
Sharma, A., Sethi, G., Tambuwala, M. M., Aljabali, A. A. A., Chellappan, D. K., Dua, K., et al. (2021). Circadian rhythm disruption and Alzheimer’s disease: the dynamics of a vicious cycle. Curr. Neuropharmacol. 19, 248–264. doi: 10.2174/1570159x18666200429013041
Shokri-Kojori, E., Wang, G. J., Wiers, C. E., Demiral, S. B., Guo, M., Kim, S. W., et al. (2018). β-Amyloid accumulation in the human brain after one night of sleep deprivation. Proc. Natl. Acad. Sci. U.S.A. 115, 4483–4488. doi: 10.1073/pnas.1721694115
Siegel, J. M. (2008). Do all animals sleep? Trends Neurosci. 31, 208–213. doi: 10.1016/j.tins.2008.02.001
Spinedi, E., and Cardinali, D. P. (2019). Neuroendocrine-metabolic dysfunction and sleep disturbances in neurodegenerative disorders: focus on Alzheimer’s disease and melatonin. Neuroendocrinology 108, 354–364. doi: 10.1159/000494889
Sunkaria, A., and Bhardwaj, S. (2022). Sleep disturbance and Alzheimer’s disease: the glial connection. Neurochem. Res. [Epub ahead of print]. doi: 10.1007/s11064-022-03578-0
Tabuchi, M., Lone, S. R., Liu, S., Liu, Q., Zhang, J., Spira, A. P., et al. (2015). Sleep interacts with aβ to modulate intrinsic neuronal excitability. Curr. Biol. 25, 702–712. doi: 10.1016/j.cub.2015.01.016
Taipa, R., das Neves, S. P., Sousa, A. L., Fernandes, J., Pinto, C., Correia, A. P., et al. (2019). Proinflammatory and anti-inflammatory cytokines in the CSF of patients with Alzheimer’s disease and their correlation with cognitive decline. Neurobiol. Aging 76, 125–132. doi: 10.1016/j.neurobiolaging.2018.12.019
Tesoriero, C., Del Gallo, F., and Bentivoglio, M. (2019). Sleep and brain infections. Brain Res. Bull. 145, 59–74. doi: 10.1016/j.brainresbull.2018.07.002
Torres, A. K., Jara, C., Park-Kang, H. S., Polanco, C. M., Tapia, D., Alarcón, F., et al. (2021). Synaptic mitochondria: an early target of amyloid-β and tau in Alzheimer’s disease. J. Alzheimers Dis. 84, 1391–1414. doi: 10.3233/jad-215139
Tsai, C. J., Nagata, T., Liu, C. Y., Suganuma, T., Kanda, T., Miyazaki, T., et al. (2021). Cerebral capillary blood flow upsurge during REM sleep is mediated by A2a receptors. Cell Rep. 36:109558. doi: 10.1016/j.celrep.2021.109558
Tuan, L.-H., and Lee, L.-J. (2019). Microglia-mediated synaptic pruning is impaired in sleep-deprived adolescent mice. Neurobiol. Dis. 130:104517. doi: 10.1016/j.nbd.2019.104517
Turrin, N. P., and Rivest, S. (2004). Innate immune reaction in response to seizures: implications for the neuropathology associated with epilepsy. Neurobiol. Dis. 16, 321–334. doi: 10.1016/j.nbd.2004.03.010
Uddin, M. S., Kabir, M. T., Al Mamun, A., Abdel-Daim, M. M., Barreto, G. E., and Ashraf, G. M. (2019). APOE and Alzheimer’s disease: evidence mounts that targeting APOE4 may combat Alzheimer’s pathogenesis. Mol. Neurobiol. 56, 2450–2465. doi: 10.1007/s12035-018-1237-z
Uddin, M. S., Tewari, D., Mamun, A. A., Kabir, M. T., Niaz, K., Wahed, M. I. I., et al. (2020). Circadian and sleep dysfunction in Alzheimer’s disease. Ageing Res. Rev. 60:101046. doi: 10.1016/j.arr.2020.101046
Urade, Y., Eguchi, N., Qu, W. M., Sakata, M., Huang, Z. L., Chen, J. F., et al. (2003). Sleep regulation in adenosine A2A receptor-deficient mice. Neurology 61(11 Suppl. 6), S94–S96. doi: 10.1212/01.wnl.0000095222.41066.5e
Urry, E., and Landolt, H. P. (2015). Adenosine, caffeine, and performance: from cognitive neuroscience of sleep to sleep pharmacogenetics. Curr. Top. Behav. Neurosci. 25, 331–366. doi: 10.1007/7854_2014_274
Vaidyanathan, T. V., Collard, M., Yokoyama, S., Reitman, M. E., and Poskanzer, K. E. (2021). Cortical astrocytes independently regulate sleep depth and duration via separate GPCR pathways. eLife 10:e63329. doi: 10.7554/eLife.63329
van Calker, D., Biber, K., Domschke, K., and Serchov, T. (2019). The role of adenosine receptors in mood and anxiety disorders. J. Neurochem. 151, 11–27. doi: 10.1111/jnc.14841
van der Kant, R., Goldstein, L. S. B., and Ossenkoppele, R. (2020). Amyloid-β-independent regulators of tau pathology in Alzheimer disease. Nat. Rev. Neurosci. 21, 21–35. doi: 10.1038/s41583-019-0240-3
Van Egroo, M., Narbutas, J., Chylinski, D., Villar González, P., Maquet, P., Salmon, E., et al. (2019). Sleep-wake regulation and the hallmarks of the pathogenesis of Alzheimer’s disease. Sleep 42:zsz017. doi: 10.1093/sleep/zsz017
Vanderheyden, W. M., Goodman, A. G., Taylor, R. H., Frank, M. G., Van Dongen, H. P. A., and Gerstner, J. R. (2018a). Astrocyte expression of the Drosophila TNF-alpha homologue, Eiger, regulates sleep in flies. PLoS Genet. 14:e1007724. doi: 10.1371/journal.pgen.1007724
Vanderheyden, W. M., Lim, M. M., Musiek, E. S., and Gerstner, J. R. (2018b). Alzheimer’s disease and sleep-wake disturbances: amyloid, astrocytes, and animal models. J. Neurosci. 38, 2901–2910. doi: 10.1523/jneurosci.1135-17.2017
Veatch, O. J., Keenan, B. T., Gehrman, P. R., Malow, B. A., and Pack, A. I. (2017). Pleiotropic genetic effects influencing sleep and neurological disorders. Lancet Neurol. 16, 158–170. doi: 10.1016/s1474-4422(16)30339-8
Verghese, P. B., Castellano, J. M., Garai, K., Wang, Y., Jiang, H., Shah, A., et al. (2013). ApoE influences amyloid-β (Aβ) clearance despite minimal apoE/Aβ association in physiological conditions. Proc. Natl. Acad. Sci. U.S.A. 110, E1807–E1816. doi: 10.1073/pnas.1220484110
Vitiello, M. V., Bliwise, D. L., and Prinz, P. N. (1992). Sleep in Alzheimer’s disease and the sundown syndrome. Neurology 42(7 Suppl. 6), 83–93; discussion 93–94.
Vogels, T., Murgoci, A.-N., and Hromádka, T. (2019). Intersection of pathological tau and microglia at the synapse. Acta Neuropathol. Commun. 7:109. doi: 10.1186/s40478-019-0754-y
Volicer, L., Harper, D. G., Manning, B. C., Goldstein, R., and Satlin, A. (2001). Sundowning and circadian rhythms in Alzheimer’s disease. Am. J. Psychiatry 158, 704–711. doi: 10.1176/appi.ajp.158.5.704
Wang, C., and Holtzman, D. M. (2020). Bidirectional relationship between sleep and Alzheimer’s disease: role of amyloid, tau, and other factors. Neuropsychopharmacology 45, 104–120. doi: 10.1038/s41386-019-0478-5
Wang, C., Xiong, M., Gratuze, M., Bao, X., Shi, Y., Andhey, P. S., et al. (2021). Selective removal of astrocytic APOE4 strongly protects against tau-mediated neurodegeneration and decreases synaptic phagocytosis by microglia. Neuron 109, 1657–1674.e7. doi: 10.1016/j.neuron.2021.03.024
Weil, Z. M., Norman, G. J., Karelina, K., Morris, J. S., Barker, J. M., Su, A. J., et al. (2009). Sleep deprivation attenuates inflammatory responses and ischemic cell death. Exp. Neurol. 218, 129–136. doi: 10.1016/j.expneurol.2009.04.018
Wood, L. B., Winslow, A. R., Proctor, E. A., McGuone, D., Mordes, D. A., Frosch, M. P., et al. (2015). Identification of neurotoxic cytokines by profiling Alzheimer’s disease tissues and neuron culture viability screening. Sci. Rep. 5:16622. doi: 10.1038/srep16622
Wright, K. P. Jr., Drake, A. L., Frey, D. J., Fleshner, M., Desouza, C. A., Gronfier, C., et al. (2015). Influence of sleep deprivation and circadian misalignment on cortisol, inflammatory markers, and cytokine balance. Brain Behav. Immun. 47, 24–34. doi: 10.1016/j.bbi.2015.01.004
Wu, H., Dunnett, S., Ho, Y. S., and Chang, R. C. (2019). The role of sleep deprivation and circadian rhythm disruption as risk factors of Alzheimer’s disease. Front. Neuroendocrinol. 54:100764. doi: 10.1016/j.yfrne.2019.100764
Wu, K. M., Zhang, Y. R., Huang, Y. Y., Dong, Q., Tan, L., and Yu, J. T. (2021). The role of the immune system in Alzheimer’s disease. Ageing Res. Rev. 70:101409. doi: 10.1016/j.arr.2021.101409
Xin, J., Wang, C., Cheng, X., Xie, C., Zhang, Q., Ke, Y., et al. (2021). CX3C-chemokine receptor 1 modulates cognitive dysfunction induced by sleep deprivation. Chin. Med. J. 135, 205–215. doi: 10.1097/cm9.0000000000001769
Yamasu, K., Shimada, Y., Sakaizumi, M., Soma, G., and Mizuno, D. (1992). Activation of the systemic production of tumor necrosis factor after exposure to acute stress. Eur. Cytokine Netw. 3, 391–398.
Ye, L., Huang, Y., Zhao, L., Li, Y., Sun, L., Zhou, Y., et al. (2013). IL-1β and TNF-α induce neurotoxicity through glutamate production: a potential role for neuronal glutaminase. J. Neurochem. 125, 897–908. doi: 10.1111/jnc.12263
Yu, J., Cho, E., Kwon, H., Jeon, J., Seong Sin, J., Kwon Park, J., et al. (2021). Akt and calcium-permeable AMPA receptor are involved in the effect of pinoresinol on amyloid β-induced synaptic plasticity and memory deficits. Biochem. Pharmacol. 184:114366. doi: 10.1016/j.bcp.2020.114366
Zhao, B., Liu, P., Wei, M., Li, Y., Liu, J., Ma, L., et al. (2019). Chronic sleep restriction induces Aβ accumulation by disrupting the balance of Aβ production and clearance in rats. Neurochem. Res. 44, 859–873. doi: 10.1007/s11064-019-02719-2
Zhao, H., Wu, H., He, J., Zhuang, J., Liu, Z., Yang, Y., et al. (2016). Frontal cortical mitochondrial dysfunction and mitochondria-related β-amyloid accumulation by chronic sleep restriction in mice. Neuroreport 27, 916–922. doi: 10.1097/wnr.0000000000000631
Keywords: sleep disturbance, Alzheimer’s disease, astrocyte, microglia, amyloid beta
Citation: Xiao S-Y, Liu Y-J, Lu W, Sha Z-W, Xu C, Yu Z-H and Lee S-D (2022) Possible Neuropathology of Sleep Disturbance Linking to Alzheimer’s Disease: Astrocytic and Microglial Roles. Front. Cell. Neurosci. 16:875138. doi: 10.3389/fncel.2022.875138
Received: 13 February 2022; Accepted: 10 May 2022;
Published: 09 June 2022.
Edited by:
Oscar Gonzalez-Perez, University of Colima, MexicoReviewed by:
Stephen Beesley, Florida State University, United StatesRocio Elizabeth Gonzalez-Castañeda, University of Guadalajara, Mexico
Copyright © 2022 Xiao, Liu, Lu, Sha, Xu, Yu and Lee. This is an open-access article distributed under the terms of the Creative Commons Attribution License (CC BY). The use, distribution or reproduction in other forums is permitted, provided the original author(s) and the copyright owner(s) are credited and that the original publication in this journal is cited, in accordance with accepted academic practice. No use, distribution or reproduction is permitted which does not comply with these terms.
*Correspondence: Shin-Da Lee, shinda@mail.cmu.edu.tw
†ORCID: Shu-Yun Xiao, orcid.org/0000-0003-1803-4244; Yi-Jie Liu, orcid.org/0000-0001-5417-2287; Wang Lu, orcid.org/0000-0001-6107-305X; Zhong-Wei Sha, orcid.org/0000-0002-5359-758X; Che Xu, orcid.org/0000-0001-7399-1528; Zhi-Hua Yu, orcid.org/0000-0001-5326-5545; Shin-Da Lee, orcid.org/0000-0002-8393-8349
‡These authors have contributed equally to this work