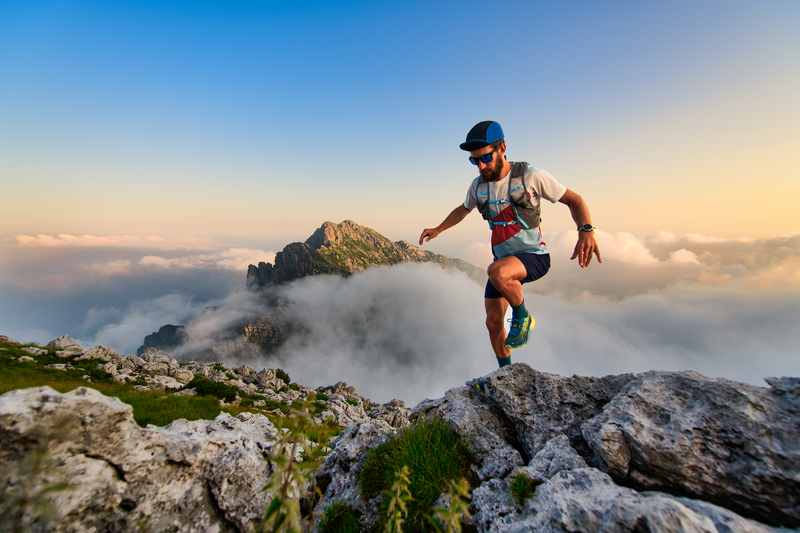
95% of researchers rate our articles as excellent or good
Learn more about the work of our research integrity team to safeguard the quality of each article we publish.
Find out more
REVIEW article
Front. Cell. Neurosci. , 07 March 2022
Sec. Non-Neuronal Cells
Volume 16 - 2022 | https://doi.org/10.3389/fncel.2022.850866
This article is part of the Research Topic The Role of Astrocytes in Stroke View all 7 articles
Astrocytes are essential in maintaining normal brain functions such as blood brain barrier (BBB) homeostasis and synapse formation as the most abundant cell type in the central nervous system (CNS). After the stroke, astrocytes are known as reactive astrocytes (RAs) because they are stimulated by various damage-associated molecular patterns (DAMPs) and cytokines, resulting in significant changes in their reactivity, gene expression, and functional characteristics. RAs perform multiple functions after stroke. The inflammatory response of RAs may aggravate neuro-inflammation and release toxic factors to exert neurological damage. However, RAs also reduce excitotoxicity and release neurotrophies to promote neuroprotection. Furthermore, RAs contribute to angiogenesis and axonal remodeling to promote neurological recovery. Therefore, RAs’ biphasic roles and mechanisms make them an effective target for functional recovery after the stroke. In this review, we summarized the dynamic functional changes and internal molecular mechanisms of RAs, as well as their therapeutic potential and strategies, in order to comprehensively understand the role of RAs in the outcome of stroke disease and provide a new direction for the clinical treatment of stroke.
Stroke is an acute cerebrovascular disease in which the blood cannot supply the brain generally due to the sudden rupture or blockage of the cerebrovascular. In 2019, stroke resulted in the death of 6.55 million people worldwide, accounting for 11.6% of the total number of deaths. About 3/4 of stroke survivors have neurological disorders of varying degrees, and 15–30% of patients are permanently disabled (Lai et al., 2002; Wu et al., 2019; GBD 2019 Stroke Collaborators, 2021). Therefore, stroke has been the second cause of death and the leading cause of severe disability globally. After the stroke, damage factors such as blood components infiltrating into the brain parenchyma, necrotic cell debris, and reactive oxygen species (ROS) often lead to neuronal degeneration or apoptosis, increasing permeability of BBB, inflammatory response, and brain edema (Iadecola et al., 2020). This is likewise the main reason for the high disability rate of stroke patients. Thus, finding effective intervention methods to control secondary brain injury (SBI) has become a vital goal to improve the prognosis of stroke patients (Chamorro et al., 2016; Lambertsen et al., 2019).
After the stroke, SBI involves vascular endothelial cells, neurons, glial cells, and other cell types, which interact to jointly determine the damage response’s occurrence, development, and outcome. Astrocytes are the most abundant subtype among these cells, highly expressing the interleukin-1 receptor (IL-1R), tumor necrosis factor receptor (TNFR), interferon-γ receptor (IFN-γR), and other inflammatory signal receptors (Lovatt et al., 2007; Wang et al., 2019), so they are one of the cells targeted for inflammatory factors. Moreover, astrocytes can also secrete CXCL12, CCl2, C1q, and other inflammatory factors to aggravate SBI (Yamada et al., 1992). Therefore, astrocytes are likely to be important targets for regulating SBI. But what are the possible roles and mechanisms of astrocytes in SBI?
Accumulating evidence supports that astrocyte undergoes significant changes after the stroke in morphology, gene expression, and cell proliferation, and these cells are known as RAs (Zisis et al., 2021). Currently, it is known that the RAs’ effects may include scarring, neurotrophic factor secretion, BBB damaging and repairing, and inhibition of synaptic formation. However, the formation and mechanisms of RAs have yet to be clearly understood. This review focuses mainly on the morphological and phenotypic changes of astrocytes after the stroke and analyses the molecular biological mechanisms behind them, reveals the pathologic mode of RAs, summarizes the application of new techniques in RAs, and outlines the therapeutic potential of astrocytes in stroke. We expect to find a comprehensive treatment strategy to reduce stroke SBI and support patient recovery based on an in-depth understanding of RAs.
After the stroke, astrocytes express multiple Toll-like receptors (TLR), including TLR4, TLR2, and TLR3, which respond to distress-associated molecular patterns (DAMPs) released by injured cells (Sofroniew, 2014; Wang et al., 2019). Besides, neurotransmitters released by damaged neurons and pro-inflammatory factors secreted by adjacent cells act together on astrocytes, leading to significant changes in astrocyte morphology, molecular expression, and other levels (Johnston, 2005; Lan et al., 2017). To distinguish them from resting astrocytes, the drastically altered astrocytes are named RAs.
Morphological changes of RAs are the most observable changes in stroke. A typical mature astrocyte has an oval body and several main branches, each divided into secondary and tertiary branches, which in turn are divided into fine processes. Each astrocyte occupies a separate, non-overlapping region in the adult brain, where astrocyte processes interact with synapses to form a delicate and complex network of processes. However, the relatively independent relationship between processes was broken after the stroke. Huang et al. (2014) found that hypertrophic processes of reactive astrocytes were overlapping and crisscrossed in the surrounding area of ischemic infarction, forming a capsule layer to cover the Infarction area. The morphological changes of RAs are spatially different. The diameter, length, and branching levels of RAs processes in the ischemic penumbra were higher than those in the contralateral hemisphere and distal astrocytes far from the ischemic core. Furthermore, the volume and branching structure of processes increased with decreasing distance from the lesion (Wagner et al., 2013). The morphological changes of RAs were likewise time-dynamic. Li induced photothrombosis-induced ischemia in mice and found that the expression level of glial fibrillary acidic protein (GFAP) in RAs was significantly increased with the progressive hypertrophy of processes in the early stage of injury, but the length of RAs did not change markedly. On the sixth day, RAs were densely packed, and their processes form linear structures and extend toward the ischemic core. After 10 days, processes extend further and RAs transition to scar-forming astrocytes, which wrap around the damaged area and separate it from normal tissue (Li et al., 2014). Astrocyte scar formation is an imperative feature of RAs’ morphologic changes after stroke. RAs secret a large amount of chondroitin sulfate proteoglycan (CSPG) and other extracellular matrix components, which together with microglia, oligodendrocytes, and other cellular components form glial scars. The glial scar can wrap around the injury site, limiting further leakage of blood components and monocyte infiltration, thereby inhibiting progressive enlargement of the ischemic penumbra (Yang, 2020). The duration of the astrocyte scar is related to the severity of the injury. Mild astrocyte activation may subside over time. In more severe cases, RAs form permanent glial scars, which inhibit axonal regeneration and are associated with poor neurological recovery after the stroke (Buss et al., 2004; Escartin et al., 2019).
Changes in the main processes of RAs during stroke have been carried out relatively detailed. Whereas limited by cell imaging technology, there is still a lack of relevant studies on the ultrastructural morphological changes of RAs endfeet and adjacent cells such as neurons and vascular endothelial cells at all stages of stroke. It is up to later researchers to complete these researches and fill in these gaps.
The original definition of RAs was closely related to GFAP (Eng et al., 1971). GFAP is one of the major components of astrocytes’ intermediate filaments. GFAP mRNA and protein expression were significantly up-regulated in astrocytes under various injury factors, so GFAP was considered a universal marker of RAs in initial studies. However, Sun found that GFAP could only stain 15% of individual astrocytes by single-cell dye injection, proving that GFAP is not an absolute marker of astrocytes (Sun and Jakobs, 2012). GFAP was also not strictly correlated with astrocyte reactivity. the expression level of GFAP in the suprachiasmatic nucleus fluctuated with circadian rhythm, suggesting that the changes of GFAP expression may be related to normal physiological functions (Gerics et al., 2006). These indicated that GFAP could not be fully and accurately reflect the morphology and reaction state of RAs, and its role as a general marker of RAs was limited.
Recent transcription studies have identified many astrocytes specific genes. Cahoy used fluorescence-activated cell sorting (FACS) to isolate astrocytes from S100B-EGFP Reporter mice and microarray analysis of over 20,000 genes at different postnatal ages from day 1 to 30. It was found that Aldh1L1 was more widely expressed in astrocytes than GFAP (Cahoy et al., 2008). Previous literature report that astrocytes almost entirely express SOX9 expression in the adult brain and that its expression is not down-regulated in aging astrocytes. Notably, SOX9 expression increases with astrocyte activation in multiple disease models (Sun et al., 2017). Compared with GFAP, these markers are more specific for some types of RAs and have been widely used in the studies of various CNS diseases. Zhang and Lundquist both used SOX9 as a marker of RAs in their studies (Lundquist et al., 2019; Zhang Q. et al., 2021). Götz’s study on brain injury and Michalovicz’s study on the neurotoxicity of RAs also used Aldh1L1 to specifically label RAs (Michalovicz et al., 2019; Götz et al., 2021).
Based on current studies, these markers have shown excellent performance in studying RAs. The development of single-cell sequencing technology also holds out the hope of finding new markers with higher specificity and sensitivity. In addition, we need to explore the change characteristics of different markers in different lesion sites and disease stages and build a comprehensive and efficient RAs marker system to serve for future research on RAs in stroke.
Previous studies have discovered that RAs activate multiple signaling pathways and have complex molecular expression patterns, with opposite or similar functional states. According to the functional characteristics, RAs can be divided into two types, namely, neuroinflammatory type induced by lipopolysaccharide and neuroprotective type induced by ischemic stroke (Zamanian et al., 2012). This is analogous to microglia changing their phenotypes and functions according to environmental changes (Loane and Kumar, 2016; Bai et al., 2020). Therefore, referring to the M1/M2-like polarization classification of microglia, researchers classified RAs into C3d+/GFAP+ A1 and S100A10+/GFAP+ A2 phenotypes (Liddelow and Barres, 2017; Zhang H. et al., 2021). Since then, the study of RAs has been incorporated into the A1/A2 paradigm, and a series of A1/A2 related genes and marker molecules have been proposed (see Table 1). In general, the NF-κB signaling pathway mediates the formation of neuroinflammatory astrocytes, which damage neurons by various ways, such as upregulating complement cascade genes, secreting inflammatory cytokines and saturated fatty acids that induce neuronal apoptosis, and reducing the secretion of GPCG4/6, SPARCL1, and ThBS1/2 with neurotrophic function (Stevens et al., 2007; Liddelow et al., 2017; Guttenplan et al., 2021). In contrast, neuroprotective type astrocytes mediated by JAK2/STAT3 signaling pathway up-regulate many neurotrophic factors and promote neuronal survival and growth (Zhu et al., 2013; Rakers et al., 2019), suggesting that this type of astrocytes may have a “beneficial” repair function.
However, it should be realized that the A1/A2 paradigm is a simple and questionable classification method. On the one hand, we do not fully understand the function of A1/A2 astrocytes. Depletion of C3d+/GFAP+ A1 aggravated cortical degeneration after moderate controlled cortical impact (CCI) (Myer et al., 2006). At the same time, neuron-derived neurotrophic factor (NDNF) released by S100A10+/GFAP+ A2 can be bound to the P75(NTR) receptor to induce neuronal apoptosis (Lee et al., 2001). On the other hand, RAs may have a more detailed phenotypic classification. In recent years, with the development of single-cell sequencing technology, RAs has shown significant heterogeneity at the transcriptomic level in senility (Boisvert et al., 2018) and many CNS diseases such as Huntington’s Disease, Alzheimer’s disease, and chronic traumatic brain injury (Al-Dalahmah et al., 2020; Pan et al., 2020; Chancellor et al., 2021). Particularly, Hasel’s recent single-cell sequencing of LPS-induced RAs revealed that RAs could be divided into nine subpopulations with distinct gene expression and some subgroups appear in different CNS diseases (Hasel et al., 2021). In the study of ischemic stroke, astrocytes in ischemic penumbra can be divided into seven subgroups and proposed related pathological mechanisms and therapeutic targets (Guo et al., 2021). The spatiotemporal heterogeneity of RAs brings us many questions. First, why do astrocytes show different activation states when receiving the same stimulus at the same time? Second, what triggers turnover between different phenotypes? Finally, is the phenotypic turnover over time due to the transformation of an “old phenotype” into a “new phenotype,” or to the activation of inactive astrocytes into a “new phenotype?”
Therefore, we speculated that RAs might have a more complex model of action in stroke. For example, (1) In the normal brain, astrocytes may have a pre-state of nerve injury or nerve protection. (2) A1 and A2 may be subdivided into more detailed subtypes or there may be new phenotypes beyond A1 and A2. (3) The activation state of RAs may be related to the degree, time, and location of the injury. In order to answer and verify these questions and hypotheses, we need to collect comprehensive multidimensional data of RAs using multiple techniques in the future to establish dynamic models of RAs phenotypes and identify specific functional patterns of different RAs.
As a neurovascular unit (NVU) component, astrocytes interact with most of the cellular components of the CNS by extending many fine processes. Consequently, astrocytes can serve as bridges between different cells to regulate the transmission of information and substances and to support the CNS functioning normally such as maintenance of internal environment homeostasis, BBB conservation, and neuronal signaling modulation (Simard et al., 2003). After the stroke, a series of cellular and molecular events induced by the breakthrough of blood components into the brain parenchyma or the sudden interruption of blood flow and subsequent reperfusion dramatically alter the astrocyte’s support function. In response to injury, the gene expression level of astrocytes changes observably, and a large number of bioactive molecules are transcribed, translated, assembled, and transported to the membrane or extracellular. These biomolecules not limit the damage scale and promote the removal of necrotic material but also lead to “secondary damage” that aggravates pathological reactions.
After the occurrence of stroke, drastic changes in the internal environment stimulate the injury and the surrounding area cells to release a large number of inflammatory factors (see Table 2), forming an “inflammatory factor storm.” RAs are both the upstream cell and the target cell of inflammatory cytokines and may be involved in the occurrence and elimination of the inflammatory response through various pathways. Our study found that proteinase-activated receptor 4 (PAR4) expressed on the cell membrane of astrocytes detects thrombin released by tissues and activates Tab2/NF-κB signaling pathway to initiate inflammatory injury mechanism (Luo et al., 2021). In addition, RAs produces and releases a large number of inflammatory mediators [such as TNF-α, IL-1α and IFN-γ (Nayak et al., 2012; Doll et al., 2015)] and free radicals [such as NO, superoxide dismutase and peroxynitrite (Gouix et al., 2014)], which directly or indirectly induce neuroinflammation leading to neuronal apoptosis and necrotic death. RAs also secret anti-inflammatory cytokines that inhibit the inflammatory process. Peng et al. (2020) found that DJ-1 (also known as Park7) is highly expressed in astrocytes around the infarct area, and DJ-1 negatively regulates the inflammatory response by promoting the interaction between SHP-1 and TRAF6, thus inducing the dissociation of NLRX1 and TRAF6. Mtlik et al. observed upregulation of mesencephalic astrocyte-derived neurotrophic factor (MANF) and brain dopamine neurotrophic factor (CDNF) in animal stroke models, which can prevent ER stress-induced overactivation and inhibit secretion of pro-inflammatory cytokines IL-1β, TNF-α, and IL-6 (Shen et al., 2012; Cheng et al., 2013; Zhao et al., 2013; Mätlik et al., 2018).
As a bridge between many cell types, astrocytes’ involvement in regulating inflammatory responses is closely related to their interactions with them and other cell types. In previous studies, for instance, this process was considered inseparable from astrocyte and microglia reciprocal action (Sacristán, 2020). Microglia can initiate inflammatory astrocyte activation. In experimental and human stroke, IL-6, IL-1α, and TNFα are abundantly secreted by microglia, these inflammatory factors promoted the transformation of astrocytes to neuroinflammation phenotype, which lost the ability to promote neuronal survival and growth, synaptogenesis, and phagocytosis as well as induced the death of neurons and oligodendrocytes (Lambertsen et al., 2012; Liddelow et al., 2017). On the other hand, RAs similarly regulate the functional status of microglia. Il-15 expression in astrocytes was significantly up-regulated in intracerebral hemorrhage (ICH) mouse models, and IL-15 predisposed microglia to an inflammatory phenotype (Shi et al., 2020). Transforming growth factor-β (TGF-β) secreted by RAs is one of many cytokines that effectively regulate inflammatory response and inhibit post-stroke inflammatory response by inhibiting the NF-κB signal of microglia (Cekanaviciute et al., 2014).
In the context of inflammation, astrocytes act as regulators or amplifiers of the internal environment of the CNS, constituting a complex and dynamic system of reactions. However, most of the current studies focus on the effect of a certain inflammatory molecule on neuroinflammation, and lack of studies on the comprehensive effect of multiple factors, such as the interference of age and gender on the intensity and type of inflammatory response. In the future, we need to further investigate the influence of multiple factors such as age, gender, area of injury, and current treatment methods on secondary inflammation after the stroke, as well as the role that astrocytes play in this process, in order to find effective ways to alleviate or contain neuroinflammation to improve the prognosis and the quality of life of patients.
Within the CNS, the astrocytes endfeet covers almost all capillaries and form an astrocyte boundary membrane outside endothelial cells in the mature brain. The normal morphology and function of the astrocyte boundary membrane are essential in maintaining the integrity of the BBB. knockdown of laminin on astrocyte boundary membrane resulted in decreased expression of tight junction protein in vascular endothelial cells and increased permeability of BBB (Yao et al., 2014). The maintenance of normal BBB function also requires astrocytes regulation. Astrocytes participate in the transport of substances between the CNS and the circulatory system, which prevents the invasion of harmful substances such as circulating antigens (Iadecola and Nedergaard, 2007; Nuriya et al., 2013) and regulate the two-way exchange of water as well as solutes on both sides of the BBB (Fukuda and Badaut, 2012; Shi Z. F. et al., 2021). What is more, astrocytes sense changes in neuronal metabolism and activate their calcium channels, releasing transmitters to regulate blood vessel diameter and blood flow to meet neuronal oxygen and energy needs (Gordon et al., 2007, 2011; Marina et al., 2020; Bonvento and Bolaños, 2021).
Nevertheless, after the stroke, BBB damage leads to continuous leakage of blood components, which leads astrocytes to activate and convert dramatically. Moreover, the activated astrocytes further aggravate the damage degree of BBB (Figure 1). RAs secrete many inflammatory factors in the acute stage of stroke, which directly or indirectly aggravates the functional damage of BBB. Argaw found that inflammatory mediator IL-1β significantly increased the expression of vascular endothelial growth factor A (VEGF-A) in RAs (Argaw et al., 2012). VEGF-A induces down-regulated claudin-5 and occludin in endothelial cells, which ultimately destroy tight junctions between endothelial cells and significantly increase the extravasation of fibrinogen, albumin, and Ig. In the early ICH, heme and thrombin in plasma enter the brain parenchyma through the broken BBB. Heme activates TLR2 on astrocytes surface (Min et al., 2017), thrombin binds to PAR-1 on astrocytes endfeet (Rajput et al., 2014). Both induce increased expression of matrix metalloproteinases (MMPs) in astrocytes, which are thought to be closely related to basement membrane degradation and BBB integrity destruction (Wiese et al., 2012). Our study found that the adiponectin receptor 1 (APNR1) is expressed explicitly on RAs around capillary after ICH. Specific activation of APNR1 can significantly reduce the damage of the BBB (Wu et al., 2020). In addition, astrocytes respond to changes in osmotic pressure induced by BBB destruction through AQP4. The expression of AQP4 in astrocytes endfeet was significantly increased in the ischemic core and penumbra 1 h after vascular occlusion, and AQP4 spread over the astrocyte membrane surface 48 h later. These two periods coincide with the peak of cerebral edema (Manley et al., 2000). Moreover, AQP4 loss in mice can reduce astrocytes swelling and brain edema and improve neurological recovery after ischemia stroke (Shi X. et al., 2021).
Figure 1. The bilateral roles of reactive astrocytes on the neurons and BBB in the different stages of stroke. In the acute stage of stroke, RAs releases inflammatory factors (such as IL-β) to disrupt tight junction and BBB structure, resulting in the infiltration of immune cells. Meanwhile, saturated fatty acids and inflammatory cytokines from RAs damage synapses and induce neuronal necrosis or apoptosis. In the subacute and chronic phases, RAs release cytokines such as VEGF-A to repair BBB and promote angiogenesis. On the other hand, neurotrophic factors derived from RAs such as BNDF promote axonal regeneration and synaptic reconstruction (created with BioRender.com).
In another aspect, other literature has reported that RAs can promote stroke recovery by repairing BBB or promoting angiogenesis (Figure 1). When BBB injury occurs, the intact astrocyte boundary membrane can partially compensate and restore BBB function. After the stroke, astrocytes up-regulate the expression of claudin 1, Claudin 4, and other tight junction proteins in the boundary membrane formed by astrocytes. Alternatively, the Sonic hedgehog (Shh) release may up-regulate capillary endothelial tight junction protein and initiate compensatory repair, promoting BBB recovery (Tian and Kyriakides, 2009; Wang et al., 2014; Liebner et al., 2018). Whereas, when the physical connection between the astrocyte boundary membrane and vascular endothelial cells is structurally damaged, the impaired BBB function cannot be compensated, leading to the aggravation of the secondary brain injury (Horng et al., 2017). In ICH, RAs upregulates heme oxygenase-1 (HO-1), which can resolve heme that flow into the brain parenchyma. Ho-1 overexpression can significantly improve BBB leakage and neurological dysfunction (Chen-Roetling et al., 2017). RAs can also promote stroke recovery by promoting angiogenesis. VEGF-C and VEGF-A secreted by RAs specifically bind to their receptors FLT-4 and FLT-1, respectively, to promote angiogenesis and contribute to spontaneous reperfusion after ischemic stroke (Gu et al., 2001). Notably, high mobility group protein B1 (HMGB-1) has been implicated in inducing inflammatory immune response after brain injury. Hayakawa found that HMGB-1 released by astrocytes promotes endothelial progenitor cell (EPC) mediated neurovascular remodeling in ischemic stroke models. Inhibition of HMGB1 by siRNA can block the pro-angiogenic response of EPC, decrease angiogenesis in damaged areas, and aggravate neurological deficits (Hayakawa et al., 2012).
The bidirectional function of RAs on BBB may be due to differences in the epigenetics of astrocytes at different stages of the stroke (Figure 1). This requires researchers to further clarify the epigenetic states such as genomic methylation and acetylation of RAs cells at different periods and the mechanisms involved. Secondly, in the normal brain, astrocytes are involved in regulating cerebral blood flow to meet the needs of brain metabolism, but there are still few studies on the changes of astrocytes’ blood flow regulation function in disease conditions. Exploration of it will help us further understand the related mechanisms of vascular remodeling and blood flow recovery after stroke and find emerging therapeutic targets.
Astrocytes are regarded as the support cells of neurons, and their dynamic interactions with neurons are crucial to facilitating the formation and stability of synapses and regulating their structure and functions (Nishida and Okabe, 2007). In embryos, contact between embryonic hippocampal neurons and astrocytes activates the neuron’s protein kinase C (PKC) signal, promoting the formation of excitatory synapses (Hama et al., 2004). Astrocytes also phagocytose unnecessary excitatory synaptic connections in the adult hippocampus, critical for supporting cognitive function (Lee et al., 2021). Secondly, astrocytes can sense and stick to synapses, and protrude into the nerve sheath to wrap around synapses. A single cortical astrocyte encloses an average of four neuron bodies and contacts 300–600 neuronal dendrites, forming synaptic “islands” through which astrocytes can synchronize and regulate neural networks (Bushong et al., 2002; Allen, 2014; Yang et al., 2019). Astrocytes can quickly clear neurotransmitters released from synapses and limit their “overflow” to other synapses through physical barriers so that these transmitters cannot interfere with the transmission of other nerve signals (Chen and Swanson, 2003; Dallérac et al., 2018; Todd and Hardingham, 2020).
After the stroke, the supporting function of astrocytes to neurons is significantly changed. In addition to regulating the state of neurons by intervening in the inflammatory response and BBB leakage, there is also evidence that RAs themselves can directly interfere in the survival and repair of injured neurons (Figure 1). The traditional view is that RAs have significant neurotoxicity, and the formation of RAs after stroke is associated with worsening neurological prognosis. Guttenplan demonstrated that the C3d+/GFAP+ A1 release of saturated lipids activates adipocyte apoptosis-related pathways that directly drive neuronal and oligodendrocyte apoptosis (Guttenplan et al., 2021). RAs release S-100β to enhance the expression of inducible nitric oxide synthase (INOS), leading to NO-mediated neuronal death (Zhao and Rempe, 2010). In addition, RAs interfere with neuronal repair and synaptic reconstruction after the stroke. Scarring of RAs is also thought to be a significant obstacle to axon regeneration in the CNS. The physical barrier formed by the scar prevents the new axons from passing through the damaged area. Hara proved that blocking type I collagen formation to prevent astrocyte scar formation can improve axon regeneration and restore neural function (Hara et al., 2017). New studies have shown that RAs phagocytose newly formed synapses and inhibit the recovery of nerve function after the stroke. Especially in the ischemic stroke model, astrocytes transform into phagocytic phenotypes by upregulation ABCA1 and its pathway-related molecules MEGF10 and GULP1. Phagocytic astrocytes can engulf synaptic components marked by presynaptic and postsynaptic proteins. Inhibition of this pathway can reduce the phagocytosis of astrocytes, improve neurobehavioral outcomes and alleviate brain injury (Schilling et al., 2005; Morizawa et al., 2017; Shi X. et al., 2021).
A new view suggests that injury factors activate downstream pathways of astrocytes and have neuroprotective functions (Figure 1). Ischemic stroke induces the secretion of thrombin from neurons, which binds to PAP1 on the astrocyte surface to induce the production of neuroprotective phenotype astrocytes and block neuronal death induced by oxygen and glucose deprivation (OGD) (Rajput et al., 2020). RAs can also release a variety of neurotrophic factors. RAs highly expressed BDNF, nerve growth factor (NGF), and fibroblast growth factor (FGF) can activate TrkB receptor, TrkA receptor, and FGFR on the surface of neurons, respectively, improve the tolerance of neurons to injury factors and promote neuronal survival (Tokumine et al., 2003; Tejeda et al., 2019). Clinical studies have also confirmed that the release level of the neurotrophic factor is positively correlated with the outcome of stroke patients (Sobrino et al., 2020). RAs are a pivotal participant in regulating synaptic reconstruction after stroke. In focal ischemia model mice, astrocytes significantly increased Thrombospondins 1 and 2 (TSP-1/2) expression and inhibition of TSP-1/2 expression showed decreased synaptic density and axonal sprouting defects (Liauw et al., 2008). Moreover, astrocytes form a network of connections between the nerve germinal center (sublateral ventricle) and the ischemic area and secrete stromal cell-derived factor-1 (SDF-1) that is the chemokine of neuroblasts, guiding neuroblasts migrating to the cerebral infarction area (Thored et al., 2006; Zhang et al., 2007, 2018). To some extent, neuroblasts can differentiate into neurons and repair defective neurons.
In the study of the damage and repair of neurons by RAs in the stroke, we found that many processes are associated with the promotion and reconstruction of neurons by astrocytes during nervous system development. Many related genes are reactivated, transcribed, and expressed by injury factors. Compared to the growth process, the intense stimulation of stroke disrupts the regulatory balance. However, which factors are involved in the reactivation process and the underlying mechanisms still need to be further explored and discovered by future researchers. This not only helps us to further understand the supporting mechanism of astrocytes for neurons but also helps us to find ways to reconstruct the balance of astrocyte regulation after the stroke.
Over the past few years, several complementary techniques and tools have encouraged progress in exploring astrocytes’ molecular mechanisms, signaling pathways, and functional patterns (Yu et al., 2020). First of all, in combination with a specific mouse lineage and anti-astrocyte specific antibodies, astrocytes can be specifically isolated and purified by FACS, immunostaining, and activated magnetic cell sorting to improve the accuracy and efficiency of single-cell transcription sequencing (Orre et al., 2014; Holt and Olsen, 2016; Zhang et al., 2016; Holt et al., 2019). Secondly, the latest technology has enabled direct analysis of mRNA transcribed in specific populations of cells in the brain without isolating cells. A technique called translational ribosomal affinity purification (TRAP) is the gene-targeted expression of EGFP-labeled ribosomal protein L10a, which can be obtained using EGFP-specific antibodies, enabling sequencing of associated ribosomal mRNA (Doyle et al., 2008). RiboTag is a related method (Sanz et al., 2009). Currently, RiboTag can be deployed in astrocyte around the lesion by astrocyte-specific RiboTag AAV vector to analyze the changes in astrocyte transcription levels after the onset of disease (Yu et al., 2018; Nagai et al., 2019). Finally, the latest visualization techniques can provide a more intuitive view of astrocyte ultrastructure. Super-resolution microscopy, combined with mathematical reconstruction to improve spatial resolution below the refractive index limit of the optical microscope, is capable of imaging the tiny projections of astrocytes and certain synaptic proteins within them. This approach is able to visualize astrocyte structure at tens of nanometers, which has excellent potential for observing astrocyte synaptic changes after stroke (Heller et al., 2020). The application of new technologies and tools in the CNS promises to research and raises further scientific questions to improve researchers’ understanding of astrocyte function after stroke.
For decades, stroke treatment has focused on alleviating irreversible neuronal damage, but therapies have failed to provide significant clinical benefits. In recent years, the NVU composed of neurons, glial cells, and vascular systems has gradually been regarded as an essential factor affecting various neurological diseases, including stroke (Guo and Lo, 2009). Therefore, future therapies should expand the scope of neuroprotection to cover the entire NVU. Astrocytes, as a large cell population in contact with all components of NVU, provide broad prospects for clinical stroke treatment (see Table 3).
As mentioned above, different phenotypes of astrocytes have their functional characteristics. Can we promote stroke recovery by modulating the phenotype of RAs toward neuroprotective type? In vitro and animal models, related interventions have been found to inhibit the inflammatory response, reduce BBB damage, alleviate injury and surrounding edema volume, and improve neurological function. For example, Liu M. et al. (2020) found that cottonseed oil (CSO) can inhibit the C3d+/GFAP+ astrocytes by blocking the TLR4/NF-κB pathway and reducing the infarct volume and inflammatory response in the middle cerebral artery occlusion (MCAO) rat model. The glucagon-like peptide-1 receptor (GLP-1R) agonist Exendin4 (EX-4) binds to astrocyte surface GLP-1R to protect the BBB and reduce secondary inflammation caused by cerebral ischemia. Shan demonstrated that EX-4 improved neurological deficit scores in the rat model of MCAO, reduced infarct size, and reduced levels of VEGF-A, MMP-9, CXCL-1, and MCP-1 derived from astrocytes (Shan et al., 2019). Our study found that classical antioxidant and anti-inflammatory adiponectin mimicking peptides can be enriched on the surface of the astrocyte membrane around BBB and inhibit the inflammatory damage of RAs on BBB and neurons by activating AMPK-DRP1 signaling (Wu et al., 2020). In the study of RAs phenotype, numerous intervention sites have been exposed, and the intervention effects of each site may be unrelated to or affect each other, which undoubtedly brings difficulties for researchers and clinicians to choose. In future translational clinical studies, researchers need to carefully identify the interrelationships and effects of each point to develop the most effective comprehensive intervention measures.
Traditionally, astrocyte scarring is the main reason for the failure of axon regeneration in severe central nerve injury. Inhibition of scar formation can improve axonal regeneration and nerve function. Previous studies have illustrated that the main mechanism of inhibition of axon regeneration by astrocyte scar is the production of CSPG (Silver and Miller, 2004). However, the up-regulated CSPG4 and CSPG5 in astrocyte scar can also promote axon growth, and only when the scar formed by astrocytes is present, the related genes of axon growth will be activated (Anderson et al., 2016). The specific relationship between glial scar and axon regeneration as well as the prognosis may be related to the injury site, duration of the stroke, and the response degree of astrocytes. Previous therapies that inhibit scar formation by inhibiting astrocyte reactivity have not achieved the desired results. Therefore, the opposite strategy has been proposed to enhance RAs’ reactivity and promote their neuroprotective function. The monoamine oxidase B inhibitor selegiline can strengthen the reaction of astrocytes to injury and increase the expression of NGF, which has been proved to alleviate ischemic injury of cortical tissues after MCAO in mice and improve the prognosis of patients (Bartolo et al., 2015; Nardai et al., 2015). Whether inhibition of astrocyte scar formation can improve the prognosis of stroke patients still requires further exploration by researchers.
Given that scars are indeed a physical barrier to axon regeneration, we hypothesized that the best strategy might be to exert the protective function of astrocytes in the early stage and select appropriate intervention sites to inhibit scar formation in the middle or late stages. For example, ephrin-A5 expressed by RAs in the peri-infarction region can inhibit axon regeneration, and inhibition of ephrin-A5 in the peri-infarction area can promote axon growth on day seven after stroke (Overman et al., 2012), which provides a long treatment time window so that has broad clinical prospects.
In recent years, stem cell therapy using embryonic stem cells or induced pluripotent stem cells to generate neurons has been considered to fill in the defective neurons and restore normal brain function in the damaged area (Yu et al., 2019; Sun et al., 2020; Asgari Taei et al., 2021). However, this cell transplantation method faces risks such as immune rejection, tumor formation, and differentiation uncertainty (Lee et al., 2013; Lukovic et al., 2014). Recent studies have discovered that astrocytes provide a new possibility for stem cells in stroke treatment. Astrocytes can induce stem cell proliferation and differentiation. In the co-culture of human umbilical cord mesenchymal stem cells (huc-Mscs) with LPS-induced RAs, it was found that huc-Mscs showed a higher S phase ratio, and BDNF secreted by astrocytes could induce the neural differentiation of exogenous huc-Mscs (Shi et al., 2016). In addition, astrocytes may have the potential to transform directly into neurons to repair damaged areas. Neural stem cells can migrate from the sub-ventricular zone (SVZ) region to the site of injury, where they differentiate into RAs subsets, and these SVZ-derived RAs can be transformed into neurons in vivo through forced expression of Ascl1 (Doetsch et al., 1999). Meanwhile, astrocytes can also reverse into radial glial stem cell rNSC and then differentiate into neurons (Niu et al., 2015). Surprisingly, neuroD1 can promote the direct transformation of astrocytes into functional neurons (Zamboni et al., 2020), and these neurons produced by astrocytes have a favorable functional state such as can participate in the establishment of synaptic networks and electrical signal transmission (Berninger et al., 2007; Zhang et al., 2015). However, the transformation of astrocytes into neurons is still controversial. Wang et al. (2021) found that overexpression of NeuroD1 does not change the fate of astrocytes according to cell lineage tracing, but NeuroD1 affects the specificity of the GFAP promoter, which is ultimately expressed in neurons. Nevertheless, the role of astrocytes on stem cells and their dryness is still a significant research area in the field of neural repair in the future.
Chinese herbal medicines (CHM) have a long tradition in treating stroke and related disorders. Multiple in vitro and in vivo studies have shown that many CHM can promote angiogenesis, inhibit inflammation and protect neural function through astrocytes (see Table 4). Ginkgo Diterpene Lactones (GDL) are extracts of active components from Ginkgoaceae leaves. GDL can inhibit astrocyte activation in rat middle cerebral artery occlusion/reperfusion (MCAO/R) and neuronal oxygen-glucose deprivation/reoxygenation (OGD/R) models and inhibit inflammation by inhibition of the TLR4/NF-κB pathway (Li et al., 2020). Ginsenosides, the bioactive components of Panax ginseng, can improve the mitochondrial oxidative phosphorylation efficiency and reduce ROS production of astrocytes in the OGD/R model, thereby protecting astrocytes (Xu et al., 2019). 1,3,7-trihydroxyxanthone is a compound isolated from Polygalae Radix, which can significantly stimulate high expression of neurotrophic factors (NGF, BDNF, etc.) in astrocytes and promote neuronal survival (Yang et al., 2018). Clinical and large-scale population epidemiological studies have proved the potential benefits of Chinese herbs and herbal formulations in the treatment of ischemic stroke (Chen et al., 2012; Hayat et al., 2015). CHM will have a broad application prospect in treating stroke in the future.
Unfortunately, all of these interventions have been unable to show the desired results in clinical trials. Above all, effective drug concentration may not be achieved in the injured area because related drugs are difficult to cross the BBB. Excitingly, a promising technology holds out the possibility of breaching the BBB’s limits. The ability of platelet membranes to cross barriers and target damage areas is transferred to microglia by membrane fusion. Ultrasound responsive IL-4 loaded liposomes were then attached to engineered microglia via bioorthogonal reaction to achieve remote directional control of specific anti-inflammatory phenotypic polarization of in situ microglia (Li et al., 2021). The broad potential of its ability to span BBB and target drug release deserves further investigation: (1) Whether this platform can be used to regulate RAs phenotypes after stroke; (2) Whether similar methods can be used to transplant astrocytes with neuroprotective phenotypes into the injured area by injection.
In the second place, as mentioned above, RAs have complex subsets and a certain temporal variation trend in the area of stroke injury. only at a single point in time for a single pathological factor intervention may be challenging to achieve satisfactory results or even counterproductive. This will require future researchers to further clarify: (1) Whether there are “threshold genes” for the phenotypic transformation of RAs, the phenotypic regulation of RAs can be achieved by manipulating them alone; (2) Whether a multi-pathway combined regulation scheme similar to “cocktail therapy” can be constructed to comprehensively regulate astrocyte function. The resolution of these problems will provide a broad clinical potential for RAs to promote the outcome of stroke.
Astrocytes are the most abundant glial cells in the CNS. They contact and communicate with other CNS components and participate in normal physiological and pathological reactions of the nervous system structurally and functionally. One of the most significant pathologic features of stroke is RAs formation induced by environmental changes in the CNS. The phenotypic and functional characteristics of RAs differed in different modes and periods of injury. They both repair damaged nerves and inhibit axon regeneration. They also play a protective role in the BBB and increase BBB leakage. Future studies should comprehensively consider the phenotypic transformation of RAs and their bidirectional effects, from which to explore multi-temporal and multi-dimensional intervention methods to ultimately achieve comprehensive treatment to reduce injury and promote repair after stroke. these may be the key to stroke treatment and improving the prognosis of patients.
All authors listed have made a substantial, direct, and intellectual contribution to the work, and approved it for publication.
This work received support from grants from the State Key Program of the National Natural Science Foundation of China (81630027 and 82130038).
The authors declare that the research was conducted in the absence of any commercial or financial relationships that could be construed as a potential conflict of interest.
All claims expressed in this article are solely those of the authors and do not necessarily represent those of their affiliated organizations, or those of the publisher, the editors and the reviewers. Any product that may be evaluated in this article, or claim that may be made by its manufacturer, is not guaranteed or endorsed by the publisher.
Ader, I., Brizuela, L., Bouquerel, P., Malavaud, B., and Cuvillier, O. (2008). Sphingosine kinase 1: a new modulator of hypoxia inducible factor 1alpha during hypoxia in human cancer cells. Cancer Res. 68, 8635–8642. doi: 10.1158/0008-5472.Can-08-0917
Al-Dalahmah, O., Sosunov, A. A., Shaik, A., Ofori, K., Liu, Y., Vonsattel, J. P., et al. (2020). Single-nucleus RNA-seq identifies Huntington disease astrocyte states. Acta Neuropathol. Commun. 8:19. doi: 10.1186/s40478-020-0880-6
Ali, C., Nicole, O., Docagne, F., Lesne, S., MacKenzie, E. T., Nouvelot, A., et al. (2000). Ischemia-induced interleukin-6 as a potential endogenous neuroprotective cytokine against NMDA receptor-mediated excitotoxicity in the brain. J. Cereb. Blood Flow Metab. 20, 956–966. doi: 10.1097/00004647-200006000-00008
Allen, N. J. (2014). Synaptic plasticity: astrocytes wrap it up. Curr. Biol. 24, R697–R699. doi: 10.1016/j.cub.2014.06.030
Anderson, M. A., Burda, J. E., Ren, Y., Ao, Y., O’Shea, T. M., Kawaguchi, R., et al. (2016). Astrocyte scar formation aids central nervous system axon regeneration. Nature 532, 195–200. doi: 10.1038/nature17623
Argaw, A. T., Asp, L., Zhang, J., Navrazhina, K., Pham, T., Mariani, J. N., et al. (2012). Astrocyte-derived VEGF-A drives blood brain barrier disruption in CNS inflammatory disease. J. Clin. Invest. 122, 2454–2468. doi: 10.1172/JCI60842
Arrieta, A., Blackwood, E. A., Stauffer, W. T., Santo Domingo, M., Bilal, A. S., Thuerauf, D. J., et al. (2020). Mesencephalic astrocyte-derived neurotrophic factor is an ER-resident chaperone that protects against reductive stress in the heart. J. Biol. Chem. 295, 7566–7583. doi: 10.1074/jbc.RA120.013345
Asgari Taei, A., Dargahi, L., Nasoohi, S., Hassanzadeh, G., Kadivar, M., and Farahmandfar, M. (2021). The conditioned medium of human embryonic stem cell-derived mesenchymal stem cells alleviates neurological deficits and improves synaptic recovery in experimental stroke. J. Cell. Physiol. 236, 1967–1979. doi: 10.1002/jcp.29981
Auernhammer, C. J., Isele, N. B., Kopp, F. B., Spoettl, G., Cengic, N., Weber, M. M., et al. (2003). Novel neurotrophin-1/B cell-stimulating factor-3 (cardiotrophin-like cytokine) stimulates corticotroph function via a signal transducer and activator of transcription-dependent mechanism negatively regulated by suppressor of cytokine signaling-3. Endocrinology 144, 1202–1210. doi: 10.1210/en.2002-220933
Bai, Q., Xue, M., and Yong, V. W. (2020). Microglia and macrophage phenotypes in intracerebral haemorrhage injury: therapeutic opportunities. Brain 143, 1297–1314. doi: 10.1093/brain/awz393
Bangsow, T., Baumann, E., Bangsow, C., Jaeger, M. H., Pelzer, B., Gruhn, P., et al. (2008). The epithelial membrane protein 1 is a novel tight junction protein of the blood-brain barrier. J. Cereb. Blood Flow Metab. 28, 1249–1260. doi: 10.1038/jcbfm.2008.19
Bartolo, M., Zucchella, C., Capone, A., Sandrini, G., and Pierelli, F. (2015). An explorative study regarding the effect of l-deprenyl on cognitive and functional recovery in patients after stroke. J. Neurol. Sci. 349, 117–123. doi: 10.1016/j.jns.2014.12.039
Berninger, B., Costa, M. R., Koch, U., Schroeder, T., Sutor, B., Grothe, B., et al. (2007). Functional properties of neurons derived from in vitro reprogrammed postnatal astroglia. J. Neurosci. 27, 8654–8664. doi: 10.1523/jneurosci.1615-07.2007
Boisvert, M. M., Erikson, G. A., Shokhirev, M. N., and Allen, N. J. (2018). The Aging Astrocyte Transcriptome from Multiple Regions of the Mouse Brain. Cell Rep. 22, 269–285. doi: 10.1016/j.celrep.2017.12.039
Bonvento, G., and Bolaños, J. P. (2021). Astrocyte-neuron metabolic cooperation shapes brain activity. Cell Metab. 33, 1546–1564. doi: 10.1016/j.cmet.2021.07.006
Boutin, H., LeFeuvre, R. A., Horai, R., Asano, M., Iwakura, Y., and Rothwell, N. J. (2001). Role of IL-1alpha and IL-1beta in ischemic brain damage. J. Neurosci. 21, 5528–5534. doi: 10.1523/jneurosci.21-15-05528.2001
Bsibsi, M., Bajramovic, J. J., Van Duijvenvoorden, E., Persoon, C., Ravid, R., Van Noort, J. M., et al. (2007). Identification of soluble CD14 as an endogenous agonist for Toll-like receptor 2 on human astrocytes by genome-scale functional screening of glial cell derived proteins. Glia 55, 473–482. doi: 10.1002/glia.20473
Bushong, E. A., Martone, M. E., Jones, Y. Z., and Ellisman, M. H. (2002). Protoplasmic astrocytes in CA1 stratum radiatum occupy separate anatomical domains. J. Neurosci. 22, 183–192. doi: 10.1523/jneurosci.22-01-00183.2002
Buss, A., Brook, G. A., Kakulas, B., Martin, D., Franzen, R., Schoenen, J., et al. (2004). Gradual loss of myelin and formation of an astrocytic scar during Wallerian degeneration in the human spinal cord. Brain 127, 34–44. doi: 10.1093/brain/awh001
Cahoy, J. D., Emery, B., Kaushal, A., Foo, L. C., Zamanian, J. L., Christopherson, K. S., et al. (2008). A transcriptome database for astrocytes, neurons, and oligodendrocytes: a new resource for understanding brain development and function. J. Neurosci. 28, 264–278. doi: 10.1523/jneurosci.4178-07.2008
Cekanaviciute, E., Dietrich, H. K., Axtell, R. C., Williams, A. M., Egusquiza, R., Wai, K. M., et al. (2014). Astrocytic TGF-β signaling limits inflammation and reduces neuronal damage during central nervous system Toxoplasma infection. J. Immunol. 193, 139–149. doi: 10.4049/jimmunol.1303284
Chamorro, Á, Dirnagl, U., Urra, X., and Planas, A. M. (2016). Neuroprotection in acute stroke: targeting excitotoxicity, oxidative and nitrosative stress, and inflammation. Lancet Neurol. 15, 869–881. doi: 10.1016/s1474-4422(16)00114-9
Chancellor, K. B., Chancellor, S. E., Duke-Cohan, J. E., Huber, B. R., Stein, T. D., Alvarez, V. E., et al. (2021). Altered oligodendroglia and astroglia in chronic traumatic encephalopathy. Acta Neuropathol. 142, 295–321. doi: 10.1007/s00401-021-02322-2
Chen, B., Chen, Z., Liu, M., Gao, X., Cheng, Y., Wei, Y., et al. (2019). Inhibition of neuronal ferroptosis in the acute phase of intracerebral hemorrhage shows long-term cerebroprotective effects. Brain Res. Bull. 153, 122–132. doi: 10.1016/j.brainresbull.2019.08.013
Chen, Y. C., Wu, J. S., Yang, S. T., Huang, C. Y., Chang, C., Sun, G. Y., et al. (2012). Stroke, angiogenesis and phytochemicals. Front. Biosci. 4:599–610. doi: 10.2741/s287
Chen, Y., and Swanson, R. A. (2003). The glutamate transporters EAAT2 and EAAT3 mediate cysteine uptake in cortical neuron cultures. J. Neurochem. 84, 1332–1339. doi: 10.1046/j.1471-4159.2003.01630.x
Cheng, L., Zhao, H., Zhang, W., Liu, B., Liu, Y., Guo, Y., et al. (2013). Overexpression of conserved dopamine neurotrophic factor (CDNF) in astrocytes alleviates endoplasmic reticulum stress-induced cell damage and inflammatory cytokine secretion. Biochem. Biophys. Res. Commun. 435, 34–39. doi: 10.1016/j.bbrc.2013.04.029
Chen-Roetling, J., Kamalapathy, P., Cao, Y., Song, W., Schipper, H. M., and Regan, R. F. (2017). Astrocyte heme oxygenase-1 reduces mortality and improves outcome after collagenase-induced intracerebral hemorrhage. Neurobiol. Dis. 102, 140–146. doi: 10.1016/j.nbd.2017.03.008
Dallérac, G., Zapata, J., and Rouach, N. (2018). Versatile control of synaptic circuits by astrocytes: where, when and how? Nat. Rev. Neurosci. 19, 729–743. doi: 10.1038/s41583-018-0080-6
Doetsch, F., Caillé, I., Lim, D. A., García-Verdugo, J. M., and Alvarez-Buylla, A. (1999). Subventricular zone astrocytes are neural stem cells in the adult mammalian brain. Cell 97, 703–716. doi: 10.1016/s0092-8674(00)80783-7
Doll, D. N., Rellick, S. L., Barr, T. L., Ren, X., and Simpkins, J. W. (2015). Rapid mitochondrial dysfunction mediates TNF-alpha-induced neurotoxicity. J. Neurochem. 132, 443–451. doi: 10.1111/jnc.13008
Doyle, J. P., Dougherty, J. D., Heiman, M., Schmidt, E. F., Stevens, T. R., Ma, G., et al. (2008). Application of a translational profiling approach for the comparative analysis of CNS cell types. Cell 135, 749–762. doi: 10.1016/j.cell.2008.10.029
Eng, L. F., Vanderhaeghen, J. J., Bignami, A., and Gerstl, B. (1971). An acidic protein isolated from fibrous astrocytes. Brain Res. 28, 351–354. doi: 10.1016/0006-8993(71)90668-8
Escartin, C., Galea, E., Lakatos, A., O’Callaghan, J. P., Petzold, G. C., Serrano-Pozo, A., et al. (2021). Reactive astrocyte nomenclature, definitions, and future directions. Nat. Neurosci. 24, 312–325. doi: 10.1038/s41593-020-00783-4
Escartin, C., Guillemaud, O., and Carrillo-de Sauvage, M. A. (2019). Questions and (some) answers on reactive astrocytes. Glia 67, 2221–2247. doi: 10.1002/glia.23687
Filppu, P., Tanjore Ramanathan, J., Granberg, K. J., Gucciardo, E., Haapasalo, H., Lehti, K., et al. (2021). CD109-GP130 interaction drives glioblastoma stem cell plasticity and chemoresistance through STAT3 activity. JCI Insight 6:e141486. doi: 10.1172/jci.insight.141486
Fu, Y., Hao, J., Zhang, N., Ren, L., Sun, N., Li, Y. J., et al. (2014). Fingolimod for the treatment of intracerebral hemorrhage: a 2-arm proof-of-concept study. JAMA Neurol. 71, 1092–1101. doi: 10.1001/jamaneurol.2014.1065
Fukuda, A. M., and Badaut, J. (2012). Aquaporin 4: a player in cerebral edema and neuroinflammation. J. Neuroinflammation 9:279. doi: 10.1186/1742-2094-9-279
Gardner, J., and Ghorpade, A. (2003). Tissue inhibitor of metalloproteinase (TIMP)-1: the TIMPed balance of matrix metalloproteinases in the central nervous system. J. Neurosci. Res. 74, 801–806. doi: 10.1002/jnr.10835
GBD 2019 Stroke Collaborators (2021). Global, regional, and national burden of stroke and its risk factors, 1990-2019: a systematic analysis for the Global Burden of Disease Study 2019. Lancet Neurol. 20, 795–820. doi: 10.1016/S1474-4422(21)00252-0
Gerics, B., Szalay, F., and Hajós, F. (2006). Glial fibrillary acidic protein immunoreactivity in the rat suprachiasmatic nucleus: circadian changes and their seasonal dependence. J. Anat. 209, 231–237. doi: 10.1111/j.1469-7580.2006.00593.x
Gordon, G. R., Howarth, C., and MacVicar, B. A. (2011). Bidirectional control of arteriole diameter by astrocytes. Exp. Physiol. 96, 393–399. doi: 10.1113/expphysiol.2010.053132
Gordon, G. R., Mulligan, S. J., and MacVicar, B. A. (2007). Astrocyte control of the cerebrovasculature. Glia 55, 1214–1221. doi: 10.1002/glia.20543
Götz, S., Bribian, A., López-Mascaraque, L., Götz, M., Grothe, B., and Kunz, L. (2021). Heterogeneity of astrocytes: electrophysiological properties of juxtavascular astrocytes before and after brain injury. Glia 69, 346–361. doi: 10.1002/glia.23900
Gouix, E., Buisson, A., Nieoullon, A., Kerkerian-Le Goff, L., Tauskela, J. S., Blondeau, N., et al. (2014). Oxygen glucose deprivation-induced astrocyte dysfunction provokes neuronal death through oxidative stress. Pharmacol. Res. 87, 8–17. doi: 10.1016/j.phrs.2014.06.002
Gril, B., Paranjape, A. N., Woditschka, S., Hua, E., Dolan, E. L., Hanson, J., et al. (2018). Reactive astrocytic S1P3 signaling modulates the blood-tumor barrier in brain metastases. Nat. Commun. 9:2705. doi: 10.1038/s41467-018-05030-w
Gu, W., Brännström, T., Jiang, W., Bergh, A., and Wester, P. (2001). Vascular endothelial growth factor-A and -C protein up-regulation and early angiogenesis in a rat photothrombotic ring stroke model with spontaneous reperfusion. Acta Neuropathol. 102, 216–226. doi: 10.1007/s004010100370
Guo, J., Cheng, C., Chen, C. S., Xing, X., Xu, G., Feng, J., et al. (2016). Overexpression of Fibulin-5 Attenuates Ischemia/Reperfusion Injury After Middle Cerebral Artery Occlusion in Rats. Mol. Neurobiol. 53, 3154–3167. doi: 10.1007/s12035-015-9222-2
Guo, K., Luo, J., Feng, D., Wu, L., Wang, X., Xia, L., et al. (2021). Single-Cell RNA Sequencing With Combined Use of Bulk RNA Sequencing to Reveal Cell Heterogeneity and Molecular Changes at Acute Stage of Ischemic Stroke in Mouse Cortex Penumbra Area. Front. Cell. Dev. Biol. 9:624711. doi: 10.3389/fcell.2021.624711
Guo, S., and Lo, E. H. (2009). Dysfunctional cell-cell signaling in the neurovascular unit as a paradigm for central nervous system disease. Stroke 40, S4–S7. doi: 10.1161/strokeaha.108.534388
Guttenplan, K. A., Weigel, M. K., Prakash, P., Wijewardhane, P. R., Hasel, P., Rufen-Blanchette, U., et al. (2021). Neurotoxic reactive astrocytes induce cell death via saturated lipids. Nature 599, 102–107. doi: 10.1038/s41586-021-03960-y
Hama, H., Hara, C., Yamaguchi, K., and Miyawaki, A. (2004). PKC signaling mediates global enhancement of excitatory synaptogenesis in neurons triggered by local contact with astrocytes. Neuron 41, 405–415. doi: 10.1016/s0896-6273(04)00007-8
Hara, M., Kobayakawa, K., Ohkawa, Y., Kumamaru, H., Yokota, K., Saito, T., et al. (2017). Interaction of reactive astrocytes with type I collagen induces astrocytic scar formation through the integrin-N-cadherin pathway after spinal cord injury. Nat. Med. 23, 818–828. doi: 10.1038/nm.4354
Hasel, P., Rose, I. V. L., Sadick, J. S., Kim, R. D., and Liddelow, S. A. (2021). Neuroinflammatory astrocyte subtypes in the mouse brain. Nat. Neurosci. 24, 1475–1487. doi: 10.1038/s41593-021-00905-6
Hayakawa, K., Pham, L. D., Katusic, Z. S., Arai, K., and Lo, E. H. (2012). Astrocytic high-mobility group box 1 promotes endothelial progenitor cell-mediated neurovascular remodeling during stroke recovery. Proc. Natl. Acad. Sci. U. S. A. 109, 7505–7510. doi: 10.1073/pnas.1121146109
Hayat, K., Iqbal, H., Malik, U., Bilal, U., and Mushtaq, S. (2015). Tea and its consumption: benefits and risks. Crit. Rev. Food Sci. Nutr. 55, 939–954. doi: 10.1080/10408398.2012.678949
Heller, J. P., Odii, T., Zheng, K., and Rusakov, D. A. (2020). Imaging tripartite synapses using super-resolution microscopy. Methods 174, 81–90. doi: 10.1016/j.ymeth.2019.05.024
Hol, E. M., and Pekny, M. (2015). Glial fibrillary acidic protein (GFAP) and the astrocyte intermediate filament system in diseases of the central nervous system. Curr. Opin. Cell Biol. 32, 121–130. doi: 10.1016/j.ceb.2015.02.004
Holt, L. M., and Olsen, M. L. (2016). Novel Applications of Magnetic Cell Sorting to Analyze Cell-Type Specific Gene and Protein Expression in the Central Nervous System. PLoS One 11:e0150290. doi: 10.1371/journal.pone.0150290
Holt, L. M., Stoyanof, S. T., and Olsen, M. L. (2019). Magnetic Cell Sorting for In Vivo and In Vitro Astrocyte, Neuron, and Microglia Analysis. Curr. Protoc. Neurosci. 88:e71. doi: 10.1002/cpns.71
Horng, S., Therattil, A., Moyon, S., Gordon, A., Kim, K., Argaw, A. T., et al. (2017). Astrocytic tight junctions control inflammatory CNS lesion pathogenesis. J. Clin. Invest. 127, 3136–3151. doi: 10.1172/jci91301
Houben, E., Janssens, K., Hermans, D., Vandooren, J., Van den Haute, C., Schepers, M., et al. (2020). Oncostatin M-induced astrocytic tissue inhibitor of metalloproteinases-1 drives remyelination. Proc. Natl. Acad. Sci. U. S. A. 117, 5028–5038. doi: 10.1073/pnas.1912910117
Huang, L., Wu, Z. B., Zhuge, Q., Zheng, W., Shao, B., Wang, B., et al. (2014). Glial scar formation occurs in the human brain after ischemic stroke. Int. J. Med. Sci. 11, 344–348. doi: 10.7150/ijms.8140
Iadecola, C., and Nedergaard, M. (2007). Glial regulation of the cerebral microvasculature. Nat. Neurosci. 10, 1369–1376. doi: 10.1038/nn2003
Iadecola, C., Buckwalter, M. S., and Anrather, J. (2020). Immune responses to stroke: mechanisms, modulation, and therapeutic potential. J. Clin. Invest. 130, 2777–2788. doi: 10.1172/JCI135530
Johnston, M. V. (2005). Excitotoxicity in perinatal brain injury. Brain Pathol. 15, 234–240. doi: 10.1111/j.1750-3639.2005.tb00526.x
Kim, J. H., Ko, P. W., Lee, H. W., Jeong, J. Y., Lee, M. G., Kim, J. H., et al. (2017). Astrocyte-derived lipocalin-2 mediates hippocampal damage and cognitive deficits in experimental models of vascular dementia. Glia 65, 1471–1490. doi: 10.1002/glia.23174
Kjell, J., and Götz, M. (2020). Filling the Gaps - A Call for Comprehensive Analysis of Extracellular Matrix of the Glial Scar in Region- and Injury-Specific Contexts. Front. Cell. Neurosci. 14:32. doi: 10.3389/fncel.2020.00032
Laeremans, A., Nys, J., Luyten, W., D’Hooge, R., Paulussen, M., and Arckens, L. (2013). AMIGO2 mRNA expression in hippocampal CA2 and CA3a. Brain Struct. Funct. 218, 123–130. doi: 10.1007/s00429-012-0387-4
Lai, S.-M., Studenski, S., Duncan, P. W., and Perera, S. (2002). Persisting consequences of stroke measured by the Stroke Impact Scale. Stroke 33, 1840–1844. doi: 10.1161/01.str.0000019289.15440.f2
Lambertsen, K. L., Biber, K., and Finsen, B. (2012). Inflammatory cytokines in experimental and human stroke. J. Cereb. Blood Flow Metab. 32, 1677–1698. doi: 10.1038/jcbfm.2012.88
Lambertsen, K. L., Finsen, B., and Clausen, B. H. (2019). Post-stroke inflammation-target or tool for therapy? Acta Neuropathol. 137, 693–714. doi: 10.1007/s00401-018-1930-z
Lan, X., Han, X., Li, Q., Yang, Q. W., and Wang, J. (2017). Modulators of microglial activation and polarization after intracerebral haemorrhage. Nat. Rev. Neurol. 13, 420–433. doi: 10.1038/nrneurol.2017.69
Lantoine, J., Procès, A., Villers, A., Halliez, S., Buée, L., Ris, L., et al. (2021). Inflammatory Molecules Released by Mechanically Injured Astrocytes Trigger Presynaptic Loss in Cortical Neuronal Networks. ACS Chem. Neurosci. 12, 3885–3897. doi: 10.1021/acschemneuro.1c00488
Lau, L. T., and Yu, A. C. (2001). Astrocytes produce and release interleukin-1, interleukin-6, tumor necrosis factor alpha and interferon-gamma following traumatic and metabolic injury. J. Neurotrauma 18, 351–359. doi: 10.1089/08977150151071035
Lee, A. S., Tang, C., Rao, M. S., Weissman, I. L., and Wu, J. C. (2013). Tumorigenicity as a clinical hurdle for pluripotent stem cell therapies. Nat. Med. 19, 998–1004. doi: 10.1038/nm.3267
Lee, J. H., Kim, J. Y., Noh, S., Lee, H., Lee, S. Y., Mun, J. Y., et al. (2021). Astrocytes phagocytose adult hippocampal synapses for circuit homeostasis. Nature 590, 612–617. doi: 10.1038/s41586-020-03060-3
Lee, R., Kermani, P., Teng, K. K., and Hempstead, B. L. (2001). Regulation of cell survival by secreted proneurotrophins. Science 294, 1945–1948. doi: 10.1126/science.1065057
Li, H., Zhang, N., Lin, H. Y., Yu, Y., Cai, Q. Y., Ma, L., et al. (2014). Histological, cellular and behavioral assessments of stroke outcomes after photothrombosis-induced ischemia in adult mice. BMC Neurosci. 15:58. doi: 10.1186/1471-2202-15-58
Li, X., Huang, L., Liu, G., Fan, W., Li, B., Liu, R., et al. (2020). Ginkgo diterpene lactones inhibit cerebral ischemia/reperfusion induced inflammatory response in astrocytes via TLR4/NF-κB pathway in rats. J. Ethnopharmacol. 249:112365. doi: 10.1016/j.jep.2019.112365
Li, Y., Teng, X., Yang, C., Wang, Y., Wang, L., Dai, Y., et al. (2021). Ultrasound Controlled Anti-Inflammatory Polarization of Platelet Decorated Microglia for Targeted Ischemic Stroke Therapy. Angew. Chem. Int. Ed. Engl. 60, 5083–5090. doi: 10.1002/anie.202010391
Liauw, J., Hoang, S., Choi, M., Eroglu, C., Choi, M., Sun, G. H., et al. (2008). Thrombospondins 1 and 2 are necessary for synaptic plasticity and functional recovery after stroke. J. Cereb. Blood Flow Metab. 28, 1722–1732. doi: 10.1038/jcbfm.2008.65
Liddelow, S. A., and Barres, B. A. (2017). Reactive Astrocytes: production, Function, and Therapeutic Potential. Immunity 46, 957–967. doi: 10.1016/j.immuni.2017.06.006
Liddelow, S. A., Guttenplan, K. A., Clarke, L. E., Bennett, F. C., Bohlen, C. J., Schirmer, L., et al. (2017). Neurotoxic reactive astrocytes are induced by activated microglia. Nature 541, 481–487. doi: 10.1038/nature21029
Liebner, S., Dijkhuizen, R. M., Reiss, Y., Plate, K. H., Agalliu, D., and Constantin, G. (2018). Functional morphology of the blood-brain barrier in health and disease. Acta Neuropathol. 135, 311–336. doi: 10.1007/s00401-018-1815-1
Liu, H., Hua, Y., Keep, R. F., and Xi, G. (2019). Brain Ceruloplasmin Expression After Experimental Intracerebral Hemorrhage and Protection Against Iron-Induced Brain Injury. Transl. Stroke Res. 10, 112–119. doi: 10.1007/s12975-018-0669-0
Liu, M., Xu, Z., Wang, L., Zhang, L., Liu, Y., Cao, J., et al. (2020). Cottonseed oil alleviates ischemic stroke injury by inhibiting the inflammatory activation of microglia and astrocyte. J. Neuroinflammation 17:270. doi: 10.1186/s12974-020-01946-7
Liu, Q., Cui, P., Zheng, K., Wang, J., Jiang, W., Liu, G., et al. (2020). SERPINA1 gene expression in whole blood links the rs6647 variant G allele to an increased risk of large artery atherosclerotic stroke. FASEB J. 34, 10107–10116. doi: 10.1096/fj.201903197R
Liu, Y., Han, S. S., Wu, Y., Tuohy, T. M., Xue, H., Cai, J., et al. (2004). CD44 expression identifies astrocyte-restricted precursor cells. Dev. Biol. 276, 31–46. doi: 10.1016/j.ydbio.2004.08.018
Loane, D. J., and Kumar, A. (2016). Microglia in the TBI brain: the good, the bad, and the dysregulated. Exp. Neurol. 275, 316–327. doi: 10.1016/j.expneurol.2015.08.018
Lovatt, D., Sonnewald, U., Waagepetersen, H. S., Schousboe, A., He, W., Lin, J. H., et al. (2007). The transcriptome and metabolic gene signature of protoplasmic astrocytes in the adult murine cortex. J. Neurosci. 27, 12255–12266. doi: 10.1523/jneurosci.3404-07.2007
Lukovic, D., Stojkovic, M., Moreno-Manzano, V., Bhattacharya, S. S., and Erceg, S. (2014). Perspectives and future directions of human pluripotent stem cell-based therapies: lessons from Geron’s clinical trial for spinal cord injury. Stem Cells Dev. 23, 1–4. doi: 10.1089/scd.2013.0266
Lundquist, A. J., Parizher, J., Petzinger, G. M., and Jakowec, M. W. (2019). Exercise induces region-specific remodeling of astrocyte morphology and reactive astrocyte gene expression patterns in male mice. J. Neurosci. Res. 97, 1081–1094. doi: 10.1002/jnr.24430
Luo, J., Wu, X., Liu, H., Cui, W., Guo, W., Guo, K., et al. (2021). Antagonism of Protease-Activated Receptor 4 Protects Against Traumatic Brain Injury by Suppressing Neuroinflammation via Inhibition of Tab2/NF-κB Signaling. Neurosci. Bull. 37, 242–254. doi: 10.1007/s12264-020-00601-8
Macheda, T., Roberts, K., Lyons, D. N., Higgins, E., Ritter, K. J., Lin, A. L., et al. (2019). Chronic Intermittent Hypoxia Induces Robust Astrogliosis in an Alzheimer’s Disease-Relevant Mouse Model. Neuroscience 398, 55–63. doi: 10.1016/j.neuroscience.2018.11.040
Mangold, C. A., Masser, D. R., Stanford, D. R., Bixler, G. V., Pisupati, A., Giles, C. B., et al. (2017). CNS-wide Sexually Dimorphic Induction of the Major Histocompatibility Complex 1 Pathway With Aging. J. Gerontol. A Biol. Sci. Med. Sci. 72, 16–29. doi: 10.1093/gerona/glv232
Manley, G. T., Fujimura, M., Ma, T., Noshita, N., Filiz, F., Bollen, A. W., et al. (2000). Aquaporin-4 deletion in mice reduces brain edema after acute water intoxication and ischemic stroke. Nat. Med. 6, 159–163. doi: 10.1038/72256
Marina, N., Christie, I. N., Korsak, A., Doronin, M., Brazhe, A., Hosford, P. S., et al. (2020). Astrocytes monitor cerebral perfusion and control systemic circulation to maintain brain blood flow. Nat. Commun. 11:131. doi: 10.1038/s41467-019-13956-y
Mathys, H., Davila-Velderrain, J., Peng, Z., Gao, F., Mohammadi, S., Young, J. Z., et al. (2019). Single-cell transcriptomic analysis of Alzheimer’s disease. Nature 570, 332–337. doi: 10.1038/s41586-019-1195-2
Mätlik, K., Anttila, J. E., Kuan-Yin, T., Smolander, O. P., Pakarinen, E., Lehtonen, L., et al. (2018). Poststroke delivery of MANF promotes functional recovery in rats. Sci. Adv. 4:eaa8957. doi: 10.1126/sciadv.aap8957
Mega, A., Hartmark Nilsen, M., Leiss, L. W., Tobin, N. P., Miletic, H., Sleire, L., et al. (2020). Astrocytes enhance glioblastoma growth. Glia 68, 316–327. doi: 10.1002/glia.23718
Michalovicz, L. T., Kelly, K. A., Vashishtha, S., Ben-Hamo, R., Efroni, S., Miller, J. V., et al. (2019). Astrocyte-specific transcriptome analysis using the ALDH1L1 bacTRAP mouse reveals novel biomarkers of astrogliosis in response to neurotoxicity. J. Neurochem. 150, 420–440. doi: 10.1111/jnc.14800
Min, H., Choi, B., Jang, Y. H., Cho, I. H., and Lee, S. J. (2017). Heme molecule functions as an endogenous agonist of astrocyte TLR2 to contribute to secondary brain damage after intracerebral hemorrhage. Mol. Brain 10:27. doi: 10.1186/s13041-017-0305-z
Morizawa, Y. M., Hirayama, Y., Ohno, N., Shibata, S., Shigetomi, E., Sui, Y., et al. (2017). Reactive astrocytes function as phagocytes after brain ischemia via ABCA1-mediated pathway. Nat. Commun. 8:28. doi: 10.1038/s41467-017-00037-1
Myer, D. J., Gurkoff, G. G., Lee, S. M., Hovda, D. A., and Sofroniew, M. V. (2006). Essential protective roles of reactive astrocytes in traumatic brain injury. Brain 129, 2761–2772. doi: 10.1093/brain/awl165
Nagai, J., Rajbhandari, A. K., Gangwani, M. R., Hachisuka, A., Coppola, G., Masmanidis, S. C., et al. (2019). Hyperactivity with Disrupted Attention by Activation of an Astrocyte Synaptogenic Cue. Cell 177, 1280–1292.e20. doi: 10.1016/j.cell.2019.03.019
Nardai, S., Dobolyi, A., Pál, G., Skopál, J., Pintér, N., Lakatos, K., et al. (2015). Selegiline promotes NOTCH-JAGGED signaling in astrocytes of the peri-infarct region and improves the functional integrity of the neurovascular unit in a rat model of focal ischemia. Restor. Neurol. Neurosci. 33, 1–14. doi: 10.3233/rnn-140420
Nayak, A. R., Kashyap, R. S., Kabra, D., Purohit, H. J., Taori, G. M., and Daginawala, H. F. (2012). Time course of inflammatory cytokines in acute ischemic stroke patients and their relation to inter-alfa trypsin inhibitor heavy chain 4 and outcome. Ann. Indian Acad. Neurol. 15, 181–185. doi: 10.4103/0972-2327.99707
Nishida, H., and Okabe, S. (2007). Direct astrocytic contacts regulate local maturation of dendritic spines. J. Neurosci. 27, 331–340. doi: 10.1523/jneurosci.4466-06.2007
Niu, W., Zang, T., Smith, D. K., Vue, T. Y., Zou, Y., Bachoo, R., et al. (2015). SOX2 reprograms resident astrocytes into neural progenitors in the adult brain. Stem Cell Rep. 4, 780–794. doi: 10.1016/j.stemcr.2015.03.006
Nuriya, M., Shinotsuka, T., and Yasui, M. (2013). Diffusion properties of molecules at the blood-brain interface: potential contributions of astrocyte endfeet to diffusion barrier functions. Cereb. Cortex 23, 2118–2126. doi: 10.1093/cercor/bhs198
Omari, K. M., John, G. R., Sealfon, S. C., and Raine, C. S. (2005). CXC chemokine receptors on human oligodendrocytes: implications for multiple sclerosis. Brain 128, 1003–1015. doi: 10.1093/brain/awh479
Orre, M., Kamphuis, W., Osborn, L. M., Melief, J., Kooijman, L., Huitinga, I., et al. (2014). Acute isolation and transcriptome characterization of cortical astrocytes and microglia from young and aged mice. Neurobiol. Aging 35, 1–14. doi: 10.1016/j.neurobiolaging.2013.07.008
Overman, J. J., Clarkson, A. N., Wanner, I. B., Overman, W. T., Eckstein, I., Maguire, J. L., et al. (2012). A role for ephrin-A5 in axonal sprouting, recovery, and activity-dependent plasticity after stroke. Proc. Natl. Acad. Sci. U. S. A. 109, E2230–E2239. doi: 10.1073/pnas.1204386109
Pan, J., Ma, N., Yu, B., Zhang, W., and Wan, J. (2020). Transcriptomic profiling of microglia and astrocytes throughout aging. J. Neuroinflammation 17:97. doi: 10.1186/s12974-020-01774-9
Peng, L., Zhou, Y., Jiang, N., Wang, T., Zhu, J., Chen, Y., et al. (2020). DJ-1 exerts anti-inflammatory effects and regulates NLRX1-TRAF6 via SHP-1 in stroke. J. Neuroinflammation 17:81. doi: 10.1186/s12974-020-01764-x
Pettigrew, L. C., Kasner, S. E., Albers, G. W., Gorman, M., Grotta, J. C., Sherman, D. G., et al. (2006). Safety and tolerability of arundic acid in acute ischemic stroke. J. Neurol. Sci. 251, 50–56. doi: 10.1016/j.jns.2006.09.001
Rajput, P. S., Lamb, J., Kothari, S., Pereira, B., Soetkamp, D., Wang, Y., et al. (2020). Neuron-generated thrombin induces a protective astrocyte response via protease activated receptors. Glia 68, 246–262. doi: 10.1002/glia.23714
Rajput, P. S., Lyden, P. D., Chen, B., Lamb, J. A., Pereira, B., Lamb, A., et al. (2014). Protease activated receptor-1 mediates cytotoxicity during ischemia using in vivo and in vitro models. Neuroscience 281, 229–240. doi: 10.1016/j.neuroscience.2014.09.038
Rakers, C., Schleif, M., Blank, N., Matušková, H., Ulas, T., Händler, K., et al. (2019). Stroke target identification guided by astrocyte transcriptome analysis. Glia 67, 619–633. doi: 10.1002/glia.23544
Sacristán, C. (2020). Microglia and Astrocyte Crosstalk in Immunity. Trends Immunol. 41, 747–748. doi: 10.1016/j.it.2020.07.009
Sanz, E., Yang, L., Su, T., Morris, D. R., McKnight, G. S., and Amieux, P. S. (2009). Cell-type-specific isolation of ribosome-associated mRNA from complex tissues. Proc. Natl. Acad. Sci. U. S. A. 106, 13939–13944. doi: 10.1073/pnas.0907143106
Schilling, M., Besselmann, M., Müller, M., Strecker, J. K., Ringelstein, E. B., and Kiefer, R. (2005). Predominant phagocytic activity of resident microglia over hematogenous macrophages following transient focal cerebral ischemia: an investigation using green fluorescent protein transgenic bone marrow chimeric mice. Exp. Neurol. 196, 290–297. doi: 10.1016/j.expneurol.2005.08.004
Seifert, H. A., Collier, L. A., Chapman, C. B., Benkovic, S. A., Willing, A. E., and Pennypacker, K. R. (2014). Pro-inflammatory interferon gamma signaling is directly associated with stroke induced neurodegeneration. J. Neuroimmune Pharmacol. 9, 679–689. doi: 10.1007/s11481-014-9560-2
Shan, Y., Tan, S., Lin, Y., Liao, S., Zhang, B., Chen, X., et al. (2019). The glucagon-like peptide-1 receptor agonist reduces inflammation and blood-brain barrier breakdown in an astrocyte-dependent manner in experimental stroke. J. Neuroinflammation 16:242. doi: 10.1186/s12974-019-1638-6
Shen, Y., Sun, A., Wang, Y., Cha, D., Wang, H., Wang, F., et al. (2012). Upregulation of mesencephalic astrocyte-derived neurotrophic factor in glial cells is associated with ischemia-induced glial activation. J. Neuroinflammation 9:254. doi: 10.1186/1742-2094-9-254
Sheth, K. N., Elm, J. J., Molyneaux, B. J., Hinson, H., Beslow, L. A., Sze, G. K., et al. (2016). Safety and efficacy of intravenous glyburide on brain swelling after large hemispheric infarction (GAMES-RP): a randomised, double-blind, placebo-controlled phase 2 trial. Lancet Neurol. 15, 1160–1169. doi: 10.1016/s1474-4422(16)30196-x
Shi, S. X., Li, Y. J., Shi, K., Wood, K., Ducruet, A. F., and Liu, Q. (2020). IL (Interleukin)-15 Bridges Astrocyte-Microglia Crosstalk and Exacerbates Brain Injury Following Intracerebral Hemorrhage. Stroke 51, 967–974. doi: 10.1161/strokeaha.119.028638
Shi, W., Huang, C. J., Xu, X. D., Jin, G. H., Huang, R. Q., Huang, J. F., et al. (2016). Transplantation of RADA16-BDNF peptide scaffold with human umbilical cord mesenchymal stem cells forced with CXCR4 and activated astrocytes for repair of traumatic brain injury. Acta Biomater. 45, 247–261. doi: 10.1016/j.actbio.2016.09.001
Shi, X., Luo, L., Wang, J., Shen, H., Li, Y., Mamtilahun, M., et al. (2021). Stroke subtype-dependent synapse elimination by reactive gliosis in mice. Nat. Commun. 12:6943. doi: 10.1038/s41467-021-27248-x
Shi, Z. F., Fang, Q., Chen, Y., Xu, L. X., Wu, M., Jia, M., et al. (2021). Methylene blue ameliorates brain edema in rats with experimental ischemic stroke via inhibiting aquaporin 4 expression. Acta Pharmacol. Sin. 42, 382–392. doi: 10.1038/s41401-020-0468-5
Silver, J., and Miller, J. H. (2004). Regeneration beyond the glial scar. Nat. Rev. Neurosci. 5, 146–156. doi: 10.1038/nrn1326
Simard, M., Arcuino, G., Takano, T., Liu, Q. S., and Nedergaard, M. (2003). Signaling at the gliovascular interface. J. Neurosci. 23, 9254–9262. doi: 10.1523/jneurosci.23-27-09254.2003
Sivenius, J., Sarasoja, T., Aaltonen, H., Heinonen, E., Kilkku, O., and Reinikainen, K. (2001). Selegiline treatment facilitates recovery after stroke. Neurorehabil. Neural Repair 15, 183–190. doi: 10.1177/154596830101500305
Sobrino, T., Rodríguez-Yáñez, M., Campos, F., Iglesias-Rey, R., Millán, M., de la Ossa, N. P., et al. (2020). Association of High Serum Levels of Growth Factors with Good Outcome in Ischemic Stroke: a Multicenter Study. Transl. Stroke Res. 11, 653–663. doi: 10.1007/s12975-019-00747-2
Sofroniew, M. V. (2014). Multiple roles for astrocytes as effectors of cytokines and inflammatory mediators. Neuroscientist 20, 160–172. doi: 10.1177/1073858413504466
Stevens, B., Allen, N. J., Vazquez, L. E., Howell, G. R., Christopherson, K. S., Nouri, N., et al. (2007). The classical complement cascade mediates CNS synapse elimination. Cell 131, 1164–1178. doi: 10.1016/j.cell.2007.10.036
Sun, D., and Jakobs, T. C. (2012). Structural remodeling of astrocytes in the injured CNS. Neuroscientist 18, 567–588. doi: 10.1177/1073858411423441
Sun, J., Huang, Y., Gong, J., Wang, J., Fan, Y., Cai, J., et al. (2020). Transplantation of hPSC-derived pericyte-like cells promotes functional recovery in ischemic stroke mice. Nat. Commun. 11:5196. doi: 10.1038/s41467-020-19042-y
Sun, W., Cornwell, A., Li, J., Peng, S., Osorio, M. J., Aalling, N., et al. (2017). SOX9 Is an Astrocyte-Specific Nuclear Marker in the Adult Brain Outside the Neurogenic Regions. J. Neurosci. 37, 4493–4507. doi: 10.1523/jneurosci.3199-16.2017
Tejeda, G. S., Esteban-Ortega, G. M., San Antonio, E., Vidaurre, Ó.G., and Díaz-Guerra, M. (2019). Prevention of excitotoxicity-induced processing of BDNF receptor TrkB-FL leads to stroke neuroprotection. EMBO Mol. Med. 11:e9950. doi: 10.15252/emmm.201809950
Thored, P., Arvidsson, A., Cacci, E., Ahlenius, H., Kallur, T., Darsalia, V., et al. (2006). Persistent production of neurons from adult brain stem cells during recovery after stroke. Stem Cells 24, 739–747. doi: 10.1634/stemcells.2005-0281
Tian, W., and Kyriakides, T. R. (2009). Matrix metalloproteinase-9 deficiency leads to prolonged foreign body response in the brain associated with increased IL-1beta levels and leakage of the blood-brain barrier. Matrix Biol. 28, 148–159. doi: 10.1016/j.matbio.2009.02.002
Todd, A. C., and Hardingham, G. E. (2020). The Regulation of Astrocytic Glutamate Transporters in Health and Neurodegenerative Diseases. Int. J. Mol. Sci. 21:9607. doi: 10.3390/ijms21249607
Tokumine, J., Kakinohana, O., Cizkova, D., Smith, D. W., and Marsala, M. (2003). Changes in spinal GDNF, BDNF, and NT-3 expression after transient spinal cord ischemia in the rat. J. Neurosci. Res. 74, 552–561. doi: 10.1002/jnr.10760
Touma, C., Gassen, N. C., Herrmann, L., Cheung-Flynn, J., Büll, D. R., Ionescu, I. A., et al. (2011). FK506 binding protein 5 shapes stress responsiveness: modulation of neuroendocrine reactivity and coping behavior. Biol. Psychiatry 70, 928–936. doi: 10.1016/j.biopsych.2011.07.023
Toyoda, K., Uchiyama, S., Yamaguchi, T., Easton, J. D., Kimura, K., Hoshino, H., et al. (2019). Dual antiplatelet therapy using cilostazol for secondary prevention in patients with high-risk ischaemic stroke in Japan: a multicentre, open-label, randomised controlled trial. Lancet Neurol. 18, 539–548. doi: 10.1016/s1474-4422(19)30148-6
Tsai, T. H., Lu, C. H., Wallace, C. G., Chang, W. N., Chen, S. F., Huang, C. R., et al. (2015). Erythropoietin improves long-term neurological outcome in acute ischemic stroke patients: a randomized, prospective, placebo-controlled clinical trial. Crit. Care 19:49. doi: 10.1186/s13054-015-0761-8
Uno, M., Kitazato, K. T., Suzue, A., Matsuzaki, K., Harada, M., Itabe, H., et al. (2005). Inhibition of brain damage by edaravone, a free radical scavenger, can be monitored by plasma biomarkers that detect oxidative and astrocyte damage in patients with acute cerebral infarction. Free Radic. Biol. Med. 39, 1109–1116. doi: 10.1016/j.freeradbiomed.2005.06.001
Van Doorn, R., Van Horssen, J., Verzijl, D., Witte, M., Ronken, E., Van Het Hof, B., et al. (2010). Sphingosine 1-phosphate receptor 1 and 3 are upregulated in multiple sclerosis lesions. Glia 58, 1465–1476. doi: 10.1002/glia.21021
Wagner, D. C., Scheibe, J., Glocke, I., Weise, G., Deten, A., Boltze, J., et al. (2013). Object-based analysis of astroglial reaction and astrocyte subtype morphology after ischemic brain injury. Acta Neurobiol. Exp. 73, 79–87.
Wang, F. J., Wang, S. X., Chai, L. J., Zhang, Y., Guo, H., and Hu, L. M. (2018). Xueshuantong injection (lyophilized) combined with salvianolate lyophilized injection protects against focal cerebral ischemia/reperfusion injury in rats through attenuation of oxidative stress. Acta Pharmacol. Sin. 39, 998–1011. doi: 10.1038/aps.2017.128
Wang, J., Chen, Z., Walston, J. D., Gao, P., Gao, M., and Leng, S. X. (2019). Interferon-γ Potentiates α-Synuclein-induced Neurotoxicity Linked to Toll-like Receptors 2 and 3 and Tumor Necrosis Factor-α in Murine Astrocytes. Mol. Neurobiol. 56, 7664–7679. doi: 10.1007/s12035-019-1567-5
Wang, L. L., Serrano, C., Zhong, X., Ma, S., Zou, Y., and Zhang, C. L. (2021). Revisiting astrocyte to neuron conversion with lineage tracing in vivo. Cell 184, 5465–5481.e16. doi: 10.1016/j.cell.2021.09.005
Wang, Y., Jin, S., Sonobe, Y., Cheng, Y., Horiuchi, H., Parajuli, B., et al. (2014). Interleukin-1β induces blood-brain barrier disruption by downregulating Sonic hedgehog in astrocytes. PLoS One 9:e110024. doi: 10.1371/journal.pone.0110024
Wiese, S., Karus, M., and Faissner, A. (2012). Astrocytes as a source for extracellular matrix molecules and cytokines. Front. Pharmacol. 3:120. doi: 10.3389/fphar.2012.00120
Wilhelmus, M. M., Otte-Höller, I., Wesseling, P., de Waal, R. M., Boelens, W. C., and Verbeek, M. M. (2006). Specific association of small heat shock proteins with the pathological hallmarks of Alzheimer’s disease brains. Neuropathol. Appl. Neurobiol. 32, 119–130. doi: 10.1111/j.1365-2990.2006.00689.x
Wu, S., Wu, B., Liu, M., Chen, Z., Wang, W., Anderson, C. S., et al. (2019). Stroke in China: advances and challenges in epidemiology, prevention, and management. Lancet Neurol. 18, 394–405. doi: 10.1016/S1474-4422(18)30500-3
Wu, X., Luo, J., Liu, H., Cui, W., Guo, K., Zhao, L., et al. (2020). Recombinant Adiponectin Peptide Ameliorates Brain Injury Following Intracerebral Hemorrhage by Suppressing Astrocyte-Derived Inflammation via the Inhibition of Drp1-Mediated Mitochondrial Fission. Transl. Stroke Res. 11, 924–939. doi: 10.1007/s12975-019-00768-x
Xu, M., Ma, Q., Fan, C., Chen, X., Zhang, H., and Tang, M. (2019). Ginsenosides Rb1 and Rg1 Protect Primary Cultured Astrocytes against Oxygen-Glucose Deprivation/Reoxygenation-Induced Injury via Improving Mitochondrial Function. Int. J. Mol. Sci. 20:6086. doi: 10.3390/ijms20236086
Xue, B., Jiao, J., Zhang, L., Li, K. R., Gong, Y. T., Xie, J. X., et al. (2007). Triptolide upregulates NGF synthesis in rat astrocyte cultures. Neurochem. Res. 32, 1113–1119. doi: 10.1007/s11064-006-9253-1
Yamada, T., Kawamata, T., Walker, D. G., and McGeer, P. L. (1992). Vimentin immunoreactivity in normal and pathological human brain tissue. Acta Neuropathol. 84, 157–162. doi: 10.1007/bf00311389
Yang, H., Xu, W., Zhao, W., Gu, M., and Wang, W. (2018). 1,3,7-Trihydroxyxanthone, derived from Polygalae Radix, a herbal medicine, stimulates the expression of neurotrophic factors in rat astrocyte primary cultures via cAMP- and ERK-dependent pathways. Biomed. Pharmacother. 98, 762–768. doi: 10.1016/j.biopha.2017.12.085
Yang, J., Vitery, M. D. C., Chen, J., Osei-Owusu, J., Chu, J., and Qiu, Z. (2019). Glutamate-Releasing SWELL1 Channel in Astrocytes Modulates Synaptic Transmission and Promotes Brain Damage in Stroke. Neuron 102, 813–827.e6. doi: 10.1016/j.neuron.2019.03.029
Yang, X. (2020). Chondroitin sulfate proteoglycans: key modulators of neuronal plasticity, long-term memory, neurodegenerative, and psychiatric disorders. Rev. Neurosci. 31, 555–568. doi: 10.1515/revneuro-2019-0117
Yao, Y., Chen, Z. L., Norris, E. H., and Strickland, S. (2014). Astrocytic laminin regulates pericyte differentiation and maintains blood brain barrier integrity. Nat. Commun. 5:3413. doi: 10.1038/ncomms4413
Yu, X., Nagai, J., and Khakh, B. S. (2020). Improved tools to study astrocytes. Nat. Rev. Neurosci. 21, 121–138. doi: 10.1038/s41583-020-0264-8
Yu, X., Taylor, A. M. W., Nagai, J., Golshani, P., Evans, C. J., Coppola, G., et al. (2018). Reducing Astrocyte Calcium Signaling In Vivo Alters Striatal Microcircuits and Causes Repetitive Behavior. Neuron 99, 1170–1187.e9. doi: 10.1016/j.neuron.2018.08.015
Yu, Y., Qin, N., Lu, X. A., Li, J., Han, X., Ni, X., et al. (2019). Human embryonic stem cell-derived cardiomyocyte therapy in mouse permanent ischemia and ischemia-reperfusion models. Stem Cell Res. Ther. 10:167. doi: 10.1186/s13287-019-1271-4
Zamanian, J. L., Xu, L., Foo, L. C., Nouri, N., Zhou, L., Giffard, R. G., et al. (2012). Genomic analysis of reactive astrogliosis. J. Neurosci. 32, 6391–6410. doi: 10.1523/jneurosci.6221-11.2012
Zamboni, M., Llorens-Bobadilla, E., Magnusson, J. P., and Frisén, J. (2020). A Widespread Neurogenic Potential of Neocortical Astrocytes Is Induced by Injury. Cell Stem Cell 27, 605–617.e5. doi: 10.1016/j.stem.2020.07.006
Zhang, H., Xue, W., Xue, X., Fan, Y., Yang, Y., Zhao, Y., et al. (2021). Spatiotemporal dynamic changes, proliferation, and differentiation characteristics of Sox9-positive cells after severe complete transection spinal cord injury. Exp. Neurol. 337:113556. doi: 10.1016/j.expneurol.2020.113556
Zhang, L., Yin, J. C., Yeh, H., Ma, N. X., Lee, G., Chen, X. A., et al. (2015). Small Molecules Efficiently Reprogram Human Astroglial Cells into Functional Neurons. Cell Stem Cell 17, 735–747. doi: 10.1016/j.stem.2015.09.012
Zhang, Q., Liu, C., Shi, R., Zhou, S., Shan, H., Deng, L., et al. (2021). Blocking C3d+/GFAP+ A1 Astrocyte Conversion with Semaglutide Attenuates Blood-Brain Barrier Disruption in Mice after Ischemic Stroke. Aging Dis. 2021. doi: 10.14336/AD.2021.1029
Zhang, R. L., LeTourneau, Y., Gregg, S. R., Wang, Y., Toh, Y., Robin, A. M., et al. (2007). Neuroblast division during migration toward the ischemic striatum: a study of dynamic migratory and proliferative characteristics of neuroblasts from the subventricular zone. J. Neurosci. 27, 3157–3162. doi: 10.1523/jneurosci.4969-06.2007
Zhang, Y., Sloan, S. A., Clarke, L. E., Caneda, C., Plaza, C. A., Blumenthal, P. D., et al. (2016). Purification and Characterization of Progenitor and Mature Human Astrocytes Reveals Transcriptional and Functional Differences with Mouse. Neuron 89, 37–53. doi: 10.1016/j.neuron.2015.11.013
Zhang, Y., Zhang, H., Lin, S., Chen, X., Yao, Y., Mao, X., et al. (2018). SDF-1/CXCR7 Chemokine Signaling is Induced in the Peri-Infarct Regions in Patients with Ischemic Stroke. Aging Dis. 9, 287–295. doi: 10.14336/ad.2017.1112
Zhao, H., Liu, Y., Cheng, L., Liu, B., Zhang, W., Guo, Y. J., et al. (2013). Mesencephalic astrocyte-derived neurotrophic factor inhibits oxygen-glucose deprivation-induced cell damage and inflammation by suppressing endoplasmic reticulum stress in rat primary astrocytes. J. Mol. Neurosci. 51, 671–678. doi: 10.1007/s12031-013-0042-4
Zhao, Y., and Rempe, D. A. (2010). Targeting astrocytes for stroke therapy. Neurotherapeutics 7, 439–451. doi: 10.1016/j.nurt.2010.07.004
Zheng, R. F., Du, Y. W., Zeng, C., Wang, H. F., Xing, J. G., and Xu, M. (2020). Total flavones of Dracocephalum moldavica L. protect astrocytes against H(2)O(2)-induced apoptosis through a mitochondria-dependent pathway. BMC Complement Med. Ther. 20:78. doi: 10.1186/s12906-020-2846-4
Zhu, H., Zou, L., Tian, J., Du, G., and Gao, Y. (2013). SMND-309, a novel derivative of salvianolic acid B, protects rat brains ischemia and reperfusion injury by targeting the JAK2/STAT3 pathway. Eur. J. Pharmacol. 714, 23–31. doi: 10.1016/j.ejphar.2013.05.043
Keywords: stroke, reactive astrocyte, polarization, blood brain barrier (BBB), neuro-inflammation
Citation: Li L, Zhou J, Han L, Wu X, Shi Y, Cui W, Zhang S, Hu Q, Wang J, Bai H, Liu H, Guo W, Feng D and Qu Y (2022) The Specific Role of Reactive Astrocytes in Stroke. Front. Cell. Neurosci. 16:850866. doi: 10.3389/fncel.2022.850866
Received: 08 January 2022; Accepted: 15 February 2022;
Published: 07 March 2022.
Edited by:
Anwen Shao, Zhejiang University, ChinaReviewed by:
Maria Cristina Ortega, National Paraplegic Hospital, SpainCopyright © 2022 Li, Zhou, Han, Wu, Shi, Cui, Zhang, Hu, Wang, Bai, Liu, Guo, Feng and Qu. This is an open-access article distributed under the terms of the Creative Commons Attribution License (CC BY). The use, distribution or reproduction in other forums is permitted, provided the original author(s) and the copyright owner(s) are credited and that the original publication in this journal is cited, in accordance with accepted academic practice. No use, distribution or reproduction is permitted which does not comply with these terms.
*Correspondence: Yan Qu, eWFucXUwMTIzQGZtbXUuZWR1LmNu
†These authors have contributed equally to this work and share first authorship
Disclaimer: All claims expressed in this article are solely those of the authors and do not necessarily represent those of their affiliated organizations, or those of the publisher, the editors and the reviewers. Any product that may be evaluated in this article or claim that may be made by its manufacturer is not guaranteed or endorsed by the publisher.
Research integrity at Frontiers
Learn more about the work of our research integrity team to safeguard the quality of each article we publish.