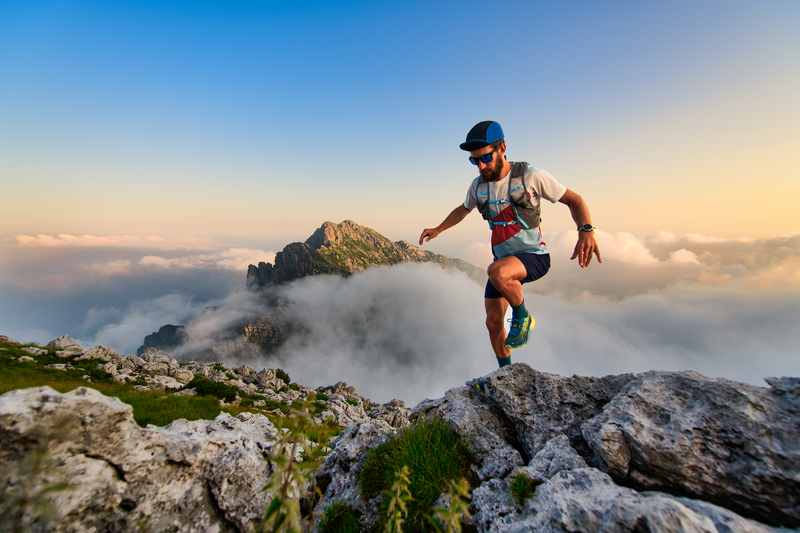
95% of researchers rate our articles as excellent or good
Learn more about the work of our research integrity team to safeguard the quality of each article we publish.
Find out more
OPINION article
Front. Cell. Neurosci. , 31 January 2022
Sec. Non-Neuronal Cells
Volume 16 - 2022 | https://doi.org/10.3389/fncel.2022.831747
This article is part of the Research Topic Glial Tiling in the Nervous System View all 5 articles
Microglia are brain-resident macrophages that carry out immune surveillance, support neurogenesis and neuronal survival, shape the neuronal network, and maintain tissue homeostasis (Nimmerjahn et al., 2005; Hanisch and Kettenmann, 2007; Sierra et al., 2010; Tremblay et al., 2010; Schafer et al., 2012; Ueno et al., 2013; Squarzoni et al., 2014; Schafer and Stevens, 2015; Diaz-Aparicio et al., 2020). The adult resident pool of microglia is primarily derived from yolk sac (YS) erythromyeloid progenitors (EMPs) that have clonally proliferated within the brain parenchyma during development (Alliot et al., 1999; Ginhoux et al., 2010; Hashimoto et al., 2013; Gomez Perdiguero et al., 2015). To maintain their cell density in adulthood, the resident microglial cells undergo local clonal self-renewal (Ajami et al., 2007; Askew et al., 2017; Réu et al., 2017; Tay et al., 2017). A study on parabiotic chimeric mice revealed that the resident microglial population is exclusively replenished by locally derived microglial clones with no evidence of contribution from peripheral myeloid progenitors (Ajami et al., 2007). Clonal expansion of non-ablated residual microglia was also found to be responsible for re-establishing steady state microglial cell densities following a pharmacological ablation (Huang et al., 2018). Homeostatic microglia continuously monitor the brain environment, scavenge dying cells and cellular debris, and rapidly respond to any tissue damage (Kreutzberg, 1996; Streit et al., 1999). In response to acute pathology, resident microglia rapidly accumulate around the lesion via clonal microgliosis (Streit et al., 1999; Ladeby et al., 2005; Ajami et al., 2007; Ransohoff, 2007). Microgliosis at the site of CNS damage has been shown to be governed by signaling molecules like colony-stimulating factor-1 (CSF1), fractalkine receptor (CX3CR1) and purinergic receptor P2Y12 (P2RY12) (Guan et al., 2016; Gu et al., 2016; Peng et al., 2016). To repair damage, microglia elicit proinflammatory cytokines and later transition to anti-inflammatory phenotypes (Colton, 2009; Lloyd et al., 2019). Several studies have suggested that clinical recovery of acute lesions is accompanied by the resolution of proliferated microglia by migration and cell death (Dihné et al., 2001; Wilson et al., 2004; Tay et al., 2017; Lloyd et al., 2019). However, in severe or chronic neurodegenerative pathologies like Alzheimer's disease (AD), Parkinson's disease (PD), multiple sclerosis (MS), and motor neuron diseases, microglial clonal expansion has been persistently observed around lesions and plaques (Glass et al., 2010; Streit et al., 2020). In AD and MS animal models, microglial proliferation around the Aβ plaques and demyelinating neurons was actively promoted by CSF1R and triggering receptor expressed on myeloid cells 2 (TREM2) (Cantoni et al., 2015; Olmos-Alonso et al., 2016; Wang et al., 2016; Jay et al., 2017; Gushchina et al., 2018; Zhao et al., 2018). CSF1R, transforming growth factor beta (TGFβ), and purinergic signaling pathways that influence microglial cell densities are impaired in neurodegenerative diseases (Gómez-Nicola et al., 2013; Von Bernhardi et al., 2015; Olmos-Alonso et al., 2016; Pietrowski et al., 2021). Taken together, the capacity for microglial clonal expansion, proliferation, or renewal, clearly play an important function across CNS development, health, and disease. Although clonal expansion and renewal of microglia appears necessary for physiological brain development, maintenance of CNS health, and response to acute damage, whether microglial clones are beneficial or detrimental in chronic neurodegeneration remains unclear.
In this opinion article, we discuss the implications of the formation of microglial clones in health and disease, independent of peripheral myeloid recruitment to the CNS in similar contexts (Figure 1). Several studies have claimed that microglia surrounding plaques and lesions exert detrimental effects and exacerbate disease conditions (Streit et al., 2009; Lassmann et al., 2012; Keren-Shaul et al., 2017; Krasemann et al., 2017; Shahidehpour et al., 2021). Considering that the restoration of tissue homeostasis coincides with the resolution of microglial clones to regain steady state microglial tiling (Dihné et al., 2001; Wilson et al., 2004; Tay et al., 2017; Lloyd et al., 2019), we hypothesize that unresolved microglial clones contribute to the prolongation of neurodegenerative states in chronic neuropathologies. As a hallmark of CNS pathology, microgliosis is likely important to limit tissue damage and infection and for local repair, as is typical in inflammatory responses of tissue-resident macrophages (Jenkins et al., 2011). A timely resolution of excess microglia resulting from clonal expansion is expected to aid or accompany the restoration of homeostasis and clinical recovery. However, sustained presence of reactive microglial clones at high densities around lesions or plaques with no signs of resolution likely lead to neurotoxic outcomes. To explore our hypothesis, we examine various contexts in which microglial clonal expansion has taken place and consider if strategic targeting of microglial clones could ameliorate chronic disease states.
Figure 1. Microglial clonality in development, health, and disease. Yolk sac EMPs enter the mouse neuroepithelium from E8 to E11.5 where they clonally expand and colonize the brain niche. An additional influx of Hoxb8+ microglial precursors from the AGM and fetal liver at E12.5 invade and populate the brain between E14.5 and E15.5 to make up the rest of the postnatal resident microglial population. Microglia clonally repopulate after ablation via self-renewal (left panel). In acute injury, microglia respond by microgliosis (clonal proliferation) at the site of damage (center panel). With clinical recovery, clones of microglia are reorganized by microglial cell migration and cell death (center panel). In chronic neurodegeneration such as AD, PD and MS, microglial clones persist around Aβ plaques, neurofibrillary tangles (tauopathy), and demyelinating neurons (right panel). Altered gene regulation and signaling pathways mentioned in the text are indicated. Created with BioRender.com.
Resident microglia of the CNS arise from clones of early EMPs during development. Starting from embryonic day 8 (E8), the first microglial progenitor was observed to migrate from the YS into the neural tube (Alliot et al., 1999). Microglial precursors positive for the F4/80 macrophage marker and proliferating cell nuclear antigen (PCNA) indicated that their active proliferation led to rapid microglial population of the brain parenchyma during embryonic and postnatal stages (Alliot et al., 1999). Fate mapping experiments identified YS EMPs as a common origin for all tissue-resident macrophages from brain microglia to other long-lasting tissue macrophages in the heart, liver, kidney, lungs, and intestines (Ginhoux et al., 2010; Hashimoto et al., 2013; Gomez Perdiguero et al., 2015). Yolk sac-derived microglial progenitors were reported to enter the brain parenchyma in a single wave up to E11.5 and locally proliferate to form the eventual adult resident microglial population (Ginhoux et al., 2010). However, the drastic increase in microglial cell number between E14.5 and E15.5, despite a decline in proliferating microglia, suggested the possibility of a second source of microglial cells that contribute to the resident microglial pool (Swinnen et al., 2013). Notably, genetic targeting of microglial progenitors from the YS have never reached close to 100 % (Ginhoux et al., 2010; Goldmann et al., 2016). Vav1-Cre+: dicerfl/fl mice were used to study the potential contribution of post-YS definitive hematopoiesis to resident microglia by targeting the microRNA-processing ribonuclease Dicer to deplete any microglia that arise after E11 (Fehrenbach et al., 2018). Dicer knock-out in Vav1-expressing hematopoietic cells caused a reduction of ~40% of total IBA1+ microglia in comparison to controls at postnatal day (P) 1, suggesting a contribution of post-YS hematopoiesis to the microglial population and/or defective microglial recruitment in the mutants (Fehrenbach et al., 2018). Another interesting finding was the presence of the Hoxb8+ microglial subpopulation, which makes up ~25% of the total microglial population in the adult cortex (Chen et al., 2010; De et al., 2018). These microglia were found to be of the Hoxb8 progenitor lineage, which originates in the aorta-gonad-mesonephros (AGM) and fetal liver, where these progenitors selectively proliferate and infiltrate the developing brain at E12.5 (De et al., 2018). Collectively, YS, AGM and fetal liver microglial precursors clonally self-renew to maintain the steady state microglial pool (Figure 1).
Microglia influence early embryonic forebrain development and the wiring of neuronal circuits (Li et al., 2019). At P3, F4/80+ microglia appear to play a role in eliminating apoptotic Purkinje cells of the developing cerebellum (Marin-Teva et al., 2004). In the first two postnatal weeks, microglia were described to aid synaptogenesis as well as actively prune synapses and engulf synaptic elements via CR3/C3 and fractalkine signaling (Paolicelli et al., 2011; Schafer et al., 2012; Miyamoto et al., 2016). Defective microglia and axonogenesis in Cx3cr1−/−, CR3−/−, and DAP12−/− mice suggested that forebrain microglia modulate dopaminergic axonal outgrowth and shape the laminar positioning of neocortical intraneuronal subsets prior to birth (Squarzoni et al., 2014). From P2 to P7, several studies have reported a specific proliferative CD11c+ CX3CR1+ GPNMB+ CLEC7A+ microglial subset within the developing white matter that was proposed to support myelination and neurogenesis in an insulin-like growth factor 1-dependent manner (Hagemeyer et al., 2017; Wlodarczyk et al., 2017; Li et al., 2019). The brief appearance of these proliferative white matter-associated microglia temporally aligned with the onset of myelination and the death of ~50% of newly formed oligodendrocytes (Barres et al., 1992; Trapp et al., 1997). This proliferative microglial subset purportedly phagocytoses dead oligodendrocytes to free up extracellular space for developing neurons and regulate homeostasis, suggesting a supportive role of these transient perinatal microglial clones (Li et al., 2019). The over two-fold increase in CD11b+ microglia peaked by the second postnatal week, followed by a 50% decline in microglial cell density due to reduced microglial proliferation and increased apoptosis from the third to sixth postnatal week, thereafter achieving steady state numbers (Nikodemova et al., 2015). In summary, microglia are basically clonal derivatives of early macrophage precursors from YS and non-YS hematopoietic sources, resulting in a heterogeneous population of microglia in the brain parenchyma. Furthermore, the various perinatal clonal microglial subsets play a supportive and beneficial role in early neuronal network development and maturation, synapse formation, synaptic pruning, and oligodendrogenesis (Barres et al., 1992; Li et al., 2019; Bennett and Bennett, 2020).
The ability of microglia to clonally self-renew in every niche of the brain without recruitment of macrophages from other brain-associated compartments or bone marrow stem cells suggests a certain level of plasticity for microglia to maintain their normal cell population in the CNS (Tay et al., 2017). Adult resident microglia undergo proliferation and apoptosis to maintain steady state cell densities (Hashimoto et al., 2013; Askew et al., 2017). On average, microglia self-renew at a proliferation rate of 0.69% in mice and 2% in humans (Askew et al., 2017). In different brain regions, adult resident microglia were predicted to randomly and completely turnover in every 8, 15, and 41 months in the murine olfactory bulb, hippocampus, and cortex, respectively (Tay et al., 2017). The majority of human cortical microglia have an average lifespan of 4.2 years (Réu et al., 2017). Signaling through the CSF1R and interleukin (IL)-1 receptors were shown to be necessary for the maintenance and self-renewal of the microglial population (Bruttger et al., 2015; Zhan et al., 2019). Furthermore, CX3CR1 signaling appeared to be required for the regulation of homeostatic microglial cell density (Zhan et al., 2014).
Microglial depletion studies by genetic or pharmacological approaches have revealed that residual microglial clones reestablish the complete microglial population by clonal expansion (Figure 1). However, transient removal of microglia from the brain is not without adverse consequences. Genetic ablation of 99% of microglia involved administering diphtheria toxin to mouse lines with the expression of diphtheria toxin receptor regulated by the promoter activity of the Cx3cr1 gene, later leading to loss of synaptic structural plasticity (Parkhurst et al., 2013). A pharmacological method involving the blockade of CSF1R with an inhibitor also resulted in microglial ablation (Elmore et al., 2014; Spangenberg et al., 2019). Sudden loss of microglia in the adult murine brain creates a dramatic change in the brain environment and a drastic downregulation of certain proinflammatory cytokines (e.g., tumor necrosis factor (TNF)-α and IL-1β), chemokines (CXCL9, CXCL10, CCL2, CCL5, and CCL7), and costimulatory molecules (CD80 and CD86) (Bruttger et al., 2015). Studies have identified glia to be major producers of TNF-α, which is also necessary for maintaining synaptic strength and excitatory synapses (Beattie et al., 2002; Stellwagen and Malenka, 2006). Microglia also produce brain-derived neurotrophic factor (BDNF) that mediates synaptic plasticity and synaptogenesis (Parkhurst et al., 2013; Zhou et al., 2019). Purinergic signaling between microglia and neurons was shown to control neuronal activity and confer neuroprotection (Eyo et al., 2014; Badimon et al., 2020). Cortical two-photon imaging of awake mice revealed that noradrenergic signaling through microglial β2-adrenergic receptors affects microglial process dynamics and surveillance (Liu et al., 2019; Stowell et al., 2019). Therefore, the loss of microglia could disrupt physiological neuronal network functions. Transient microglial ablation using the CSF1R inhibitor PLX5622 showed that the absence of microglia in the adult mouse brain caused odor response deficits in the developing granule cells of the olfactory bulb (Wallace et al., 2020). Post-mortem human homozygous CSF1R mutants revealed that complete absence of microglia was comorbid with underdeveloped corpus callosum and several structural anomalies of the brain (Oosterhof et al., 2019). In contrast to a hereditary lack of microglia, the CNS niche was instantaneously repopulated within a very short span of time after experimental microglial depletion in mouse models, indicating an innate inclination to restore healthy microglial tiling whenever possible (Varvel et al., 2012; Elmore et al., 2014; Han et al., 2017). In the case of partial (80%) microglial depletion, repopulation of microglial cells after ablation was contributed by the remaining internal source of parenchymal microglia (Bruttger et al., 2015; Askew et al., 2017). NF-κB signaling contributes to the restoration of homeostatic microglial cell density achieved through self-renewal, local clonal expansion, and the activation of maturation programs (Zhan et al., 2019). Notably, some microglial ablation studies have shown that peripheral myeloid cells partially repopulate the microglial niche in the absence of CNS irradiation (Varvel et al., 2012; Cronk et al., 2018), potentially also via clonal expansion (Shemer et al., 2018). Altogether, we can infer that in the process of regaining steady state, the CNS does not remain deprived of microglia for long periods in the absence of any interventions to block their survival. This is evident from the instantaneous proliferation and repopulation of these important CNS-resident macrophages by residual microglia. Collectively, clonal renewal and expansion of microglia within the brain parenchyma appear to be necessary and protective against damaging impacts on the CNS, which do not seem to tolerate any prolonged absence of microglia.
Microgliosis is the active proliferation of microglial cells in response to pathology. This was initially demonstrated in a sciatic nerve injury model, where neuroglia were reported to proliferate in the spinal cord of irradiated rats after sciatic nerve transection (Gilmore, 1975). Following sciatic nerve injury, the increase in anti-CD11b antibody (OX-42)-stained cells in the spinal cord and brain stem areas suggested the proliferation of microglial cells (Eriksson et al., 1993). In other examples, 3 days after peripheral nerve injury, the BrdU-labeled cells that were mostly IBA1+ microglia in the injured spinal cord increased by 24 and 11 folds in the dorsal and ventral horn, respectively, in comparison to the intact rat brain (Echeverry et al., 2008). Ten days after intraorbital optic nerve transection, there was a peak in the number of BrdU+ F4/80+ retinal microglia (Wohl et al., 2010). Yuan and colleagues described a morphologically unique BrdU+ IBA1+ “rod” microglia that underwent local microgliosis peaking at 7 days following optic nerve transection, without evidence of peripheral monocyte infiltration (Yuan et al., 2015). Clonally expanded Ki67+ IBA1+ microglia, also indicated by fluorescent Confetti markers, were observed within the injured facial nucleus in the facial nerve axotomy acute neurodegeneration model where the number of microglial cells increased by eight folds (Tay et al., 2017). At 3–5 days post excitotoxic lesions, a high number of IB-4+ microglial cells, which were mostly PCNA-positive indicating active proliferation, were found in the ipsilateral substantia nigra pars reticulata (Dihné et al., 2001). Peripheral nerve injury models showed the influence of CSF1R signaling in microgliosis, which is also partially regulated by CX3CR1 and purinergic P2Y12 signaling (Guan et al., 2016; Gu et al., 2016; Peng et al., 2016). Following acute demyelination in the mouse spinal cord, single-cell RNA-sequencing (scRNAseq) revealed a subpopulation of microglia with an activated profile characterized by an increase in interferon signaling (Plemel et al., 2020).
In acute CNS pathologies, clonally expanded microglial populations were observed to resolve (Figure 1). Clinical recovery is accompanied by the reorganization of accumulated microglial clones in the area of injury or damage. In demyelination mouse models based on parabiosis and white matter demyelinating lesions, a transition from proinflammatory to an increasingly anti-inflammatory microglial cell population occurs at the initiation of remyelination in the white matter regions (Miron et al., 2013). Microglial subsets near the lesion sites displayed proinflammatory phenotypes such as high levels of expression of cytokines, including IL1 (specifically IL1β), IL-6, and TNFα (Basu et al., 2002; Li et al., 2003; Yang et al., 2004; Sato et al., 2012). In the lysophosphatidylcholine-induced demyelination mouse model, the transition from a proinflammatory to a pro-regenerative microglial phenotype is accompanied by the necroptosis of proinflammatory microglia before the onset of remyelination (Lloyd et al., 2019). By 10 days post-lesion, pro-regenerative microglia upregulate genes associated with remyelination (e.g., Matn2, Osm, Fgf1, and Cd300lf ) and myelination (e.g., Bmp1, Cd69, and Fabp5) (Lloyd et al., 2019). In contrast to proinflammatory phenotypes, microglia transition to express anti-inflammatory cytokines such as IL-4, IL-10, and TGFβ, to suppress inflammation and elicit neuroprotective effects (Park et al., 2007; Colton, 2009; Fenn et al., 2014; Lively et al., 2016; Zöller et al., 2018). Following 10 days of excitotoxic lesion, a high number of previously IB-4+ PCNA+ microglial cells in the ipsilateral substantia nigra pars reticulata appeared to be apoptotic as indicated by TUNEL staining (Dihné et al., 2001). Apoptotic microglia were also observed around 12 months post-trauma in human head injury patients (Wilson et al., 2004). Corresponding to clinical recovery after facial nerve axotomy, some microglia near the lesion in the facial nucleus underwent apoptosis, or were removed by cell migration during the re-establishment of steady state microglial tiling and morphology (Tay et al., 2017). Concomitant with the onset of recovery, bulk RNAseq and scRNAseq analyses revealed the differential regulation of genes implicated in lipid mediation and cell migration, whereas genes belonging to the homeostatic microglial signature such as Cst3 and Sparc were downregulated (Tay et al., 2017, 2018). Taken together, we can infer the following: microglial clones resulting from local microgliosis at an acute lesion site respond to changes in the brain milieu by eliciting pro- and anti-inflammatory molecules required for tissue repair. While the specific mechanisms driving the migration and cell death of excess microglia resulting from clonal expansion are unclear, the phenomena suggest the tendency for microglia to reorganize and restore their homeostasis alongside clinical recovery.
In chronic neurodegeneration, persistent microglial clones can be seen accumulating at lesions and plaques (Figure 1), unlike CNS pathologies discussed above where clinical recovery accompanies the resolution of excess microglial cells. Microgliosis is consistently observed in chronic CNS pathologies. This could also be characterized by local microglial recruitment to the lesion followed by clonal proliferation in disease affected brain areas (Davalos et al., 2005; Füger et al., 2017). In both murine experimental autoimmune encephalomyelitis (EAE) mouse model and human MS pathology, the increase in microglial cell numbers was a result of local microgliosis around demyelinating lesions and dying neurons (Ajami et al., 2011; Zrzavy et al., 2017; Jordão et al., 2019). There is evidence for Ki67+ IBA1+ microglial clonal expansion in the temporal cortex of human AD brains (Gómez-Nicola et al., 2013; Olmos-Alonso et al., 2016). A progressive increase in IBA1+ BrdU+ microglia was found near amyloid-β (Aβ) plaques, suggesting microglial clonal expansion in APP/PS1 mice (Olmos-Alonso et al., 2016).
Further studies have demonstrated that microglia located near lesions exhibit a dysfunctional phenotype in chronic pathologies. K-means clustering of the transcriptomic data obtained from FCRLS+ microglia in wildtype aging and disease mouse models including SOD1G93A, APP-PS1, and EAE, showed two major gene clusters (Krasemann et al., 2017). One cluster displayed the loss of 68 homeostatic microglial genes that included Csf1r, Cx3Cr1, Gpr34, Hexb, Mertk, Olfml3, P2ry12, Tgfbr1, Tgfb1, and Tmem119, together with a loss of transcription factors such as Mef2a, Mafb, Jun, Sall1, and Egr1. The other cluster showed the upregulation of 28 inflammatory molecules, including Spp1, Itgax, Axl, Lilrb4, Clec7a, Ccl2, Csf1, and Apoe, among which Apoe was most upregulated (Krasemann et al., 2017). CLEC7A+ P2RY12− microglia that specifically associated with neuritic Aβ plaques were named microglial neurodegenerative phenotype (“MGnD”) by the authors, in contrast to “M0-homeostatic” adult microglia. The authors proposed that the transition from “M0-homeostatic” to “MGnD” was closely linked to neuritic dystrophy, and that the switch was regulated by TREM2-APOE signaling (Krasemann et al., 2017). Single-cell RNAseq studies showed a disease-associated microglial (“DAM”) subset localized near AD plaques that downregulated P2RY12/P2RY13, CX3CR1, and TMEM119 (Keren-Shaul et al., 2017). In cases of AD, dementia with Lewy bodies, as well as limbic-predominant age-related TDP-43 encephalopathy, neuropathological change associated strongly with dystrophic microglia with altered iron homeostasis, rather than with hypertrophic microglia (Shahidehpour et al., 2021). Chronic microglial activation caused oxidative bursts that led to the progression of MS (Lassmann et al., 2012). Reactive microglial populations that upregulate Il-1α, TNF or C1q were shown to promote the neurotoxic phenotype of astrocytes in vitro (Liddelow et al., 2017). This observation was recapitulated in disease affected areas of postmortem AD, PD, MS, and amyotrophic lateral sclerosis (ALS) human tissues, in which C3+ GFAP+ S100β+ reactive astrocytes were abundant (Liddelow et al., 2017). Furthermore, scRNAseq revealed the upregulation of TNF in late-response microglial populations found in the hippocampus of CK-p25 mice in late stages of neurodegeneration (Mathys et al., 2017). The authors proposed that these microglia could be programmed to transition into a neurotoxic population during later stages of pathology, as they were found to upregulate this cytokine already at an early stage of neurodegeneration (Mathys et al., 2017). Another deleterious impact of microglial populations in AD was demonstrated in a mouse model, where Aβ-carrying microglial cells were claimed to induce Aβ plaques in healthy tissue (d'Errico et al., 2021). In vivo imaging of Irf8+/+ Cx3cr1+/− 5xFAD mice showed that motile microglia transported Aβ in response to laser-induced focal tissue injury, leading to the build-up of Aβ plaques at lesion sites. Migration of microglia containing Aβ from old Cx3cr1+/− 5xFAD brain slice to healthy young wildtype brain tissue further argues for the active involvement of microglia in disease propagation (d'Errico et al., 2021). Altogether, these studies point toward detrimental effects of microglial subpopulations in promoting and propagating chronic neurodegenerative diseases. On the contrary, several scRNAseq studies revealed that among lesion-associated microglia, a subset of microglial cells expressed genes that are associated with the homeostatic phenotype (Keren-Shaul et al., 2017; Mathys et al., 2017; Tay et al., 2018). Interestingly, not all microglia that surround lesions contribute to the aggravation of pathology. It is currently unclear if microglia expressing “MGnD”, “DAM”, and similar non-homeostatic microglial phenotypes could be clonally related, or if clonally expanded microglia display transcriptomic heterogeneity in response to their microenvironment. Instead of naming microglia as the major contributors to chronic neuroinflammation, several studies have shown that microglia surrounding the Aβ plaques form a compact physical barrier around them to limit further local neuronal damage (Condello et al., 2015; Yuan et al., 2016; Spangenberg et al., 2019). Moreover, microglial TREM2-mediated clearance of protein aggregates of human TAR-DNA binding protein 43 kDa (TDP-43) was neuroprotective in mouse models for the motor neuron degenerative disease ALS (Spiller et al., 2018; Xie et al., 2021). Taken together, microglial subpopulations near lesions or plaques can potentially have both beneficial and detrimental effects in chronic neurodegeneration. We speculate that it is the persistence of unresolved reactive and proinflammatory microglial clones that aggravates disease and prevents recovery.
Considering microglial clonality in CNS development and homeostasis, we can conclude that the presence of adult microglia is the result of clonally expanding microglial progenitors that colonize the brain parenchyma during development. Based on the rapid repopulation and re-establishment of homeostatic microglia by clonal expansion of residual cells after ablation, it appears that local sources of CSF1R signaling are sufficient to drive the restoration of homeostatic microglial tiling. In acute CNS diseases, clinical recovery coincides with the resolution of microglial clones that had resulted from microgliosis near the sites of damage. On the other hand, in severe chronic neurodegenerative diseases, prevalent microgliosis with no signs of resolution of excess proinflammatory microglia are observed alongside altered CSF1R, TGFβ, and purinergic signaling that influence microglial cell densities and their homeostatic gene signature (Gómez-Nicola et al., 2013; Von Bernhardi et al., 2015; Olmos-Alonso et al., 2016; Zöller et al., 2018; Pietrowski et al., 2021). While current literature does not describe any ongoing resolution of microglial clones near chronic lesions in diseased brains, this does not exclude the low-level existence of resolving microglial clones around sites of neurodegeneration. To reveal such resolution events, we propose approaches similar to partial targeting of microglial cells by Confetti labeling to spatially and temporally address the clonality and resolution of microglia in diseases (Tay et al., 2017). We hypothesize that unresolved microglial subpopulations, in particular those carrying a proinflammatory phenotype and surrounding the lesions over prolonged periods promote neuroinflammation, exacerbate disease conditions, and impede recovery. An alternative explanation is that these microglial subpopulations become dysfunctional, fail to overcome the complex microenvironment of the disease, and do not transition from an adverse proinflammatory state to a pro-regenerative phenotype. To investigate if partial amelioration of disease could be achieved by disrupting microglial clonal expansion in different disease contexts, streamlined strategies to selectively or transiently target, for example, CSF1R, TGFβ or purinergic signaling could be tested (Gómez-Nicola et al., 2013; Olmos-Alonso et al., 2016; Easley-Neal et al., 2019; Obst et al., 2020; Pons et al., 2020; Pietrowski et al., 2021). Finally, while microglia are clonally-related due to their YS, AGM, and fetal liver hematopoietic origins, we acknowledge their fascinating heterogeneity in CNS development, aging and diseases that would make for another interesting review (Grabert et al., 2016; Matcovitch-Natan et al., 2016; Keren-Shaul et al., 2017; Mathys et al., 2017; Tay et al., 2018; Hammond et al., 2019).
AVM wrote the manuscript and designed the figure. TLT supervised the project and extensively revised the manuscript. All authors contributed to the article and approved the submitted version.
TLT was supported by the Klaus Tschira Boost Fund (KT-10), FRIAS Junior Fellowship, University of Freiburg Research Innovation Fund, Wissenschaftliche Gesellschaft Freiburg (Helmut Holzer Prize), German Research Foundation (EXC 1086), and Ministry of Economics, Science and Arts of Baden-Württemberg (Sustainability Program for Projects of the Excellence Initiative II).
The authors declare that the research was conducted in the absence of any commercial or financial relationships that could be construed as a potential conflict of interest.
All claims expressed in this article are solely those of the authors and do not necessarily represent those of their affiliated organizations, or those of the publisher, the editors and the reviewers. Any product that may be evaluated in this article, or claim that may be made by its manufacturer, is not guaranteed or endorsed by the publisher.
The authors thank M. Petrich, R. Phadke, O. Bouadi, and Drs. H. Vecchiarelli, K. Bisht, and B. Appiah for their valuable feedback. In memory of Purushottam M. Bhat (1967–2019) and Marc Spelleken (1964–2021).
Ajami, B., Bennett, J. L., Krieger, C., McNagny, K. M., and Rossi, F. M. V. (2011). Infiltrating monocytes trigger EAE progression, but do not contribute to the resident microglia pool. Nat. Neurosci. 14, 1142–1149. doi: 10.1038/nn.2887
Ajami, B., Bennett, J. L., Krieger, C., Tetzlaff, W., and Rossi, F. M. V. (2007). Local self-renewal can sustain CNS microglia maintenance and function throughout adult life. Nat. Neurosci. 10, 1538–1543. doi: 10.1038/nn2014
Alliot, F., Godin, I., and Pessac, B. (1999). Microglia derive from progenitors, originating from the yolk sac, and which proliferate in the brain. Brain Res. Dev. Brain Res. 117, 145–152. doi: 10.1016/S0165-3806(99)00113-3
Askew, K., Li, K., Olmos-Alonso, A., Garcia-Moreno, F., Liang, Y., Richardson, P., et al. (2017). Coupled proliferation and apoptosis maintain the rapid turnover of microglia in the adult brain. Cell Rep. 18, 391–405. doi: 10.1016/j.celrep.2016.12.041
Badimon, A., Strasburger, H. J., Ayata, P., Chen, X., Nair, A., Ikegami, A., et al. (2020). Negative feedback control of neuronal activity by microglia. Nature. 586, 417–423. doi: 10.1038/s41586-020-2777-8
Barres, B. A., Hart, I. K., Coles, H. S., Burne, J. F., Voyvodic, J. T., Richardson, W. D., et al. (1992). Cell death and control of cell survival in the oligodendrocyte lineage. Cell. 70, 31–46. doi: 10.1016/0092-8674(92)90531-G
Basu, A., Krady, J. K., O'Malley, M., Styren, S. D., DeKosky, S. T., and Levison, S. W. (2002). The type 1 interleukin-1 receptor is essential for the efficient activation of microglia and the induction of multiple proinflammatory mediators in response to brain injury. J. Neurosci. 22, 6071. doi: 10.1523/JNEUROSCI.22-14-06071.2002
Beattie, E. C., Stellwagen, D., Morishita, W., Bresnahan, J. C., Ha, B. K., Von Zastrow, M., et al. (2002). Control of Synaptic Strength by Glial TNFα. Science 295, 2282–2285. doi: 10.1126/science.1067859
Bennett, M. L., and Bennett, F. C. (2020). The influence of environment and origin on brain resident macrophages and implications for therapy. Nat. Neurosci. 23, 157–166. doi: 10.1038/s41593-019-0545-6
Bruttger, J., Karram, K., Wörtge, S., Regen, T., Marini, F., Hoppmann, N., et al. (2015). Genetic cell ablation reveals clusters of local self-renewing microglia in the mammalian central nervous system. Immunity 43, 92–106. doi: 10.1016/j.immuni.2015.06.012
Cantoni, C., Bollman, B., Licastro, D., Xie, M., Mikesell, R., Schmidt, R., et al. (2015). TREM2 regulates microglial cell activation in response to demyelination in vivo. Acta Neuropathol. 129, 429–447. doi: 10.1007/s00401-015-1388-1
Chen, S.-K., Tvrdik, P., Peden, E., Cho, S., Wu, S., Spangrude, G., et al. (2010). Hematopoietic origin of pathological grooming in Hoxb8 mutant mice. Cell 141, 775–785. doi: 10.1016/j.cell.2010.03.055
Colton, C. A. (2009). Heterogeneity of microglial activation in the innate immune response in the brain. J. Neuroimmune Pharmacol. 4, 399–418. doi: 10.1007/s11481-009-9164-4
Condello, C., Yuan, P., Schain, A., and Grutzendler, J. (2015). Microglia constitute a barrier that prevents neurotoxic protofibrillar Aβ42 hotspots around plaques. Nat. Commun. 6, 6176. doi: 10.1038/ncomms7176
Cronk, J. C., Filiano, A. J., Louveau, A., Marin, I., Marsh, R., Ji, E., et al. (2018). Peripherally derived macrophages can engraft the brain independent of irradiation and maintain an identity distinct from microglia. J. Exp. Med. 215, 1627–1647. doi: 10.1084/jem.20180247
Davalos, D., Grutzendler, J., Yang, G., Kim, J. V., Zuo, Y., Jung, S., et al. (2005). ATP mediates rapid microglial response to local brain injury in vivo. Nat. Neurosci. 8, 752–758. doi: 10.1038/nn1472
De, S., Van Deren, D., Peden, E., Hockin, M., Boulet, A., Titen, S., et al. (2018). Two distinct ontogenies confer heterogeneity to mouse brain microglia. Development. 145. doi: 10.1242/dev.152306
d'Errico, P., Ziegler-Waldkirch, S., Aires, V., Hoffmann, P., Mezö, C., Erny, D., et al. (2021). Microglia contribute to the propagation of Aβ into unaffected brain tissue. Nat. Neurosci. doi: 10.1038/s41593-021-00951-0
Diaz-Aparicio, I., Paris, I., Sierra-Torre, V., Plaza-Zabala, A., Rodríguez-Iglesias, N., Márquez-Ropero, M., et al. (2020). Microglia actively remodel adult hippocampal neurogenesis through the phagocytosis secretome. J. Neurosci. 40, 1453. doi: 10.1523/JNEUROSCI.0993-19.2019
Dihné, M., Block, F., Korr, H., and Töpper, R. (2001). Time course of glial proliferation and glial apoptosis following excitotoxic CNS injury. Brain Res. 902, 178–189. doi: 10.1016/S0006-8993(01)02378-2
Easley-Neal, C., Foreman, O., Sharma, N., Zarrin, A. A., and Weimer, R. M. (2019). CSF1R Ligands IL-34 and CSF1 are differentially required for microglia development and maintenance in white and gray matter brain regions. Front. Immunol. 10, 2199. doi: 10.3389/fimmu.2019.02199
Echeverry, S., Shi, X. Q., and Zhang, J. (2008). Characterization of cell proliferation in rat spinal cord following peripheral nerve injury and the relationship with neuropathic pain. J. Pain 135, 37–47. doi: 10.1016/j.pain.2007.05.002
Elmore, M. R. P., Najafi, A. R., Koike, M. A., Dagher, N. N., Spangenberg, E. E., Rice, R. A., et al. (2014). Colony-stimulating factor 1 receptor signaling is necessary for microglia viability, unmasking a microglia progenitor cell in the adult brain. Neuron 82, 380–397. doi: 10.1016/j.neuron.2014.02.040
Eriksson, N. P., Persson, J. K. E., Svensson, M., Arvidsson, J., Molander, C., and Aldskogius, H. (1993). A quantitative analysis of the microglial cell reaction in central primary sensory projection territories following peripheral nerve injury in the adult rat. Exp. Brain Res. 96, 19–27. doi: 10.1007/BF00230435
Eyo, U. B., Peng, J., Swiatkowski, P., Mukherjee, A., Bispo, A., and Wu, L.-J. (2014). Neuronal hyperactivity recruits microglial processes via neuronal NMDA receptors and microglial P2Y12 receptors after status epilepticus. J. Neurosci. 34, 10528. doi: 10.1523/JNEUROSCI.0416-14.2014
Fehrenbach, M. K., Tjwa, M., Bechmann, I., and Krueger, M. (2018). Decreased microglial numbers in Vav1-Cre+:dicer knock-out mice suggest a second source of microglia beyond yolk sac macrophages. Ann. Anat. 218, 190–198. doi: 10.1016/j.aanat.2018.03.004
Fenn, A. M., Hall, J. C. E., Gensel, J. C., Popovich, P. G., and Godbout, J. P. (2014). IL-4 signaling drives a unique arginase+/IL-1β+ microglia phenotype and recruits macrophages to the inflammatory CNS: consequences of age-related deficits in IL-4Rα after traumatic spinal cord injury. J. Neurosci. 34, 8904–8917. doi: 10.1523/JNEUROSCI.1146-14.2014
Füger, P., Hefendehl, J. K., Veeraraghavalu, K., Wendeln, A.-C., Schlosser, C., Obermüller, U., et al. (2017). Microglia turnover with aging and in an Alzheimer's model via long-term in vivo single-cell imaging. Nat. Neurosci. 20, 1371–1376. doi: 10.1038/nn.4631
Gilmore, S. A. (1975). Proliferation of non-neuronal cells in spinal cords of irradiated, immature rats following transection of the sciatic nerve. Anat. Rec. 181, 799–811. doi: 10.1002/ar.1091810411
Ginhoux, F., Greter, M., Leboeuf, M., Nandi, S., See, P., Gokhan, S., et al. (2010). Fate mapping analysis reveals that adult microglia derive from primitive macrophages. Science. 330, 841–845. doi: 10.1126/science.1194637
Glass, C. K., Saijo, K., Winner, B., Marchetto, M. C., and Gage, F. H. (2010). Mechanisms underlying inflammation in neurodegeneration. Cell. 140, 918–934. doi: 10.1016/j.cell.2010.02.016
Goldmann, T., Wieghofer, P., Jordão, M. J. C., Prutek, F., Hagemeyer, N., Frenzel, K., et al. (2016). Origin, fate and dynamics of macrophages at central nervous system interfaces. Nat. Immunol. 17, 797–805. doi: 10.1038/ni.3423
Gomez Perdiguero, E., Klapproth, K., Schulz, C., Busch, K., Azzoni, E., Crozet, L., et al. (2015). Tissue-resident macrophages originate from yolk-sac-derived erythro-myeloid progenitors. Nature. 518, 547–551. doi: 10.1038/nature13989
Gómez-Nicola, D., Fransen, N. L., Suzzi, S., and Perry, V. H. (2013). Regulation of microglial proliferation during chronic neurodegeneration. J. Neurosci. 33, 2481–2493. doi: 10.1523/JNEUROSCI.4440-12.2013
Grabert, K., Michoel, T., Karavolos, M. H., Clohisey, S., Baillie, J. K., Stevens, M. P., et al. (2016). Microglial brain region–dependent diversity and selective regional sensitivities to aging. Nat. Neurosci. 19, 504–516. doi: 10.1038/nn.4222
Gu, N., Peng, J., Murugan, M., Wang, X., Eyo, U. B., Sun, D., et al. (2016). Spinal Microgliosis Due to Resident Microglial Proliferation Is Required for Pain Hypersensitivity after Peripheral Nerve Injury. Cell Rep. 16, 605–614. doi: 10.1016/j.celrep.2016.06.018
Guan, Z., Kuhn, J. A., Wang, X., Colquitt, B., Solorzano, C., Vaman, S., et al. (2016). Injured sensory neuron-derived CSF1 induces microglial proliferation and DAP12-dependent pain. Nat. Neurosci. 19, 94–101. doi: 10.1038/nn.4189
Gushchina, S., Pryce, G., Yip, P. K., Wu, D., Pallier, P., Giovannoni, G., et al. (2018). Increased expression of colony-stimulating factor-1 in mouse spinal cord with experimental autoimmune encephalomyelitis correlates with microglial activation and neuronal loss. Glia 66, 2108–2125. doi: 10.1002/glia.23464
Hagemeyer, N., Hanft, K.-M., Akriditou, M.-A., Unger, N., Park, E. S., Stanley, E. R., et al. (2017). Microglia contribute to normal myelinogenesis and to oligodendrocyte progenitor maintenance during adulthood. Acta Neuropathol. 134, 441–458. doi: 10.1007/s00401-017-1747-1
Hammond, T. R., Dufort, C., Dissing-Olesen, L., Giera, S., Young, A., Wysoker, A., et al. (2019). Single-cell RNA sequencing of microglia throughout the mouse lifespan and in the injured brain reveals complex cell-state changes. Immunity. 50, 253–271.e6. doi: 10.1016/j.immuni.2018.11.004
Han, J., Harris, R. A., and Zhang, X.-M. (2017). An updated assessment of microglia depletion: current concepts and future directions. Mol. Brain. 10, 25. doi: 10.1186/s13041-017-0307-x
Hanisch, U.-K., and Kettenmann, H. (2007). Microglia: active sensor and versatile effector cells in the normal and pathologic brain. Nat. Neurosci. 10, 1387–1394. doi: 10.1038/nn1997
Hashimoto, D., Chow, A., Noizat, C., Teo, P., Beasley, M. B., Leboeuf, M., et al. (2013). Tissue-resident macrophages self-maintain locally throughout adult life with minimal contribution from circulating monocytes. Immunity 38, 792–804. doi: 10.1016/j.immuni.2013.04.004
Huang, Y., Xu, Z., Xiong, S., Sun, F., Qin, G., Hu, G., et al. (2018). Repopulated microglia are solely derived from the proliferation of residual microglia after acute depletion. Nat. Neurosci. 21, 530–540. doi: 10.1038/s41593-018-0090-8
Jay, T. R., Hirsch, A. M., Broihier, M. L., Miller, C. M., Neilson, L. E., Ransohoff, R. M., et al. (2017). Disease progression-dependent effects of TREM2 deficiency in a mouse model of Alzheimer's disease. J. Neurosci. 37, 637. doi: 10.1523/JNEUROSCI.2110-16.2016
Jenkins, S. J., Ruckerl, D., Cook, P. C., Jones, L. H., Finkelman, F. D., Rooijen, N. van, et al. (2011). Local macrophage proliferation, rather than recruitment from the blood, is a signature of TH2 inflammation. Science. doi: 10.1126/science.1204351
Jordão, M. J. C., Sankowski, R., Brendecke, S. M., Sagar, Locatelli, G., Tai, Y.-H., et al. (2019). Single-cell profiling identifies myeloid cell subsets with distinct fates during neuroinflammation. Science. 363. doi: 10.1126/science.aat7554
Keren-Shaul, H., Spinrad, A., Weiner, A., Matcovitch-Natan, O., Dvir-Szternfeld, R., Ulland, T. K., et al. (2017). A unique microglia type associated with restricting development of Alzheimer's disease. Cell 169, 1276-1290.e17. doi: 10.1016/j.cell.2017.05.018
Krasemann, S., Madore, C., Cialic, R., Baufeld, C., Calcagno, N., El Fatimy, R., et al. (2017). The TREM2-APOE pathway drives the transcriptional phenotype of dysfunctional microglia in neurodegenerative diseases. Immunity 47, 566-581.e9. doi: 10.1016/j.immuni.2017.08.008
Kreutzberg, G. W. (1996). Microglia: a sensor for pathological events in the CNS. Trends Neurosci. 19, 312–318. doi: 10.1016/0166-2236(96)10049-7
Ladeby, R., Wirenfeldt, M., Garcia-Ovejero, D., Fenger, C., Dissing-Olesen, L., Dalmau, I., et al. (2005). Microglial cell population dynamics in the injured adult central nervous system. Brain Res. Rev. 48, 196–206. doi: 10.1016/j.brainresrev.2004.12.009
Lassmann, H., van Horssen, J., and Mahad, D. (2012). Progressive multiple sclerosis: pathology and pathogenesis. Nat. Rev. Neurol. 8, 647–656. doi: 10.1038/nrneurol.2012.168
Li, Q., Cheng, Z., Zhou, L., Darmanis, S., Neff, N. F., Okamoto, J., et al. (2019). Developmental heterogeneity of microglia and brain myeloid cells revealed by deep single-Cell RNA sequencing. Neuron 101, 207-223.e10. doi: 10.1016/j.neuron.2018.12.006
Li, Y., Liu, L., Barger, S. W., and Griffin, W. S. T. (2003). Interleukin-1 mediates pathological effects of microglia on tau phosphorylation and on synaptophysin synthesis in cortical neurons through a p38-MAPK pathway. J. Neurosci. 23, 1605. doi: 10.1523/JNEUROSCI.23-05-01605.2003
Liddelow, S. A., Guttenplan, K. A., Clarke, L. E., Bennett, F. C., Bohlen, C. J., Schirmer, L., et al. (2017). Neurotoxic reactive astrocytes are induced by activated microglia. Nature 541, 481–487. doi: 10.1038/nature21029
Liu, Y. U., Ying, Y., Li, Y., Eyo, U. B., Chen, T., Zheng, J., et al. (2019). Neuronal network activity controls microglial process surveillance in awake mice via norepinephrine signaling. Nat. Neurosci. 22, 1771–1781. doi: 10.1038/s41593-019-0511-3
Lively, S., Hutchings, S., and Schlichter, L. C. (2016). Molecular and cellular responses to interleukin-4 treatment in a rat model of transient ischemia. J. Neuropathol. Exp. Neurol. 75, 1058–1071. doi: 10.1093/jnen/nlw081
Lloyd, A. F., Davies, C. L., Holloway, R. K., Labrak, Y., Ireland, G., Carradori, D., et al. (2019). Central nervous system regeneration is driven by microglia necroptosis and repopulation. Nat. Neurosci. 22, 1046–1052. doi: 10.1038/s41593-019-0418-z
Marin-Teva, J. L., Dusart, I., Colin, C., Gervais, A., van Rooijen, N., and Mallat, M. (2004). Microglia promote the death of developing purkinje cells. Neuron 41, 535–547. doi: 10.1016/S0896-6273(04)00069-8
Matcovitch-Natan, O., Winter, D. R., Giladi, A., Vargas Aguilar, S., Spinrad, A., Sarrazin, S., et al. (2016). Microglia development follows a stepwise program to regulate brain homeostasis. Science. 353, aad8670. doi: 10.1126/science.aad8670
Mathys, H., Adaikkan, C., Gao, F., Young, J. Z., Manet, E., Hemberg, M., et al. (2017). Temporal tracking of microglia activation in neurodegeneration at single-cell resolution. Cell Rep. 21, 366–380. doi: 10.1016/j.celrep.2017.09.039
Miron, V. E., Boyd, A., Zhao, J.-W., Yuen, T. J., Ruckh, J. M., Shadrach, J. L., et al. (2013). M2 microglia and macrophages drive oligodendrocyte differentiation during CNS remyelination. Nat. Neurosci. 16, 1211–1218. doi: 10.1038/nn.3469
Miyamoto, A., Wake, H., Ishikawa, A. W., Eto, K., Shibata, K., Murakoshi, H., et al. (2016). Microglia contact induces synapse formation in developing somatosensory cortex. Nat. Commun. 7, 12540. doi: 10.1038/ncomms12540
Nikodemova, M., Kimyon, R. S., De, I., Small, A. L., Collier, L. S., and Watters, J. J. (2015). Microglial numbers attain adult levels after undergoing a rapid decrease in cell number in the third postnatal week. J. Neuroimmunol. 278, 280–288. doi: 10.1016/j.jneuroim.2014.11.018
Nimmerjahn, A., Kirchhoff, F., and Helmchen, F. (2005). Resting microglial cells are highly dynamic surveillants of brain parenchyma in vivo. Science. 308, 1314–1318. doi: 10.1126/science.1110647
Obst, J., Simon, E., Martin-Estebane, M., Pipi, E., Barkwill, L. M., Gonzalez-Rivera, I., et al. (2020). Inhibition of IL-34 unveils tissue-selectivity and is sufficient to reduce microglial proliferation in a model of chronic neurodegeneration. Front. Immunol. 11, 2360. doi: 10.3389/fimmu.2020.579000
Olmos-Alonso, A., Schetters, S. T. T., Sri, S., Askew, K., Mancuso, R., Vargas-Caballero, M., et al. (2016). Pharmacological targeting of CSF1R inhibits microglial proliferation and prevents the progression of Alzheimer's-like pathology. Brain J. Neurol. 139, 891–907. doi: 10.1093/brain/awv379
Oosterhof, N., Chang, I. J., Karimiani, E. G., Kuil, L. E., Jensen, D. M., Daza, R., et al. (2019). Homozygous mutations in CSF1R cause a pediatric-onset leukoencephalopathy and can result in congenital absence of microglia. Am. J. Hum. Genet. 104, 936–947. doi: 10.1016/j.ajhg.2019.03.010
Paolicelli, R. C., Bolasco, G., Pagani, F., Maggi, L., Scianni, M., Panzanelli, P., et al. (2011). Synaptic pruning by microglia is necessary for normal brain development. Science 333, 1456–1458. doi: 10.1126/science.1202529
Park, K. W., Lee, H. G., Jin, B. K., and Lee, Y. B. (2007). Interleukin-10 endogenously expressed in microglia prevents lipopolysaccharide-induced neurodegeneration in the rat cerebral cortex in vivo. Exp. Mol. Med. 39, 812–819. doi: 10.1038/emm.2007.88
Parkhurst, C. N., Yang, G., Ninan, I., Savas, J. N., Yates, J. R. 3rd, Lafaille, J. J., et al. (2013). Microglia promote learning-dependent synapse formation through brain-derived neurotrophic factor. Cell 155, 1596–1609. doi: 10.1016/j.cell.2013.11.030
Peng, J., Gu, N., Zhou, L., B Eyo, U., Murugan, M., Gan, W.-B., et al. (2016). Microglia and monocytes synergistically promote the transition from acute to chronic pain after nerve injury. Nat. Commun. 7, 12029. doi: 10.1038/ncomms12029
Pietrowski, M. J., Gabr, A. A., Kozlov, S., Blum, D., Halle, A., and Carvalho, K. (2021). Glial purinergic signaling in neurodegeneration. Front. Neurol. 12, 743. doi: 10.3389/fneur.2021.654850
Plemel, J. R., Stratton, J. A., Michaels, N. J., Rawji, K. S., Zhang, E., Sinha, S., et al. (2020). Microglia response following acute demyelination is heterogeneous and limits infiltrating macrophage dispersion. Sci. Adv. 6, eaay6324. doi: 10.1126/sciadv.aay6324
Pons, V., Laflamme, N., Préfontaine, P., and Rivest, S. (2020). Role of macrophage colony-stimulating factor receptor on the proliferation and survival of microglia following systemic nerve and cuprizone-induced injuries. Front. Immunol. 11, 47. doi: 10.3389/fimmu.2020.00047
Ransohoff, R. M. (2007). Microgliosis: the questions shape the answers. Nat. Neurosci. 10, 1507–1509. doi: 10.1038/nn1207-1507
Réu, P., Khosravi, A., Bernard, S., Mold, J. E., Salehpour, M., Alkass, K., et al. (2017). The lifespan and turnover of microglia in the human brain. Cell Rep. 20, 779–784. doi: 10.1016/j.celrep.2017.07.004
Sato, A., Ohtaki, H., Tsumuraya, T., Song, D., Ohara, K., Asano, M., et al. (2012). Interleukin-1 participates in the classical and alternative activation of microglia/macrophages after spinal cord injury. J. Neuroinflammation 9, 65. doi: 10.1186/1742-2094-9-65
Schafer, D. P., Lehrman, E. K., Kautzman, A. G., Koyama, R., Mardinly, A. R., Yamasaki, R., et al. (2012). Microglia sculpt postnatal neural circuits in an activity and complement-dependent manner. Neuron 74, 691–705. doi: 10.1016/j.neuron.2012.03.026
Schafer, D. P., and Stevens, B. (2015). Microglia function in central nervous system development and plasticity. Cold Spring Harb. Perspect. Biol. 7, a020545–a020545. doi: 10.1101/cshperspect.a020545
Shahidehpour, R. K., Higdon, R. E., Crawford, N. G., Neltner, J. H., Ighodaro, E. T., Patel, E., et al. (2021). Dystrophic microglia are associated with neurodegenerative disease and not healthy aging in the human brain. Neurobiol. Aging 99, 19–27. doi: 10.1016/j.neurobiolaging.2020.12.003
Shemer, A., Grozovski, J., Tay, T. L., Tao, J., Volaski, A., Süß, P., et al. (2018). Engrafted parenchymal brain macrophages differ from microglia in transcriptome, chromatin landscape and response to challenge. Nat. Commun. 9, 5206. doi: 10.1038/s41467-018-07548-5
Sierra, A., Encinas, J. M., Deudero, J. J. P., Chancey, J. H., Enikolopov, G., Overstreet-Wadiche, L. S., et al. (2010). Microglia shape adult hippocampal neurogenesis through apoptosis-coupled phagocytosis. Cell Stem Cell 7, 483–495. doi: 10.1016/j.stem.2010.08.014
Spangenberg, E., Severson, P. L., Hohsfield, L. A., Crapser, J., Zhang, J., Burton, E. A., et al. (2019). Sustained microglial depletion with CSF1R inhibitor impairs parenchymal plaque development in an Alzheimer's disease model. Nat. Commun. 10, 3758. doi: 10.1038/s41467-019-11674-z
Spiller, K. J., Restrepo, C. R., Khan, T., Dominique, M. A., Fang, T. C., Canter, R. G., et al. (2018). Microglia-mediated recovery from ALS-relevant motor neuron degeneration in a mouse model of TDP-43 proteinopathy. Nat. Neurosci. 21, 329–340. doi: 10.1038/s41593-018-0083-7
Squarzoni, P., Oller, G., Hoeffel, G., Pont-Lezica, L., Rostaing, P., Low, D., et al. (2014). Microglia modulate wiring of the embryonic forebrain. Cell Rep. 8, 1271–1279. doi: 10.1016/j.celrep.2014.07.042
Stellwagen, D., and Malenka, R. C. (2006). Synaptic scaling mediated by glial TNF-α. Nature 440, 1054–1059. doi: 10.1038/nature04671
Stowell, R. D., Sipe, G. O., Dawes, R. P., Batchelor, H. N., Lordy, K. A., Whitelaw, B. S., et al. (2019). Noradrenergic signaling in the wakeful state inhibits microglial surveillance and synaptic plasticity in the mouse visual cortex. Nat. Neurosci. 22, 1782–1792. doi: 10.1038/s41593-019-0514-0
Streit, W. J., Braak, H., Xue, Q.-S., and Bechmann, I. (2009). Dystrophic (senescent) rather than activated microglial cells are associated with tau pathology and likely precede neurodegeneration in Alzheimer's disease. Acta Neuropathol. 118, 475–485. doi: 10.1007/s00401-009-0556-6
Streit, W. J., Khoshbouei, H., and Bechmann, I. (2020). Dystrophic microglia in late-onset Alzheimer's disease. Glia 68, 845–854. doi: 10.1002/glia.23782
Streit, W. J., Walter, S. A., and Pennell, N. A. (1999). Reactive microgliosis. Prog. Neurobiol. 57, 563–581. doi: 10.1016/S0301-0082(98)00069-0
Swinnen, N., Smolders, S., Avila, A., Notelaers, K., Paesen, R., Ameloot, M., et al. (2013). Complex invasion pattern of the cerebral cortex by microglial cells during development of the mouse embryo. Glia 61, 150–163. doi: 10.1002/glia.22421
Tay, T. L., Mai, D., Dautzenberg, J., Fernández-Klett, F., Lin, G., Sagar, et al. (2017). A new fate mapping system reveals context-dependent random or clonal expansion of microglia. Nat. Neurosci. 20, 793–803. doi: 10.1038/nn.4547
Tay, T. L., Sagar Dautzenberg, J., Grün, D., and Prinz, M. (2018). Unique microglia recovery population revealed by single-cell RNAseq following neurodegeneration. Acta Neuropathol. Commun. 6, 87–87. doi: 10.1186/s40478-018-0584-3
Trapp, B. D., Nishiyama, A., Cheng, D., and Macklin, W. (1997). Differentiation and death of premyelinating oligodendrocytes in developing rodent brain. J. Cell Biol. 137, 459–468. doi: 10.1083/jcb.137.2.459
Tremblay, M.-È., Lowery, R. L., and Majewska, A. K. (2010). Microglial interactions with synapses are modulated by visual experience. PLOS Biol. 8, e1000527. doi: 10.1371/journal.pbio.1000527
Ueno, M., Fujita, Y., Tanaka, T., Nakamura, Y., Kikuta, J., Ishii, M., et al. (2013). Layer V cortical neurons require microglial support for survival during postnatal development. Nat. Neurosci. 16, 543–551. doi: 10.1038/nn.3358
Varvel, N. H., Grathwohl, S. A., Baumann, F., Liebig, C., Bosch, A., Brawek, B., et al. (2012). Microglial repopulation model reveals a robust homeostatic process for replacing CNS myeloid cells. Proc. Natl. Acad. Sci. 109, 18150–18155. doi: 10.1073/pnas.1210150109
Von Bernhardi, R., Cornejo, F., Parada, G., and Eugenin, J. (2015). Role of TGFβ signaling in the pathogenesis of Alzheimer's disease. Front. Cell. Neurosci. 9, 426. doi: 10.3389/fncel.2015.00426
Wallace, J., Lord, J., Dissing-Olesen, L., Stevens, B., and Murthy, V. N. (2020). Microglial depletion disrupts normal functional development of adult-born neurons in the olfactory bulb. eLife. 9, e50531. doi: 10.7554/eLife.50531
Wang, Y., Ulland, T. K., Ulrich, J. D., Song, W., Tzaferis, J. A., Hole, J. T., et al. (2016). TREM2-mediated early microglial response limits diffusion and toxicity of amyloid plaques. J. Exp. Med. 213, 667–675. doi: 10.1084/jem.20151948
Wilson, S., Raghupathi, R., Saatman, K. E., MacKinnon, M.-A., McIntosh, T. K., and Graham, D. I. (2004). Continued in situ DNA fragmentation of microglia/macrophages in white matter weeks and months after traumatic brain injury. J. Neurotrauma. 21, 239–250. doi: 10.1089/089771504322972031
Wlodarczyk, A., Holtman, I. R., Krueger, M., Yogev, N., Bruttger, J., Khorooshi, R., et al. (2017). A novel microglial subset plays a key role in myelinogenesis in developing brain. EMBO J. 36, 3292–3308. doi: 10.15252/embj.201696056
Wohl, S. G., Schmeer, C. W., Witte, O. W., and Isenmann, S. (2010). Proliferative response of microglia and macrophages in the adult mouse eye after optic nerve lesion. Invest. Ophthalmol. Vis. Sci. 51, 2686–2696. doi: 10.1167/iovs.09-4537
Xie, M., Liu, Y. U., Zhao, S., Zhang, L., Bosco, D. B., Pang, Y.-P., et al. (2021). TREM2 interacts with TDP-43 and mediates microglial neuroprotection against TDP-43-related neurodegeneration. Nat. Neurosci. doi: 10.21203/rs.3.rs-133413/v1
Yang, L., Blumbergs, P. C., Jones, N. R., Manavis, J., Sarvestani, G. T., and Ghabriel, M. N. (2004). Early expression and cellular localization of proinflammatory cytokines interleukin-1β, interleukin-6, and tumor necrosis factor-α in human traumatic spinal cord injury. Spine 29. doi: 10.1097/00007632-200405010-00004
Yuan, P., Condello, C., Keene, C. D., Wang, Y., Bird, T. D., Paul, S. M., et al. (2016). TREM2 Haplodeficiency in Mice and Humans Impairs the Microglia Barrier Function Leading to Decreased Amyloid Compaction and Severe Axonal Dystrophy. Neuron. 90, 724–739. doi: 10.1016/j.neuron.2016.05.003
Yuan, T.-F., Liang, Y.-X., Peng, B., Lin, B., and So, K.-F. (2015). Local proliferation is the main source of rod microglia after optic nerve transection. Sci. Rep. 5, 10788. doi: 10.1038/srep10788
Zhan, L., Krabbe, G., Du, F., Jones, I., Reichert, M. C., Telpoukhovskaia, M., et al. (2019). Proximal recolonization by self-renewing microglia re-establishes microglial homeostasis in the adult mouse brain. PLoS Biol. 17, e3000134–e3000134. doi: 10.1371/journal.pbio.3000134
Zhan, Y., Paolicelli, R. C., Sforazzini, F., Weinhard, L., Bolasco, G., Pagani, F., et al. (2014). Deficient neuron-microglia signaling results in impaired functional brain connectivity and social behavior. Nat. Neurosci. 17, 400–406. doi: 10.1038/nn.3641
Zhao, Y., Wu, X., Li, X., Jiang, L.-L., Gui, X., Liu, Y., et al. (2018). TREM2 Is a Receptor for β-Amyloid that Mediates Microglial Function. Neuron. 97, 1023–1031.e7. doi: 10.1016/j.neuron.2018.01.031
Zhou, L.-J., Peng, J., Xu, Y.-N., Zeng, W.-J., Zhang, J., Wei, X., et al. (2019). Microglia are indispensable for synaptic plasticity in the spinal dorsal horn and chronic pain. Cell Rep. 27, 3844-3859.e6. doi: 10.1016/j.celrep.2019.05.087
Zöller, T., Schneider, A., Kleimeyer, C., Masuda, T., Potru, P. S., Pfeifer, D., et al. (2018). Silencing of TGFβ signalling in microglia results in impaired homeostasis. Nat. Commun. 9, 4011. doi: 10.1038/s41467-018-06224-y
Keywords: microglia, clonality, development, homeostasis, repopulation, neurodegeneration
Citation: Manjally AV and Tay TL (2022) Attack of the Clones: Microglia in Health and Disease. Front. Cell. Neurosci. 16:831747. doi: 10.3389/fncel.2022.831747
Received: 08 December 2021; Accepted: 10 January 2022;
Published: 31 January 2022.
Edited by:
Sarah Kucenas, University of Virginia, United StatesReviewed by:
Long-Jun Wu, Mayo Clinic, United StatesCopyright © 2022 Manjally and Tay. This is an open-access article distributed under the terms of the Creative Commons Attribution License (CC BY). The use, distribution or reproduction in other forums is permitted, provided the original author(s) and the copyright owner(s) are credited and that the original publication in this journal is cited, in accordance with accepted academic practice. No use, distribution or reproduction is permitted which does not comply with these terms.
*Correspondence: Tuan Leng Tay, dGx0YXlAYnUuZWR1
Disclaimer: All claims expressed in this article are solely those of the authors and do not necessarily represent those of their affiliated organizations, or those of the publisher, the editors and the reviewers. Any product that may be evaluated in this article or claim that may be made by its manufacturer is not guaranteed or endorsed by the publisher.
Research integrity at Frontiers
Learn more about the work of our research integrity team to safeguard the quality of each article we publish.