- 1Molecular Endocrinology and Pharmacology, Harry Perkins Institute of Medical Research and Centre for Medical Research, The University of Western Australia, Nedlands, WA, Australia
- 2Australian Research Council Centre for Personalised Therapeutics Technologies, Melbourne, VIC, Australia
- 3Australian Research Council Centre for Personalised Therapeutics Technologies, Perth, WA, Australia
- 4Florey Institute of Neuroscience and Mental Health, Parkville, VIC, Australia
- 5Department of Biochemistry and Pharmacology, School of Biomedical Sciences, Faculty of Medicine, Dentistry and Health Sciences, The University of Melbourne, Parkville, VIC, Australia
- 6Department of Molecular Medicine, The Scripps Research Institute, La Jolla, CA, United States
- 7Melbourne Dementia Research Centre, Florey Institute of Neuroscience and Mental Health, The University of Melbourne, Parkville, VIC, Australia
- 8Dimerix Limited, Nedlands, WA, Australia
- 9School of Biomedical Sciences, The University of Western Australia, Nedlands, WA, Australia
The orexin system comprises two G protein-coupled receptors, OX1 and OX2 receptors (OX1R and OX2R, respectively), along with two endogenous agonists cleaved from a common precursor (prepro-orexin), orexin-A (OX-A) and orexin-B (OX-B). For the receptors, a complex array of signaling behaviors has been reported. In particular, it becomes obvious that orexin receptor coupling is very diverse and can be tissue-, cell- and context-dependent. Here, the early signal transduction interactions of the orexin receptors will be discussed in depth, with particular emphasis on the direct G protein interactions of each receptor. In doing so, it is evident that ligands, additional receptor-protein interactions and cellular environment all play important roles in the G protein coupling profiles of the orexin receptors. This has potential implications for our understanding of the orexin system’s function in vivo in both central and peripheral environments, as well as the development of novel agonists, antagonists and possibly allosteric modulators targeting the orexin system.
Introduction
The orexin system was discovered in 1998 by two independent groups, De Lecea et al. (1998) and Sakurai et al. (1998). De Lecea et al. (1998) described an mRNA located within the hypothalamus that encodes for a peptide named prepro-hypocretin/orexin. Prepro-hypocretin/orexin, when cleaved, produces two highly conserved peptides termed hypocretin 1 and hypocretin 2. These peptides are also known as orexin-A (OX-A) and orexin-B (OX-B) respectively, and will be referred to using this terminology throughout this review. De Lecea et al. (1998) went on to show that Prepro-hypocretin/orexin was present specifically and exclusively in a limited population of cells in the dorsal and lateral hypothalamus (3,000–7,000 in rodents, up to 70,000 in humans), with immunoelectron microscopy studies indicating prepro-hypocretin/orexin associates with presynaptic vesicles. From these results, it was proposed that the OX-A and OX-B peptides were potential neurotransmitters, with their precursor’s localization in the lateral hypothalamus suggesting a role in energy homeostasis. Sakurai et al. (1998) also identified the presence of prepro-hypocretin/orexin mRNA within hypothalamic areas implicated in feeding behavior and demonstrated that administration of OX-A or OX-B into the lateral ventricles of rats resulted in increased food intake. However, Sakurai et al. (1998) first utilized reporter cell lines to de-orphanize G protein-coupled receptors (GPCRs), identifying the OX-A and OX-B peptides and the cognate OX1 and OX2 receptors (OX1R and OX2R, respectively) (Figure 1). This was part of a larger GPCR de-orphanization program (which included the orphan HFGAN72 sequence that corresponds to OX1R, allowing identification of two related expressed sequence tags that correspond to OX2R), conducted at the time in collaboration with Smith Kline Beecham, before their merger with Glaxo. Hence the two groups discovered the orexin system by two complementary approaches, identification of either the neuropeptides or the receptors first and then their respective receptor or neuropeptide counterparts in turn.
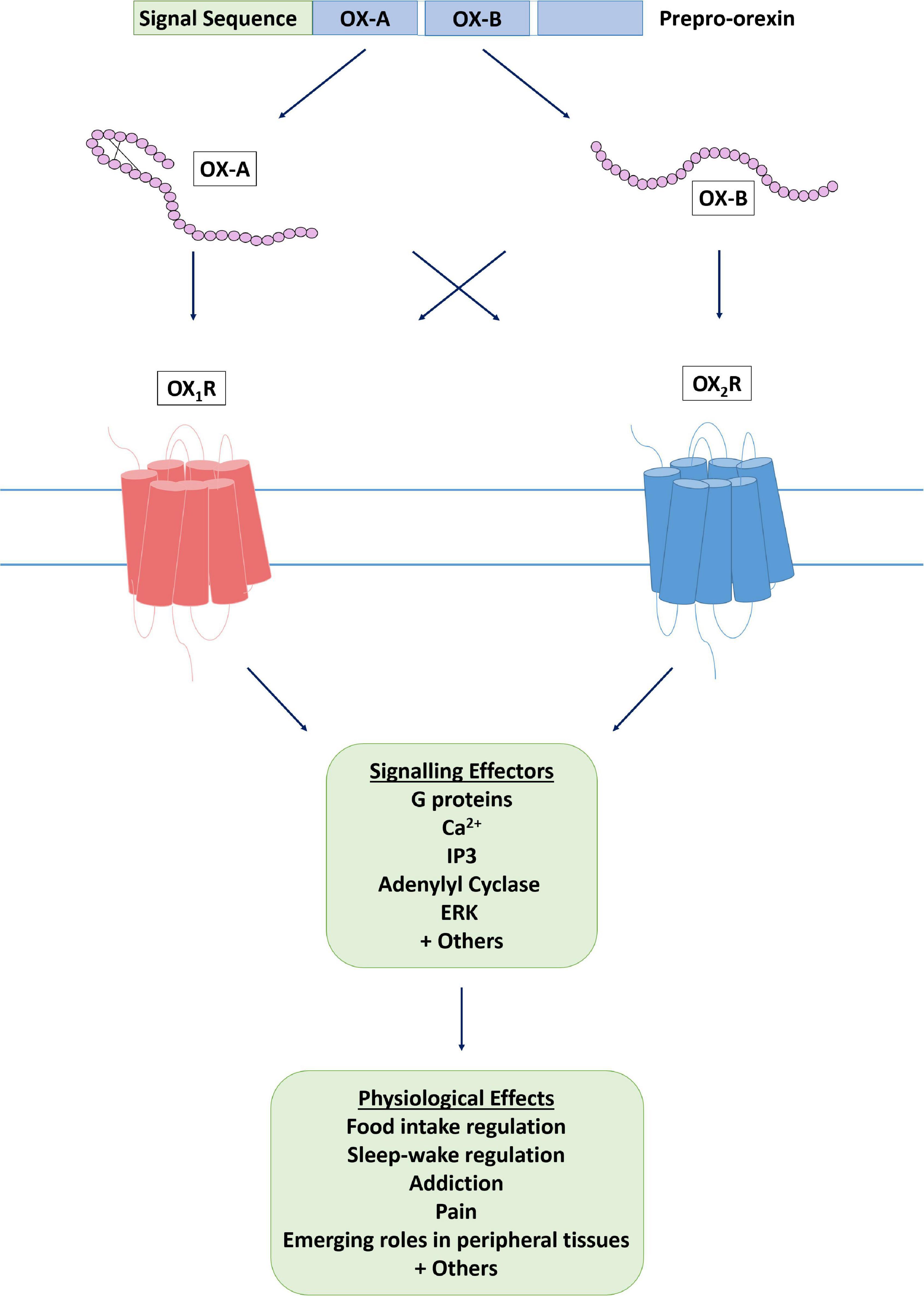
Figure 1. The orexin system. The orexin system consists of two endogenous peptide agonists, OX-A and OX-B, cleaved from the common precursor peptide prepro-orexin. Both agonists have affinity for the two orexin receptors, OX1R and OX2R, although it has been suggested that OX-B has some OX2R selectivity, whereas OX-A is not selective. Following agonist binding, an active conformation of the receptor is stabilized and effector proteins are recruited to induce a cellular response.
The discovery of OX-A and OX-B and the subsequent de-orphanization of OX1R and OX2R forms the basis of the orexin/hypocretin system as it is understood today. With regards to nomenclature, while hypocretin and orexin can be used interchangeably, it is most widely accepted to refer to related genes by the hypocretin name (in agreement with HUGO) while peptides and receptors are referred to by the orexin name (as recommended by IUPHAR, see Alexander et al. (2021), Coleman et al. (2021), so as to recognize the contributions of both discovery studies (De Lecea et al., 1998; Sakurai et al., 1998).
As previously mentioned, upon initial discovery, the orexin system was implicated in food intake regulation due to the prevalence of prepro-hypocretin/orexin and orexin receptors within the lateral hypothalamus – a brain region implicated in the regulation of food intake (De Lecea et al., 1998; Sakurai et al., 1998) – and subsequently the obese phenotype of orexin knockout mice. This localization evidence, combined with early observations that intracerebroventricular (ICV) administration of orexins resulted in an increase in feeding behavior in rats, heavily implicated the orexin system as a regulator of food intake (Sakurai et al., 1998; Edwards et al., 1999; Yamanaka et al., 2000). Further antagonist studies also supported this, with OX1R antagonists decreasing the orexigenic effect of OX-A administration (Haynes et al., 2000).
Further functional characterization and neuronal circuitry studies confirmed the orexin system as an important regulator of feeding behavior but also revealed orexin involvement in the regulation of other homeostatic mechanisms, in particular arousal and sleep-wake regulation (Peyron et al., 1998, 2000; Chemelli et al., 1999; Date et al., 1999; Edwards et al., 1999; Lin et al., 1999; Nambu et al., 1999; Yamanaka et al., 2000; Muroya et al., 2004; Sakurai and Mieda, 2011; Li and de Lecea, 2020). The focus of research into the orexin system took a marked pivot upon the observation that knockout of the prepro-hypocretin gene in mice produced a phenotype that exhibited striking similarities to human narcolepsy with cataplexy (Chemelli et al., 1999), also known as type 1 narcolepsy (NT1) (Mahoney et al., 2019). At the time, narcolepsy had no known cause and as such, this observation led to the rapid uptake of research into the orexin system’s role in the regulation of sleep (Lin et al., 1999; Scammell et al., 2000; Estabrooke et al., 2001; Saper et al., 2001; Sakurai, 2007; Tsujino and Sakurai, 2009). From this research came the discovery that the orexin system is a critical regulator of sleep-wake cycles, with the loss of orexin neurons (Thannickal et al., 2000, 2009; Hara et al., 2001; Honda et al., 2009) and the resulting loss of endogenous orexin (Nishino et al., 2000, 2001; Peyron et al., 2000; Ripley et al., 2001; Kanbayashi et al., 2002; Mignot et al., 2002) responsible for the rapid cycling between sleep and wake states, both during day and night, characteristic of human narcolepsy. The underlying cause of orexin neuron loss in human narcolepsy is still unknown, however autoimmune-related degenerative processes may play a role (Thannickal et al., 2000; Cvetkovic-Lopes et al., 2010; Lecendreux et al., 2017; Ramberger et al., 2017; Latorre et al., 2018; Luo et al., 2018; Bassetti et al., 2019; Lippert et al., 2019; Mahoney et al., 2019; Pedersen et al., 2019).
Additionally, the role of the orexin system in sleep-wake regulation was further validated when narcoleptic dogs were reported as having non-functional mutated OX2R (Lin et al., 1999; Hungs et al., 2001). This is particularly interesting, as similar receptor mutations have not been reported to cause narcolepsy in humans (Nishino et al., 2000, 2001; Peyron et al., 2000; Liblau et al., 2015). Indeed, NT1 is specifically linked to the loss of orexin producing cells in the hypothalamus and the resulting absence of measurable orexin in the cerebrospinal fluid of NT1 patients. Interestingly, orexin receptor mutant mice show different sleep phenotypes depending upon the receptor(s) mutated. OX1R knockout results in almost normal sleep-wake patterns, OX2R knockout results in a mild sleep phenotype with some sleep fractionation and very rare cataplectic events, whereas the double receptor knockout replicates the orexin peptide knockout (NT1) phenotype, with frequent cataplectic attacks, fast transitions from wake to REM sleep and sleep fragmentation (Willie et al., 2003; Mang et al., 2012; Hoyer and Jacobson, 2013; Jacobson et al., 2017, 2019). In particular, the sleep phenotype of the OX2R knockout mouse (Willie et al., 2003) did not replicate that of the orexin peptide knockout (Diniz Behn et al., 2010), nor that of the double receptor knockout, which both have fast transitions from wake to REM and cataplexy characteristic of NT1 in humans. The contributing role of OX1R to REM sleep modulation, was later confirmed in multiple studies showing that dual orexin receptor antagonists (DORAs) in rodents and humans over proportionally trigger REM sleep, whether during the active or inactive phase (Betschart et al., 2013; Hoyer and Jacobson, 2013; Hoyer et al., 2013). It has also become clear that DORAs have the ability to trigger cataplectic episodes in rodents, dogs and humans (Mahoney et al., 2020), and hence are contraindicated in patients suffering from NT1. This is also evident from studies combining OX1R and OX2R antagonists in rodents (Dugovic et al., 2009), where REM sleep is more affected than with an OX2R antagonist alone. Hence, there is contribution of both receptors to the phenotype displayed, however, the mechanistic basis for each receptors’ contribution is not yet understood, whether related to signaling and/or cellular/pathway crosstalk.
As these studies demonstrated that blockade/knockout of both receptors had a more pronounced effect on sleep than blockade/knockout of only one of the receptors, this led pharmaceutical and biotech companies to develop DORAs as a novel treatment for insomnia (Brisbare-Roch et al., 2007; Cox et al., 2010; Bettica et al., 2012; Coleman et al., 2012; Beuckmann et al., 2017; Berger et al., 2020; Boss et al., 2020). The critical role of OX2R was further evidenced in subsequent receptor antagonism/agonism studies performed in various transgenic mice which led to the alternate hypothesis that OX2R antagonism may produce more physiological sleep than DORAs (Mang et al., 2012; Betschart et al., 2013; Hoyer and Jacobson, 2013; Hoyer et al., 2013; Dugovic et al., 2014; Bonaventure et al., 2015; Clark et al., 2020). The DORA almorexant did not affect sleep-wake patterns in OX2R knockout mice, and was completely inactive in double receptor knockout mice, which incidentally confirmed that almorexant’s hypnotic effects were mediated exclusively by orexin receptors (Mang et al., 2012). Almorexant had major hypnotic effects in OX1R knockout mice, comparable to those seen in wildtype mice. However, a closer look at the polysomnography data suggested that almorexant’s effects on sleep were even more pronounced in the OX1R knockout (Mang et al., 2012). Along the same lines, OX-A produced no effect in double receptor knockout but had stimulatory effects in OX1R knockout mice, whereas it produced no obvious effects in OX2R knockout mice, emphasizing again a major role of the OX2R in wake-related activities (Mang et al., 2012). One unusual feature of a number of these DORAs is their very slow dissociation rates from the receptors, which result in sustained receptor occupancy and explain their long duration of action on top of rather long pharmacokinetic features (Mang et al., 2012; Callander et al., 2013; Farkas, 2013; Hoyer and Jacobson, 2013; Jacobson et al., 2014).
Since these initial studies revealing the orexin system’s role in critical homeostatic mechanisms, the system’s impact has been further expanded to include roles in addiction, pain, anxiety, panic, depression, binge eating and potentially post-traumatic stress disorder (PTSD) among others (Nakamura et al., 2000; Piccoli et al., 2012; Tsujino and Sakurai, 2013; Fitch et al., 2014; Li et al., 2014; Roh et al., 2014; Sakurai, 2014; Abbas et al., 2015; Bonaventure et al., 2015, 2017; Bonnavion et al., 2015; Soya et al., 2017; Steiner et al., 2018; Kaufmann et al., 2020). Additionally, a role for orexins and orexin receptors in peripheral tissues has also been suggested (Kirchgessner and Liu, 1999; Karteris et al., 2001, 2004, 2005; Mazzocchi et al., 2001; Randeva et al., 2001; Patel et al., 2018).
Mounting evidence has now demonstrated that classical G protein-dependent signaling at the plasma membrane is not the only way most GPCRs transduce signals. β-arrestin-dependent signaling (Smith and Rajagopal, 2016), signaling from GPCRs sequestered within subcellular compartments (Irannejad and von Zastrow, 2014; Thomsen et al., 2018) and bias toward specific signaling pathways due to ligand or cellular environment (Smith et al., 2018) have all been observed. Additionally, with the use of newly developed biosensors to probe G protein coupling to receptors [Wan et al., 2018; Laschet et al., 2019; Avet et al., 2020 (unpublished); Olsen et al., 2020], promiscuity in G protein coupling has been revealed to be more common than previously thought. Despite these shifts in dogma surrounding GPCR signaling, investigation of these concepts in relation to the orexin receptors has been very limited, with the understanding of their signaling properties mostly confined to the classical perspective. Here, the current understanding of orexin receptor interactions with direct effectors such as G proteins and β-arrestins, as well as the subsequent implications of these interactions for the signaling of the orexin system, will be discussed.
Calcium and Intracellular Messengers
Calcium Ion Movement
Ever since the orexin system’s discovery, activation of the orexin receptors has been strongly associated with a rise in intracellular Ca2+ (Sakurai et al., 1998). This is a hallmark of the receptors’ activation in all recombinant systems. Early studies aimed to elucidate the origin of the rise in cytoplasmic Ca2+ in order to give insight into the cellular mechanisms and transduction cascades involved in orexin receptor signaling.
Van Den Pol et al. (1998) used the fluorescent free Ca2+ indicator Fura-2 to perform imaging on hypothalamic neurons to characterize Ca2+ elevations upon stimulation with orexins. They observed that depletion of intracellular Ca2+ stores by pre-treatment with the endoplasmic reticulum Ca2+ ATPase inhibitor thapsigargin had no significant effect on the orexin-induced response. However, stimulation of cells in the presence of a chelating agent (EGTA) abolished the rise in intracellular Ca2+ concentration in response to stimulation with orexins.
Smart et al. (1999) subsequently utilized a Fluorescence Imaging Plate Reader (FLIPR) along with CHO cells transfected with OX1R or OX2R to characterize the rise in intracellular Ca2+. When OX1R was stimulated with 1 μM OX-A, a rapid peak in intracellular Ca2+ followed by a slow decline in the elevated Ca2+ level was observed. It is noted that similar results were observed for OX-B at OX2R, however these data were not shown. In contrast to Van Den Pol et al. (1998)’s findings, Smart et al. (1999) observed a rise in intracellular Ca2+ in the absence of extracellular Ca2+, however also observed that the Ca2+ response became more transient. Depletion of intracellular Ca2+ stores abolished the OX1R response to OX-A and OX-B. In addition, this study demonstrated that inhibition of phospholipase C (PLC) by U73122 abolished the Ca2+ response. These results were suggested to indicate that the orexins stimulate the release of intracellular Ca2+ stores for the rapid peak response as well as extracellular Ca2+ influx for the prolonged response, resulting in the typical biphasic pattern.
Lund et al. (2000) however, support extracellular Ca2+ influx as the primary mechanism of intracellular Ca2+ increase as a result of OX1R stimulation. Lund et al. (2000) demonstrate OX1R-mediated activation of cation channels and the resultant rise in intracellular Ca2+ acting synergistically with Gαq activation to increase PLC activation. These results suggest that inositol trisphosphate (IP3)-mediated Ca2+ release from intracellular stores is secondary and dependent upon extracellular Ca2+ influx for OX1R. This finding is reiterated in Larsson et al. (2005) and Johansson et al. (2007), who also implicate transient receptor potential cation channels (TRPCs) as mediating Ca2+ influx. Interestingly, these studies demonstrate the Ca2+ influx dependency of the response decreases at high concentrations of OX-A (0.1 μM and above), however the physiological relevance of this is unclear.
From these initial studies, subsequent works aimed to further elucidate the mechanisms underlying the robust Ca2+ response characteristic of orexin receptor activation. Studies in recombinant cells largely support a primary role for extracellular Ca2+ influx (Peltonen et al., 2009; Putula et al., 2014; Wang et al., 2014; Nagahara et al., 2015), however, divergences in function have been described between these recombinant systems and measurements in neurons. Indeed, there is conflicting evidence between neuronal studies (Van Den Pol et al., 1998; Uramura et al., 2001; Kohlmeier et al., 2004, 2008; Muroya et al., 2004; Kukkonen and Leonard, 2014). In summary, it appears that the orexin-induced intracellular Ca2+ increase is primarily a result of extracellular Ca2+ influx, with a small contribution from intracellular Ca2+ release, with cell- and tissue-specific effects also likely.
Downstream Signaling Interactions
Orexin receptor activation has been documented to result in an enormously varied set of downstream interactions, involving many key cascades within the signaling milieu. Studies investigating responses downstream of G protein activation indicate the involvement of multiple concurrent signaling mechanisms. The activation of effectors classically thought of as directly downstream of G protein activation are often used as indicators of specific G protein activity, despite the two not always being directly correlated. The activation of PLC, the production of diacylglycerol (DAG) and IP3 and an increase in intracellular Ca2+ have been widely characterized upon stimulation of orexin receptors. The activation of adenylate cyclase and production of cyclic adenosine monophosphate (cAMP) have also been characterized in some studies (Randeva et al., 2001; Tang et al., 2008; Kukkonen, 2016a,b).
Further downstream, activation of extracellular signal-regulated kinases (ERKs) (Ammoun et al., 2006a), phospholipase A2 (PLA2) (Turunen et al., 2012), phospholipase D (PLD) (Jäntti et al., 2012) and p38 mitogen-activated protein kinases (Ammoun et al., 2006b) among others (Ammoun et al., 2006a; Turunen et al., 2012) have been reported, once again highlighting the diverse signaling capabilities of the orexin system, the functional consequences of which are still being elucidated. To date, much of this research has been conducted within recombinant cell environments with a bias toward studying OX-A-induced OX1R function. Therefore an important part of future research will be examining these aspects of orexin receptor signaling within primary cell models and native tissue, as well as identifying how much of the OX-A-induced OX1R-derived signaling patterns can be applied to OX2R- and OX-B-induced signaling. Kukkonen (2016b) began to address this, characterizing the signaling effectors activated by OX2R in CHO cells and comparing the profile to that of OX1R. They observed the involvement of the same effectors described for OX1R with minor differences in potency for some signaling pathways. However, they did not investigate the effect of OX-B-induced stimulation and as such, any ligand-dependent effects at OX2R on the signaling pathways investigated remain to be characterized in such detail.
Further information on the downstream effectors and signaling cascades implicated in orexin function is summarized in Kukkonen and Leonard (2014) and Leonard and Kukkonen (2014).
G Proteins
As a result of receptor activation leading to increased intracellular Ca2+ levels and PLC activation, the orexin receptors were initially thought to couple to Gαq with subsequent PLC-IP3 signaling (Sakurai et al., 1998; Lund et al., 2000; Holmqvist et al., 2002). Further research expanded the G protein-coupling partners of the orexin receptors, with evidence of varying degrees of coupling to Gαq, Gαi/o and Gαs, depending upon the experimental conditions. Given this variation in the reported coupled G proteins, there is uncertainty around the relevance of these reports to endogenous function as the literature contains multiple accounts of conflicting evidence. A number of these discrepancies can be attributed, at least in part, to the varied cell and tissue conditions under which orexin signaling has been studied. This possible tissue- and cell-dependent variation in the G protein coupling indicates that the effector proteins may be influenced by different environmental conditions (Randeva et al., 2001; Caillol et al., 2003; Woldan-Tambor et al., 2011). Other factors may also contribute to these perceived differences, including the stimulating ligand used, ligand concentration and experimental method. Of particular note is the abundance of G protein coupling data that has been inferred based upon assays that detect the activation of downstream messengers of G proteins. This has been historically necessary due to the lack of techniques to study G protein coupling directly. However, given the well-characterized promiscuity of the orexin receptors’ G protein coupling [Avet et al., 2020 (unpublished)], and the possibility for cross-regulation between classical signaling cascades (Houslay and Kolch, 2000; Halls and Cooper, 2011), caution must be used when applying these data to determine G protein coupling. Here, we will look in-depth at the evidence for G protein coupling to each of the orexin receptors.
G Protein Coupling to Both Receptors
In their discovery study, Sakurai et al. (1998) suggested that the orexin receptors couple to Gαq due to the generation of OX-A or OX-B concentration-dependent increases in intracellular Ca2+ in CHO cells stably transfected with OX1R or OX2R. Van Den Pol et al. (1998) subsequently gave indirect evidence for coupling to Gαq, as inhibition of PKC using the inhibitor bisindolylmaleimide abolished orexin-induced intracellular Ca2+ increase. However, which specific orexin receptor was mediating this effect in the hypothalamic neurons was not suggested or investigated.
Randeva et al. (2001) detected OX-A-induced activation of Gαq, Gαs, and Gαi in human adult adrenal gland membrane preparations using a GTP-azidoanilide-labeling and subsequent immunoprecipitation technique. Subsequent functional analysis showed a concentration-dependent increase in both cAMP and IP3 production upon treatment with OX-A. These findings are in agreement with previous data detailing increased cAMP levels in adrenal cells upon stimulation with orexins (Malendowicz et al., 1999; Mazzocchi et al., 2001). Randeva et al. (2001) also characterized the receptors present within the adrenal tissue. Using RT-PCR, the expression of OX2R, but not OX1R, was observed and this was confirmed using various additional methods, as was the presence of prepro-orexin. The orexin expression pattern in adrenal tissue is contested however, as discussed in the “Signaling in Peripheral Cell and Tissue Environments” section below.
Zhu et al. (2003) utilized neuronal hybrid cells (BIM cells) stably expressing OX1R-EGFP or OX2R-EGFP to measure the effect of orexin stimulation on cAMP and intracellular Ca2+ levels. From the trends observed in these pathways, Zhu et al. (2003) suggest coupling of both receptors to Gαq, OX2R but not OX1R coupling to Gαi, and coupling of neither receptor to Gαs. However, as this study only investigated effectors downstream of G protein coupling, and had limited use of pathway modulators [such as pertussis toxin (PTX) and cholera toxin (CTX)], these suggestions should be treated with caution. The lack of data on the effect of OX-B stimulation on cAMP in this study is also of note. A bias toward studying OX-A-induced responses is present within the literature, with markedly less data available on the effects of OX-B. This is unfortunate, as ligand-specific effects have been reported for the orexin system and therefore important signaling events have potentially been missed due to the omission of OX-B in such studies. Ligand-specific effects that have been elucidated are discussed in detail in the “Endogenous Ligands” section below.
OX1R G Protein Coupling
Multiple studies followed that aimed to investigate the coupling of OX1R to G proteins and the associated signaling cascades. Holmqvist et al. (2005) utilized CHO cells stably expressing OX1R to study the response to orexins of multiple downstream G protein effectors. CTX (which constitutively activates Gαs) and PTX were used to probe for potential G protein mediation with both positive and negative regulation of cAMP production observed. Robust and concentration-dependent intracellular Ca2+ elevation and inositol phosphate production was also observed. From these results, the authors suggest that in CHO cells, OX1R couples to a minimum of three G proteins: Gαi/o, Gαs, and Gαq.
Magga et al. (2006) used immunoprecipitation of C-terminally FLAG-tagged OX1R and subsequent immunoblotting with Gα subunit-specific antibodies to investigate the G protein coupling partners of OX1R in HEK293 cells. Gαq showed the strongest signal for co-immunoprecipitation with OX1R-FLAG and this interaction was detected in all experiments. Co-immunoprecipitation was also observed with Gαi and Gαs but this was not detected in all experiments (6/11 and 2/3 independent experiments respectively) with no detection of Gαo (0/5). Subsequent functional analysis showed stimulation of HEK293 cells stably expressing OX1R-FLAG with OX-A resulting in no change to cAMP levels from basal or when pre-treated with forskolin. From this study, it is not clear if OX1R couples to Gαs or Gαi in HEK293 cells. While the co-immunoprecipitation data indicate weak coupling may be present, the functional data were unable to support this.
Woldan-Tambor et al. (2011) observed robust cAMP production in rat cerebral cortex astrocyte cultures upon stimulation with OX-A. This effect was not seen upon treatment with the moderately OX2R selective agonist [Ala11,D-Leu15]-OX-B and was partially inhibited by an OX1R-selective antagonist (SB 40812), but not by the OX2R-selective antagonist (TCS OX2 29).
OX2R G Protein Coupling
Research focused specifically on OX2R came somewhat later than that on OX1R. Using dominant negative G proteins in HEK293 cells stably expressing OX2R, Tang et al. (2008) observed Gαq, Gαs and Gαi involvement in orexin-induced ERK activation. Even though involvement of all three G proteins in downstream effector activation was detected, there was evidence for ligand-specific bias in the activated G proteins and cascades. This is discussed in detail in the “Endogenous Ligands” section, along with potential limitations of the methodologies used in the study.
These findings were in stark contrast to the findings of Urbańska et al. (2012) in primary cultures of rat cortical neurons which showed no effect of orexin stimulation on cAMP levels, suggesting no direct activation via Gαs or any indirect activation, e.g., via PLC. They did, however, observe inhibition of forskolin-induced cAMP accumulation with orexin stimulation, which was completely reversed by PTX treatment, as well as inhibited by an OX2R-selective antagonist (TCS OX2 29) but not an OX1R-selective antagonist (SB 408124). These results suggest OX2R-mediated activation of Gαi is observed upon stimulation with orexins in this particular cell model.
Kukkonen (2016a) revisited the coupling partners of both OX1R and OX2R in CHO cells by investigating the effect of G protein inhibitors on downstream signaling effectors. It was observed that for both receptors, Gαq was responsible for the vast majority of responses, including intracellular Ca2+ accumulation, which was abolished with UBO-QIC (Gαq inhibitor) treatment. Their results also gave evidence for Gαs-mediated adenylate cyclase activity with OX-A stimulation at both receptors. Additionally, Kukkonen (2016b) suggested that OX2R has weaker coupling to Gαs relative to OX1R in CHO cells, as only weak adenylate cyclase activity could be detected upon stimulation with OX-A. Interestingly, UBO-QIC and PTX were found to reduce and abolish, respectively, the inhibitory effect of OX-A stimulation on adenylate cyclase for OX1R and OX2R, indicating this effect that has classically been attributed to Gαi may also be partially mediated by Gαq. It is noteworthy that while these studies provide an excellent comparison of OX1R and OX2R G protein coupling and downstream signaling, no investigation with OX-B stimulation was pursued. Given that biased agonism of OX-A and OX-B to specific G protein signaling cascades has been reported previously (Tang et al., 2008), this would be an interesting aspect to investigate further.
More recently, Baker and Proudman (2021) reported a biphasic cAMP response to OX-A and OX-B in CHO cells expressing human OX2R but not in OX1R expressing cells. The inhibitory, but not the stimulatory component, was PTX sensitive. Both OX-A and OX-B were highly potent activators of Ca2+ release, inositol phosphate (IP) accumulation and ERK1/2 activity, with moderate or significant selectivity for OX-B. Interestingly, in the same study compound C (Nagahara et al., 2015) was potent and OX2R selective in the latter three assays, but had no effect on cAMP accumulation, suggesting biased signaling. Furthermore, the potency values of OX-B varied between OX2R-mediated Ca2+ release, IP accumulation, ERK1/2 activity and cAMP inhibition and activation (pEC50 = 10.36, 9.84, 11.08, 9.68, and 7.85). Similar differences were observed for OX-A in the same assays (pEC50 = 9.72, 9.28, 10.47, 9.54, and 7.72). The potency variations of OX-A and OX-B at the OX1R were less marked. Altogether, these data would suggest that natural and synthetic agonists can display pathway selectivity at human OX2R, with a range of potency and/or apparent efficacy values that are signaling dependent. Similar although less marked differences were noticed at OX1R, except that cAMP was not affected by any of the ligands tested.
Signaling in Peripheral Cell and Tissue Environments
Studies often characterize the presence of orexins and orexin receptors within cells or tissue by utilizing techniques such as RT-PCR, western blot and fluorescence in situ hybridization (FISH). In some cases, a predominant receptor subtype can be identified, allowing for signaling characteristics to be attributed as predominantly OX1R- or OX2R-mediated. However, when both receptors are detected, dissecting receptor-specific signaling becomes more difficult and requires the use of receptor-specific antagonists or other tools. While orexin receptors can be located within the same areas of the CNS, they most often show distinct, complementary distribution (Marcus et al., 2001; Sakurai and Mieda, 2011). As such, research using CNS-derived cells and tissues rarely encounter this issue, with signaling observations often attributable to the one present orexin receptor subtype. In contrast, expression of both receptors appears to be more common within the periphery, and as such, techniques to investigate the contribution of each receptor are necessary in these studies.
In addition, there are conflicting data on the expression of orexin receptors within peripheral environments, making analysis of results in these studies difficult (Heinonen et al., 2008; Kukkonen, 2013). Perhaps the most prominent example of this is in adrenal gland tissue and cells, in which some studies report only OX2R expression (Karteris et al., 2001; Randeva et al., 2001) while others report expression of both OX1R and OX2R (Lopez et al., 1999; Spinazzi et al., 2005).
These conflicting reports may be attributable to both lack of binding to orexin receptors and lack of specificity of anti-orexin receptor peptide antibodies (Kukkonen, 2013), although inter-species and intra-organ (e.g., adrenal cortex vs. medulla) differences may also contribute (Heinonen et al., 2008). As such, while signaling behaviors are sometimes attributed to specific receptors, this analysis should be approached with caution.
Adrenal Tissue
One of the earliest studies to investigate peripheral orexin signaling and G protein coupling was the previously mentioned study by Randeva et al. (2001) which gave evidence for OX2R coupling to Gαq, Gαs, and Gαi, but not Gαo in human adult adrenal tissue. A similar study in human fetal adrenal tissue supported the finding of OX2R, but not OX1R expression within human adrenal glands. However, interestingly, upon stimulation with OX-A, coupling of Gαs and Gαi but not Gαq was detected by GTP-azidoanilide labeling (Karteris et al., 2001). Why this difference between fetal and adult coupling was observed is unclear and warrants further investigation, although it is not uncommon to observe differences in protein expression between fetal and adult tissues. Also of note, both these studies investigated OX-A-induced responses only, as OX-B was not tested.
Contrasting results subsequently gave evidence for coupling of Gαq, Gαs, Gαi as well as Gαo in rat adrenal cortex and also the hypothalamus (Karteris et al., 2005), however, this may represent a species-specific difference. Remarkably, this study also showed changes to the G protein activation profile upon OX-A stimulation following 24 h of food deprivation. Subsequent work to further interrogate this change in G protein activation would assist in understanding the implications of this finding, for example, analysis of the contribution of each receptor. However, this study serves as yet another example of the potentially fluid and highly variable nature of orexin signaling.
Ramanjaneya et al. (2008) investigated orexin signaling in the H295R adrenocortical cell line. Steroidogenic acute regulatory protein (StAR) is a transporter protein important to acute steroid synthesis. Ramanjaneya et al. (2008) demonstrated that both orexin ligands upregulate expression of StAR in H295R cells. Using dominant negative G proteins, which it should be noted can have issues of non-specificity (Kukkonen, 2004), they subsequently suggested the involvement of Gαq, Gαs, and Gαi in OX-A-induced StAR expression, but only Gαq and Gαs involvement in OX-B-induced StAR expression. An OX1R-selective antagonist was used to determine the role of each orexin receptor in these responses, with OX1R found to be mediating OX-A-induced coupling, and both receptors, but predominantly OX1R, mediating the OX-B-induced response. In a second study, Ramanjaneya et al. (2009) investigated the regulation of mitogen-activated protein kinase (MAPK) activation by the orexin system, showing distinct G protein coupling to ERK/MAPK cascade regulation for both ligands. They suggested Gαq, Gαs, and Gαi involvement that was predominantly OX1R-mediated, with minor input from OX2R for both ligands’ effects. Although these studies did not investigate any possible activation or involvement of Gαo within this cell model, they regardless give insight into the potential complexity of orexin-G protein coupling within adrenal tissue.
Orexin receptors have been detected within many peripheral tissues (Johren et al., 2001; Heinonen et al., 2008), however G protein coupling of the orexin system within these tissues has not been widely investigated, with the most in-depth analysis found in the adrenal glands as discussed above. Future research in a range of peripheral tissues may shed light on the enigmatic orexin G protein coupling systems. Furthermore, an area of orexin research that has been reinvigorated by peripheral tissue studies pertains to the roles of the two orexin ligands and the potential differences in their signaling and function. Further discussion of this can be found in the “Endogenous Ligands” section.
Non-G Protein Modulation
While much attention has been given to G protein activation and the subsequent signaling cascades, the role of other effector proteins in orexin pharmacology has been less thoroughly interrogated. Important receptor accessory proteins, such as arrestins, have the ability to impact signaling behavior, and therefore understanding these interactions could reveal important insights into the function of orexin receptors.
β-Arrestins
Both orexin receptors have been shown to interact robustly with β-arrestins. Along with their classical role in signal cessation and receptor internalization, β-arrestins also scaffold secondary signaling platforms (Lefkowitz and Shenoy, 2005; DeWire et al., 2007).
Evidence that β-arrestin2 plays a role in orexin signaling through ERK pathways is given by Milasta et al. (2005). Notably, ERK1/2 phosphorylation was reduced and the time-scale of ERK1/2 phosphorylation shortened with a mutant OX1R that reduced agonist-induced β-arrestin2 recruitment. This was despite no significant changes to the induction of increased intracellular Ca2+, indicating these changes do not result from general reduced signaling capacity. Additionally, while β-arrestin2-dependent internalization of the mutated receptor was minimally impacted, colocalization of OX1R and βarrestin-2 within endosomal compartments following internalization was not observed with the mutant receptor, in contrast to the wildtype OX1R. This study did not investigate whether the same patterns could be observed with OX2R or OX-B treatment, however subsequent studies suggest OX1R and OX2R have distinct interaction profiles with β-arrestin2.
In COS-7 cells, Dalrymple et al. (2011) observed β-arrestins to be recruited with a higher potency to OX2R compared to OX1R in response to OX-A. Using a kinetic BRET assay, OX1R underwent relatively transient interactions with arrestin-ubiquitin complexes compared to OX2R that showed more stable and prolonged interactions. Additionally, ERK1/2 phosphorylation in response to OX-A stimulation was similar for both receptors in the short term (up to 10 min post-stimulation), however, OX1R exhibited lower levels of sustained ERK1/2 phosphorylation compared to OX2R (Figure 2). Dalrymple et al. (2011) suggested β-arrestins may form a more stable secondary signaling platform with OX2R than with OX1R, indicating a mechanism that may play a role in the diverging functional profiles of the two receptors.
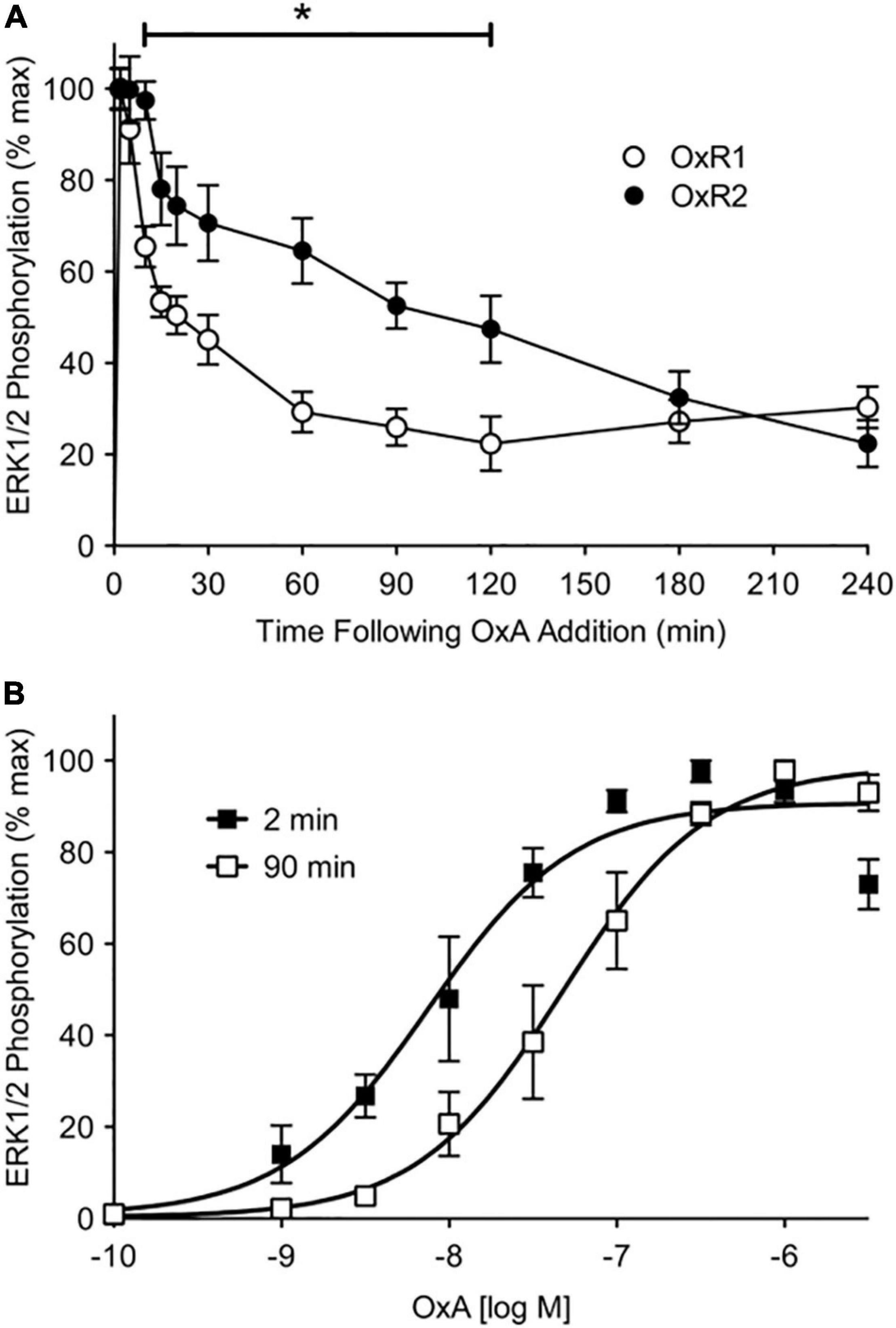
Figure 2. ERK1/2 phosphorylation data for OX1R (OxR1) and OX2R (OxR2) stably transfected in HEK293 cells. (A) Stably transfected HEK293 cells were treated with OX-A (OxA) and measured over a 4-h period. Data were normalized to time-matched vehicle treatments and are expressed as a percentage of the maximal response induced at 2 min post-agonist treatment. Data are expressed as mean ± SE of four independent experiments. *p < 0.05 between OX1R and OX2R from 10 to 120 min post-agonist stimulation. (B) Concentration-response data were collected at 2- and 90-min post OX-A treatment of OX2R-expressing cells. Data are expressed as a percentage of the maximal response induced at the time point (mean ± S.E. of four independent experiments). Reproduced from Dalrymple et al. (2011) under a Creative Commons Attribution 4.0 International License. Full terms provided at https://creativecommons.org/licenses/by/4.0/.
Possible structural determinants of the orexin-β-arrestin interaction profiles were investigated by Jaeger et al. (2014). Using site-specific mutagenesis to isolate the contribution of serine and threonine residues in the C-terminal tail of OX2R, they showed that OX2R has two C-terminal serine/threonine clusters that are phosphorylated by GRKs, creating a strong affinity for the receptor with β-arrestin. This is in contrast to OX1R which has only one C-terminal serine/threonine cluster important for GRK phosphorylation, resulting in the less stable interactions reported by Dalrymple et al. (2011). This difference in C-terminal phosphorylation sites is also suggested to be the structural determinant underpinning the slower recycling rate of OX2R observed by Dalrymple et al. (2011), with OX2R requiring more significant dephosphorylation post-internalization to dissociate from β-arrestin, resulting in the receptor remaining within the cell for longer. These results were produced using only OX-A stimulation. Whether different structural determinants may be involved in OX-B-induced β-arrestin2 interactions is unknown. Regardless, these foundational studies give insight into the role of β-arrestin in orexin signaling and demonstrate the potential importance of non-G protein interactions.
With the exception of arrestins, research investigating the potential role of accessory proteins in the control of orexin receptor signaling is sparse. Further in-depth investigation into the role of such proteins could uncover further modulators of orexin signaling. This could ultimately aid in explaining the complex signaling and functional characteristics described for the orexin system.
Heteromerization
Heteromerization has been suggested to play a functional role in the orexin system, with particular focus on heteromerization of orexin receptors with opioid and cannabinoid receptors (Thompson et al., 2017). However, it is of note when considering these reports that these studies rely heavily on recombinant expression, which is prone to overexpression artifacts. Reported functional interactions between orexin receptors and other GPCRs are listed in Table 1.
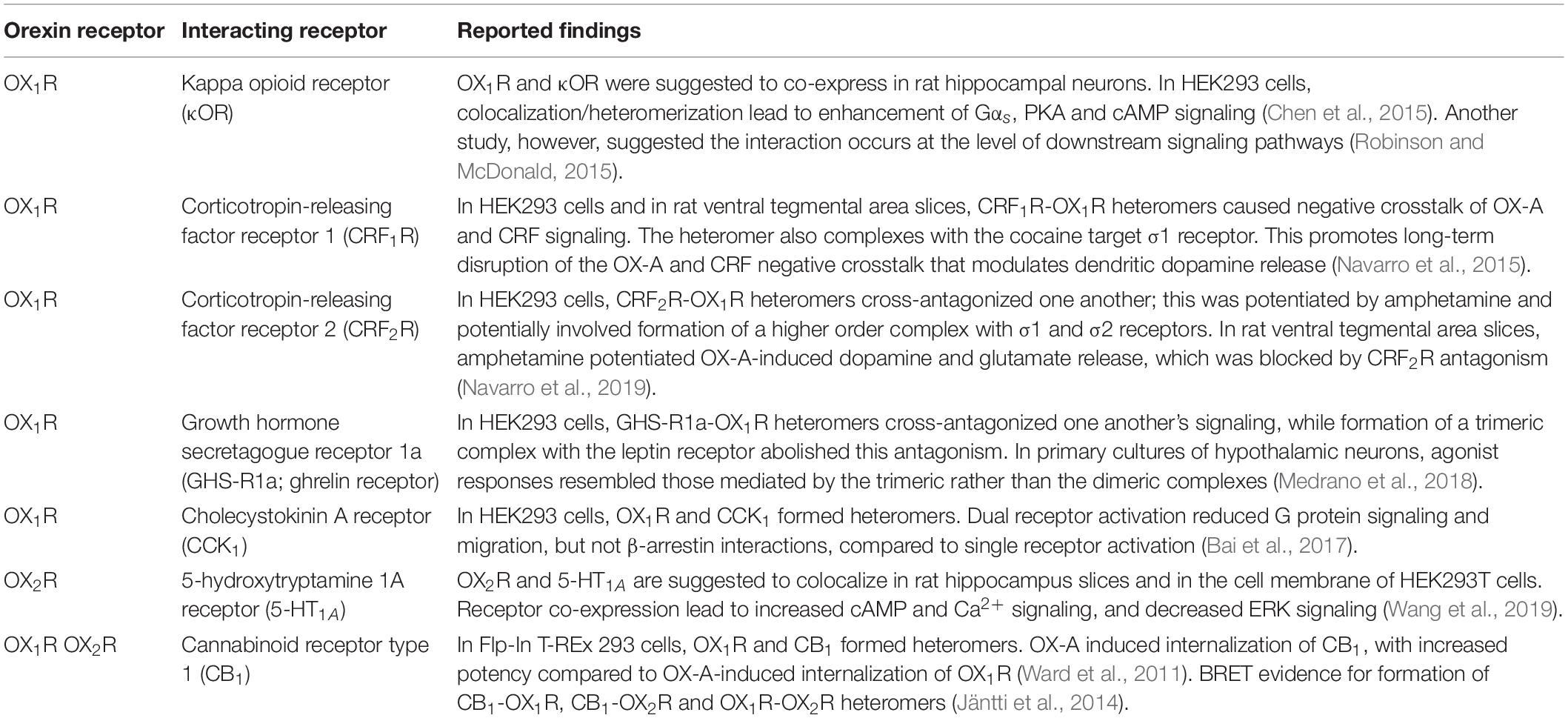
Table 1. Select reported interactions between orexin receptors and other GPCRs in native and recombinant environments.
The effects of orexin receptor heteromerization include cross-activation and inhibition, as well as novel signaling mechanisms that are different from those of the monomeric receptor. An example of this is seen with the kappa opioid receptor (κOR)-OX1R heteromer, in which signaling through Gαs is observed in response to OX-A or dynorphin A, with a reduction in Gαi and Gαq signaling through κOR and OX1R, respectively, upon heteromerization (Chen et al., 2015). Heteromerization resulting in novel signaling is strong evidence of a functional interaction between the two receptors that may result in physiologically relevant applications. Given the wide range of reported heteromers from the orexin system, the formation of higher order receptor oligomers is likely to be an important aspect of the orexin system’s complex in vivo functions, and may underlie some of the reported signaling variation in different cell and tissue types. Further research is needed to determine the extent of the influence of heteromerization on these factors, as well as further characterize the novel signaling properties of specific orexin heteromers.
Ligands
Endogenous Ligands
The orexin system has two known endogenous ligands, OX-A and OX-B, both resulting from a common precursor, prepro-orexin. OX-A is a 33 amino acid peptide with two intrachain disulfide bonds, while OX-B is a 28 amino acid linear peptide. Both orexins have a C-terminal amide group (Sakurai et al., 1998). In their first report of hypocretin/orexin peptides, De Lecea et al. (1998) reported the fast neuroexcitatory effects of the native OX-B (1 μM) in various rat hypothalamic neuronal cultures and the absence of effects of OX-B in four different hippocampal dentate granule neuronal cultures. In these early studies, OX-A activity was not tested.
In CHO and HEK293 cells, OX1R shows preferential binding of OX-A over OX-B while at OX2R, OX-A and OX-B have similar affinities in radioligand binding experiments (Sakurai et al., 1998). Similar trends are reflected in the potency of the ligands at the two receptors, with OX-A and OX-B showing equivocal potency at OX2R in IP production assays while OX-B shows significantly lower potency at OX1R (Holmqvist et al., 2002; Dalrymple et al., 2011; Kocan et al., 2011). Despite these characterizations, many nuanced ligand-specific effects have been reported, the roles of which remain to be elucidated. In other words, the putative receptor selectivity of OX-B needs to be re-considered, depending on cellular environment and/or transduction mechanism considered. The data reported by different groups in a variety of cells (whether collected from recombinant systems or from primary cultures) and/or assays, demonstrate variations in apparent affinity, potency and to some extent efficacy (see above, e.g., Section “OX2R G protein coupling”). Therefore, the purported “selectivities” for endogenous and synthetic ligands, especially agonists, show marked variations and the use of “selective” when describing such ligands must be taken with caution. An obvious consequence of this, is that effects mediated by endogenous orexin receptors need to be characterized using adequate pharmacological tools, preferably by measuring rank orders of potency of several antagonists with various degrees of selectivity, rather than the limited use of poorly selective agonists.
From observations on the effects of both ligands on second messenger cascades in CHO cells, Holmqvist et al. (2005) demonstrated that OX-A and OX-B do not exhibit the canonical affinity-efficacy relationship at OX1R that had been previously described. While the relative potencies of the ligands were as expected for the Gαq-mediated cascades, for the Gαs-mediated cascades the relative potencies of the two ligands were observed to be similar. Tang et al. (2008) also observed biased agonism of OX-A and OX-B in their activation of G proteins in HEK293 cells stably expressing OX2R. In HEK293 cells, OX-B exhibited bias toward Gαq-mediated pathways while OX-A seemed to favor Gαs- and Gαi-mediated pathways of ERK activation. Neither OX-A nor OX-B showed total bias to a specific pathway for ERK activation, with activity detected for all three G protein cascades that were screened with both OX-A and OX-B. However, it is also of note that this study utilized dominant-negative G proteins, which have been reported to have non-specific effects (Kukkonen, 2004).
Gorojankina et al. (2007) also noted differences in the action of the orexin ligands within an immortalized cell line of olfactory sensory neuron lineage (Murrell and Hunter, 1999) transfected with OX1R-EGFP or OX2R-EGFP. In this cell line, OX-B produced a synergistic increase in cAMP production with co-treatment of forskolin, however, this was not observed with treatment of OX-A. This was observed with both receptors.
A differential role for OX-A and OX-B in peripheral tissues, in particular adrenal tissue, was postulated early on, when OX-A but not OX-B stimulation resulted in a concentration-dependent increase in basal cAMP production (Mazzocchi et al., 2001). Initially this was thought to be due to the presence of OX1R with only low level detection of OX2R (Mazzocchi et al., 2001), however further research conflicted with these findings (Randeva et al., 2001). In contrast, OX-B was observed to be more potent in stimulating IP3 production in human testicular membrane preparations (Karteris et al., 2004). While it is suggested this indicates a predominantly OX2R-mediated effect, no further investigation was conducted.
The possible implications of such differences are highlighted by Patel et al. (2018) in which OX-B, but not OX-A, was found to produce contractile shortening through OX2R in cardiomyocytes. It was further observed that OX-B exerted a cardioprotective effect in rat heart models. The G protein-based signaling mechanisms underlying these effects were not thoroughly investigated in this study, however the phosphoinositide 3-kinase/protein kinase B (PI3K/Akt) and ERK1/2/MAPK pathways were implicated as mediators of the OX-B-induced cardioprotective effects observed.
Experiments further investigating these ligand-dependent biases, including effects on other cellular machinery and translation of findings into higher-order cell or tissue environments, would give great insight into the relative roles of OX-A and OX-B.
Exogenous Ligands
The majority of drug discovery efforts targeting the orexin system have been focused on the development of antagonists as treatments for insomnia and other sleep and mental health disorders. This has led to the development of selective and potent small molecule orexin receptor antagonists as shown in Table 2. Antagonist research has focused on DORAs which have been, at least initially, preferred for clinical applications, since double receptor knockout mice had the most pronounced sleep phenotypes (Mang et al., 2012). However, there is evidence that dual antagonism can promote cataplexy-like symptoms in rodents (Black et al., 2013) and the Food and Drug Administration (FDA) has issued a warning about such events in humans (Farkas, 2013; Hoyer and Jacobson, 2013). Suvorexant and lemborexant are the only orexin-targeted drugs on the market for the treatment of insomnia, with the New Drug Application for Idorsia’s daridorexant (ACT-541468) just being approved by the FDA. Multiple other DORAs and selective orexin receptor antagonists (SORAs), are currently under development/in clinical trials for the treatment of a variety of CNS disorders, such as depression, anxiety, panic or PTSD (Fitch et al., 2014; Bonaventure et al., 2015, 2017; Boss et al., 2020; Kaufmann et al., 2020; Maehara et al., 2020; Zammit et al., 2020b). Alongside the development of antagonists for clinical use, highly selective and potent orexin antagonists have also been produced for research applications as these compounds are highly desirable for probing receptor-specific effects (Nagase et al., 2017).
In contrast to antagonist research that has focused on non-selective compounds, much of the research relating to orexin receptor agonists is focused on OX2R-selective agonists. This is in part due to evidence indicating that stimulation of OX1R in the ventral tegmental area increases dopaminergic transmission, raising concerns that OX1R-targeted agonists may result in dependency issues (Nakamura et al., 2000; Yukitake et al., 2019). For the agonists that have been developed (Nagahara et al., 2015; Turku et al., 2016, 2019; Leino et al., 2018; Rinne et al., 2018), comprehensive analysis of their pharmacological profiles has not been conducted. Studies have mainly relied upon Ca2+ measurements to determine receptor selectivity and agonist activity, since until recently, FLIPR has been a traditional workhorse for drug screening in industry. Given the apparent differences between OX-A and OX-B at both orexin receptors, detailed pharmacological profiling of these new synthetic agonists will hopefully assist in developing our understanding of the pharmacological function of the orexin receptors.
Initial reports indicate further optimization may be needed before some of the proposed agonists are suitable for clinical applications (Irukayama-Tomobe et al., 2017), however the OX2R-selective agonist YNT-185 has shown positive results in ameliorating morphine-induced sedation without affecting analgesia in a rat model (Toyama et al., 2018). Additionally, the OX2R-selective agonist TAK-925 has shown wake-promoting effects in wild-type mice, but not OX2R-knockout mice, indicating target-specific effects in an in vivo model (Yukitake et al., 2019) as was expected from original studies performed in a variety of orexin receptor knockout mice (Willie et al., 2003; Mang et al., 2012).
As the orexin system has been demonstrated to stimulate multiple downstream signaling pathways, there is exciting potential for therapeutically harnessing any existing ligand-induced bias. An example of this potential is seen in Yukitake et al. (2019) in which the OX2R-selective agonist TAK-925 was characterized using an array of methods.
With respect to phospholipase C activity, TAK-925 and the endogenous ligands had equal potency and maximum response. For cAMP response element-binding protein (CREB) and ERK phosphorylation, the potencies for all three ligands were essentially the same but the maximum response to TAK-925 was somewhat lower. With respect to Ca2+ elevation and β-arrestin2 recruitment, the endogenous ligands had equal potency while the potency of TAK-925 was 30–50- fold lower; all ligands had the same maximum response. No data were presented on the relative potency of the agonist on non-Gαq mediated effects. As more is revealed about the interplay of multiple G proteins in orexin signaling, the potential for biased agonists may become a particularly interesting consideration for the therapeutic targeting of this system.
The development of orexin-targeted therapeutics, especially agonists, is still in the early stages with much more work needed to explore their therapeutic potential. Interest in orexin-targeted therapeutics has remained strong since initial research began in the early 2000’s. Significant efforts have been made in recent years to produce novel small molecule orexin agonists and antagonists which hold exciting potential for future therapeutics. The translation of these compounds to clinical use has been recently demonstrated with Lemborexant being approved in the US and Japan, and the FDA approving daridorexant in January 2022, as well as other compounds, e.g., seltorexant, being in late stage clinical development (Dauvilliers et al., 2020; Hoyer et al., 2020; Kaufmann et al., 2020; Muehlan et al., 2020a,b; Zammit et al., 2020a,b).
Conclusion
Research into orexin pharmacology, while fragmented, presents a body of interesting and exciting research, which demonstrates many complex signaling behaviors that could hold vital insights into orexin system function and GPCR pharmacology as a whole. The recent development of various biophysical assay panels (Wan et al., 2018; Avet et al., 2020; Olsen et al., 2020) allows for monitoring of the effect of receptor stimulation on a wide range of GPCR effectors, in live cells and in real-time. Applying technologies such as these to study orexin receptor signaling will allow investigation of orexin receptor pharmacology with greater detail and precision. Indeed, the elucidation of orexin function in peripheral environments, and the development of novel, small molecule ligands in recent years demonstrates that there is undoubtedly much still left to be discovered about this system.
Author Contributions
All authors listed have made a substantial, direct, and intellectual contribution to the work, and approved it for publication.
Funding
This work was supported by an Australian Government Research Training Program Scholarship and a University of Western Australia Baillieu Research Scholarship (ND), an Australian Research Council Industrial Transformation Training Centre IC170100016 Postdoctoral Fellowship (EJ), and an NHMRC Ideas Grant APP20033770 (DH and LJ).
Conflict of Interest
KP is Chief Scientific Advisor to Dimerix, of which he has a shareholding.
The remaining authors declare that the research was conducted in the absence of any commercial or financial relationships that could be construed as a potential conflict of interest.
Publisher’s Note
All claims expressed in this article are solely those of the authors and do not necessarily represent those of their affiliated organizations, or those of the publisher, the editors and the reviewers. Any product that may be evaluated in this article, or claim that may be made by its manufacturer, is not guaranteed or endorsed by the publisher.
References
Abbas, M. G., Shoji, H., Soya, S., Hondo, M., Miyakawa, T., and Sakurai, T. (2015). Comprehensive behavioral analysis of male Ox1r(-/-) mice showed implication of orexin receptor-1 in mood, anxiety, and social behavior. Front. Behav. Neurosci. 9:324. doi: 10.3389/fnbeh.2015.00324
Alexander, S. P., Christopoulos, A., Davenport, A. P., Kelly, E., Mathie, A., Peters, J. A., et al. (2021). The Concise Guide to PHARMACOLOGY 2021/22: G protein−coupled receptors. Br. J. Pharmacol. 178, S27–S156. doi: 10.1111/bph.15538
Ammoun, S., Johansson, L., Ekholm, M. E., Holmqvist, T., Danis, A. S., Korhonen, L., et al. (2006a). OX1 orexin receptors activate extracellular signal-regulated kinase in Chinese hamster ovary cells via multiple mechanisms: the role of Ca2+ influx in OX1 receptor signaling. Mol. Endocrinol. 20, 80–99. doi: 10.1210/me.2004-0389
Ammoun, S., Lindholm, D., Wootz, H., Åkerman, K. E., and Kukkonen, J. P. (2006b). G-protein-coupled OX1 orexin/hcrtr-1 hypocretin receptors induce caspase-dependent and-independent cell death through p38 mitogen-/stress-activated protein kinase. J. Biol. Chem. 281, 834–842. doi: 10.1074/jbc.M508603200
Asahi, S., Egashira, S.-I., Matsuda, M., Iwaasa, H., Kanatani, A., Ohkubo, M., et al. (2003). Development of an orexin-2 receptor selective agonist, [Ala11, D-Leu15] orexin-B. Bioorg. Med. Chem. Lett. 13, 111–113. doi: 10.1016/s0960-894x(02)00851-x
Avet, C., Mancini, A., Breton, B., Le Gouill, C., Hauser, A. S., Normand, C., et al. (2020). Selectivity landscape of 100 therapeutically relevant GPCR profiled by an effector translocation-based BRET platform. bioRxiv [Preprint]. doi: 10.1101/2020.04.20.052027
Bai, B., Chen, X., Zhang, R., Wang, X., Jiang, Y., Li, D., et al. (2017). Dual-agonist occupancy of orexin receptor 1 and cholecystokinin A receptor heterodimers decreases G-protein–dependent signaling and migration in the human colon cancer cell line HT-29. Biochim. Biophys. Acta Mol. Cell Res. 1864, 1153–1164. doi: 10.1016/j.bbamcr.2017.03.003
Baker, J., and Proudman, R. (2021). Pharmacological analysis of orexin A, orexin B and two other orexin agonists for the human orexin-1 and orexin-2 receptors. Br. J. Pharmacol. 178, 4969–4970.
Bassetti, C. L., Adamantidis, A., Burdakov, D., Han, F., Gay, S., Kallweit, U., et al. (2019). Narcolepsy—clinical spectrum, aetiopathophysiology, diagnosis and treatment. Nat. Rev. Neurol. 15, 519–539. doi: 10.1038/s41582-019-0226-9
Berger, B., Brooks, S., Zuiker, R., Richard, M., Muehlan, C., and Dingemanse, J. (2020). Pharmacological interactions between the dual orexin receptor antagonist daridorexant and ethanol in a double-blind, randomized, placebo-controlled, double-dummy, four-way crossover phase i study in healthy subjects. CNS Drugs 34, 1253–1266. doi: 10.1007/s40263-020-00768-8
Betschart, C., Hintermann, S., Behnke, D., Cotesta, S., Fendt, M., Gee, C. E., et al. (2013). Identification of a novel series of orexin receptor antagonists with a distinct effect on sleep architecture for the treatment of insomnia. J. Med. Chem. 56, 7590–7607. doi: 10.1021/jm4007627
Bettica, P., Squassante, L., Zamuner, S., Nucci, G., Danker-Hopfe, H., and Ratti, E. (2012). The orexin antagonist SB-649868 promotes and maintains sleep in men with primary insomnia. Sleep 35, 1097–1104. doi: 10.5665/sleep.1996
Beuckmann, C. T., Suzuki, M., Ueno, T., Nagaoka, K., Arai, T., and Higashiyama, H. (2017). In vitro and in silico characterization of lemborexant (E2006), a novel dual orexin receptor antagonist. J. Pharmacol. Exp. Ther. 362, 287–295. doi: 10.1124/jpet.117.241422
Black, S. W., Morairty, S. R., Fisher, S. P., Chen, T.-M., Warrier, D. R., and Kilduff, T. S. (2013). Almorexant promotes sleep and exacerbates cataplexy in a murine model of narcolepsy. Sleep 36, 325–336. doi: 10.5665/sleep.2442
Bonaventure, P., Dugovic, C., Shireman, B., Preville, C., Yun, S., Lord, B., et al. (2017). Evaluation of JNJ-54717793 a novel brain penetrant selective orexin 1 receptor antagonist in two rat models of panic attack provocation. Front. Pharmacol. 8:357. doi: 10.3389/fphar.2017.00357
Bonaventure, P., Shelton, J., Yun, S., Nepomuceno, D., Sutton, S., Aluisio, L., et al. (2015). Characterization of JNJ-42847922, a selective orexin-2 receptor antagonist, as a clinical candidate for the treatment of insomnia. J. Pharmacol. Exp. Ther. 354, 471–482. doi: 10.1124/jpet.115.225466
Bonnavion, P., Jackson, A. C., Carter, M. E., and De Lecea, L. (2015). Antagonistic interplay between hypocretin and leptin in the lateral hypothalamus regulates stress responses. Nat. Commun. 6:6266. doi: 10.1038/ncomms7266
Boss, C., Gatfield, J., Brotschi, C., Heidmann, B., Sifferlen, T., von Raumer, M., et al. (2020). Front cover: the quest for the best dual orexin receptor antagonist (Daridorexant) for the treatment of insomnia disorders. ChemMedChem 15, 2286–2305. doi: 10.1002/cmdc.202000453
Brisbare-Roch, C., Dingemanse, J., Koberstein, R., Hoever, P., Aissaoui, H., Flores, S., et al. (2007). Promotion of sleep by targeting the orexin system in rats, dogs and humans. Nat. Med. 13, 150–155. doi: 10.1038/nm1544
Caillol, M., Aioun, J., Baly, C., Persuy, M.-A., and Salesse, R. (2003). Localization of orexins and their receptors in the rat olfactory system: possible modulation of olfactory perception by a neuropeptide synthetized centrally or locally. Brain Res. 960, 48–61. doi: 10.1016/s0006-8993(02)03755-1
Callander, G. E., Olorunda, M., Monna, D., Schuepbach, E., Langenegger, D., Betschart, C., et al. (2013). Kinetic properties of “dual” orexin receptor antagonists at OX1R and OX2R orexin receptors. Front. Neurosci. 7:230. doi: 10.3389/fnins.2013.00230
Chemelli, R. M., Willie, J. T., Sinton, C. M., Elmquist, J. K., Scammell, T., Lee, C., et al. (1999). Narcolepsy in orexin knockout mice: molecular genetics of sleep regulation. Cell 98, 437–451. doi: 10.1016/s0092-8674(00)81973-x
Chen, J., Zhang, R., Chen, X., Wang, C., Cai, X., Liu, H., et al. (2015). Heterodimerization of human orexin receptor 1 and kappa opioid receptor promotes protein kinase A/cAMP-response element binding protein signaling via a Gαs-mediated mechanism. Cell. Signal. 27, 1426–1438. doi: 10.1016/j.cellsig.2015.03.027
Clark, J. W., Brian, M., Drummond, S. P., Hoyer, D., and Jacobson, L. H. (2020). Effects of orexin receptor antagonism on human sleep architecture: a systematic review. Sleep Med. Rev. 53:101332. doi: 10.1016/j.smrv.2020.101332
Coleman, P., de Lecea, L., Gotter, A., Hagan, J., Hoyer, D., Kilduff, T., et al. (2021). Orexin receptors in GtoPdb v. IUPHAR/BPS Guide Pharmacol. CITE 2021. doi: 10.2218/gtopdb/F51/2021.3
Coleman, P. J., Schreier, J. D., Cox, C. D., Breslin, M. J., Whitman, D. B., Bogusky, M. J., et al. (2012). Discovery of [(2R, 5R)−5−{[(5−Fluoropyridin−2−yl) oxy] methyl}−2−methylpiperidin−1−yl][5−methyl−2−(pyrimidin−2−yl) phenyl] methanone (MK−6096): a dual orexin receptor antagonist with potent sleep−promoting properties. ChemMedChem 7, 415–424. doi: 10.1002/cmdc.201200025
Cox, C. D., Breslin, M. J., Whitman, D. B., Schreier, J. D., McGaughey, G. B., Bogusky, M. J., et al. (2010). Discovery of the dual orexin receptor antagonist [(7 R)-4-(5-chloro-1, 3-benzoxazol-2-yl)-7-methyl-1, 4-diazepan-1-yl][5-methyl-2-(2 H-1, 2, 3-triazol-2-yl) phenyl] methanone (MK-4305) for the treatment of insomnia. J. Med. Chem. 53, 5320–5332. doi: 10.1021/jm100541c
Cvetkovic-Lopes, V., Bayer, L., Dorsaz, S., Maret, S., Pradervand, S., Dauvilliers, Y., et al. (2010). Elevated Tribbles homolog 2–specific antibody levels in narcolepsy patients. J. Clin. Invest. 120, 713–719. doi: 10.1172/JCI41366
Dalrymple, M. B., Jaeger, W. C., Eidne, K. A., and Pfleger, K. D. (2011). Temporal profiling of orexin receptor-arrestin-ubiquitin complexes reveals differences between receptor subtypes. J. Biol. Chem. 286, 16726–16733. doi: 10.1074/jbc.M111.223537
Date, Y., Ueta, Y., Yamashita, H., Yamaguchi, H., Matsukura, S., Kangawa, K., et al. (1999). Orexins, orexigenic hypothalamic peptides, interact with autonomic, neuroendocrine and neuroregulatory systems. Proc. Natl. Acad. Sci. U.S.A. 96, 748–753. doi: 10.1073/pnas.96.2.748
Dauvilliers, Y., Zammit, G., Fietze, I., Mayleben, D., Seboek Kinter, D., Pain, S., et al. (2020). Daridorexant, a new dual orexin receptor antagonist to treat insomnia disorder. Ann. Neurol. 87, 347–356. doi: 10.1002/ana.25680
De Lecea, L., Kilduff, T., Peyron, C., Gao, X.-B., Foye, P., Danielson, P., et al. (1998). The hypocretins: hypothalamus-specific peptides with neuroexcitatory activity. Proc. Natl. Acad. Sci. U.S.A. 95, 322–327. doi: 10.1073/pnas.95.1.322
DeWire, S. M., Ahn, S., Lefkowitz, R. J., and Shenoy, S. K. (2007). Beta-arrestins and cell signaling. Annu. Rev. Physiol. 69, 483–510.
Diniz Behn, C. G., Klerman, E. B., Mochizuki, T., Lin, S. C., and Scammell, T. E. (2010). Abnormal sleep/wake dynamics in orexin knockout mice. Sleep 33, 297–306. doi: 10.1093/sleep/33.3.297
Dugovic, C., Shelton, J. E., Aluisio, L. E., Fraser, I. C., Jiang, X., Sutton, S. W., et al. (2009). Blockade of orexin-1 receptors attenuates orexin-2 receptor antagonism-induced sleep promotion in the rat. J. Pharmacol. Exp. Ther. 330, 142–151. doi: 10.1124/jpet.109.152009
Dugovic, C., Shelton, J. E., Yun, S., Bonaventure, P., Shireman, B. T., and Lovenberg, T. W. (2014). Orexin-1 receptor blockade dysregulates REM sleep in the presence of orexin-2 receptor antagonism. Front. Neurosci. 8:28. doi: 10.3389/fnins.2014.00028
Edwards, C., Abusnana, S., Sunter, D., Murphy, K., Ghatei, M., and Bloom, S. (1999). The effect of the orexins on food intake: comparison with neuropeptide Y, melanin-concentrating hormone and galanin. J. Endocrinol. 160, R7–12. doi: 10.1677/joe.0.160r007
Estabrooke, I. V., McCarthy, M. T., Ko, E., Chou, T. C., Chemelli, R. M., Yanagisawa, M., et al. (2001). Fos expression in orexin neurons varies with behavioral state. J. Neurosci. 21, 1656–1662. doi: 10.1523/JNEUROSCI.21-05-01656.2001
Farkas, R. (2013). Suvorexant Safety and Efficacy. Available online at: http://www.fda.gov/downloads/AdvisoryCommittees/CommitteesMeetingMaterials/Drugs/PeripheralandCentralNervousSystemDrugsAdvisoryCommittee/UCM354215.pdf (Accessed on May 08, 2013).
Fitch, T. E., Benvenga, M. J., Jesudason, C. D., Zink, C., Vandergriff, A. B., Menezes, M., et al. (2014). LSN2424100: a novel, potent orexin-2 receptor antagonist with selectivity over orexin-1 receptors and activity in an animal model predictive of antidepressant-like efficacy. Front. Neurosci. 8:5. doi: 10.3389/fnins.2014.00005
Gorojankina, T., Grébert, D., Salesse, R., Tanfin, Z., and Caillol, M. (2007). Study of orexins signal transduction pathways in rat olfactory mucosa and in olfactory sensory neurons-derived cell line Odora: multiple orexin signalling pathways. Regul. Pept. 141, 73–85. doi: 10.1016/j.regpep.2006.12.012
Halls, M. L., and Cooper, D. M. (2011). Regulation by Ca2+-signaling pathways of adenylyl cyclases. Cold Spring Harb. Perspect. Biol. 3:a004143. doi: 10.1101/cshperspect.a004143
Hara, J., Beuckmann, C. T., Nambu, T., Willie, J. T., Chemelli, R. M., Sinton, C. M., et al. (2001). Genetic ablation of orexin neurons in mice results in narcolepsy, hypophagia, and obesity. Neuron 30, 345–354. doi: 10.1016/s0896-6273(01)00293-8
Haynes, A. C., Jackson, B., Chapman, H., Tadayyon, M., Johns, A., Porter, R. A., et al. (2000). A selective orexin-1 receptor antagonist reduces food consumption in male and female rats. Regul. Pept. 96, 45–51. doi: 10.1016/s0167-0115(00)00199-3
Heinonen, M., Purhonen, A., Mäkelä, K., and Herzig, K. (2008). Functions of orexins in peripheral tissues. Acta Physiol. 192, 471–485. doi: 10.1111/j.1748-1716.2008.01836.x
Holmqvist, T., Åkerman, K. E., and Kukkonen, J. P. (2002). Orexin signaling in recombinant neuron−like cells. FEBS Lett. 526, 11–14. doi: 10.1016/s0014-5793(02)03101-0
Holmqvist, T., Johansson, L., Östman, M., Ammoun, S., Åkerman, K. E., and Kukkonen, J. P. (2005). OX1 orexin receptors couple to adenylyl cyclase regulation via multiple mechanisms. J. Biol. Chem. 280, 6570–6579. doi: 10.1074/jbc.M407397200
Honda, M., Arai, T., Fukazawa, M., Honda, Y., Tsuchiya, K., Salehi, A., et al. (2009). Absence of ubiquitinated inclusions in hypocretin neurons of patients with narcolepsy. Neurology 73, 511–517. doi: 10.1212/WNL.0b013e3181b2a6af
Houslay, M. D., and Kolch, W. (2000). Cell-type specific integration of cross-talk between extracellular signal-regulated kinase and cAMP signaling. Mol. Pharmacol. 58, 659–668. doi: 10.1124/mol.58.4.659
Hoyer, D., Allen, A., and Jacobson, L. H. (2020). Hypnotics with novel modes of action. Br. J. Clin. Pharmacol. 86, 244–249. doi: 10.1111/bcp.14180
Hoyer, D., Dürst, T., Fendt, M., Jacobson, L. H., Betschart, C., Hintermann, S., et al. (2013). Distinct effects of IPSU and suvorexant on mouse sleep architecture. Front. Neurosci. 7:235. doi: 10.3389/fnins.2013.00235
Hoyer, D., and Jacobson, L. H. (2013). Orexin in sleep, addiction and more: is the perfect insomnia drug at hand? Neuropeptides 47, 477–488. doi: 10.1016/j.npep.2013.10.009
Hungs, M., Fan, J., Lin, L., Lin, X., Maki, R. A., and Mignot, E. (2001). Identification and functional analysis of mutations in the hypocretin (orexin) genes of narcoleptic canines. Genome Res. 11, 531–539. doi: 10.1101/gr.gr-1610r
Irannejad, R., and von Zastrow, M. (2014). GPCR signaling along the endocytic pathway. Curr. Opin. Cell Biol. 27, 109–116. doi: 10.1016/j.ceb.2013.10.003
Irukayama-Tomobe, Y., Ogawa, Y., Tominaga, H., Ishikawa, Y., Hosokawa, N., Ambai, S., et al. (2017). Nonpeptide orexin type-2 receptor agonist ameliorates narcolepsy-cataplexy symptoms in mouse models. Proc. Natl. Acad. Sci. U.S.A. 114, 5731–5736. doi: 10.1073/pnas.1700499114
Jacobson, L., Brian, M., Cornthwaite-Duncan, L., Lin, I., Keenan, R., and Daykin, H. (2019). Delineating the influence of orexin 1 and 2 receptors on sleep architecture and aversive memory. J. Sleep Res. 28:27.
Jacobson, L. H., Callander, G. E., and Hoyer, D. (2014). Suvorexant for the treatment of insomnia. Expert Rev. Clin. Pharmacol. 7, 711–730.
Jacobson, L. H., Chen, S., Mir, S., and Hoyer, D. (2017). Orexin OX2 receptor antagonists as sleep aids. Curr. Top. Behav. Neurosci. 33, 105–136. doi: 10.1007/7854_2016_47
Jaeger, W., Armstrong, S., Hill, S., and Pfleger, K. D. (2014). Biophysical detection of diversity and bias in GPCR function. Front. Endocrinol. 5:26. doi: 10.3389/fendo.2014.00026
Jäntti, M., Putula, J., Somerharju, P., Frohman, M., and Kukkonen, J. (2012). OX1 orexin/hypocretin receptor activation of phospholipase D. Br. J. Pharmacol. 165, 1109–1123. doi: 10.1111/j.1476-5381.2011.01565.x
Jäntti, M. H., Mandrika, I., and Kukkonen, J. P. (2014). Human orexin/hypocretin receptors form constitutive homo-and heteromeric complexes with each other and with human CB1 cannabinoid receptors. Biochem. Biophys. Res. Commun. 445, 486–490. doi: 10.1016/j.bbrc.2014.02.026
Johansson, L., Ekholm, M. E., and Kukkonen, J. P. (2007). Regulation of OX1 orexin/hypocretin receptor−coupling to phospholipase C by Ca2+ influx. Br. J. Pharmacol. 150, 97–104. doi: 10.1038/sj.bjp.0706959
Johren, O., Neidert, S. J., Kummer, M., Dendorfer, A., and Dominiak, P. (2001). Prepro-orexin and orexin receptor mRNAs are differentially expressed in peripheral tissues of male and female rats. Endocrinology 142, 3324–3331. doi: 10.1210/endo.142.8.8299
Kanbayashi, T., Inoue, Y., Chiba, S., Aizawa, R., Saito, Y., Tsukamoto, H., et al. (2002). CSF hypocretin−1 (orexin−A) concentrations in narcolepsy with and without cataplexy and idiopathic hypersomnia. J. Sleep Res. 11, 91–93. doi: 10.1046/j.1365-2869.2002.00284.x
Karteris, E., Chen, J., and Randeva, H. S. (2004). Expression of human prepro-orexin and signaling characteristics of orexin receptors in the male reproductive system. J. Clin. Endocrinol. Metab. 89, 1957–1962. doi: 10.1210/jc.2003-031778
Karteris, E., Machado, R. J., Chen, J., Zervou, S., Hillhouse, E. W., and Randeva, H. S. (2005). Food deprivation differentially modulates orexin receptor expression and signaling in rat hypothalamus and adrenal cortex. Am. J. Physiol. Endocrinol. Metab. 288, E1089–E1100. doi: 10.1152/ajpendo.00351.2004
Karteris, E., Randeva, H., Grammatopoulos, D., Jaffe, R., and Hillhouse, E. (2001). Expression and coupling characteristics of the CRH and orexin type 2 receptors in human fetal adrenals. J. Clin. Endocrinol. Metab. 86, 4512–4519. doi: 10.1210/jcem.86.9.7849
Kaufmann, P., Ort, M., Golor, G., Kornberger, R., and Dingemanse, J. (2020). First−in−human study with ACT−539313, a novel selective orexin−1 receptor antagonist. Br. J. Clin. Pharmacol. 86, 1377–1386. doi: 10.1111/bcp.14251
Kirchgessner, A. L., and Liu, M.-T. (1999). Orexin synthesis and response in the gut. Neuron 24, 941–951. doi: 10.1016/s0896-6273(00)81041-7
Kocan, M., Dalrymple, M., Seeber, R., Feldman, B., and Pfleger, K. (2011). Enhanced BRET technology for the monitoring of agonist-induced and agonist-independent interactions between GPCRs and β-arrestins. Front. Endocrinol. 1:12. doi: 10.3389/fendo.2010.00012
Kohlmeier, K. A., Inoue, T., and Leonard, C. S. (2004). Hypocretin/orexin peptide signaling in the ascending arousal system: elevation of intracellular calcium in the mouse dorsal raphe and laterodorsal tegmentum. J. Neurophysiol. 92, 221–235. doi: 10.1152/jn.00076.2004
Kohlmeier, K. A., Watanabe, S., Tyler, C. J., Burlet, S., and Leonard, C. S. (2008). Dual orexin actions on dorsal raphe and laterodorsal tegmentum neurons: noisy cation current activation and selective enhancement of Ca2+ transients mediated by L-type calcium channels. J. Neurophysiol. 100, 2265–2281. doi: 10.1152/jn.01388.2007
Kukkonen, J. P. (2004). Regulation of receptor-coupling to (multiple) G proteins. a challenge for basic research and drug discovery. Recept. Channels 10, 167–183. doi: 10.3109/10606820490926151
Kukkonen, J. P. (2013). Physiology of the orexinergic/hypocretinergic system: a revisit in 2012. Am. J. Physiol. Cell Physiol. 304, C2–C32. doi: 10.1152/ajpcell.00227.2012
Kukkonen, J. P. (2016a). G-protein-dependency of orexin/hypocretin receptor signalling in recombinant Chinese hamster ovary cells. Biochem. Biophys. Res. Commun. 476, 379–385. doi: 10.1016/j.bbrc.2016.05.130
Kukkonen, J. P. (2016b). OX2 orexin/hypocretin receptor signal transduction in recombinant Chinese hamster ovary cells. Cell. Signal. 28, 51–60. doi: 10.1016/j.cellsig.2015.11.009
Kukkonen, J. P., and Leonard, C. (2014). Orexin/hypocretin receptor signalling cascades. Br. J. Pharmacol. 171, 314–331. doi: 10.1111/bph.12324
Larsson, K. P., Peltonen, H. M., Bart, G., Louhivuori, L. M., Penttonen, A., Antikainen, M., et al. (2005). Orexin-A-induced Ca2+ entry evidence for involvement of trpc channels and protein kinase C regulation. J. Biol. Chem. 280, 1771–1781. doi: 10.1074/jbc.M406073200
Laschet, C., Dupuis, N., and Hanson, J. (2019). A dynamic and screening-compatible nanoluciferase-based complementation assay enables profiling of individual GPCR–G protein interactions. J. Biol. Chem. 294, 4079–4090. doi: 10.1074/jbc.RA118.006231
Latorre, D., Kallweit, U., Armentani, E., Foglierini, M., Mele, F., Cassotta, A., et al. (2018). T cells in patients with narcolepsy target self-antigens of hypocretin neurons. Nature 562, 63–68. doi: 10.1038/s41586-018-0540-1
Lecendreux, M., Churlaud, G., Pitoiset, F., Regnault, A., Tran, T. A., Liblau, R., et al. (2017). Narcolepsy type 1 is associated with a systemic increase and activation of regulatory T cells and with a systemic activation of global T cells. PLoS One 12:e0169836. doi: 10.1371/journal.pone.0169836
Lefkowitz, R. J., and Shenoy, S. K. (2005). Transduction of receptor signals by ß-arrestins. Science 308, 512–517. doi: 10.1126/science.1109237
Leino, T. O., Turku, A., Yli-Kauhaluoma, J., Kukkonen, J. P., Xhaard, H., and Wallén, E. A. (2018). Azulene-based compounds for targeting orexin receptors. Eur. J. Med. Chem. 157, 88–100. doi: 10.1016/j.ejmech.2018.07.040
Leonard, C., and Kukkonen, J. P. (2014). Orexin/hypocretin receptor signalling: a functional perspective. Br. J. Pharmacol. 171, 294–313. doi: 10.1111/bph.12296
Li, J., Hu, Z., and de Lecea, L. (2014). The hypocretins/orexins: integrators of multiple physiological functions. Br. J. Pharmacol. 171, 332–350. doi: 10.1111/bph.12415
Li, S.-B., and de Lecea, L. (2020). The hypocretin (orexin) system: from a neural circuitry perspective. Neuropharmacology 167:107993. doi: 10.1016/j.neuropharm.2020.107993
Liblau, R. S., Vassalli, A., Seifinejad, A., and Tafti, M. (2015). Hypocretin (orexin) biology and the pathophysiology of narcolepsy with cataplexy. Lancet Neurol. 14, 318–328. doi: 10.1016/S1474-4422(14)70218-2
Lin, L., Faraco, J., Li, R., Kadotani, H., Rogers, W., Lin, X., et al. (1999). The sleep disorder canine narcolepsy is caused by a mutation in the hypocretin (orexin) receptor 2 gene. Cell 98, 365–376. doi: 10.1016/s0092-8674(00)81965-0
Lippert, J., Young, P., Gross, C., Meuth, S. G., Dräger, B., Schirmacher, A., et al. (2019). Specific T-cell activation in peripheral blood and cerebrospinal fluid in central disorders of hypersomnolence. Sleep 42:zsy223. doi: 10.1093/sleep/zsy223
Lopez, M., Senaris, R., Gallego, R., Garcia-Caballero, T., Lago, F., Seoane, L., et al. (1999). Orexin receptors are expressed in the adrenal medulla of the rat. Endocrinology 140, 5991–5994. doi: 10.1210/endo.140.12.7287
Lund, P.-E., Shariatmadari, R., Uustare, A., Detheux, M., Parmentier, M., Kukkonen, J. P., et al. (2000). The orexin OX1 receptor activates a novel Ca2+ influx pathway necessary for coupling to phospholipase C. J. Biol. Chem. 275, 30806–30812. doi: 10.1074/jbc.M002603200
Luo, G., Ambati, A., Lin, L., Bonvalet, M., Partinen, M., Ji, X., et al. (2018). Autoimmunity to hypocretin and molecular mimicry to flu in type 1 narcolepsy. Proc. Natl. Acad. Sci. U.S.A. 115, E12323–E12332. doi: 10.1073/pnas.1818150116
Maehara, S., Yuge, N., Higashi, C., Ota, T., Furukawa, J., and Takeuchi, T. (2020). Pharmacological characterization of a novel potent, selective, and orally active orexin 2 receptor antagonist. SDM−878. Neuropsychopharmacol. Rep. 40, 182–189. doi: 10.1002/npr2.12105
Magga, J., Bart, G., Oker-Blom, C., Kukkonen, J. P., Åkerman, K. E., and Näsman, J. (2006). Agonist potency differentiates G protein activation and Ca2+ signalling by the orexin receptor type 1. Biochem. Pharmacol. 71, 827–836. doi: 10.1016/j.bcp.2005.12.021
Mahoney, C. E., Cogswell, A., Koralnik, I. J., and Scammell, T. E. (2019). The neurobiological basis of narcolepsy. Nat. Rev. Neurosci. 20, 83–93. doi: 10.1038/s41583-018-0097-x
Mahoney, C. E., Mochizuki, T., and Scammell, T. E. (2020). Dual orexin receptor antagonists increase sleep and cataplexy in wild type mice. Sleep 43:zsz302. doi: 10.1093/sleep/zsz302
Malendowicz, L. K., Tortorella, C., and Nussdorfer, G. G. (1999). Orexins stimulate corticosterone secretion of rat adrenocortical cells, through the activation of the adenylate cyclase-dependent signaling cascade. J. Steroid Biochem. Mol. Biol. 70, 185–188. doi: 10.1016/s0960-0760(99)00110-7
Mang, G. M., Dürst, T., Bürki, H., Imobersteg, S., Abramowski, D., Schuepbach, E., et al. (2012). The dual orexin receptor antagonist almorexant induces sleep and decreases orexin-induced locomotion by blocking orexin 2 receptors. Sleep 35, 1625–1635. doi: 10.5665/sleep.2232
Marcus, J. N., Aschkenasi, C. J., Lee, C. E., Chemelli, R. M., Saper, C. B., Yanagisawa, M., et al. (2001). Differential expression of orexin receptors 1 and 2 in the rat brain. J. Comp. Neurol. 435, 6–25. doi: 10.1002/cne.1190
Mazzocchi, G., Malendowicz, L., Gottardo, L., Aragona, F., and Nussdorfer, G. (2001). Orexin A stimulates cortisol secretion from human adrenocortical cells through activation of the adenylate cyclase-dependent signaling cascade. J. Clin. Endocrinol. Metab. 86, 778–782. doi: 10.1210/jcem.86.2.7233
Medrano, M., Aguinaga, D., Reyes-Resina, I., Canela, E. I., Mallol, J., Navarro, G., et al. (2018). Orexin A/Hypocretin modulates leptin receptor-mediated signaling by allosteric modulations mediated by the ghrelin ghs-r1a receptor in hypothalamic neurons. Mol. Neurobiol. 55, 4718–4730. doi: 10.1007/s12035-017-0670-8
Mignot, E., Lammers, G. J., Ripley, B., Okun, M., Nevsimalova, S., Overeem, S., et al. (2002). The role of cerebrospinal fluid hypocretin measurement in the diagnosis of narcolepsy and other hypersomnias. Arch. Neurol. 59, 1553–1562. doi: 10.1001/archneur.59.10.1553
Milasta, S., Evans, N. A., Ormiston, L., Wilson, S., Lefkowitz, R. J., and Milligan, G. (2005). The sustainability of interactions between the orexin-1 receptor and β-arrestin-2 is defined by a single C-terminal cluster of hydroxy amino acids and modulates the kinetics of ERK MAPK regulation. Biochem. J. 387, 573–584. doi: 10.1042/BJ20041745
Muehlan, C., Boehler, M., Brooks, S., Zuiker, R., van Gerven, J., and Dingemanse, J. (2020a). Clinical pharmacology of the dual orexin receptor antagonist ACT-541468 in elderly subjects: exploration of pharmacokinetics, pharmacodynamics and tolerability following single-dose morning and repeated-dose evening administration. J. Psychopharmacol. 34, 326–335. doi: 10.1177/0269881119882854
Muehlan, C., Zuiker, R., Peeters, P., Rowles, R., and Dingemanse, J. (2020b). Pharmacokinetics and pharmacodynamics of the dual orexin receptor antagonist daridorexant in Japanese and Caucasian subjects. J. Clin. Psychopharmacol. 40, 157–166. doi: 10.1097/JCP.0000000000001182
Muroya, S., Funahashi, H., Yamanaka, A., Kohno, D., Uramura, K., Nambu, T., et al. (2004). Orexins (hypocretins) directly interact with neuropeptide Y, POMC and glucose−responsive neurons to regulate Ca2+ signaling in a reciprocal manner to leptin: orexigenic neuronal pathways in the mediobasal hypothalamus. Eur. J. Neurosci. 19, 1524–1534. doi: 10.1111/j.1460-9568.2004.03255.x
Murrell, J. R., and Hunter, D. D. (1999). An olfactory sensory neuron line, odora, properly targets olfactory proteins and responds to odorants. J. Neurosci. 19, 8260–8270. doi: 10.1523/JNEUROSCI.19-19-08260.1999
Nagahara, T., Saitoh, T., Kutsumura, N., Irukayama-Tomobe, Y., Ogawa, Y., Kuroda, D., et al. (2015). Design and synthesis of non-peptide, selective orexin receptor 2 agonists. J. Med. Chem. 58, 7931–7937. doi: 10.1021/acs.jmedchem.5b00988
Nagase, H., Yamamoto, N., Yata, M., Ohrui, S., Okada, T., Saitoh, T., et al. (2017). Design and synthesis of potent and highly selective orexin 1 receptor antagonists with a morphinan skeleton and their pharmacologies. J. Med. Chem. 60, 1018–1040. doi: 10.1021/acs.jmedchem.6b01418
Nakamura, T., Uramura, K., Nambu, T., Yada, T., Goto, K., Yanagisawa, M., et al. (2000). Orexin-induced hyperlocomotion and stereotypy are mediated by the dopaminergic system. Brain Res. 873, 181–187. doi: 10.1016/s0006-8993(00)02555-5
Nambu, T., Sakurai, T., Mizukami, K., Hosoya, Y., Yanagisawa, M., and Goto, K. (1999). Distribution of orexin neurons in the adult rat brain. Brain Res. 827, 243–260. doi: 10.1016/s0006-8993(99)01336-0
Navarro, G., Medrano, M., Aguinaga, D., Vega-Quiroga, I., Lillo, A., Jiménez, J., et al. (2019). Differential effect of amphetamine over the corticotropin-releasing factor CRF2 receptor, the orexin OX1 receptor and the CRF2-OX1 heteroreceptor complex. Neuropharmacology 152, 102–111. doi: 10.1016/j.neuropharm.2018.11.014
Navarro, G., Quiroz, C., Moreno-Delgado, D., Sierakowiak, A., McDowell, K., Moreno, E., et al. (2015). Orexin–corticotropin-releasing factor receptor heteromers in the ventral tegmental area as targets for cocaine. J. Neurosci. 35, 6639–6653. doi: 10.1523/JNEUROSCI.4364-14.2015
Nishino, S., Ripley, B., Overeem, S., Lammers, G. J., and Mignot, E. (2000). Hypocretin (orexin) deficiency in human narcolepsy. Lancet 355, 39–40. doi: 10.1016/s0140-6736(99)05582-8
Nishino, S., Ripley, B., Overeem, S., Nevsimalova, S., Lammers, G. J., Vankova, J., et al. (2001). Low cerebrospinal fluid hypocretin (Orexin) and altered energy homeostasis in human narcolepsy. Ann. Neurol. 50, 381–388. doi: 10.1002/ana.1130
Olsen, R. H., DiBerto, J. F., English, J. G., Glaudin, A. M., Krumm, B. E., Slocum, S. T., et al. (2020). TRUPATH, an open-source biosensor platform for interrogating the GPCR transducerome. Nat. Chem. Biol. 16; 841–849. doi: 10.1038/s41589-020-0535-8
Patel, V. H., Karteris, E., Chen, J., Kyrou, I., Mattu, H. S., Dimitriadis, G. K., et al. (2018). Functional cardiac orexin receptors: role of orexin-B/orexin 2 receptor in myocardial protection. Clin. Sci. 132, 2547–2564. doi: 10.1042/CS20180150
Pedersen, N. W., Holm, A., Kristensen, N. P., Bjerregaard, A.-M., Bentzen, A. K., Marquard, A. M., et al. (2019). CD8+ T cells from patients with narcolepsy and healthy controls recognize hypocretin neuron-specific antigens. Nat. Commun. 10:837. doi: 10.1038/s41467-019-08774-1
Peltonen, H. M., Magga, J. M., Bart, G., Turunen, P. M., Antikainen, M. S., Kukkonen, J. P., et al. (2009). Involvement of TRPC3 channels in calcium oscillations mediated by OX1 orexin receptors. Biochem. Biophys. Res. Commun. 385, 408–412. doi: 10.1016/j.bbrc.2009.05.077
Peyron, C., Faraco, J., Rogers, W., Ripley, B., Overeem, S., Charnay, Y., et al. (2000). A mutation in a case of early onset narcolepsy and a generalized absence of hypocretin peptides in human narcoleptic brains. Nat. Med. 6, 991–997. doi: 10.1038/79690
Peyron, C., Tighe, D. K., Van Den Pol, A. N., De Lecea, L., Heller, H. C., Sutcliffe, J. G., et al. (1998). Neurons containing hypocretin (orexin) project to multiple neuronal systems. J. Neurosci. 18, 9996–10015. doi: 10.1523/jneurosci.18-23-09996.1998
Piccoli, L., Di Bonaventura, M. V. M., Cifani, C., Costantini, V. J., Massagrande, M., Montanari, D., et al. (2012). Role of orexin-1 receptor mechanisms on compulsive food consumption in a model of binge eating in female rats. Neuropsychopharmacology 37, 1999–2011. doi: 10.1038/npp.2012.48
Putula, J., Pihlajamaa, T., and Kukkonen, J. P. (2014). Calcium affects OX 1 orexin (hypocretin) receptor responses by modifying both orexin binding and the signal transduction machinery. Br. J. Pharmacol. 171, 5816–5828. doi: 10.1111/bph.12883
Ramanjaneya, M., Conner, A. C., Chen, J., Kumar, P., Brown, J. E., Jöhren, O., et al. (2009). Orexin-stimulated MAP kinase cascades are activated through multiple G-protein signalling pathways in human H295R adrenocortical cells: diverse roles for orexins A and B. J. Endocrinol. 202, 249–261. doi: 10.1677/JOE-08-0536
Ramanjaneya, M., Conner, A. C., Chen, J., Stanfield, P. R., and Randeva, H. S. (2008). Orexins stimulate steroidogenic acute regulatory protein expression through multiple signaling pathways in human adrenal H295R cells. Endocrinology 149, 4106–4115. doi: 10.1210/en.2007-1739
Ramberger, M., Högl, B., Stefani, A., Mitterling, T., Reindl, M., and Lutterotti, A. (2017). CD4+ T-cell reactivity to orexin/hypocretin in patients with narcolepsy type 1. Sleep 40:zsw070. doi: 10.1093/sleep/zsw070
Randeva, H., Karteris, E., Grammatopoulos, D., and Hillhouse, E. (2001). Expression of orexin-A and functional orexin type 2 receptors in the human adult adrenals: implications for adrenal function and energy homeostasis. J. Clin. Endocrinol. Metab. 86, 4808–4813. doi: 10.1210/jcem.86.10.7921
Rinne, M. K., Leino, T. O., Turku, A., Turunen, P. M., Steynen, Y., Xhaard, H., et al. (2018). Pharmacological characterization of the orexin/hypocretin receptor agonist Nag 26. Eur. J. Pharmacol. 837, 137–144. doi: 10.1016/j.ejphar.2018.09.003
Ripley, B., Overeem, S., Fujiki, N., Nevsimalova, S., Uchino, M., Yesavage, J., et al. (2001). CSF hypocretin/orexin levels in narcolepsy and other neurological conditions. Neurology 57, 2253–2258. doi: 10.1212/wnl.57.12.2253
Robinson, J. D., and McDonald, P. H. (2015). The orexin 1 receptor modulates kappa opioid receptor function via a JNK-dependent mechanism. Cell. Signal. 27, 1449–1456. doi: 10.1016/j.cellsig.2015.03.026
Roh, J. H., Jiang, H., Finn, M. B., Stewart, F. R., Mahan, T. E., Cirrito, J. R., et al. (2014). Potential role of orexin and sleep modulation in the pathogenesis of Alzheimer’s disease. J. Exp. Med. 211, 2487–2496. doi: 10.1084/jem.20141788
Sakurai, T. (2007). The neural circuit of orexin (hypocretin): maintaining sleep and wakefulness. Nat. Rev. Neurosci. 8, 171–181. doi: 10.1038/nrn2092
Sakurai, T. (2014). The role of orexin in motivated behaviours. Nat. Rev. Neurosci. 15, 719–731. doi: 10.1038/nrn3837
Sakurai, T., Amemiya, A., Ishii, M., Matsuzaki, I., Chemelli, R. M., Tanaka, H., et al. (1998). Orexins and orexin receptors: a family of hypothalamic neuropeptides and G protein-coupled receptors that regulate feeding behavior. Cell 92, 573–585. doi: 10.1016/s0092-8674(00)80949-6
Sakurai, T., and Mieda, M. (2011). Connectomics of orexin-producing neurons: interface of systems of emotion, energy homeostasis and arousal. Trends Pharmacol. Sci. 32, 451–462. doi: 10.1016/j.tips.2011.03.007
Saper, C. B., Chou, T. C., and Scammell, T. E. (2001). The sleep switch: hypothalamic control of sleep and wakefulness. Trends Neurosci. 24, 726–731. doi: 10.1016/s0166-2236(00)02002-6
Scammell, T. E., Estabrooke, I. V., McCarthy, M. T., Chemelli, R. M., Yanagisawa, M., Miller, M. S., et al. (2000). Hypothalamic arousal regions are activated during modafinil-induced wakefulness. J. Neurosci. 20, 8620–8628. doi: 10.1523/JNEUROSCI.20-22-08620.2000
Smart, D., Jerman, J., Brough, S., Rushton, S., Murdock, P., Jewitt, F., et al. (1999). Characterization of recombinant human orexin receptor pharmacology in a Chinese hamster ovary cell−line using FLIPR. Br. J. Pharmacol. 128, 1–3. doi: 10.1038/sj.bjp.0702780
Smith, J. S., Lefkowitz, R. J., and Rajagopal, S. (2018). Biased signalling: from simple switches to allosteric microprocessors. Nat. Rev. Drug Discov. 17, 243–260. doi: 10.1038/nrd.2017.229
Smith, J. S., and Rajagopal, S. (2016). The β-arrestins: multifunctional regulators of G protein-coupled receptors. J. Biol. Chem. 291, 8969–8977. doi: 10.1074/jbc.r115.713313
Soya, S., Takahashi, T. M., McHugh, T. J., Maejima, T., Herlitze, S., Abe, M., et al. (2017). Orexin modulates behavioral fear expression through the locus coeruleus. Nat. Commun. 8:1606. doi: 10.1038/s41467-017-01782-z
Spinazzi, R., Rucinski, M., Neri, G., Malendowicz, L., and Nussdorfer, G. (2005). Preproorexin and orexin receptors are expressed in cortisol-secreting adrenocortical adenomas, and orexins stimulate in vitro cortisol secretion and growth of tumor cells. J. Clin. Endocrinol. Metab. 90, 3544–3549. doi: 10.1210/jc.2004-2385
Steiner, N., Rossetti, C., Sakurai, T., Yanagisawa, M., de Lecea, L., Magistretti, P. J., et al. (2018). Hypocretin/orexin deficiency decreases cocaine abuse liability. Neuropharmacology 133, 395–403. doi: 10.1016/j.neuropharm.2018.02.010
Tang, J., Chen, J., Ramanjaneya, M., Punn, A., Conner, A. C., and Randeva, H. S. (2008). The signalling profile of recombinant human orexin-2 receptor. Cell. Signal. 20, 1651–1661. doi: 10.1016/j.cellsig.2008.05.010
Thannickal, T. C., Moore, R. Y., Nienhuis, R., Ramanathan, L., Gulyani, S., Aldrich, M., et al. (2000). Reduced number of hypocretin neurons in human narcolepsy. Neuron 27, 469–474. doi: 10.1016/s0896-6273(00)00058-1
Thannickal, T. C., Nienhuis, R., and Siegel, J. M. (2009). Localized loss of hypocretin (orexin) cells in narcolepsy without cataplexy. Sleep 32, 993–998. doi: 10.1093/sleep/32.8.993
Thompson, M. D., Sakurai, T., Rainero, I., Maj, M. C., and Kukkonen, J. P. (2017). Orexin receptor multimerization versus functional interactions: neuropharmacological implications for opioid and cannabinoid signalling and pharmacogenetics. Pharmaceuticals 10:79. doi: 10.3390/ph10040079
Thomsen, A. R., Jensen, D. D., Hicks, G. A., and Bunnett, N. W. (2018). Therapeutic targeting of endosomal G-protein-coupled receptors. Trends Pharmacol. Sci. 39, 879–891. doi: 10.1016/j.tips.2018.08.003
Toyama, S., Shimoyama, N., Tagaito, Y., Nagase, H., Saitoh, T., Yanagisawa, M., et al. (2018). Nonpeptide orexin-2 receptor agonist attenuates morphine-induced sedative effects in rats. Anesthesiology 128, 992–1003. doi: 10.1097/ALN.0000000000002161
Treiber, A., de Kanter, R., Roch, C., Gatfield, J., Boss, C., von Raumer, M., et al. (2017). The use of physiology-based pharmacokinetic and pharmacodynamic modeling in the discovery of the dual orexin receptor antagonist ACT-541468. J. Pharmacol. Exp. Ther. 362, 489–503. doi: 10.1124/jpet.117.241596
Tsujino, N., and Sakurai, T. (2009). Orexin/hypocretin: a neuropeptide at the interface of sleep, energy homeostasis, and reward system. Pharmacol. Rev. 61, 162–176. doi: 10.1124/pr.109.001321
Tsujino, N., and Sakurai, T. (2013). Role of orexin in modulating arousal, feeding, and motivation. Front. Behav. Neurosci. 7:28. doi: 10.3389/fnbeh.2013.00028
Turku, A., Borrel, A., Leino, T. O., Karhu, L., Kukkonen, J. P., and Xhaard, H. (2016). Pharmacophore model to discover OX1 and OX2 orexin receptor ligands. J. Med. Chem. 59, 8263–8275. doi: 10.1021/acs.jmedchem.6b00333
Turku, A., Leino, T. O., Karhu, L., Yli-Kauhaluoma, J., Kukkonen, J. P., Wallén, E. A., et al. (2019). Structure–Activity Relationships of 1−Benzoylazulenes at the OX1 and OX2 Orexin Receptors. ChemMedChem 14, 965–981. doi: 10.1002/cmdc.201900074
Turku, A., Rinne, M. K., Boije af Gennäs, G., Xhaard, H., Lindholm, D., and Kukkonen, J. P. (2017). Orexin receptor agonist Yan 7874 is a weak agonist of orexin/hypocretin receptors and shows orexin receptor-independent cytotoxicity. PLoS One 12:e0178526. doi: 10.1371/journal.pone.0178526
Turunen, P. M., Jäntti, M. H., and Kukkonen, J. P. (2012). OX1 orexin/hypocretin receptor signaling through arachidonic acid and endocannabinoid release. Mol. Pharmacol. 82, 156–167. doi: 10.1124/mol.112.078063
Uramura, K., Funahashi, H., Muroya, S., Shioda, S., Takigawa, M., and Yada, T. (2001). Orexin-a activates phospholipase C-and protein kinase C-mediated Ca2+ signaling in dopamine neurons of the ventral tegmental area. Neuroreport 12, 1885–1889. doi: 10.1097/00001756-200107030-00024
Urbańska, A., Sokołowska, P., Woldan-Tambor, A., Biegańska, K., Brix, B., Jöhren, O., et al. (2012). Orexins/hypocretins acting at G i protein-coupled OX 2 receptors inhibit cyclic AMP synthesis in the primary neuronal cultures. J. Mol. Neurosci. 46, 10–17. doi: 10.1007/s12031-011-9526-2
Van Den Pol, A. N., Gao, X.-B., Obrietan, K., Kilduff, T. S., and Belousov, A. B. (1998). Presynaptic and postsynaptic actions and modulation of neuroendocrine neurons by a new hypothalamic peptide, hypocretin/orexin. J. Neurosci. 18, 7962–7971. doi: 10.1523/JNEUROSCI.18-19-07962.1998
Wan, Q., Okashah, N., Inoue, A., Nehmé, R., Carpenter, B., Tate, C. G., et al. (2018). Mini G protein probes for active G protein-coupled receptors (GPCRs) in live cells. J. Biol. Chem. 293, 7466–7473. doi: 10.1074/jbc.RA118.001975
Wang, Q.-Q., Wang, C.-M., Cheng, B.-H., Yang, C.-Q., Bai, B., and Chen, J. (2019). Signaling transduction regulated by 5-hydroxytryptamine 1A receptor and orexin receptor 2 heterodimers. Cell. Signal. 54, 46–58. doi: 10.1016/j.cellsig.2018.11.014
Wang, Z., Liu, S., Kakizaki, M., Hirose, Y., Ishikawa, Y., Funato, H., et al. (2014). Orexin/hypocretin activates mTOR complex 1 (mTORC1) via an Erk/Akt-independent and calcium-stimulated lysosome v-ATPase pathway. J. Biol. Chem. 289, 31950–31959. doi: 10.1074/jbc.M114.600015
Ward, R. J., Pediani, J. D., and Milligan, G. (2011). Heteromultimerization of cannabinoid CB1 receptor and orexin OX1 receptor generates a unique complex in which both protomers are regulated by orexin A. J. Biol. Chem. 286, 37414–37428. doi: 10.1074/jbc.M111.287649
Willie, J. T., Chemelli, R. M., Sinton, C. M., Tokita, S., Williams, S. C., Kisanuki, Y. Y., et al. (2003). Distinct narcolepsy syndromes in Orexin receptor-2 and Orexin null mice: molecular genetic dissection of Non-REM and REM sleep regulatory processes. Neuron 38, 715–730. doi: 10.1016/s0896-6273(03)00330-1
Winrow, C. J., Gotter, A. L., Cox, C. D., Tannenbaum, P. L., Garson, S. L., Doran, S. M., et al. (2012). Pharmacological characterization of MK-6096–a dual orexin receptor antagonist for insomnia. Neuropharmacology 62, 978–987. doi: 10.1016/j.neuropharm.2011.10.003
Woldan-Tambor, A., Biegańska, K., Wiktorowska-Owczarek, A., and Zawilska, J. B. (2011). Activation of orexin/hypocretin type 1 receptors stimulates cAMP synthesis in primary cultures of rat astrocytes. Pharmacol. Rep. 63, 717–723. doi: 10.1016/s1734-1140(11)70583-7
Yamanaka, A., Kunii, K., Nambu, T., Tsujino, N., Sakai, A., Matsuzaki, I., et al. (2000). Orexin-induced food intake involves neuropeptide Y pathway. Brain Res. 859, 404–409. doi: 10.1016/s0006-8993(00)02043-6
Yoshida, Y., Naoe, Y., Terauchi, T., Ozaki, F., Doko, T., Takemura, A., et al. (2015). Discovery of (1 R, 2 S)-2-{[(2, 4-Dimethylpyrimidin-5-yl) oxy] methyl}-2-(3-fluorophenyl)-N-(5-fluoropyridin-2-yl) cyclopropanecarboxamide (E2006): a potent and efficacious oral orexin receptor antagonist. J. Med. Chem. 58, 4648–4664. doi: 10.1021/acs.jmedchem.5b00217
Yukitake, H., Fujimoto, T., Ishikawa, T., Suzuki, A., Shimizu, Y., Rikimaru, K., et al. (2019). TAK-925, an orexin 2 receptor-selective agonist, shows robust wake-promoting effects in mice. Pharmacol. Biochem. Behav. 187:172794. doi: 10.1016/j.pbb.2019.172794
Zammit, G., Seboek Kinter, D., Bassetti, C., Leger, D., Hermann, V., Pain, S., et al. (2020b). 0521 Daridorexant (ACT-541468), a dual orexin receptor antagonist for the treatment of insomnia disorder: double blind, randomized, phase 3 studies for efficacy and safety in adult and elderly patients. Sleep 43, A199–A200.
Zammit, G., Dauvilliers, Y., Pain, S., Kinter, D. S., Mansour, Y., and Kunz, D. (2020a). Daridorexant, a new dual orexin receptor antagonist, in elderly subjects with insomnia disorder. Neurology 94, e2222–e2232. doi: 10.1212/WNL.0000000000009475
Zhu, Y., Miwa, Y., Yamanaka, A., Yada, T., Shibahara, M., Abe, Y., et al. (2003). Orexin receptor type-1 couples exclusively to pertussis toxin-insensitive G-proteins, while orexin receptor type-2 couples to both pertussis toxin-sensitive and-insensitive G-proteins. J. Pharmacol. Sci. 92, 259–266. doi: 10.1254/jphs.92.259
Keywords: orexin, orexin 1 receptor, orexin 2 receptor, signaling, G protein, GPCR (G protein coupled receptor), arrestin
Citation: Dale NC, Hoyer D, Jacobson LH, Pfleger KDG and Johnstone EKM (2022) Orexin Signaling: A Complex, Multifaceted Process. Front. Cell. Neurosci. 16:812359. doi: 10.3389/fncel.2022.812359
Received: 10 November 2021; Accepted: 07 March 2022;
Published: 13 April 2022.
Edited by:
Terence Hébert, McGill University, CanadaReviewed by:
Karen J. Gregory, Monash University, AustraliaJuliana Dallagnol, McGill University, Canada
Copyright © 2022 Dale, Hoyer, Jacobson, Pfleger and Johnstone. This is an open-access article distributed under the terms of the Creative Commons Attribution License (CC BY). The use, distribution or reproduction in other forums is permitted, provided the original author(s) and the copyright owner(s) are credited and that the original publication in this journal is cited, in accordance with accepted academic practice. No use, distribution or reproduction is permitted which does not comply with these terms.
*Correspondence: Kevin D. G. Pfleger, a2V2aW4ucGZsZWdlckB1d2EuZWR1LmF1; Elizabeth K. M. Johnstone, bGl6LmpvaG5zdG9uZUB1d2EuZWR1LmF1