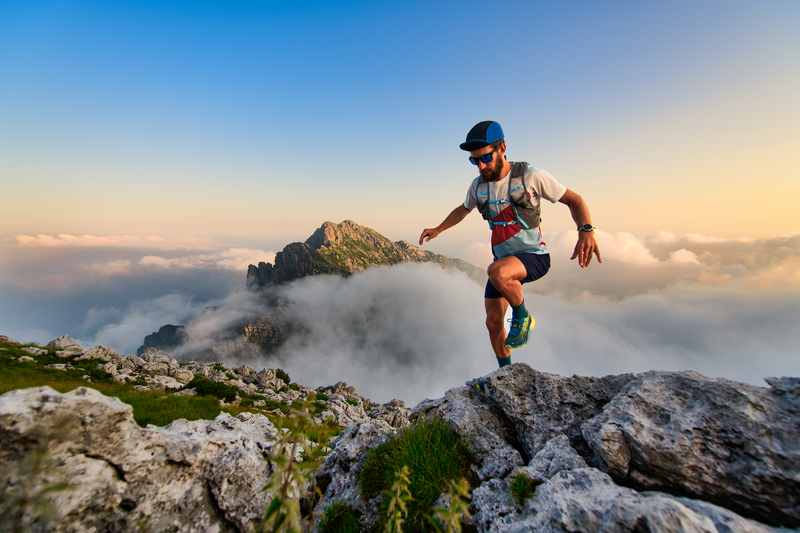
94% of researchers rate our articles as excellent or good
Learn more about the work of our research integrity team to safeguard the quality of each article we publish.
Find out more
REVIEW article
Front. Cell. Neurosci. , 18 January 2022
Sec. Cellular Neurophysiology
Volume 15 - 2021 | https://doi.org/10.3389/fncel.2021.754110
This article is part of the Research Topic Neurological and Psychiatric Disorders: The Role of Clathrin-Mediated Endocytosis (CME) and Related Intracellular Trafficking View all 6 articles
Endocytosis is a process essential to the health and well-being of cell. It is required for the internalisation and sorting of “cargo”—the macromolecules, proteins, receptors and lipids of cell signalling. Clathrin mediated endocytosis (CME) is one of the key processes required for cellular well-being and signalling pathway activation. CME is key role to the recycling of synaptic vesicles [synaptic vesicle recycling (SVR)] in the brain, it is pivotal to signalling across synapses enabling intracellular communication in the sensory and nervous systems. In this review we provide an overview of the general process of CME with a particular focus on two key proteins: clathrin and dynamin that have a central role to play in ensuing successful completion of CME. We examine these two proteins as they are the two endocytotic proteins for which small molecule inhibitors, often of known mechanism of action, have been identified. Inhibition of CME offers the potential to develop therapeutic interventions into conditions involving defects in CME. This review will discuss the roles and the current scope of inhibitors of clathrin and dynamin, providing an insight into how further developments could affect neurological disease treatments.
Clathrin mediated endocytosis (CME) is ubiquitous process in all eukaryotic cells. It is the major mechanism for sorting and internalisation of various macromolecules, proteins and lipids and controlling signalling pathway activation. CME plays a key role in the recycling of synaptic vesicles [synaptic vesicle recycling (SVR)] in the brain, allowing the continued signalling across synapses that is vital for intracellular communication in the sensory and nervous systems. Our focus is on the small molecules published to date as potential probe molecules. Major aspects of the biology roles of clathrin mediated endocytosis, we expect are covered within other manuscripts within this issue.
In CME, clathrin polymerises to act as a coat to mediate the internalisation of hormones, nutrients and receptors, while dynamin mediated scission of the vesicle by self-assembling into rings forming a collar around the neck of the vesicle (Royle, 2006; Anggono and Robinson, 2009; Robinson, 2015; Kaksonen and Roux, 2018; Milosevic, 2018). Dynamin and clathrin are vital to CME and play a role in the efficient recycling of synaptic vesicles. SVR mediates the proper function of nervous and sensory systems. These proteins are believed to be contribute towards multiple neurological conditions including, but not limited to, epilepsy (Chin et al., 1995; Di Paolo et al., 2002; Kim et al., 2002), schizophrenia (Schubert et al., 2012), Huntington's (McAdam et al., 2020), Parkinson's (Inoshita and Imai, 2015; Vidyadhara et al., 2019), and Alzheimer's disease (Rafii and Aisen, 2009; Wu and Yao, 2009; Palmer, 2011; Alsaqati et al., 2018). It is highly unlikely, given the complex nature of these conditions, that CME inhibition or defect is the sole disease progenitor. This review will provide an overview of selected health conditions linked with defects in CME/SVR and discuss the current scope of inhibitors of clathrin and dynamin. It is not intended to be a comprehensive listing of human diseases that maybe associated with endocytosis, but to provide an insight into how further development of these compounds could potentially affect the treatment of neurological diseases.
Given that of all the proteins involved in endocytosis, clathrin and dynamin are essentially the only two systems to which there have been reported small molecule inhibitors, our focus is centred on these two proteins. This work describes the structure and function of dynamin and clathrin in endocytosis across clathrin mediated and synaptic vesicle endocytosis; the potential role of SVE in neurological diseases and the current state of the art in developing CME (and SVE) inhibitors via targeting of dynamin and clathrin.
Clathrin, unsurprisingly, is critical to achieving a successful CME outcome (Roth and Porter, 1964; Pearse, 1975). It is principally involved in CME, mitosis, and SVR (Kaksonen and Roux, 2018; Mettlen et al., 2018). Clathrin activity requires the assembly of a macromolecular complex, a triskelion. Each leg is of ca 475Å length and 20Å thickness and radiates from a central hub which contains a helical tripod and QLMLT amino acid sequence (Figures 1A,B) (Ungewickell et al., 1981; Kirchhausen et al., 2014). The clathrin heavy chain (CHC) makes up the length of each leg and associates with a clathrin light chain (CLC) (Kaksonen and Roux, 2018). In humans, two CHC isoforms (CHC17 and CHC22) exist, with 85% amino acid homology. CHC17 is ubiquitous and involved in membrane trafficking and mitosis, while CHC22 is located within skeletal muscle (Kirchhausen et al., 2014; Kaksonen and Roux, 2018). Two 60% homologous CLCs exist, both can associate with CHC17 but not with CHC22 (Royle, 2006; Kirchhausen et al., 2014; Kaksonen and Roux, 2018). The CLC binds to the CHC at a series of zig-zags, where hydrophobic residues of the CLC face the surface of the heavy chain. The CLC has two flexible regions, which lie near the curved knee and the central vertex of the CHC when bound. The C-terminal of the CLC sits close to the vertex of the CHC triskelion (Kirchhausen and Toyoda, 1993; Kirchhausen et al., 2014).
Figure 1. (A) Schematic representation of the clathrin triskelion (top view). The heavy chain is displayed in dark blue, the light chain in light blue, and the terminal domain in purple. (B) Side view of clathrin triskelion showing the location of the tripod and QLMLT amino acid sequence at the central vertex. (C) Schematic representation of three clathrin triskelions bound in lattice formation. Each singular triskelion represented in a different colour (blue, green, and purple) (Fotin et al., 2004a). (D) Top view of the CTD, consisting of seven blades. The binding sites are shown: Yellow: Site 1 (clathrin box), orange: Site 2 (W-box), green: Site 3 (aL2 binding site), purple: Site 4, red: Site 5. (E) Bottom view of the CTD, showing the seven blades and five binding sites (Alsaqati et al., 2018).
The CHC comprises six segments; proximal, knee, distal, ankle, linker and terminal domain (Figure 1A) (Kirchhausen et al., 2014). The whole leg adopts a stacked hairpin structure containing a ~145-residue motif of five hairpins, repeated seven times (Robinson, 2015). These legs are flexible, to allow the formation of differing diameter coats of hexagons and pentagons by the polymerisation of clathrin triskelions (Kirchhausen, 2000). In the lattice, the proximal segment of each leg passes beside the proximal segment of the adjacent triskelion, the central vertex forming the points of the lattice, with the contour of each leg spiralling inwards and the terminal domain sitting towards the inside edge of the lattice (Figure 1C) (Fotin et al., 2004a; Kirchhausen et al., 2014; Robinson, 2015; Kaksonen and Roux, 2018).
The clathrin terminal domain (CTD) (resides 1-330) comprises seven β-sheets, stacked around a central axis in a propeller-like structure (Figures 1D,E) (Ter Haar et al., 1998). The propeller has an elliptical cross section due to increased spacing between blades 1 and 7, and 3 and 4, occupied with short helical segments. Differences in inter-strand loops gives rise to variation in the number of residues in each blade (Robinson, 2015). The terminal domain projects inwards, towards the membrane allowing interactions with adaptor proteins at a number of binding sites to direct incorporation of cargo (Table 1 details the key binding residues at each binding site). Adaptors bind to the CTD via short linear peptide sequences (Collette et al., 2009; Robinson, 2015).
Table 1. Summary of the currently known and proposed binding sites of the clathrin terminal domain, detailing key residues, and binding examples.
The CTD is connected to the distal leg by a short zig-zag linker of alpha helices followed by the ankle region (Lemmon and Traub, 2012; Kirchhausen et al., 2014). The ankle also contains the binding site of ADP-ribosylation factor-binding protein (GGA1) and adaptor protein (AP) 1 and AP-2 beta chains (Knuehl et al., 2006). This binding site has an unknown non-linear motif, but contains key residues C682, G710 (Knuehl et al., 2006).
Site directed mutagenesis of clathrin determined an interaction with β-arrestin and arrestion3, where the first 100 residues were sufficient for binding in vitro. This fragment corresponds to blades 1 and 2 of the propeller, with key residues (Q89, F91, K96, K98) found to lie in the groove between the blades (Figures 1D,E) (Ter Haar et al., 1998; Collette et al., 2009). This is Site 1, also known as the clathrin box. The principle contact sites gave a sequence known as the clathrin box motif; LΦXΦ[DE]where Φ is a hydrophobic reside and alternative residues are bracketed, [] (Lemmon and Traub, 2012). The β-2 subunit of adaptor protein 2 (AP-2) also contains this motif, which overlaps with another clathrin binding motif for site 3 possibly allowing AP-2 binding at both sites 1 and 3 (Muenzner et al., 2017).
A second binding site on clathrin, the W-box (Ramjaun and Mcpherson, 1996) site, binds proteins containing the W-box motif; PWXXW (where X is any amino acid), such as amphiphysin and sorting nexin 9 (SNX9) (Royle, 2006; Popova et al., 2013). Located in the deep pocket at the centre of the top surface the key resides of the W-box include F27, Q152, I154, and I170 (Figures 1D,E) (Miele et al., 2004; Collette et al., 2009; Chen et al., 2020).
Site 3, the aL2 (arrestin 2L) binding site is a shallow hydrophobic groove between blades 4 and 5 defined by W164, L183, R188, V190, Q192, I194, 231, E232, T235, and K245 (Figures 1D,E). The binding motif [LI][LI]GXL, observed in arrestin 2L has also been found in other trafficking accessory proteins such as the adaptins subunits of AP-1 and AP-2 (Muenzner et al., 2017), synaptotagmin and synaptojanin, however it is currently unknown if these proteins use this motif to interact with clathrin (Kang et al., 2009).
A triple knockout of sites 1–3, comprising a 2 point mutations in the W-box (Q152L, Il54Q), and a2L binding site (R188A, Q192A) and a 4 point mutation in the clathrin box (T87A, Q89A, K96E, K98E) retained CME function, suggesting the presence of a fourth binding site on the CTD (Willox and Royle, 2012). A region on the surface exposed blade 7 at E11, was identified as site 4, although the binding motif and protein partners are currently unknown (Figures 1D,E) (Willox and Royle, 2012).
A fifth proposed site lies between blades 5 and 6, lined with residues N175, G179, R221, Q23, F252, and F260 (Figure 2B). This site was suggested by a molecular dynamics investigation of the potential binding poses of the marine sesquiterpenoid, bolinaquinone. In a protein-pull down experiment, bolinaquinone was reported as a specific inhibitor of clathrin (Abdel-Hamid and McCluskey, 2014; Ghods et al., 2018).
Figure 2. (A) Domain structure of the human dynamin superfamily. All DSPs contain a GTPase domain, a middle domain (MD), and a GTPase effector domain (GED). The MD and GED comprises the stalk region of the dynamin structure. Additionally, most dynamins contain a domain for interactions with lipid membranes, such as a pleckstrin-homology (PH) domain or trans membrane domain (T). Classical dynamins contain a PH domain and also a C-terminal proline-rich domain (PRD). Other family members may have different sequences at the N-terminal or in between the MD and GED domains. Human dynamin superfamily members are grouped according to their domain structure into; classical dynamins (dynamin 1-3), Dynamin related proteins (examples shown; MxA/MxB, Mitofusin 1 and 2, and OPA1), and guanylate-binding proteins GBPs (examples shown; Atlastin 1-3 and GBP 1-5). Structural components are colour coordinated; red: bundle signalling element (BSE), green: G-domain, light blue: middle domain, yellow: PH domain, dark blue: GED, purple: transmembrane domain (T), grey: PRD (Praefcke and McMahon, 2004; Ford et al., 2011), pink: mitochondria localisation signal (MLS) and orange: coiled coil motif (CC). (B) Domain Structure of human dynamin 1 (Ford et al., 2011). Classical domain names are indicated below. (C) Ribbon representation of human dynamin 1. Domains represented by colours coordinating to the domain structure in A. GTPase domain (green), BSE (red), stalk region consisting of the middle domain (light blue) and GED (dark blue) and the PH domain (yellow). Hinge 1 and 2 are labelled (Ford et al., 2011).
Dynamin, a 100-kDa large GTPase and mechanochemical enzyme, is recruited post clathrin coated vesicle formation and is critical to vesicle scission and ultimately recycling of the clathrin coated pits (Robinson et al., 1987; Shpetner and Vallee, 1989; Popova et al., 2013). Dynamin differs significantly from traditional GTPases (Obar et al., 1990), and is the founding member of the dynamin superfamily of large GTPases (Anggono and Robinson, 2009). Dynamin, in addition to its CME role (Praefcke and McMahon, 2004), in involved in SVR (Ferguson and De Camilli, 2012; Antonny et al., 2016), caveolae internalization (Oh et al., 1998), and possibly vesicle trafficking in and out of the Golgi (Llorente et al., 1998; Nicoziani et al., 2000).
Dynamin spans species, from bacteria to human (Hinshaw, 2000; Anggono and Robinson, 2009; Ramachandran and Schmid, 2018). Dynamin and dynamin-related proteins (DRPs) are distinguished from traditional GTPases by a larger GTPase domain, their unique ability to self-assemble and disassemble–to which their GTP hydrolysis mechanism is distinctly associated (Hinshaw and Schmid, 1995; Marks et al., 2001; Chappie et al., 2010; Faelber et al., 2011). They have low GTP-binding affinities (Hinshaw and Schmid, 1995; Gasper et al., 2009), high GTPase activity and the ability to bind and tubulate lipid membranes (Takei et al., 1995; Hinshaw, 2000; Danino et al., 2004; Roux et al., 2006).
Structurally the dynamin superfamily proteins (DSPs) are classified as: the classical dynamins (Nakata et al., 1993; Sontad et al., 1994; Gray et al., 2003), the dynamin-related proteins (DRPs) and the guanylate-binding proteins (GBPs)/atlastins (Prakash et al., 2000a,b). The DRPs include dynamin-like proteins (Dlp) (Labrousse et al., 1999), Mx proteins (MxA, MxB) (Staeheli et al., 1986; Janzen et al., 2000; Kochs et al., 2002), optic atrophy 1 (OPA1) (Olichon et al., 2002; Satoh et al., 2003) and mitofusins (Rapaport et al., 1998; Santel and Fulller, 2000) (Figure 2). Many DSPs are involved in membrane re-modelling events including fission, fusion and intercellular tracking of vesicles and large organelles. Others such as the Mx proteins have roles independent of membrane properties like viral resistance to host cells (Ramachandran and Schmid, 2018). DLPs, also known as non-classical dynamins, are believed to assist in the recruitment of the classical dynamins to the membrane for vesicle scission (Singh et al., 2017).
Common to all DSPs are the large N-terminal GTPase domain (~300 amino acids), the middle domain (MD), and the GTPase effector domain (GED) (Figure 2A). Most DSPs contain additional domains that are tailored to their specific cellular functions or subcellular location. In the classical dynamins, the three basic set of domains is supplemented by two additional functional domains—a pleckstrin-homology (PH) domain and a proline-rich domain (PRD) at the C-terminus (Praefcke and McMahon, 2004; Anggono and Robinson, 2009).
There are three mammalian dynamin genes—dynamin 1, 2, and 3 which are known as the “classical dynamins” (Cao et al., 1998; Praefcke and McMahon, 2004). These dynamins share the same domain organisation and 80% overall homology, but they have distinctive expression patterns, with variation between isoforms primarily observed within the PRD (Ferguson and De Camilli, 2012; Jimah and Hinshaw, 2019).
Dynamin 1 is predominately expressed at high levels in the central nervous system, where it is concentrated in the presynaptic nerve terminals. Although not generally not present in non-neuronal tissues (Nakata et al., 1991; Ferguson et al., 2007; Anggono and Robinson, 2009), dynamin 1 has been detected in cultured cell lines (Liu et al., 2008; Ferguson et al., 2009) and in the testes (Zhou et al., 2017). In the brain, dynamin 1 is expressed at ~100-fold greater than other isoforms and in fact, is among the most abundant neuronal proteins in the brain (Anggono and Robinson, 2009). Dynamin 2 is ubiquitously expressed in all tissues where it plays a major role in mitosis (Nakata et al., 1991; Cook et al., 1994; Chircop et al., 2011; Smith and Chircop, 2012). Dynamin 3 is found predominantly in the testes and brain, where it is found both pre- and post- synaptically, and in low levels in the lungs (Nakata et al., 1993; Cao et al., 1998; Gray et al., 2003; Raimondi et al., 2011; Ferguson and De Camilli, 2012).
All three dynamins play a role in vesicle scission during the specialised neuronal function of rapid SVR (Anggono and Robinson, 2009). Dynamin 1 mediates the majority of synaptic vesicle endocytosis (SVE), particularly during a depolarization stimulus (Anggono and Robinson, 2009; Clayton et al., 2009) where dynamin 1 and several other endocytic proteins are constitutively phosphorylated in resting synapses and upon nerve stimulation. In addition to the role in CME, dynamin also plays a role in clathrin independent synaptic vesicle recycling pathways including clathrin independent fast endocytosis, fast endophilin mediated endocytosis and ultrafast endocytosis, where dynamin is involved in both scission and membrane curvature (Chanaday et al., 2019; Renard and Boucrot, 2021). Dynamin 1 is rapidly dephosphorylated (at S774 and S778) by the calcium and calmodulin-dependent phosphatase calcineurin (Cousin and Robinson, 2001) which is predicted to facilitate dynamin interactions with endocytic proteins to promote endocytosis (Anggono et al., 2006), specifically this dephosphorylation is believed to play a role in triggering activity dependent bulk endocytosis (Anggono et al., 2006; Cheung and Cousin, 2013). Phosphorylation and dephosphorylation may also play a role in regulation of activity in dynamin 1. Up to a 12-fold increase in GTPase activity has been shown to be caused by phosphorylation of dynamin 1 by protein kinase C, while the dephosphorylation results in inhibition of GTPase activity. Dephosphorylation also directs dynamin to the membrane, and the inhibition of GTPase activity may allow time for binding at the neck of a forming vesicle prior to GTPase hydrolysis to facilitate scission (Hinshaw, 2000).
Dynamin 2 plays a major role in SVR, mainly in the slow endocytosis that occurs after nerve stimulation has ceased (Anggono and Robinson, 2009). In neurons and non-neuronal cells, dynamin 2 mediates CME and mitosis (Chircop et al., 2011; Smith and Chircop, 2012; Kaksonen and Roux, 2018; Mettlen et al., 2018; Bhave et al., 2020). Dynamin 2 also has roles in caveolae budding (Henley et al., 1998; Oh et al., 1998), phagocytosis (Gold et al., 1999), podosome formation (Ochoa et al., 2000) and in endocytosis-independent cellular functions, such as actin dynamics (Orth and McNiven, 2003) and cytokinesis (Van Dam and Stoorvogel, 2002) and mitosis (Chircop et al., 2011; Smith and Chircop, 2012).
Dynamin 3 is proposed to have a specific postsynaptic role, forming specialised endocytic sites that locally recycle AMPA (α-amino-3-hydroxy-5-methyl-4-isoxazole propionic acid) receptors found in dendritic spine tips (Lu et al., 2007). This is consistent with a report of dynamin 3 binding to the ENA-VASP-homology 1 (EVH1) domain of the postsynaptic protein Homer through a PXXF motif (Gray et al., 2003). This motif is not present in dynamin 1 and dynamin 2. This preferential enrichment was not confirmed by another study which highlighted the overlapping presynaptic roles of dynamin 3 with dynamin 1 (Raimondi et al., 2011). Both dynamin 1 and 3 play critical roles in neurotransmission with individual conditional dynamin knockouts in mice having no effect on the development nerve terminals in mouse brain (calix of Held). A combined dynamin 1 and 3 knockdown results in a progressive synaptic transmission loss. This suggests that one or more of dynamin 1, 2 and 3 can rescue synaptic transmission caused by deletion of one dynamin isoform, but not of two. In turn this suggests a degree of functional dynamin isoform redundancy.
Classical dynamins comprise an extended structure with five domains; amino-terminal GTP hydrolysis (GTPase) domain, a “middle” domain (MD), a pleckstrin-homology (PH) domain, a GTPase effector domain (GED), and a proline-rich carboxy-terminal (PR) domain (Figure 2B) (Hinshaw, 2000; Ford et al., 2011; Ferguson and De Camilli, 2012; Kong et al., 2018; Jimah and Hinshaw, 2019).
The GTPase domain sits on a long helical “stalk” composed of anti-parallel helices from the middle domain to the N-terminal region of GED (Chappie et al., 2010, 2011; Faelber et al., 2011), linking the GTPase domain to the lipid interacting domains and providing interfaces for higher-order assembly (Jimah and Hinshaw, 2019). The PH domain forms the vertex or “foot” of the stalk hairpin and binds membranes (Ferguson et al., 1994). Linking the GTPase domain and “stalk” is a α-helical bundle, referred to as the bundle signalling element (BSE) (Chappie et al., 2010) or neck (Low and Löwe, 2006), which is formed from non-contiguous sequences at the N- and C-terminal helix of the GTPase domain, as well as the carboxy terminal of the GED (Chappie et al., 2010; Ramachandran and Schmid, 2018; Jimah and Hinshaw, 2019). The PRD domain emerges at the boundary between BSE and the GTPase domain and is expected to be unfolded, most likely projecting away from the membrane where it might interact with other proteins (Figure 2C) (Ferguson and De Camilli, 2012).
The GTPase domain is the most highly conserved region of DSPs (Warnock and Schmid, 1996; van der Bliek, 1999; Anggono and Robinson, 2009), but at ~300 residues is considerably larger than traditional GTPases. The GTPase domain includes splice variations that confer DSP-specific biochemical and biophysical properties and as well as biological functions (Vetter and Wittinghofer, 2001; Ramachandran and Schmid, 2018). The DSP GTPase domain is activated by a nucleotide-dependent dimerization (GAD), thus does not require guanine-exchange factors (GEFs) or GTPase-activating proteins (GAPs), as required by Ras GTPases (Gasper et al., 2009; Chappie et al., 2010). This is due to their unusually high GTPase activity and low affinity for GTP (Praefcke and McMahon, 2004).
Four GTP-binding motifs (G1-G4) exist in the GTPase domain: G1, or P-loop, binds the phosphate; a G2 threonine enables GTP hydrolysis by coordinating a Mg2+; G3 also coordinates the Mg2+ ion via aspartate and glycine binds the γ-phosphate; and G4 binds the nucleotide base (Niemann et al., 2001; Bramkamp, 2012). The conservation of these motifs is absolute except for the G4 motif in guanylate-binding proteins (Praefcke and McMahon, 2004). K44A, T65A, T141D, and K142A GTPase domain mutations inhibit endocytosis (Damke et al., 1994; Marks et al., 2001; Song et al., 2004).
Several DSPs have two elongated α-helical bundle domains (the BSE), adjacent to the GTPase domain (Jimah and Hinshaw, 2019). The BSE is composed of helices derived from the N- and C-terminal of the GTPase domain and the C-terminal of GED (Ramachandran and Schmid, 2018). In fission, the BSE connects the GTPase domain to the membrane-distal end of the stalk and undergoes a swivel-like motion relative to the GTPase domain during GTP hydrolysis cycles. In fusion, DSPs contain a functionally equivalent nucleotide-responsive flexible hinge in the bipartite helical-bundle (HB) stalk that bounds directly against the GTPase domain (Ramachandran and Schmid, 2018). These conformational shifts are believed responsible for the DSP power stroke in fission and fusion (Chappie et al., 2009, 2010; Yan et al., 2018). An A738T mutation in the BSE suppresses the temperature-sensitive Drosophila Shibire mutants which occur within the GTPase domain and cause defects in GTP binding and endocytosis (Kosaka and Ikeda, 1983; Ramaswami et al., 1993; Narayanan et al., 2005).
The middle domain (MD) of dynamin lacks sequence homology to any known protein structural motif (Hinshaw, 2000; Anggono and Robinson, 2009). This domain is involved in the formation of dynamin tetramers required for the higher-order self-assembly of tetramers into rings and helices (Faelber et al., 2011; Reubold et al., 2015; Kong et al., 2018). The middle domain comprises a N-terminal and C-terminal region (Muhlberg et al., 1997). Between dynamin isoforms the N-terminal region significantly more conserved than the C-terminal region (Warnock and Schmid, 1996). The C-terminal region accounts for the alternative splice sites for all three classical dynamins. A synthetic equivalent of the N-terminal region adopts an α-helical coiled-coil structure that forms stable tetramers in solution and has been implicated in dynamin-dynamin assembly (Okamoto et al., 1999; Smirnova et al., 1999). It also makes extensive contacts with the self-assembling GED (Anggono and Robinson, 2009).
The GED, or coiled-coil domain is involved in protein-protein interactions (Lupas et al., 1991; Okamoto et al., 1999). This domain binds to the GTPase domain, to the MD, and to itself to form homodimers (Muhlberg et al., 1997; Okamoto et al., 1999; Zhang and Hinshaw, 2001). These interactions drive dynamin self-assembly. The addition of an isolated GED to an unassembled dynamin stimulated its GTPase catalytic activity by up to 100-fold. The GED acts as a dynamin GAP, stimulating both dynamin assembly and GTPase activity (Muhlberg et al., 1997; Sever et al., 1999). The GED, along with the middle domain, make up the “stalk.”
Among the DSPs only the classical dynamins contain the PH domain (Anggono and Robinson, 2009). This domain contains a seven stranded β-sheet sandwich, with a dynamin hairpin, and three variable loops, ending in the C terminal α-helix (Ferguson et al., 1994; Timm et al., 1994). The PH domain exhibits high homology with pleckstrin, a major PCK substrate in platelets and is found in many proteins (Hinshaw, 2000). Most variation is observed in the three (variable) loops. Each presents a predominantly hydrophobic region, to promote membrane interactions, polymerisation dynamins and membrane curvature with a positive charge within the binding site (Ramachandran et al., 2009; Liu et al., 2011). Dynamins PH domain shows low lipid specificity, but favours binding to phosphatidylinositol-4,5-biphosphate (PI(4,5)P2) (Ferguson et al., 1994; Zheng et al., 1996; Anggono and Robinson, 2009). PI(4,5)P2 binding to dynamin produces the highest activation of its GTPase activity. Lipid binding is significantly enhanced when the PH domain is oligomerized (Salim et al., 1996; Klein et al., 1998). Dynamin with PH-domain mutants that impair phosphoinositide binding (PI(4,5)P2) exert dominant negative effects on CME (Lee et al., 1999; Vallis et al., 1999). The PH domain is required for membrane localisation and CME.
Only classical dynamins possess a PRD. Comprising several BAR and SH3 domain binding sites (defined by a PXXP motif), it is a protein-protein interaction domain for a variety of signalling and cytoskeletal proteins (Mcpherson, 1999; Simpson et al., 1999; Anggono and Robinson, 2009; Ramachandran and Schmid, 2018). These function to recruit dynamin to endocytic sites and coordinate dynamin's function with these other factors during endocytosis (Shpetner et al., 1996; Lundmark and Carlsson, 2004; Anggono et al., 2006; Anggono and Robinson, 2009). For example, amphiphysin directs dynamin to the coated pits by binding to both dynamin and the AP-2 α-subunit complex (David et al., 1996; Shupliakov et al., 1997). Dynamin lacking the PRD cannot rescue endocytic defects in dynamin-knockout fibroblasts (Ferguson et al., 2009). A major function of PRD is to target dynamin to its site of action in the cell. Overexpression of Dyn1ΔPRD results in a failure of dynamin to accumulate at clathrin coated pits (CCP) (Anggono and Robinson, 2009). The PRD also enables dynamin recruitment by key partners such as syndapin that may be involved in membrane tubulation and at the synapse (Qualmann et al., 1999). Dynamin 1 PRD is also the site for its phosphorylation and regulation of its activity in nerve terminals (Anggono et al., 2006).
Dynamin self-assembles in vitro into rings and helices (Hinshaw and Schmid, 1995). Cryogenic electron microscopy revealed that the dynamin polymer unit is an anti-parallel dimer, with two GTPase domains linked to one side of the cross and the PH domains linked to the other side (Antonny et al., 2016; Kong et al., 2018). Dimerization is mediated by the stalks (MD and GED), which form the cross and provides the interface for further polymerisation (Figure 3). While the simplest dynamin unit is the dimer, in solution dynamin was found to exists as a tetramer (Morlot and Roux, 2013) that fluctuates between a monomer-tetramer equilibrium under physiological salt conditions (Binns et al., 1999; Hinshaw, 2000). These tetramers are capable of further self-assembly into rings or helices (Figures 3B,C) (Hinshaw and Schmid, 1995; Muhlberg et al., 1997; Binns et al., 1999).
Figure 3. Structure of dynamin dimer and tetramer. (A) Crystal structure of a dimer showing the stalk interface (interface 1) needed for self-assembly (Reubold et al., 2015). (B) Crystal structure of a tetramer showing the stalk interfaces (interfaces 1, 2, and 3) needed for self-assembly. GTPase domain (green), BSE (red), stalk region consists of the middle domain (light blue) and GED (dark blue) and the PH domain (yellow) (Reubold et al., 2015). (C) Structure of dynamin and its assembly into oligomers. Ball and stick representation of a dynamin dimer and tetramer, showing how the dimer polymerize into a tetramer and how tetramers further assemble into a helix around a membrane tube. The GTPase domain is represented in green, BSE in red, stalk in blue, and PH domain in yellow (Chen et al., 2020).
Dynamin rings (or stacks of rings) eventuate under low salt conditions and no underlying template (Hinshaw and Schmid, 1995). In the presence of a template, e.g., liposomes, microtubules, or other suitable membrane templates, dynamin forms helices (Shpetner and Vallee, 1989; Stowell et al., 1999; Roux et al., 2010). The helical structure occurs due to interactions between the stalk dimers, which drive the assembly into the polymer of the expected size, as observed in the molecular dynamics of the assembly process (Faelber et al., 2011). The dimers polymerise in a curved alignment allowing the formation of the macromolecular helix (Faelber et al., 2011). The stalks form the core of the dynamin helix, with the GTPase domains facing outside and the PH domains on the inside bound to the membrane tube (Figure 3C) (Zhang and Hinshaw, 2001; Chen et al., 2004; Mears et al., 2007). Helix constriction, as seen in membrane fission in endocytosis, is believed to be the result of a conformation change at the flexible hinge of the BSE (Ford et al., 2011). This conformational change would also result in the PH domain inserting into the lipid bilayer, causing additional curvature stress on the membrane assisting in membrane fission (Ramachandran et al., 2009).
Dynamin self-assembly produces up to a 1000-fold increase in its GTPase activity (Anggono and Robinson, 2009). Of the isoforms, dynamin 2 has the highest intrinsic GTPase activity (3–10-fold higher than dynamin 1) and also a higher stimulated GTPase activity under self-assembly promoting conditions (Höning et al., 1997; Warnock et al., 1997). This oligomerization explains dynamin's ability to tubulate membrane bilayers under appropriate conditions by forming a continuous membrane coat around them (Takei et al., 1995; Sweitzer and Hinshaw, 1998; Roux et al., 2006) and its ability to associate with tubular templates which facilitate with its assembly (Shpetner and Vallee, 1989; Stowell et al., 1999; Marks et al., 2001; Roux et al., 2010). Dynamin polymerization in solution is favoured by binding to non-hydrolysable analogues of GTP, such as GTPγS, GDP·AlF, or GMPPCP, while GTP hydrolysis favours disassembly of the dynamin oligomers and release of its subunits from the plasma membrane (Hinshaw and Schmid, 1995; Carr and Hinshaw, 1997; Marks et al., 2001; Danino et al., 2004). In the absence of a nucleotide, purified dynamin assembles into a helix of ~50 nm outer diameter with a helical pitch between 10 nm and 20 nm, surrounding a 20 nm radius membrane tubule (Sweitzer and Hinshaw, 1998; Takei et al., 1998; Chen et al., 2004; Danino et al., 2004). Dynamin assembly and hydrolysis of GTP is critical for completion of CME.
Endocytosis spans a number mechanistically varied pathways including phagocytosis, micropinocytosis, caveolae-mediated endocytosis, and CME (McMahon and Boucrot, 2011; Kaksonen and Roux, 2018). CME is heavily implicated in nutrient uptake, signal transduction, synaptic vesicle recycling, maintenance of cell polarity, and antigen presentation (Mellman and Warren, 2000). CME can be broken up into five major stages and can be explained by examining the mechanism of cargo internalisation (Figure 4).
Figure 4. Schematic diagram of CCV formation occurring in the following steps; Nucleation, cargo selection, coat formation, vesicle scission, and uncoating.
The first step of CME is the nucleation of clathrin coated pits and vesicles (CCP and CCV), the exact mechanism of which remains to be elucidated. Key endocytic proteins include adaptor protein 2 (AP-2), FCH domain only (FCHO) proteins, epidermal growth factor receptors (EGFRs) and intersectins. All have binding domains for PI(4,5)P2, which is believed to act in the recruitment of endocytic proteins to the membrane (Höning et al., 2005; Reider et al., 2009; Stimpson et al., 2009; Henne et al., 2010; Taylor et al., 2011; Umasankar et al., 2012). It is still unclear if these components are all essential and which initiates the nucleation process. The AP-2 complex is known to be involved in the nucleation process for recruitment of clathrin, but whether the complex is necessary for initiation to occur is disputed (Motley et al., 2003; Godlee and Kaksonen, 2013). Depletion of AP-2 leads to a general block of transferrin (Tf) uptake (Godlee and Kaksonen, 2013), but this conflicts with other studies on the uptake of other major cargos, e.g., low-density lipoprotein receptor LDLR, EGFR and influenza (Motley et al., 2003). It may be that different cargo depend on different clathrin adaptors. Clathrin can be recruited to the membrane by other adaptors including adaptor protein 180 (AP180) and epsin, which have been found to recruit clathrin via synthetic lipid monolayer (Ford et al., 2001). These alternative adaptors may also work with AP-2 as some are found to bind to the AP-2 complex.
Depletion of FCHO reduced the lifetime of nucleation sites. CCPs still formed in the absence of FCHO, but were observed to abort, resulting in incomplete pit formation, and furthermore they were observed to grow faster in the presence of FHCO (Cocucci et al., 2012). FCHOs are also known to interact with epidermal growth factor receptor substrate 15 (eps15) and intersectin, both of which are important in progression of CCP formation (Benmerah et al., 1999; Koh et al., 2007; Pechstein et al., 2010). Epsin and intersectin, are believed to aid in the clustering of FCHO (Henne et al., 2010; Godlee and Kaksonen, 2013), additionally FCHO proteins and epsin, are often present selectively at the outer rim of the assembling coat and are proposed to have a role in stabilisation the curvature of the bilayer, via the F-Bar domain of FCHO (Tebar et al., 1996; Saffarian et al., 2009; Henne et al., 2010). FCHO proteins are necessary to stabilise the growing CCPs.
CME generates the necessary vesicles to ensure transport of a wide variety of cargo across the plasma membrane. Protein components of the clathrin coat recruit cargo by binding to specific sites of different transmembrane cargo molecules, enriching the concentration of specific cargo at the site of the forming vesicle (Kaksonen and Roux, 2018). Recognition and internalisation of such a wide range of cargo requires the existence of many adaptor proteins. In CME, these adaptors are known as clathrin-associated sorting proteins (CLASPs). Clathrin and CLASPs binding allows the sorting proteins to become engrained in the polyhedral scaffold and engage in recognition of sorting signals to recruit cargo. CLAPSs are categorised by their structural identity as oligomeric or monomeric (Traub and Bonifacino, 2013).
AP-2 is the best understood cargo selective clathrin adaptor (Traub, 2009). AP-2 is a key player in CCP initiation, where it is able to bind to clathrin and PI(4,5)P2 (Cocucci et al., 2012). The AP-2 protein family comprises AP-1, AP-3, AP-4 and AP-5. AP-1 acts in essentially the same role as AP-2, but in CME at intracellular membranes, rather than the plasma membrane where AP-2 is observed. AP-3 has a role in the sorting of proteins to lysosomes (Hirst and Robinson, 1998). It is capable of acting with or without association with clathrin. AP-4 has no clathrin association, and the role of AP-5 is at this stage unclear (Robinson, 2015).
The AP complexes are each made up of four non-identical polypeptide chains arranged into a block-like shape, with two symmetrical appendages connected by a flexible hinge-like stalk (Figure 5) (Hirst and Robinson, 1998; Traub, 2009). AP-2 comprises an α subunit (100 kDa), β2 subunit (100 kDa), μ2 subunit (50 kDa), and σ2 subunit (17 kDa) (Scarmato and Kirchhausen, 1990; Collins et al., 2002; Traub, 2009). The two hinge appendages (α and β2 subunits) are involved in interaction with clathrin and other adaptors, while the central block-like core mediates sorting signal recognition and binding to PI(4,5)P2 (Traub, 2009).
Figure 5. Structure of Adaptor complexes (AP) 1-5. The hinge, truck and ear regions are labelled on AP-1, and the subunits are labelled on each adaptor protein.
In addition to the AP complexes, a wide range of dimeric and monomeric CLASPs have also been characterised. Some examples of dimeric sorting proteins include FCHO1 and eps15, and monomeric CLASPs include Low Density Lipoprotein Receptor Adaptor Protein 1 (LDLRAP1 or ARH), Disabled homologue (Dab), Numb, β-arrestin and epsin (Traub and Bonifacino, 2013). CLASPs identify and recruit cargo through the use of endocytic signals, typically linear amino acid motifs (Ohno et al., 1995; Olusanya et al., 2001; Höning et al., 2005; McMahon and Boucrot, 2011; Taylor et al., 2011; Traub and Bonifacino, 2013) and covalent modifications, such as ubiquitination (Sorkina et al., 2006) and phosphorylation (Santini et al., 2002; Edeling et al., 2006; Burtey et al., 2007; Marchese et al., 2008; Reider et al., 2009; Traub and Bonifacino, 2013).
Coat assembly occurs as clathrin triskelia begin to self-polymerise to a polyhedral cage around the cargo (Kaksonen and Roux, 2018), leading to a CCV that is still connected to the plasma membrane by a narrow “neck” (Young, 2007). As polymerisation occurs curvature effectors such as EPS15 and epsin are displaced to the edges of the forming vesicle (Tebar et al., 1996), to assist in the stabilisation of the coat (McMahon and Boucrot, 2011). There are two competing models of membrane bending to form the CCP proposed (Kaksonen and Roux, 2018); the constant curvature model (Kirchhausen, 2009; Saffarian et al., 2009; Cocucci et al., 2012) and the constant area model (Avinoam et al., 2015; Haucke and Kozlov, 2018).
The constant curvature model was originally proposed, where clathrin would polymerise directly onto a curved membrane, maturation occurring via an increase in the area of the clathrin coated membrane. The area increases as clathrin self assembles, while the curvature of the membrane remains constant throughout maturation of the CCV (Kirchhausen, 2009; Saffarian et al., 2009; Cocucci et al., 2012). Many in vitro findings are consistent with the constant curvature model as increased tension and bending rigidity blocked clathrin polymerisation (Saleem et al., 2015), however in vivo flat clathrin patches have repeatedly been observed (Heuser, 1980; Mettlen et al., 2010), leading to the proposal of the constant area mode.
The constant area model requires the formation of a clathrin lattice on a planar or nearly planar area of membrane prior to the initiation of membrane bending (Avinoam et al., 2015). Molecular rearrangements and exchange of clathrins results in the hexagon/pentagon curved coat (Kirchhausen, 1993; Avinoam et al., 2015), increasing the curvature while the area remains largely unchanged (Avinoam et al., 2015). The exchange of clathrin at the CCP is observed during both early and late stages of endocytosis (Kirchhausen et al., 2014) and is supported by the results that blocking the turnover of clathrin arrests CCP formation at all stages (Von Kleist et al., 2011). More recent studies have also supported this model showing curvature beginning after assembly of a flat clathrin lattice (Bucher et al., 2018; Scott et al., 2018). However, the number of clathrin bonds that would need to undergo modification to form a pentagon from a hexagon paired with the affinity of these bonds renders this an energetically costly process (Kirchhausen, 1993; Nossal, 2001). It is likely that the process occurs by a combination of these models, as both models have been simultaneously observed, showing curvature prior to clathrin polymerisation, and curvature after clathrin polymerisation (Haucke and Kozlov, 2018; Scott et al., 2018).
The integration of accessory proteins such as epsin or clathrin assembly lymphoid myeloid leukaemia protein (CALM) within the lattice may assist in the curvature (Ford et al., 2002; McMahon and Boucrot, 2011; Miller et al., 2015; Kaksonen and Roux, 2018). Actin filaments are also able to contribute to membrane curvature, via localised polymerisation at the membrane and coupling with the clathrin coat to force the bending of the membrane (Carlsson and Bayly, 2014; Kaksonen and Roux, 2018).
Scission of the narrow neck that connects the almost fully formed membrane bound vesicle allows completion of cargo trafficking. This is mediated by dynamin as a helical collar around the neck of the formed vesicle (Kaksonen and Roux, 2018). Dynamin recruitment to the vesicle is believed to occur by BAR domain containing proteins, e.g., amphiphysin, endophilin, sorting nexin 9 (SNX9) via binding at the PRD (McMahon and Boucrot, 2011). GTP hydrolysis modulates the helical structure of dynamin, (Sweitzer and Hinshaw, 1998) and membrane tension facilitates the constriction that reduces the radius of the membrane in its helix (Stowell et al., 1999; Roux et al., 2006). There are two current models of dynamin fission step (Antonny et al., 2016); two-stage dynamin-catalysed fission model (disassembly model) and the constrictase/ratchet model (Figure 6).
Figure 6. Two models for dynamin-mediated membrane fission. (A) Two-stage fission model, where constriction is mediated by assembly and fission by disassembly. (B) The constriction/ratchet model, where constriction is achieved by active sliding of the helical turns and induce fission (Chen et al., 2020).
The two-stage dynamin-catalysed fission model suggests that in the first stage, assembled dynamin in a specific nucleotide-driven conformation adopts a super-constricted state enabling the formation of a hemi-fission intermediate (Bashkirov et al., 2008; Pucadyil and Schmid, 2008; Boucrot et al., 2012). This stage likely corresponds to the GDP + Pi transition state, when GTPase domains across adjacent rungs form their highest affinity interactions (Chappie et al., 2011). Release of Pi from the GTP-bound state in the second stage would then release the scaffold, as seen by negative-stain electron microscopy, allowing for the hemi-fission intermediate to proceed to complete fission (Figure 6A) (Stowell et al., 1999; Danino et al., 2004; Mattila et al., 2015).
The constrictase/ratchet model suggests dynamin functions as a motor. The energy obtained from GTP hydrolysis would be spent in mechanical work to slide adjacent turns of the dynamin helix (Figure 6B). In this model, GTPase domains, which binds dynamin through direct interactions, could act as molecular motors, and by the dimerizations-power strokes-dissociations cycles powered by GTP hydrolysis, would trigger relative sliding of the helical turn, leading to constriction and twisting of the helix. Fission results from membrane destabilisation arising when the constricted ring disassembles (Sweitzer and Hinshaw, 1998; Praefcke and McMahon, 2004; Ferguson and De Camilli, 2012; Antonny et al., 2016). Studies have shown that GTP binding induces trans-dimerization of dynamin GTPase domains via an interface across the nucleotide-binding site (Chappie et al., 2011). BSE senses the nucleotide loading status of the GTPase domain resulting in an open conformation in the presence of GTP and a 70° rotation to a closed state in the absence of nucleotide. Potentially this acts as a power stroke for dynamin during constriction and twisting (Chappie et al., 2009, 2010, 2011; Ford et al., 2011).
The final step in CME involves the release of endocytic proteins by uncoating allowing these materials to be recycled and the vesicle to fuse with its target membrane for cargo trafficking (Kaksonen and Roux, 2018). Auxilin binds within the clathrin lattice at the points of overlapping ankles, which lie under the tripod of the C-terminal of the adjacent triskelion (Fotin et al., 2004b; Kaksonen and Roux, 2018) recruiting heat shock cognate (Hsc70) to a critical point of interaction of the clathrin lattice (Schlossman et al., 1984; Umeda et al., 2000; Fotin et al., 2004b). Auxilin binding is believed to distort the clathrin lattice (Xing et al., 2010), allowing Hsc70 recruitment and binding at the QLMLT sequence under the tripod of the central vertex (Rapoport et al., 2009), increasing distortion and strain at the point where three ankles cross over each other under the tripod (Rapoport et al., 2009; Xing et al., 2010). This Hsc70 mediated strain between clathrin triskelion is one of the two possible mechanisms for uncoating understood at this stage. The other model suggests the collision resulting from the recruitment of Hsc70 produced a localised pressure in the disrupted lattice (Xing et al., 2010) to mediate uncoating (Sousa et al., 2016; Kaksonen and Roux, 2018).
Neurotransmission between nerve cells is at the core of intracellular communication in the sensory and nervous systems. Non-peptide neurotransmitters are released from small, specialised round organelles called synaptic vesicles (SVs), involved in the signalling across synapses (Wu et al., 2014; Milosevic, 2018). SVs are essential in preserving key neuronal properties, but there are a limited number of SVs in the brain due to the small size of presynaptic terminal. Thus, synapses reply on controlled recycling of SV (SVR) membranes and proteins. SVR is fundamental to synaptic transmission (Lu et al., 2009; Chanaday et al., 2019).
At pre-synapses SVs are organised into four “pools”; the readily releasable pool (RRP), recycling pool, reserve pool and resting pool (Chanaday et al., 2019). The RRP consist of SVs that are physically in contact with the plasma membrane and are immediately available for fusion. Upon the arrival of electrical membrane potential (action potential), Ca2+ channel opening is induced, and Ca2+ concentration elevation stimulates fusion of these SVs in the RRP. The RRP requires constant replenishment, which occurs either by recruitment of new SVs from the reserve pool, or the rapid reuse of fused SVs (Holderith et al., 2012; Guo et al., 2015).
SVR involves vesicle exocytosis from the RRP, which is followed by retrieval via endocytosis (Lu et al., 2009; Denker and Rizzoli, 2010; Kaeser and Regehr, 2017) to ensure a constant supply of neurotransmitter filled SVs. Following endocytosis, new SVs required rapid refilling with neurotransmitters and prepared for fusion (Blakely and Edwards, 2012; Farsi et al., 2017). Vacuolar H+ ATPase (vATPase) and vesicular neurotransmitter transporters are the main components involved in the filling of new SVs. vATPase acts by forming an electrochemical gradient across the membrane, which allows the transporters to move the neurotransmitters into the SVs. The transporters also determine the type of neurotransmitters content in the SV. Recycling of these key components is believed to be coupled with SVR (Blakely and Edwards, 2012; Farsi et al., 2017; Chanaday et al., 2019).
Investigations in a range of different systems, indicated an essential role of clathrin and suggested CME is one of the major mechanism for SVR (Heuser and Reese, 1973; Maycox et al., 1992; Morgan et al., 1999, 2000, 2001; Granseth et al., 2006; Heerssen et al., 2008; Kasprowicz et al., 2008). CME at the synapses is a specialised form of the membrane trafficking pathway that occurs in all cells, occurring via the same five steps (nucleation, cargo selection, coat formation, scission, uncoating) as detailed above. However, the rate of endocytosis during SVR differs vastly from endocytosis at the plasma membrane (Morris and Schmid, 1995), and unlike the heterogeneous vesicles observed at the plasma membrane CME at the synapses results in extremely uniform vesicles (Milosevic, 2018). Synaptic vesicles are internalised in seconds following neurotransmission, and are able to be re-loaded with neurotransmitters within 1–2 min; in contrast, the half time of internalisation of transferrin (Tf) at the plasma membrane is 3–5 min (Morris and Schmid, 1995). Clathrin independent mechanisms of SVR will not be discussed in further detail here, for a more complete overview refer to recent reviews in this area (Cremona and De Camilli, 1997; Soykan et al., 2016; Watanabe and Boucrot, 2017; Chanaday et al., 2019; Casamento and Boucrot, 2020; Renard and Boucrot, 2021).
SVR originally was thought to comprise only “kiss and run” and CME. Kiss and run occurs quickly (<1–2 s) compared to CME (10–30 s) (Chanaday et al., 2019). In addition, kiss and run does not require clathrin-associated proteins, and instead acts through SV briefly contacting the plasma membrane via a small fusion pore, which allows the release of small molecules. The SVs do not completely flatten to the membrane and are then able to be quickly recycled (Fesce et al., 1994; Gandhi and Stevens, 2003; Wightman and Haynes, 2004; Zhang et al., 2009; Wen et al., 2017). SVR is now know to encompass activity dependent bulk endocytosis (ADBE) (Holt et al., 2003; Wu and Wu, 2007), clathrin independent fast endocytosis (CIFE) (Delvendahl et al., 2016), fast endophilin mediated endocytosis (FEME) (Boucrot et al., 2015; Casamento and Boucrot, 2020; Renard and Boucrot, 2021), ultrafast endocytosis (UFE) (Clayton and Cousin, 2009; Clayton et al., 2009; Holderith et al., 2012; Watanabe and Boucrot, 2017; Gan and Watanabe, 2018).
ADBE occurs in response to longer bursts of intense activity and retrieves large areas of membrane within 1–2 s via a clathrin independent mechanism (Clayton et al., 2008; Clayton and Cousin, 2009; Kononenko and Haucke, 2015; Gan and Watanabe, 2018; Chanaday et al., 2019). Large intracellular endosomes are formed by inducing actin-driven membrane invaginations at sites where synaptic vesicle cargo is clusters. These endosomes average 150 nm, but can reach up to 500 nm in size and non-specifically retrieve all molecules within that region of the membrane (Clayton and Cousin, 2009; Kononenko and Haucke, 2015; Nicholson-Fish et al., 2015). In mammalian, invertebrate and amphibian synapses, ADBE is triggered by high stimuli and increased Ca2+ concentrations. Increased Ca2+ levels in nerve terminals activate calcineurin, which dephosphorylates and activates many endocytic proteins, including synapnin-1 and dynamin-1 (Cheung and Cousin, 2013). In weakly stimulated neurons ADBE is inhibited by the phosphorylation of these endocytic proteins by kinases [including Cyclin dependent kinase 5 (Cdk5) and glycogen synthase kinase 3 β (GSK3β)] (Evans and Cousin, 2007; Smillie et al., 2013). Some studies have indicated ADBE can occur independently of dynamin (Raimondi et al., 2011; Wu et al., 2014).
UFE can occur as fast as 50 ms after exocytosis (Watanabe et al., 2013a,b; Zhou et al., 2014; Delvendahl et al., 2016). UFE studies in C. elegans (neuromuscular junctions) and in mouse (hippocampal synapses) and have shown sites of UFE as the edges of the active zone, and is predominant as physiological temperatures (Watanabe et al., 2014; Soykan et al., 2017). UFE results in larger vesicles (80 nm) and does not require clathrin. Although not requiring clathrin, many of the proteins required for CME are also involved in ultrafast endocytosis. Synaptojanin 1, endophilin A and dynamin 1 are involved in vesicle budding and scission, while actin is also essential (Watanabe et al., 2014; Watanabe and Boucrot, 2017; Chanaday et al., 2019). There are also reports of CIFE, mediated by dynamin and actin which additionally shares many features of UFE (Delvendahl et al., 2016).
FEME is also clathrin independent but requires many CME proteins, notably regulation by endophilin. FEME occurs within 1–10 s and is triggered on activation of specific receptors, including G-protein coupled receptors, interleukin-2 receptor (IL2R) and some receptor tyrosine kinases (Boucrot et al., 2015). This pathway occurs through a similar progression of steps as CME and has been suggested to function in the post-synaptic SVR (Boucrot et al., 2015; Watanabe and Boucrot, 2017; Casamento and Boucrot, 2020).
All the known components of CME, including clathrin and dynamin, are expressed in higher levels (10–50-fold) in neuronal cells, possibly allowing the accelerated rate of CME observed, by increasing the number of coated pits and efficiency of assembly (Morris and Schmid, 1995). Neuron-specific inserts are observed in both clathrin light chains (Brodsky et al., 1991), and also in the β-subunit of AP-2 complex, along with the neuron specific dynamin 1 (Robinson et al., 1994), and the α-subunit of AP-2 (Robinson, 1992; Morris and Schmid, 1995). There are also neuron specific endocytic components, not generally observed in CME at the plasma membrane such as neuron specific assembly protein AP-3, which is ca 4 times more efficient at stimulating clathrin assembly (Lindner and Ungewickell, 1992; Morris and Schmid, 1995).
In addition to its role in CME recycling of synapses, clathrin also mediates the steps involved in filling SVs with neurotransmitters, as sorting of neurotransmitter transporters requires clathrin and adaptor proteins (AP-1, AP-2, and AP-3) (Blakely and Edwards, 2012; Silm et al., 2019). Each SVs must contain a proper set of neurotransmitter transporters to ensure the SV is filled with the correct neurotransmitter content (Chanaday et al., 2019). Additionally clathrin may play a role in determining timely acidification, and therefore neurotransmitter loading. Acidification of SVs requires uncoating of the clathrin cage, suggesting removal of this coat ensures the timing of neurotransmitter loading occurs as soon as the SV is reformed (Farsi et al., 2018).
The efficient and effective recycling of SVs is critical for proper function of sensory and nervous systems, and synaptic physiology (Milosevic, 2018). Various neurological conditions have proposed reliance on SVR and possibly CME, such as Parkinson's (Inoshita and Imai, 2015; Vidyadhara et al., 2019), Alzheimer's (Rafii and Aisen, 2009; Wu and Yao, 2009; Palmer, 2011; Alsaqati et al., 2018), epilepsy (Chin et al., 1995; Di Paolo et al., 2002; Kim et al., 2002), Huntington's disease (McAdam et al., 2020), and schizophrenia (Schubert et al., 2012).
It is important to recognise that the aetiology of neuronal disease is typically complex. It is thus difficult to assign defects in a single process or protein as being the cause of the disease, but in the exemplars (note that this is not intended as a comprehensive or exhaustive list of endocytosis related human diseases) shown below, a defect in endocytosis has been reported.
Alzheimer's disease, affects >37 million people globally, is a progressive neurological disorder that affects the daily lives of sufferers through cognitive impairment and memory loss (Rafii and Aisen, 2009). Early symptoms include issues with short term memory, spatial orientation, attention, confusion and changes in mood and personality (Palmer, 2011).
The amyloid precursor protein (APP), a type of glycoprotein, is produced by the neuron and has several roles in the development and function of the neuron (Mattson, 1997). APP can undergo cleavage by either non-amyloidogenic processing (α-secretase and γ-secretase) or amyloidogenic processing (β-secretase and γ-secretase). The latter of these results in production of amyloid-β peptide (Aβ) (Alsaqati et al., 2018), which along with neurofibrillary tangles, are central to the pathogenesis of Alzheimer's disease (Rafii and Aisen, 2009). It is suggested that endocytosis and intracellular sorting determines the processing of APP, and that amyloidogenic processing occurs within endosomes, the production of Aβ is therefore dependent on internalisation of APP via CME (Alsaqati et al., 2018). One of the most notable abnormalities in AD is changes in the endocytic process (Milosevic, 2018). An increase in endocytosis with age has been reported, leading to increased internalisation of APP and subsequently production of Aβ, potentially explaining why age is the most important risk factor of AD (Milosevic, 2018). Further, it has been demonstrated that the proteins involved in CME are upregulated with ageing, including dynamin and clathrin (Alsaqati et al., 2018).
Huntington's Disease (HD) is a genetic neurodegenerative disease with main symptoms including dementia, ataxia and chorea. The disease is caused by a mutation in the huntingtin (HTT) gene, resulting in dysfunction of the huntingtin protein (Htt) leading to specific degeneration of spatial medium spiny neuron, causing psychiatric, motor and cognitive symptoms in addition to disturbances in the mitochondrial electron transport chain (Zeviani and Carelli, 2005; Bates et al., 2015). Genetic studies have shown a link between Htt and CME (Harjes and Wanker, 2003; Zeviani and Carelli, 2005; Singh et al., 2017; McAdam et al., 2020). The aggregation of the mutated Htt protein has been shown to cause inhibition of CME, inhibiting the internalisation of receptors and recycling of SVs affecting neuronal function (Yu et al., 2014). Htt has been observed to interact with a range of proteins implicated in SVR, specifically clathrin-mediated SVR. The Htt aggregate sequesters HSC70, an essential protein in CME, specifically in the uncoating process, rendering it unable to act in this process and inhibiting endocytosis (Yu et al., 2014). Htt has additionally been shown to interact directly with AP-2, and is involved in recruitment of AP-2 to the membrane. A loss of this docking function, due to mutation of the proteins results in reduced recruitment of AP-2 and impairment of CME.
Charcot Marie Tooth (CMT) disease is one of the most common inherited neurological diseases affecting 1 in 2,500 people. Symptoms include muscle weakness and foot ulcers, often causing infection, due to the characteristic impaired sensory and motor neuronal functions (Szigeti and Lupski, 2009; Singh et al., 2017). CMT has been linked to mutations of intracellular trafficking related genes, specifically in the PHD of dynamin 2 (Durieux et al., 2010).
Similarly, autosomal-dominant Centronuclear myopathy (CNM), an inherited neuromuscular disorder, has been speculated to result from mutations in dynamin 2 (Bitoun et al., 2005; Jungbluth et al., 2008). CNM is characterised by delayed motor milestones, muscle weakness, progressive muscle wasting, cognitive impairment, extraocular muscle palsy and frequently limited eye movement (Bitoun et al., 2005; Jungbluth et al., 2008; Sidiropoulos et al., 2012; Singh et al., 2017). Currently there is no effective treatment available, however DNM2 (dynamin gene) knockdowns have been shown to improve muscle mass and restore muscle structure (Buono et al., 2018). This led to investigation of dynamin 2 knockdown mediated by antisense oligonucleotide (ASOs), the lead candidate (developed by DynaCure) is currently undergoing human trials. Mice studies showed the ASO knockdown resulted in a reduction of dynamin 2 levels in muscle tissue and prevented myopathy from developing, while ASO treatment of affected mice lead to the correction of muscle defects (Tasfaout et al., 2017).
Parkinson's Disease (PD) is an age-dependent neurodegenerative disorder associated with tremors, slow movement, sleep disturbances, cognitive difficulties and depression (Inoshita and Imai, 2015; Singh et al., 2017). These symptoms occur as a result of dopamine depletion and loss of dopamine neurons in the mid brain. Although the specific molecular mechanism that causes the neurodegeneration is unclear, recent developments have indicated that many of the causative PD genes are involved in regulation of vesicular trafficking, including SVR (Inoshita and Imai, 2015). For example, mutations of an E3 ubiquitin protein ligase, Parkin, results in early onset autosomal recessive PD. Association between Parkin and endophilin, the endocytotic protein that binds and directs dynamin to the necks of CCVs, indicated the possible role of Parkin in regulation SVR and pathogenesis of PD. Late onset autosomal dominant PD is believed to be associated with a mutation in leucine-rich repeat kinase 2 (LRRK2). LRRK2 also interacts with endophilin, and is involved in regulation of SV dynamics, with additional links to dynamin (Inoshita and Imai, 2015; Singh et al., 2017).
Affecting ~50 million people worldwide, epilepsy is a central nervous system disorder with spontaneous seizures as the main symptom (Li et al., 2015; Vannini et al., 2020). In lesional epilepsy, seizures develop in response to brain damage (Pitkänen and Immonen, 2014), while in non-lesional epilepsy they are the result of altered synaptic function (Farisello et al., 2013; Vannini et al., 2020). These seizures are caused by high frequency, synchronous and uncontrolled synaptic transmission in the brain (Li et al., 2015; Vannini et al., 2020). SVR plays a clear role in this abnormal synaptic transmission by maintaining the neurotransmission of the central synapses (Li et al., 2015).
Due to their role in SVR, CME and its components have implications in altered synaptic function. In addition there is significant evidence of dynamins role in epileptic seizures (Anggono et al., 2006; Appenzeller et al., 2014). Mutations in dynamin 1 have been observed to cause epileptic symptoms, while in both animal models and human epilepsy dynamin 1 is upregulated and may contribute to the development of seizures, with dynamin inhibition, by the small molecule inhibitor Dynasore, exhibiting anti-epileptic effects (Appenzeller et al., 2014; Li et al., 2015; Vannini et al., 2020). Moreover the clinically used levetiracetam (KeppraTM) is believed to act directly at synaptic vessel protein 2 (SV2A) (Lynch et al., 2004; Klein et al., 2020; Contreras-García et al., 2021).
Affecting ~0.5–1.0% of the worldwide population, schizophrenia is a debilitating neuropsychiatric disorder characterised by a breakdown in thinking, attributed to defects in synaptic transmission. Several schizophrenia-susceptibility genes have been identified, including dysbindin (dystrobrecin-binding protein 1) (Chen et al., 2008). This gene is known to effect neurotransmission, resulting in the cognitive dysfunctions associated with the disorder and is also believed to effect hippocampal dopamine levels. Dysbindin is involved in processes that are closely related CME (Chen et al., 2008; Schubert et al., 2012). Additionally, key aspects of the neuropathology, such as white matter changes, synaptic dysfunction and abnormal neurodevelopment are all influenced by CME and clathrin dependent processes (Schubert et al., 2012). The phenothiazine-based antipsychotic drugs, such as chlorpromazine, are now known to strongly inhibit CME, via inhibition of dynamin (Daniel et al., 2015). First and second generation antipsychotics are also known to interact with β-arrestin, another clathrin interacting protein involved in CME (Masri et al., 2008; Schubert et al., 2012; Singh et al., 2017).
Currently there are no available treatments to cure neurodegenerative diseases, with treatments aiming to support patients, manage symptoms and halt disease progression (Durães et al., 2018; Overcoming Gaps in the Treatment of Neurodegenerative Disease, 2020). Drug development in this area has focused primarily on postsynaptic targets and G-protein coupled receptors, leaving the investigation of endocytic machinery as potential drug targets an underexplored, but promising avenue.
The inhibition of CME through genetic and chemical means has been used to explore and reveal the molecular components required for, and also the consequences of, blocking CME. Studies into the inhibition of CME have highlighted its potential medical importance, e.g., in the entry of bacterial and viral pathogens into cells (Dutta and Donaldson, 2012), psychiatric disorders (schizophrenia and bipolar disorder) (Schubert et al., 2012) and neurodegenerative diseases [e.g., Parkinson's (Vidyadhara et al., 2019) and Alzheimer's disease] (Alsaqati et al., 2018). Viral pathogens, such as the influenza virus (Yang et al., 2011), Ebola virus (Bhattacharyya et al., 2010), African Swine fever virus (ASFV) (Hernaez and Alonso, 2010), Simian Haemorrhagic fever virus (Caı̀ et al., 2015), HIV (Aggarwal et al., 2017), and Semliki Forest virus (SFV) (Doxsey et al., 1987) have been found to hijack CME to enter cells (Harper et al., 2013). Also known to enter via CME are some bacterial pathogens and yeast including Staphylococcus aureus (Veiga et al., 2010) and uropathogenic Escherichia coli (Eto et al., 2008), and Toxins such as Anthrax toxin (from Bacillus anthracis) (Abrami et al., 2010) and diphtheria toxin (from Corynebacterium diphtheria) (Skretting et al., 1999; Harper et al., 2013). As CME is one of the major endocytic pathways involved in SVR, investigation of mechanisms of inhibition of CME may provide understanding of the synapse function along with the potential of therapeutic targets, improving knowledge of neurodegenerative processes. CME machinery is a promising target for development of new therapeutics for neurodegenerative disorders (Konno et al., 2012; Schubert et al., 2012; Oliver and Reddy, 2019), specifically this section will focus on inhibitors of dynamin and clathrin, along with general CME inhibitors.
Classical inhibition of CME includes environmental stimuli, which act as general cellular perturbants, such as cytosolic acidification, potassium depletion, and hypertonic treatment. These methods, while gleaning information into the endocytic process, also effect many non-clathrin mediated pathways as well as many non-endocytic processes resulting in unknown global effects on the cells (Dutta and Donaldson, 2012).
Hypertonic sucrose (0.4–0.5 M) results in the dispersion of regular clathrin lattices and the formation of microcages (Carpentier et al., 1989; Ivanov, 2008), but has additionally be found to interfere with all three major internalisation pathways and cause cell shrinkage (Ivanov, 2008; Dutta and Donaldson, 2012). Potassium ion (K+) depletion is another classical method of CME inhibition. Once K+ reached a critical level in cells, the number of clathrin coated pits is reduced by ~80%, and CME rate is decreased by 70–95% (Carpentier et al., 1989; Ivanov, 2008). This is believed to be cause by aggregation of clathrin in the cytoplasm, and although is thought to be more selective to clathrin dependent endocytic pathways (Ivanov, 2008). A range of side effects are observed including reduced protein and DNA synthesis (Dutta and Donaldson, 2012). Similarity, cytosol acidification is observed to cause a range of side effects, however its mode of action against CME differs, the clathrin coated vesicles are “frozen” at the cell surface when the pH is <6.5 (Cosson et al., 1989; Dutta and Donaldson, 2012).
There have also been genetic approaches employed to inhibit CME by altering the expression of specific protein, such as using the expression of mutants or siRNA-mediated depletion of proteins. Expression of a mutant form of dynamin (K44A, patterned after a temperature sensitive mutant in Drosophila) has been shown to inhibit CME, however this also resulted in an increased the rate of clathrin-independent fluid endocytosis (Van der Bliek et al., 1993; Damke et al., 1994). Additional studies show expression of the CTD of clathrin, the carboxyl-terminal clathrin binding domain of AP180 (AP180C) or a truncated from of Eps15 (lacking the epsin homology domain) are all able to inhibit CME (Benmerah et al., 1998; Liu et al., 1998; Zhao et al., 2001). Unfortunately overexpression of proteins (wild-type and mutants) is believed to lead to a range of possible indirect off target effects (Dutta and Donaldson, 2012).
Another approach is the knockdown of proteins by siRNA (e.g., siRNA of clathrin has been shown to block the formation of CCPs) (Hinrichsen et al., 2003), however the considerable time (3–7 days) required to deplete cells, and the cell adaptions and altered gene expression possible in this time are significant drawbacks to this approach (Motley et al., 2003; Dutta and Donaldson, 2012). These drawbacks mean it is difficult to ensure CME is the only impacted pathway. The “knock-sideways” approach was developed to circumvent some of these drawbacks, and involved the translocation of clathrin (to the mitochondria) to deplete cells of clathrin, although there are concerns of the diversion of other proteins in the process, resulting in off-target effects (Robinson et al., 2010; Dutta and Donaldson, 2012).
Although genetic approaches are useful for studies into the understanding of mechanisms of CME, these also resulted in a range of side effects and are not methods that can be readily translated to a therapeutic use.
Firstly, a note on the use of small molecule inhibitors. All small molecules have potential off-target effects, even those published as specific inhibitors (Hopkins, 2008; Hafner et al., 2019; Oña and Bouso, 2020). To ameliorate these potential effects and to strengthen evidence associated with the observed phenotypes, that are ascribed to the inhibition of the target protein, it is strongly recommended (a) that more than one chemical scaffold that inhibits the target protein is used in the biochemical study (it is unlikely that two (or more) chemical scaffolds will display the same off target effects); and (b) inactive control compounds using as close a structural analogue as possible is used (chemically similar but with no in vitro target efficacy). Combined these approaches can significantly improved target validation. A listing of the known dynamin and clathrin inhibitors with inhibition data (where published) is provided in Table 2.
Monodansylcadaverine (MDC, 1) (Figure 7) is known to stabilise CCV to uncoating and inhibit CME. MDC (1) displays a range of side effects believed to be due to its inhibitory action against enzymes of the transglutaminase family, which may affect the organisation and dynamics of the actin cytoskeleton. Some studies have also observed inhibition of macropinocytosis and phagocytosis by MDC (1) (Schlegel et al., 1982; Kang et al., 1995; Singh et al., 2003; Mishra and Murphy, 2004). Similar to MDC (1), chloroquine (2) and phenothiazine (3) (Figure 7) are hydrophobic amines which inhibit CME via affecting the function and formation of CCVs (Dutta and Donaldson, 2012). The modes of action of MDC (1) and chloroquine (2) are largely unknown; however, phenothiazine (3) has been shown to block dynamin (Daniel et al., 2015; Chew et al., 2020). These compounds have been observed to exhibit off target effects and non-specificity to clathrin dependent endocytic pathways (Chen et al., 2009; Dutta and Donaldson, 2012).
Figure 7. Structures of small molecules CME inhibitors; monodansylcadaverine (MDC, 1), chloroquine (2), phenothiazine (3), Phenylarsine oxide (PAO, 4), Ikarugamycin (5), and bolinaquinone (6).
Phenylarsine oxide (PAO, 4) inhibits CME at 1–20 μM, via an unknown mechanism (Figure 7). PAO (4) exhibits a range of serious side effects, which result in the disorganisation of action cytoskeleton, along with non-specific as inhibition of macropinocytosis and phagocytosis (Francesco Retta et al., 1996; Gerhard et al., 2003; Singh et al., 2003).
Ikarugamycin (5) (Figure 7) displays broad-spectrum antibiotic and antiprotozoal activity (Jomon et al., 1972). It was subsequently found to be cytotoxic (IC50 = 0.22 μM, HL-60 cells) (Popescu et al., 2011). Ikarugamycin (5) reversibly inhibits transferrin endocytosis in H1299 cells (IC50 of 2.7 ± 0.3 μM) (Hasumi et al., 1992; Elkin et al., 2016). Comparable activity is conserved across multiple human cell lines (H1299, HCC366, H1437, ARPE-19, and HBEC3KT), and Ikarugamycin (5) showed no inhibition of albumin uptake [via Caveolae-Mediated Endocytosis (CavME)] or CD44 and CD59 uptake [via clathrin independent endocytosis (CIE)] over a 1–4 μM concentration range (Elkin et al., 2016). However, despite the promising indication of CME selectivity of Ikarugamycin (5), cytotoxicity is observed at concentration below CME activity and the mode of action is currently unknown (Elkin et al., 2016).
The clinical use of small molecules, drugs, is a major goal in therapeutic interventions. However, prior to attaining this outcome, it is often essential to validate the target-disease nexus and the therapeutic suitability of the proposed approaches. In this regard, the development of probe molecule is often the first step in this process. These probe molecules may be the ultimate drug candidate, but it is more common that these molecules are used in the initial target validation steps. They are most often not regarded as drugs. During this interrogation of a pathway it is generally considered prudent that multiple probes (and inactive controls as described above) be used in confirming (or not) the expected phenotype from inhibiting the target protein. The current palette of inhibitors targeting clathrin and dynamin are best described as early-stage probe molecules.
The marine sesquiterpene hydroxyquinone, bolinaquinone (6) (Figure 7) (De Guzman et al., 1998), was identified as a possible clathrin inhibitor in a proteomics experiment. The selective pull-down of clathrin after attachment to agarose beads at the methoxy position identified (6) as a clathrin inhibitor (Margarucci et al., 2011). Initial studies showed in vitro cytotoxicity against a human colon cell line and broad spectrum anti-inflammatory effect (Lucas et al., 2003; Petronzi et al., 2011). Albumin internalisation studies on THP1 (human acute lymphoma) additionally validate the CME activity of (6), which was found to be non-cytotoxic at concentrations <100 μm (Margarucci et al., 2011). A computational docking study investigated the possible CTD binding site of bolinaquinone (6), leading to the identification of a proposed new fifth site between blades 5 and 6.
Endosidin 9 (ES9, 7) (Figure 8E) is a known endocytic inhibitor and inhibits the uptake of a tracer dye (FM4-64) in Arabidopsis root epidermal cells (IC50 = 5 μM). As ES9 (7) is a protonophone, inhibition may be the result of cytosolic acidification, however it is believed the activity may be structure driven as CME activity is still observed at high apoplectic pH (Dejonghe et al., 2019). ES9 (7) led to the synthesis of several related analogues including a non-protonophore lead; ES9-17 (8). In silico modelling studies led to the proposal that ES9 (7) and ES9-17 (8) bind in site 1 of the CTD (Figure 8A). This binding site was confirmed by the determination of the CTD co-crystal with ES9 (7), indicating it is likely ES9-17 (8) is binding at site 1 also (Figure 8B). ES9-17 (8) effectively inhibits CME in Arabidopsis, and to date is the only known small molecule inhibitor allowing reversible inhibition of CME in Arabidopsis. ES9-17 (8) was also found to inhibit transferrin uptake in human cells (HeLa cells) after treatment with 30 μM, however at this stage there is no reported IC50 (Dejonghe et al., 2019).
Figure 8. Binding site and structures of small molecule clathrin inhibitors. (A) Top view of the CTD, showing Site 1 (in yellow) the binding site of ES9 (7), ES9-17 (8), Pitstop® 1 (9), and Pitstop® 2 (10) (Ter Haar et al., 1998). (B) ES9 in site 1 of the CTD (Dejonghe et al., 2019). (C) Pitstop® 1 in site 1 of the CTD. (D) Pitstop® 2 in site 1 of the CTD (Von Kleist et al., 2011). (E) Chemical structures of ES9 (7), ES9-17 (8), Pitstop® 1 (9), and Pitstop® 2 (10).
The Pitstop® series of compounds were identified via screening of a library of ~17,000 small molecules, leading to the synthesis of two structurally distinct leads. Subsequent focused library development and screening afforded Pitstop® 1 (9) and Pitstop® 2 (10) (Von Kleist et al., 2011; Robertson et al., 2016) (Figure 8E). These compounds have been found to inhibit the CTD and formation of clathrin coated pits, which in turn inhibited the CME entry of HIV and also interfered with SVR (Von Kleist et al., 2011). Although showing inhibition of transferrin uptake Pitstop® 1 (9) (IC50 = 18 μm, CME) possess limited cellular uptake, while Pitstop® 2 (10) exhibits cell permeability and inhibits transferrin uptake into HeLa cells (IC50 = 12–15 μm) (Smith et al., 2013). X-ray co-crystals of the Pitstop®–CTD complex revealed that although structurally different they both bind at Site-1 between blades 1 and 2 lying off-set relative to each other in a hydrophobic cavity comprising I52, I62, I80, I93, L82, and F91 (Figures 8A,C,D) (Von Kleist et al., 2011).
There has been some speculation about the specificity and mode of action of Pitstop® 2 (10). Previous results from knockout studies have observed the ability of CME to function with any one site functional (Lemmon and Traub, 2012). This has raised the question of how inhibition at the one known binding site of Pitstop® 2 (10) results in CME inhibition. Additionally, it has been demonstrated with various point mutation on the CTD that Pitstop® 2's inhibitory action was unhindered. Notable studies with a C+ mutant [which altered the key residues involved in the clathrin box site (site 1)] found Pitstop® 2 (10) activity to be conserved. It is thus possible that Pitstop® 2 (10) is able to bind at all sites on the CTD, which would explain the inhibition of CME (Elferink, 1979; Lemmon and Traub, 2012; Willox and Royle, 2012), or there is a second site of Pitstop® 2 (10) action. However, molecular docking studies performed with the altered sites alongside the existing Pitstop® 2/CTD co-crystal postulate that these altered amino acids are not involved in binding at all, and thus would not be sufficient to prevent Pitstop® 2 (10) inhibition but may in fact result in increased binding affinity (Robertson et al., 2016). Another study suggested the non-specificity of Pitstop® 2 (10) due to the observed inhibition of MHCI (major histocompatibility complex I) internalisation into HeLa cells, believed to occur via a clathrin-independent endocytosis (CIE) (Dutta et al., 2012; Willox et al., 2014). However, AP-2 μ2 and CHC knockdown studies also resulted in reduced entry to cells, confirming the MHCI pathway is clathrin/AP-2 dependent (Stahlschmidt et al., 2014). The specificity is further reinforced when observing Shiga toxin entry, a Clathrin independent process, of which Pitstop® 2 (10) provides no inhibition (Von Kleist et al., 2011; Dutta et al., 2012).
Overexpression of the CTD results in CME inhibition, shown using a CTD and distal leg (TDD) construct, which resulted in decreased transferrin uptake and additionally showed no significant inhibition of CIE (CD44 and CD59 uptake) (Chen et al., 2020). Overexpression of the TDD inhibited initiation and stabilisation of CCPs and blocked late-stage maturation of formed CCPs by interfering with the clathrin-AP-2 and clathrin-SNX9 interactions. The overexpressed terminal domain (TDD) is not incorporated into the clathrin/AP-2 coat, but rather binds AP-2 and SNX9, sequestering these proteins from normal CME functions, corresponding to an increase in the observation of AP-2 deficient CCP formation (Chen et al., 2020). This led to the design of a series of peptides encoding the key residues of each binding site (Chen et al., 2020). Two of these, Cbox2 and Wbox2, substantially and reversibly inhibited CME. Of the peptides designed, Cbox2 and Wbox2 were the only that encompassed the entire bonding motif, by taking into account discontinuous residues on adjacent blades (Figure 9). Of the peptides, Wbox2 showed the greatest inhibition (IC50 ~ 3 μM, Tf uptake in ARPE19/HPV16 cells), which was attributed to AP-2 and SNX9 binding following pulldown studies (Chen et al., 2020). However, Wbox2 was non-specific and found to inhibit clathrin independent endocytosis (CD44 and CD59 uptake) proposed to be due to either the upregulation of CIE during CTD overexpression or that SNX9 is required for CIE, and inhibition by Wbox2 sequesters it from CIE (Chen et al., 2020).
Figure 9. (A) Top view of the CTD, showing the binding sites of interest; Site 1 (clathrin box) in yellow and Site 2 (W-box) in orange (Ter Haar et al., 1998). (B) Amino acid sequences of Cbox2 (red) and Wbox2 (orange) shown on the CTD (Chen et al., 2020). (C) Peptide sequence of Cbox2 and Wbox2, TAT (transactivator of transcription) sequence in black and CTD derived amino acids in red. Bold and underlined are the key resides (corresponding to the key residues of Site 1 and 2) (Chen et al., 2020).
A primary screen of lipid like compounds resulted in the first class of compounds to be described as specific dynamin inhibitors (Hill et al., 2004). Originally long chain primary amines, it was predicted the hydrophobic molecular may potentially inhibit dynamin through interaction with the PH domain (Hill et al., 2004). The corresponding ammonium salts, octadecyl trimethyl ammonium bromide (OcTMAB, 11) and myristyl trimethyl ammonium bromide (MiTMAB, 12) (Figure 10A) were found to be the most potent with dynamin 1 IC50 values of 1.9 ± 0.24 μM and 3.1 ± 0.2 μM, respectively (Hill et al., 2004; Quan et al., 2007). Studies found these compounds disrupted the interaction of dynamin 1 with lipids, affecting the stimulation of GTPase activity (Quan et al., 2007). MiTMAB (12) was found to compete with PS binding to the PH domain of dynamin, as inhibition was not observed when using a recombinant dynamin 1 lacking the PH domain (dynamin 1-ΔPH). Additionally a sedimentation assay revealed MiTMAB (12) did not affect the self-assembly of dynamin (Quan et al., 2007). Cell based assays confirmed the inhibitory activity, specifically internalisation of transferrin and epidermal growth factor (EFG) into HeLa, Her14, A431, and COS-7 cells, mediated by dynamin 2 along with SVE inhibition mediated by dynamin as observed via styryl dye uptake measurements and synaptic vesicle depletion measurements (by electron microscopy) (Quan et al., 2007; Hill et al., 2010). These compounds also resulted in a significant reduction in the proliferation and viability of cancer cells, across a diverse panel of cell lines (Hopkins, 2008; Robinson et al., 2010).
Figure 10. Structures of, (A) long chain ammonium salt dynamin inhibitors OcTMAB (11), MiTMAB (12) and RTIL-13 (13); and (B) anti-psychotics and Selective Seretonin Reuptake as dynamin inhibitors; chlorpromazine (14), fluvoxamine (15), sertraline (16), citalopram (17), trifluoperazine (18), haloperidol (19), and clozapine (20).
While developing new RTILs for the solubilisation of the dynamin PH domain for dynamic combination chemistry (DCC) assembly reactions, screening for potential dynamin inhibitors found RTIL-13 [4-(N,N-dimethyl-N-octadecyl-N-ethyl)-4-aza-10-oxatricyclo[5.2.1]decane-3,5-dione bromide, 13], with an IC50 of 2.3 ± 0.3 μM (Figure 10A). The presence of an alkyl ammonium group revealed the structural similarity to MiTMAB (12) and led to the proposal of a similar mode of action, however this is currently not confirmed (Zhang et al., 2008; Eschenburg and Reubold, 2018).
Chlorpromazine (14) (Figure 10B), used to treat schizophrenia, inhibits CME originally thought to be due to inhibition of the clathirn-AP-2 complex (Wang et al., 1993). However, chlorpromazine's CME inhibition (14) occurs via dynamin inhibition (Daniel et al., 2015). The surfactant nature of MiTMAB (12) (a cationic surfactant at high concentrations) is shared with some pharmacologically active cationic amphiphilic compounds. Paired with the identification of chlorpromazine (14) as a CME inhibitor, this led to investigation of the inhibitory activity of a panel of selective serotonin reuptake inhibitors (SSRIs) and antidepressants against dynamin GTPase. The IC50 values of SSRI's were compared to the value obtained for MiTMAB (12) in this study, which gave an IC50 of 24.1 ± 9.4 μM (Otomo et al., 2008). The value was significantly less potent than the previously reported 3.1 ± 0.2 μM (Hill et al., 2004; Quan et al., 2007), a discrepancy believed to be observed due to the use of Dyn-His6 GTPase (from E. coli) in this study (Otomo et al., 2008) compared to native dynamin 1 (from sheep brain) (Hill et al., 2004; Quan et al., 2007). Two SSRI's displayed inhibitory effects greater that MiTMAB (12) [IC50 24.1 ± 9.4 μM, against Dyn-His6 GTPase (from E. coli)]; fluvoxamine (15) (IC50 14.7 ± 1.6 μM) and sertraline (16) (IC50 7.3 ± 1.0 μM) (Otomo et al., 2008) (Figure 10B). Sertraline (16), along with chlorpromazine (14), blocked transferrin and cholera toxin subunit (CTB) endocytosis in HeLa cells. The related SSRI, citalopram (17), had no effect on endocytosis (Figure 10B) (Takahashi et al., 2010). The clinical efficacy of SSRI's means that these compounds are normally dosed at concentrations <100 time that required for in vitro CME inhibition. Caution is thus required even in using these SSRI's as probes to examine dynamin (and CME) inhibition. Given the potency differential, this would feasible only if the resultant phenotype was confirmed through the use of other dynamin inhibitors and the appropriate inactive control compounds. Realistically, though, the use of SSRI's to interrogate dynamin and CME is not encouraged.
The dynamin GTPase activity of a range of anti-psychotic drugs (APDs), both typical and atypical, were investigated. Typical APDs include chlorpromazine (14) and other phenothiazine derived compounds. All nine clinically used phenothiazine compounds were observed to inhibit dynamin 1 with the most potent being trifluoperazine (18) with an IC50 of 2.6 ± 0.7 μM. These compounds additionally inhibited dynamin 2 with similar IC50 values. Atypical APDs represent a more structurally diverse class of compounds of which five were investigated. Of these haloperidol (19) and clozapine (20) (Figure 10B), displayed improved potency for dynamin 2 (IC50 = 6.5 μM and 5.3 μM, respectively), over dynamin 1 (IC50 = 19.0 ± 2.2 μM and 28.2 ± 1.2 μM, respectively) with the remaining showing no activity (Daniel et al., 2015). Many of these compounds are highly non-specific, with activity against dopamine receptors (Ban, 2007) and phagocytosis (Elferink, 1979) among others, indicating multiple off target effects.
Bis-T (21) (Figure 11) is the most potent of the dimeric tryphostins (tyrosine phosphorylation inhibitors) reported as dynamin inhibitors, with an IC50 of 1.7 ± 0.2 μM (Hill et al., 2005). Structural modification to replace the nitrile group with a more drug like isostere, gave the Iminodyns, the first sub-micromolar potent dynamin inhibitors (Hill et al., 2010). The five most potent in this series were investigated further. Imidodyn 22 (23) inhibited the endocytosis (CME) of Texas Red-Transferrin (Tf-TxR) in human bone osteosarcoma epithelial cells (U2OS), with an IC50 of 10.7 ± 4.5 μM (Hill et al., 2010). Significant SVR inhibition of styryl dye FM4-64 uptake, with Iminodyn 21 (22), 22 (23) and 23 (24) (Figure 11) was noted, with Iminodyn 23 (24) the most potent (IC50 40.4 ± 08 μM). Michaelis-Menten kinetics suggested that Iminodyn 22 (23) is an uncompetitive inhibitor of dynamin 1 (Hill et al., 2010). Iminodyn 22 (23) has since been used as a tool to investigate the role of dynamin in a range of processes, including elucidating new dynamin interactions [e.g., Nitric oxide synthase 1 (NOS1β)], dynamins role in phagosome scission and F-actin (filamentous) assembly and in the investigation viral cellular entry (dengue virus in macrophages) (Ayala-Nunez et al., 2016; Hyndman et al., 2016; Marie-Anaïs et al., 2016; Muranen et al., 2017).
Figure 11. Structure of Bis-T (21) Iminodyn 21 (22), Iminodyn 22 (23), Iminodyn 23 (24), Dynasore (25), Dyngo 4a® (26), Dyngo 6a® (27), Rhodadyn A1 (28), Rhodadyn D10 (29), Rhodadyn C10 (30), Pthaladyn 23 (31), Naphthaladyn 29 (32), and Quinone 45 (33). The common N,N-dimethyl-3-phenoxypropan-1-amine head group of Rhodadyn D10 (29) and Rhodadyn C10 (30) is shown in red.
Macia et al identified Dynasore (25) (Figure 11), as a fast-acting, cell permeable, non-competitive small molecule inhibitor of dynamin 1 and 2 (Macia et al., 2006). The mechanism of action is unknown, Dynasore (25) did not affect GTP binding, dynamin self-assembly or oligomerisation but did disrupt dynamin dependent endocytic functions of the cell. Dynasore (25) arrested the formation of clathrin coated pits and vesicles and has since been used as a chemical probe to analyse the effect of dynamin on endocytosis in cells and inhibitory effects of dynamin in vitro (Kirchhausen et al., 2008). Unfortunately, Dynasore (25) was limited by non-specific binding properties. Binding to serum proteins resulted in a loss of inhibition. Further Dynasore (25) binds to trace levels of detergents, such as Tween-80, which are commonly used in biological assays (Mccluskey et al., 2013).
McCluskey et al. developed a second generation library of Dynasore (25) analogues with the objective to reduce non-specific binding and cytotoxicity. Dyngo 4a® (26) and Dyngo 6a® (27) (Figure 11), dynamin 1 IC50s of 0.38 ± 0.05 μM and 3.2 ± 0.3 μM, respectively [~30 times more potent than Dynasore (25) which has a dynamin 1 IC50 of 12.4 ± 1.5 μM], with reduced non-specific binding and cytotoxicity relative to Dynasore (Mccluskey et al., 2013). Unlike Dynasore (25), these compounds did not display off target protein-protein interactions with amphiphysin/clathrin or AP-2 and displayed a preference for the helical assembled dynamin, although there is still some debate regarding the specificity of the dyngo compounds (Park et al., 2013; Basagiannis et al., 2017; Persaud et al., 2018). This can, in part be off-set through the use of the inactive control compound, Dyngo-ϕ. Dyngo 4a® (26) inhibits SVE and activity-dependent bulk endocytosis (ADBE) at presynaptic nerve terminal (Mccluskey et al., 2013).
Selective optimization of the side activities of a lead from the Pitstop® 2 (10) family afforded the rhodadyns as dynamin inhibitors (no clathrin inhibition off-target activity). Focused library development based on Rhodadyn A1 (28) (dynamin 1 IC50 134 ± 21 μM), resulted in 13 analogues with dynamin 1 IC50s of ≤ 10 μM (dynamin 1) (Robertson et al., 2012). CME inhibition was also analysed using Texas red uptake assay. The most potent, Rhodadyn D10 (29), blocked Texas red uptake in U2OS cells with an IC50 of 5.9 ± 1.0 μM (dynamin 1 IC50 4.5 ± 0.8 μM). Common to the two most potent analogues [Rhodadyn C10 (30) and D10 (29) (Figure 11)] and to the dynole and pyrimidine series of compounds was the N,N-dimethyl-3-aminopropan-1-amine head group both contained (shown in red in Figure 11) (Rhodadyn C10 (30) dynamin 1 IC50 7.1 ± 1.0 μM). The introduction of a terminal carboxylate moiety abolished CME activity (Robertson et al., 2012).
The Naphthaladyns and Pthaladyns were the first classes of dynamin inhibitor targeting the GTPase domain to be rationally designed. The Pthaladyns were developed from virtual screening lead of a homology model of the human dynamin 1 GTPase domain (Odell et al., 2010; Macgregor et al., 2014a; Abdel-Hamid et al., 2015). No pthaladyn-based dynamin 1 inhibitor blocked CME. This was attributed to poor cell permeability or their rapid degradation (Odell et al., 2010). Pthaladyn 23 (31) did return an SVE IC50 of 12.9 ± 5.9 μM (FM4-64 uptake in synaptosomes), similar to the dynamin 1 inhibition observed for this compound (IC50 = 17.4 ± 5.8 μM) (Odell et al., 2010). However, in an assay examining the effects of pthaladyn 23 (31) in synaptosomes, an IC50(SVE) of 743 μM was observed (Daniel et al., 2012).
Modelling led side activity optimization of the 1,8-naphthalimide scaffold found in the Pitstop® 1 (9) family, via imide modification resulted in the development of a library of Naphthaladyns (Macgregor et al., 2014a; Abdel-Hamid et al., 2015). Naphthaladyn 29 (32) (Figure 11), displayed a dynamin 1 IC50 of 18.5 ± 1.7 μM, a CME IC50 of 66 μm (inhibition of Tf-a594 internalisation in U2OS cells) inhibition of internalisation and was GTP domain competitive (Abdel-Hamid et al., 2015).
Also identified by in silico screening, as with the Pthaladyns, the Quinodyns arose from the same in silico library screening of the human dynamin GTPase domain model that afforded the Pthaladyns. Dynamin activity was confirmed using a minimal dynamin construct (containing the GTPase domain and BSE) (Chappie et al., 2011; Macgregor et al., 2014b). Quinone 45 (33) (Figure 11) inhibited GTPase dynamin 1 (IC50 of 11.1 ± 3.6 μm) and inhibited transferrin uptake with an IC50 of 36 ± 16 μm (Macgregor et al., 2014b).
A small library of substituted pyrimidines, the Pyrimidyns, were designed based on library screening and resulted in the identification of Pyrimidyn 1 (34) as a modest dynamin 1 inhibitor (IC50 35.3 ± 7.1 μM) (McGeachie et al., 2013). The two most potent compounds from subsequent focused library development showed a 30-fold increase in dynamin 1 inhibition, with Pyrimidyn 6 (35) and Pyrimidyn 7 (36) (IC50s of 1.4 ± 0.2 μM and 1.1 ± 0.05 μM, respectively) (Figure 12) (McGeachie et al., 2013). Both Pyrimidyn 6 (35) and Pyrimidyn 7 (36) were found to obstruct interaction of dynamin with phospholipids, and also the binding of GTP to dynamin concluding that they target two different domains simultaneously, the GTPase and PH domain, via a dual mechanism of action. Pyrimidyn 6 (35) and Pyrimidyn 7 (36) were also found to have a potent inhibitory action against CME with IC50 values of 19.3 ± 3.5 μM and 12.1 ± 2.1 μM, respectively (McGeachie et al., 2013).
Figure 12. Structures of Pyrimidyn 1 (34), Pyrimidyn 6 (35), Pyrimidyn 7 (36), Dynole 34-1™ (37), Dynole 34-2™ (38), dynole 24 (39), and dynole 25 (40) are shown.
The Dynoles™ are a class of dynamin inhibitors, designed by structural simplification of the lead, staurosporine. This resulted in structures with a single indole and an alkyl dimethyl amino moiety. This initial library produced only moderate dynamin 1 inhibitors, with the introduction of a C-3 alkyl chain yielding a 10–30-fold potency increase (Hill et al., 2009). The most active compound in the series, Dynole 34-1™ (37) (dynamin 1 IC50 = 3.33 ± 0.75 μM) was highly lipophilic (Figure 12). It was hypothesised that removal of the C2-methyl of Dynole 34-1™ (37) would result a more favourable LogP, while retaining activity. This gave Dynole 34-2™ (38) (Figure 12), with a 3-fold increase in potency (IC50 of 1.30 ± 0.30 μM) (Hill et al., 2009). A second generation of Dynole compounds, elicited two compounds, dynole 24 (39) and dynole 25 (40) (Figure 12) that displayed greatly reduced cell toxicity, with sub-micromolar potency against dynamin 1 (IC50 0.56 ± 0.09 μM and 0.76 ± 0.05 μM, respectively) (Gordon et al., 2013). In-cell testing for CME (mediated by dynamin 2) showed compound dynole 24 (39) had an IC50 3 times more potent that Dynole 34-2™ (38) [IC50 of 1.9 ± 0.3 μM for dynole 24 (39) compared to 5.0 ± 0.9 μM for Dynole 34-2™ (38)] (Gordon et al., 2013). Dynole 24 (39) was also found to be 4.4-fold more selective towards dynamin 1 over dynamin 2 (compared to Dynole 34-2™ (38) which displays 2-fold selectivity for dynamin 1) (Gordon et al., 2013).
Bisphosphonates (BPs) are synthetic analogues of phyrophosphate, which can be sub-classified into two classes, depending on the presence or absence of a nitrogen in the structure; non-nitrogen containing BPs (NN-BPs) and nitrogen containing BPs (N-BPs) (Reszka and Rodan, 2003). Some compounds in this class are used as prescriptions drugs against the loss of bone mass arising from a range of medical conditions (Maraka and Kennel, 2015). N-BPs, such as alendronate (41), zoledronate (42), risedronate (43), and ibandronate (44) are the most widely prescribed, however some NN-BPs are also used, including etidronate (45), clodronate (46) and tiludronate (47) (Figure 13) (Maraka and Kennel, 2015). Dynamin 2 was identified as a possible target for BP drugs, and it was determined that three different BPs, alendronate (41), zoledronate (42), and etidronate (45), inhibited the uptake of transferrin as well as adenovirus and simian virus 40 (Masaike et al., 2010). Titration experiments with acidic phospholipids indicated BPs bind to the PH domain, however the proposed mechanism is still debated (Masaike et al., 2010; Eschenburg and Reubold, 2018).
Figure 13. Bisphosphonates (BPs) as dynamin inhibitors. Nitrogen containing BPs (N-BPs); alendronate (41), zoledronate (42), risedronate (43), and ibandronate (44) and Non-nitrogen containing BPs (NN-BPs); etidronate (45), clodronate (46) and tiludronate (47). Alendronate (41), zoledronate (42), and etidronate (45) are believed to inhibited CME via dynamin inhibition.
In terms of clathrin inhibitory potency, the co-crystal structures of Pitstop® 1 and Pitstop® 2 and the clathrin terminal domain enables the identification of the key residues involved in inhibitor binding. The inhibition of the CTD interaction with amphiphysin 2 depends on the disruption of a protein-protein interaction. This more complex than traditional small molecule inhibitor development as the protein-protein interface is typically large and shallow. This is further complicated within site 1—the Pitstop® binding site which is lined predominately with hydrophobic residues: I52, I62, I80, I93, L82 and F91 (Figures 8A,C,D). Most probably, increased binding would most result from increased analogue hydrophobicity, which is counter to the introduction of drug like properties. Alternatively, given that Pitstop® 1 and Pitstop® 2 bind in the same site, but offset to each other, chimeric analogues are possible, but these will still, most likely, suffer from increased hydrophobicity, and in this instance, increased molecular weights again pushing these compounds out with the physicochemical properties associated with drugs.
In terms of drug development, dynamin inhibitors appear to offer greater scope for development. There have been multiple inhibitors across multiple chemical scaffolds and modes of dynamin inhibition reported. Of those reported to date, those targeting the PH domain are probably the least likely to be developed to clinical candidates. Despite CEREP ExpresS screening showing limited problematic off target effects, the wide spread prevalence of PH domains in proteins raises the spectre of off-target effects that would be difficult to design away from and gain dynamin selectivity. The efficacy of APDs against dynamin is typically at a level 100x higher (as is the efficacy of SSRI's) than the primary protein targets, and while a number of these analogues are known to accumulate and can be found at high concentrations, the difference between primary and off-target effect (dynamin) renders their therapeutic use for endocytosis modulation highly questionable. However, it is always important to know that the side-effect severity is associated with the extent of the medical need.
The in vivo efficacy noted with dynole 34-2 suggests that on-going development in this family of compounds may generate a true drug lead, but while tolerated and synergistic with current chemotherapy regimes in mouse models of T-ALL and MAL, the current lack of known binding site within dynamin limits the potential for a rational drug design approach. While efficacious in a cytotoxicity trial, this does not automatically translate to prophylactic or chronic exposure safety and efficacy. The Bis-T and Dyngo series of compounds are also limited the same lack of knowledge with respect to dynamin binding site.
Most promising are those analogues that target the dynamin GTPase domain. As noted this domain is significantly larger than that observed with traditional Ras-like GTPases, as such protein selectivity is theoretically achievable. To date Dynamin GTPase competitive analogues have been reported across three chemical scaffolds: the pthaladyns, Naphthaladyn and Quinodyns. The latter, Quinodyns, contain a promiscuous quinone moiety and while analogues of this nature are clinically used, they are difficult compounds too develop due to their potential off-target effects. The SAR around both the Pthaladyns and Naphthaladyns demonstrate that increased efficacy is feasible. Recent structural reports of the dynamin crystal structure and more importantly the cryo-EM structure of fully assembled helical dynamin permit more accurate rational design and virtual screening programs to be developed. The modelling approach, with the Pthaladyns and Naphthaladyns led the introduction of key structural motifs that saw increases in potency across both scaffolds of 10–20-fold. Activity increases were evident in the introduction of substituted amides and substituted N-imides with the Pthaladyns. The removal of naphthalene substituents and the introduction of aromatic imides with the Naphthaladyns removed clathrin inhibition while enhancing dynamin potency.
In this review, the process of CME has been discussed in order to illuminate the function of two key proteins; clathrin and dynamin. These proteins play essential roles with clathrin forming the being the main component of CCV and CCPs and dynamin required during scission, allowing the formed vesicle to pinch off from the membrane and travel to its destination. Although CME does not account for all SVR, this process does play a key role in the endocytic cycle upon which various neurological conditions rely (e.g., Parkinson's, Alzheimer's, epilepsy, and schizophrenia).
With no treatments currently available to cure neurodegenerative diseases investigating these endocytic processes, focusing on clathrin and dynamin provides new therapeutic targets for neurological diseases. General cellular perturbants along with genetic approaches to clathrin and dynamin inhibitors have been used to provide insight into the mechanisms of pathways, however for therapeutic use novel small molecule inhibitors seem the most auspicious approach. Building on the compounds discussed in this review, the development of new and potent clathrin and dynamin inhibitor is an extremely promising avenue for the development of new therapeutics targeting endocytosis, for treatments of neurological diseases.
A common comment relates to the potency of such analogues, here from sub-micromolar to micromolar and how this potency is not appropriate for their use in a clinical setting. Another is that endocytosis is a critical process, and inhibition by this criteria should be lethal. However, total inhibition of protein function is not the goal of a small molecule, more often these “drugs” seek to modulate protein activity enabling endogenous systems to regain balance, of systems are temporally inhibited only until the affected protein can be regenerated by the host system, e.g., in the case of covalent inhibitors. If this was not the case, protein kinase inhibitors and immunosuppressive agents would not be of clinical value. Moreover, it has been shown that transient endocytosis inhibition by Stemetil is tolerated and elicited a favourable response in ADCC adjuvant treatment with cetuximab in head and neck cancer.
These inhibitors may ultimately lead to the clinical use of endocytosis modulators in humans. While endocytosis is a critical mechanism in maintaining cell and thus host well-being, there is growing evidence that transient modulation is not only feasible but may have positive therapeutic outcomes. Chew et al. (2020) report on blocking CME as an effective strategy to improve the clinical response to antibody dependent cellular cytotoxicity mediating antibodies in humans; Tremblay et al. (2020) report that the dynamin inhibitor, dynole 34-4 is highly synergistic with existing chemotherapy in mouse models of T-ALL and AML; Powell et al. (2021) report that endocytosis inhibition attenuates inflammatory pain like behaviour; and Jensen et al. (2017) also reported on an endocytosis mediated effect as a viable therapeutic target for prolonged pain relief. While these findings should be considered positive, it is prudent to temper the enthusiasm that may be apparent with the realisation that the path to the clinic is long and winding. Multiple drug targets show such early-stage progress, but for a myriad of reasons fail to gain a foothold in a clinical setting. Time, money and extensive future studies will be required to deliver safe and efficacious CME inhibitors as therapeutics.
All authors listed have made a substantial, direct, and intellectual contribution to the work and approved it for publication.
Funding from the Australian Research Council and the National Health and Medical Research Council (Australia) is gratefully acknowledged.
AM has a commercial compound supply agreement with Abcam (UK) which includes some of the compounds mentioned in this article.
The remaining authors declare that the research was conducted in the absence of any commercial or financial relationships that could be construed as a potential conflict of interest.
All claims expressed in this article are solely those of the authors and do not necessarily represent those of their affiliated organizations, or those of the publisher, the editors and the reviewers. Any product that may be evaluated in this article, or claim that may be made by its manufacturer, is not guaranteed or endorsed by the publisher.
Abdel-Hamid, M. K., Macgregor, K. A., Odell, L. R., Chau, N., Mariana, A., Whiting, A., et al. (2015). 1,8-naphthalimide derivatives: new leads against dynamin I GTPase activity. Org. Biomol. Chem. 13, 8016–8028. doi: 10.1039/C5OB00751H
Abdel-Hamid, M. K., and McCluskey, A. (2014). In silico docking, molecular dynamics and binding energy insights into the bolinaquinone-clathrin terminal domain binding site. Molecules 19, 6609–6622. doi: 10.3390/molecules19056609
Abrami, L., Bischofberger, M., Kunz, B., Groux, R., and Van Der Goot, F. G. (2010). Endocytosis of the anthrax toxin is mediated by clathrin, actin and unconventional adaptors. PLoS Pathog. 6:e1000792. doi: 10.1371/journal.ppat.1000792
Aggarwal, A., Hitchen, T. L., Ootes, L., McAllery, S., Wong, A., Nguyen, K., et al. (2017). HIV infection is influenced by dynamin at 3 independent points in the viral life cycle. Traffic 18, 392–410. doi: 10.1111/tra.12481
Alsaqati, M., Thomas, R. S., and Kidd, E. J. (2018). Proteins involved in endocytosis are upregulated by ageing in the normal human brain: implications for the development of Alzheimer's disease. J. Gerontol. Ser. A Biol. Sci. Med. Sci. 73, 289–298. doi: 10.1093/gerona/glx135
Anggono, V., and Robinson, P. J. (2009). “Dynamin,” in Encyclopedia of Neuroscience, eds L. R. Squire. (Amsterdam: Elsevier), 725–735.
Anggono, V., Smillie, K. J., Graham, M. E., Valova, V. A., Cousin, M. A., and Robinson, P. J. (2006). Syndapin I is the phosphorylation-regulated dynamin I partner in synaptic vesicle endocytosis. Nat. Neurosci. 9, 752–760. doi: 10.1038/nn1695
Antonny, B., Burd, C., De Camilli, P., Chen, E., Daumke, O., Faelber, K., et al. (2016). Membrane fission by dynamin: what we know and what we need to know. EMBO J. 35, 2270–2284. doi: 10.15252/embj.201694613
Appenzeller, S., Balling, R., Barisic, N., Baulac, S., Caglayan, H., Craiu, D., et al. (2014). De novo mutations in synaptic transmission genes including DNM1 cause epileptic encephalopathies. Am. J. Hum. Genet. 95, 360–370. doi: 10.1016/j.ajhg.2014.08.013
Avinoam, O., Schorb, M., Beese, C. J., Briggs, J. A. G., and Kaksonen, M. (2015). Endocytic Sites mature by continuous bending and remodeling of the clathrin coat. Science. (80-.). 348, 1369–1372. doi: 10.1126/science.aaa9555
Ayala-Nunez, N. V., Hoornweg, T. E., Van De Pol, D. P. I., Sjollema, K. A., Flipse, J., Van Der Schaar, H. M., et al. (2016). How antibodies alter the cell entry pathway of dengue virus particles in macrophages. Sci. Rep. 6, 1–15. doi: 10.1038/srep28768
Ban, T. A. (2007). Fifty years chlorpromazine: a historical perspective. Neuropsychiatr. Dis. Treat. 3, 495–500. Available online at: https://www.dovepress.com/fifty-years-chlorpromazine-a-historical-perspective-peer-reviewed-article-NDT
Basagiannis, D., Zografou, S., Galanopoulou, K., and Christoforidis, S. (2017). Dynasore impairs VEGFR2 signalling in an endocytosis-independent manner. Sci. Rep. 7, 1–11. doi: 10.1038/srep45035
Bashkirov, P. V., Akimov, S. A., Evseev, A. I., Schmid, S. L., Zimmerberg, J., and Frolov, V. A. (2008). GTPase cycle of dynamin is coupled to membrane squeeze and release, leading to spontaneous fission. Cell 135, 1276–1286. doi: 10.1016/j.cell.2008.11.028
Bates, G. P., Dorsey, R., Gusella, J. F., Hayden, M. R., Kay, C., Leavitt, B. R., et al. (2015). Huntington disease. Nat. Rev. Dis. Prim. 1:15005. doi: 10.1038/nrdp.2015.5
Benmerah, A., Bayrou, M., Carf-Bensussan, N., and Dautry-Varsat, A. (1999). Inhibition of clathrin-coated pit assembly by an Eps15 mutant. J. Cell Sci. 112, 1303–1311. doi: 10.1242/jcs.112.9.1303
Benmerah, A., Lamaze, C., Bègue, B., Schmid, S. L., Dautry-Varsat, A., and Cerf-Bensussan, N. (1998). Ap-2/Eps15 interaction is required for receptor-mediated endocytosis. J. Cell Biol. 140, 1055–1062. doi: 10.1083/jcb.140.5.1055
Bhattacharyya, S., Warfieldc, K. L., Ruthelc, G., Bavaric, S., Amanc, M. J., and Hope, T. J. (2010). Ebola virus uses clathrin mediated endocytosis as an entry pathway. Virology 401, 1–28. doi: 10.1016/j.virol.2010.02.015
Bhave, M., Mettlen, M., Wang, X., and Schmid, S. L. (2020). Early and nonredundant functions of dynamin isoforms in clathrin-mediated endocytosis. Mol. Biol. Cell 31, 2035–2047. doi: 10.1091/mbc.E20-06-0363
Binns, D. D., Barylko, B., Grichine, N., Atkinson, M. A. L., Helms, M. K., Jameson, D. M., et al. (1999). Correlation between self-association modes and GTPase activation of dynamin. J. Protein Chem. 18, 277–290. doi: 10.1023/A:1021083211267
Bitoun, M., Maugenre, S., Jeannet, P. Y., Lacène, E., Ferrer, X., Laforêt, P., et al. (2005). Mutations in dynamin 2 cause dominant centronuclear myopathy. Nat. Genet. 37, 1207–1209. doi: 10.1038/ng1657
Blakely, R. D., and Edwards, R. H. (2012). Vesicular and plasma membrane transporters for neurotransmitters. Cold Spring Harb. Perspect. Biol. 4:a005595. doi: 10.1101/cshperspect.a005595
Boucrot, E., Ferreira, A. P. A., Almeida-Souza, L., Debard, S., Vallis, Y., Howard, G., et al. (2015). Endophilin marks and controls a clathrin-independent endocytic pathway. Nature 517, 460–465. doi: 10.1038/nature14067
Boucrot, E., Pick, A., Çamdere, G., Liska, N., Evergren, E., McMahon, H. T., et al. (2012). Membrane fission is promoted by insertion of amphipathic helices and is restricted by crescent BAR domains. Cell 149, 124–136. doi: 10.1016/j.cell.2012.01.047
Bramkamp, M. (2012). Structure and function of bacterial dynamin-like proteins. Biol. Chem. 393, 1203–1214. doi: 10.1515/hsz-2012-0185
Brodsky, F. M., Hill, B. L., Acton, S. L., Näthke, I., Wong, D. H., Ponnambalam, S., et al. (1991). Clathrin light chains: arrays of protein motifs that regulate coated-vesicle dynamics. Trends Biochem. Sci. 16, 208–213. doi: 10.1016/0968-0004(91)90087-C
Bucher, D., Frey, F., Sochacki, K. A., Kummer, S., Bergeest, J. P., Godinez, W. J., et al. (2018). Clathrin-adaptor ratio and membrane tension regulate the flat-to-curved transition of the clathrin coat during endocytosis. Nat. Commun. 9:1109. doi: 10.1038/s41467-018-03533-0
Buono, S., Ross, J. A., Tasfaout, H., Levy, Y., Kretz, C., Tayefeh, L., et al. (2018). Reducing dynamin 2 (DNM2) rescues DNM2-related dominant centronuclear myopathy. Proc. Natl. Acad. Sci. U.S.A. 115, 11066–11071. doi: 10.1073/pnas.1808170115
Burtey, A., Schmid, E. M., Ford, M. G. J., Rappoport, J. Z., Scott, M. G. H., Marullo, S., et al. (2007). The conserved isoleucine-valine-phenylalanine motif couples activation state and endocytic functions of β-arrestins. Traffic 8, 914–931. doi: 10.1111/j.1600-0854.2007.00578.x
Caì, Y., Postnikova, E. N., Bernbaum, J. G., Yú, S., Mazur, S., Deiuliis, N. M., et al. (2015). Simian hemorrhagic fever virus cell entry is dependent on CD163 and uses a clathrin-mediated endocytosis-like pathway. J. Virol. 89, 844–856. doi: 10.1128/JVI.02697-14
Cao, H., Garcia, F., and Mcniven, M. A. (1998). Differential distribution of dynamin isoforms in mammalian cells. Mol. Biol. Cell. 9, 2595–2609. doi: 10.1091/mbc.9.9.2595
Carlsson, A. E., and Bayly, P. V. (2014). Force generation by endocytic actin patches in budding yeast. Biophys. J. 106, 1596–1606. doi: 10.1016/j.bpj.2014.02.035
Carpentier, J., -L; Sawano, F., Geiger, D., Gorden, P., Perrelet, A., and Orci, L. (1989). Potassium depletion and hypertonic medium reduce “non-coated” and clathrin-coated pit formation, as well as endocytosis through these two gates. J. Cell. Physiol. 138, 519–526. doi: 10.1002/jcp.1041380311
Carr, J. F., and Hinshaw, J. E. (1997). Dynamin assembles into spirals under physiological salt conditions upon the addition of GDP and γ-phosphate analogues. J. Biol. Chem. 272, 28030–28035. doi: 10.1074/jbc.272.44.28030
Casamento, A., and Boucrot, E. (2020). Molecular mechanism of fast endophilin-mediated endocytosis. Biochem. J. 477, 2327–2345. doi: 10.1042/BCJ20190342
Chanaday, N. L., Cousin, M. A., Milosevic, I., Watanabe, S., and Morgan, J. R. (2019). The synaptic vesicle cycle revisited: new insights into the modes and mechanisms. J. Neurosci. 39, 8209–8216. doi: 10.1523/JNEUROSCI.1158-19.2019
Chappie, J. S., Acharya, S., Leonard, M., Schmid, S. L., and Dyda, F. G. (2010). Domain dimerization controls dynamin's assembly-stimulated GTPase activity. Nature 465, 435–440. doi: 10.1038/nature09032
Chappie, J. S., Acharya, S., Liu, Y.-W., Leonard, M., Pucadyil, T. J., Schmid, S. L., et al. (2009). An intramolecular signaling element that modulates dynamin function in vitro and in vivo. Mol. Biol. Cell 20, 3561–3571. doi: 10.1091/mbc.e09-04-0318
Chappie, J. S., Mears, J. A., Fang, S., Leonard, M., Schmid, S. L., Milligan, R. A., et al. (2011). A pseudoatomic model of the dynamin polymer identifies a hydrolysis-dependent powerstroke. Cell 147, 209–222. doi: 10.1016/j.cell.2011.09.003
Chen, C., Hou, W., Liu, I., Hsiao, G., Huang, S. S., and Huang, J. S. (2009). Inhibitors of clathrin-dependent endocytosis enhance TGF β signaling and responses. J. Cell Sci. 122, 1863–1871. doi: 10.1242/jcs.038729
Chen, X. W., Feng, Y. Q., Hao, C. J., Guo, X. L., He, X., Zhou, Z. Y., et al. (2008). DTNBP1, a schizophrenia susceptibility gene, affects kinetics of transmitter release. J. Cell Biol. 181, 791–801. doi: 10.1083/jcb.200711021
Chen, Y. J., Zhang, P., Egelman, E. H., and Hinshaw, J. E. (2004). The stalk region of dynamin drives the constriction of dynamin tubes. Nat. Struct. Mol. Biol. 11, 574–575. doi: 10.1038/nsmb762
Chen, Z., Mino, R. E., Mettlen, M., Michaely, P., Bhave, M., Reed, D. K., et al. (2020). Wbox2: a clathrin terminal domain derived peptide inhibitor of clathrin-mediated endocytosis. J. Cell Biol. 219:e201908189. doi: 10.1083/jcb.201908189
Cheung, G., and Cousin, M. A. (2013). Synaptic vesicle generation from activity-dependent bulk endosomes requires calcium and calcineurin. J. Neurosci. 33, 3370–3379. doi: 10.1523/JNEUROSCI.4697-12.2013
Chew, H. Y., De Lima, P. O., Gonzalez Cruz, J. L., Banushi, B., Echejoh, G., Hu, L., et al. (2020). Endocytosis inhibition in humans to improve responses to ADCC-mediating antibodies. Cell 180, 895–914.e27. doi: 10.1016/j.cell.2020.02.019
Chin, L., Shupliakovt, O., Brodint, L., Sihra, T. S., Hvalbyi, I., Jensen, V., et al. (1995). Impairment of synaptic vesicle clustering and of synaptic transmission, and increased seizure propensity, in synapsin i-deficient mice. Neurobiology 92, 9235–9239. doi: 10.1073/pnas.92.20.9235
Chircop, M., Sarcevic, B., Larsen, M. R., Malladi, C. S., Chau, N., Zavortink, M., et al. (2011). Phosphorylation of dynamin II at serine-764 is associated with cytokinesis. Biochim. Biophys. Acta Mol. Cell Res. 1813, 1689–1699. doi: 10.1016/j.bbamcr.2010.12.018
Clayton, E. L., Anggono, V., Smillie, K. J., Chau, N., Robinson, P. J., and Cousin, M. A. (2009). The phospho-dependent dynamin-syndapin interaction triggers activity-dependent bulk endocytosis of synaptic vesicles. J. Neurosci. 29, 7706–7717. doi: 10.1523/JNEUROSCI.1976-09.2009
Clayton, E. L., and Cousin, M. A. (2009). The molecular physiology of activity-dependent bulk endocytosis of synaptic vesicles. J. Neurochem. 111, 901–914. doi: 10.1111/j.1471-4159.2009.06384.x
Clayton, E. L., Evans, G. J. O., and Cousin, M. A. (2008). Bulk synaptic vesicle endocytosis is rapidly triggered during strong stimulation. J. Neurosci. 28, 6627–6632. doi: 10.1523/JNEUROSCI.1445-08.2008
Cocucci, E., Aguet, F., Boulant, S., and Kirchhausen, T. (2012). The first five seconds in the life of a clathrin-coated Pit. Cell 150, 495–507. doi: 10.1016/j.cell.2012.05.047
Collette, J. R., Chi, R. J., Boettner, D. R., Fernandez-Golbano, I. M., Plemel, R., Merz, A. J., et al. (2009). Clathrin functions in the absence of the terminal domain binding site for adaptor-associated clathrin-box motifs. Mol. Biol. Cell 20, 3401–3413. doi: 10.1091/mbc.e08-10-1082
Collins, B. M., McCoy, A. J., Kent, H. M., Evans, P. R., and Owen, D. J. (2002). Molecular architecture and functional model of the endocytic AP2 complex. Cell 109, 523–535. doi: 10.1016/S0092-8674(02)00735-3
Contreras-García, I. J., Gómez-Lira, G., Phillips-Farfán, B. V., Pichardo-Macías, L. A., García-Cruz, M. E., Chávez-Pacheco, J. L., et al. (2021). Synaptic vesicle protein 2a expression in glutamatergic terminals is associated with the response to levetiracetam treatment. Brain Sci. 11:531. doi: 10.3390/brainsci11050531
Cook, T. A., Urrutia, R., and Mcnivent, M. A. (1994). Identification of dynamin 2, an isoform ubiquitously expressed in rat tissues (endocytosis/vesicular transport/molecular cloning/liver). Cell Biol. 91, 644–648. doi: 10.1073/pnas.91.2.644
Cosson, P., De Curtis, I., Pouyssegur, J., Griffiths, G., and Davoust, J. (1989). Low cytoplasmic PH inhibits endocytosis and transport from the Trans-golgi network to the cell surface. J. Cell Biol. 108, 377–387. doi: 10.1083/jcb.108.2.377
Cousin, M. A., and Robinson, P. J. (2001). The dephosphins: dephosphorylation by calcineurin triggers synaptic vesicle endocytosis. Trends Neurosci. 24, 659–665. doi: 10.1016/S0166-2236(00)01930-5
Cremona, O., and De Camilli, P. (1997). Synaptic vesicle endocytosis. Curr. Opin. Neurobiol. 7, 323–330. doi: 10.1016/S0959-4388(97)80059-1
Damke, H., Baba, T., Warnock, D. E., and Schmid, S. L. (1994). Induction of mutant dynamin specifically blocks endocytic coated vesicle formation. J. Cell Biol. 127, 915–934. doi: 10.1083/jcb.127.4.915
Daniel, J. A., Chau, N., Abdel-Hamid, M. K., Hu, L., von Kleist, L., Whiting, A., et al. (2015). Phenothiazine-derived antipsychotic drugs inhibit dynamin and clathrin-mediated endocytosis. Traffic 16, 635–654. doi: 10.1111/tra.12272
Daniel, J. A., Malladi, C. S., Kettle, E., McCluskey, A., and Robinson, P. J. (2012). Analysis of synaptic vesicle endocytosis in synaptosomes by high-content screening. Nat. Protoc. 7, 1649–1655. doi: 10.1038/nprot.2012.070
Danino, D., Moon, K. H., and Hinshaw, J. E. (2004). Rapid constriction of lipid bilayers by the mechanochemical enzyme dynamin. J. Struct. Biol. 147, 259–267. doi: 10.1016/j.jsb.2004.04.005
David, C., Mcpherson, P. S., Mundigl, O., and De Camillit, P. (1996). A role of amphiphysin in synaptic vesicle endocytosis suggested by its binding to dynamin in nerve terminals (SH3 domain/adaptin/Grb2/clathrin/RVS genes). Cell Biol. 93, 331–335. doi: 10.1073/pnas.93.1.331
De Guzman, F. S., Copp, B. R., Mayne, C. L., Concepcion, G. P., Mangalindan, G. C., Barrows, L. R., et al. (1998). Bolinaquinone: a novel cytotoxic sesquiterpene hydroxyquinone from a philippine dysidea sponge have been isolated from various sponges. 3-16 the ses-quiterpene moiety of these metabolites usually have the normal drimane skeleton, as exemplified by spo. J. Org. Chem. 63, 8042–8044. doi: 10.1021/jo981037t
Dejonghe, W., Sharma, I., Denoo, B., De Munck, S., Lu, Q., Mishev, K., et al. (2019). Disruption of endocytosis through chemical inhibition of clathrin heavy chain function. Nat. Chem. Biol. 15, 641–649. doi: 10.1038/s41589-019-0262-1
Delvendahl, I., Vyleta, N. P., von Gersdorff, H., and Hallermann, S. (2016). Fast, temperature-sensitive and clathrin-independent endocytosis at central synapses. Neuron 90, 492–498. doi: 10.1016/j.neuron.2016.03.013
Denker, A., and Rizzoli, S. O. (2010). Synaptic vesicle pools: an update. Front. Synaptic Neurosci. 2:135. doi: 10.3389/fnsyn.2010.00135
Di Paolo, G., Sankaranarayanan, S., Wenk, M. R., Daniell, L., Perucco, E., Caldarone, B. J., et al. (2002). Decreased synaptic vesicle recycling efficiency and cognitive deficits in amphiphysin 1 knockout mice. Neuron 33, 789–804. doi: 10.1016/S0896-6273(02)00601-3
Doxsey, S. J., Brodsky, F. M., Blank, G. S., and Helenius, A. (1987). Inhibition of endocytosis by anti-clathrin antibodies. Cell 50, 453–463. doi: 10.1016/0092-8674(87)90499-5
Durães, F., Pinto, M., and Sousa, E. (2018). Old drugs as new treatments for neurodegenerative diseases. Pharmaceuticals 11, 1–21. doi: 10.3390/ph11020044
Durieux, A. C., Prudhon, B., Guicheney, P., and Bitoun, M. (2010). Dynamin 2 and human diseases. J. Mol. Med. 88, 339–350. doi: 10.1007/s00109-009-0587-4
Dutta, D., and Donaldson, J. G. (2012). Search for inhibitors of endocytosis. Cell. Logist. 2, 203–208. doi: 10.4161/cl.23967
Dutta, D., Williamson, C. D., Cole, N. B., and Donaldson, J. G. (2012). Pitstop 2 is a potent inhibitor of clathrin-independent endocytosis. PLoS ONE 7, 1–9. doi: 10.1371/journal.pone.0045799
Edeling, M. A., Mishra, S. K., Keyel, P. A., Steinhauser, A. L., Collins, B. M., Roth, R., et al. (2006). Molecular switches involving the AP-2 2 appendage regulate endocytic cargo selection and clathrin coat assembly. Dev. Cell 10, 329–342. doi: 10.1016/j.devcel.2006.01.016
Elferink, J. G. (1979). R. chlorpromazine inhibits phagocytosis and exocytosis in rabbit polymorphonuclear leukocytes. Biochem. Pharmacol. 28, 965–968. doi: 10.1016/0006-2952(79)90287-9
Elkin, S. R., Oswald, N. W., Reed, D. K., Mettlen, M., MacMillan, J. B., and Schmid, S. L. (2016). Ikarugamycin: a natural product inhibitor of clathrin-mediated endocytosis. Traffic 17, 1139–1149. doi: 10.1111/tra.12425
Eschenburg, S., and Reubold, T. F. (2018). Modulation of dynamin function by small molecules. Biol. Chem. 399, 1421–1432. doi: 10.1515/hsz-2018-0257
Eto, D. S., Gordon, H. B., Dhakal, B. K., Jones, T. A., and Mulvey, M. A. (2008). Clathrin, AP-2, and the NPXY-binding subset of alternate endocytic adaptors facilitate fimh-mediated bacterial invasion of host cells. Cell. Microbiol. 10, 2553–2567. doi: 10.1111/j.1462-5822.2008.01229.x
Evans, G. J. O., and Cousin, M. A. (2007). Activity-dependent control of slow synaptic vesicle endocytosis by cyclin-dependent kinase 5. J. Neurosci. 27, 401–411. doi: 10.1523/JNEUROSCI.3809-06.2007
Faelber, K., Posor, Y., Gao, S., Held, M., Roske, Y., Schulze, D., et al. (2011). Crystal structure of nucleotide-free dynamin. Nature 477, 556–562. doi: 10.1038/nature10369
Farisello, P., Boido, D., Nieus, T., Medrihan, L., Cesca, F., Valtorta, F., et al. (2013). Synaptic and extrasynaptic origin of the excitation/inhibition imbalance in the hippocampus of synapsin I/II/III knockout mice. Cereb. Cortex 23, 581–593. doi: 10.1093/cercor/bhs041
Farsi, Z., Gowrisankaran, S., Krunic, M., Rammner, B., Woehler, A., Lafer, E. M., et al. (2018). Clathrin coat controls synaptic vesicle acidification by blocking vacuolar ATpase activity. Elife 7, 1–18. doi: 10.7554/eLife.32569
Farsi, Z., Jahn, R., and Woehler, A. (2017). Proton electrochemical gradient: driving and regulating neurotransmitter uptake. BioEssays 39, 1–9. doi: 10.1002/bies.201600240
Ferguson, K. M., Lemmon, M. A., Bchlessinger, J., and Slgler, P. B. (1994). Crystal structure at 2.2 a resolution of the pleckstrin homology domain from human dynamin. Cell 79, 199–209. doi: 10.1016/0092-8674(94)90190-2
Ferguson, S., Raimondi, A., Paradise, S., Shen, H., Mesaki, K., Ferguson, A., et al. (2009). Coordinated actions of actin and BAR proteins upstream of dynamin at endocytic clathrin-coated pits. Dev. Cell 17, 811–822. doi: 10.1016/j.devcel.2009.11.005
Ferguson, S. M., Brasnjo, G., Hayashi, M., Wölfel, M., Collesi, C., Giovedi, S., et al. (2007). A selective activity-dependent requirement for dynamin 1 in synaptic vesicle endocytosis. Science. (80-.). 316, 570–574. doi: 10.1126/science.1140621
Ferguson, S. M., and De Camilli, P. (2012). Dynamin, a membrane-remodelling GTPase. Nat. Rev. Mol. Cell Biol. 13, 75–88. doi: 10.1038/nrm3266
Fesce, R., Grohovaz, F., Valtorta, F., and Meldolesi, J. (1994). Neurotransmitter release: fusion or “kiss-and-run”? Trends Cell Biol. 4, 1–4. doi: 10.1016/0962-8924(94)90025-6
Ford, M. G. J., Jenni, S., and Nunnari, J. (2011). The crystal structure of dynamin. Nature 477, 561–566. doi: 10.1038/nature10441
Ford, M. G. J., Mills, I. G., Peter, B. J., Vallis, Y., Praefcke, G. J. K., Evans, P. R., et al. (2002). Curvature of clathrin-coated pits driven by epsin. Nature 419, 361–366. doi: 10.1038/nature01020
Ford, M. G. J., Pearse, B. M. F., Higgins, M. K., Vallis, Y., Owen, D. J., Gibson, A., et al. (2001). Simultaneous binding of PtdIns(4,5)P2 and clathrin byAP180 in the nucleation ofclathrin lattices on membranes. Science. (80-.). 291, 1051–1055. doi: 10.1126/science.291.5506.1051
Fotin, A., Cheng, Y., Grigorieff, N., Walz, T., Harrison, S. C., and Kirchhausen, T. (2004b). Structure of an auxilin-bound clathrin coat and its implications for the mechanism of uncoating. Nature 432, 649–653. doi: 10.1038/nature03078
Fotin, A., Cheng, Y., Sliz, P., Grigorieff, N., Harrison, S. C., Kirchhausen, T., et al. (2004a). Molecular model for a complete clathrin lattice from electron cryomicroscopy. Nature 432, 573–579. doi: 10.1038/nature03079
Francesco Retta, S., Barry, S. T., Critchley, D. R., Defilippi, P., Silengo, L., and Tarone, G. (1996). Focal adhesion and stress fiber formation is regulated by tyrosine phosphatase activity. Exp. Cell Res. 229, 307–317. doi: 10.1006/excr.1996.0376
Gan, Q., and Watanabe, S. (2018). Synaptic vesicle endocytosis in different model systems. Front. Cell. Neurosci. 12:171. doi: 10.3389/fncel.2018.00171
Gandhi, S. P., and Stevens, C. F. (2003). Three modes of synaptic vesicular recycling revealed by single-vesicle imaging. Nature 423, 607–613. doi: 10.1038/nature01677
Gasper, R., Meyer, S., Gotthardt, K., Sirajuddin, M., and Wittinghofer, A. (2009). It takes two to tango: regulation of G proteins by dimerization. Nat. Rev. Mol. Cell Biol. 10, 423–429. doi: 10.1038/nrm2689
Gerhard, R., John, H., Aktories, K., and Just, I. (2003). Thiol-modifying phenylarsine oxide inhibits guanine nucleotide binding of rho but not of rac GTPases. Mol. Pharmacol. 63, 1349–1355. doi: 10.1124/mol.63.6.1349
Ghods, A., Gilbert, J., Baker, J. R., Russell, C. C., Sakoff, J. A., and McCluskey, A. (2018). A focused library synthesis and cytotoxicity of quinones derived from the natural product bolinaquinone. R. Soc. Open Sci. 5, 171–189. doi: 10.1098/rsos.171189
Godlee, C., and Kaksonen, M. (2013). From uncertain beginnings: initiation mechanisms of clathrin-mediated endocytosis. J. Cell Biol. 203, 717–725. doi: 10.1083/jcb.201307100
Gold, E. S., Underhill, D. M., Morrissette, N. S., Guo, J., Mcniven, M. A., and Aderem, A. (1999). Dynamin 2 is required for phagocytosis in macrophages. J. Exp. Med. 190, 1849–1856. doi: 10.1084/jem.190.12.1849
Gordon, C. P., Venn-Brown, B., Robertson, M. J., Young, K. A., Chau, N., Mariana, A., et al. (2013). Development of second-generation indole-based dynamin GTPase inhibitors. J. Med. Chem. 56, 46–59. doi: 10.1021/jm300844m
Granseth, B., Odermatt, B., Royle, S. J. J., and Lagnado, L. (2006). Clathrin-mediated endocytosis is the dominant mechanism of vesicle retrieval at hippocampal synapses. Neuron 51, 773–786. doi: 10.1016/j.neuron.2006.08.029
Gray, N. W., Fourgeaud, L., Huang, B., Chen, J., Cao, H., Oswald, B. J., et al. (2003). Dynamin 3 is a component of the postsynapse, where it interacts with MGluR5 and homer integral role in synaptic vesicle recycling [4, 9]. we and others identified dynamin 2 (Dyn2) as a ubiquitously expressed isoform that can mediate multiple endocytic. Curr. Biol. 13, 510–515. doi: 10.1016/S0960-9822(03)00136-2
Guo, J., Ge, J. L., Hao, M., Sun, Z. C., Wu, X. S., Zhu, J. B., et al. (2015). A three-pool model dissecting readily releasable pool replenishment at the calyx of held. Sci. Rep. 5, 1–8. doi: 10.1038/srep09517
Hafner, M., Mills, C. E., Subramanian, K., Chen, C., Chung, M., Boswell, S. A., et al. (2019). Multiomics profiling establishes the polypharmacology of FDA-approved CDK4/6 inhibitors and the potential for differential clinical activity. Cell Chem. Biol. 26, 1067–1080.e8. doi: 10.1016/j.chembiol.2019.05.005
Harjes, P., and Wanker, E. E. (2003). The hunt for huntingtin function: interaction partners tell many different stories. Trends Biochem. Sci. 28, 425–433. doi: 10.1016/S0968-0004(03)00168-3
Harper, C. B., Popoff, M. R., McCluskey, A., Robinson, P. J., and Meunier, F. A. (2013). Targeting membrane trafficking in infection prophylaxis: dynamin inhibitors. Trends Cell Biol. 23, 90–101. doi: 10.1016/j.tcb.2012.10.007
Hasumi, K., Shinohara, C., Naganuma, S., and Endo, A. (1992). Inhibition of the uptake of oxidized low-density lipoprotein in macrophage J774 by the antibiotic ikarugamycin. Eur. J. Biochem. 205, 841–846. doi: 10.1111/j.1432-1033.1992.tb16848.x
Haucke, V., and Kozlov, M. M. (2018). Membrane remodeling in clathrin-mediated endocytosis. J. Cell Sci. 131:jcs216812. doi: 10.1242/jcs.216812
Heerssen, H., Fetter, R. D., and Davis, G. W. (2008). Clathrin dependence of synaptic-vesicle formation at the drosophila neuromuscular junction. Curr. Biol. 18, 401–409. doi: 10.1016/j.cub.2008.02.055
Henley, J. R., Krueger, E. W. A., Oswald, B. J., and Mcniven, M. A. (1998). Dynamin-mediated internalization of caveolae. J. Cell Biol. 141, 85–99. doi: 10.1083/jcb.141.1.85
Henne, W. M., Boucrot, E., Meinecke, M., Evergren, E., Vallis, Y., Mittal, R., et al. (2010). FCHo proteins are nucleators of clathrin-mediated endocytosis. Science. (80-.). 328, 1281–1284. doi: 10.1126/science.1188462
Hernaez, B., and Alonso, C. (2010). Dynamin- and clathrin-dependent endocytosis in african swine fever virus entry. J. Virol. 84, 2100–2109. doi: 10.1128/JVI.01557-09
Heuser, E., and Reese, T. S. (1973). Evidence for recycling of synaptic vesicle membrane during transmitter release at the frog neuromuscular junction. J. Cell Biol. 57, 15–344. doi: 10.1083/jcb.57.2.315
Heuser, J. (1980). Three-dimensional visualization of coated vesicle formation in fibroblasts. J. Cell Biol. 84, 560–583. doi: 10.1083/jcb.84.3.560
Hill, T., Odell, L. R., Edwards, J. K., Graham, M. E., McGeachie, A. B., Rusak, J., et al. (2005). Small molecule inhibitors of dynamin I GTPase activity: development of dimeric tyrphostins. J. Med. Chem. 48, 7781–7788. doi: 10.1021/jm040208l
Hill, T. A., Gordon, C. P., McGeachie, A. B., Venn-Brown, B., Odell, L. R., Chau, N., et al. (2009). Inhibition of dynamin mediated endocytosis by the dynoles–synthesis and functional activity of a family of indoles. J. Med. Chem. 52, 3762–3773. doi: 10.1021/jm900036m
Hill, T. A., Mariana, A., Gordon, C. P., Odell, L. R., Robertson, M. J., McGeachie, A. B., et al. (2010). Iminochromene inhibitors of dynamins I and II GTPase activity and endocytosis. J. Med. Chem. 53, 4094–4102. doi: 10.1021/jm100119c
Hill, T. A., Odell, L. R., Quan, A., Abagyan, R., Ferguson, G., Robinson, P. J., et al. (2004). Long chain amines and long chain ammonium salts as novel inhibitors of dynamin GTPase activity. Bioorganic Med. Chem. Lett. 14, 3275–3278. doi: 10.1016/j.bmcl.2004.03.096
Hinrichsen, L., Harborth, J., Andrees, L., Weber, K., and Ungewickell, E. J. (2003). Effect of clathrin heavy chain- and α-adaptin-specific small inhibitory RNAs on endocytic accessory proteins and receptor trafficking in HeLa cells. J. Biol. Chem. 278, 45160–45170. doi: 10.1074/jbc.M307290200
Hinshaw, J. E. (2000). Dynamin and its role in membrane fission. Annu. Rev. Cell Dev. Biol 16, 483–519. doi: 10.1146/annurev.cellbio.16.1.483
Hinshaw, J. E., and Schmid, S. L. (1995). Dynamin self-assembles into rings suggesting a mechanism for coated vesicle budding. Nature 9, 190–192. doi: 10.1038/374190a0
Hirst, J., and Robinson, M. S. (1998). Clathrin and Adaptors. Biochim. Biophys. Acta Mol. Cell Res. 1404, 173–193. doi: 10.1016/S0167-4889(98)00056-1
Holderith, N., Lorincz, A., Katona, G., Rózsa, B., Kulik, A., Watanabe, M., et al. (2012). Release probability of hippocampal glutamatergic terminals scales with the size of the active zone. Nat. Neurosci. 15, 988–997. doi: 10.1038/nn.3137
Holt, M., Cooke, A., Wu, M. M., and Lagnado, L. (2003). Bulk membrane retrieval in the synaptic terminal of retinal bipolar cells. J. Neurosci. 23, 1329–1339. doi: 10.1523/JNEUROSCI.23-04-01329.2003
Höning, S., Ricotta, D., Krauss, M., Späte, K., Spolaore, B., Motley, A., et al. (1997). Phosphatidylinositol (4,5)-bisphosphate-dependent activation of dynamins I and II lacking the proline/arginine-rich domains. J. Biol. Chem. 272, 25999–26004. doi: 10.1074/jbc.272.41.25999
Höning, S., Ricotta, D., Krauss, M., Späte, K., Spolaore, B., Motley, A., et al. (2005). Phosphatidylinositol-(4,5)-bisphosphate regulates sorting signal recognition by the clathrin-associated adaptor complex AP2. Mol. Cell 18, 519–531. doi: 10.1016/j.molcel.2005.04.019
Hopkins, A. L. (2008). Network pharmacology: the next paradigm in drug discovery. Nat. Chem. Biol. 4, 682–690. doi: 10.1038/nchembio.118
Hyndman, K. A., Arguello, A. M., Morsing, S. K. H., and Pollock, J. S. (2016). Dynamin-2 is a novel NOS1β interacting protein and negative regulator in the collecting duct. Am. J. Physiol. Regul. Integr. Comp. Physiol. 310, R570–R577. doi: 10.1152/ajpregu.00008.2015
Inoshita, T., and Imai, Y. (2015). Regulation of vesicular trafficking by Parkinson's disease-associated genes. AIMS Mol. Sci. 2, 461–475. doi: 10.3934/molsci.2015.4.461
Ivanov, A. I. (2008). Pharmacological inhibition of endocytic pathways: is it specific enough to be useful? Methods Mol. Biol. 440, 15–33. doi: 10.1007/978-1-59745-178-9_2
Janzen, C., Kochs, G., and Haller, O. (2000). A monomeric GTPase-negative MxA mutant with antiviral activity. J. Virol. 74, 8202–8206. doi: 10.1128/JVI.74.17.8202-8206.2000
Jensen, D. D., Lieu, T. M., Halls, M. L., Veldhuis, N. A., Imlach, W. L., Mai, Q. N., et al. (2017). Neurokinin 1 receptor signaling in endosomes mediates sustained nociception and is a viable therapeutic target for prolonged pain relief. Sci. Transl. Med. 9:eaal3447. doi: 10.1126/scitranslmed.aal3447
Jimah, J. R., and Hinshaw, J. E. (2019). Structural insights into the mechanism of dynamin superfamily proteins. Trends Cell. Biol. 29, 257–273. doi: 10.1016/j.tcb.2018.11.003
Jomon, K., Kuroda, Y., Heiichi, S., and Ajisaka, M. (1972). A new antibiotic: ikarugamycin. J. Antibiot. (Tokyo). 25, 271–280. doi: 10.7164/antibiotics.25.271
Joshi, S., Perera, S., Gilbert, J., Smith, C. M., Mariana, A., Gordon, C. P., et al. (2010). The Dynamin inhibitors MiTMAB and OcTMAB induce cytokinesis failure and inhibit cell proliferation in human cancer cells. Mol. Cancer Ther. 9, 1995–2006. doi: 10.1158/1535-7163.MCT-10-0161
Jungbluth, H., Wallgren-Pettersson, C., and Laporte, J. (2008). Centronuclear (Myotubular) myopathy. Orphanet, J. Rare Dis. 3, 1–13. doi: 10.1186/1750-1172-3-26
Kaeser, P. S., and Regehr, W. G. (2017). The readily releasable pool of synaptic vesicles. Curr. Opin. Neurobiol. 43, 63–70. doi: 10.1016/j.conb.2016.12.012
Kaksonen, M., and Roux, A. (2018). Mechanisms of clathrin-mediated endocytosis. Nat. Rev. Mol. Cell Biol. 19, 313–326. doi: 10.1038/nrm.2017.132
Kang, D. S., Kern, R. C., Puthenveedu, M. A., von Zastrow, M., Williams, J. C., and Benovic, J. L. (2009). Structure of an arrestin2-clathrin complex reveals a novel clathrin binding domain that modulates receptor trafficking. J. Biol. Chem. 284, 29860–29872. doi: 10.1074/jbc.M109.023366
Kang, S. J., Shin, K. S., Song, W. K., Ha, D. B., Chung, C. H., and Kang, M. S. (1995). Involvement of transglutaminase in myofibril assembly of chick embryonic myoblasts in culture. J. Cell Biol. 130, 1127–1136. doi: 10.1083/jcb.130.5.1127
Kasprowicz, J., Kuenen, S., Miskiewicz, K., Habets, R. L. P., Smitz, L., and Verstreken, P. (2008). Inactivation of clathrin heavy chain inhibits synaptic recycling but allows bulk membrane uptake. J. Cell Biol. 182, 1007–1016. doi: 10.1083/jcb.200804162
Kim, W. T., Chang, S., Daniell, L., Cremona, O., Di Paolo, G., and De Camilli, P. (2002). Delayed reentry of recycling vesicles into the fusion-competent synaptic vesicle pool in synaptojanin 1 knockout mice. Proc. Natl. Acad. Sci.U.S.A. 99, 17143–17148. doi: 10.1073/pnas.222657399
Kirchhausen, T. (1993). Coated pits and coated vesicles.-sorting it all out. Curr. Opin. Struct. Biol. 3, 182–188. doi: 10.1016/S0959-440X(05)80150-2
Kirchhausen, T. (2000). Clathrin. Annu. Rev. Biochem. 69, 699–727. doi: 10.1146/annurev.biochem.69.1.699
Kirchhausen, T. (2009). Imaging endocytic clathrin structures in living cells. Trends Cell Biol. 19, 596–605. doi: 10.1016/j.tcb.2009.09.002
Kirchhausen, T., Macia, E., and Pelish, H. E. (2008). Use of dynasore, the small molecule inhibitor of dynamin, in the regulation of endocytosis. Methods Enzymol. 438, 77–93. doi: 10.1016/S0076-6879(07)38006-3
Kirchhausen, T., Owen, D., and Harrison, S. C. (2014). Molecular structure, function, and dynamics of clathrin-mediated membrane traffic. Cold Spring Harb. Perspect. Biol. 6:a016725. doi: 10.1101/cshperspect.a016725
Kirchhausen, T., and Toyoda, T. (1993). Immunoelectron microscopic evidence for the extended conformation of light chains in clathrin trimers. J. Biol. Chem. 268, 10268–10273. doi: 10.1016/S0021-9258(18)82199-8
Klein, D. E., Lee, A., Frank, D. W., Marks, M. S., and Lemmon, M. A. (1998). The pleckstrin homology domains of dynamin isoforms require oligomerization for high affinity phosphoinositide binding. J. Biol. Chem. 273, 27725–27733. doi: 10.1074/jbc.273.42.27725
Klein, P., Friedman, A., Hameed, M. Q., Kaminski, R. M., Bar-Klein, G., Klitgaard, H., et al. (2020). repurposed molecules for antiepileptogenesis: missing an opportunity to prevent epilepsy? Epilepsia 61, 359–386. doi: 10.1111/epi.16450
Knuehl, C., Chen, C. Y., Manalo, V., Hwang, P. K., Ota, N., and Brodsky, F. M. (2006). Novel binding sites on clathrin and adaptors regulate distinct aspects of coat assembly. Traffic 7, 1688–1700. doi: 10.1111/j.1600-0854.2006.00499.x
Kochs, G., Haener, M., Aebi, U., and Haller, O. (2002). Self-assembly of human MxA GTPase into highly ordered dynamin-like oligomers. J. Biol. Chem. 277, 14172–14176. doi: 10.1074/jbc.M200244200
Koh, T. W., Korolchuk, V. I., Wairkar, Y. P., Jiao, W., Evergren, E., Pan, H., et al. (2007). Eps15 and Dap160 control synaptic vesicle membrane retrieval and synapse development. J. Cell Biol. 178, 309–322. doi: 10.1083/jcb.200701030
Kong, L., Sochacki, K. A., Wang, H., Fang, S., Canagarajah, B., Kehr, A. D., et al. (2018). Cryo-EM of the dynamin polymer assembled on lipid membrane. Nature 560, 258–262. doi: 10.1038/s41586-018-0378-6
Konno, M., Hasegawa, T., Baba, T., Miura, E., Sugeno, N., Kikuchi, A., et al. (2012). Suppression of dynamin GTPase decreases -synuclein uptake by neuronal and oligodendroglial cells: a potent therapeutic target for synucleinopathy. Mol. Neurodegener. 7, 1–16. doi: 10.1186/1750-1326-7-38
Kononenko, N. L., and Haucke, V. (2015). Molecular mechanisms of presynaptic membrane retrieval and synaptic vesicle reformation. Neuron 85, 484–496. doi: 10.1016/j.neuron.2014.12.016
Kosaka, T., and Ikeda, K. (1983). Possible temperature-dependent blockage of synaptic vesicle recycling induced by a single gene mutation in drosophila. J. Neurobiol. 14, 207–225. doi: 10.1002/neu.480140305
Labrousse, A. M., Zappaterra, M. D., Rube, D. A., and van der Bliek, A. M. (1999). C. elegans dynamin-related protein DRP-1 controls severing of the mitochondrial outer membrane. Mol. Cell 4, 815–826. doi: 10.1016/S1097-2765(00)80391-3
Lee, A., Frank, D. W., Marks, M. S., and Lemmon, M. A. (1999). Dominant-negative inhibition of receptor-mediated endocytosis by a dynamin-1 mutant with a defective pleckstrin homology domain results and discussion. Curr. Biol. 9, 261–264. doi: 10.1016/S0960-9822(99)80115-8
Lemmon, S. K., and Traub, L. M. (2012). Getting in touch with the clathrin terminal domain. Traffic 13, 511–519. doi: 10.1111/j.1600-0854.2011.01321.x
Li, Y. Y., Chen, X. N., Fan, X. X., Zhang, Y. J., Gu, J., Fu, X. W., et al. (2015). Upregulated dynamin 1 in an acute seizure model and in epileptic patients. Synapse 69, 67–77. doi: 10.1002/syn.21788
Lindner, R., and Ungewickell, E. (1992). Clathrin-associated proteins of bovine brain coated vesicles. An analysis of their number and assembly-promoting activity. J. Biol. Chem. 267, 16567–16573. doi: 10.1016/S0021-9258(18)42040-6
Liu, S. H., Marks, M. S., and Brodsky, F. M. (1998). A dominant-negative clathrin mutant differentially affects trafficking of molecules with distinct sorting motifs in the class II major histocompatibility complex (MHC) pathway. J. Cell Biol. 140, 1023–1037. doi: 10.1083/jcb.140.5.1023
Liu, Y.-W., Surka, M. C., Schroeter, T., Lukiyanchuk, V., and Schmid, S. L. (2008). Isoform and splice-variant specific functions of dynamin-2 revealed by analysis of conditional knock-out cells. Mol. Biol. Cell 19, 5347–5359. doi: 10.1091/mbc.e08-08-0890
Liu, Y. W., Neumann, S., Ramachandran, R., Ferguson, S. M., Pucadyil, T. J., and Schmid, S. L. (2011). Differential curvature sensing and generating activities of dynamin isoforms provide opportunities for tissue-specific regulation. Proc. Natl. Acad. Sci. U. S. A. 108, E234–E242. doi: 10.1073/pnas.1102710108
Llorente, A., Rapak, A., Schmid, S. L., Van Deurs, B., Sandvig, K., Damke, H., et al. (1998). Expression of mutant dynamin inhibits toxicity and transport of endocytosed ricin to the golgi apparatus. J. Cell Biol. 140, 69–80. doi: 10.1083/jcb.140.3.553
Low, H. H., and Löwe, J. (2006). A bacterial dynamin-like protein. Nature 444, 766–769. doi: 10.1038/nature05312
Lu, J., Helton, T. D., Blanpied, T. A., Rácz, B., Newpher, T. M., Weinberg, R. J., et al. (2007). Postsynaptic positioning of endocytic zones and AMPA receptor cycling by physical coupling of dynamin-3 to homer. Neuron 55, 874–889. doi: 10.1016/j.neuron.2007.06.041
Lu, W., Huan, M., Sheng, Z. H., and Mochida, S. (2009). Dynamin and activity regulate synaptic vesicle recycling in sympathetic neurons. J. Biol. Chem. 284, 1930–1937. doi: 10.1074/jbc.M803691200
Lucas, R., Giannini, C., D'Auria, M. V., and Pay,á, M. (2003). Modulatory effect of bolinaquinone, a marine sesquiterpenoid, on acute and chronic inflammatory processes. J. Pharmacol. Exp. Ther. 304, 1172–1180. doi: 10.1124/jpet.102.045278
Lundmark, R., and Carlsson, S. R. (2004). Regulated membrane recruitment of dynamin-2 mediated by sorting nexin 9. J. Biol. Chem. 279, 42694–42702. doi: 10.1074/jbc.M407430200
Lupas, A., Van Dyke, M., and Stock, J. (1991). Predicting coiled coils from protein sequences. Science. (80-.). 252, 1162–1164. doi: 10.1126/science.252.5009.1162
Lynch, B. A., Lambeng, N., Nocka, K., Kensel-Hammes, P., Bajjalieh, S. M., Matagne, A., et al. (2004). The synaptic vesicle is the protein SV2A is the binding site for the antiepileptic drug levetiracetam. Proc. Natl. Acad. Sci. U.S.A. 101, 9861–9866. doi: 10.1073/pnas.0308208101
Macgregor, K. A., Abdel-Hamid, M. K., Odell, L. R., Chau, N., Whiting, A., Robinson, P. J., et al. (2014b). Development of quinone analogues as dynamin GTPase inhibitors. Eur. J. Med. Chem. 85, 191–206. doi: 10.1016/j.ejmech.2014.06.070
Macgregor, K. A., Robertson, M. J., Young, K. A., Von Kleist, L., Stahlschmidt, W., Whiting, A., et al. (2014a). Development of 1,8-naphthalimides as clathrin inhibitors. J. Med. Chem. 57, 131–143. doi: 10.1021/jm4015263
Macia, E., Ehrlich, M., Massol, R., Boucrot, E., Brunner, C., and Kirchhausen, T. (2006). Dynasore, a cell-permeable inhibitor of dynamin. Dev. Cell 10, 839–850. doi: 10.1016/j.devcel.2006.04.002
Maraka, S., and Kennel, K. A. (2015). Bisphosphonates for the prevention and treatment of osteoporosis. BMJ 351:h3783. doi: 10.1136/bmj.h3783
Marchese, A., Paing, M. M., Temple, B. R. S., and Trejo, J. A. (2008). G Protein-coupled receptor sorting to endosomes and lysosomes. Annu. Rev. Pharmacol. Toxicol. 48, 601–629. doi: 10.1146/annurev.pharmtox.48.113006.094646
Margarucci, L., Monti, M. C., Fontanella, B., Riccio, R., and Casapullo, A. (2011). Chemical proteomics reveals bolinaquinone as a clathrin-mediated endocytosis inhibitor. Mol. Biosyst. 7, 480–485. doi: 10.1039/C0MB00126K
Marie-Anaïs, F., Mazzolini, J., Herit, F., and Niedergang, F. (2016). Dynamin-actin cross talk contributes to phagosome formation and closure. Traffic 17, 487–499. doi: 10.1111/tra.12386
Marks, B., Stowell, M. H. B., Vallis, Y., Mills, I. G., Gibson, A., Hopkins, C. R., et al. (2001). GTPase activity of dynamin and resulting conformation change are essential for endocytosis. Nature 410, 231–235. doi: 10.1038/35065645
Masaike, Y., Takagi, T., Hirota, M., Yamada, J., Ishihara, S., Yung, T. M. C., et al. (2010). Identification of dynamin-2-mediated endocytosis as a new target of osteoporosis drugs, bisphosphonates. Mol. Pharmacol. 77, 262–269. doi: 10.1124/mol.109.059006
Masri, B., Salahpour, A., Didriksen, M., Ghisi, V., Beaulieu, J.-M., Gainetdinov, R. R., et al. (2008). Antagonism of dopamine D2 receptor/β-arrestin 2 interaction is a common property of clinically effective antipsychotics. Proc. Natl. Acad. Sci. U.S.A. 105, 13656–13661. doi: 10.1073/pnas.0803522105
Mattila, J. P., Shnyrova, A. V., Sundborger, A. C., Hortelano, E. R., Fuhrmans, M., Neumann, S., et al. (2015). A hemi-fission intermediate links two mechanistically distinct stages of membrane fission. Nature 524, 109–113. doi: 10.1038/nature14509
Mattson, M. P. (1997). Cellular actions of β-amyloid precursor protein and its soluble and fibrillogenic derivatives. Physiol. Rev. 77, 1081–1115. doi: 10.1152/physrev.1997.77.4.1081
Maycox, P. R., Link, E., Reetz, A., Morris, S. A., and Jahn, R. (1992). Clathrin-coated vesicles in nervous tissue are involved primarily in synaptic vesicle recycling. J. Cell Biol. 118, 1379–1388. doi: 10.1083/jcb.118.6.1379
McAdam, R. L., Morton, A., Gordon, S. L., Alterman, J. F., Khvorova, A., Cousin, M. A., et al. (2020). Loss of huntingtin function slows synaptic vesicle endocytosis in striatal neurons from the HttQ140/Q140 mouse model of huntington's disease. Neurobiol. Dis. 134:104637. doi: 10.1016/j.nbd.2019.104637
Mccluskey, A., Daniel, J. A., Hadzic, G., Chau, N., Clayton, E. L., Mariana, A., et al. (2013). Building a better dynasore: the dyngo compounds potently inhibit dynamin and endocytosis. Traffic 14, 1272–1289. doi: 10.1111/tra.12119
McGeachie, A. B., Odell, L. R., Quan, A., Daniel, J. A., Chau, N., Hill, T. A., et al. (2013). Pyrimidyn compounds: dual-action small molecule pyrimidine-based dynamin inhibitors. ACS Chem. Biol. 8, 1507–1518. doi: 10.1021/cb400137p
McMahon, H. T., and Boucrot, E. (2011). Molecular mechanism and physiological functions of clathrin-mediated endocytosis. Nat. Rev. Mol. Cell Biol. 12, 517–533. doi: 10.1038/nrm3151
Mcpherson, P. S. (1999). Regulatory role of SH3 domain-mediated proteinprotein interactions in synaptic vesicle endocytosis. Cell. Signal 11:229238. doi: 10.1016/S0898-6568(98)00059-X
Mears, J. A., Ray, P., and Hinshaw, J. E. (2007). A corkscrew model for dynamin constriction. Structure 15, 1190–1202. doi: 10.1016/j.str.2007.08.012
Mellman, I., and Warren, G. (2000). The road taken: past and future review foundations of membrane traffic. Cell 100, 99–112. doi: 10.1016/S0092-8674(00)81687-6
Mettlen, M., Chen, P., Srinivasan, S., Danuser, G., and Schmid, S. L. (2018). Regulation of clathrin-mediated endocytosis. Annu. Rev. Biochem. 87, 871–896. doi: 10.1146/annurev-biochem-062917-012644
Mettlen, M., Loerke, D., Yarar, D., Danuser, G., and Schmid, S. L. (2010). Cargo- and adaptor-specific mechanisms regulate clathrin-mediated endocytosis. J. Cell Biol. 188, 919–933. doi: 10.1083/jcb.200908078
Miele, A. E., Watson, P. J., Evans, P. R., Traub, L. M., and Owen, D. J. (2004). Two distinct interaction motifs in amphiphysin bind two independent sites on the clathrin terminal domain β-propeller. Nat. Struct. Mol. Biol. 11, 242–248. doi: 10.1038/nsmb736
Miller, S. E., Mathiasen, S., Bright, N. A., Pierre, F., Kelly, B. T., Kladt, N., et al. (2015). CALM regulates clathrin-coated vesicle size and maturation by directly sensing and driving membrane curvature. Dev. Cell 33, 163–175. doi: 10.1016/j.devcel.2015.03.002
Milosevic, I. (2018). Revisiting the role of clathrin-mediated endoytosis in synaptic vesicle recycling. Front. Cell. Neurosci. 12:27. doi: 10.3389/fncel.2018.00027
Mishra, S., and Murphy, L. J. (2004). Tissue transglutaminase has intrinsic kinase activity. Identification of transglutaminase 2 as an insulin-like growth factor-binding protein-3 kinase. J. Biol. Chem. 279, 23863–23868. doi: 10.1074/jbc.M311919200
Morgan, J. R., Prasad, K., Hao, W., Augustine, G. J., and Lafer, E. M. (2000). A conserved clathrin assembly motif essential for synaptic vesicle endocytosis. J. Neurosci. 20, 8667–8676. doi: 10.1523/JNEUROSCI.20-23-08667.2000
Morgan, J. R., Prasad, K., Jin, S., Augustine, G. J., and Lafer, E. M. (2001). Uncoating of clathrin-coated vesicles in presynaptic terminals: roles for Hsc70 and auxilin. Neuron 32, 289–300. doi: 10.1016/S0896-6273(01)00467-6
Morgan, J. R., Zhao, X., Womack, M., Prasad, K., Augustine, G. J., and Lafer, E. M. (1999). A role for the clathrin assembly domain of AP180 in synaptic vesicle endocytosis. J. Neurosci. 19, 10201–10212. doi: 10.1523/JNEUROSCI.19-23-10201.1999
Morlot, S., and Roux, A. (2013). Mechanics of dynamin-mediated membrane fission. Annu. Rev. Biophys. 42, 629–649. doi: 10.1146/annurev-biophys-050511-102247
Morris, S. A., and Schmid, S. L. (1995). The ferrari of endocytosis? Curr. Biol. 5, 113–115. doi: 10.1016/S0960-9822(95)00028-5
Motley, A., Bright, N. A., Seaman, M. N. J., and Robinson, M. S. (2003). Clathrin-mediated endocytosis in AP-2-depleted cells. J. Cell Biol. 162, 909–918. doi: 10.1083/jcb.200305145
Muenzner, J., Traub, L. M., Kelly, B. T., and Graham, S. C. (2017). Cellular and viral peptides bind multiple sites on the n-terminal domain of clathrin. Traffic 18, 44–57. doi: 10.1111/tra.12457
Muhlberg, A. B., Warnock, D. E., and Schmid, S. L. (1997). Domain structure and intramolecular regulation of dynamin GTPase. EMBO J. 16, 6676–6683. doi: 10.1093/emboj/16.22.6676
Muranen, T., Iwanicki, M. P., Curry, N. L., Hwang, J., DuBois, C. D., Coloff, J. L., et al. (2017). Starved epithelial cells uptake extracellular matrix for survival. Nat. Commun. 8, 1–12. doi: 10.1038/ncomms13989
Nakata, T., Iwamoto, A., Noda, Y., Takemura, R., Yoshikura, H., and Hirokawa, N. (1991). Predominant and developmentally regulated expression of dynamin in neurons. Neuron 7, 46–469. doi: 10.1016/0896-6273(91)90298-E
Nakata, T., Takemura, R., and Hirokawa, N. (1993). A novel member of the dynamin family of gtp-binding proteins is expressed specifically in the testis. J. Cell Sci. 105, 1–5. doi: 10.1242/jcs.105.1.1
Narayanan, R., Leonard, M., Byeong, D. S., Schmid, S. L., and Ramaswami, M. (2005). An internal GAP domain negatively regulates presynaptic dynamin in vivo: a two-step model for dynamin function. J. Cell Biol. 169, 117–126. doi: 10.1083/jcb.200502042
Nicholson-Fish, J. C., Kokotos, A. C., Gillingwater, T. H., Smillie, K. J., and Cousin, M. A. (2015). VAMP4 is an essential cargo molecule for activity-dependent bulk endocytosis. Neuron 88, 973–984. doi: 10.1016/j.neuron.2015.10.043
Nicoziani, P., Vilhardt, F., Llorente, A., Hilout, L., Courtoy, P. J., Sandvig, K., et al. (2000). Role for dynamin in late endosome dynamics and trafficking of the cation-independent mannose 6-phosphate receptor. Mol. Biol. Cell 11, 481–495. doi: 10.1091/mbc.11.2.481
Niemann, H. H., Knetsch, M. L. W., Scherer, A., Manstein, D. J., and Kull, F. J. (2001). Crystal Structure of a Dynamin GTPase Domain in Both Nucleotide-Free and GDP-Bound Forms. EMBO J. 20, 5813–5821. doi: 10.1093/emboj/20.21.5813
Nossal, R. (2001). Energetics of clathrin basket assembly. Traffic 2, 138–147. doi: 10.1034/j.1600-0854.2001.020208.x
Obar, R. A., Collins, C. A., Hammarback, J. A., Shpetner, H., and Vallee, R. B. (1990). Molecular cloning of the microtubule-associated mechanochemical enzyme dynamin reveals homology with a new family of GYP-binding proteins. Nature 347, 256–261. doi: 10.1038/347256a0
Ochoa, G.-C., Slepnev, V. I., Neff, L., Ringstad, N., Takei, K., Daniell, L., et al. (2000). A functional link between dynamin and the actin cytoskeleton at podosomes. J. Cell Biol. 150, 377–389. doi: 10.1083/jcb.150.2.377
Odell, L. R., Howan, D., Gordon, C. P., Robertson, M. J., Chau, N., Mariana, A., et al. (2010). The pthaladyns: GTP competitive inhibitors of dynamin I and II GTPase derived from virtual screening. J. Med. Chem. 53, 5267–5280. doi: 10.1021/jm100442u
Oh, P., Mcintosh, D. P., and Schnitzer, J. E. (1998). Dynamin at the neck of caveolae mediates their budding to form transport vesicles by gtp-driven fission from the plasma membrane of endothelium. J. Cell Biol. 141, 101–114. doi: 10.1083/jcb.141.1.101
Ohno, H., Stewart, J., Fournier, M.-C., Bosshart, H., Rhee, I., Miyatake, S., et al. (1995). Interaction of tyrosine-based sorting signals with clathrin-associated proteins. Science. (80-.). 269, 1872–1875. doi: 10.1126/science.7569928
Okamoto, P. M., Tripet, B., Litowski, J., Hodges, R. S., and Vallee, R. B. (1999). Multiple distinct coiled-coils are involved in dynamin self-assembly. J. Biol. Chem. 274, 10277–10286. doi: 10.1074/jbc.274.15.10277
Olichon, lien;, Emorine, L. J., Descoins, E., Pelloquin, L., Brichese, L., Gas, N., Guillou, E., et al. (2002). The human dynamin-related protein OPA1 is anchored to the mitochondrial inner membrane facing the inter-membrane space. Fed. Eur. Biochem. Soc. 523, 171–176. doi: 10.1016/S0014-5793(02)02985-X
Oliver, D., and Reddy, P. H. (2019). Dynamics of dynamin-related protein 1 in Alzheimer's disease and other neurodegenerative diseases. Cells 8, 1–20. doi: 10.3390/cells8090961
Olusanya, O., Andrews, P. D., Swedlow, J. R., and Smythe, E. (2001). Phosphorylation of threonine 156 of the 2 subunit of the AP2 complex is essential for endocytosis in vitro and in vivo. Curr. Biol. 5, 896–900. doi: 10.1016/S0960-9822(01)00240-8
Oña, G., and Bouso, J. C. (2020). Therapeutic potential of natural psychoactive drugs for central nervous system disorders: a perspective from polypharmacology. Curr. Med. Chem. 28, 53–68. doi: 10.2174/0929867326666191212103330
Orth, J. D., and McNiven, M. A. (2003). Dynamin at the actin-membrane interface. Curr. Opin. Cell Biol. 15, 31–39. doi: 10.1016/S0955-0674(02)00010-8
Otomo, M., Takahashi, K., Miyoshi, H., Osada, K., Nakashima, H., and Yamaguchi, N. (2008). Some selective serotonin reuptake inhibitors inhibit dynamin I guanosine triphosphatase (GTPase). Biol. Pharm. Bull. 31, 1489–1495. doi: 10.1248/bpb.31.1489
Overcoming Gaps in the Treatment of Neurodegenerative Disease (2020). EBioMedicine 60:103088. doi: 10.1016/j.ebiom.2020.103088
Palmer, A. M. (2011). Neuroprotective therapeutics for Alzheimers disease: progress and prospects. Trends Pharmacol. Sci. 32, 141–147. doi: 10.1016/j.tips.2010.12.007
Park, R. J., Shen, H., Liu, L., Liu, X., Ferguson, S. M., and De Camilli, P. (2013). Dynamin triple knockout cells reveal off target effects of commonly used dynamin inhibitors. J. Cell Sci. 126, 5305–5312. doi: 10.1242/jcs.138578
Pearse, B. M. F. (1975). Coated vesicles from pig brain: purification and biochemical characterization. J. Mol. Biol. 97, 93–98. doi: 10.1016/S0022-2836(75)80024-6
Pechstein, A., Bacetic, J., Vahedi-Faridi, A., Gromova, K., Sundborger, A., Tomlin, N., et al. (2010). Regulation of synaptic vesicle recycling by complex formation between intersectin 1 and the clathrin adaptor complex AP2. Proc. Natl. Acad. Sci. U. S. A. 107, 4206–4211. doi: 10.1073/pnas.0911073107
Persaud, A., Cormerais, Y., Pouyssegur, J., and Rotin, D. (2018). Dynamin inhibitors block activation of MTORC1 by amino acids independently of dynamin. J. Cell Sci. 131:jcs211755. doi: 10.1242/jcs.211755
Petronzi, C., Filosa, R., Peduto, A., Monti, M. C., Margarucci, L., Massa, A., et al. (2011). Structure-based design, synthesis and preliminary anti-inflammatory activity of bolinaquinone analogues. Eur. J. Med. Chem. 46, 488–496. doi: 10.1016/j.ejmech.2010.11.028
Pitkänen, A., and Immonen, R. (2014). Epilepsy related to traumatic brain injury. Neurotherapeutics 11, 286–296. doi: 10.1007/s13311-014-0260-7
Popescu, R., Heiss, E. H., Ferk, F., Peschel, A., Knasmueller, S., Dirsch, V. M., et al. (2011). Ikarugamycin induces DNA damage, intracellular calcium increase, P38 MAP kinase activation and apoptosis in HL-60 human promyelocytic leukemia cells. Mutat. Res. Fundam. Mol. Mech. Mutagen. 709–710, 60–66. doi: 10.1016/j.mrfmmm.2011.03.001
Popova, N.V., Deyev, I. E., and Petrenko, A. G. (2013). Clathrin-mediated endocytosis and adaptor proteins. Acta Nat. 5, 62–73. doi: 10.32607/20758251-2013-5-3-62-73
Powell, R., Young, V. A., Pryce, K. D., Sheehan, G. D., Bonsu, K., Ahmed, A., et al. (2021). Inhibiting endocytosis in CGRP+ nociceptors attenuates inflammatory pain-like behavior. Nat. Commun. 12, 1–15. doi: 10.1038/s41467-021-26100-6
Praefcke, G. J. K., and McMahon, H. T. (2004). The dynamin superfamily: universal membrane tubulation and fission molecules? Nat. Rev. Mol. Cell Biol. 5, 133–147. doi: 10.1038/nrm1313
Prakash, B., Praefcke, G. J. K., Renault, L., Wittinghofer, A., and Herrmann, C. (2000b). Structure of human guanylate-binding protein 1 representing a unique class of GTP-binding proteins. Nature 403, 567–571. doi: 10.1038/35000617
Prakash, B., Renault, L., Praefcke, G. J. K., Herrmann, C., and Wittinghofer, A. (2000a). Triphosphate structure of guanylate-binding protein 1and implications for nucleotide binding and GTPase mechanism. EMBO J. 19, 4555–4564. doi: 10.1093/emboj/19.17.4555
Pucadyil, T. J., and Schmid, S. L. (2008). Real-time visualization of dynamin-catalyzed membrane fission and vesicle release. Cell 135, 1263–1275. doi: 10.1016/j.cell.2008.11.020
Qualmann, B., Roos, J., Digregorio, P. J., and Kelly, R. B. (1999). Syndapin I, a synaptic dynamin-binding protein that associates with the neural wiskott-aldrich syndrome protein. Mol. Biol. Cell 10, 501–513. doi: 10.1091/mbc.10.2.501
Quan, A., McGeachie, A. B., Keating, D. J., Van Dam, E. M., Rusak, J., Chau, N., et al. (2007). Myristyl trimethyl ammonium bromide and octadecyl trimethyl ammonium bromide are surface-active small molecule dynamin inhibitors that block endocytosis mediated by dynamin I or dynamin II? Mol. Pharmacol. 72, 1425–1439. doi: 10.1124/mol.107.034207
Rafii, M. S., and Aisen, P. S. (2009). Recent developments in Alzheimer's disease therapeutics. BMC Med. 7, 1–4. doi: 10.1186/1741-7015-7-7
Raimondi, A., Ferguson, S. M., Lou, X., Armbruster, M., Paradise, S., Giovedi, S., et al. (2011). Overlapping role of dynamin isoforms in synaptic vesicle endocytosis. Neuron 70, 1100–1114. doi: 10.1016/j.neuron.2011.04.031
Ramachandran, R., Pucadyil, T. J., Liu, Y.-W., Acharya, S., Leonard, M., Lukiyanchuk, V., et al. (2009). Membrane insertion of the pleckstrin homology domain variable loop 1 is critical for dynamin-catalyzed vesicle scission. Mol. Biol. Cell 20, 4630–4639. doi: 10.1091/mbc.e09-08-0683
Ramachandran, R., and Schmid, S. L. (2018). The dynamin superfamily. Curr. Biol. 28, R411–R416. doi: 10.1016/j.cub.2017.12.013
Ramaswami, M., Rao, S., Van Der Bliek, A., Kelly, R. B., and Krishnan, K. S. (1993). Genetic studies on dynamin function in drosophila. J. Neurogenet. 9, 73–87. doi: 10.3109/01677069309083451
Ramjaun, A. R., and Mcpherson, P. S. (1996). Multiple amphiphysin II splice variants display differential clathrin binding: identification of two distinct clathrin-binding sites. J. Neurochem. 70, 2369–2376. doi: 10.1046/j.1471-4159.1998.70062369.x
Rapaport, D., Brunner, M., Neupert, W., and Westermann, B. (1998). Fzo1p is a mitochondrial outer membrane protein essential for the biogenesis of functional mitochondria in saccharomyces cerevisiae*. J. Biol. Chem. 273, 20150–20155. doi: 10.1074/jbc.273.32.20150
Rapoport, I., Boll, W., Yu, A., Kirchhausen, T., and Schmid, S. (2009). A motif in the clathrin heavy chain required for the Hsc70/auxilin uncoating reaction. Mol. Biol. Cell 19, 406–413. doi: 10.1091/mbc.e07-09-0870
Reider, A., Barker, S. L., Mishra, S. K., Im, Y. J., Maldonado-Báez, L., Hurley, J. H., et al. (2009). Syp1 is a conserved endocytic adaptor that contains domains involved in cargo selection and membrane tubulation. EMBO J. 28, 3103–3116. doi: 10.1038/emboj.2009.248
Renard, H. F., and Boucrot, E. (2021). Unconventional endocytic mechanisms. Curr. Opin. Cell Biol. 71, 120–129. doi: 10.1016/j.ceb.2021.03.001
Reszka, A. A., and Rodan, G. A. (2003). Mechanism of action of bisphosphonates. Curr. Osteoporos. Rep. 1, 45–52. doi: 10.1007/s11914-003-0008-5
Reubold, T. F., Faelber, K., Plattner, N., Posor, Y., Ketel, K., Curth, U., et al. (2015). Crystal structure of the dynamin tetramer. Nature 525, 404–408. doi: 10.1038/nature14880
Robertson, M. J., Hadzic, G., Ambrus, J., Pom,è, D. Y., Hyde, E., Whiting, A., et al. (2012). The rhodadyns, a new class of small molecule inhibitors of dynamin gtpase activity. ACS Med. Chem. Lett. 3, 352–356. doi: 10.1021/ml200284s
Robertson, M. J., Horatscheck, A., Sauer, S., Von Kleist, L., Baker, J. R., Stahlschmidt, W., et al. (2016). 5-Aryl-2-(naphtha-1-Yl)sulfonamido-thiazol-4(5: H)-ones as clathrin inhibitors. Org. Biomol. Chem. 14, 11266–11278. doi: 10.1039/C6OB02308H
Robinson, M. S. (2015). Forty years of clathrin-coated vesicles. Traffic 16, 1210–1238. doi: 10.1111/tra.12335
Robinson, M. S., Sahlender, D. A., and Foster, S. D. (2010). Rapid inactivation of proteins by rapamycin-induced rerouting to mitochondria. Dev. Cell 18, 324–331. doi: 10.1016/j.devcel.2009.12.015
Robinson, P. J., Hauptschein, R., Lovenberg, W., and Dunkley, P. R. (1987). Dephosphorylation of synaptosomal proteins P96 and P139 Is regulated by both depolarization and calcium, but not by a rise in cytosolic calcium alone. J. Neurochem. 48, 187–195. doi: 10.1111/j.1471-4159.1987.tb13146.x
Robinson, P. J., Liu, J.-P., Powell, K. A., Fykse, E. M., and Sudhof, T. C. (1994). Phosphorylation of Dynamin I and Synaptic-Veside Recycling. Trends Neurosci. 17, 348–353. doi: 10.1016/0166-2236(94)90179-1
Roth, T. F., and Porter, K. R. (1964). Yolk protein uptake in the oocyte of mosquito Aedes aegypti L. J. Cell Biol. 20, 313–332. doi: 10.1083/jcb.20.2.313
Roux, A., Koster, G., Lenz, M., Sorre, B., Manneville, J. B., Nassoy, P., et al. (2010). Membrane curvature controls dynamin polymerization. Proc. Natl. Acad. Sci. U. S. A. 107, 4141–4146. doi: 10.1073/pnas.0913734107
Roux, A., Uyhazi, K., Frost, A., and De Camilli, P. (2006). GTP-dependent twisting of dynamin implicates constriction and tension in membrane fission. Nature 441, 528–531. doi: 10.1038/nature04718
Royle, S. J. (2006). The cellular functions of clathrin. Cell. Mol. Life Sci. 63, 1823–1832. doi: 10.1007/s00018-005-5587-0
Saffarian, S., Cocucci, E., and Kirchhausen, T. (2009). Distinct dynamics of endocytic clathrin-coated pits and coated plaques. PLoS Biol. 7:e1000191. doi: 10.1371/journal.pbio.1000191
Saleem, M., Morlot, S., Hohendahl, A., Manzi, J., Lenz, M., and Roux, A. (2015). A balance between membrane elasticity and polymerization energy sets the shape of spherical clathrin coats. Nat. Commun. 6:6249. doi: 10.1038/ncomms7249
Salim, K., Bottomley, M. J., Querfurth, E., Zvelebil, M. J., Gout, I., Scaife, R., et al. (1996). Distinct specificity in the recognition of phosphoinositides by the pleckstrin homology domains of dynamin and bruton's tyrosine kinase. EMBO J. 15, 6241–6250. doi: 10.1002/j.1460-2075.1996.tb01014.x
Santel, A., and Fulller, M. T. (2000). Control of mitochondrial morphology by a humanmitofusin. J. Cell Sci. 114, 867–874. doi: 10.1242/jcs.114.5.867
Santini, F., Gaidarov, I., and Keen, J. H. (2002). G protein-coupled receptor/arrestin3 modulation of the endocytic machinery. J. Cell Biol. 156, 665–676. doi: 10.1083/jcb.200110132
Satoh, M., Hamamoto, T., Seo, N., Kagawa, Y., and Endo, H. (2003). Differential sublocalization of the dynamin-related protein OPA1 isoforms in mitochondria. Biochem. Biophys. Res. Commun. 300, 482–493. doi: 10.1016/S0006-291X(02)02874-7
Scarmato, P., and Kirchhausen, T. (1990). Analysis of clathrin light chain-heavy chain interactions using truncated mutants of rat liver light chain LCB3*. J. Biol. Chem. 265:36614666. doi: 10.1016/S0021-9258(19)39644-9
Schlegel, R., Dickson, R. B., Willingham, M. C., and Pastan, I. H. (1982). Amantadine and dansylcadaverine inhibit vesicular stomatitis virus uptake and receptor-mediated endocytosis of 2-macroglobulin. Proc. Natl. Acad. Sci. U.S.A. 79, 2291–2295. doi: 10.1073/pnas.79.7.2291
Schlossman, D. M., Schmid, S. L., Braell, W. A., and Rothman, J. E. (1984). An enzyme that removes clathrin coats : purification of an uncoating ATPase. J. Cell Biol. 99, 723–733. doi: 10.1083/jcb.99.2.723
Schubert, K. O., Föcking, M., Prehn, J. H. M., and Cotter, D. R. (2012). Hypothesis review: are clathrin-mediated endocytosis and clathrin-dependent membrane and protein trafficking core pathophysiological processes in schizophrenia and bipolar disorder. Mol. Psychiatry 17, 669–681. doi: 10.1038/mp.2011.123
Scott, B. L., Sochacki, K. A., Low-Nam, S. T., Bailey, E. M., Luu, Q. A., Hor, A., et al. (2018). Membrane bending occurs at all stages of clathrincoat assembly and defines endocytic dynamics. Nat. Commun. 9:419. doi: 10.1038/s41467-018-02818-8
Sever, S., Muhlberg, A. B., and Schmid, S. L. (1999). Impairment of dynamin's GAP domain stimulates receptor-mediated endocytosis. Nature 398, 481–486. doi: 10.1038/19024
Shpetner, H. S., Herskovits, J. S., and Vallee, R. B. (1996). A binding site for SH3 domains targets dynamin to coated pits. J. Biol. Chem. 271, 13–16. doi: 10.1074/jbc.271.1.13
Shpetner, H. S., and Vallee, R. B. (1989). Identification of dynamin, a novel mechanochemical enzyme that mediates interactions between microtubules. Cell 59, 421–432. doi: 10.1016/0092-8674(89)90027-5
Shupliakov, O., Löw, P., Grabs, D., Gad, H., Chen, H., David, C., et al. (1997). Synaptic vesicle endocytosis impaired by disruption of dynamin–SH3Domain interactions. Science. (80-.). 276, 259–263. doi: 10.1126/science.276.5310.259
Sidiropoulos, P. N. M., Miehe, M., Bock, T., Tinelli, E., Oertli, C. I., Kuner, R., et al. (2012). Dynamin 2 mutations in charcot-marie-tooth neuropathy highlight the importance of clathrin-mediated endocytosis in myelination. Brain 135, 1395–1411. doi: 10.1093/brain/aws061
Silm, K., Yang, J., Marcott, P. F., Asensio, C. S., Eriksen, J., Guthrie, D. A., et al. (2019). Synaptic vesicle recycling pathway determines neurotransmitter content and release properties. Neuron 102, 786–800.e5. doi: 10.1016/j.neuron.2019.03.031
Simpson, F., Hussain, N. K., Qualmann, B., Kelly, R. B., Kay, B. K., McPherson, P. S., et al. (1999). SH3-domain-containing proteins function at distinct steps in clathrin-coated vesicle formation. Nat. Cell Biol. 1, 119–124. doi: 10.1038/10091
Singh, M., Jadhav, H. R., and Bhatt, T. (2017). Dynamin functions and ligands: classical mechanisms behind. Mol. Pharmacol. 91, 123–134. doi: 10.1124/mol.116.105064
Singh, U. S., Pan, J., Kao, Y. L., Joshi, S., Young, K. L., and Baker, K. M. (2003). Tissue transglutaminase mediates activation of RhoA and MAP kinase pathways during retinoic acid-induced neuronal differentiation of SH-SY5Y cells. J. Biol. Chem. 278, 391–399. doi: 10.1074/jbc.M206361200
Skretting, G., Torgersen, M. L., Van Deurs, B., and Sandvig, K. (1999). Endocytic mechanisms responsible for uptake of GPI-linked diphtheria toxin receptor. J. Cell Sci. 112, 3899–3909. doi: 10.1242/jcs.112.22.3899
Smillie, K. J., Pawson, J., Perkins, E. M., Jackson, M., and Cousin, M. A. (2013). Control of synaptic vesicle endocytosis by an extracellular signalling molecule. Nat. Commun. 4, 1–10. doi: 10.1038/ncomms3394
Smirnova, E., Shurland, D. L., Newman-Smith, E. D., Pishvaee, B., and Van Der Bliek, A. M. (1999). A Model for dynamin self-assembly based on binding between three different protein domains. J. Biol. Chem. 274, 14942–14947. doi: 10.1074/jbc.274.21.14942
Smith, C. M., and Chircop, M. (2012). Clathrin-mediated endocytic proteins are involved in regulating mitotic progression and completion. Traffic 13, 1628–1641. doi: 10.1111/tra.12001
Smith, C. M., Haucke, V., McCluskey, A., Robinson, P. J., and Chircop, M. (2013). Inhibition of clathrin by pitstop 2 activates the spindle assembly checkpoint and induces cell death in dividing hela cancer cells. Mol. Cancer 12, 1–15. doi: 10.1186/1476-4598-12-4
Song, B. D., Leonard, M., and Schmid, S. L. (2004). Dynamin GTPase domain mutants that differentially affect GTP binding, GTP hydrolysis, and clathrin-mediated endocytosis. J. Biol. Chem. 279, 40431–40436. doi: 10.1074/jbc.M407007200
Sontad, J.-M., Fykses, E. M., Ushkaryovs, Y., Liu,5, J.-P., Robinson, P. J., and Sudhofsn, T. C. (1994). Differential expression and regulation of multiple dynamins*. J. Biol. Chem. 269, 4547–4554. doi: 10.1016/S0021-9258(17)41812-6
Sorkina, T., Miranda, M., Dionne, K. R., Hoover, B. R., Zahniser, N. R., and Sorkin, A. (2006). RNA interference screen reveals an essential role of Nedd4-2 in dopamine transporter ubiquitination and endocytosis. J. Neurosci. 26, 8195–8205. doi: 10.1523/JNEUROSCI.1301-06.2006
Sousa, R., Liao, H. S., Cuéllar, J., Jin, S., Valpuesta, J. M., Jin, A. J., et al. (2016). Clathrin-coat disassembly illuminates the mechanisms of Hsp70 force generation. Nat. Struct. Mol. Biol. 23, 821–829. doi: 10.1038/nsmb.3272
Soykan, T., Kaempf, N., Sakaba, T., Vollweiter, D., Goerdeler, F., Puchkov, D., et al. (2017). Synaptic vesicle endocytosis occurs on multiple timescales and is mediated by formin-dependent actin assembly. Neuron 93, 854–866.e4. doi: 10.1016/j.neuron.2017.02.011
Soykan, T., Maritzen, T., and Haucke, V. (2016). Modes and mechanisms of synaptic vesicle recycling. Curr. Opin. Neurobiol. 39, 17–23. doi: 10.1016/j.conb.2016.03.005
Staeheli, P., Haller, O., Boll, W., Lindenmann, J., and Weissmann, C. (1986). Mx protein: constitutive expression in 3t3 cells transformed with cloned Mx CDNA confers selective resistance to influenza virus. Cell 44, 147–158. doi: 10.1016/0092-8674(86)90493-9
Stahlschmidt, W., Robertson, M. J., Robinson, P. J., McCluskey, A., and Haucke, V. (2014). Clathrin terminal domain-ligand interactions regulate sorting of mannose 6-phosphate receptors mediated by AP-1 and GGA adaptors. J. Biol. Chem. 289, 4906–4918. doi: 10.1074/jbc.M113.535211
Stimpson, H. E. M., Toret, C. P., Cheng, A. T., Pauly, B. S., Drubin, D. G., and Schmid, S. L. (2009). Early-arriving syp1p and ede1p function in endocytic site placement and formation in budding yeast. Mol. Biol. Cell 20, 4640–4651. doi: 10.1091/mbc.e09-05-0429
Stowell, M. H. B., Marks, B., Wigge, P., and Mcmahon, H. T. (1999). Nucleotide-dependent conformational changes in dynamin: evidence for a mechanochemical molecular spring. Nat. Cell Biol. 1, 27–32. doi: 10.1038/8997
Sweitzer, S. M., and Hinshaw, J. E. (1998). Dynamin undergoes a GTP-dependent conformational change causing vesiculation. Cell 93, 1021–1029. doi: 10.1016/S0092-8674(00)81207-6
Szigeti, K., and Lupski, J. R. (2009). Charcot-marie-tooth disease. Eur. J. Hum. Genet. 17, 703–710. doi: 10.1038/ejhg.2009.31
Takahashi, K., Miyoshi, H., Otomo, M., Osada, K., Yamaguchi, N., and Nakashima, H. (2010). Suppression of dynamin GTPase activity by sertraline leads to inhibition of dynamin-dependent endocytosis. Biochem. Biophys. Res. Commun. 391, 382–387. doi: 10.1016/j.bbrc.2009.11.067
Takei, K., Haucke, V., Slepnev, V., Farsad, K., Salazar, M., Chen, H., et al. (1998). Generation of coated intermediates of clathrin-mediated endocytosis on protein-free liposomes. Cell 94, 131–141. doi: 10.1016/S0092-8674(00)81228-3
Takei, K., McPherson, P. S., Schmid, S. L., and De Camilli, P. (1995). Tubular membrane invaginations coated by dynamin rings are induced by GTP-ΓS in nerve terminals. Nature 374, 186–190. doi: 10.1038/374186a0
Tasfaout, H., Buono, S., Guo, S., Kretz, C., Messaddeq, N., Booten, S., et al. (2017). Antisense oligonucleotide-mediated Dnm2 knockdown prevents and reverts myotubular myopathy in mice. Nat. Commun. 8, 1–13. doi: 10.1038/ncomms15661
Taylor, M. J., Perrais, D., and Merrifield, C. J. (2011). A high precision survey of the molecular dynamics of mammalian clathrin-mediated endocytosis. PLoS Biol. 9:e1000604. doi: 10.1371/journal.pbio.1000604
Tebar, F., Sorkina, T., Sorkin, A., Ericsson, M., and Kirchhausen, T. (1996). Eps15 is a component of clathrin-coated pits and vesicles and is located at the rim of coated pits. J. Biol. Chem. 271, 28727–28730. doi: 10.1074/jbc.271.46.28727
Ter Haar, E., Musacchio, A., Harrison, S. C., and Kirchhausen, T. (1998). Atomic structure of clathrin: a β propeller terminal domain joins an α zigzag linker. Cell 95, 563–573. doi: 10.1016/S0092-8674(00)81623-2
Timm, D., Salim, K., Gout, I., Guruprasad, L., Waterfield, M., and Blundell, T. (1994). Crystal structure of the pleckstrin homology domain from dynamin. Nat. Struct. Biol. 1, 782–788. doi: 10.1038/nsb1194-782
Traub, L. M. (2009). Tickets to ride: selecting cargo for clathrin-regulated internalization. Nat. Rev. Mol. Cell Biol. 10, 583–596. doi: 10.1038/nrm2751
Traub, L. M., and Bonifacino, J. S. (2013). Cargo recognition in clathrin-mediated endocytosis. Cold Spring Harb. Perspect. Biol. 5:a016790. doi: 10.1101/cshperspect.a016790
Tremblay, C. S., Chiu, S. K., Saw, J., McCalmont, H., Litalien, V., Boyle, J., et al. (2020). Small molecule inhibition of dynamin-dependent endocytosis targets multiple niche signals and impairs leukemia stem cells. Nat. Commun. 11:6211. doi: 10.1038/s41467-020-20091-6
Umasankar, P. K., Sanker, S., Thieman, J. R., Chakraborty, S., Wendland, B., Tsang, M., et al. (2012). Distinct and separable activities of the endocytic clathrin-coat components Fcho1/2 and AP-2 in developmental patterning. Nat. Cell Biol. 14, 488–501. doi: 10.1038/ncb2473
Umeda, A., Meyerholz, A., and Ungewickell, E. (2000). Identification of the universal cofactor (Auxilin 2) in clathrin coat dissociation. Eur. J. Cell Biol. 79, 336–342. doi: 10.1078/S0171-9335(04)70037-0
Ungewickell, E., Branton, D., Ernst, U., and Daniel, B. (1981). Assembly units of clathrin coats. Nature 289, 420–422. doi: 10.1038/289420a0
Vallis, Y., Wigge, P., Marks, B., Evans, P. R., and Mcmahon, H. T. (1999). Importance of the pleckstrin homology domain of dynamin in clathrin-mediated endocytosis results and discussion overexpression of dynamin-1 lacking its ph domain blocks endocytosis in COS cells. Curr. Biol. 9, 257–260. doi: 10.1016/S0960-9822(99)80114-6
Van Dam, E. M., and Stoorvogel, W. (2002). Dynamin-dependent transferrin receptor recycling by endosome-derived clathrin-coated vesicles. Mol. Biol. Cell 13, 169–182. doi: 10.1091/mbc.01-07-0380
van der Bliek, A. M. (1999). Functional diversity in the dynamin family. Trends Cell Biol. 9, 96–102. doi: 10.1016/S0962-8924(98)01490-1
Van der Bliek, A. M., Redelmeier, T. E., Damke, H., Tisdale, E. J., Meyerowitz, E. M., and Schmid, S. L. (1993). Mutations in human dynamin block an intermediate stage in coated vesicle formation. J. Cell Biol. 122, 553–563. doi: 10.1083/jcb.122.3.553
Vannini, E., Restani, L., Dilillo, M., McDonnell, L. A., Caleo, M., and Marra, V. (2020). Synaptic vesicles dynamics in neocortical epilepsy. Front. Cell. Neurosci. 14:606142. doi: 10.3389/fncel.2020.606142
Veiga, E., Guttman, J. A., Bonazzi, M., Toledo-arana, A., Lin, A. E., Enninga, J., et al. (2010). Invasive and adherent bacterial pathogens Co-Opt host clathrin for infection. Cell 2, 340–351. doi: 10.1016/j.chom.2007.10.001
Vetter, I. R., and Wittinghofer, A. (2001). The guanine nucleotide-binding switch in three dimensions. Science. (80-.). 294, 1299–1304. doi: 10.1126/science.1062023
Vidyadhara, D. J., Lee, J. E., and Chandra, S. S. (2019). Role of the endolysosomal system in Parkinson's disease. J. Neurochem. 150, 487–506. doi: 10.1111/jnc.14820
Von Kleist, L., Stahlschmidt, W., Bulut, H., Gromova, K., Puchkov, D., Robertson, M. J., et al. (2011). Role of the clathrin terminal domain in regulating coated pit dynamics revealed by small molecule inhibition. Cell 146, 471–484. doi: 10.1016/j.cell.2011.06.025
Wang, L.-H. H., Rothberg, K. G., and Anderson, R. G. W. W. (1993). Mis-assembly of clathrin lattices on endosomes reveals a regulatory switch for coated pit formation. J. Cell Biol. 123, 1107–1117. doi: 10.1083/jcb.123.5.1107
Warnock, D. E., Baba, T., and Schmid, S. L. (1997). Ubiquitously expressed dynamin-II has a higher intrinsic GTPase activity and a greater propensity for self-assembly than neuronal dynamin-I. Mol. Biol. Cell 8, 2553–2562. doi: 10.1091/mbc.8.12.2553
Warnock, D. E., and Schmid, S. L. (1996). Dynamin GTPase, a force-generating molecular switch. BioEssays 18, 885–893. doi: 10.1002/bies.950181107
Watanabe, S., and Boucrot, E. (2017). Fast and ultrafast endocytosis. Curr. Opin. Cell Biol. 47, 64–71. doi: 10.1016/j.ceb.2017.02.013
Watanabe, S., Liu, Q., Davis, M. W., Hollopeter, G., Thomas, N., Jorgensen, N. B., et al. (2013b). Ultrafast endocytosis at caenorhabditis elegans neuromuscular junctions. Elife 2:e00723. doi: 10.7554/eLife.00723.023
Watanabe, S., Rost, B. R., Camacho-Pérez, M., Davis, M. W., Söhl-Kielczynski, B., Rosenmund, C., et al. (2013a). Ultrafast endocytosis at mouse hippocampal synapses. Nature 504, 242–247. doi: 10.1038/nature12809
Watanabe, S., Trimbuch, T., Camacho-Pérez, M., Rost, B. R., Brokowski, B., Söhl-Kielczynski, B., et al. (2014). Clathrin regenerates synaptic vesicles from endosomes. Nature 515, 228–233. doi: 10.1038/nature13846
Wen, X., Saltzgaber, G. W., and Thoreson, W. B. (2017). Kiss-and-run is a significant contributor to synaptic exocytosis and endocytosis in photoreceptors. Front. Cell. Neurosci. 11:286. doi: 10.3389/fncel.2017.00286
Wightman, R. M., and Haynes, C. L. (2004). Synaptic vesicles really do kiss and run. Nat. Neurosci. 7, 321–322. doi: 10.1038/nn0404-321
Willox, A. K., and Royle, S. J. (2012). Functional analysis of interaction sites on the n-terminal domain of clathrin heavy chain. Traffic 13, 70–81. doi: 10.1111/j.1600-0854.2011.01289.x
Willox, A. K., Sahraoui, Y. M. E., and Royle, S. J. (2014). Non-specificity of pitstop 2 in clathrin-mediated endocytosis. Biol. Open 3, 326–331. doi: 10.1242/bio.20147955
Wu, F., and Yao, P. J. (2009). Clathrin-mediated endocytosis and Alzheimer's disease: an update. Ageing Res. Rev. 8, 147–149. doi: 10.1016/j.arr.2009.03.002
Wu, W., and Wu, L.-G. (2007). Rapid bulk endocytosis and its kinetics of fission pore closure at a central synapse. Proc. Natl. Acad. Sci.U.S.A. 104, 10234–10239. doi: 10.1073/pnas.0611512104
Wu, Y., O'Toole, E. T., Girard, M., Ritter, B., Messa, M., Liu, X., et al. (2014). A dynamin 1-, dynamin 3- and clathrin-independent pathway of synaptic vesicle recycling mediated by bulk endocytosis. Elife 3:e01621. doi: 10.7554/eLife.01621.018
Xing, Y., Böcking, T., Wolf, M., Grigorieff, N., Kirchhausen, T., and Harrison, S. C. (2010). Structure of clathrin coat with bound Hsc70 and auxilin: mechanism of Hsc70-facilitated disassembly. EMBO J. 29, 655–665. doi: 10.1038/emboj.2009.383
Yan, L., Qi, Y., Huang, X., Yu, C., Lan, L., Guo, X., et al. (2018). Structural basis for GTP hydrolysis and conformational change of MFN1 in mediating membrane fusion. Nat. Struct. Mol. Biol. 25, 233–243. doi: 10.1038/s41594-018-0034-8
Yang, N., Hong, X., Yang, P., Ju, X., Wang, Y., Tang, J., et al. (2011). The 2009 pandemic A/Wenshan/01/2009 H1N1 induces apoptotic cell death in human airway epithelial cells. J. Mol. Cell Biol. 3, 221–229. doi: 10.1093/jmcb/mjr017
Young, A. (2007). Structural insights into the clathrin coat. Semin. Cell Dev. Biol. 18, 448–458. doi: 10.1016/j.semcdb.2007.07.006
Yu, A., Shibata, Y., Shah, B., Calamini, B., Lo, D. C., and Morimoto, R. I. (2014). Protein aggregation can inhibit clathrin-mediated endocytosis by chaperone competition. Proc. Natl. Acad. Sci. U.S.A. 111, E1481–E1490. doi: 10.1073/pnas.1321811111
Zeviani, M., and Carelli, V. (2005). Dominance in mitochondrial disorders. J. Inherit. Metab. Dis. 28, 287–299. doi: 10.1007/s10545-005-0307-3
Zhang, J., Lawrance, G. A., Chau, N., Robinson, P. J., and McCluskey, A. (2008). From spanish fly to room-temperature ionic liquids (RTILs): synthesis, thermal stability and inhibition of dynamin 1 GTPase by a novel class of RTILs. New J. Chem. 32, 28–36. doi: 10.1039/B707092F
Zhang, P., and Hinshaw, J. E. (2001). Three-dimensional reconstruction of dynamin in the constricted state. Nat. Cell Biol. 3, 922–926. doi: 10.1038/ncb1001-922
Zhang, Q., Li, Y., and Tsien, R. W. (2009). The dynamic control of kiss-and-run and vesicular reuse probed with single nanoparticles. Science. (80-.). 323, 1448–1453. doi: 10.1126/science.1167373
Zhao, X., Greener, T., Al-Hasani, H., Cushman, S. W., Eisenberg, E., and Greene, L. E. (2001). Expression of auxilin or AP180 inhibits endocytosis by mislocalizing clathrin: evidence for formation of nascent pits containing AP1 or AP2 but not clathrin. J. Cell Sci. 114, 353–365. doi: 10.1242/jcs.114.2.353
Zheng, J., Cahill, S. M., Lemmon, M. A., Fushman, D., Schlessinger, J., and Cowburn, D. (1996). Identification of the binding site for acidic phospholipids on the PH domain of dynamin: implications for stimulation of GTPase activity. J. Mol. Biol. 255, 14–21. doi: 10.1006/jmbi.1996.0002
Zhou, L., McInnes, J., and Verstreken, P. (2014). Ultrafast synaptic endocytosis cycles to the center stage. Dev. Cell 28, 5–6. doi: 10.1016/j.devcel.2013.12.017
Keywords: clathrin mediated endocytosis (CME), clathrin, dynamin, inhibitors, synaptic vesicle recycling (SVR), neurological disorders
Citation: Prichard KL, O'Brien NS, Murcia SR, Baker JR and McCluskey A (2022) Role of Clathrin and Dynamin in Clathrin Mediated Endocytosis/Synaptic Vesicle Recycling and Implications in Neurological Diseases. Front. Cell. Neurosci. 15:754110. doi: 10.3389/fncel.2021.754110
Received: 05 August 2021; Accepted: 10 December 2021;
Published: 18 January 2022.
Edited by:
Daniela Zizioli, University of Brescia, ItalyReviewed by:
Karen Janet Smillie, University of Edinburgh, United KingdomCopyright © 2022 Prichard, O'Brien, Murcia, Baker and McCluskey. This is an open-access article distributed under the terms of the Creative Commons Attribution License (CC BY). The use, distribution or reproduction in other forums is permitted, provided the original author(s) and the copyright owner(s) are credited and that the original publication in this journal is cited, in accordance with accepted academic practice. No use, distribution or reproduction is permitted which does not comply with these terms.
*Correspondence: Adam McCluskey, YWRhbS5tY2NsdXNrZXlAbmV3Y2FzdGxlLmVkdS5hdQ==
†ORCID: Kate L. Prichard orcid.org/0000-0003-0458-5037
Nicholas S. O'Brien orcid.org/0000-0003-2110-8830
Sari R. Murcia orcid.org/0000-0001-9040-1032
Jennifer R. Baker orcid.org/0000-0002-9560-301ZX
Adam McCluskey orcid.org/0000-0001-7125-863X
Disclaimer: All claims expressed in this article are solely those of the authors and do not necessarily represent those of their affiliated organizations, or those of the publisher, the editors and the reviewers. Any product that may be evaluated in this article or claim that may be made by its manufacturer is not guaranteed or endorsed by the publisher.
Research integrity at Frontiers
Learn more about the work of our research integrity team to safeguard the quality of each article we publish.