- 1Neuroscience Institute, National Research Council (IN-CNR), Padua, Italy
- 2Department of Biomedical Sciences, University of Padua, Padua, Italy
The glial cells astrocytes have long been recognized as important neuron-supporting elements in brain development, homeostasis, and metabolism. After the discovery that the reciprocal communication between astrocytes and neurons is a fundamental mechanism in the modulation of neuronal synaptic communication, over the last two decades astrocytes became a hot topic in neuroscience research. Crucial to their functional interactions with neurons are the cytosolic Ca2+ elevations that mediate gliotransmission. Large attention has been posed to the so-called Ca2+microdomains, dynamic Ca2+ changes spatially restricted to fine astrocytic processes including perisynaptic astrocytic processes (PAPs). With presynaptic terminals and postsynaptic neuronal membranes, PAPs compose the tripartite synapse. The distinct spatial-temporal features and functional roles of astrocyte microdomain Ca2+ activity remain poorly defined. However, thanks to the development of genetically encoded Ca2+ indicators (GECIs), advanced microscopy techniques, and innovative analytical approaches, Ca2+ transients in astrocyte microdomains were recently studied in unprecedented detail. These events have been observed to occur much more frequently (∼50–100-fold) and dynamically than somatic Ca2+ elevations with mechanisms that likely involve both IP3-dependent and -independent pathways. Further progress aimed to clarify the complex, dynamic machinery responsible for astrocytic Ca2+ activity at microdomains is a crucial step in our understanding of the astrocyte role in brain function and may also reveal astrocytes as novel therapeutic targets for different brain diseases. Here, we review the most recent studies that improve our mechanistic understanding of the essential features of astrocyte Ca2+ microdomains.
Introduction
Studies performed over the last two decades have revealed that astrocytes are intrinsic elements in brain circuits that through reciprocal signaling with neurons contribute to fundamental phenomena in brain function (Araque et al., 2014). Intracellular Ca2+ transients, which are evoked by different neurotransmitters, are fundamental for astrocyte signaling, releasing gliotransmitters (Bezzi and Volterra, 2001) that differently modulate synaptic transmission (Di Castro et al., 2011; Panatier et al., 2011), long-term synaptic plasticity (Henneberger et al., 2010; Gómez-Gonzalo et al., 2015; Vignoli et al., 2016; Papouin et al., 2017; Robin et al., 2018), cognitive functions, and behavior (Santello et al., 2019; Kofuji and Araque, 2021; Nagai et al., 2021).
Initially centered on astrocytic soma or main processes (Cornell-Bell et al., 1990; Parpura et al., 1994), early investigations then focused on small processes, revealing that Ca2+ elevations can either remain restricted locally or eventually propagate to the main processes and soma (Pasti et al., 1997). Localized Ca2+ events were first described as microdomains in Bergmann glia processes (Grosche et al., 1999). Then, microdomains were observed in different ex vivo and in vivo brain preparations, becoming the focus of several recent studies and reviews (Volterra et al., 2014; Bazargani and Attwell, 2016; Semyanov et al., 2020). A strict and shared definition of microdomains is currently missing because of the lack of knowledge of these events. Like others, we use the term microdomains to describe Ca2+ events that are restricted to small portions of individual astrocyte territories that can be assigned to astrocytic thin processes with high-resolution imaging techniques.
While the molecular mechanisms and physiological roles of astrocyte Ca2+ microdomains remain poorly defined, the development of GECIs and advanced microscopy techniques revealed a high complexity of microdomain spatial and temporal profiles (Shigetomi et al., 2013a; Srinivasan et al., 2015; Agarwal et al., 2017; Bindocci et al., 2017). Although generally much slower than neuronal Ca2+ events, Ca2+ microdomains in astrocytes may exhibit fast kinetics on a timescale similar to neurons (Stobart et al., 2018). Notably, most studies on microdomains have to face technical limitations due to the nanoscopic size and the intrinsic remarkable dynamics of these structures (Semyanov et al., 2020). These limitations include: (i) the resolution of optical microscopy, which should be ideally paralleled by super-resolution microscopy; (ii) the type and concentration of the Ca2+ indicator used that buffers intracellular Ca2+ transients; (iii) the possible photostimulation or photodamage; (iv) the use of 2D scanning as opposed to ideal 3D acquisition; (v) the type of analysis that has to consider the highly dynamic spatial-temporal profile of these events. Despite these limitations, several recent studies revealed that the intracellular Ca2+ activity occurring in ultra-small processes (i.e., microdomains), where “the most important calcium transients occur,” is crucial for the dynamic cooperation with neurons (Bazargani and Attwell, 2016). In this review, we highlight the most recent advances in the different aspects that characterize astrocyte Ca2+ microdomains.
The Locus of Ca2+ Microdomains
Elucidating the fine anatomy of astrocytic processes is crucial for understanding how PAPs contact synapses and affect neuronal functions. Astrocyte complex morphology is mainly composed of nanoscopic irregular protrusions that are highly fluid and plastic (Bernardinelli et al., 2014; Rusakov, 2015) and account for the majority of astrocyte volume (75–85%) (Bushong et al., 2002; Bindocci et al., 2017). These structures are below the resolution of optical microscopy with smaller processes 30–50 nm in size (Rusakov, 2015). A shared nomenclature for these structures is missing, with different terms being used, including terms such as leaflets (Gavrilov et al., 2018), nodes, and shafts (Arizono et al., 2020), or more generically gliapil, in analogy with neuropil (Bindocci et al., 2017). Serial electron microscopy (EM) with 3D reconstruction (Ventura and Harris, 1999; Calì et al., 2019) revealed the intracellular organization of astrocytic organelles, while EM imaging of astrocytes after fluorescence dye-loading through a patch pipette has revealed the fine details of PAPs (Bushong et al., 2002).
The nanoscopic size of these structures is an obstacle for functional imaging of Ca2+ activity and innovative imaging approaches are needed (Heller and Rusakov, 2017). A recent study using 3D-super resolution microscopy combined with Ca2+ imaging revealed that astrocyte processes form a spongiform domain composed of a meshwork of fine structures with nodes and shafts that may form loops of reconnecting processes (Arizono et al., 2020). In line with a previous study (Shigetomi et al., 2013a), Arizono et al. (2020) found that nodes and shafts are equally present in the entire astrocyte territory irrespective of the distance from the soma. Astrocyte morphology is thus like a sponge with the meshwork of ultrathin small processes that cover the surrounding territory (Rusakov, 2015; Arizono et al., 2020), in contrast with the classic view of star shaped cells. Analysis of the spatial relationship with excitatory synapses revealed that most PAPs are nodes, i.e., compartmentalized structures (0.07–0.7 μm2), where the majority of localized Ca2+ transients occur and may or may not propagate to nearby shafts. A strict correlation was reported between node and spine size, and also between Ca2+ transients and spine size (Arizono et al., 2020), suggesting that PAPs are morphologically and functionally correlated with their synaptic partners. Accordingly, nodes would be the “primary site” of Ca2+ signaling in excitatory tripartite synapses and may represent specialized compartments endowed with all the machinery needed for governing astrocyte-neuron dynamic interactions. Of note, a significant portion of spines is in contact with shafts (35% compared to 55% contacting nodes), which could differ in terms of Ca2+ sources and handling from nodes.
The Genesis of Ca2+ Microdomains
Calcium transients at astrocyte soma and main processes have been studied by conventional bulk-loading with Ca2+-sensitive membrane-permeable chemical dyes. This approach is, however, inadequate for revealing Ca2+ changes at fine PAPs. The only available, though invasive, tool to study Ca2+ signaling at PAPs was the microinjection of chemical dyes into individual astrocytes through glass pipettes (Rungta et al., 2016). However, GECIs recently emerged as a powerful tool to study Ca2+ dynamics also in astrocytic microdomains (Shigetomi et al., 2013a; Kanemaru et al., 2014). The release of Ca2+ from the endoplasmic reticulum (ER) into the cytosol can be initiated by stimulation of metabotropic Gq G-protein-coupled receptors (GPCRs) through activation of PLC, production of IP3, and activation of IP3 receptors (IP3Rs) on ER membranes (Perea et al., 2009). Notably, Gi coupled GPCRs, such as GABAB receptors, can induce Ca2+ transients in astrocytes through an intracellular pathway that seems to converge also on IP3Rs signaling (Mariotti et al., 2016, 2018; Durkee et al., 2019; Caudal et al., 2020).
Historically, PAPs have been considered structures without organelles. However, through the use of serial block-face scanning EM, one or more subcellular organelles, including ER and mitochondria, were detected in about 40% of cortical PAPs (Aboufares El Alaoui et al., 2021). Consistent with the presence of ER in PAPs, deletion of the IP3R type 2 (IP3R2), which is known to be enriched in astrocytes, partially reduces the number or amplitude (∼60–70% of reduction) of spontaneous Ca2+ microdomains (Kanemaru et al., 2014; Srinivasan et al., 2015; Stobart et al., 2016; Agarwal et al., 2017, but see Rungta et al., 2016). In these mice, the level of impairment of Ca2+ microdomain responses to different GPCR agonists or electrical stimuli is, instead, variable among different studies (Kanemaru et al., 2014; Srinivasan et al., 2015; Stobart et al., 2016; Agarwal et al., 2017). However, the IP3R2 seems not to be the only astrocytic receptor mediating Ca2+ release from the ER. Okubo et al. recently used the ER luminal Ca2+ indicator G-CEPIA1er to visualize spontaneous and evoked ER Ca2+ release in astrocytes. While in fine processes of wild-type (WT) mice they observed spontaneous Ca2+ elevations with cytosolic GCaMP6f and spontaneous ER Ca2+ release with G-CEPIA1er, in IP3R2–/– mice they could detect spontaneous cytosolic Ca2+ elevations without decreases in ER Ca2+ levels. In contrast, in fine processes of IP3R2–/– mice, Gq receptor activation evoked a significant Ca2+ release from ER, although attenuated with respect to WT. Interestingly, this IP3R2-independent Ca2+ release induced low cytosolic Ca2+ elevations but robust Ca2+ transients in mitochondria (Okubo et al., 2019). Accordingly, in IP3R2–/– mice, IP3R1/3 and/or ryanodine receptors (Parpura et al., 2011; Sherwood et al., 2017) possibly account for residual IP3R2-independent Ca2+ release. Because evidence of ER cisternae is present in only ∼45% of PAPs, other ER-independent mechanisms must occur to allow Ca2+ dynamics in ER-free PAPs (Aboufares El Alaoui et al., 2021). For instance, Bergles’s laboratory has recently described that mitochondria are important players in astrocytic Ca2+ microdomains, in WT and IP3R2–/– mice. The authors demonstrated that Ca2+ microdomains can arise through Ca2+ efflux from mitochondria after transient openings of the mitochondrial permeability transition pore (mPTP). The opening of mPTP is facilitated by the production of reactive oxygen species owing to a rise in the metabolic demand after increased neuronal activity, suggesting a direct link between metabolic rate and Ca2+ microdomains (Agarwal et al., 2017). Although Ca2+ microdomains rely in part on Ca2+ release from intracellular stores, they may depend also on transmembrane Ca2+ influxes (Rungta et al., 2016). The substantial contribution of Ca2+ influxes from the extracellular space to spontaneous Ca2+ transients in PAPs has been established with Ca2+-free buffers (Srinivasan et al., 2015; Rungta et al., 2016; Gómez-Gonzalo et al., 2018).
Plasma membrane channels and transporters have been proposed to contribute to Ca2+ microdomain activity. Among these, the involvement of the TRPA1 channel has been described (Shigetomi et al., 2012, 2013b), though a clear role of this channel has not been conclusively established (Rungta et al., 2016). A promising candidate to directly link neuronal activity with Ca2+ microdomains is the Na+/Ca2+ exchanger (NCX). Na+-dependent neurotransmitter transporters localized in PAPs, such as the GABA transporter GAT3 (Minelli et al., 1996) or the glutamate transporter GLT-1 (Danbolt et al., 1992; Sakers et al., 2017), can generate Na+ microdomains (Felix et al., 2020) which in turn can trigger Ca2+ elevations in PAPs through the reverse function of the NCX, as recently described in the hippocampus after exogenous GABA applications (Boddum et al., 2016). However, the role of this mechanism in Ca2+ microdomains, both spontaneous and evoked, has been poorly investigated (Matos et al., 2018).
Although Ca2+ microdomains can have a pure intracellular or extracellular Ca2+ source, a cooperativity that finely shapes Ca2+ transients may occur among different sources. The release of Ca2+ from the ER typically triggers activation of store-operated Ca2+ entry (SOCE). During SOCE, depletion of ER Ca2+ stores activates the Ca2+ sensor stromal interaction molecules (STIMs) to translocate to the junctional ER-plasmatic membranes, where STIMs interact with and activate the store-operated Ca2+-release-activated Ca2+ channels (CRAC channels) formed by Orai proteins. Recently, Toth et al. (2019) observed that the frequency and amplitude of Ca2+ transients evoked by thrombin in PAPs from Orai1–/– mice were substantially attenuated, suggesting that Ca2+ microdomains rely on coordinated Ca2+ release from intracellular stores and transmembrane Ca2+ influxes from the extracellular space.
Even though knowledge is rapidly increasing (Figure 1), a complete picture of the Ca2+ microdomain essential features is lacking. Key questions remain unsolved. For example, which proteins are involved in Ca2+ influxes shaping microdomains? Astrocytes are mechanosensitive cells and the high motility of astrocyte processes (Bernardinelli et al., 2014) may expose them to physical forces that, in turn, may activate Ca2+-permeable mechanosensitive channels. Some plausible candidates that mediate mechanically induced Ca2+ microdomains have been described in astrocyte cultures and include TRPC1 (Reyes et al., 2013), TRPV4 (Turovsky et al., 2020), and Piezo1 (Velasco-Estevez et al., 2020) channels. Furthermore, are Ca2+ influxes modulated by activation of GPCRs? In addition to the IP3R-dependent signaling, GPCRs trigger different second messenger pathways, such as DAG-PKC and cAMP-PKA, that may regulate proteins mediating Ca2+ events.
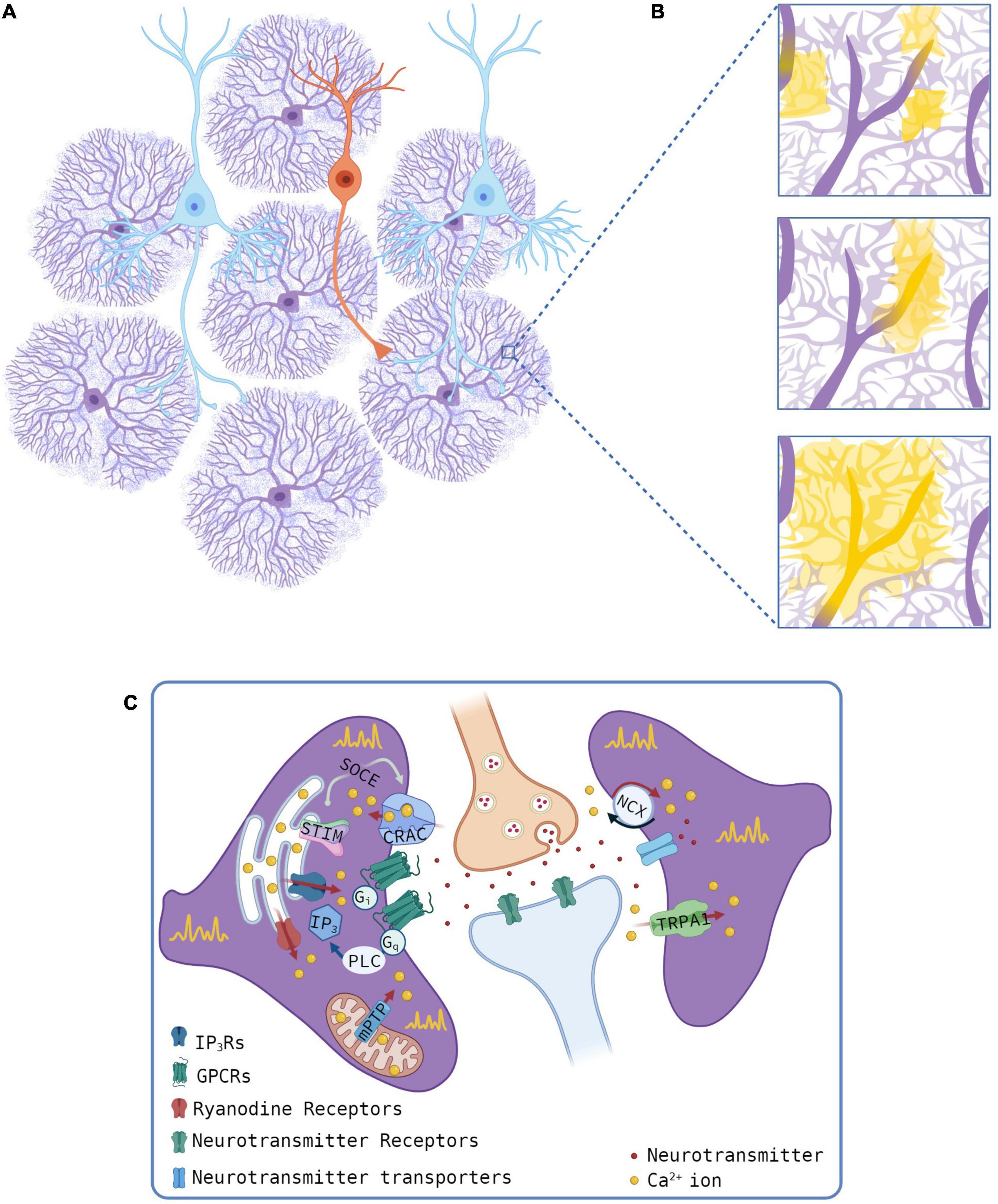
Figure 1. Sources of Ca2+ transients at microdomains. (A,B) Schematic of astrocyte-neuron network (A, pyramidal neurons, cyan, and interneuron, orange) and microdomain Ca2+ events (B). Yellow regions for different Ca2+ events, as observed with optical microscopy, and the presumed thin processes (light purple) as revealed by super-resolution imaging studies. (C) Mechanisms of astrocyte Ca2+ transients at microdomains showing intracellular (left) or extracellular (right) Ca2+ sources. Gliotransmitters are omitted. Created with QmlvUmVuZGVyLmNvbS4=
The intracellular signaling mechanism that links activation of GPCRs to an increase in Ca2+ microdomains in IP3R2–/– mice is also undefined. A partially unaltered neuronal function and the lack of somatic Ca2+ elevations in IP3R2–/– mice have been used to cast doubts about the role of astrocytic Ca2+ signaling in brain function (Fiacco and McCarthy, 2018). The observation of a richer Ca2+ activity at microdomains than at soma and the relative preservation of evoked Ca2+ microdomains in IP3R2–/– mice have turned attention to the relevance of astrocytic Ca2+ signaling in the modulation of neuronal function (Bazargani and Attwell, 2016; Savtchouk and Volterra, 2018). However, further evidence on the mechanisms of evoked Ca2+ microdomains in IP3R2–/– mice is necessary to unambiguously support a role for IP3-independent astrocytic Ca2+ signaling in neuronal function. The development of new tools to impair astrocytic Ca2+ signaling in an IP3R-independent manner (Yu et al., 2018) will help to better understand the molecular mechanism of Ca2+ microdomain generation.
The Analysis of Ca2+ Signals in Astrocytic Microdomains
Many experimental and analytical approaches have been developed to properly dissect the features of Ca2+ signals at the level of astrocytic microdomains. The measurement of Ca2+ fluctuations in astrocytic soma is relatively simple, owing to the easy identification of the somatic area. Analysis of Ca2+ signals in astrocytic microdomains, instead, is complex because their morphological features cannot be accurately defined under routinely used confocal or 2-photon microscopy techniques. For this reason, their identification has been handled based on the detection of Ca2+ changes rather than on morphological criteria. We can, indeed, gather information on microdomain Ca2+ signaling by imaging the fluorescent Ca2+ signal without, however, obtaining indications of fine process morphological details. The fluorophores of choice for this purpose have been the cytosolic or sub-membrane GECIs of the GCaMP family which revealed unexpectedly a high number of localized Ca2+ events throughout astrocyte distal territories (Shigetomi et al., 2013b) albeit without an estimate of the actual Ca2+ concentration. The increased complexity of Ca2+ signals revealed by GECIs, however, demanded a corresponding breakthrough in the analytical approach to properly evaluate the Ca2+ events. In this context, Khakh’s laboratory performed pioneering work in the decoding of astrocytic Ca2+ activity at the level of microdomains. Published in 2015, GECIquant represents the first software written to specifically analyze Ca2+ transients in GCaMP expressing astrocytes. Using a threshold-based criterion for signal amplitude, GECIquant obtains a semi-automated detection of the regions of interest (ROIs) containing Ca2+ fluctuations (Srinivasan et al., 2015). The software works at single cell level allowing us to distinguish between somatic Ca2+ fluctuations, waves involving principal processes, and sparkly signals at microdomains, providing as principal output, the raw fluorescence data along time from each region. Traces obtained through GECIquant can be further processed to obtain additional features, such as the amplitude and frequency of the Ca2+ events for each ROI, as performed in Mariotti et al. (2018), where a MATLAB script was used to identify significant peaks, based on additional amplitude thresholds along global and local baselines (Mariotti et al., 2018).
In 2017, CaSCaDe software was developed, overcoming the need for two scripts (Agarwal et al., 2017). CaSCaDe, like GECIquant, performs a semi-automated analysis, in this case using a machine-learning-based algorithm to identify Ca2+ microdomains. Time-series images are processed to remove background noise and binarized using an amplitude threshold. Microdomains are selected based on additional criteria of amplitude and duration. Besides producing a spatial map of active regions, the software provides information about the number, frequency, amplitude, and time course of events.
Weber’s laboratory worked to develop a processing suite aimed at simplifying the analysis workflows. This is the main purpose of CHIPS, a MATLAB based open-source toolbox that gathers different algorithms and provides the user with options for a number of analysis types. CHIPS offers helpful pre-processing functions, such as motion correction and denoising. Different algorithms can then be applied to analyze data and plot the resulting outputs (Barrett et al., 2018).
All the analytical procedures described so far rely on the concept of ROIs applied to microdomains, defined by spatial coordinates and measurable along different time series. However, constraining microdomains in defined boundaries may result in signal detection inaccuracy. Regions involved in Ca2+ variations may become larger than the defined ROI, leading the signal to invade other territories, finally resulting in signal fragmentation among adjacent ROIs. Conversely, larger ROIs may fail to faithfully capture the signal amplitude if Ca2+ increases only in a portion of the ROI.
The turning point in this concern was represented by AQuA software (Wang et al., 2019), which reverses the logic at the basis of ROI detection by adopting an event-based analytical approach, as already suggested in Wu et al. (2014). Calcium events are thus captured in their dynamic changes along space and time, revealing signals that can propagate and modify their size or shape, expressing different tracts of the same, composite fingerprint.
AQuA software does not include pre-processing functions but offers suggestions about pre-processing steps to be considered. A valuable feature is the possibility to choose between different data type pre-sets, depending on the Ca2+ indicator used and on the acquisition signal to noise ratio, with optimized but user-adjustable parameters. AQuA can work also on a single cell level and isolate events in astrocytic processes, but without distinction between principal branches and thin processes. The identification of events is achieved through different image processing steps, including thresholding and smoothing, and the application of a number of algorithms that aim to define the temporal limits and spatial propagation features of individual events. The user proceeds step-by-step and can inspect traces and spatial boundaries of the events along time, as training which is recommended to achieve the best parameters to apply to the specific experimental datasets. AQuA outputs a broad and rich collection of features for detected events, such as area, amplitude, duration, frequency, and propagation features, revealing itself as a valuable tool for astrocytic Ca2+ analysis.
While the approaches described so far refer to 2D imaging, it is worth mentioning that a study from Volterra’s group focused on the analysis of astrocytic Ca2+ activity recorded through 3D imaging. This approach captures the entire extension of individual astrocytes, adding a significant step of complexity to the comprehension of Ca2+ signal dynamics (Bindocci et al., 2017).
All in all, the development of different analytical approaches confirms the important role acquired by astrocytes in the studies of brain function. In the near future, analytical and experimental advances will enable us to unveil new types of modulation exerted by astrocytes.
Discussion
Over the last decade, the combined use of innovative tools has provided compelling evidence that astrocyte dynamic interactions with neurons have fundamental roles in brain function. The different players involved in this interplay are beginning to be elucidated, particularly regarding Ca2+ activities at microdomains. In this restricted and elusive subcellular region, an unexpected complexity has emerged. Here, the stage of Ca2+ activity has been populated by new actors but many questions remain unanswered. For example, how these players interact? How Ca2+ events propagate from fine to major processes? What is the role of spontaneous microdomain Ca2+ activity in brain functions? The use of different types of preparations, Ca2+ detection techniques, and analytical tools in different brain regions has provided invaluable results that, however, cannot always be easily compared. New shared techniques and analytical tools are thus needed. Elucidating all the mechanisms of neuron-astrocyte interplay at tripartite synapses is crucial to understanding brain functions and dysfunctions (Pekny et al., 2016), and will possibly unveil new therapeutic targets. We believe that a new decade of great discoveries has just begun.
Author Contributions
AL wrote the introduction with GL and the chapter on the Analysis of Ca2+ events with MZ. VH created the figure. AC wrote the chapter on the locus of Ca2+ with GL. MZ wrote chapter on the Analysis of Ca2+ events with AL. GC contributed to the manuscript. MG-G wrote the chapter on the genesis of Ca2+ events. GL wrote the abstract, introduction, the chapter on the site of Ca2+ events with AC, and the discussion with the help of all other authors. All authors contributed to the article and approved the submitted version.
Funding
The work was supported by the Marie Skłodowska-Curie ITN, EU-Glia Ph.D. to GC and VH, Cariparo Foundation Excellence 2018 to GC, PRIN 2018 to GC.
Conflict of Interest
The authors declare that the research was conducted in the absence of any commercial or financial relationships that could be construed as a potential conflict of interest.
Acknowledgments
We thank Gian Michele Ratto for helpful discussion on analytical approaches to Ca2+ transients in astrocyte microdomains.
References
Aboufares El Alaoui, A., Jackson, M., Fabri, M., de Vivo, L., and Bellesi, M. (2021). Characterization of subcellular organelles in cortical perisynaptic astrocytes. Front. Cell. Neurosci. 14:573944. doi: 10.3389/fncel.2020.573944
Agarwal, A., Wu, P. H., Hughes, E. G., Fukaya, M., Tischfield, M. A., Langseth, A. J., et al. (2017). Transient opening of the mitochondrial permeability transition pore induces microdomain calcium transients in astrocyte processes. Neuron 93, 587.e–605.e. doi: 10.1016/j.neuron.2016.12.034
Araque, A., Carmignoto, G., Haydon, P. G., Oliet, S. H. R., Robitaille, R., and Volterra, A. (2014). Gliotransmitters travel in time and space. Neuron 81, 728–739. doi: 10.1016/j.neuron.2014.02.007
Arizono, M., Inavalli, V. V. G. K., Panatier, A., Pfeiffer, T., Angibaud, J., Levet, F., et al. (2020). Structural basis of astrocytic Ca2+ signals at tripartite synapses. Nat. Commun. 11:1906. doi: 10.1038/s41467-020-15648-4
Barrett, M. J. P., Ferrari, K. D., Stobart, J. L., Holub, M., and Weber, B. (2018). CHIPS: an extensible toolbox for cellular and hemodynamic two-photon image analysis. Neuroinformatics 16, 145–147. doi: 10.1007/s12021-017-9344-y
Bazargani, N., and Attwell, D. (2016). Astrocyte calcium signaling: the third wave. Nat. Neurosci. 19, 182–189. doi: 10.1038/nn.4201
Bernardinelli, Y., Muller, D., and Nikonenko, I. (2014). Astrocyte-synapse structural plasticity. Neural Plast. 2014:232105. doi: 10.1155/2014/232105
Bezzi, P., and Volterra, A. (2001). A neuron–glia signalling network in the active brain. Curr. Opin. Neurobiol. 11, 387–394. doi: 10.1016/S0959-4388(00)00223-3
Bindocci, E., Savtchouk, I., Liaudet, N., Becker, D., Carriero, G., and Volterra, A. (2017). Three-dimensional Ca2+ imaging advances understanding of astrocyte biology. Science 356:eaai8185. doi: 10.1126/science.aai8185
Boddum, K., Jensen, T. P., Magloire, V., Kristiansen, U., Rusakov, D. A., Pavlov, I., et al. (2016). Astrocytic GABA transporter activity modulates excitatory neurotransmission. Nat. Commun. 7:13572. doi: 10.1038/ncomms13572
Bushong, E. A., Martone, M. E., Jones, Y. Z., and Ellisman, M. H. (2002). Protoplasmic astrocytes in CA1 stratum radiatum occupy separate anatomical domains. J. Neurosci. 22, 183–192. doi: 10.1523/jneurosci.22-01-00183.2002
Calì, C., Agus, M., Kare, K., Boges, D. J., Lehväslaiho, H., Hadwiger, M., et al. (2019). 3D cellular reconstruction of cortical glia and parenchymal morphometric analysis from serial block-face electron microscopy of juvenile rat. Prog. Neurobiol. 183:101696. doi: 10.1016/j.pneurobio.2019.101696
Caudal, L. C., Gobbo, D., Scheller, A., and Kirchhoff, F. (2020). The paradox of astroglial Ca2 + signals at the interface of excitation and inhibition. Front. Cell. Neurosci. 14:609947. doi: 10.3389/fncel.2020.609947
Cornell-Bell, A. H., Finkbeiner, S. M., Cooper, M. S., and Smith, S. J. (1990). Glutamate induces calcium waves in cultured astrocytes: long-range glial signaling. Science 247, 470–473. doi: 10.1126/science.1967852
Danbolt, N. C., Storm-Mathisen, J., and Kanner, B. I. (1992). An [Na+ + K+]coupledl-glutamate transporter purified from rat brain is located in glial cell processes. Neuroscience 51, 295–310. doi: 10.1016/0306-4522(92)90316-T
Di Castro, M. A., Chuquet, J., Liaudet, N., Bhaukaurally, K., Santello, M., Bouvier, D., et al. (2011). Local Ca2+ detection and modulation of synaptic release by astrocytes. Nat. Neurosci. 14, 1276–1284. doi: 10.1038/nn.2929
Durkee, C. A., Covelo, A., Lines, J., Kofuji, P., Aguilar, J., and Araque, A. (2019). G i/o protein-coupled receptors inhibit neurons but activate astrocytes and stimulate gliotransmission. Glia 67, 1076–1093. doi: 10.1002/glia.23589
Felix, L., Delekate, A., Petzold, G. C., and Rose, C. R. (2020). Sodium fluctuations in astroglia and their potential impact on astrocyte function. Front. Physiol. 11:871. doi: 10.3389/fphys.2020.00871
Fiacco, T. A., and McCarthy, K. D. (2018). Multiple lines of evidence indicate that gliotransmission does not occur under physiological conditions. J. Neurosci. 38, 3–13. doi: 10.1523/JNEUROSCI.0016-17.2017
Gavrilov, N., Golyagina, I., Brazhe, A., Scimemi, A., Turlapov, V., and Semyanov, A. (2018). Astrocytic coverage of dendritic spines, dendritic shafts, and axonal boutons in hippocampal neuropil. Front. Cell. Neurosci. 12:248. doi: 10.3389/fncel.2018.00248
Gómez-Gonzalo, M., Navarrete, M., Perea, G., Covelo, A., Martín-Fernández, M., Shigemoto, R., et al. (2015). Endocannabinoids induce lateral long-term potentiation of transmitter release by stimulation of gliotransmission. Cereb. Cortex 25, 3699–3712. doi: 10.1093/cercor/bhu231
Gómez-Gonzalo, M., Zehnder, T., Requie, L. M., Bezzi, P., and Carmignoto, G. (2018). Insights into the release mechanism of astrocytic glutamate evoking in neurons NMDA receptor-mediated slow depolarizing inward currents. Glia 66, 2188–2199. doi: 10.1002/glia.23473
Grosche, J., Matyash, V., Möller, T., Verkhratsky, A., Reichenbach, A., and Kettenmann, H. (1999). Microdomains for neuron-glia interaction: parallel fiber signaling to Bergmann glial cells. Nat. Neurosci. 2, 139–143. doi: 10.1038/5692
Heller, J. P., and Rusakov, D. A. (2017). The nanoworld of the tripartite synapse: insights from super-resolution microscopy. Front. Cell. Neurosci. 11:374. doi: 10.3389/fncel.2017.00374
Henneberger, C., Papouin, T., Oliet, S. H. R., and Rusakov, D. A. (2010). Long-term potentiation depends on release of d-serine from astrocytes. Nature 463, 232–236. doi: 10.1038/nature08673
Kanemaru, K., Sekiya, H., Xu, M., Satoh, K., Kitajima, N., Yoshida, K., et al. (2014). In Vivo visualization of subtle, transient, and local activity of astrocytes using an ultrasensitive Ca2+ indicator. Cell Rep. 8, 311–318. doi: 10.1016/j.celrep.2014.05.056
Kofuji, P., and Araque, A. (2021). Astrocytes and behavior. Annu. Rev. Neurosci. 44, 49–67. doi: 10.1146/annurev-neuro-101920-112225
Mariotti, L., Losi, G., Lia, A., Melone, M., Chiavegato, A., Gómez-Gonzalo, M., et al. (2018). Interneuron-specific signaling evokes distinctive somatostatin-mediated responses in adult cortical astrocytes. Nat. Commun. 9:82. doi: 10.1038/s41467-017-02642-6
Mariotti, L., Losi, G., Sessolo, M., Marcon, I., and Carmignoto, G. (2016). The inhibitory neurotransmitter GABA evokes long-lasting Ca2+ oscillations in cortical astrocytes. Glia 64, 363–373. doi: 10.1002/glia.22933
Matos, M., Bosson, A., Riebe, I., Reynell, C., Vallée, J., Laplante, I., et al. (2018). Astrocytes detect and upregulate transmission at inhibitory synapses of somatostatin interneurons onto pyramidal cells. Nat. Commun. 9:4254. doi: 10.1038/s41467-018-06731-y
Minelli, A., DeBiasi, S., Brecha, N. C., Zuccarello, L. V., and Conti, F. (1996). GAT-3, a high-affinity GABA plasma membrane transporter, is localized to astrocytic processes, and it is not confined to the vicinity of GABAergic synapses in the cerebral cortex. J. Neurosci. 16, 6255–6264. doi: 10.1523/jneurosci.16-19-06255.1996
Nagai, J., Yu, X., Papouin, T., Cheong, E., Freeman, M. R., Monk, K. R., et al. (2021). Behaviorally consequential astrocytic regulation of neural circuits. Neuron 109, 576–596. doi: 10.1016/j.neuron.2020.12.008
Okubo, Y., Kanemaru, K., Suzuki, J., Kobayashi, K., Hirose, K., and Iino, M. (2019). Inositol 1,4,5-trisphosphate receptor type 2-independent Ca 2+ release from the endoplasmic reticulum in astrocytes. Glia 67, 113–124. doi: 10.1002/glia.23531
Panatier, A., Vallée, J., Haber, M., Murai, K. K., Lacaille, J. C., and Robitaille, R. (2011). Astrocytes are endogenous regulators of basal transmission at central synapses. Cell 146, 785–798. doi: 10.1016/j.cell.2011.07.022
Papouin, T., Dunphy, J., Tolman, M., Foley, J. C., and Haydon, P. G. (2017). Astrocytic control of synaptic function. Philos. Trans. R. Soc. B Biol. Sci. 372:20160154. doi: 10.1098/rstb.2016.0154
Parpura, V., Basarsky, T. A., Liu, F., Jeftinija, K., Jeftinija, S., and Haydon, P. G. (1994). Glutamate-mediated astrocyte–neuron signalling. Nature 369, 744–747. doi: 10.1038/369744a0
Parpura, V., Grubišic, V., and Verkhratsky, A. (2011). Ca2+ sources for the exocytotic release of glutamate from astrocytes. Biochim. Biophys. Acta Mol. Cell Res. 1813, 984–991. doi: 10.1016/j.bbamcr.2010.11.006
Pasti, L., Volterra, A., Pozzan, T., and Carmignoto, G. (1997). Intracellular calcium oscillations in astrocytes: a highly plastic, bidirectional form of communication between neurons and astrocytes in situ. J. Neurosci. 17, 7817–7830. doi: 10.1523/jneurosci.17-20-07817.1997
Pekny, M., Pekna, M., Messing, A., Steinhäuser, C., Lee, J.-M., Parpura, V., et al. (2016). Astrocytes: a central element in neurological diseases. Acta Neuropathol. 131, 323–345. doi: 10.1007/s00401-015-1513-1
Perea, G., Navarrete, M., and Araque, A. (2009). Tripartite synapses: astrocytes process and control synaptic information. Trends Neurosci. 32, 421–431.
Reyes, R. C., Verkhratsky, A., and Parpura, V. (2013). TRPC1-mediated Ca2+ and Na+ signalling in astroglia: differential filtering of extracellular cations. Cell Calcium 54, 120–125. doi: 10.1016/j.ceca.2013.05.005
Robin, L. M., Oliveira da Cruz, J. F., Langlais, V. C., Martin-Fernandez, M., Metna-Laurent, M., Busquets-Garcia, A., et al. (2018). Astroglial CB1 receptors determine synaptic D-serine availability to enable recognition memory. Neuron 98, 935.e–944.e. doi: 10.1016/j.neuron.2018.04.034
Rungta, R. L., Bernier, L. P., Dissing-Olesen, L., Groten, C. J., LeDue, J. M., Ko, R., et al. (2016). Ca2+ transients in astrocyte fine processes occur via Ca2+ influx in the adult mouse hippocampus. Glia 64, 2093–2103. doi: 10.1002/glia.23042
Rusakov, D. A. (2015). Disentangling calcium-driven astrocyte physiology. Nat. Rev. Neurosci. 16, 226–233. doi: 10.1038/nrn3878
Sakers, K., Lake, A. M., Khazanchi, R., Ouwenga, R., Vasek, M. J., Dani, A., et al. (2017). Astrocytes locally translate transcripts in their peripheral processes. Proc. Natl. Acad. Sci. U.S.A. 114, E3830–E3838. doi: 10.1073/pnas.1617782114
Santello, M., Toni, N., and Volterra, A. (2019). Astrocyte function from information processing to cognition and cognitive impairment. Nat. Neurosci. 22, 154–166. doi: 10.1038/s41593-018-0325-8
Savtchouk, I., and Volterra, A. (2018). Gliotransmission: beyond black-and-white. J. Neurosci. 38, 14–25. doi: 10.1523/JNEUROSCI.0017-17.2017
Semyanov, A., Henneberger, C., and Agarwal, A. (2020). Making sense of astrocytic calcium signals from acquisition to interpretation. Nat. Rev. Neurosci. 21, 551–564. doi: 10.1038/s41583-020-0361-8
Sherwood, M. W., Arizono, M., Hisatsune, C., Bannai, H., Ebisui, E., Sherwood, J. L., et al. (2017). Astrocytic IP3Rs: contribution to Ca2+ signalling and hippocampal LTP. Glia 65, 502–513. doi: 10.1002/glia.23107
Shigetomi, E., Bushong, E. A., Haustein, M. D., Tong, X., Jackson-Weaver, O., Kracun, S., et al. (2013a). Imaging calcium microdomains within entire astrocyte territories and endfeet with GCaMPs expressed using adeno-associated viruses. J. Gen. Physiol. 141, 633–647. doi: 10.1085/jgp.201210949
Shigetomi, E., Jackson-Weaver, O., Huckstepp, R. T., O’Dell, T. J., and Khakh, B. S. (2013b). TRPA1 channels are regulators of astrocyte basal calcium levels and long-term potentiation via constitutive d-serine release. J. Neurosci. 33, 10143–10153. doi: 10.1523/JNEUROSCI.5779-12.2013
Shigetomi, E., Tong, X., Kwan, K. Y., Corey, D. P., and Khakh, B. S. (2012). TRPA1 channels regulate astrocyte resting calcium and inhibitory synapse efficacy through GAT-3. Nat. Neurosci. 15, 70–80. doi: 10.1038/nn.3000
Srinivasan, R., Huang, B. S., Venugopal, S., Johnston, A. D., Chai, H., Zeng, H., et al. (2015). Ca2+ signaling in astrocytes from Ip3r2 -/- mice in brain slices and during startle responses in vivo. Nat. Neurosci. 18, 708–717. doi: 10.1038/nn.4001
Stobart, J. L., Ferrari, K. D., Barrett, M. J. P., Glück, C., Stobart, M. J., Zuend, M., et al. (2018). Cortical circuit activity evokes rapid astrocyte calcium signals on a similar timescale to neurons. Neuron 98, 726.e–735.e. doi: 10.1016/j.neuron.2018.03.050
Stobart, J. L., Ferrari, K. D., Barrett, M. J. P., Stobart, M. J., Looser, Z. J., Saab, A. S., et al. (2016). Long-term in vivo calcium imaging of astrocytes reveals distinct cellular compartment responses to sensory stimulation. Cereb. Cortex 28, 184–198. doi: 10.1093/cercor/bhw366
Toth, A. B., Hori, K., Novakovic, M. M., Bernstein, N. G., Lambot, L., and Prakriya, M. (2019). CRAC channels regulate astrocyte Ca2+ signaling and gliotransmitter release to modulate hippocampal GABAergic transmission. Sci. Signal. 12:eaaw5450. doi: 10.1126/scisignal.aaw5450
Turovsky, E. A., Braga, A., Yu, Y., Esteras, N., Korsak, A., Theparambil, S. M., et al. (2020). Mechanosensory signaling in astrocytes. J. Neurosci. 40, 9364–9371. doi: 10.1523/JNEUROSCI.1249-20.2020
Velasco-Estevez, M., Rolle, S. O., Mampay, M., Dev, K. K., and Sheridan, G. K. (2020). Piezo1 regulates calcium oscillations and cytokine release from astrocytes. Glia 68, 145–160. doi: 10.1002/glia.23709
Ventura, R., and Harris, K. M. (1999). Three-dimensional relationships between hippocampal synapses and astrocytes. J. Neurosci. 19, 6897–6906. doi: 10.1523/jneurosci.19-16-06897.1999
Vignoli, B., Battistini, G., Melani, R., Blum, R., Santi, S., Berardi, N., et al. (2016). Peri-synaptic glia recycles brain-derived neurotrophic factor for LTP stabilization and memory retention. Neuron 92, 873–887. doi: 10.1016/j.neuron.2016.09.031
Volterra, A., Liaudet, N., and Savtchouk, I. (2014). Astrocyte Ca2+ signalling: an unexpected complexity. Nat. Rev. Neurosci. 15, 327–335. doi: 10.1038/nrn3725
Wang, Y., DelRosso, N. V., Vaidyanathan, T. V., Cahill, M. K., Reitman, M. E., Pittolo, S., et al. (2019). Accurate quantification of astrocyte and neurotransmitter fluorescence dynamics for single-cell and population-level physiology. Nat. Neurosci. 22, 1936–1944. doi: 10.1038/s41593-019-0492-2
Wu, Y., Tang, X., Arizono, M., Bannai, H., Shih, P., Dembitskaya, Y., et al. (2014). Cell calcium spatiotemporal calcium dynamics in single astrocytes and its modulation by neuronal activity. Cell Calcium 55, 119–129. doi: 10.1016/j.ceca.2013.12.006
Keywords: astrocytes, calcium, tripartite synapses, microdomains, gliotransmission
Citation: Lia A, Henriques VJ, Zonta M, Chiavegato A, Carmignoto G, Gómez-Gonzalo M and Losi G (2021) Calcium Signals in Astrocyte Microdomains, a Decade of Great Advances. Front. Cell. Neurosci. 15:673433. doi: 10.3389/fncel.2021.673433
Received: 27 February 2021; Accepted: 23 April 2021;
Published: 07 June 2021.
Edited by:
Christian Lohr, University of Hamburg, GermanyReviewed by:
Christian Henneberger, University of Bonn, GermanyValentin Nägerl, UMR 5297 Institut Interdisciplinaire de Neurosciences (IINS), France
Copyright © 2021 Lia, Henriques, Zonta, Chiavegato, Carmignoto, Gómez-Gonzalo and Losi. This is an open-access article distributed under the terms of the Creative Commons Attribution License (CC BY). The use, distribution or reproduction in other forums is permitted, provided the original author(s) and the copyright owner(s) are credited and that the original publication in this journal is cited, in accordance with accepted academic practice. No use, distribution or reproduction is permitted which does not comply with these terms.
*Correspondence: Marta Gómez-Gonzalo, bWFydGEuZ29tZXpnb256YWxvQGNuci5pdA==; Gabriele Losi, Z2FicmllbGUubG9zaUBiaW8udW5pcGQuaXQ=
†These authors have contributed equally to this work