- Department of Neonatology, Charité—Universitätsmedizin Berlin, Berlin, Germany
The neurotransmitter GABA and its receptors assume essential functions during fetal and postnatal brain development. The last trimester of a human pregnancy and early postnatal life involves a vulnerable period of brain development. In the second half of gestation, there is a developmental shift from depolarizing to hyperpolarizing in the GABAergic system, which might be disturbed by preterm birth. Alterations of the postnatal GABA shift are associated with several neurodevelopmental disorders. In this in vivo study, we investigated neurogenesis in the dentate gyrus (DG) in response to daily administration of pharmacological GABAA (DMCM) and GABAB (CGP 35348) receptor inhibitors to newborn rats. Six-day-old Wistar rats (P6) were daily injected (i.p.) to postnatal day 11 (P11) with DMCM, CGP 35348, or vehicle to determine the effects of both antagonists on postnatal neurogenesis. Due to GABAB receptor blockade by CGP 35348, immunohistochemistry revealed a decrease in the number of NeuroD1 positive intermediate progenitor cells and a reduction of proliferative Nestin-positive neuronal stem cells at the DG. The impairment of hippocampal neurogenesis at this stage of differentiation is in line with a significantly decreased RNA expression of the transcription factors Pax6, Ascl1, and NeuroD1. Interestingly, the number of NeuN-positive postmitotic neurons was not affected by GABAB receptor blockade, although strictly associated transcription factors for postmitotic neurons, Tbr1, Prox1, and NeuroD2, displayed reduced expression levels, suggesting impairment by GABAB receptor antagonization at this stage of neurogenesis. Antagonization of GABAB receptors decreased the expression of neurotrophins (BDNF, NT-3, and NGF). In contrast to the GABAB receptor blockade, the GABAA receptor antagonization revealed no significant changes in cell counts, but an increased transcriptional expression of Tbr1 and Tbr2. We conclude that GABAergic signaling via the metabotropic GABAB receptor is crucial for hippocampal neurogenesis at the time of rapid brain growth and of the postnatal GABA shift. Differentiation and proliferation of intermediate progenitor cells are dependent on GABA. These insights become more pertinent in preterm infants whose developing brains are prematurely exposed to spostnatal stress and predisposed to poor neurodevelopmental disorders, possibly as sequelae of early disruption in GABAergic signaling.
Introduction
Very preterm-born children may suffer from significant deficits in executive function, processing speed, and intelligence (Brydges et al., 2018). The risk for neurodevelopmental disorders such as attention-deficit/hyperactivity disorder (ADHD), autism spectrum disorder (ASD), and anxiety are increased two to four times in preterm children, as compared to term-born controls (Rogers et al., 2018). Altered GABAergic signaling has been implicated in the pathogenesis of ASD (Gaetz et al., 2014; Tanifuji et al., 2017), ADHD (Naaijen et al., 2017), as well as anxiety (Nuss, 2015). Pharmacological antagonization and agonization of γ-aminobutyric acid (GABA) receptors in the neonatal animal model of the mouse during postnatal brain development corroborate an important role of GABA and their receptors in programming neurobehavioral phenotypes in adulthood (Salari and Amani, 2017).
While human brain growth reaches its highest velocity at birth (Watson et al., 2006), the so-called “brain growth spurts” in rodents peak at the seventh postnatal day (Semple et al., 2013). Postnatal rat pups may therefore serve as a model for the human stage of brain development, corresponding to the last trimester of pregnancy. Both in humans and rodents, the hippocampus undergoes developmental changes close to birth (Semple et al., 2013). During the formation of the dentate gyrus (DG) neural progenitor cells (NPC) are generated and form a proliferative zone that remains active during postnatal stages, becoming the site of adult hippocampal neurogenesis called the subgranular zone (SGZ; Paridaen and Huttner, 2014). Supplemented new neurons form highly complex neural circuits, supporting a role for hippocampal neurogenesis in memory, learning, and behavior (Deng et al., 2010; Anacker and Hen, 2017).
The last trimester of a human pregnancy and early postnatal life involves a period of brain development with neuronal organization and maturation, such as neurogenesis, migration, dendritogenesis, synaptogenesis, and plasticity of developing neurons. These processes are regulated by neurotransmitters such as GABA and glutamate, which remain at risk of disruption after preterm birth (Malik et al., 2013). In humans, the phase of rapid brain growth starts at gestational week 28, and peaks at the time of birth. In the rat model, this phase occurs from postnatal day (P)4 to P11 and peaks at P7 (Dobbing and Sands, 1979; Semple et al., 2013) which makes early postnatal pups useful as model organisms in studies of human neuronal development, corresponding roughly to the last trimester of pregnancy. These neurodevelopmental processes are highly vulnerable and clinically relevant, as they can be affected by oxidative stress (hyperoxia, hypoxia) or various necessary medical interventions (Malik et al., 2013; Steinhorn et al., 2015; Duerden et al., 2016; Isokawa, 2016). In addition to neurotransmission and developmentally mediated excitatory-inhibitory transition of GABA action during the perinatal period (Ben-Ari, 2018), GABA and its receptors assume multiple essential functions during fetal and postnatal brain development (Cellot and Cherubini, 2013; Wu and Sun, 2015; Tang et al., 2021). In the rat, the main generation of hippocampal granular cells starts around birth and peaks during the first postnatal week (Altman and Bayer, 1990). In line with this, GABAergic transmission changes from excitatory to inhibitory also during the end of the first postnatal week (Rivera et al., 1999; Khirug et al., 2005). Neurobehavioral disorders, including autism, are more common in survivors of preterm birth and have been associated with decreased GABA concentrations, underscoring the importance of in vivo examination of GABA changes during early postnatal life in preterm infants (Ream and Lehwald, 2018; Basu et al., 2021). Peerboom and Wierenga (2021) postulated that the postnatal shift from depolarizing to hyperpolarizing GABA is a pivotal event in brain development and its timing affects brain function throughout life. Altered timing of the postnatal GABA shift is associated with several neurodevelopmental disorders (Schulte et al., 2018). In addition, preterm birth itself, as well as pharmacologic drugs used in the preterm infant, influence GABA receptor associated pathways (Shaw et al., 2015; Steinhorn et al., 2015). Extremely and very preterm infants showed reduced GABA concentrations in the brain measured by magnetic resonance imaging (Kwon et al., 2014; Basu et al., 2020). The developmental timeline of the GABAergic system becomes more relevant in preterm infants whose developing brains are prematurely exposed to extra uterine stress, and predisposed to neurological disorders, perhaps in part as sequela of early derangement in GABAergic systems.
Despite the postnatal developmental differences in GABAergic signaling, there are many similarities in the generalized course of neural maturation in early development and adulthood (Song et al., 2012). A complex interaction of the intrinsic programs of neuronal stem cells (NSCs) and their progressively produced progenitors (NPC) regulates neurogenesis, which is orchestrated by intrinsic pathways and extracellular signaling molecules (Faigle and Song, 2013; Bjornsson et al., 2015). As shown schematically in Figure 5, hippocampal neurogenesis originates from NPC and leads to granule cell neurons, which go through different stages leads to granule cell neurons after progressing through the stages of NSC/type-1 cells, NPC/type-2a cells, neuroblast/type-2b cells, immature-mitotic neuron/type-3 cells as well as postmitotic-immature and mature granular neurons (Kempermann et al., 2004). NSCs are the shared NPC of both neurons and astrocytes and therefore express the astrocytic markers glial fibrillary acidic protein (GFAP) and Scl1a3 as well as the neuronal marker nestin (DeCarolis et al., 2013; Berg et al., 2018; Vieira et al., 2018). NSCs express Sox2 to maintain their multipotency and proliferation capacity (Mercurio et al., 2019). Sox2 represses the expression of NeuroD1 and therefore prevents the cells’ progression in neurogenesis, preserving their self-renewal capacity (Kuwabara et al., 2009). A key regulator of the mainstay of NSCs after their transition to asymmetric neurogenic division is the Notch target gene Hes5 (Lugert et al., 2010). By directly changing the expression of genes associated with self-renewal and differentiation [e.g., Sox2 (Wen et al., 2008), Ngn2, and NeuroD1 (Scardigli et al., 2003; Shimojo et al., 2008)], Pax6 is essential to regulating the NPCs’ proliferation (Maekawa et al., 2005). NSCs give rise to intermediate progenitor cells, which express as a specific marker Tbr2. Tbr2 labels type-2 cells, while Tbr1 is expressed by immature granule neurons (Englund et al., 2005; Hodge et al., 2008; Nicola et al., 2015). Additionally, type-2a cells express the proneural markers Ascl1 (also known as Mash1) and Ngn2 (Amador-Arjona et al., 2015; Pérez-Domínguez et al., 2018). At the late stage of typ-2a cells, Ngn2 is downregulated, whilst Tbr2 expression in typ-2b cells persists (Roybon et al., 2009). Type-2b cells start to express NeuroD1, a crucial transcription factor for neurogenesis during hippocampal development that marks the transition from amplifying progenitor to neuroblast (Kuwabara et al., 2009). NeuroD1 is necessary for further survival and maturation of neurons (Pérez-Domínguez et al., 2018). Type-3 cells are less proliferative and may migrate and exit the cell cycle before full maturation into granule neurons (Nicola et al., 2015). NeuroD2 starts to be expressed just after NeuroD1 and continues to be highly expressed in postmitotic mature neurons (Roybon et al., 2009). Postmitotic neurons following further differentiation express as specific markers NeuN, Tbr1 (Englund et al., 2005) and NeuroD2, of which the latter is necessary for cell cycle regulation and survival of neurons (Olson et al., 2001; Wilke et al., 2012). Granule cell maturation necessarily depends on Prox1 expression, which starts with type-2b cells and is maintained further in differentiation (Lavado et al., 2010). Different types of signals, including glutamatergic and GABAergic signals from local neural networks, mediate these complex neurogenic processes.
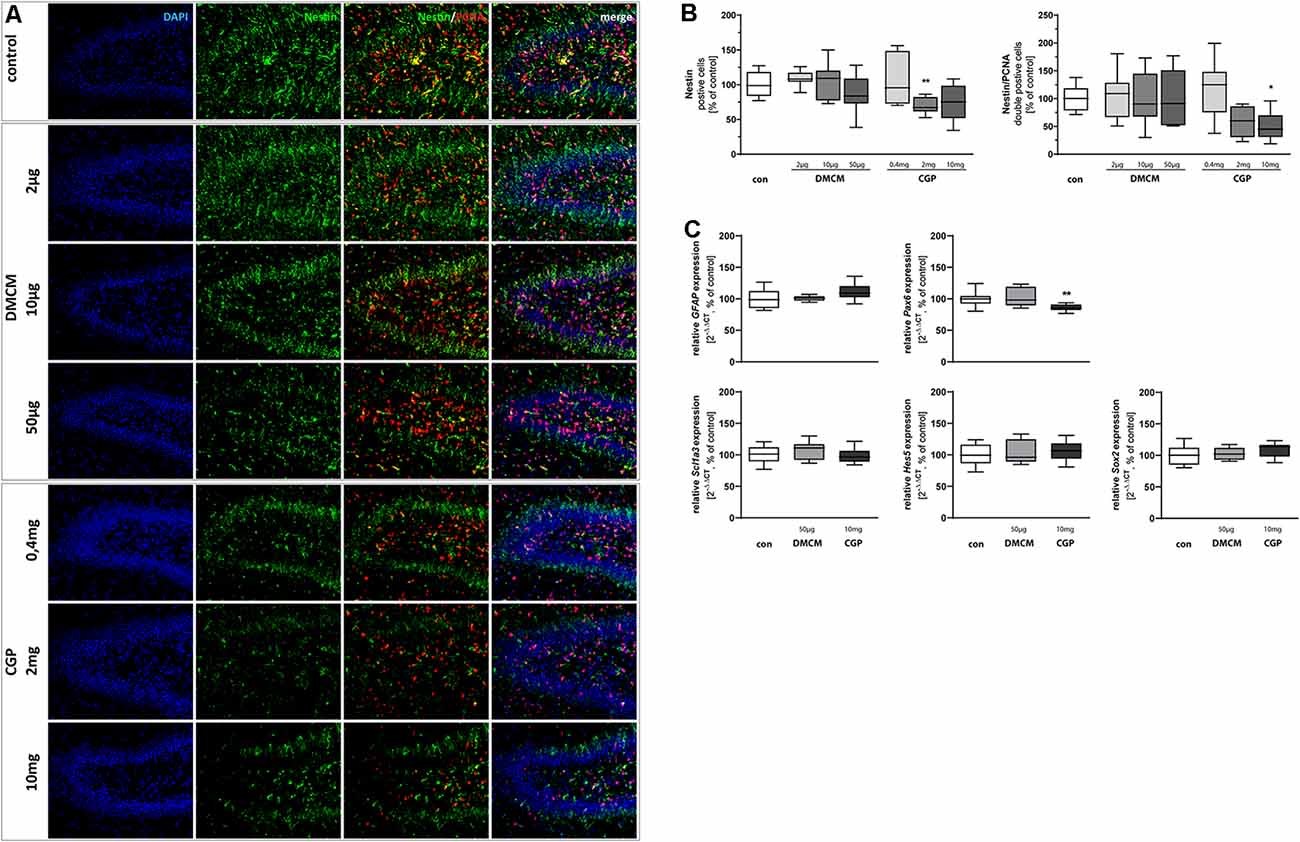
Figure 1. Representative hippocampal paraffin sections (A) of control animals, DMCM hydrochloride (DMCM) in doses of either 2 μg/kg, 10 μg/kg or 50 μg/kg, and CGP 35348 in doses of either 0.4 mg/kg, 2 mg/kg or 10 mg/kg treated rat pups at P11 co-labeled with DAPI, Nestin, and PCNA. Application of GABAB receptor antagonist CGP 2 mg/kg decreased Nestin positive progenitor cells in the dentate gyrus (DG). Application of CGP 10 mg/kg decreased the number of proliferating Nestin/PCNA double positive cells. Quantification of (B) Nestin and Nestin/PCNA double positive cells in sum of the DG in comparison to control group (100% white bars). Data are expressed relative to the control group as mean ± SEM of n = 10 each group. The 100% values are for Nestin+ 83.5 cell counts and for Nestin+PCNA+ 13.3 cell counts. *p < 0.05 and **p < 0.01 vs. control (Brown-Forsythe test for Nestin+, Kruskal–Wallis test for Nestin+PCNA+). Expressions of (C) glial fibrillary acidic protein (GFAP), Scl1a3, Hes5, and Sox2 are not affected by the application of DMCM or CGP. Pax6 expression is diminished in CGP treated animals. The relative mRNA expressions of markers were measured by quantitative real-time PCR in rat brain homogenates with DMCM 50 μg/kg (gray bars) or CGP 10 mg/kg (black bars) application relative to control (white bars). Bars represent the relative mRNA quantification based on internal standard HPRT. Data shown as mean ± SEM, n = 9–10. **p < 0.01 vs. control (Brown-Forsythe test for GFAP, one-way analysis of variance (ANOVA) for Scl1a3, Hes5, Sox2, and Pax6).
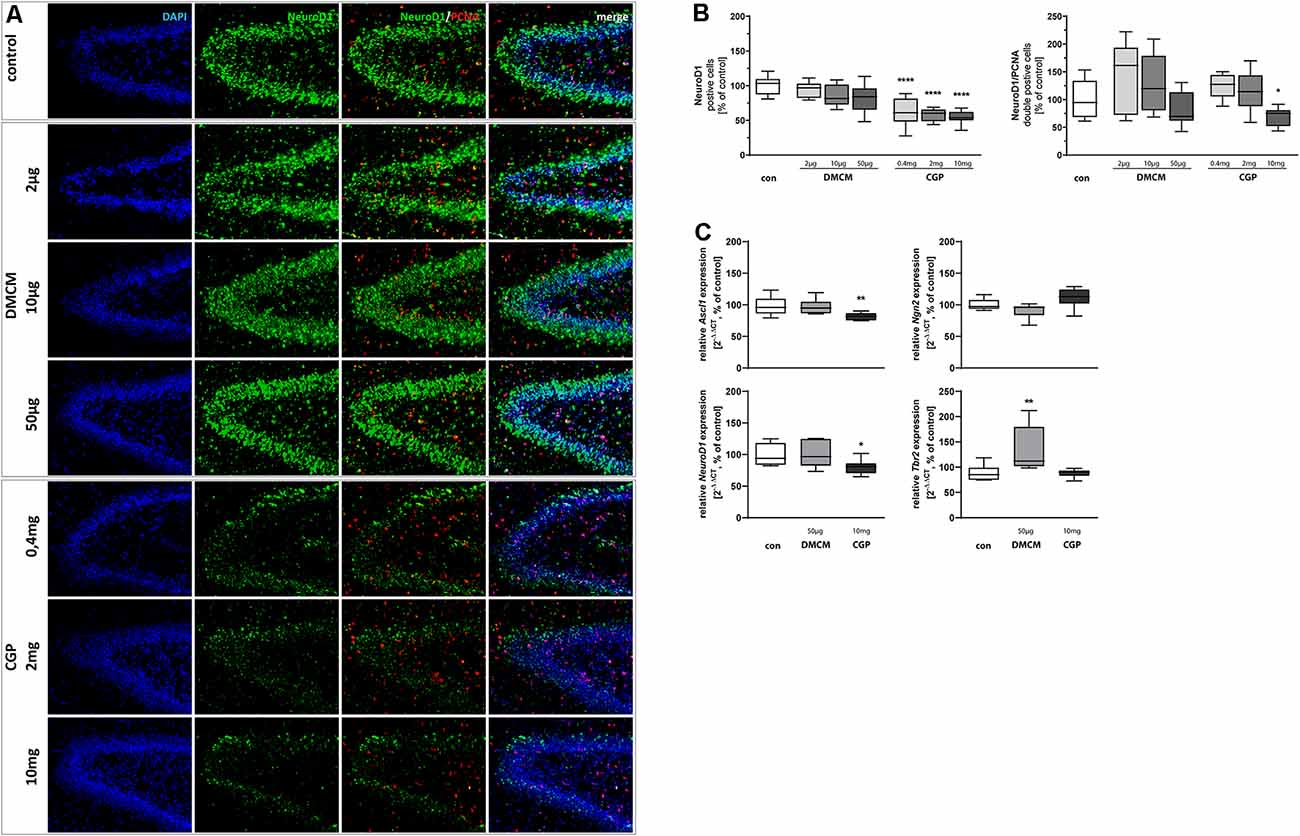
Figure 2. Representative hippocampal paraffin sections (A) of control animals, DMCM hydrochloride (DMCM) in doses of either 2 μg/kg, 10 μg/kg or 50 μg/kg, and CGP 35348 in doses of either 0.4 mg/kg, 2 mg/kg or 10 mg/kg treated rat pups at P11 co-labeled with DAPI, NeuroD1, and PCNA. Application of GABAB receptor antagonist CGP decreased NeuroD1 positive progenitor cells in the DG and NeuroD1/PCNA double positive cells in the group with the highest dose of 10 mg/kg. Quantification of (B) NeuroD1 and NeuroD1/PCNA double positive cells in sum of the DG in comparison to control group (100% white bars). Data are expressed relative to the control group as mean ± SEM of n = 10 each group. The 100% values are for NeuroD1+ 308.9 cell counts and for NeuroD1+PCNA+ 7.7 cell counts. *p < 0.05 and ****p < 0.0001 vs. control (one-way ANOVA for NeuroD1+, Brown-Forsythe test for NeuroD1+PCNA+). Expressions of (C) Ascl1 and NeuroD1 are reduced in CGP treated animals and expression of Tbr2 is increased in DMCM treated animals. Ngn2 does not get affected by GABA receptor antagonists. The relative mRNA expressions of markers were measured by quantitative real-time PCR in rat brain homogenates with DMCM 50 μg/kg (gray bars) or CGP 10 mg/kg (black bars) application relative to control (white bars). Bars represent the relative mRNA quantification based on internal standard HPRT. Data shown as mean ± SEM, n = 9–10. *p < 0.05 and **p < 0.01 vs. control (one-way ANOVA for Ascl1 and Tbr2, Kruskal–Wallis test for NeuroD1, Brown-Forsythe test for Ngn2).
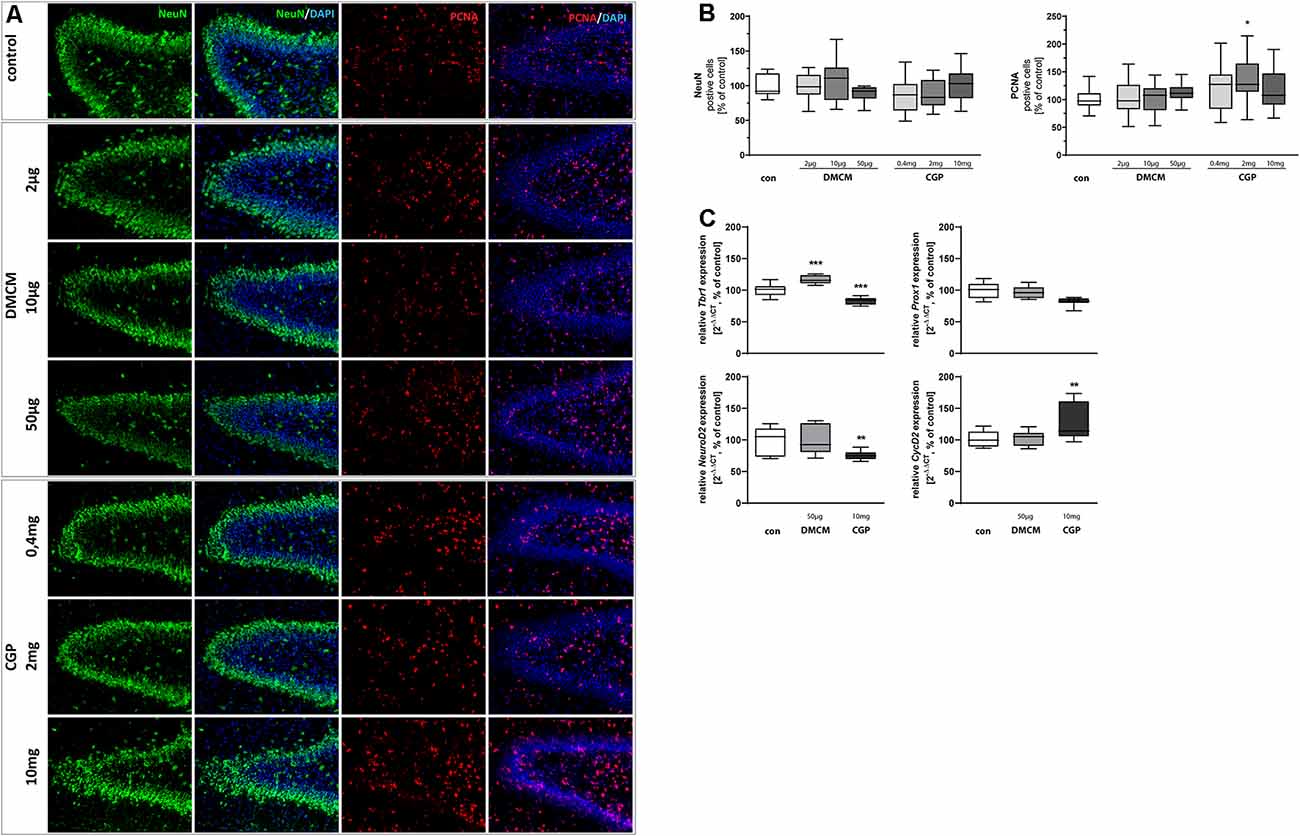
Figure 3. Representative hippocampal paraffin sections (A) of control animals, DMCM hydrochloride (DMCM) in doses of either 2 μg/kg, 10 μg/kg or 50 μg/kg, and CGP 35348 in doses of either 0.4 mg/kg, 2 mg/kg or 10 mg/kg treated rat pups at P11 co-labeled with DAPI, NeuN, and PCNA. Application of GABA receptor antagonists did not affect cell counts for postmitotic NeuN+ neurons at the DG. Application of CGP 2 mg/kg led to an increased number of proliferating PCNA+ cells. Quantification of (B) NeuN and PCNA positive cells in sum of the DG in comparison to control group (100% white bars). Data are expressed relative to the control group as mean ± SEM of n = 10 each group. The 100% values are for NeuN+ 143.0 cell counts and for PCNA+ 81.8 cell counts. *p < 0.05 vs. control (Brown-Forsythe test). Expressions of (C) Tbr1 and NeuroD2 are reduced and expression of CycD2 is increased in CGP treated animals. Expression of Tbr1 is increased in DMCM treated animals. GABA receptor antagonists do not affect the expression of Prox1. The relative mRNA expressions of markers were measured by quantitative real-time PCR in rat brain homogenates with DMCM 50 μg/kg (gray bars) or CGP 10 mg/kg (black bars) application relative to control (white bars). Bars represent the relative mRNA quantification based on internal standard HPRT. Data shown as mean ± SEM, n = 9–10. **p < 0.01 and ***p < 0.001 vs. control (Brown-Forsythe test for CycD2 and NeuroD2, one-way ANOVA for Tbr1).
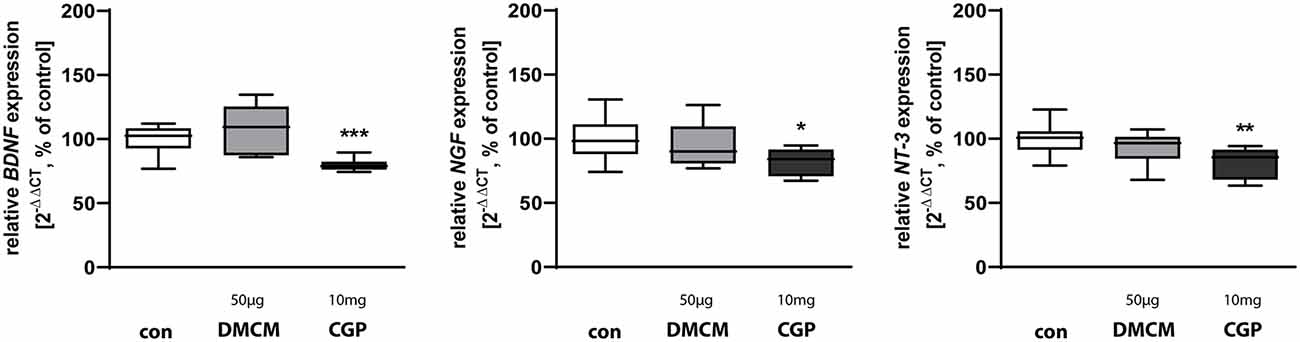
Figure 4. Expression of neurotrophins BDNF, NGF, and NT-3 is reduced in CGP treated animals. The relative mRNA expression of markers was measured by quantitative real-time PCR in rat brain homogenates with DMCM 50 μg/kg (gray bars) or CGP 10 mg/kg (black bars) application relative to control (white bars). Bars represent the relative mRNA quantification based on internal standard HPRT. Data shown as mean ± SEM, n = 9–10. *p < 0.05, **p < 0.01, and ***p < 0.001 vs. control (Brown-Forsythe test for BDNF, one-way ANOVA for NGF and NT-3).
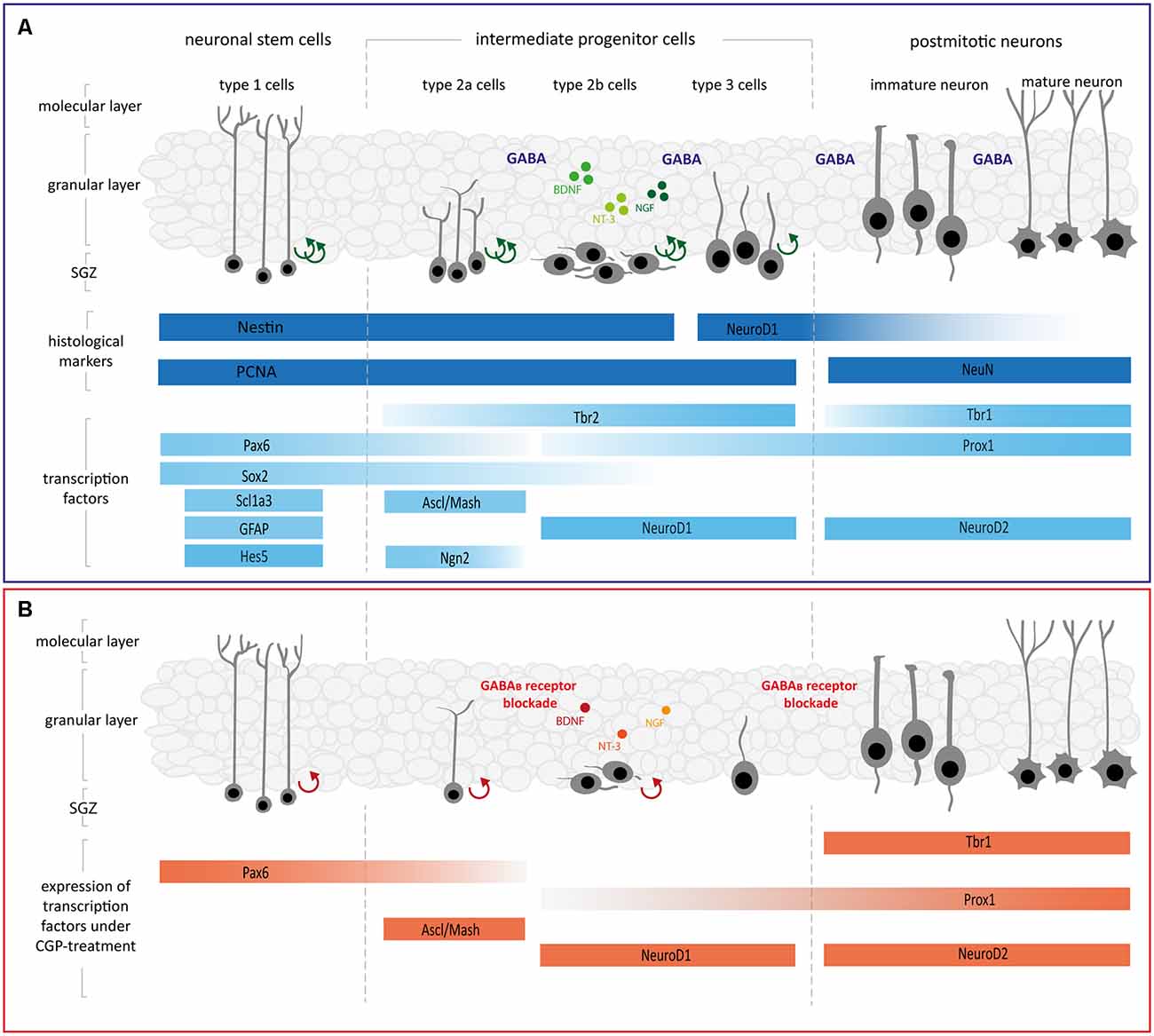
Figure 5. (A) Schematic diagram illustrating the different phases of neurogenesis in the DG. Asymmetrically dividing neuronal stem cells situated at the subgranular zone (SGZ) of the DG give rise to intermediate progenitor cells type-2a, type-2b, and type-3, which are highly proliferative, and their progeny determined for neuronal fate. Following further differentiation, intermediate progenitors extend processes, mature, exit the cell cycle and finally differentiate into mature neurons. Most of the regulators play an important role in self-renewal, proliferation, and fate specification during neurogenesis (see main text for details, modeled after Roybon et al., 2009; Lugert et al., 2010; Berg et al., 2018; Vieira et al., 2018; Hevner, 2019). (B) Schematic diagram depicting how the GABAB receptor blockade changes single steps of hippocampal neurogenesis. GABAB receptor blockade with CGP led to reductions in proliferating Nestin+ as well as NeuroD1+ cells, furthermore, expression of Pax6, Ascl1, NeuroD1, Tbr1, Prox1, and NeuroD2 was decreased (see main text for details, modeled after Roybon et al., 2009; Lugert et al., 2010; Berg et al., 2018; Vieira et al., 2018; Hevner, 2019). Abbreviations: Ascl1, achaete-scute family bHLH transcription factor 1; BDNF, brain-derived neurotrophic factor; CycD2, cyclin D2; GABA, γ-aminobutyric acid; GFAP, glial fibrillary acidic protein; Hes5, hairy-enhancer-of-split 5; NeuroD1/2, neurogenic differentiation 1/ 2; Ngn2, neurogenin 2; NGF, nerve growth factor; NT-3, neurotrophin 3; Pax6, paired box 6; Prox1, prospero homeobox 1; Sox2, SRY-box transcription factor 2; Scl1a3, solute carrier family 1 member 3; SGZ, subgranule zone; Tbr1/2, T-box brain transcription factor 1/2.
The neurotransmitter GABA, in tandem with the neurotransmitter glutamate and its receptors, is essential for balancing excitation and inhibition and subject to various changes during pre- and postnatal development (Takesian and Hensch, 2013). The ionotropic GABAA receptor and the metabotropic GABAB receptor are two main subtypes of GABA receptors. Berg et al. (2013) postulated that in addition to the progression of neural stem cells into functionally integrated mature neurons, cell cycle regulation and cell differentiation might be part of the functions of neurotransmitters. Various neurotransmitter receptors are expressed on different neuronal cell types (Pocock and Kettenmann, 2007). The functional roles for GABA during adult neurogenesis are already well described in part (Ge et al., 2006; Giachino et al., 2014; Song et al., 2016; Catavero et al., 2018), but it is poorly understood which steps in hippocampal neurogenesis at the time of brain growth spurts are vulnerable to disturbance of GABAergic signaling. Since the referenced evidence suggests that modulation of the GABA receptors during early life induces behavioral abnormalities in later life, we hypothesized that neonatal pharmacological blockade of GABAA and GABAB receptors during excitatory to inhibitory switch of GABA signaling may alter neuronal proliferation, differentiation, and maturation of hippocampal neurons in newborn rat pups.
Materials and Methods
Animal Welfare
Time-pregnant Wistar rat dams were obtained from the Department of Experimental Medicine (FEM, Charité—Universitätsmedizin Berlin, Germany). The rat litters were housed with lactating mother under temperature- and humidity-controlled 12 h/12 h light/dark cycle conditions with ad libitum access to food and water. All animal experimental procedures were approved by the local animal welfare authorities (LAGeSo, approval number G-0075/18) and followed institutional guidelines as well as ARRIVE guidelines.
Drug Administration
Rat pups were cross-gender randomly assigned into a control group with 0.9% saline and six verum groups with GABAA receptor antagonist DMCM (4-Ethyl-6,7-dimethoxy-9H-pyrido[3,4-b]indole-3-carboxylic acid methyl ester) hydrochloride administered at three dosages (2 μg/kg, 10 μg/kg, or 50 μg/kg body weight; Tocris, cat. no. 3083, Wiesbaden-Nordenstadt, Germany), and with GABAB receptor antagonist CPG 35348 at 0.4 mg/kg, 2 mg/kg, or 10 mg/kg body weight (Tocris, cat. no. 1245), respectively. Beginning at postnatal day 6 (P6) the rat pups were intraperitoneally injected (i.p.) with saline, DMCM, or CGP 35348 daily on six consecutive days (P6 to P11) with a weight-adapted volume of 0.1 ml per 10 g of body weight. The dosage of the two GABA receptor antagonists was selected to exclude seizures and shakiness. Behavioral seizure responses were monitored for 30 min after drug administration. No pups died or scored for seizure activity. Animals were sacrificed within 12 h following the last injection. For dose-dependent histological analysis of GABA receptor antagonization, each group entailed 10 animals. Gene expression analyses were done in animals receiving the highest concentration of GABA receptor antagonists (DMCM hydrochloride 50 μg/kg and CGP 35348 10 mg/kg body weight (i.p.), respectively) and included nine to 10 animals. The two substances used are hereinafter referred to as DMCM and CGP.
Tissue Preparation
For histological analysis, as previously described (Endesfelder et al., 2018), at postnatal day 11 (P11) rat pups were transcardially perfused with ice-cold phosphate-buffered saline (PBS, pH 7.4), followed by 4% paraformaldehyde (PFA) in PBS under anesthesia of ketamine (100 mg/kg), xylazine (20 mg/kg), and acepromazine (3 mg/kg). The removed brain tissues were post-fixed in 4% PFA at 4°C for 24 h. Afterward they were transferred to PBS (pH 7.4) and stored at 4°C until paraffin embedding. In preparation for immunohistochemical analyses, the brains were embedded in paraffin. For this purpose, the tissues were first washed under running water for 4 h. This was followed by various dehydrating incubations of the brain tissue with increasing alcohol (ethanol) concentrations (70% for 4 h and 80% overnight at room temperature, 96% and two changes of 100% for each 1 h at 40°C) and chloroform (100% ethanol/chloroform (1:1) and two changes of chloroform for each 1 h at 40°C). Finally, the tissues were immersed in two changes of paraffin (1 h and overnight) at 60°C, cooled, and stored at room temperature.
For gene expression analysis, animals were transcardially perfused with ice-cold PBS (pH 7.4). After decapitation, the olfactory bulb and cerebellum were removed, and brain hemispheres were snap-frozen in liquid nitrogen and stored at −80°C.
RNA Extraction and Quantitative Real-Time PCR
The gene expression analysis was performed as previously described (Endesfelder et al., 2020). In short, total RNA was isolated from frozen tissue of the whole hemisphere by acidic phenol/chloroform-extraction (peqGOLD RNAPureTM; PEQLAB Biotechnologie, cat. no. 30-1010, Erlangen, Germany). 2 μg of DNase-treated RNA was reverse transcribed. In real time the PCR products of the following genes were quantified: achaete-scute family bHLH transcription factor 1 (Ascl1), brain-derived neurotrophic factor (BDNF), cyclin D2 (CycD2), GFAP, hairy-enhancer-of-split 5 (Hes5), hypoxanthine-guanine phosphoribosyl-transferase (HPRT), neurogenic differentiation 1 (NeuroD1), neurogenic differentiation 2 (NeuroD2), neurogenin 2 (Ngn2), nerve growth factor (NGF), neurotrophin 3 (NT-3), paired box 6 (Pax6), prospero homeobox 1 (Prox1), SRY-box transcription factor 2 (Sox2), solute carrier family 1 member 3 (Scl1a3), T-box brain transcription factor 1 (Tbr1), and T-box brain transcription factor 2 (Tbr2).
Table 1 shows the sequences of dye-labeled fluorogenic reporter oligonucleotides used for real time amplification. Probes were labeled with the fluorescent reporter 6-carboxy-fluorescein (6-FAM) at the 5′ end and the fluorescent quencher carboxytetramethylrhodamine (TAMRA) at the 3′ end. PCR and detection were performed with qPCR BIO Mix Hi-ROX (NIPPON Genetics Europe, cat. no. PB20.22-51, Düren, Germany) with HPRT used as an internal reference. The expression of target genes was analyzed according to the 2−ΔΔCT method (Livak and Schmittgen, 2001) with the StepOnePlus real-time PCR system (Applied Biosystems/Life Technologies, Carlsbad, CA, USA).
Immunohistochemistry
Paraffin-embedded coronal sections of the brains were serially cut into 5 μm sections and mounted onto Super Frost Plus-coated slides (Menzel, Braunschweig, Germany). The sections were deparaffinized in Roti-Histol twice for 10 min each. The PFA-fixed tissues were dehydrated through incubation in aqueous solutions of decreasing ethanol concentration. The slices were immersed for 3 min each in ethanol (100%, 100%, 90%, 80%, 70%). To demask intracellular epitopes, sections were fixed in citrate buffer (pH 6.0) in a microwave oven for 10 min at 600 W. The sections then were cooled down at room temperature for 30 min and were washed three times afterward in PBS for NeuN/DAPI- and Nestin/PCNA/DAPI-staining and in Tris-buffered saline (TBS) for NeuroD1/PCNA/DAPI-staining. For NeuN/DAPI- and Nestin/PCNA/DAPI-staining blocking solution [3% bovine serum albumin (BSA), 0.2% TX-100 in PBS] was applicated to each section for 60 min. For NeuroD1/PCNA/DAPI staining, blocking solution (5%BSA, 0.5% TX-100 in TBS) was applied instead. Sections were washed again in PBS for NeuN/DAPI- and Nestin/PCNA/DAPI-staining, and in TBS for NeuroD1/PCNA-staining before the primary antibody was applied overnight at 4°C.
Primary antibody, monoclonal mouse-anti-rat NeuN IgG (Merck Millipore, cat. no. MAB377, Darmstadt, Germany) diluted 1:200 in antibody diluent (Zymed Laboratories, San Francisco, CA, USA) or polyclonal goat anti-rat Nestin IgG (R&D, AF2736, Minneapolis, USA) diluted 1:20 in antibody diluent and monoclonal mouse anti-rat PCNA IgG (abcam, ab29, Cambridge, UK) diluted 1:1,000 in antibody diluent, or monoclonal mouse anti-rat NeuroD1 (abcam, ab60704) diluted 1:200 in antibody diluent and polyclonal rabbit anti-rat PCNA (abcam, ab152112) diluted 1:50 in antibody diluent was applied to each slide.
For NeuN/DAPI-staining sections were washed three times in PBS before the fluorescein-conjugated secondary antibody goat anti-mouse-IgG Alexa Fluor 488 (Thermo Fisher Scientific, Waltham, MA, USA, A11029, Rockford, IL, USA) diluted 1:200 in carrier solution was applied for 60 min in darkness at room temperature.
For Nestin/PCNA/DAPI- staining sections were washed three times in PBS before donkey anti-goat Alexa Fluor 488 (Thermo Fisher Scientific, Waltham, MA, USA, A11055) diluted 1:200 in antibody diluent was applicated for 4 h. Sections then were washed three times in PBS and goat anti-mouse Alexa Fluor 594 (Thermo Fisher Scientific, Waltham, MA, USA, A11032) diluted 1:200 in antibody diluent applicated for 60 min.
For NeuroD1/PCNA/DAPI- staining sections were washed three times in TBS before goat anti-mouse Alexa Fluor 488 (Thermo Fisher Scientific, Waltham, MA, USA, A11029) diluted 1:200 in antibody diluent was applied for 2 h. The sections were again washed three times in TBS and goat anti-rabbit Alexa Fluor 594 (Thermo Fisher Scientific, Waltham, MA, USA, A11037) diluted 1:200 in antibody diluent was applied for 60 min. Aqueous 4′,6-diamidino-2-phenylidole (DAPI; Sigma-Aldrich, #32670, Taufkirchen, Deutschland) 1:1,000 was applied for 10 min. After washing three times in PBS for NeuN/DAPI- and Nestin/PCNA/DAPI-staining, and three times in TBS for NeuroD1/PCNA/DAPI-staining, the sections were mounted and stored overnight at 4°C.
Images were acquired blinded on Keyence compact fluorescent microscope BZ 9000 with BZ-II Viewer software (Keyence, Osaka, Japan) using 10x objective lenses and individual files stitched automatically for each RGB color. Pictures were taken with the same exposure time and contrast/brightness parameters. Imaging files were analyzed and quantified in Adobe Photoshop software (Adobe Photoshop CS3 Extended). Double- (NeuN/DAPI) and triple-labeled (Nestin or NeuroD1/PCNA/DAPI) images in the DG were quantified by first outlining the hilus, GCL, and SGZ as a region of interest (ROI) at 10× magnification in Adobe Photoshop using DAPI to initially identify the cell-dense GCL. The area of the complete hilus, GCL, and SGZ of the DG, with an imaginary cut at the beginning of CA3, was counted manually for each staining up to four sections per animal separately. For multi-channel images, distinct channels were overlaid using the “Merge Channel”-function (overlaid images are indicated as “merge” in figures). To count the co-labeled positive cells, the different RGB channels were used overlapping. For each quantified marker, two different investigators for reproducibility repeated counts. The fluorescence signal for single reactivity and co-localization of immunoreactivity was counted individually using the markers function in the Adobe Photoshop software at 40× magnification. Mean values per sample were calculated by averaging the values of all sections of the same animal and were used to compare the cell counts of neuronal marker of GABA receptor antagonist treated animal vs. or control animals. For the representative imaging of immunohistological stainings, a background minimization with black balance identical for all images was performed.
Statistical Analyses
Box and whisker plots represent the interquartile range (box) with the line representing the median, while whiskers show the data variability outside the upper and lower quartiles. Groups were compared using one-way analysis of variance (ANOVA), based on a partially non-Gaussian distribution with the Kruskal–Wallis test or based on the assumption that groups do not have equal variances with the Brown-Forsythe test. Depending on which ANOVA test was used, multiple comparisons of means were carried out using Bonferroni’s, Dunn’s, or Dunnett’s T3 post hoc test. A p value of <0.05 was considered significant. All graphics and statistical analyses were performed using the GraphPad Prism 8.0 software (GraphPad Software, La Jolla, CA, USA).
Results
GABAB Receptor Antagonist Reduces Intermediate Progenitor Cells
To investigate the effect of GABAergic signaling on early progenitor cells at the beginning of neuronal cell lineage development, we stained for Nestin. This marker is expressed by NSCs (Nicola et al., 2015). The GABAB receptor antagonist CGP administered in a dose of 2 mg/kg led to a decreased number of Nestin+ cells at the DG. The proliferating Nestin+/PCNA+ double-stained cells were significantly diminished in animals treated with the highest dose of GABAB receptor antagonist CGP (10 mg/kg). The administration of the GABAA receptor antagonist DMCM, in contrast, did not significantly alter the number of Nestin+ or Nestin+/PCNA+ double-stained cells (Figures 1A,B). The expression of the NSC marker Pax6 was reduced in CGP-treated (10 mg/kg) animals. Multipotent NSCs, besides their neuronal qualities, also have astrocytic properties and thus express GFAP and Scl1a3 (DeCarolis et al., 2013; Berg et al., 2018). The expression of those astrocytic markers, GFAP and Scl1a3, was unchanged by either GABA receptor blockade (Figure 1C). By the expression of Hes5 and Sox2, NSCs inhibit neuronal differentiation and maintain their crucial multipotency and proliferation capacity (Pérez-Domínguez et al., 2018). The blockade of GABA receptors did not interfere with the expression of Hes5 and Sox2.
GABAB Receptor Blockade Weakens Number and Proliferation of Intermediate Progenitor Cells
Ongoing neuronal differentiation of NSCs’ progeny leads to intermediate progenitor cells of type 2a. Those still dividing Nestin+ type-2a cells express Ascl1, a transcription factor sufficient to induce neuronal differentiation (Vasconcelos and Castro, 2014). We found decreased expression of Ascl1 in CGP-treated animals. Ngn2 is another transcription factor up-regulated in type-2a cells (Pérez-Domínguez et al., 2018). Ngn2 expression was not affected by the administration of GABA receptor antagonists (Figure 2C). The neuronal fate choice of intermediate progenitor cells leads to decreasing expression of Nestin while type-2b cells and neuroblast-like type-3 cells become NeuroD1 positive (Nicola et al., 2015). NeuroD1+ cells were reduced in all groups treated with CGP, indicating that intermediate progenitor cells at the differentiation stages of type-2b cells and neuroblast-like type-3 cells are especially affected by the GABAB receptor blockade. The highest dose of CGP with 10 mg/kg also led to reduced proliferation of NeuroD1+/PCNA+ double-stained cells (Figures 2A,B). In line with these results, qPCR showed a significant reduction of NeuroD1 at RNA gene expression level in CGP-treated animals. The expression of Tbr2, a broader marker for all type-2 cells, was not altered by CGP. DMCM-treatment on the contrary did not affect the number of NeuroD1+ and NeuroD1+/PCNA+ cells and did not change Ascl1 or NeuroD1 expression, but led to an increased Tbr2 expression.
Postmitotic Neurons Are Less Affected by GABA Receptor Blockade
NeuN staining (Vieira et al., 2018) identified postmitotic neurons. The number of postmitotic neurons in the DG was not significantly affected by the administration of GABA receptor antagonists (Figures 3A,B). The expression of Tbr1, however, another marker for postmitotic neurons, was reduced in CCP-treated animals and increased in DMCM-treated ones. NeuroD2, a third marker for postmitotic neurons, showed decreased expression after CGP-treatment and was not affected by DMCM. Prox1, involved in granule cell maturation (Lavado et al., 2010), was not altered by the administration of GABA receptor antagonists (Figure 3C).
GABAB Receptor Antagonists Affected Hippocampal Proliferation Capacity
The highly regulated ability of precursor cells to proliferate and self-renew is pivotal for the functioning of hippocampal neurogenesis up to adult age (Berg et al., 2018). In order to investigate the dependency of hippocampal proliferative capacity on GABA receptor blockade, slices were stained for the endogenous proliferation marker PCNA. The number of proliferating and therefore PCNA+ cells increased after treatment with 2 mg/kg CGP, while it was not altered in DMCM-treated groups (Figures 3A,B). It should be noted that despite a significant difference in the CGP-treated animals compared to the control, the variance is very high. Additionally, we observed an increased expression of CycD2 in CGP-treated animals.
GABAB Receptor Blockade Reduces Expression of Neurotrophins
Neurotrophins play important roles in defining the hippocampal neurogenic niche (Faigle and Song, 2013). Brain-derived neurotrophic factor (BDNF) affects structural plasticity, dendritic spine growth, and has long–term effects on LTP and learning (Leal et al., 2015; Gonçalves et al., 2016). Nerve growth factor NGF as well as neurotrophin 3 NT-3 are involved in the regulation of synaptic plasticity (Leal et al., 2015). All tested neurotrophic factors, BDNF, NGF, and NT-3, were downregulated in rats treated with CGP while DMCM did not alter the expression of any of these neurotrophic factors (Figure 4).
Briefly, we observed a reduction of NPC, especially of type-2b and type-3, caused by GABAB receptor blockade. In addition, the proliferation of progenitors at these stages in hippocampal neurogenesis was diminished. Gene expression analysis revealed a reduced expression of multiple transcription factors involved in neuronal fate choice (see schematic diagram in Figure 5). After GABAA receptor blockade, in contrast, there was no significant impairment of neurogenesis observed on a cellular level and changes in RNA-expression affected only Tbr 1 and Tbr 2, leading to an overexpression of these factors.
Discussion
In the present study, we investigated the effects of a GABAA and GABAB receptor antagonist on postnatal hippocampal neurogenesis in newborn rats, during the developmental depolarizing-to-hyperpolarizing switch in response to the neurotransmitter GABA (Ben-Ari, 2018). Our data suggest that antagonizing GABAB receptor activity impaired the progression of neural progenitors to mitotic immature neurons, reduced the expression of neurotrophins, and increased the proliferation capacity. In contrast, antagonizing GABAA receptors did not affect postnatal hippocampal neurogenesis at a cellular level.
GABA is one of the most abundant neurotransmitters in the central nervous system. GABAergic neurons are the main source of GABA; additionally, synthesis occurs in glial cells (Angulo et al., 2008; Héja et al., 2012). The subtypes of GABAB receptors, GABAB1a/b and GABAB2, were shown to be located both pre- and postsynaptically, indicating a more modulatory function in the developing brain (López-Bendito et al., 2004; Evenseth et al., 2020). Various experimental models suggest that GABAB receptor antagonists modulate hippocampal-linked learning, memory, and behavior (Cryan and Kaupmann, 2005; Heaney and Kinney, 2016).
During neuronal lineage development, intermediate progenitor cells have been described to successively express a cascade of transcription factors starting with Pax6 expression in type-1 and type-2a cells, and further involving Ngn2, Tbr2, NeuroD1, Tbr1, and Prox1, which is expressed from the stage of type-2b cells onwards (see schematic overview in Figure 5; Gonçalves et al., 2016; Pérez-Domínguez et al., 2018; Hevner, 2019). First, GABAB receptor blockade reduced the total Nestin+ and proliferating Nestin+ cells in the DG, depending on concentration. Nestin is widely used as a marker for proliferating neuroepithelial and progenitor cells as well as some astrocytes in the hippocampus (Von Bohlen und Halbach, 2011; Wilhelmsson et al., 2019). Further, Nestin is essential for the survival and self-renewal of NSC (Park et al., 2010). Quiescent type-1 NSC and active NSC differ in frequency of proliferation, while both cell types share the characteristic expression of the glial cell marker GFAP and neural progenitor marker Nestin (Lugert et al., 2010). Neither GFAP nor the astrocytic markers SCL1a3/GLAST were impaired in gene expression by the antagonization of the GABAB receptor. Sox2-expressing cells in the SGZ generate differentiated and identical cells, indicating their multipotent properties and importance for self-renewal (Suh et al., 2007). Similar to Sox2, Hes5-positive cells specifically inhabit the SGZ, with the property of NPC in the neurogenic niche (Lugert et al., 2010). Both transcription factors of type-1 cells, Sox2 and Hes5, were not affected by the blockade of GABA. This is in line with the thesis that type-1 NSCs are not affected as a whole, while the proliferating subpopulation of type-1 NSC depends on GABAergic signaling.
It is interesting that Pax6, classifying newly born cells from the differentiation state in SGZ and regulating self-renewal of NSC (Maekawa et al., 2005), was significantly reduced by the performed GABAB receptor blockade. The impact on Nestin+ NSC and Pax6 expression suggests that GABAB receptor-mediated GABA signals may be central in determining the neuronal fate and proliferation of NSCs. NSCs self-renew to generate asymmetrically a new daughter NSC and a daughter NSC with properties of intermediate NPC (type-2 cells; Gonçalves et al., 2016). The maintenance of pluripotency in NPC by Sox2 is well-defined (Favaro et al., 2009). Sox2 binds to the regulatory regions of a number of genes involved in neuronal differentiation (Lodato et al., 2013), including proneural genes such as Ascl1 (Vasconcelos and Castro, 2014) and NeuroD1 (Kuwabara et al., 2009). The substitution of Sox2 by neurogenic signals at the regulatory promotor regions is required for activation of proneural genes and therefore downregulation of Sox2 a precondition for further neuronal differentiation (Kuwabara et al., 2009). GABA antagonists did not interfere with the level of Sox2 expression in the postnatal DG.
One step further in neuronal differentiation, changes caused by GABA receptor blockade were detectable. Mediators of intermediate progenitor cells, Ascl1, NeuroD1 as well as proliferating NeuroD1+ cells were significantly decreased under GABAB receptor blockade. Ascl1 is a proneural transcription factor and is expressed in mitotic NPC (Uda et al., 2007). The transcription factor NeuroD1, which may act as a neuronal determination gene, is expressed in the middle and late stages of NPC (Von Bohlen und Halbach, 2011). Interestingly, Ascl1 is able to play two opposing roles. On the one hand, it promotes proliferation and on the other supports cell-cycle exit and differentiation (Andersen et al., 2014). Ascl1 enables the transcription of multiple proneuronal target genes (Vasconcelos and Castro, 2014). A decreased expression of Ascl1 after GABAB receptor blockade might explain the observed delay in NPC differentiation. This does not necessarily lead to decreasing proliferation per se. Although the proportion of mitotic maturing neurons (NeuroD1+/PCNA+ cells) was reduced, the overall proliferation capacity (PCNA+ cells, CycD2) was induced. This could be due to Ascl1’s interaction with Notch target genes in neural precursor cells via downstream mediators (Andersen et al., 2014), similar to Hes5 (Lugert et al., 2010). Hes proteins activated via the Notch signaling pathway act as repressors of proneural transcription factors. Due to their self-regulation and short half-lives, the cellular expression of Hes proteins is not constant and thus leads to fluctuating expression of downstream target genes, such as Ngn2 and Ascl1 (Lugert et al., 2010; Vasconcelos and Castro, 2014). Expressions that can be changed in this way could promote proliferation, while steady conditions lead to the progression of differentiation.
Current measurement technology at a certain point is not sufficiently capable of detecting oscillating expressions of these mediators. However, our findings support the hypothesis that NeuroD1, which is directly regulated by Pax6 (Thakurela et al., 2016), and Ascl1 are significantly reduced in expression, while proliferation is increased. Typically, NeuroD1 expression peaks in early neuroblasts/type-2b and type-3 cells. Diminished NeuroD1 expression seems to be partly responsible for the stagnation of differentiation and progression of neurogenesis (Gao et al., 2009).
A possible explanation for the observed general boost in proliferation capacity beyond the transcriptional regulation of hippocampal neurogenesis might be GABAergic signaling itself. GABAergic signals through GABA receptors as well as the loss of GABAA and GABAB receptor subtypes were described to increase proliferation of NSC in the adult DG (Song et al., 2012; Giachino et al., 2014). GABAB receptor function is associated with suppressed proliferation of adult hippocampal neurogenesis (Felice et al., 2012). The impact of GABAB receptor blockade already in postnatal development with excitatory GABA on NPC associate transcription factors suggests that GABAB receptor-mediated signals may be important for initiating transcription of proneural mediators and generate mitotic neuroblasts.
Nevertheless, mitotic immature neuroblasts/type-2b cells express Tbr2 (Hodge et al., 2012). Before the neuroblasts exit the cell cycle to become mature granular neurons, they start expressing Tbr1, Prox1, and NeuroD2 (Gonçalves et al., 2016). Downregulation of Tbr2 is the precondition for NPC to differentiate into postmitotic immature neurons and this coincides with upregulation of Tbr1, which is strongly associated with new mature neurons (Hodge et al., 2012). Late neuroblasts/type-3 cells and postmitotic granular neurons express the transcription factor Prox1 (Li et al., 2009). NeuroD2 induces a neural phenotype, while its possible involvement in terminal differentiation is still being investigated (Ravanpay et al., 2010). Considering the crucial tasks of these transcription factors for final neurogenic termination, a significant downregulation of gene expression would suggest a reduction in the number of mature neurons. Controversially, GABAB receptor antagonization sustainably reduced the expression of relevant transcription factors (Prox1, Tbr1, NeuroD2), but did not affect the NeuN+ neurons in DG.
Prox1 was applied as a dentate granule neuron lineage marker (Rubin and Kessaris, 2013). Several studies (Lavado et al., 2010; Iwano et al., 2012) suggested Prox1 to act pleiotropically and modulate multiple targets as well as signaling pathways possibly through dysregulation of Notch signaling. Postnatal deletion as well as a conditional knockout of Prox1 in mice results in decreased counts of NPC subtypes (type-2a/b cells) and NSC/ type-1 cells (Lavado et al., 2010). Further, overexpression of NeuroD1, but not of either Ngn2 or NeuroD2, was able to drive the exclusive production of new neurons in the adult hippocampus (Richetin et al., 2015). A milieu dominated by the differentiation-driving effects of NeuroD1 might overlay the stage-specific action of NeuroD2. Particularly since NeuroD2 is required for the survival of hippocampal neurons (Olson et al., 2001), one might expect apoptosis-associated mediators to increase; however, in our study neither the expression profiles of apoptosis-associated mediators, effector caspase 3 (Casp3), and apoptosis-inducing factor (AIF), nor the total DAPI+ cell counts showed changes after switching off GABA receptor signaling compared to the control animals (see Supplementary Figures 1A,B).
The temporal delay in the differentiation of the NPC in our model of GABAB receptor blockade is in line with a hypothesis formulated by Lugert et al. (2012). Instead of the amplification of type-2a intermediate progenitors as seen previously, though, they proposed that divisions of neural stem cells and early neuroblasts drive DG neurogenesis (Lugert et al., 2012). Mitotic NSCs in the DG provide neuronal progenitor expansion and initiate neurogenic differentiation, a process requiring more than 3 weeks under homeostatic conditions. After the initiation of neurogenesis, cells remain at the immature NPC developing stage for some weeks. In this phase, neuroblasts adapt to physiological or pathophysiological stimuli that may affect their maturation and differentiation. The NPC population reacts to specific noxae and, if required, rapidly initiates the production of many neurons (Dranovsky et al., 2011; Lugert et al., 2012). The hypothesis that NPCs remain in an immature state for a long period and retain their ability to expand neuronal cells and continue neurogenesis in order to quickly provide missing neurons when needed could support our observation of an unchanged population of mature neurons after GABA receptor blockade despite massive impairment of intermediate neurons. A hypothetical subsequent effect on the proportion of mature neurons resulting from a reduced differentiation capacity of maturing intermediary neurons did not occur at time P15 (see Supplementary Figure 2).
The described impact of transcription factors associated with mature granular neurons suggests that GABAB receptor-mediated GABA signals might be important for the maintenance of the NPC cell pool by the initiation of pleiotropic interactions. Since glial markers were not influenced by GABAA or GABAB receptor antagonization, only the neural line seems to be affected. Interestingly GABAA receptor blockade did not lead to any changes similar to those caused by GABAB receptor blockade. Instead, it resulted in an overexpression of Tbr2 and Tbr1. There seem to be essential differences regarding the influence of GABA and its mediation via the respective receptors. Considering the GABA receptor density, a significant increase of GABAA receptor occurs between P10 and P20, while the GABAB receptor density peaks between P0 and P10 at P5 (Behuet et al., 2019). Due to the animal model we used, an effect of GABA blockade via the GABAB receptor seems more probable. GABA-mediated effects via the GABAA receptor require further investigation.
Intrinsic factors including neurotransmitters (Catavero et al., 2018), hormones (Triviño-Paredes et al., 2016), and neurotrophic factors (Lee et al., 2002) influence hippocampal neurogenesis. Neurotrophic factors, such as NT-3, NGF, and BDNF, are key players in the stimulation of NSC proliferation and differentiation (Ding et al., 2013). Interestingly, GABAB receptor peak coincides with the time course of synaptogenesis, which is regulated by neurotrophins such as BDNF (Gaiarsa and Porcher, 2013). Coordinated effects of GABA and neurotrophins are important for the long–term survival of newborn neurons (Vilar and Mira, 2016). Waterhouse et al. (2012) suggested a reciprocal interaction between neurotransmitters and neurotrophic factors and showed that BDNF promotes progenitor cell differentiation and maturation by enhancing the release of GABA in the SGZ, resulting in increased differentiation and maturation of progenitor cells. Glial cells and GABAergic neurons are not sources for the secretion of neurotrophins themselves. BDNF is provided by pyramidal neurons or mossy fibers of hippocampal granular cells (Danzer and Mcnamara, 2004). BDNF is a crucial regulator of synapse development (Cohen-Cory et al., 2010) and shapes the development of neuronal circuits, as well as the construction of inhibitory connections throughout life (Gottmann et al., 2009). Similar findings were reported for NGF and NT-3, both involved in several critical processes in the developing brain (Shimazu et al., 2006; Frielingsdorf et al., 2007). We found that the inactivation of metabotropic GABAB receptors downregulates the transcription of BDNF, NGF, and NT-3. Currently, studies on the effects of GABAB receptor blockade on the neurotrophic response are limited. Overexpression of BDNF in hippocampal cultures results in an earlier maturation of inhibitory synapses by inducing both presynaptic and postsynaptic structural and functional modifications to enhance GABAergic transmission (Vicario-Abejón et al., 1998). Badurek et al. (2020) show that early disruption of Trkb signaling, the receptor for BDNF, from immature mouse hippocampal dentate granule cells affects the integration and maturation of newly formed DGCs in the hippocampal circuitry, and drives a premature shift from depolarizing to hyperpolarizing GABAergic actions. Conditional NT-3 knockout in mice goes along with an impairment of neuronal differentiation (Shimazu et al., 2006). Intracerebroventricular treatment with NGF increases hippocampal neurogenesis and enhances the survival of new neurons in young and aged rats (Frielingsdorf et al., 2007). Likewise, following GABAB receptor activation and regulated secretion of BDNF increases the number of postsynaptic GABAA receptor subunits (Kuczewski et al., 2011). This implies complex interactions between GABAB receptor activation and neurotrophins to contribute to the functional and structural maturation of the developing hippocampus. A decreased neurotrophic response following GABAB receptor antagonization appears to be consistent with the stagnant maturation of NPC. Neurotrophins may have important roles in hippocampal neurogenesis and developmental plasticity (Porcher et al., 2018). Imbalance in neurotransmission seems to lead to the reduction of neurotrophin transcription; therefore, further investigations on the precise mechanisms of the relationship between neurotrophic growth factors and GABAergic transmission are required.
Taken together, GABA influences the development and functioning of the GABAergic network mainly via GABAB receptor during the first 10 postnatal days. As a result of the limited signal transduction of GABA via the GABAB receptor, impairment of neurogenesis advanced, which did not affect the final termination to mature neurons, and diminished neurotrophin signals. This could imply that although antagonization of GABAB receptors recruited quiescent cells to the active proliferative stem cell pool, progression towards terminal differentiation was not induced. This is in line with Giachino et al.’s (2014) proposal that GABAB receptor activity controls the number of proliferating NPC in the adult hippocampus. In sum, GABA and the GABAB receptors’ transmission affect neural stem cell and progenitor cell proliferation in the developing hippocampus.
The involvement of GABA receptors in neurogenesis was linked to the modulation of cognitive processes, like memory formation, executive function, learning, and intelligence. Imbalances during GABAergic transmission in neuronal circuits, such as drugs or oxygen, can affect these vulnerable phases of brain development and disrupt homeostatic control (Vertkin et al., 2015; Friedman and Kahen, 2019). Failure in neuronal homeostasis has been linked to pathophysiological mechanisms of various brain disorders (Marín, 2012; Kim and Yoon, 2017), like autism, hyperactivity, inattention, social and emotional incompetence, which are also associated with preterm birth (Fatemi et al., 2009; Arpino et al., 2010; Hashemi et al., 2017). For a deeper understanding of postnatal brain development and improving the understanding of GABA signaling in correspondence to preterm birth, further analysis will be required to establish which cues regulate the various stages of neurogenesis beyond NSC expansion, NPC proliferation, and maturation as well as the survival of newly formed neurons. These may open new therapeutic strategies to alleviate behavioral impairments and neurological disorders, perhaps in part as sequela of early derangement in GABAergic systems.
Limitations of the Study
Altogether, these observations point to GABA as one of the major players in the early formation of neuronal circuits in the developing brain. Since GABAB receptors are expressed in hippocampal progenitor cells as well as throughout the adult neurogenic lineage, it is possible that antagonization of GABA receptor signaling affects non-hippocampal neuronal cells. For gene expression analysis, we used the complete hemisphere and removed the olfactory bulb and cerebellum. The data collected cannot be adequately and exclusively associated with the hippocampus and we are aware that the transcriptional markers are also expressed in other brain regions of the developing brain (Rodier, 1980; Rice and Barone, 2000; Lavado and Oliver, 2007; Bedogni et al., 2010; Hsieh, 2012; Miyoshi et al., 2015). The main proliferating niche during the phase of rapid brain growth is the DG (Stefovska et al., 2008). A study by Stefovska et al. (2008) showed in the developing brain (P0 to P15), that the in vivo modulation of GABA receptors changes the proliferation capacity in different brain regions, such as cortical sections, thalamus, and in the DG (SGZ and granular cell layer) to the same extent. Not only newly generated neurons in the proliferative neurogenic niches of the immature brain express GABA receptors, so that an impairment after systemic administration of GABA antagonists or agonists can act on different reaction pathways, on different cell types and can affect cells practically in all parts of postnatal brain. Nevertheless, postnatal cell proliferation is age-dependent and most pronounced in the cerebellum and the SGZ of the DG. Outside the cerebellum and DG, proliferating new neurons become non-neuronal cells, like glia cells. The neurotransmitter GABA constitutes a developmental signal during stages of embryonic neurogenesis, progenitor proliferation, neuronal migration, and neurite outgrowth (Wang and Kriegstein, 2009; Xing and Huttner, 2020). Differences in the transcription of neurogenesis-associated genes and specifically neuronal lineage associated cells are then less expected in the whole brain RNA or protein extract (if removal of olfactory bulb and cerebellum) as all are affected to the same extent. Significant change of transcript levels may prove the influence of GABAergic interruptions in the neonatal brain, including possible already migrated cells, in whole hemisphere homogenate. Further studies could advance our observed data with region-specific transcription analyses in the developing brain.
Data Availability Statement
The original contributions presented in the study are included in the article/Supplementary Material, further inquiries can be directed to the corresponding author.
Ethics Statement
The animal study was reviewed and approved by LAGeSo, Berlin, Germany, approval number G-0075/18.
Author Contributions
TScheuer and SE conceived the ideas. TScheuer designed the experiments. SE, CG, and TScheuer executed the experiments. CG and SE wrote the first draft of the manuscript and approved the final draft. TScheuer and SE performed the animal studies. CG performed and analyzed immunohistological staining and qPCR. TSchmitz and CB revised the manuscript and contributed to the critical discussion. All authors have contributed to the article and approved the submitted version.
Funding
This work was supported by the Deutsche Forschungsgemeinschaft (SCHE 2078/2-1) and received no specific grant from any funding agency in the public, commercial, or not-for-profit sectors.
Conflict of Interest
The authors declare that the research was conducted in the absence of any commercial or financial relationships that could be construed as a potential conflict of interest.
Publisher’s Note
All claims expressed in this article are solely those of the authors and do not necessarily represent those of their affiliated organizations, or those of the publisher, the editors and the reviewers. Any product that may be evaluated in this article, or claim that may be made by its manufacturer, is not guaranteed or endorsed by the publisher.
Acknowledgments
Technical support and advice of Evelyn Strauß and Ruth Hermann are gratefully acknowledged.
Supplementary Material
The Supplementary Material for this article can be found online at: https://www.frontiersin.org/articles/10.3389/fncel.2021.651072/full#supplementary-material.
SUPPLEMENTARY FIGURE 1 | Quantification of (A) DAPI positive cells in sum of the DG of control animals (100% white bars), DMCM hydrochloride (DMCM) in doses of either 2 μg/kg, 10 μg/kg or 50 μg/kg, and CGP 35348 in doses of either 0.4 mg/kg, 2 mg/kg or 10 mg/kg treated rat pups at P11. Data are expressed relative to the control group as mean ± SEM of n = 10 each group. The 100% values are 594.1 cell counts (Brown-Forsythe test). Expressions of (B) AIF and Casp3 are not affected by the application of DMCM or CGP. The relative mRNA expressions of markers were measured by quantitative real-time PCR in rat brain homogenates with DMCM 50 μg/kg (gray bars) or CGP 10 mg/kg (black bars) application relative to control (white bars). Bars represent the relative mRNA quantification based on internal standard HPRT. Data shown as mean ± SEM, n = 9–10 (one-way ANOVA).
SUPPLEMENTARY FIGURE 2 | Representative hippocampal paraffin sections (A) of control animals, DMCM hydrochloride (DMCM) in dose of 50 μg/kg and CGP 35348 in dose of 10 mg/kg treated rat pups at P15 co-labeled with DAPI and NeuN. Application of GABA receptor antagonists did not affect cell counts for postmitotic NeuN+ neurons at the DG. Quantification of (B) NeuN positive cells in sum of the DG in comparison to control group (100% white bars). Data are expressed relative to the control group as mean ± SEM of n = 10 each group. The 100% values are for NeuN+ 187.3 cell counts (one-way ANOVA).
References
Altman, J., and Bayer, S. A. (1990). Migration and distribution of two populations of hippocampal granule cell precursors during the perinatal and postnatal periods. J. Comp. Neurol. 301, 365–381. doi: 10.1002/cne.903010304
Amador-Arjona, A., Cimadamore, F., Huang, C. T., Wright, R., Lewis, S., Gage, F. H., et al. (2015). SOX2 primes the epigenetic landscape in neural precursors enabling proper gene activation during hippocampal neurogenesis. Proc. Natl. Acad. Sci. U S A 112, E1936–E1945. doi: 10.1073/pnas.1421480112
Anacker, C., and Hen, R. (2017). Adult hippocampal neurogenesis and cognitive flexibility—linking memory and mood. Nat. Rev. Neurosci. 18, 335–346. doi: 10.1038/nrn.2017.45
Andersen, J., Urbán, N., Achimastou, A., Ito, A., Simic, M., Ullom, K., et al. (2014). A transcriptional mechanism integrating inputs from extracellular signals to activate hippocampal stem cells. Neuron 83, 1085–1097. doi: 10.1016/j.neuron.2014.08.004
Angulo, M. C., Le Meur, K., Kozlov, A. S., Charpak, S., and Audinat, E. (2008). GABA, a forgotten gliotransmitter. Prog. Neurobiol. 86, 297–303. doi: 10.1016/j.pneurobio.2008.08.002
Arpino, C., Compagnone, E., Montanaro, M. L., Cacciatore, D., De Luca, A., Cerulli, A., et al. (2010). Preterm birth and neurodevelopmental outcome: a review. Childs Nerv. Syst. 26, 1139–1149. doi: 10.1007/s00381-010-1125-y
Badurek, S., Griguoli, M., Asif-Malik, A., Zonta, B., Guo, F., Middei, S., et al. (2020). Immature dentate granule cells require Ntrk2/Trkb for the formation of functional hippocampal circuitry. iScience 23:101078. doi: 10.1016/j.isci.2020.101078
Basu, S. K., Pradhan, S., Du Plessis, A. J., Ben-Ari, Y., and Limperopoulos, C. (2021). GABA and glutamate in the preterm neonatal brain: in-vivo measurement by magnetic resonance spectroscopy. NeuroImage 238:118215. doi: 10.1016/j.neuroimage.2021.118215
Basu, S. K., Pradhan, S., Jacobs, M. B., Said, M., Kapse, K., Murnick, J., et al. (2020). Age and sex influences gamma-aminobutyric acid concentrations in the developing brain of very premature infants. Sci. Rep. 10:10549. doi: 10.1038/s41598-020-67188-y
Bedogni, F., Hodge, R. D., Elsen, G. E., Nelson, B. R., Daza, R. A., Beyer, R. P., et al. (2010). Tbr1 regulates regional and laminar identity of postmitotic neurons in developing neocortex. Proc. Natl. Acad. Sci. U S A 107, 13129–13134. doi: 10.1073/pnas.1002285107
Behuet, S., Cremer, J. N., Cremer, M., Palomero-Gallagher, N., Zilles, K., and Amunts, K. (2019). Developmental changes of glutamate and GABA receptor densities in wistar rats. Front. Neuroanat. 13:100. doi: 10.3389/fnana.2019.00100
Ben-Ari, Y. (2018). Oxytocin and vasopressin, and the GABA developmental shift during labor and birth: friends or foes? Front. Cell. Neurosci. 12:254. doi: 10.3389/fncel.2018.00254
Berg, D. A., Belnoue, L., Song, H., and Simon, A. (2013). Neurotransmitter-mediated control of neurogenesis in the adult vertebrate brain. Development 140, 2548–2561. doi: 10.1242/dev.088005
Berg, D. A., Bond, A. M., Ming, G. L., and Song, H. (2018). Radial glial cells in the adult dentate gyrus: what are they and where do they come from? F1000Res. 7:277. doi: 10.12688/f1000research.12684.1
Bjornsson, C. S., Apostolopoulou, M., Tian, Y., and Temple, S. (2015). It takes a village: constructing the neurogenic niche. Dev. Cell 32, 435–446. doi: 10.1016/j.devcel.2015.01.010
Brydges, C. R., Landes, J. K., Reid, C. L., Campbell, C., French, N., and Anderson, M. (2018). Cognitive outcomes in children and adolescents born very preterm: a meta-analysis. Dev. Med. Child Neurol. 60, 452–468. doi: 10.1111/dmcn.13685
Catavero, C., Bao, H., and Song, J. (2018). Neural mechanisms underlying GABAergic regulation of adult hippocampal neurogenesis. Cell Tissue Res. 371, 33–46. doi: 10.1007/s00441-017-2668-y
Cellot, G., and Cherubini, E. (2013). Functional role of ambient GABA in refining neuronal circuits early in postnatal development. Front. Neural Circuits 7:136. doi: 10.3389/fncir.2013.00136
Cohen-Cory, S., Kidane, A. H., Shirkey, N. J., and Marshak, S. (2010). Brain-derived neurotrophic factor and the development of structural neuronal connectivity. Dev. Neurobiol. 70, 271–288. doi: 10.1002/dneu.20774
Cryan, J. F., and Kaupmann, K. (2005). Don’t worry ‘B’ happy!: a role for GABA(B) receptors in anxiety and depression. Trends Pharmacol. Sci. 26, 36–43. doi: 10.1016/j.tips.2004.11.004
Danzer, S. C., and Mcnamara, J. O. (2004). Localization of brain-derived neurotrophic factor to distinct terminals of mossy fiber axons implies regulation of both excitation and feedforward inhibition of CA3 pyramidal cells. J. Neurosci. 24, 11346–11355. doi: 10.1523/JNEUROSCI.3846-04.2004
DeCarolis, N. A., Mechanic, M., Petrik, D., Carlton, A., Ables, J. L., Malhotra, S., et al. (2013). In vivo contribution of nestin- and GLAST-lineage cells to adult hippocampal neurogenesis. Hippocampus 23, 708–719. doi: 10.1002/hipo.22130
Deng, W., Aimone, J. B., and Gage, F. H. (2010). New neurons and new memories: how does adult hippocampal neurogenesis affect learning and memory? Nat. Rev. Neurosci. 11, 339–350. doi: 10.1038/nrn2822
Ding, J., He, Z., Ruan, J., Ma, Z., Liu, Y., Gong, C., et al. (2013). Role of ciliary neurotrophic factor in the proliferation and differentiation of neural stem cells. J. Alzheimers Dis. 37, 587–592. doi: 10.3233/JAD-130527
Dobbing, J., and Sands, J. (1979). Comparative aspects of the brain growth spurt. Early Hum. Dev. 3, 79–83. doi: 10.1016/0378-3782(79)90022-7
Dranovsky, A., Picchini, A. M., Moadel, T., Sisti, A. C., Yamada, A., Kimura, S., et al. (2011). Experience dictates stem cell fate in the adult hippocampus. Neuron 70, 908–923. doi: 10.1016/j.neuron.2011.05.022
Duerden, E. G., Guo, T., Dodbiba, L., Chakravarty, M. M., Chau, V., Poskitt, K. J., et al. (2016). Midazolam dose correlates with abnormal hippocampal growth and neurodevelopmental outcome in preterm infants. Ann. Neurol. 79, 548–559. doi: 10.1002/ana.24601
Endesfelder, S., Strauss, E., Bendix, I., Schmitz, T., and Buhrer, C. (2020). Prevention of oxygen-induced inflammatory lung injury by caffeine in neonatal rats. Oxid. Med. Cell. Longev. 2020:3840124. doi: 10.1155/2020/3840124
Endesfelder, S., Weichelt, U., Schiller, C., Winter, K., Von Haefen, C., and Buhrer, C. (2018). Caffeine protects against anticonvulsant-induced impaired neurogenesis in the developing rat brain. Neurotox Res. 34, 173–187. doi: 10.1007/s12640-018-9872-8
Englund, C., Fink, A., Lau, C., Pham, D., Daza, R. A., Bulfone, A., et al. (2005). Pax6, Tbr2 and Tbr1 are expressed sequentially by radial glia, intermediate progenitor cells, and postmitotic neurons in developing neocortex. J. Neurosci. 25, 247–251. doi: 10.1523/JNEUROSCI.2899-04.2005
Evenseth, L. S. M., Gabrielsen, M., and Sylte, I. (2020). The GABA(B) receptor-structure, ligand binding and drug development. Molecules 25:3093. doi: 10.3390/molecules25133093
Faigle, R., and Song, H. (2013). Signaling mechanisms regulating adult neural stem cells and neurogenesis. Biochim. Biophys. Acta 1830, 2435–2448. doi: 10.1016/j.bbagen.2012.09.002
Fatemi, S. H., Reutiman, T. J., Folsom, T. D., and Thuras, P. D. (2009). GABA(A) receptor downregulation in brains of subjects with autism. J. Autism Dev. Disord. 39, 223–230. doi: 10.1007/s10803-008-0646-7
Favaro, R., Valotta, M., Ferri, A. L. M., Latorre, E., Mariani, J., Giachino, C., et al. (2009). Hippocampal development and neural stem cell maintenance require Sox2-dependent regulation of SHH. Nat. Neurosci. 12, 1248–1256. doi: 10.1038/nn.2397
Felice, D., O’Leary, O. F., Pizzo, R. C., and Cryan, J. F. (2012). Blockade of the GABA(B) receptor increases neurogenesis in the ventral but not dorsal adult hippocampus: relevance to antidepressant action. Neuropharmacology 63, 1380–1388. doi: 10.1016/j.neuropharm.2012.06.066
Friedman, L. K., and Kahen, B. A. (2019). Chronic subconvulsive activity during early postnatal life produces autistic behavior in the absence of neurotoxicity in the juvenile weanling period. Behav. Brain Res. 374:112046. doi: 10.1016/j.bbr.2019.112046
Frielingsdorf, H., Simpson, D. R., Thal, L. J., and Pizzo, D. P. (2007). Nerve growth factor promotes survival of new neurons in the adult hippocampus. Neurobiol. Dis. 26, 47–55. doi: 10.1016/j.nbd.2006.11.015
Gaetz, W., Bloy, L., Wang, D. J., Port, R. G., Blaskey, L., Levy, S. E., et al. (2014). GABA estimation in the brains of children on the autism spectrum: measurement precision and regional cortical variation. NeuroImage 86, 1–9. doi: 10.1016/j.neuroimage.2013.05.068
Gaiarsa, J.-L., and Porcher, C. (2013). Emerging neurotrophic role of GABAB receptors in neuronal circuit development. Front. Cell. Neurosci. 7:206. doi: 10.3389/fncel.2013.00206
Gao, Z., Ure, K., Ables, J. L., Lagace, D. C., Nave, K. A., Goebbels, S., et al. (2009). Neurod1 is essential for the survival and maturation of adult-born neurons. Nat. Neurosci. 12, 1090–1092. doi: 10.1038/nn.2385
Ge, S., Goh, E. L., Sailor, K. A., Kitabatake, Y., Ming, G. L., and Song, H. (2006). GABA regulates synaptic integration of newly generated neurons in the adult brain. Nature 439, 589–593. doi: 10.1038/nature04404
Giachino, C., Barz, M., Tchorz, J. S., Tome, M., Gassmann, M., Bischofberger, J., et al. (2014). GABA suppresses neurogenesis in the adult hippocampus through GABAB receptors. Development 141, 83–90. doi: 10.1242/dev.102608
Gonçalves, J. T., Schafer, S. T., and Gage, F. H. (2016). Adult neurogenesis in the hippocampus: from stem cells to behavior. Cell 167, 897–914. doi: 10.1016/j.cell.2016.10.021
Gottmann, K., Mittmann, T., and Lessmann, V. (2009). BDNF signaling in the formation, maturation and plasticity of glutamatergic and GABAergic synapses. Exp. Brain Res. 199, 203–234. doi: 10.1007/s00221-009-1994-z
Hashemi, E., Ariza, J., Rogers, H., Noctor, S. C., and Martínez-Cerdeño, V. (2017). The number of parvalbumin-expressing interneurons is decreased in the prefrontal cortex in autism. Cereb. Cortex 27, 1931–1943. doi: 10.1093/cercor/bhw021
Heaney, C. F., and Kinney, J. W. (2016). Role of GABA(B) receptors in learning and memory and neurological disorders. Neurosci. Biobehav. Rev. 63, 1–28. doi: 10.1016/j.neubiorev.2016.01.007
Héja, L., Nyitrai, G., Kékesi, O., Dobolyi, A., Szabó, P., Fiáth, R., et al. (2012). Astrocytes convert network excitation to tonic inhibition of neurons. BMC Biol. 10:26. doi: 10.1186/1741-7007-10-26
Hevner, R. F. (2019). Intermediate progenitors and Tbr2 in cortical development. J. Anat. 235, 616–625. doi: 10.1111/joa.12939
Hodge, R. D., Kowalczyk, T. D., Wolf, S. A., Encinas, J. M., Rippey, C., Enikolopov, G., et al. (2008). Intermediate progenitors in adult hippocampal neurogenesis: Tbr2 expression and coordinate regulation of neuronal output. J. Neurosci. 28, 3707–3717. doi: 10.1523/JNEUROSCI.4280-07.2008
Hodge, R. D., Nelson, B. R., Kahoud, R. J., Yang, R., Mussar, K. E., Reiner, S. L., et al. (2012). Tbr2 is essential for hippocampal lineage progression from neural stem cells to intermediate progenitors and neurons. J. Neurosci. 32, 6275–6287. doi: 10.1523/JNEUROSCI.0532-12.2012
Hsieh, J. (2012). Orchestrating transcriptional control of adult neurogenesis. Genes Dev. 26, 1010–1021. doi: 10.1101/gad.187336.112
Isokawa, M. (2016). Caffeine-induced suppression of gabaergic inhibition and calcium-independent metaplasticity. Neural Plast. 2016:1239629. doi: 10.1155/2016/1239629
Iwano, T., Masuda, A., Kiyonari, H., Enomoto, H., and Matsuzaki, F. (2012). Prox1 postmitotically defines dentate gyrus cells by specifying granule cell identity over CA3 pyramidal cell fate in the hippocampus. Development 139, 3051–3062. doi: 10.1242/dev.080002
Kempermann, G., Jessberger, S., Steiner, B., and Kronenberg, G. (2004). Milestones of neuronal development in the adult hippocampus. Trends Neurosci. 27, 447–452. doi: 10.1016/j.tins.2004.05.013
Khirug, S., Huttu, K., Ludwig, A., Smirnov, S., Voipio, J., Rivera, C., et al. (2005). Distinct properties of functional KCC2 expression in immature mouse hippocampal neurons in culture and in acute slices. Eur. J. Neurosci. 21, 899–904. doi: 10.1111/j.1460-9568.2005.03886.x
Kim, Y. S., and Yoon, B.-E. (2017). Altered GABAergic signaling in brain disease at various stages of life. Exp. Neurobiol. 26, 122–131. doi: 10.5607/en.2017.26.3.122
Kuczewski, N., Fuchs, C., Ferrand, N., Jovanovic, J. N., Gaiarsa, J. L., and Porcher, C. (2011). Mechanism of GABAB receptor-induced BDNF secretion and promotion of GABAA receptor membrane expression. J. Neurochem. 118, 533–545. doi: 10.1111/j.1471-4159.2011.07192.x
Kuwabara, T., Hsieh, J., Muotri, A., Yeo, G., Warashina, M., Lie, D. C., et al. (2009). Wnt-mediated activation of NeuroD1 and retro-elements during adult neurogenesis. Nat. Neurosci. 12, 1097–1105. doi: 10.1038/nn.2360
Kwon, S. H., Scheinost, D., Lacadie, C., Benjamin, J., Myers, E. H., Qiu, M., et al. (2014). GABA, resting-state connectivity and the developing brain. Neonatology 106, 149–155. doi: 10.1159/000362433
Lavado, A., and Oliver, G. (2007). Prox1 expression patterns in the developing and adult murine brain. Dev. Dyn. 236, 518–524. doi: 10.1002/dvdy.21024
Lavado, A., Lagutin, O. V., Chow, L. M. L., Baker, S. J., and Oliver, G. (2010). Prox1 is required for granule cell maturation and intermediate progenitor maintenance during brain neurogenesis. PLoS Biol. 8:e1000460. doi: 10.1371/journal.pbio.1000460
Leal, G., Afonso, P. M., Salazar, I. L., and Duarte, C. B. (2015). Regulation of hippocampal synaptic plasticity by BDNF. Brain Res. 1621, 82–101. doi: 10.1016/j.brainres.2014.10.019
Lee, J., Duan, W., and Mattson, M. P. (2002). Evidence that brain-derived neurotrophic factor is required for basal neurogenesis and mediates, in part, the enhancement of neurogenesis by dietary restriction in the hippocampus of adult mice. J. Neurochem. 82, 1367–1375. doi: 10.1046/j.1471-4159.2002.01085.x
Li, G., Kataoka, H., Coughlin, S. R., and Pleasure, S. J. (2009). Identification of a transient subpial neurogenic zone in the developing dentate gyrus and its regulation by Cxcl12 and reelin signaling. Development 136, 327–335. doi: 10.1242/dev.025742
Livak, K. J., and Schmittgen, T. D. (2001). Analysis of relative gene expression data using real-time quantitative PCR and the 2−ΔΔCT method. Methods 25, 402–408. doi: 10.1006/meth.2001.1262
Lodato, M. A., Ng, C. W., Wamstad, J. A., Cheng, A. W., Thai, K. K., Fraenkel, E., et al. (2013). SOX2 co-occupies distal enhancer elements with distinct POU factors in ESCs and NPCs to specify cell state. PLoS Genet. 9:e1003288. doi: 10.1371/journal.pgen.1003288
López-Bendito, G., Shigemoto, R., Kulik, A., Vida, I., Fairén, A., and Luján, R. (2004). Distribution of metabotropic GABA receptor subunits GABAB1a/b and GABAB2 in the rat hippocampus during prenatal and postnatal development. Hippocampus 14, 836–848. doi: 10.1002/hipo.10221
Lugert, S., Basak, O., Knuckles, P., Haussler, U., Fabel, K., Götz, M., et al. (2010). Quiescent and active hippocampal neural stem cells with distinct morphologies respond selectively to physiological and pathological stimuli and aging. Cell Stem Cell 6, 445–456. doi: 10.1016/j.stem.2010.03.017
Lugert, S., Vogt, M., Tchorz, J. S., Müller, M., Giachino, C., and Taylor, V. (2012). Homeostatic neurogenesis in the adult hippocampus does not involve amplification of Ascl1(high) intermediate progenitors. Nat. Commun. 3:670. doi: 10.1038/ncomms1670
Maekawa, M., Takashima, N., Arai, Y., Nomura, T., Inokuchi, K., Yuasa, S., et al. (2005). Pax6 is required for production and maintenance of progenitor cells in postnatal hippocampal neurogenesis. Genes Cells 10, 1001–1014. doi: 10.1111/j.1365-2443.2005.00893.x
Malik, S., Vinukonda, G., Vose, L. R., Diamond, D., Bhimavarapu, B. B., Hu, F., et al. (2013). Neurogenesis continues in the third trimester of pregnancy and is suppressed by premature birth. J. Neurosci. 33, 411–423. doi: 10.1523/JNEUROSCI.4445-12.2013
Marín, O. (2012). Interneuron dysfunction in psychiatric disorders. Nat. Rev. Neurosci. 13, 107–120. doi: 10.1038/nrn3155
Mercurio, S., Serra, L., and Nicolis, S. K. (2019). More than just stem cells: functional roles of the transcription factor Sox2 in differentiated glia and neurons. Int. J. Mol. Sci. 20:4540. doi: 10.3390/ijms20184540
Miyoshi, G., Young, A., Petros, T., Karayannis, T., Mckenzie Chang, M., Lavado, A., et al. (2015). Prox1 regulates the subtype-specific development of caudal ganglionic eminence-derived GABAergic cortical interneurons. J. Neurosci. 35, 12869–12889. doi: 10.1523/JNEUROSCI.1164-15.2015
Naaijen, J., Bralten, J., Poelmans, G., Glennon, J. C., Franke, B., and Buitelaar, J. K. (2017). Glutamatergic and GABAergic gene sets in attention-deficit/hyperactivity disorder: association to overlapping traits in ADHD and autism. Transl. Psychiatry 7:e999. doi: 10.1038/tp.2016.273
Nicola, Z., Fabel, K., and Kempermann, G. (2015). Development of the adult neurogenic niche in the hippocampus of mice. Front. Neuroanat. 9:53. doi: 10.3389/fnana.2015.00053
Nuss, P. (2015). Anxiety disorders and GABA neurotransmission: a disturbance of modulation. Neuropsychiatr. Dis. Treat. 11, 165–175. doi: 10.2147/NDT.S58841
Olson, J. M., Asakura, A., Snider, L., Hawkes, R., Strand, A., Stoeck, J., et al. (2001). NeuroD2 is necessary for development and survival of central nervous system neurons. Dev. Biol. 234, 174–187. doi: 10.1006/dbio.2001.0245
Paridaen, J. T., and Huttner, W. B. (2014). Neurogenesis during development of the vertebrate central nervous system. EMBO Rep. 15, 351–364. doi: 10.1002/embr.201438447
Park, D., Xiang, A. P., Mao, F. F., Zhang, L., Di, C. G., Liu, X. M., et al. (2010). Nestin is required for the proper self-renewal of neural stem cells. Stem Cells 28, 2162–2171. doi: 10.1002/stem.541
Peerboom, C., and Wierenga, C. J. (2021). The postnatal GABA shift: a developmental perspective. Neurosci. Biobehav. Rev. 124, 179–192. doi: 10.1016/j.neubiorev.2021.01.024
Pérez-Domínguez, M., Tovar-Y-Romo, L. B., and Zepeda, A. (2018). Neuroinflammation and physical exercise as modulators of adult hippocampal neural precursor cell behavior. Rev. Neurosci. 29, 1–20. doi: 10.1515/revneuro-2017-0024
Pocock, J. M., and Kettenmann, H. (2007). Neurotransmitter receptors on microglia. Trends Neurosci. 30, 527–535. doi: 10.1016/j.tins.2007.07.007
Porcher, C., Medina, I., and Gaiarsa, J. L. (2018). Mechanism of BDNF modulation in GABAergic synaptic transmission in healthy and disease brains. Front. Cell. Neurosci. 12:273. doi: 10.3389/fncel.2018.00273
Ravanpay, A. C., Hansen, S. J., and Olson, J. M. (2010). Transcriptional inhibition of REST by NeuroD2 during neuronal differentiation. Mol. Cell. Neurosci. 44, 178–189. doi: 10.1016/j.mcn.2010.03.006
Ream, M. A., and Lehwald, L. (2018). Neurologic consequences of preterm birth. Curr. Neurol. Neurosci. Rep. 18:48. doi: 10.1007/s11910-018-0862-2
Rice, D., and Barone, S. Jr. (2000). Critical periods of vulnerability for the developing nervous system: evidence from humans and animal models. Environ. Health Perspect. 108, 511–533. doi: 10.1289/ehp.00108s3511
Richetin, K., Leclerc, C., Toni, N., Gallopin, T., Pech, S., Roybon, L., et al. (2015). Genetic manipulation of adult-born hippocampal neurons rescues memory in a mouse model of Alzheimer’s disease. Brain 138, 440–455. doi: 10.1093/brain/awu354
Rivera, C., Voipio, J., Payne, J. A., Ruusuvuori, E., Lahtinen, H., Lamsa, K., et al. (1999). The K+/Cl- co-transporter KCC2 renders GABA hyperpolarizing during neuronal maturation. Nature 397, 251–255. doi: 10.1038/16697
Rodier, P. M. (1980). Chronology of neuron development: animal studies and their clinical implications. Dev. Med. Child Neurol. 22, 525–545. doi: 10.1111/j.1469-8749.1980.tb04363.x
Rogers, C. E., Lean, R. E., Wheelock, M. D., and Smyser, C. D. (2018). Aberrant structural and functional connectivity and neurodevelopmental impairment in preterm children. J. Neurodev. Disord. 10:38. doi: 10.1186/s11689-018-9253-x
Roybon, L., Hjalt, T., Stott, S., Guillemot, F., Li, J. Y., and Brundin, P. (2009). Neurogenin2 directs granule neuroblast production and amplification while NeuroD1 specifies neuronal fate during hippocampal neurogenesis. PLoS One 4:e4779. doi: 10.1371/journal.pone.0004779
Rubin, A. N., and Kessaris, N. (2013). PROX1: a lineage tracer for cortical interneurons originating in the lateral/caudal ganglionic eminence and preoptic area. PLoS One 8:e77339. doi: 10.1371/journal.pone.0077339
Salari, A.-A., and Amani, M. (2017). Neonatal blockade of GABA-A receptors alters behavioral and physiological phenotypes in adult mice. Int. J. Dev. Neurosci. 57, 62–71. doi: 10.1016/j.ijdevneu.2017.01.007
Scardigli, R., Bäumer, N., Gruss, P., Guillemot, F., and Le Roux, I. (2003). Direct and concentration-dependent regulation of the proneural gene Neurogenin2 by Pax6. Development 130, 3269–3281. doi: 10.1242/dev.00539
Schulte, J. T., Wierenga, C. J., and Bruining, H. (2018). Chloride transporters and GABA polarity in developmental, neurological and psychiatric conditions. Neurosci. Biobehav. Rev. 90, 260–271. doi: 10.1016/j.neubiorev.2018.05.001
Semple, B. D., Blomgren, K., Gimlin, K., Ferriero, D. M., and Noble-Haeusslein, L. J. (2013). Brain development in rodents and humans: identifying benchmarks of maturation and vulnerability to injury across species. Prog. Neurobiol. 106–107, 1–16. doi: 10.1016/j.pneurobio.2013.04.001
Shaw, J. C., Palliser, H. K., Walker, D. W., and Hirst, J. J. (2015). Preterm birth affects GABAA receptor subunit mRNA levels during the foetal-to-neonatal transition in guinea pigs. J. Dev. Orig. Health Dis. 6, 250–260. doi: 10.1017/S2040174415000069
Shimazu, K., Zhao, M., Sakata, K., Akbarian, S., Bates, B., Jaenisch, R., et al. (2006). NT-3 facilitates hippocampal plasticity and learning and memory by regulating neurogenesis. Learn. Mem. 13, 307–315. doi: 10.1101/lm.76006
Shimojo, H., Ohtsuka, T., and Kageyama, R. (2008). Oscillations in notch signaling regulate maintenance of neural progenitors. Neuron 58, 52–64. doi: 10.1016/j.neuron.2008.02.014
Song, J., Olsen, R. H., Sun, J., Ming, G. L., and Song, H. (2016). Neuronal circuitry mechanisms regulating adult mammalian neurogenesis. Cold Spring Harb. Perspect. Biol. 8:a018937. doi: 10.1101/cshperspect.a018937
Song, J., Zhong, C., Bonaguidi, M. A., Sun, G. J., Hsu, D., Gu, Y., et al. (2012). Neuronal circuitry mechanism regulating adult quiescent neural stem-cell fate decision. Nature 489, 150–154. doi: 10.1038/nature11306
Stefovska, V. G., Uckermann, O., Czuczwar, M., Smitka, M., Czuczwar, P., Kis, J., et al. (2008). Sedative and anticonvulsant drugs suppress postnatal neurogenesis. Ann. Neurol. 64, 434–445. doi: 10.1002/ana.21463
Steinhorn, R., McPherson, C., Anderson, P. J., Neil, J., Doyle, L. W., and Inder, T. (2015). Neonatal morphine exposure in very preterm infants-cerebral development and outcomes. J. Pediatr. 166, 1200.e4–1207.e4. doi: 10.1016/j.jpeds.2015.02.012
Suh, H., Consiglio, A., Ray, J., Sawai, T., D’Amour, K. A., and Gage, F. H. (2007). in vivo fate analysis reveals the multipotent and self-renewal capacities of Sox2+ neural stem cells in the adult hippocampus. Cell Stem Cell 1, 515–528. doi: 10.1016/j.stem.2007.09.002
Takesian, A. E., and Hensch, T. K. (2013). Balancing plasticity/stability across brain development. Prog. Brain Res. 207, 3–34. doi: 10.1016/B978-0-444-63327-9.00001-1
Tang, X., Jaenisch, R., and Sur, M. (2021). The role of GABAergic signalling in neurodevelopmental disorders. Nat. Rev. Neurosci. 22, 290–307. doi: 10.1038/s41583-021-00443-x
Tanifuji, S., Akasaka, M., Kamei, A., Araya, N., Asami, M., Matsumoto, A., et al. (2017). Temporal brain metabolite changes in preterm infants with normal development. Brain Dev. 39, 196–202. doi: 10.1016/j.braindev.2016.10.006
Thakurela, S., Tiwari, N., Schick, S., Garding, A., Ivanek, R., Berninger, B., et al. (2016). Mapping gene regulatory circuitry of Pax6 during neurogenesis. Cell Discov. 2:15045. doi: 10.1038/celldisc.2015.45
Triviño-Paredes, J., Patten, A. R., Gil-Mohapel, J., and Christie, B. R. (2016). The effects of hormones and physical exercise on hippocampal structural plasticity. Front. Neuroendocrinol. 41, 23–43. doi: 10.1177/2048872620935399
Uda, M., Ishido, M., and Kami, K. (2007). Features and a possible role of Mash1-immunoreactive cells in the dentate gyrus of the hippocampus in the adult rat. Brain Res. 1171, 9–17. doi: 10.1016/j.brainres.2007.06.099
Vasconcelos, F. F., and Castro, D. S. (2014). Transcriptional control of vertebrate neurogenesis by the proneural factor Ascl1. Front. Cell. Neurosci. 8:412. doi: 10.3389/fncel.2014.00412
Vertkin, I., Styr, B., Slomowitz, E., Ofir, N., Shapira, I., Berner, D., et al. (2015). GABAB receptor deficiency causes failure of neuronal homeostasis in hippocampal networks. Proc. Natl. Acad. Sci. U S A 112, E3291–E3299. doi: 10.1073/pnas.1424810112
Vicario-Abejón, C., Collin, C., McKay, R. D., and Segal, M. (1998). Neurotrophins induce formation of functional excitatory and inhibitory synapses between cultured hippocampal neurons. J. Neurosci. 18, 7256–7271. doi: 10.1523/JNEUROSCI.18-18-07256.1998
Vieira, M. S., Santos, A. K., Vasconcellos, R., Goulart, V. A. M., Parreira, R. C., Kihara, A. H., et al. (2018). Neural stem cell differentiation into mature neurons: mechanisms of regulation and biotechnological applications. Biotechnol. Adv. 36, 1946–1970. doi: 10.1016/j.biotechadv.2018.08.002
Vilar, M., and Mira, H. (2016). Regulation of neurogenesis by neurotrophins during adulthood: expected and unexpected roles. Front. Neurosci. 10:26. doi: 10.3389/fnins.2016.00026
Von Bohlen und Halbach, O. (2011). Immunohistological markers for proliferative events, gliogenesis, and neurogenesis within the adult hippocampus. Cell Tissue Res. 345, 1–19. doi: 10.1007/s00441-011-1196-4
Wang, D. D., and Kriegstein, A. R. (2009). Defining the role of GABA in cortical development. J. Physiol. 587, 1873–1879. doi: 10.1113/jphysiol.2008.167635
Waterhouse, E. G., An, J. J., Orefice, L. L., Baydyuk, M., Liao, G. Y., Zheng, K., et al. (2012). BDNF promotes differentiation and maturation of adult-born neurons through GABAergic transmission. J. Neurosci. 32, 14318–14330. doi: 10.1523/JNEUROSCI.0709-12.2012
Watson, R. E., Desesso, J. M., Hurtt, M. E., and Cappon, G. D. (2006). Postnatal growth and morphological development of the brain: a species comparison. Birth Defects Res. B Dev. Reprod. Toxicol. 77, 471–484. doi: 10.1002/bdrb.20090
Wen, J., Hu, Q., Li, M., Wang, S., Zhang, L., Chen, Y., et al. (2008). Pax6 directly modulate Sox2 expression in the neural progenitor cells. Neuroreport 19, 413–417. doi: 10.1097/WNR.0b013e3282f64377
Wilhelmsson, U., Lebkuechner, I., Leke, R., Marasek, P., Yang, X., Antfolk, D., et al. (2019). Nestin regulates neurogenesis in mice through notch signaling from astrocytes to neural stem Cells. Cereb. Cortex 29, 4050–4066. doi: 10.1093/cercor/bhy284
Wilke, S. A., Hall, B. J., Antonios, J. K., Denardo, L. A., Otto, S., Yuan, B., et al. (2012). NeuroD2 regulates the development of hippocampal mossy fiber synapses. Neural Dev. 7:9. doi: 10.1186/1749-8104-7-9
Wu, C., and Sun, D. (2015). GABA receptors in brain development, function, and injury. Metab. Brain Dis. 30, 367–379. doi: 10.1007/s11011-014-9560-1
Keywords: GABA, postnatal neurogenesis, brain development, hippocampus, rat—brain
Citation: Gustorff C, Scheuer T, Schmitz T, Bührer C and Endesfelder S (2021) GABAB Receptor-Mediated Impairment of Intermediate Progenitor Maturation During Postnatal Hippocampal Neurogenesis of Newborn Rats. Front. Cell. Neurosci. 15:651072. doi: 10.3389/fncel.2021.651072
Received: 08 January 2021; Accepted: 12 July 2021;
Published: 06 August 2021.
Edited by:
Francesco Moccia, University of Pavia, ItalyReviewed by:
Rebecca Hodge, Allen Institute for Brain Science, United StatesEniko Račeková, Slovak Academy of Sciences, Slovakia
Copyright © 2021 Gustorff, Scheuer, Schmitz, Bührer and Endesfelder. This is an open-access article distributed under the terms of the Creative Commons Attribution License (CC BY). The use, distribution or reproduction in other forums is permitted, provided the original author(s) and the copyright owner(s) are credited and that the original publication in this journal is cited, in accordance with accepted academic practice. No use, distribution or reproduction is permitted which does not comply with these terms.
*Correspondence: Stefanie Endesfelder, c3RlZmFuaWUuZW5kZXNmZWxkZXJAY2hhcml0ZS5kZQ==