- Department of Biomedical Sciences, Florida State University College of Medicine, Tallahassee, FL, United States
Neutrophils are short-lived cells of the innate immune system and the first line of defense at the site of an infection and tissue injury. Pattern recognition receptors on neutrophils recognize pathogen-associated molecular patterns or danger-associated molecular patterns, which recruit them to the destined site. Neutrophils are professional phagocytes with efficient granular constituents that aid in the neutralization of pathogens. In addition to phagocytosis and degranulation, neutrophils are proficient in creating neutrophil extracellular traps (NETs) that immobilize pathogens to prevent their spread. Because of the cytotoxicity of the associated granular proteins within NETs, the microbes can be directly killed once immobilized by the NETs. The role of neutrophils in infection is well studied; however, there is less emphasis placed on the role of neutrophils in tissue injury, such as traumatic spinal cord injury. Upon the initial mechanical injury, the innate immune system is activated in response to the molecules produced by the resident cells of the injured spinal cord initiating the inflammatory cascade. This review provides an overview of the essential role of neutrophils and explores the contribution of neutrophils to the pathologic changes in the injured spinal cord.
Introduction
Innate immunity is the first line of defense against foreign agents and self-tissue injury (Buchmann, 2014; Hato and Dagher, 2015). The innate response is much faster than adaptive immunity and can be initiated immediately or within a few hours (Hato and Dagher, 2015). The innate immune response results in inflammation to control the infection or injury and signal the recruitment of relevant immune cells, which aid in clearing the pathogens and cell debris while promoting tissue healing and recovery (Newton and Dixit, 2012; Cui et al., 2014; Spiering, 2015). The components of the innate immune system that aid in its function are granulocytes, monocytes, natural killer cells, and the complement system (Spiering, 2015). Neutrophils, also known as polymorphonuclear leukocytes, are the key players of the innate immune system and the first immune cells to arrive at the site of infection and injury (Kobayashi and DeLeo, 2009; Rosales et al., 2017; Kovtun et al., 2018). In humans, neutrophils are produced at a rate of 1 × 1011 cells per day and are the most abundant granulocytes, comprising 60–70% of all blood leukocytes and have a short life span of fewer than 24 h in the bloodstream (Hong et al., 2012; Mayadas et al., 2014; McCracken and Allen, 2014; Sheshachalam et al., 2014). In mice, neutrophils are the most common granulocytes and are produced at a rate of 1 × 107 cell per day, comprising 20–30% of all blood leukocytes (O’Connell et al., 2015; Ng et al., 2019). Mature circulating neutrophils are destined for apoptosis and clearance by macrophages (Mϕ) in the liver, spleen, and bone marrow to maintain homeostasis (Savill et al., 1989; Fox et al., 2010; Hong et al., 2012; Greenlee-Wacker, 2016). This review describes the involvement of neutrophils in different pathological states with a focus on spinal cord injury (SCI).
SCI is a traumatic and detrimental condition that can result in temporary or permanent paralysis in injured patients (Kumar et al., 2018). An estimated 700,000 new SCI cases arise per year worldwide, resulting in a global incidence of 10 cases per 100,000 people (Jin et al., 2019). The vast majority of SCI cases are traumatic and caused by accidents in traffic, sports, falls, and violence (Alizadeh et al., 2019). The major phases of injury response after SCI can be categorized into the primary phase and secondary phase of injury (Ahuja et al., 2017; Alizadeh et al., 2019). Immediately after an SCI, the resulting initial mechanical damage, commonly referred to as primary injury, is characterized by a mechanical force acting on the spinal cord, resulting in immediate hemorrhage, cell death, vascular damage, ischemia, tissue disruption, edema, and the physical disruption of neurons at the site of injury (Oyinbo, 2011; Singh et al., 2012). The primary phase initiates a series of molecular changes at the tissue and cellular levels contributing to the secondary injury cascade, resulting in further permanent damage and neurological dysfunction. Secondary injury can be further divided into the acute, the subacute, and the chronic subphases (Badhiwala et al., 2018).
Inflammatory Response: A Call for Neutrophils
The first cells to be recruited to the injury site are neutrophils (Figure 1; Popovich and Jones, 2003; Oyinbo, 2011; Kubota et al., 2012; Wang et al., 2015; Guo et al., 2016). To respond to a pathogenic invasion or tissue damage, pattern recognition receptors (PRRs) on neutrophils recognize pathogen-associated molecular patterns (PAMPs) or danger-associated molecular patterns (DAMPs) (Suresh and Mosser, 2013; Amarante-Mendes et al., 2018). The PRRs activate downstream signaling pathways such as the mitogen-activated protein kinase and nuclear factor κB (NF-κB) pathways responsible for upregulating proinflammatory cytokines and chemokines (Chen and Nunez, 2010; Kigerl et al., 2014; Venereau et al., 2015; Pouwels et al., 2017; Kaur et al., 2019). PAMPs, DAMPs, and their respective receptors on neutrophils are summarized in Table 1 (Parroche et al., 2007; Mogensen, 2009; Chen and Nunez, 2010; Kumar et al., 2011; Venereau et al., 2015; Roh and Sohn, 2018; Wang, 2018).
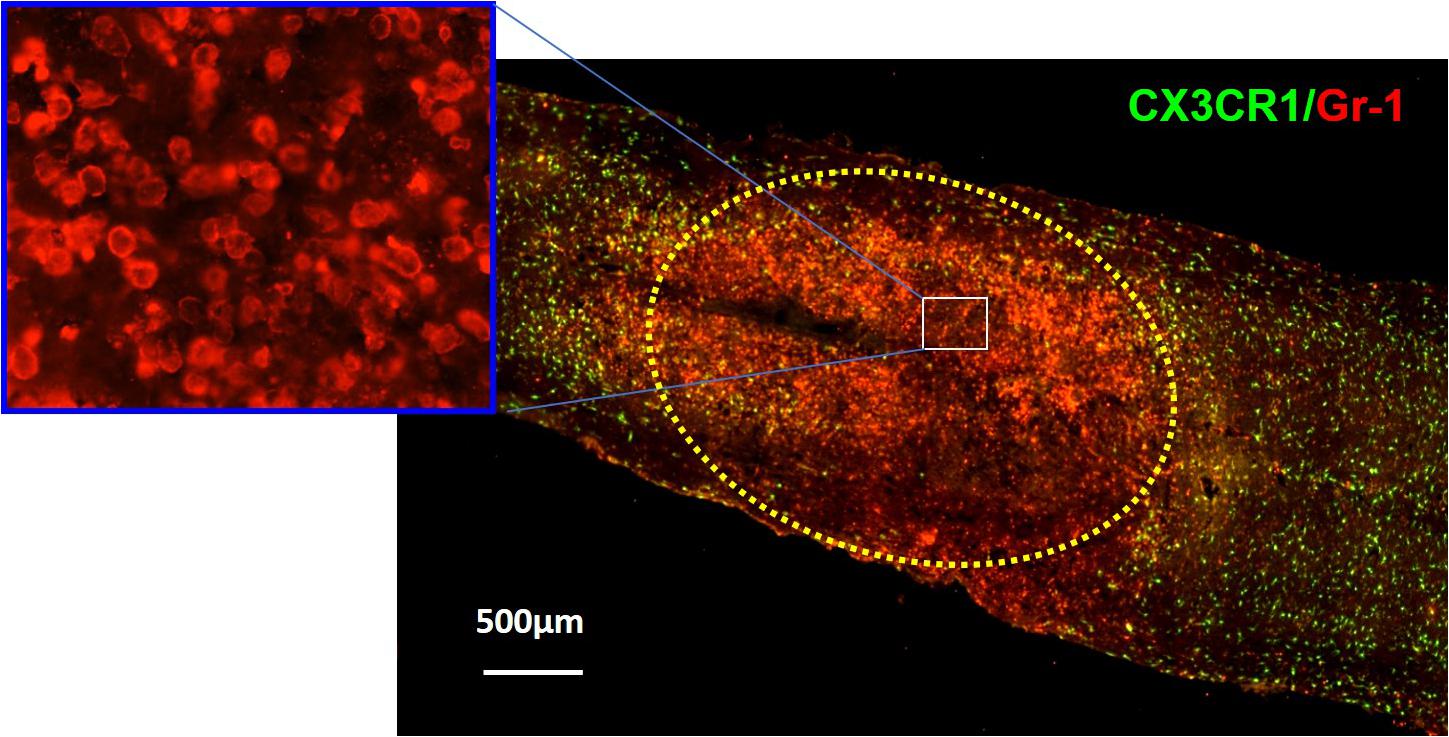
Figure 1. Immunohistochemical analysis showing neutrophils (red) in the injury site at 24 h after SCI in CX3CR1GFP/+ mice where CX3CR1 is a marker for microglia, and Gr-1 is a marker for neutrophils. The injury lesion is circled with dotted lines. Although neutrophils can be detected as early as 3 h postinjury and peak 12 h postinjury, they remain elevated up to 24 h postinjury and decrease in concentration 3 days post-SCI. Adapted from Wang et al. (2015).
Tissue damage in SCI is first detected by resident cells in the spinal cord, such as glial cells and microvascular cells, resulting in proinflammatory chemokine expression that attracts neutrophils to the injured area (Anwar et al., 2016; Alizadeh et al., 2019). The most common proinflammatory cytokines and chemokines that facilitate neutrophil recruitment include interleukin 1α (IL-1α), IL-β, IL-8, tumor necrosis factor (TNF), granulocyte colony-stimulating factor, CCL3, CXCL1, CXCL2, and CXCL5 (Kobayashi et al., 2018; Pelisch et al., 2020). IL-1β is a crucial initial proinflammatory cytokine produced after injury. Within an hour after contusion SCI in mice, astrocytes and microglia release IL-1β, which peaks in expression 12 h postinjury, correlating with the peak of infiltrated neutrophils to the injured area (Pineau and Lacroix, 2007; Saiwai et al., 2010; Kubota et al., 2012). IL-1β binds IL-1R on the cells, activating the production of proinflammatory cytokines through the NF-κB pathway (Zhang and Fuller, 2000; Albrecht et al., 2007). Astrocytes produce two important neutrophil chemoattractants, CXCL1 and CXCL2 (Pineau et al., 2010). Deletion of IL-1R in mice showed a significant reduction in infiltration of neutrophils to the injured spinal cord (Pineau et al., 2010). Administration of IL-1 receptor antagonist (IL-1RA) inhibits IL-1 signaling and suppresses neutrophil infiltration in the injured spinal cord (Yates et al., 2021). SCI mice treated with colony-stimulating factor 1 receptor antagonist PLX5622 have significantly reduced neutrophil infiltration in the injured spinal cord (Li et al., 2020). Additionally, deletion of MyD88, an adapter molecule of IL-1R and an intermediate protein in the activation of the NF-κB pathway, showed low expression of CXCL2 and no expression of CXCL1, resulting in low recruitment of neutrophils in mouse SCI (Gasse et al., 2007; Pineau et al., 2010). IKK-β is a regulatory unit of the NF-κB pathway. When bound to NF-κB molecules, it keeps this pathway inactive as it prevents NF-κB molecules from translocating into the nucleus (Karin, 1999; Rothwarf and Karin, 1999; Hacker and Karin, 2006). Similarly to the deletion of Myd88, myeloid cell–specific IKK-β–deficient mice showed decreased CXCL1 expression in the injured spinal cord and less neutrophil recruitment, strengthening the importance of these chemoattractants for the neutrophil infiltration via the NF-κB pathway (Kang et al., 2011). Moreover, neutrophil infiltration into the injured spinal cord is also attributed to the tight regulation of the receptor for the complement activation product 3a, C3aR1. In C3aR1 knockout (KO) mice, CXCL1 level increases 2 h post-SCI and remains elevated after the injury, suggesting that C3aR1 negatively regulates neutrophil mobilization by acting as the antagonist for neutrophil chemotactic signals (Brennan et al., 2019).
Major Functions of Neutrophils in the Injured Spinal Cord
The role of neutrophils at the injured spinal cord is not well understood. As mentioned earlier, upon SCI, there is physical damage to the tissue, which generates cell debris (Oyinbo, 2011; Singh et al., 2012). What is the exact role of neutrophils in response to the present debris in the injured area is yet to be elucidated. However, there are three major mechanisms by which the neutrophils generally respond to an inflammation and/or infection: degranulation, phagocytosis, and formation of neutrophil extracellular traps (NETs) (Rosales, 2020).
Degranulation
Very little is known about the role of degranulation in the pathophysiology of SCI, but a valuable lesson can be learned from its general role in other diseases. Degranulation of neutrophils is when granules directly translocate and fuse with the plasma membrane and release their contents into the extracellular space (Lacy, 2006). Upon an extracellular stimulus, secretory vesicles are mobilized and regulate circulating neutrophil transformation to an activated state (Table 2) where they then secrete their granular contents into the extracellular space (Borregaard et al., 1990; Ramadass and Catz, 2016). The purpose of degranulation into the extracellular space is to kill the extracellular enemy (Yaseen et al., 2017). Degranulation is stimulated upon ligand binding, such as IL-8, to its G-protein–coupled receptor CXCR1/2 (Barlic et al., 2000). Translocation of the granules to the neutrophil plasma membrane depends on the actin remodeling and microtubule assembly (Burgoyne and Morgan, 2003). Additionally, it requires increase in intracellular Ca2+ concentration and hydrolysis of ATP and GTP (Lacy, 2006). Fusion of granules with the plasma membrane deposits granular component cytochrome b558 onto the plasma membrane and stimulates assembly of nicotinamide adenine dinucleotide phosphate (NADPH) and reactive oxygen species (ROS) production (McLeish et al., 2013). Rab-GTPase family regulates secretion of granular contents in a time-dependent and granule-specific manner (Ramadass and Catz, 2016). Neutrophils’ primary granules contain a spectrum of serine proteases listed in Table 3, which effectively kill the pathogen (Teng et al., 2017). Although these granular contents are potent weapons to kill pathogens, they are also toxic to the tissue (Kruger et al., 2015). As infiltrated neutrophils are accumulated in the demyelinating lesion core (Figure 1), where the area for new blood vessel formation occurs, further studies are needed to know the functional consequences of neutrophil degranulation in the pathogenesis of SCI, such as destruction of myelin sheath and breakdown of the blood–spinal cord barrier (BSCB).
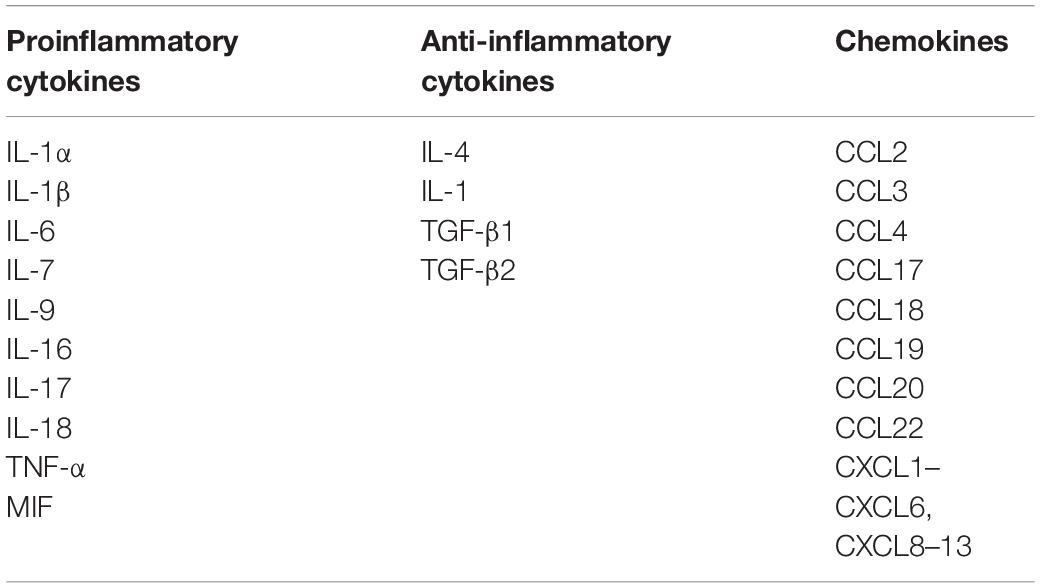
Table 2. Common cytokines and chemokines expressed constitutively or upon activation of neutrophils.
Phagocytosis of Cell Debris
Phagocytosis is a cellular process for engulfing and eliminating self or nonself particles. Particles opsonized with immunoglobulins (Ig), IgG or IgM, and complement factors are phagocytosed more effectively via Fcγ receptors and complement receptors (CRs) on neutrophils, respectively (Flannagan et al., 2009; Yu et al., 2016; Kobayashi et al., 2018). Stages of phagocytosis start with the formation of the phagosome and then continue with the maturation of the phagosome and finally with the phagolysosome formation (Flannagan et al., 2009).
Myelin is an extension of oligodendrocytes’ plasma membrane in the central nervous system (CNS) (Bunge, 1968; Hildebrand et al., 1993; Simons and Nave, 2015). This extended plasma membrane wraps around axons to create compact myelin sheaths (Demerens et al., 1996; Williamson and Lyons, 2018; Stadelmann et al., 2019). Myelin sheaths enable fast salutatory conduction of action potentials, acting as electrical insulators and allowing for impulse propagation along the axon diameter (Ford et al., 2015; Hughes and Appel, 2016; Williamson and Lyons, 2018). Myelin contains approximately 70% lipids and 30% proteins (Roots et al., 1991; Jackman et al., 2009; Fledrich et al., 2018). Proteolipid protein and myelin basic protein together make up 80% of the myelin protein by weight (Price et al., 1997; Wrathall et al., 1998; Arvanitis et al., 2002). Other important myelin proteins include myelin-associated glycoprotein, NogoA family proteins, oligodendrocyte myelin glycoprotein, and chondroitin sulfate proteoglycans, which are important for neuron regeneration and recovery after SCI (Baldwin and Giger, 2015).
Myelin debris, which is generated from the breakdown of myelin sheaths immediately after SCI, persists in the injury site and contributes to regeneration failure because it contains molecules that strongly inhibit axon regeneration and remyelination (Chen et al., 2000; Filbin, 2003; Kotter et al., 2006; Syed et al., 2016). Moreover, myelin debris is actively involved in inflammatory responses during SCI progression (Jeon et al., 2008; Sun et al., 2010; Wang et al., 2015). Therefore, clearance of myelin debris from the injury site is critical for axon regeneration, remyelination, and inflammation resolution. Infiltrating bone marrow–derived Mϕ (BMDMϕ) and resident microglia are the two major professional phagocytes for myelin debris clearance. Complement-3 receptor (CR3), Mac-2 (Glactin-3), CD36, scavenger receptor AI/II (SRAI/II), and triggering receptor expressed on myeloid cells 2 (TREM2) have been proposed as receptors for myelin debris phagocytosis by Mϕ and microglia (Kuhlmann et al., 2002; Napoli and Neumann, 2010; Sun et al., 2010; Zhou et al., 2014; Wang et al., 2015; Kopper and Gensel, 2018). The semiprofessional phagocytes, such as astrocytes and endothelial cells, can engulf myelin debris as well (Zhou et al., 2019; Konishi et al., 2020; Wang et al., 2020). We recently demonstrated that newly formed microvessels and lining microvascular endothelial cells in the injured spinal cord can engulf IgG-opsonized myelin debris (Zhou et al., 2019).
The role of neutrophils with respect to phagocytosing myelin debris post-SCI is not clear; however, engulfment of myelin debris by neutrophils has been studied in Wallerian degeneration (WD), a degeneration associated with the breakdown of the myelin sheath (Qin et al., 2012; Lindborg et al., 2017). In a mouse model of WD, deletion of neutrophils resulted in a significant lack of myelin debris clearance (Lindborg et al., 2017). Despite the results that neutrophils facilitate compensatory mechanism of clearance of myelin debris in a collaboration with clearance activity of Schwann cells in peripheral nervous system, there is no direct evidence showing that neutrophils are responsible for clearance of myelin debris, and the receptors utilized by neutrophils for myelin debris uptake are as of yet unknown (Lindborg et al., 2017). As a consequence, there are gaps in our knowledge that prevents us from understanding the full functional capacity of neutrophils in SCI in addition to their contribution to secondary tissue damage and recovery post-injury.
Neutrophil Extracellular Traps
In 2004, it was discovered that neutrophils release extracellular fibers that contained granular proteins and chromatin that trap bacteria (Brinkmann et al., 2004). Most commonly, NETs have been described as a response mechanism to kill extracellular pathogens (Brinkmann et al., 2004; Beiter et al., 2006; Ramos-Kichik et al., 2009; Papayannopoulos et al., 2010; Pilsczek et al., 2010; Juneau et al., 2011; Kaplan and Radic, 2012; Manda et al., 2014; Rosales et al., 2016). It is dependent on the size of the microbe and whether the microbe was able to avoid phagocytosis (Estua-Acosta et al., 2019). The release of NETs is also deadly to the neutrophils themselves and is thus classified as a type of cell death called NETosis, or suicidal NETs (Steinberg and Grinstein, 2007; Papayannopoulos, 2018). NETosis is stimulated by binding molecules to the receptors on the neutrophils such as Toll-like receptors (TLRs), FcRs, and CRs (Brinkmann et al., 2004; Munks et al., 2010; Garcia-Romo et al., 2011; Kaplan and Radic, 2012). Neutrophils about to undergo NETosis display distinctive morphological features that differ from their natural appearance (Fuchs et al., 2007; Vorobjeva and Pinegin, 2014; Scieszka et al., 2020).
Molecules that can stimulate NETs, referred to as sterile stimuli, include cytokines, DNA/RNA and histones, crystals, autoantibodies, and immune complexes (Keshari et al., 2012; Schorn et al., 2012; Behnen et al., 2014; Yalavarthi et al., 2015; Shrestha et al., 2019). NETs are initiated as NADPH is activated following the binding of proinflammatory cytokines, such as TNF-α and TLR-binding molecules (El-Benna et al., 2008; Stoiber et al., 2015). NADPH forms ROS, which can easily be converted to radicals spontaneously or via superoxide dismutase (Bedard and Krause, 2007; Stoiber et al., 2015). An increase in ROS stimulates the release of elastase from the membrane complex of azurophilic granules into the cytosol, activating their proteolytic activity and translocating them into the nucleus, where they aid in chromatin decondensation. Before their translocation into the nucleus, active elastase in the cytosol binds actin and degrades it. This contributes to the plasma membrane and nuclear membrane permeability and the release of NETs into the extracellular space (Metzler et al., 2014; Thiam et al., 2020). NETs are eventually degraded by DNase 1 and cleared by Mϕ (Gupta and Kaplan, 2016). The most important contents of the NETs include but are not limited to (1) granular components: elastase, lactoferrin, azurocidin, cathepsin G, myeloperoxidase (MPO), defensins, and lysozyme; (2) nuclear components: histones H2A, H2B, H3, H4, and myeloid nuclear differentiation agents; (3) cytoplasmic components: S100 calcium-binding proteins A8, A9, and A12; (4) cytoskeletal components: actin, myosin, plastin, and cytokeratin; (5) catalase peroxisome; and (6) glycolytic enzymes enolase and transketolase (Kaplan and Radic, 2012; Vorobjeva and Pinegin, 2014; Estua-Acosta et al., 2019).
There are no current NET formation reports in the injured spinal cord; however, it has been reported that infiltrated neutrophils in CNS release NETs, which may contribute to the blood–brain barrier damage and neural injury in some CNS disorders such as neurodegeneration, multiple sclerosis, traumatic brain injury (TBI), and ischemic stroke (Tillack et al., 2013; Perez-de-Puig et al., 2015; Laridan et al., 2017; Pietronigro et al., 2017; Valles et al., 2017; Ducroux et al., 2018; Farkas et al., 2019; Novotny et al., 2020; Vaibhav et al., 2020). Vaibhav et al. (2020) reported recently that NET formation worsens TBI outcomes, which is regulated by TLR4 and downstream kinase peptidylarginine deiminase 4 (PAD4). Importantly, therapeutically targeting NETs by administration of recombinant human DNase-I degrades NETs and improves neurological outcomes (Vaibhav et al., 2020). As little is known about the mechanisms of NET formation and how it interacts with other CNS resident cells in SCI, it is critical to determine to what degree this neutrophilic defense process partakes in progression of secondary injury in SCI. The research on the clear mechanistic view of NET formation could lead to identifying new targets for therapeutic interventions to treat not only SCI but also other CNS disorders.
Potentially Detrimental Roles of Infiltrating Neutrophils in SCI
In SCI, neutrophilic MPO activity can be measured within 3 h of the SCI and lasts up to 3 days postinjury. Within 1 day post-SCI, abundant neutrophils can be detected at the injury lesion (Figure 1; Wang et al., 2015). As neutrophils have not been extensively studied in SCI, not much information is available on this topic; however, there are reports regarding the general recruitment of neutrophils to an area of peripheral injury. Infiltration of neutrophils to the injury site has mainly been described as a negative event due to exposure of the injured area to the neutrophilic tissue-damaging factors. Of the four different granules in the neutrophils (Table 3), the most toxic are azurophilic, which cause tissue damage (Lacy, 2006; Nguyen et al., 2007; Kumar and Sharma, 2010). Neutrophils also secrete ROS and proteases such as metalloproteinases (MMPs), contributing to secondary tissue damage (Trivedi et al., 2006). For example, MMP9 facilitates neutrophil penetration of white and gray matter in the injured spinal cord (Fleming et al., 2006). During the first 3 days after injury, there is an increase in MMP9 and NADPH marker gp91phox, in the necrotic and apoptotic areas. Both MMP9 and NADPH contribute to the inflammatory response and secondary injury as they generate byproducts such as hydrochlorous acid (HOCl), which cause tissue damage in the surrounding area (Fleming et al., 2006).
A constituent of azurophilic granules of neutrophils is the enzyme elastase, which can create a lot of damage to the surrounding tissues (Doring, 1994; Borregaard and Cowland, 1997; Lominadze et al., 2005). Additionally, elastase can induce cell damage and dysfunction, degrade extracellular matrix proteins, and cause cell death by interfering with normal cellular pathways. Elastase is released into the surrounding tissue after neutrophils degranulate or neutrophils release NETs, resulting in inflammation (Kumar et al., 2018). In chronic inflammation, elastase is present outside neutrophils in high concentrations as elastase inhibitor, α1-proteinase inhibitor, can be easily inactivated by the proteolytic and oxidative attack, but the details of this mechanism remain unknown. In the case of SCI, in response to proinflammatory cytokines, elastase disrupts the neurovascular unit by inducing endothelial cell apoptosis and degrading endothelial cell junction proteins (Kumar et al., 2018). Inhibition of elastase by the administration of sivelestat, an inhibitor of human neutrophil elastase, after an SCI, resulted in the rescue of angiopoietin-1, a vascular growth factor responsible for anti-inflammatory effects and reduction of vessel permeability (Kumar et al., 2018).
MPO is another enzyme of the neutrophils’ azurophilic granules (Borregaard and Cowland, 1997; Lominadze et al., 2005; Kato, 2016). Following the degranulation of neutrophils, MPO is responsible for the generation of various acids, depending on the ions present in the environment, such as HOCl, to kill bacteria (Kato, 2016). MPO has been referred to as the local mediator of tissue damage and inducer of the inflammatory response (Aratani, 2018). It has been reported that MPO worsens secondary injury (Kubota et al., 2012). MPO-KO mice had less HOCl in the injured area, more intact myelin in the core of the injury, and an overall decrease in the production of proinflammatory cytokines such as IL-6, IL-1β, and TNF-α, all of which are contributors to secondary injury of SCI (Hausmann, 2003; Kubota et al., 2012). Locomotor function of MPO-KO mice was significantly better than that of wild-type (WT) mice, indicating an overall increase in functional recovery of MPO-KO injured mice (Kubota et al., 2012). We previously demonstrated that Mϕ that have taken up myelin debris have decreased phagocytic capacity for apoptotic neutrophils (Wang et al., 2015). Nonengulfed apoptotic neutrophils undergo secondary necrosis and release MPO and elastase, which might be involved in inflammation and secondary injury after SCI (Smith, 1999; Wang et al., 2015).
Leukotriene B4 (LB4) is a proinflammatory moderator that induces recruitment of neutrophils through LTB4 receptor 1 (BLT1) on the neutrophils (Crooks and Stockley, 1998). LB4 is produced from arachidonic acid and activated in the lesion area of SCI (Xu et al., 1990). It has been shown that LB4 is involved in the tissue damage caused by neutrophils (Crooks and Stockley, 1998). Neutrophil infiltration in BLT1-KO mice was significantly less than that in WT mice (Saiwai et al., 2010). Coculture of neural cells with neutrophils isolated from the lesion area showed a significant increase in neural cell death compared to neural cells cocultured with circulating neutrophils suggesting that the toxicity present in the lesion area is possibly because of infiltrated neutrophils (Saiwai et al., 2010).
In the earlier section, we discuss neutrophils’ ability to form NETs in response to inflammation and/or infection. Because of the nature of the components that make up the NETs, there is a great potential for tissue damage in this neutrophilic response (Villanueva et al., 2011; Saffarzadeh et al., 2012; Carmona-Rivera et al., 2015; Sorensen and Borregaard, 2016; Brinkmann, 2018). As infiltrated neutrophils are accumulated in lesion core (Figure 1), where the demyelinating area for new blood vessel formation is located, it is likely that NET formation and NETosis may be involved in the pathogenesis of SCI such as destruction of myelin sheath and BSCB breakdown if NETs were to be detected in SCI.
Potentially Beneficial Roles of Infiltrating Neutrophils in SCI
Although recruitment of neutrophils is thought of as damaging for the injured tissue, there is also a positive aspect of that recruitment. As first responders to the injured site, they can initiate clearance of debris and produce proinflammatory signals that recruit other immune cells such as Mϕ to eliminate leftover debris and contribute to tissue healing (Trivedi et al., 2006). Defective neutrophil recruitment to the injury site, mediated by the absence in the expression of esophageal cancer–related gene 4 (ECRG4) causing suppression of CD44, shows impaired wound healing process (Dorschner et al., 2020). A study reported that depletion of Ly6G/Gr-1 neutrophils in SCI mice resulted in the abolition of neutrophil infiltration into the injured spinal cord, lack of appropriate proinflammatory response, and impairment of injury recovery (Stirling et al., 2009).
Neutrophils are a heterogeneous cell population essential for immune defense versatile in their defense mechanisms. Heterogeneity of neutrophils is defined by the maturity of the cells, activation state, and discrete subsets such as low-density neutrophils, immunomodulatory neutrophils, and neutrophils expressing surface maker CD177 (Grieshaber-Bouyer and Nigrovic, 2019; Ng et al., 2019). A recent study reports a new subset of neutrophils with axon regenerative properties (Sas et al., 2020). This subset is characterized as CD14+Ly6Glo granulocytes resembling immature neutrophils. In SCI, this new subset promoted axonal regrowth, passing beyond the injury site and exhibiting neuroprotective and proregenerative functions (Sas et al., 2020). Therefore, heterogeneity of neutrophils offers new therapeutic opportunities.
The secretory leukocyte protease inhibitor (SLPI) is a serine protease inhibitor and a member of the innate immune system with an anti-inflammatory role (Doumas et al., 2005). It protects the tissue from damage by forming protease–antiprotease complexes (Ying et al., 1994). SLPI is expressed by neutrophils and is an important factor in SCI recovery and the improvement of locomotor activity by pausing further degradation of tissue matrix and thereby preventing further secondary damage (Ghasemlou et al., 2010). Recombinant SLPI treatment in SCI mice showed a reduced lesion size in the epicenter of the injury and reduced myelin loss, indicating a lesser degree of secondary injury (Ghasemlou et al., 2010). Overexpression of SLPI inhibited proinflammatory signals and significantly improved locomotor activity (Ghasemlou et al., 2010; Svensson et al., 2017).
Conclusion
Although we started to understand the events in secondary injury better, we have yet to uncover the early contributors of secondary injury and why secondary injury has such irreversible consequences. Neutrophils are the first immune cells to infiltrate the injured spinal cord (Popovich and Jones, 2003; Oyinbo, 2011; Kubota et al., 2012; Guo et al., 2016). The role of neutrophils in innate immunity has been well studied, their response to the pathogenic invasion is well characterized, and their infiltration into the injured tissue has been thoroughly reported. Nevertheless, their role in SCI and their contribution to the secondary injury cascade in SCI are not well understood. The roles of neutrophils in SCI may be context-dependent. We understand that neutrophils are important in propagation of the inflammatory response and recruitment of other immune cells, such as BMDMϕ to the injured area for clearance of damaged cells and cellular debris (Prame Kumar et al., 2018). However, it remains unclear what are the other cellular functions of neutrophils in the injured area. Understanding the role of neutrophils at the different stages of SCI could lead to major advances in determining the timeline of the irreversible and detrimental changes following an SCI and allow us to pinpoint the best therapeutic target.
Author Contributions
SZ contributed to data collection, critical analysis of the literature, and writing the manuscript. MA and GH contributed to the discussion. YR contributed to the conception, design, critical analysis of the literature, and writing the manuscript. All authors contributed to the article and approved the submitted version.
Funding
This work was supported by the National Science Foundation (DMS-1661727) to YR.
Conflict of Interest
The authors declare that the research was conducted in the absence of any commercial or financial relationships that could be construed as a potential conflict of interest.
References
Ahuja, C. S., Wilson, J. R., Nori, S., Kotter, M. R. N., Druschel, C., Curt, A., et al. (2017). Traumatic spinal cord injury. Nat. Rev. Dis. Primers 3:17018. doi: 10.1038/nrdp.2017.18
Albrecht, U., Yang, X., Asselta, R., Keitel, V., Tenchini, M. L., Ludwig, S., et al. (2007). Activation of NF-kappaB by IL-1beta blocks IL-6-induced sustained STAT3 activation and STAT3-dependent gene expression of the human gamma-fibrinogen gene. Cell Signal 19, 1866–1878. doi: 10.1016/j.cellsig.2007.04.007
Alizadeh, A., Dyck, S. M., and Karimi-Abdolrezaee, S. (2019). Traumatic spinal cord injury: an overview of pathophysiology, models and acute injury mechanisms. Front. Neurol. 10:282. doi: 10.3389/fneur.2019.00282
Amarante-Mendes, G. P., Adjemian, S., Branco, L. M., Zanetti, L. C., Weinlich, R., and Bortoluci, K. R. (2018). Pattern recognition receptors and the host cell death molecular machinery. Front. Immunol. 9:2379. doi: 10.3389/fimmu.2018.02379
Anwar, M. A., Al Shehabi, T. S., and Eid, A. H. (2016). Inflammogenesis of secondary spinal cord injury. Front. Cell Neurosci. 10:98. doi: 10.3389/fncel.2016.00098
Aratani, Y. (2018). Myeloperoxidase: its role for host defense, inflammation, and neutrophil function. Arch. Biochem. Biophys. 640, 47–52. doi: 10.1016/j.abb.2018.01.004
Arvanitis, D. N., Yang, W., and Boggs, J. M. (2002). Myelin proteolipid protein, basic protein, the small isoform of myelin-associated glycoprotein, and p42MAPK are associated in the Triton X-100 extract of central nervous system myelin. J. Neurosci. Res. 70, 8–23. doi: 10.1002/jnr.10383
Badhiwala, J. H., Ahuja, C. S., and Fehlings, M. G. (2018). Time is spine: a review of translational advances in spinal cord injury. J. Neurosurg. Spine 30, 1–18. doi: 10.3171/2018.9.SPINE18682
Baldwin, K. T., and Giger, R. J. (2015). Insights into the physiological role of CNS regeneration inhibitors. Front. Mol. Neurosci. 8:23. doi: 10.3389/fnmol.2015.00023
Barlic, J., Andrews, J. D., Kelvin, A. A., Bosinger, S. E., DeVries, M. E., Xu, L., et al. (2000). Regulation of tyrosine kinase activation and granule release through beta-arrestin by CXCRI. Nat. Immunol. 1, 227–233. doi: 10.1038/79767
Bedard, K., and Krause, K. H. (2007). The NOX family of ROS-generating NADPH oxidases: physiology and pathophysiology. Physiol. Rev. 87, 245–313. doi: 10.1152/physrev.00044.2005
Behnen, M., Leschczyk, C., Moller, S., Batel, T., Klinger, M., Solbach, W., et al. (2014). Immobilized immune complexes induce neutrophil extracellular trap release by human neutrophil granulocytes via FcgammaRIIIB and Mac-1. J. Immunol. 193, 1954–1965. doi: 10.4049/jimmunol.1400478
Beiter, K., Wartha, F., Albiger, B., Normark, S., Zychlinsky, A., and Henriques-Normark, B. (2006). An endonuclease allows Streptococcus pneumoniae to escape from neutrophil extracellular traps. Curr. Biol. 16, 401–407. doi: 10.1016/j.cub.2006.01.056
Borregaard, N., Christensen, L., Bejerrum, O. W., Birgens, H. S., and Clemmensen, I. (1990). Identification of a highly mobilizable subset of human neutrophil intracellular vesicles that contains tetranectin and latent alkaline phosphatase. J. Clin. Invest. 85, 408–416. doi: 10.1172/JCI114453
Borregaard, N., and Cowland, J. B. (1997). Granules of the human neutrophilic polymorphonuclear leukocyte. Blood 89, 3503–3521.
Brennan, F. H., Jogia, T., Gillespie, E. R., Blomster, L. V., Li, X. X., Nowlan, B., et al. (2019). Complement receptor C3aR1 controls neutrophil mobilization following spinal cord injury through physiological antagonism of CXCR2. JCI Insight 4, e98254. doi: 10.1172/jci.insight.98254
Brinkmann, V. (2018). Neutrophil extracellular traps in the second decade. J. Innate Immun. 10, 414–421. doi: 10.1159/000489829
Brinkmann, V., Reichard, U., Goosmann, C., Fauler, B., Uhlemann, Y., Weiss, D. S., et al. (2004). Neutrophil extracellular traps kill bacteria. Science 303, 1532–1535. doi: 10.1126/science.1092385
Buchmann, K. (2014). Evolution of innate immunity: clues from invertebrates via fish to mammals. Front. Immunol. 5:459. doi: 10.3389/fimmu.2014.00459
Bunge, R. P. (1968). Glial cells and the central myelin sheath. Physiol. Rev. 48, 197–251. doi: 10.1152/physrev.1968.48.1.197
Burgoyne, R. D., and Morgan, A. (2003). Secretory granule exocytosis. Physiol. Rev. 83, 581–632. doi: 10.1152/physrev.00031.2002
Carmona-Rivera, C., Zhao, W., Yalavarthi, S., and Kaplan, M. J. (2015). Neutrophil extracellular traps induce endothelial dysfunction in systemic lupus erythematosus through the activation of matrix metalloproteinase-2. Ann. Rheum. Dis. 74, 1417–1424. doi: 10.1136/annrheumdis-2013-204837
Chen, G. Y., and Nunez, G. (2010). Sterile inflammation: sensing and reacting to damage. Nat. Rev. Immunol. 10, 826–837. doi: 10.1038/nri2873
Chen, M. S., Huber, A. B., van der Haar, M. E., Frank, M., Schnell, L., Spillmann, A. A., et al. (2000). Nogo-A is a myelin-associated neurite outgrowth inhibitor and an antigen for monoclonal antibody IN-1. Nature 403, 434–439. doi: 10.1038/35000219
Crooks, S. W., and Stockley, R. A. (1998). Leukotriene B4. Int. J. Biochem. Cell Biol. 30, 173–178. doi: 10.1016/s1357-2725(97)00123-4
Cui, J., Chen, Y., Wang, H. Y., and Wang, R. F. (2014). Mechanisms and pathways of innate immune activation and regulation in health and cancer. Hum. Vaccin. Immunother. 10, 3270–3285. doi: 10.4161/21645515.2014.979640
Demerens, C., Stankoff, B., Logak, M., Anglade, P., Allinquant, B., Couraud, F., et al. (1996). Induction of myelination in the central nervous system by electrical activity. Proc. Natl. Acad. Sci. U.S.A. 93, 9887–9892. doi: 10.1073/pnas.93.18.9887
Doring, G. (1994). The role of neutrophil elastase in chronic inflammation. Am. J. Respir. Crit. Care Med. 150(6 Pt 2), S114–S117. doi: 10.1164/ajrccm/150.6_Pt_2.S114
Dorschner, R. A., Lee, J., Cohen, O., Costantini, T., Baird, A., and Eliceiri, B. P. (2020). ECRG4 regulates neutrophil recruitment and CD44 expression during the inflammatory response to injury. Sci. Adv. 6:eaay0518. doi: 10.1126/sciadv.aay0518
Doumas, S., Kolokotronis, A., and Stefanopoulos, P. (2005). Anti-inflammatory and antimicrobial roles of secretory leukocyte protease inhibitor. Infect. Immun. 73, 1271–1274. doi: 10.1128/IAI.73.3.1271-1274.2005
Ducroux, C., Di Meglio, L., Loyau, S., Delbosc, S., Boisseau, W., Deschildre, C., et al. (2018). Thrombus neutrophil extracellular traps content impair tpa-induced thrombolysis in acute ischemic stroke. Stroke 49, 754–757. doi: 10.1161/STROKEAHA.117.019896
El-Benna, J., Dang, P. M., and Gougerot-Pocidalo, M. A. (2008). Priming of the neutrophil NADPH oxidase activation: role of p47phox phosphorylation and NOX2 mobilization to the plasma membrane. Semin. Immunopathol. 30, 279–289. doi: 10.1007/s00281-008-0118-3
Estua-Acosta, G. A., Zamora-Ortiz, R., Buentello-Volante, B., Garcia-Mejia, M., and Garfias, Y. (2019). Neutrophil extracellular traps: current perspectives in the eye. Cells 8:979. doi: 10.3390/cells8090979
Farkas, A. Z., Farkas, V. J., Gubucz, I., Szabo, L., Balint, K., Tenekedjiev, K., et al. (2019). Neutrophil extracellular traps in thrombi retrieved during interventional treatment of ischemic arterial diseases. Thromb. Res. 175, 46–52. doi: 10.1016/j.thromres.2019.01.006
Filbin, M. T. (2003). Myelin-associated inhibitors of axonal regeneration in the adult mammalian CNS. Nat. Rev. Neurosci. 4, 703–713. doi: 10.1038/nrn1195
Flannagan, R. S., Cosio, G., and Grinstein, S. (2009). Antimicrobial mechanisms of phagocytes and bacterial evasion strategies. Nat. Rev. Microbiol. 7, 355–366. doi: 10.1038/nrmicro2128
Fledrich, R., Abdelaal, T., Rasch, L., Bansal, V., Schutza, V., Brugger, B., et al. (2018). Targeting myelin lipid metabolism as a potential therapeutic strategy in a model of CMT1A neuropathy. Nat. Commun. 9:3025. doi: 10.1038/s41467-018-05420-0
Fleming, J. C., Norenberg, M. D., Ramsay, D. A., Dekaban, G. A., Marcillo, A. E., Saenz, A. D., et al. (2006). The cellular inflammatory response in human spinal cords after injury. Brain 129(Pt 12), 3249–3269. doi: 10.1093/brain/awl296
Ford, M. C., Alexandrova, O., Cossell, L., Stange-Marten, A., Sinclair, J., Kopp-Scheinpflug, C., et al. (2015). Tuning of Ranvier node and internode properties in myelinated axons to adjust action potential timing. Nat. Commun. 6:8073. doi: 10.1038/ncomms9073
Fox, S., Leitch, A. E., Duffin, R., Haslett, C., and Rossi, A. G. (2010). Neutrophil apoptosis: relevance to the innate immune response and inflammatory disease. J. Innate Immun. 2, 216–227. doi: 10.1159/000284367
Fuchs, T. A., Abed, U., Goosmann, C., Hurwitz, R., Schulze, I., Wahn, V., et al. (2007). Novel cell death program leads to neutrophil extracellular traps. J. Cell Biol. 176, 231–241. doi: 10.1083/jcb.200606027
Garcia-Romo, G. S., Caielli, S., Vega, B., Connolly, J., Allantaz, F., Xu, Z., et al. (2011). Netting neutrophils are major inducers of type I IFN production in pediatric systemic lupus erythematosus. Sci. Transl. Med. 3:73ra20. doi: 10.1126/scitranslmed.3001201
Gasse, P., Mary, C., Guenon, I., Noulin, N., Charron, S., Schnyder-Candrian, S., et al. (2007). IL-1R1/MyD88 signaling and the inflammasome are essential in pulmonary inflammation and fibrosis in mice. J. Clin. Invest. 117, 3786–3799. doi: 10.1172/JCI32285
Ghasemlou, N., Bouhy, D., Yang, J., Lopez-Vales, R., Haber, M., Thuraisingam, T., et al. (2010). Beneficial effects of secretory leukocyte protease inhibitor after spinal cord injury. Brain 133(Pt 1), 126–138. doi: 10.1093/brain/awp304
Greenlee-Wacker, M. C. (2016). Clearance of apoptotic neutrophils and resolution of inflammation. Immunol. Rev. 273, 357–370. doi: 10.1111/imr.12453
Grieshaber-Bouyer, R., and Nigrovic, P. A. (2019). Neutrophil heterogeneity as therapeutic opportunity in immune-mediated disease. Front. Immunol. 10:346. doi: 10.3389/fimmu.2019.00346
Guo, L., Rolfe, A. J., Wang, X., Tai, W., Cheng, Z., Cao, K., et al. (2016). Rescuing macrophage normal function in spinal cord injury with embryonic stem cell conditioned media. Mol. Brain 9:48. doi: 10.1186/s13041-016-0233-3
Gupta, S., and Kaplan, M. J. (2016). The role of neutrophils and NETosis in autoimmune and renal diseases. Nat. Rev. Nephrol. 12, 402–413. doi: 10.1038/nrneph.2016.71
Hacker, H., and Karin, M. (2006). Regulation and function of IKK and IKK-related kinases. Sci. STKE 2006:re13. doi: 10.1126/stke.3572006re13
Hato, T., and Dagher, P. C. (2015). How the innate immune system senses trouble and causes trouble. Clin. J. Am. Soc. Nephrol. 10, 1459–1469. doi: 10.2215/CJN.04680514
Hausmann, O. N. (2003). Post-traumatic inflammation following spinal cord injury. Spinal Cord 41, 369–378. doi: 10.1038/sj.sc.3101483
Hildebrand, C., Remahl, S., Persson, H., and Bjartmar, C. (1993). Myelinated nerve fibres in the CNS. Prog. Neurobiol. 40, 319–384. doi: 10.1016/0301-0082(93)90015-k
Hong, C., Kidani, Y., A-Gonzalez, N., Phung, T., Ito, A., Rong, X., et al. (2012). Coordinate regulation of neutrophil homeostasis by liver X receptors in mice. J. Clin. Invest. 122, 337–347. doi: 10.1172/JCI58393
Hughes, E. G., and Appel, B. (2016). The cell biology of CNS myelination. Curr. Opin. Neurobiol. 39, 93–100. doi: 10.1016/j.conb.2016.04.013
Jackman, N., Ishii, A., and Bansal, R. (2009). Oligodendrocyte development and myelin biogenesis: parsing out the roles of glycosphingolipids. Physiology (Bethesda) 24, 290–297. doi: 10.1152/physiol.00016.2009
Jeon, S. B., Yoon, H. J., Park, S. H. I, Kim, H., and Park, E. J. (2008). Sulfatide, a major lipid component of myelin sheath, activates inflammatory responses as an endogenous stimulator in brain-resident immune cells. J. Immunol. 181, 8077–8087. doi: 10.4049/jimmunol.181.11.8077
Jin, M. C., Medress, Z. A., Azad, T. D., Doulames, V. M., and Veeravagu, A. (2019). Stem cell therapies for acute spinal cord injury in humans: a review. Neurosurg. Focus 46, E10. doi: 10.3171/2018.12.FOCUS18602
Juneau, R. A., Pang, B., Weimer, K. E., Armbruster, C. E., and Swords, W. E. (2011). Nontypeable Haemophilus influenzae initiates formation of neutrophil extracellular traps. Infect. Immun. 79, 431–438. doi: 10.1128/IAI.00660-10
Kang, J., Jiang, M. H., Min, H. J., Jo, E. K., Lee, S., Karin, M., et al. (2011). IKK-beta-mediated myeloid cell activation exacerbates inflammation and inhibits recovery after spinal cord injury. Eur. J. Immunol. 41, 1266–1277. doi: 10.1002/eji.201040582
Kaplan, M. J., and Radic, M. (2012). Neutrophil extracellular traps: double-edged swords of innate immunity. J. Immunol. 189, 2689–2695. doi: 10.4049/jimmunol.1201719
Karin, M. (1999). How NF-kappaB is activated: the role of the IkappaB kinase (IKK) complex. Oncogene 18, 6867–6874. doi: 10.1038/sj.onc.1203219
Kato, Y. (2016). Neutrophil myeloperoxidase and its substrates: formation of specific markers and reactive compounds during inflammation. J. Clin. Biochem. Nutr. 58, 99–104. doi: 10.3164/jcbn.15-104
Kaur, D., Patiyal, S., Sharma, N., Usmani, S. S., and Raghava, G. P. S. (2019). PRRDB 2.0: a comprehensive database of pattern-recognition receptors and their ligands. Database (Oxford) 2019, baz076. doi: 10.1093/database/baz076
Keshari, R. S., Jyoti, A., Dubey, M., Kothari, N., Kohli, M., Bogra, J., et al. (2012). Cytokines induced neutrophil extracellular traps formation: implication for the inflammatory disease condition. PLoS One 7:e48111. doi: 10.1371/journal.pone.0048111
Kigerl, K. A., de Rivero Vaccari, J. P., Dietrich, W. D., Popovich, P. G., and Keane, R. W. (2014). Pattern recognition receptors and central nervous system repair. Exp. Neurol. 258, 5–16. doi: 10.1016/j.expneurol.2014.01.001
Kobayashi, S. D., and DeLeo, F. R. (2009). Role of neutrophils in innate immunity: a systems biology-level approach. Wiley Interdiscip. Rev. Syst. Biol. Med. 1, 309–333. doi: 10.1002/wsbm.32
Kobayashi, S. D., Malachowa, N., and DeLeo, F. R. (2018). Neutrophils and bacterial immune evasion. J. Innate Immun. 10, 432–441. doi: 10.1159/000487756
Konishi, H., Okamoto, T., Hara, Y., Komine, O., Tamada, H., Maeda, M., et al. (2020). Astrocytic phagocytosis is a compensatory mechanism for microglial dysfunction. EMBO J. 39, e104464. doi: 10.15252/embj.2020104464
Kopper, T. J., and Gensel, J. C. (2018). Myelin as an inflammatory mediator: myelin interactions with complement, macrophages, and microglia in spinal cord injury. J. Neurosci. Res. 96, 969–977. doi: 10.1002/jnr.24114
Kotter, M. R., Li, W. W., Zhao, C., and Franklin, R. J. (2006). Myelin impairs CNS remyelination by inhibiting oligodendrocyte precursor cell differentiation. J. Neurosci. 26, 328–332. doi: 10.1523/JNEUROSCI.2615-05.2006
Kovtun, A., Messerer, D. A. C., Scharffetter-Kochanek, K., Huber-Lang, M., and Ignatius, A. (2018). Neutrophils in tissue trauma of the skin, bone, and lung: two sides of the same coin. J. Immunol. Res. 2018:8173983. doi: 10.1155/2018/8173983
Kruger, P., Saffarzadeh, M., Weber, A. N., Rieber, N., Radsak, M., von Bernuth, H., et al. (2015). Neutrophils: between host defence, immune modulation, and tissue injury. PLoS Pathog. 11:e1004651. doi: 10.1371/journal.ppat.1004651
Kubota, K., Saiwai, H., Kumamaru, H., Maeda, T., Ohkawa, Y., Aratani, Y., et al. (2012). Myeloperoxidase exacerbates secondary injury by generating highly reactive oxygen species and mediating neutrophil recruitment in experimental spinal cord injury. Spine (Phila Pa 1976) 37, 1363–1369. doi: 10.1097/BRS.0b013e31824b9e77
Kuhlmann, T., Wendling, U., Nolte, C., Zipp, F., Maruschak, B., Stadelmann, C., et al. (2002). Differential regulation of myelin phagocytosis by macrophages/microglia, involvement of target myelin, Fc receptors and activation by intravenous immunoglobulins. J. Neurosci. Res. 67, 185–190. doi: 10.1002/jnr.10104
Kumar, H., Choi, H., Jo, M. J., Joshi, H. P., Muttigi, M., Bonanomi, D., et al. (2018). Neutrophil elastase inhibition effectively rescued angiopoietin-1 decrease and inhibits glial scar after spinal cord injury. Acta Neuropathol. Commun. 6:73. doi: 10.1186/s40478-018-0576-3
Kumar, H., Kawai, T., and Akira, S. (2011). Pathogen recognition by the innate immune system. Int. Rev. Immunol. 30, 16–34. doi: 10.3109/08830185.2010.529976
Kumar, V., and Sharma, A. (2010). Neutrophils: cinderella of innate immune system. Int. Immunopharmacol. 10, 1325–1334. doi: 10.1016/j.intimp.2010.08.012
Lacy, P. (2006). Mechanisms of degranulation in neutrophils. Allergy Asthma Clin. Immunol. 2, 98–108. doi: 10.1186/1710-1492-2-3-98
Laridan, E., Denorme, F., Desender, L., Francois, O., Andersson, T., Deckmyn, H., et al. (2017). Neutrophil extracellular traps in ischemic stroke thrombi. Ann. Neurol. 82, 223–232. doi: 10.1002/ana.24993
Li, Y., Ritzel, R. M., Khan, N., Cao, T., He, J., Lei, Z., et al. (2020). Delayed microglial depletion after spinal cord injury reduces chronic inflammation and neurodegeneration in the brain and improves neurological recovery in male mice. Theranostics 10, 11376–11403. doi: 10.7150/thno.49199
Lindborg, J. A., Mack, M., and Zigmond, R. E. (2017). Neutrophils are critical for myelin removal in a peripheral nerve injury model of wallerian degeneration. J. Neurosci. 37, 10258–10277. doi: 10.1523/JNEUROSCI.2085-17.2017
Lominadze, G., Powell, D. W., Luerman, G. C., Link, A. J., Ward, R. A., and McLeish, K. R. (2005). Proteomic analysis of human neutrophil granules. Mol. Cell Proteomics 4, 1503–1521. doi: 10.1074/mcp.M500143-MCP200
Manda, A., Pruchniak, M. P., Arazna, M., and Demkow, U. A. (2014). Neutrophil extracellular traps in physiology and pathology. Cent. Eur. J. Immunol. 39, 116–121. doi: 10.5114/ceji.2014.42136
Mayadas, T. N., Cullere, X., and Lowell, C. A. (2014). The multifaceted functions of neutrophils. Annu. Rev. Pathol. 9, 181–218. doi: 10.1146/annurev-pathol-020712-164023
McCracken, J. M., and Allen, L. A. (2014). Regulation of human neutrophil apoptosis and lifespan in health and disease. J. Cell Death 7, 15–23. doi: 10.4137/JCD.S11038
McLeish, K. R., Uriarte, S. M., Tandon, S., Creed, T. M., Le, J., and Ward, R. A. (2013). Exocytosis of neutrophil granule subsets and activation of prolyl isomerase 1 are required for respiratory burst priming. J. Innate Immun. 5, 277–289. doi: 10.1159/000345992
Metzler, K. D., Goosmann, C., Lubojemska, A., Zychlinsky, A., and Papayannopoulos, V. (2014). A myeloperoxidase-containing complex regulates neutrophil elastase release and actin dynamics during NETosis. Cell Rep. 8, 883–896. doi: 10.1016/j.celrep.2014.06.044
Mogensen, T. H. (2009). Pathogen recognition and inflammatory signaling in innate immune defenses. Clin. Microbiol. Rev. 22, 240–273. doi: 10.1128/CMR.00046-08
Munks, M. W., McKee, A. S., Macleod, M. K., Powell, R. L., Degen, J. L., Reisdorph, N. A., et al. (2010). Aluminum adjuvants elicit fibrin-dependent extracellular traps in vivo. Blood 116, 5191–5199. doi: 10.1182/blood-2010-03-275529
Napoli, I., and Neumann, H. (2010). Protective effects of microglia in multiple sclerosis. Exp. Neurol. 225, 24–28. doi: 10.1016/j.expneurol.2009.04.024
Newton, K., and Dixit, V. M. (2012). Signaling in innate immunity and inflammation. Cold Spring Harb. Perspect. Biol. 4:a006049. doi: 10.1101/cshperspect.a006049
Ng, L. G., Ostuni, R., and Hidalgo, A. (2019). Heterogeneity of neutrophils. Nat. Rev. Immunol. 19, 255–265. doi: 10.1038/s41577-019-0141-8
Nguyen, H. X., O’Barr, T. J., and Anderson, A. J. (2007). Polymorphonuclear leukocytes promote neurotoxicity through release of matrix metalloproteinases, reactive oxygen species, and TNF-alpha. J. Neurochem. 102, 900–912. doi: 10.1111/j.1471-4159.2007.04643.x
Novotny, J., Oberdieck, P., Titova, A., Pelisek, J., Chandraratne, S., Nicol, P., et al. (2020). Thrombus NET content is associated with clinical outcome in stroke and myocardial infarction. Neurology 94, e2346–e2360. doi: 10.1212/WNL.0000000000009532
O’Connell, K. E., Mikkola, A. M., Stepanek, A. M., Vernet, A., Hall, C. D., Sun, C. C., et al. (2015). Practical murine hematopathology: a comparative review and implications for research. Comp. Med. 65, 96–113.
Oyinbo, C. A. (2011). Secondary injury mechanisms in traumatic spinal cord injury: a nugget of this multiply cascade. Acta Neurobiol. Exp. (Wars) 71, 281–299.
Papayannopoulos, V. (2018). Neutrophil extracellular traps in immunity and disease. Nat. Rev. Immunol. 18, 134–147. doi: 10.1038/nri.2017.105
Papayannopoulos, V., Metzler, K. D., Hakkim, A., and Zychlinsky, A. (2010). Neutrophil elastase and myeloperoxidase regulate the formation of neutrophil extracellular traps. J. Cell Biol. 191, 677–691. doi: 10.1083/jcb.201006052
Parroche, P., Lauw, F. N., Goutagny, N., Latz, E., Monks, B. G., Visintin, A., et al. (2007). Malaria hemozoin is immunologically inert but radically enhances innate responses by presenting malaria DNA to Toll-like receptor 9. Proc. Natl. Acad. Sci. U.S.A. 104, 1919–1924. doi: 10.1073/pnas.0608745104
Pelisch, N., Rosas Almanza, J., Stehlik, K. E., Aperi, B. V., and Kroner, A. (2020). CCL3 contributes to secondary damage after spinal cord injury. J. Neuroinflammation 17:362. doi: 10.1186/s12974-020-02037-3
Perez-de-Puig, I., Miro-Mur, F., Ferrer-Ferrer, M., Gelpi, E., Pedragosa, J., Justicia, C., et al. (2015). Neutrophil recruitment to the brain in mouse and human ischemic stroke. Acta Neuropathol. 129, 239–257. doi: 10.1007/s00401-014-1381-0
Pietronigro, E. C., Della Bianca, V., Zenaro, E., and Constantin, G. (2017). NETosis in Alzheimer’s Disease. Front. Immunol. 8:211. doi: 10.3389/fimmu.2017.00211
Pilsczek, F. H., Salina, D., Poon, K. K., Fahey, C., Yipp, B. G., Sibley, C. D., et al. (2010). A novel mechanism of rapid nuclear neutrophil extracellular trap formation in response to Staphylococcus aureus. J. Immunol. 185, 7413–7425. doi: 10.4049/jimmunol.1000675
Pineau, I., and Lacroix, S. (2007). Proinflammatory cytokine synthesis in the injured mouse spinal cord: multiphasic expression pattern and identification of the cell types involved. J. Comp. Neurol. 500, 267–285. doi: 10.1002/cne.21149
Pineau, I., Sun, L., Bastien, D., and Lacroix, S. (2010). Astrocytes initiate inflammation in the injured mouse spinal cord by promoting the entry of neutrophils and inflammatory monocytes in an IL-1 receptor/MyD88-dependent fashion. Brain Behav. Immun. 24, 540–553. doi: 10.1016/j.bbi.2009.11.007
Popovich, P. G., and Jones, T. B. (2003). Manipulating neuroinflammatory reactions in the injured spinal cord: back to basics. Trends Pharmacol. Sci. 24, 13–17. doi: 10.1016/s0165-6147(02)00006-8
Pouwels, S. D., van Geffen, W. H., Jonker, M. R., Kerstjens, H. A., Nawijn, M. C., and Heijink, I. H. (2017). Increased neutrophil expression of pattern recognition receptors during COPD exacerbations. Respirology 22, 401–404. doi: 10.1111/resp.12912
Prame Kumar, K., Nicholls, A. J., and Wong, C. H. Y. (2018). Partners in crime: neutrophils and monocytes/macrophages in inflammation and disease. Cell Tissue Res. 371, 551–565. doi: 10.1007/s00441-017-2753-2
Price, S. E., Sharpe, G., Boots, A., Poutsma, A., Mason, C., James, J., et al. (1997). Role of myelin basic protein and proteolipid protein genes in multiple sclerosis: single strand conformation polymorphism analysis of the human sequences. Neuropathol. Appl. Neurobiol. 23, 457–467. doi: 10.1111/j.1365-2990.1997.tb01322.x
Qin, W., Zhang, M., Piao, Y., Guo, D., Zhu, Z., Tian, X., et al. (2012). Wallerian degeneration in central nervous system: dynamic associations between diffusion indices and their underlying pathology. PLoS One 7:e41441. doi: 10.1371/journal.pone.0041441
Ramadass, M., and Catz, S. D. (2016). Molecular mechanisms regulating secretory organelles and endosomes in neutrophils and their implications for inflammation. Immunol. Rev. 273, 249–265. doi: 10.1111/imr.12452
Ramos-Kichik, V., Mondragon-Flores, R., Mondragon-Castelan, M., Gonzalez-Pozos, S., Muniz-Hernandez, S., Rojas-Espinosa, O., et al. (2009). Neutrophil extracellular traps are induced by Mycobacterium tuberculosis. Tuberculosis (Edinb) 89, 29–37. doi: 10.1016/j.tube.2008.09.009
Roh, J. S., and Sohn, D. H. (2018). Damage-Associated molecular patterns in inflammatory diseases. Immune Netw. 18:e27. doi: 10.4110/in.2018.18.e27
Roots, B. I., Cardone, B., and Pereyra, P. (1991). Isolation and characterization of the myelin-like membranes ensheathing giant axons in the earthworm nerve cord. Ann. N. Y. Acad. Sci. 633, 559–561. doi: 10.1111/j.1749-6632.1991.tb15660.x
Rosales, C. (2020). Neutrophils at the crossroads of innate and adaptive immunity. J. Leukoc. Biol. 108, 377–396. doi: 10.1002/JLB.4MIR0220-574RR
Rosales, C., Demaurex, N., Lowell, C. A., and Uribe-Querol, E. (2016). Neutrophils: their role in innate and adaptive immunity. J. Immunol. Res. 2016, 1469780. doi: 10.1155/2016/1469780
Rosales, C., Lowell, C. A., Schnoor, M., and Uribe-Querol, E. (2017). Neutrophils: their role in innate and adaptive immunity 2017. J. Immunol. Res. 2017:9748345. doi: 10.1155/2017/9748345
Rothwarf, D. M., and Karin, M. (1999). The NF-kappa B activation pathway: a paradigm in information transfer from membrane to nucleus. Sci. STKE 1999:RE1. doi: 10.1126/stke.1999.5.re1
Saffarzadeh, M., Juenemann, C., Queisser, M. A., Lochnit, G., Barreto, G., Galuska, S. P., et al. (2012). Neutrophil extracellular traps directly induce epithelial and endothelial cell death: a predominant role of histones. PLoS One 7:e32366. doi: 10.1371/journal.pone.0032366
Saiwai, H., Ohkawa, Y., Yamada, H., Kumamaru, H., Harada, A., Okano, H., et al. (2010). The LTB4-BLT1 axis mediates neutrophil infiltration and secondary injury in experimental spinal cord injury. Am. J. Pathol. 176, 2352–2366. doi: 10.2353/ajpath.2010.090839
Sas, A. R., Carbajal, K. S., Jerome, A. D., Menon, R., Yoon, C., Kalinski, A. L., et al. (2020). A new neutrophil subset promotes CNS neuron survival and axon regeneration. Nat. Immunol. 21, 1496–1505. doi: 10.1038/s41590-020-00813-0
Savill, J. S., Wyllie, A. H., Henson, J. E., Walport, M. J., Henson, P. M., and Haslett, C. (1989). Macrophage phagocytosis of aging neutrophils in inflammation. Programmed cell death in the neutrophil leads to its recognition by macrophages. J Clin Invest 83, 865–875. doi: 10.1172/JCI113970
Schorn, C., Janko, C., Latzko, M., Chaurio, R., Schett, G., and Herrmann, M. (2012). Monosodium urate crystals induce extracellular DNA traps in neutrophils, eosinophils, and basophils but not in mononuclear cells. Front. Immunol. 3:277. doi: 10.3389/fimmu.2012.00277
Scieszka, D., Lin, Y.-H., Li, W., Choudhury, S., Yu, Y., and Freire, M. (2020). Netome: the molecular characterization of neutrophil extracellular traps (NETs). bioRxiv [Preprint] doi: 10.1101/2020.05.18.102772 bioRxiv: 2020.2005.2018.102772,
Sheshachalam, A., Srivastava, N., Mitchell, T., Lacy, P., and Eitzen, G. (2014). Granule protein processing and regulated secretion in neutrophils. Front. Immunol. 5:448. doi: 10.3389/fimmu.2014.00448
Shrestha, B., Ito, T., Kakuuchi, M., Totoki, T., Nagasato, T., Yamamoto, M., et al. (2019). Recombinant thrombomodulin suppresses histone-induced neutrophil extracellular trap formation. Front. Immunol. 10:2535. doi: 10.3389/fimmu.2019.02535
Simons, M., and Nave, K. A. (2015). Oligodendrocytes: myelination and axonal support. Cold Spring Harb. Perspect. Biol. 8:a020479. doi: 10.1101/cshperspect.a020479
Singh, P. L., Agarwal, N., Barrese, J. C., and Heary, R. F. (2012). Current therapeutic strategies for inflammation following traumatic spinal cord injury. Neural Regen. Res. 7, 1812–1821. doi: 10.3969/j.issn.1673-5374.2012.23.008
Smith, M. E. (1999). Phagocytosis of myelin in demyelinative disease: a review. Neurochem. Res. 24, 261–268. doi: 10.1023/a:1022566121967
Sorensen, O. E., and Borregaard, N. (2016). Neutrophil extracellular traps – the dark side of neutrophils. J. Clin. Invest. 126, 1612–1620. doi: 10.1172/JCI84538
Stadelmann, C., Timmler, S., Barrantes-Freer, A., and Simons, M. (2019). Myelin in the central nervous system: structure, function, and pathology. Physiol. Rev. 99, 1381–1431. doi: 10.1152/physrev.00031.2018
Steinberg, B. E., and Grinstein, S. (2007). Unconventional roles of the NADPH oxidase: signaling, ion homeostasis, and cell death. Sci. STKE 2007:e11. doi: 10.1126/stke.3792007pe11
Stirling, D. P., Liu, S., Kubes, P., and Yong, V. W. (2009). Depletion of Ly6G/Gr-1 leukocytes after spinal cord injury in mice alters wound healing and worsens neurological outcome. J Neurosci. 29, 753–764. doi: 10.1523/JNEUROSCI.4918-08.2009
Stoiber, W., Obermayer, A., Steinbacher, P., and Krautgartner, W. D. (2015). the role of reactive oxygen species (ros) in the formation of extracellular traps (ETs) in humans. Biomolecules 5, 702–723. doi: 10.3390/biom5020702
Sun, X., Wang, X., Chen, T., Li, T., Cao, K., Lu, A., et al. (2010). Myelin activates FAK/Akt/NF-kappaB pathways and provokes CR3-dependent inflammatory response in murine system. PLoS One 5:e9380. doi: 10.1371/journal.pone.0009380
Suresh, R., and Mosser, D. M. (2013). Pattern recognition receptors in innate immunity, host defense, and immunopathology. Adv. Physiol. Educ. 37, 284–291. doi: 10.1152/advan.00058.2013
Svensson, D., Aidoukovitch, A., Anders, E., Jonsson, D., Nebel, D., and Nilsson, B. O. (2017). Secretory leukocyte protease inhibitor regulates human periodontal ligament cell production of pro-inflammatory cytokines. Inflamm. Res. 66, 823–831. doi: 10.1007/s00011-017-1062-2
Syed, Y. A., Zhao, C., Mahad, D., Mobius, W., Altmann, F., Foss, F., et al. (2016). Antibody-mediated neutralization of myelin-associated EphrinB3 accelerates CNS remyelination. Acta Neuropathol. 131, 281–298. doi: 10.1007/s00401-015-1521-1
Teng, T. S., Ji, A. L., Ji, X. Y., and Li, Y. Z. (2017). Neutrophils and immunity: from bactericidal action to being conquered. J. Immunol. Res. 2017:9671604. doi: 10.1155/2017/9671604
Thiam, H. R., Wong, S. L., Qiu, R., Kittisopikul, M., Vahabikashi, A., Goldman, A. E., et al. (2020). NETosis proceeds by cytoskeleton and endomembrane disassembly and PAD4-mediated chromatin decondensation and nuclear envelope rupture. Proc. Natl. Acad. Sci. U S.A 117, 7326–7337. doi: 10.1073/pnas.1909546117
Tillack, K., Naegele, M., Haueis, C., Schippling, S., Wandinger, K. P., Martin, R., et al. (2013). Gender differences in circulating levels of neutrophil extracellular traps in serum of multiple sclerosis patients. J. Neuroimmunol. 261, 108–119. doi: 10.1016/j.jneuroim.2013.05.004
Trivedi, A., Olivas, A. D., and Noble-Haeusslein, L. J. (2006). Inflammation and spinal cord injury: infiltrating leukocytes as determinants of injury and repair processes. Clin. Neurosci. Res. 6, 283–292. doi: 10.1016/j.cnr.2006.09.007
Vaibhav, K., Braun, M., Alverson, K., Khodadadi, H., Kutiyanawalla, A., Ward, A., et al. (2020). Neutrophil extracellular traps exacerbate neurological deficits after traumatic brain injury. Sci. Adv. 6:eaax8847. doi: 10.1126/sciadv.aax8847
Valles, J., Lago, A., Santos, M. T., Latorre, A. M., Tembl, J. I., Salom, J. B., et al. (2017). Neutrophil extracellular traps are increased in patients with acute ischemic stroke: prognostic significance. Thromb. Haemost. 117, 1919–1929. doi: 10.1160/TH17-02-0130
Venereau, E., Ceriotti, C., and Bianchi, M. E. (2015). DAMPs from cell death to new life. Front. Immunol. 6:422. doi: 10.3389/fimmu.2015.00422
Villanueva, E., Yalavarthi, S., Berthier, C. C., Hodgin, J. B., Khandpur, R., Lin, A. M., et al. (2011). Netting neutrophils induce endothelial damage, infiltrate tissues, and expose immunostimulatory molecules in systemic lupus erythematosus. J. Immunol. 187, 538–552. doi: 10.4049/jimmunol.1100450
Vorobjeva, N. V., and Pinegin, B. V. (2014). Neutrophil extracellular traps: mechanisms of formation and role in health and disease. Biochemistry (Mosc) 79, 1286–1296. doi: 10.1134/S0006297914120025
Wang, J. (2018). Neutrophils in tissue injury and repair. Cell Tissue Res. 371, 531–539. doi: 10.1007/s00441-017-2785-7
Wang, S., Deng, J., Fu, H., Guo, Z., Zhang, L., and Tang, P. (2020). Astrocytes directly clear myelin debris through endocytosis pathways and followed by excessive gliosis after spinal cord injury. Biochem. Biophys. Res. Commun. 525, 20–26. doi: 10.1016/j.bbrc.2020.02.069
Wang, X., Cao, K., Sun, X., Chen, Y., Duan, Z., Sun, L., et al. (2015). Macrophages in spinal cord injury: phenotypic and functional change from exposure to myelin debris. Glia 63, 635–651. doi: 10.1002/glia.22774
Williamson, J. M., and Lyons, D. A. (2018). Myelin dynamics throughout life: an ever-changing landscape? Front. Cell Neurosci. 12:424. doi: 10.3389/fncel.2018.00424
Wrathall, J. R., Li, W., and Hudson, L. D. (1998). Myelin gene expression after experimental contusive spinal cord injury. J. Neurosci. 18, 8780–8793.
Xu, J. A., Hsu, C. Y., Liu, T. H., Hogan, E. L., Perot, P. L. Jr., and Tai, H. H. (1990). Leukotriene B4 release and polymorphonuclear cell infiltration in spinal cord injury. J. Neurochem. 55, 907–912. doi: 10.1111/j.1471-4159.1990.tb04577.x
Yalavarthi, S., Gould, T. J., Rao, A. N., Mazza, L. F., Morris, A. E., Nunez-Alvarez, C., et al. (2015). Release of neutrophil extracellular traps by neutrophils stimulated with antiphospholipid antibodies: a newly identified mechanism of thrombosis in the antiphospholipid syndrome. Arthritis Rheumatol. 67, 2990–3003. doi: 10.1002/art.39247
Yaseen, R., Blodkamp, S., Luthje, P., Reuner, F., Vollger, L., Naim, H. Y., et al. (2017). Antimicrobial activity of HL-60 cells compared to primary blood-derived neutrophils against Staphylococcus aureus. J. Negat. Results Biomed. 16:2. doi: 10.1186/s12952-017-0067-2
Yates, A. G., Jogia, T., Gillespie, E. R., Couch, Y., Ruitenberg, M. J., and Anthony, D. C. (2021). Acute IL-1RA treatment suppresses the peripheral and central inflammatory response to spinal cord injury. J. Neuroinflammation 18:15. doi: 10.1186/s12974-020-02050-6
Ying, Q. L., Kemme, M., and Simon, S. R. (1994). Functions of the N-terminal domain of secretory leukoprotease inhibitor. Biochemistry 33, 5445–5450. doi: 10.1021/bi00184a013
Yu, Y., Zhou, H., Kong, Y., Pan, B., Chen, L., Wang, H., et al. (2016). The Landscape of A-to-I RNA editome is shaped by both positive and purifying selection. PLoS Genet. 12:e1006191. doi: 10.1371/journal.pgen.1006191
Zhang, Z., and Fuller, G. M. (2000). Interleukin 1beta inhibits interleukin 6-mediated rat gamma fibrinogen gene expression. Blood 96, 3466–3472.
Zhou, T., Zheng, Y., Sun, L., Badea, S. R., Jin, Y., Liu, Y., et al. (2019). Microvascular endothelial cells engulf myelin debris and promote macrophage recruitment and fibrosis after neural injury. Nat. Neurosci. 22, 421–435. doi: 10.1038/s41593-018-0324-9
Keywords: spinal cord injury, neutrophils, secondary injury, inflammation, cytokines, myelin debris
Citation: Zivkovic S, Ayazi M, Hammel G and Ren Y (2021) For Better or for Worse: A Look Into Neutrophils in Traumatic Spinal Cord Injury. Front. Cell. Neurosci. 15:648076. doi: 10.3389/fncel.2021.648076
Received: 31 December 2020; Accepted: 08 March 2021;
Published: 22 April 2021.
Edited by:
Junfang Wu, University of Maryland School of Medicine, United StatesReviewed by:
Li Cai, Rutgers, The State University of New Jersey, United StatesDylan McCreedy, Texas A&M University, United States
Copyright © 2021 Zivkovic, Ayazi, Hammel and Ren. This is an open-access article distributed under the terms of the Creative Commons Attribution License (CC BY). The use, distribution or reproduction in other forums is permitted, provided the original author(s) and the copyright owner(s) are credited and that the original publication in this journal is cited, in accordance with accepted academic practice. No use, distribution or reproduction is permitted which does not comply with these terms.
*Correspondence: Yi Ren, eWkucmVuQG1lZC5mc3UuZWR1