- Department of Molecular Physiology, Center for Integrative Physiology and Molecular Medicine, University of Saarland, Homburg, Germany
Astroglial networks constitute a non-neuronal communication system in the brain and are acknowledged modulators of synaptic plasticity. A sophisticated set of transmitter receptors in combination with distinct secretion mechanisms enables astrocytes to sense and modulate synaptic transmission. This integrative function evolved around intracellular Ca2+ signals, by and large considered as the main indicator of astrocyte activity. Regular brain physiology meticulously relies on the constant reciprocity of excitation and inhibition (E/I). Astrocytes are metabolically, physically, and functionally associated to the E/I convergence. Metabolically, astrocytes provide glutamine, the precursor of both major neurotransmitters governing E/I in the central nervous system (CNS): glutamate and γ-aminobutyric acid (GABA). Perisynaptic astroglial processes are structurally and functionally associated with the respective circuits throughout the CNS. Astonishingly, in astrocytes, glutamatergic as well as GABAergic inputs elicit similar rises in intracellular Ca2+ that in turn can trigger the release of glutamate and GABA as well. Paradoxically, as gliotransmitters, these two molecules can thus strengthen, weaken or even reverse the input signal. Therefore, the net impact on neuronal network function is often convoluted and cannot be simply predicted by the nature of the stimulus itself. In this review, we highlight the ambiguity of astrocytes on discriminating and affecting synaptic activity in physiological and pathological state. Indeed, aberrant astroglial Ca2+ signaling is a key aspect of pathological conditions exhibiting compromised network excitability, such as epilepsy. Here, we gather recent evidence on the complexity of astroglial Ca2+ signals in health and disease, challenging the traditional, neuro-centric concept of segregating E/I, in favor of a non-binary, mutually dependent perspective on glutamatergic and GABAergic transmission.
Introduction
The path that led the scientific community to agree upon the role of astrocytes in actively tuning and modulating brain activity has been one of the most challenging and fertile fields in neuroscience for the last decades. It is now widely accepted that astrocytes can sense, react to and modify the extracellular transmitter milieu both quantitatively and qualitatively, thus contributing to neural network excitability and functioning. Nevertheless, little is still known about the exact molecular mechanisms of the astrocytic response and contribution to synaptic transmission. Most studies have been characterizing astrocytes in terms of their inputs and outputs, without precise knowledge of their inner working, thus regarding them as black boxes. Nowadays, internal Ca2+ oscillations are by far considered the main read-out of astrocytic activity (Bazargani and Attwell, 2016) and are known to be induced, among others, by binding of neurotransmitters to astroglial membrane receptors and to eventually lead to the release of gliotransmitters in the extracellular space. These include glutamate (Parpura et al., 1994; Pasti et al., 1997; Kang et al., 1998; Parri et al., 2001; Angulo et al., 2004; Fellin et al., 2004), ATP (Pascual et al., 2005; Serrano et al., 2006), D-serine (Henneberger et al., 2010) and γ-aminobutyric acid (GABA; Liu et al., 2000; Kozlov et al., 2006; Lee et al., 2010; Jiménez-González et al., 2011). In this review we point out that a pile of evidence is building up against a simplistic way of considering astrocytic Ca2+ response as a linear and stereotypical process. In order to understand the brain, it is essential to regard astrocytes as active information integrators and processors.
Astroglial Ca2+ Dynamics at the Interface of Glutamatergic and Gabaergic Signaling
The astroglial role as mere responders to neuronal firing was challenged by the fact that astrocytes exhibited internal Ca2+ oscillations in hippocampal slice preparations even in presence of the neuronal voltage-gated Na+ channel blocker tetrodotoxin (Nett et al., 2002). This confirmed previous in vitro evidence of neuron-independent Ca2+ activity (Araque et al., 1999; Parri et al., 2001). These results were then similarly obtained using genetically encoded Ca2+ indicators (GECIs; Haustein et al., 2014; Bindocci et al., 2017). Several lines of evidence suggested that the spontaneous opening of a member of the transient receptor potential (TRP) family, TRPA1, and possibly of other cation channels of the same family, contribute to resting astroglial Ca2+ levels and at least a fraction of their intrinsic fluctuations (Shigetomi et al., 2011, 2013; Agarwal et al., 2017). However, neither specific TRPA1 mRNA or protein was so far detected in astrocytes (Verkhratsky et al., 2014). In line with these observations, 0 mM [Ca2+]o reduced the Ca2+ transient frequency of the gliapil by up to 75% and the knockout of the inositol triphosphate type 2 receptor (IP3R2) spared around 10% of somatic and around 40% of gliapil fluctuations without affecting the frequency of the latter (Srinivasan et al., 2015). Since tetrodotoxin prevents action potential generation and not neurotransmission itself, these results can, at least partially, be attributed to astroglial responses elicited by spontaneous neurotransmitter release, as shown for the activation of cortical astrocytes by glutamate and ATP (Palygin et al., 2010; Lalo et al., 2011).
Indeed, astroglial Ca2+ oscillations driven by extracellular inputs superimpose on and integrate intrinsic Ca2+ activity, thus making astrocytes active partners of network functioning. With some notable exceptions (Jennings et al., 2017; Xin et al., 2019), activation of G-protein coupled receptors (GPCRs) leads to intracellular Ca2+ elevations (Kofuji and Araque, 2020) not only upon stimulation with molecules commonly considered excitatory, such as glutamate, but also with the canonical inhibitory neurotransmitter GABA (Perea et al., 2016; Mariotti et al., 2018; Mederos and Perea, 2019; Nagai et al., 2019). In line with these observations and contrary to their neuronal counterpart (Huang and Thathiah, 2015), in vivo chemogenetic activation of both Gq and Gi/o DREADDs elicited Ca2+ increases in astrocytes (Durkee et al., 2019), thus challenging the long-established concept of E/I as mutually interplaying and yet still discernable processes (Isaacson and Scanziani, 2011). [Ca2+]i increase can lead to the release of glutamate (Parpura et al., 1994; Pasti et al., 1997; Kang et al., 1998; Parri et al., 2001; Angulo et al., 2004; Fellin et al., 2004) as well as of GABA (Liu et al., 2000; Kozlov et al., 2006; Lee et al., 2010; Jiménez-González et al., 2011). Notably, contrary to Ca2+ uncaging or inositol-1,4,5-trisphosphate (IP3) application, Gq-coupled receptor activation does not necessarily induce the release of gliotransmitters (Wang et al., 2013) and different Gq-coupled receptors can exert gliotransmitter release with different efficiencies (Shigetomi et al., 2008). This challenges the idea that astrocytes may act as a redundant layer that responds similarly to GPCR-mediated inputs (Guerra-Gomes et al., 2017) and suggests that astrocytes can discriminate metabotropic signaling upstream of internal Ca2+ oscillations.
The astroglial membrane receptome, responsible for transmitter-triggered Ca2+ signaling is highly diverse and is far from being fully characterized (Figure 1A). In the following, we focus on glutamatergic and GABAergic signaling, representing by far the major emblems of the black-and-white E/I dichotomy. Astrocytes can express various types of ionotropic Ca2+ permeable glutamate receptors: AMPA receptors in cortex and cerebellum (Schipke et al., 2001; Lalo et al., 2006; Saab et al., 2012) and NMDA receptors in the cortex (Schipke et al., 2001; Lalo et al., 2006; Kirchhoff, 2017), although their expression in the hippocampus is still unclear (Matthias et al., 2003; Verkhratsky and Kirchhoff, 2007; Serrano et al., 2008; Letellier et al., 2016). Kainate receptors may be absent from astroglial membranes under physiological conditions, but were reported to be inducible in a mouse model of temporal lobe epilepsy (TLE; Das et al., 2012; Vargas et al., 2013). Among metabotropic glutamate receptors, Gq-coupled mGluR5 activation results in IP3-mediated Ca2+ increase through the phospholipase C pathway (Panatier and Robitaille, 2016). mGluR5 contribution to Ca2+ oscillations seems to be restricted, at least in the adult brain, to the fine perisynaptic processes (Sun et al., 2013, 2014; Haustein et al., 2014). Astrocytes express also the Gi/o-coupled mGluR2/3, whose activation leads to inhibition of adenylate cyclase (Aronica et al., 2003; Sun et al., 2013; Figure 1A).
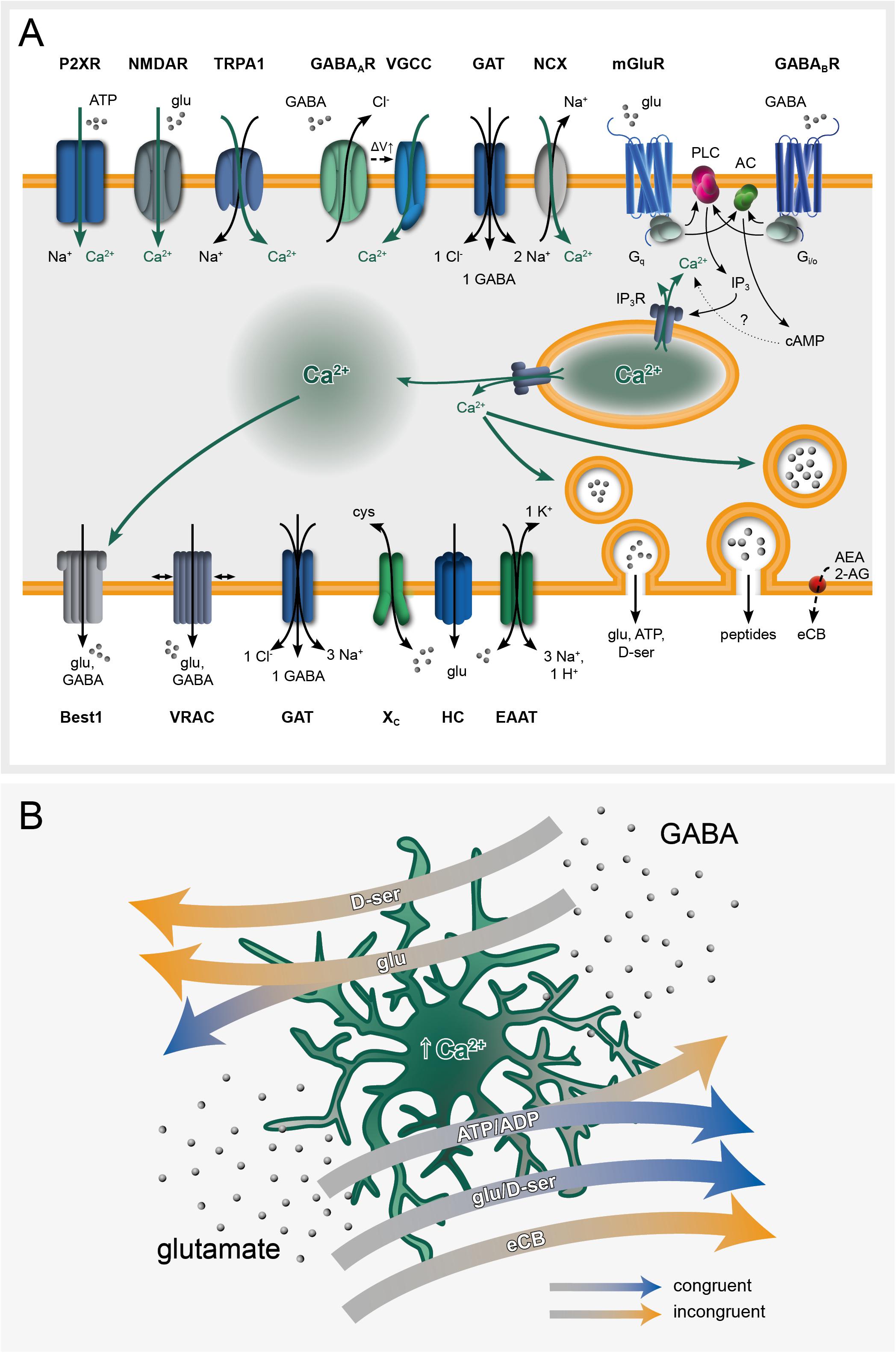
Figure 1. Ca2+ signaling at the core of astroglial black box operations. (A) Astroglial receptome coordinates glutamate and GABA-induced intracellular Ca2+ signaling and subsequent gliotransmitter release. (B) Glutamate and GABA elicit Ca2+-dependent gliotransmitter-mediated congruent and incongruent modulation of network plasticity. Congruent signaling refers to contexts in which GABA and glutamate as initial stimuli exert inhibitory or excitatory effects on the neuronal network, respectively. Vice versa, incongruent signaling designates scenarios in which GABA has an excitatory and glutamate an inhibitory final impact on the network. Both congruent and incongruent signaling may involve the same gliotransmitters (highlighted on the arrows). 2-AG, 2-arachidonoylglycerol; AC, adenylate cyclase; ADP/ATP, adenosine di/tri-phosphate; AEA, anandamide; Best1, bestrophin-1 channel; cAMP, cyclic AMP; cys, cysteine; D-ser, D-serine; EAAT, excitatory amino acid transporter; eCB, endocannabinoids; GABA, γ-aminobutyric acid; GABAAR/GABABR, GABA receptors; GAT, GABA transporter; glu, glutamate; HC, hemichannel; IP3, inositol triphosphate; IP3R, inositol triphosphate receptor; mGluR, metabotropic glutamate receptor; NCX, sodium-calcium exchanger; NMDAR, N-methyl-D-aspartate receptor; P2XR, purinergic transmitter-gated ion channels; PLC, phospholipase C; TRPA1, transient receptor potential A1; VGCC, voltage-gated calcium channel; VRAC, volume-regulated anion channel; XC, cysteine-glutamate antiporter; ΔV, membrane potential.
With respect to GABAergic signaling, astrocytes display internal Ca2+ increases following GABAA receptor-mediated depolarization through voltage-gated Ca2+ channels (VGCCs; Nilsson et al., 1993; Verkhratsky and Steinhäuser, 2000; Meier et al., 2008; Parpura et al., 2011; Verkhratsky et al., 2012). However, given the low membrane input resistance of mature astrocytes, the contribution of GABAA receptors to Ca2+ responses in vivo remains controversial. Metabotropic Gi/o-coupled GABAB receptors were extensively reported to induce intracellular Ca2+ rises in an IP3-dependent manner (Mariotti et al., 2016; Nagai et al., 2019) followed by gliotransmitter release (Serrano et al., 2006; Perea et al., 2016; Durkee et al., 2019). Although their role in the cortical astroglial response is unclear, GABA transporter (GAT)-mediated Na+ symport increases intracellular Ca2+ through the Na+/Ca2+ exchanger (Doengi et al., 2009; Boddum et al., 2016), as previously suggested for glutamate transporters (Schummers et al., 2008). Synergistic activation of different pathways is likely to occur upon GABAergic signaling, thus potentially introducing an additional level of up-stream signal discrimination (Matos et al., 2018; Figure 1A).
There is plenty of evidence that gliotransmitter release occurs (Parpura et al., 1994; Jeftinija et al., 1997; Bezzi et al., 1998) and that it is, at least partially, a Ca2+ dependent mechanism (Bezzi et al., 2004; Perea and Araque, 2005; Araque and Navarrete, 2010; Schwarz et al., 2017; Bohmbach et al., 2018; Savtchouk and Volterra, 2018). Glutamate release occurs through several pathways, including reverse operation of plasma membrane glutamate transporters (Longuemare and Swanson, 1997; Rossi et al., 2000), cystine-glutamate Xc- antiporter (Cavelier and Attwell, 2005), volume-regulated anion channels (VRACs; Kimelberg et al., 1990; Mongin and Kimelberg, 2005; Abdullaev et al., 2006; Liu et al., 2006; Ramos-Mandujano et al., 2007), P2X7 receptors (Duan et al., 2003), the Ca2+ activated anion channel bestrophin 1 (Best1; Park et al., 2009; Woo et al., 2012), hemichannels (Ye et al., 2003) and vesicular exocytosis (Jeftinija et al., 1997; Bezzi et al., 2004; Montana et al., 2006; Bowser and Khakh, 2007; Xu et al., 2007; Parpura and Zorec, 2010; Schwarz et al., 2017). In contrast to glutamate, GABA release mechanisms were less extensively addressed and remain elusive. Vesicular release of GABA seems unlikely due to the lack of GABA-containing synaptic vesicles in astrocytes (Yoon and Lee, 2014). Ex vivo electrophysiological studies using acute brain slices suggested that VRACs (Kozlov et al., 2006; Le Meur et al., 2012) as well as GATs (Barakat and Bordey, 2002; Richerson and Wu, 2003; Lee et al., 2011) can mediate GABA release. GAT2/3 are indeed involved in astroglial GABA release (Héja et al., 2012; Unichenko et al., 2013), as well as Best1 (Lee et al., 2010). Although still under debate, both GATs and Best1 could be responsible for tonic as well as phasic GABAergic astroglial signaling under physiological conditions. Remarkably, reactive astrocytes show aberrant and abundant tonic GABA release through Best1 in mouse models of Alzheimer’s disease (Jo et al., 2014). In a mouse model of TLE, the astrocyte-specific rescue of Best1 could restore tonic GABAergic inhibition and suppressed seizure susceptibility in Best1 complete knock-out mice (Pandit et al., 2020).
The Paradox of Astroglial Black Box Function in the Neural Network
The astrocytes’ black box operations typically involve an initial stimulus (most commonly synaptically released neurotransmitters), an astroglial receptor inducing an intracellular signaling cascade leading to Ca2+ elevations, a released gliotransmitter and finally the net effect on the neuronal network: excitation or inhibition. Assuming that GABA as initial stimulus would exert an inhibitory (congruent) rather than excitatory (incongruent) and glutamate an excitatory (congruent) rather than inhibitory (incongruent) effect on the network, several scenarios co-exist along this information processing thread (Figure 1B and Table 1).
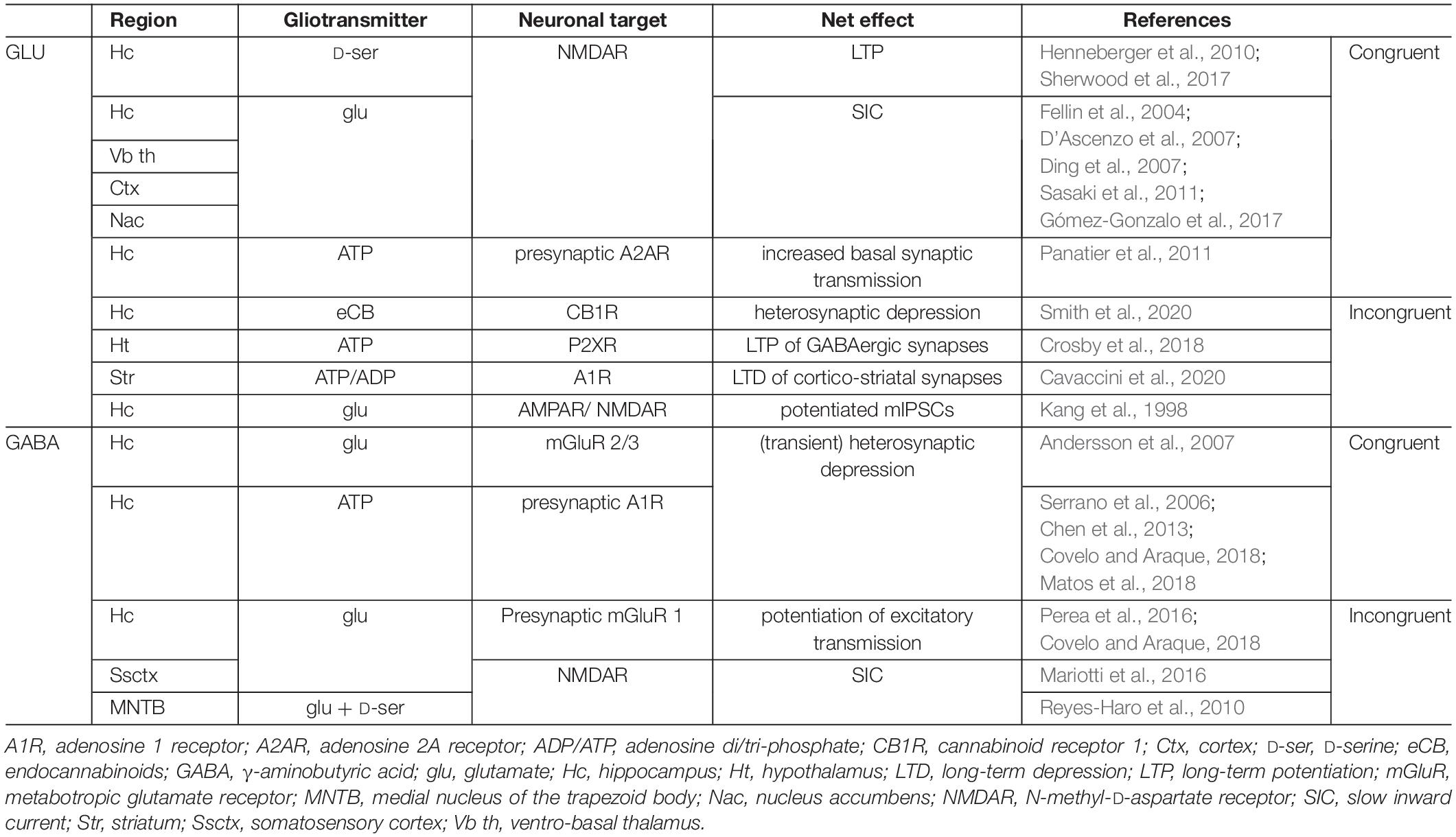
Table 1. Summary of congruent and incongruent signaling pathways evoked by glutamate and GABA, according to brain region, released gliotransmitter, and principal neuronal targets.
Congruent Signaling Preserves Input–Output Polarity
In the simplest case, initial stimulus and net result are congruent as it has been observed frequently across brain regions. Cortical, hippocampal and thalamic astrocytes stimulated by glutamate release glutamate and in turn induce NMDA receptor-dependent slow inward currents (SICs) in adjacent neurons (D’Ascenzo et al., 2007; Ding et al., 2007; Pirttimaki et al., 2011; Sasaki et al., 2011; Gómez-Gonzalo et al., 2017), resulting in neuronal synchronization and an elevated network excitability (Fellin et al., 2004). However, a congruent net result can also be obtained when the released gliotransmitter is neither glutamate nor GABA. Within the hippocampal network, both glutamate and GABA, as neurotransmitters, can stimulate ATP release from astrocytes and thereby evoke congruent consequences for the neuronal environment. Glutamate-induced ATP release enhances basal synaptic transmission at pyramidal cells (Panatier et al., 2011), while GABA-induced ATP release generates (hetero-) synaptic depression (Serrano et al., 2006; Andersson et al., 2007; Chen et al., 2013; Covelo and Araque, 2018) or up-regulation of inhibitory transmission (Matos et al., 2018). Alternatively, glutamate can stimulate astrocytes to release the NMDA receptor co-agonist D-serine, being an essential component of hippocampal long-term potentiation (LTP; Henneberger et al., 2010; Sherwood et al., 2017). Most strikingly, GABAergic stimulation can lead to astroglial release of glutamate, anticipating a reversal of the initial stimulus’ nature, yet still exhibiting a net dampening effect on the hippocampal network through potentiated inhibitory postsynaptic currents (IPSCs; Kang et al., 1998) or heterosynaptic depression (Andersson et al., 2007). Astrocytes therefore can convey the initial message of the primary stimulus to the network, sometimes via alternative routes (ATP, D-serine) and even by switching from an “inhibitory” GABAergic stimulation to glutamate release (Figure 1B and Table 1).
Incongruent Signaling Reverses Input–Output Polarity
Astrocytes can change the nature of the initial stimulus with respect to the output to the network. The most evident way to achieve this is to perform a switch between the main inhibitory and the main excitatory transmitter. Indeed, GABAergic stimulation of astrocytes can be turned into glutamatergic excitation and thereby potentiate excitatory transmission at the CA3-CA1 microcircuit (Mariotti et al., 2016; Perea et al., 2016; Covelo and Araque, 2018). Likewise, an astroglial switch is attained by including impartial transmitters in the information processing thread. Hypothalamic astrocytes receiving concomitant glutamatergic input and cholecystokinin stimulation release ATP. Acting on P2X-receptors, this astrocyte-derived purinergic stimulation is crucial for LTP of local GABAergic synapses, thereby augmenting the inhibitory tone of the network (Crosby et al., 2018). Moreover, striatal astrocytes also switch a glutamatergic input into long-term depression (LTD) mediated via purines (Cavaccini et al., 2020). Similarly, endocannabinoids released after glutamatergic activation mediate transient hippocampal heterosynaptic depression (Smith et al., 2020). Conversely, GABA-evoked astroglial Ca2+-transients induce the co-release of glutamate and D-Serine, thereby producing NMDA receptor-dependent SICs in principal neurons of the medial nucleus of the trapezoid body (MNTB, ascending auditory pathway) (Reyes-Haro et al., 2010). In contrast to hippocampal pyramidal neurons, however, the postsynaptic principal neurons of the MNTB are less susceptible to SIC-mediated synchronization. Moreover, astroglial switches modulating synaptic plasticity operate at various timescales. In contrast to mechanisms such as LTP and (transient) heterosynaptic depression acting within minutes, striatal astrocytes increase the number of excitatory synapses and enhance excitatory transmission upon GABAergic stimulation, prevailing for at least 48 h. Mechanistically, the activation of the Gi-pathway downstream of the astroglial GABAB receptor leads to an upregulation of the synaptogenic multi-domain matrix glycoprotein thrombospondin-1 in vivo (Nagai et al., 2019).
Astrocytes therefore can reverse the initial message of the primary stimulus to the network with the same mechanisms employed to convey a congruent message: by switching from an “inhibitory” GABAergic stimulation to glutamate release, using alternative routes (ATP, D-serine, endocannabinoids), but oppositely even by reproducing the initial stimulus (glutamate-induced glutamate release) (Figure 1B and Table 1). For sake of simplicity, here the concept of congruent and incongruent signaling has been mainly reduced to contexts involving glutamate or GABA as initial stimulus, followed by Ca2+-dependent gliotransmitter release. However, gliotransmitters’ impact on neuronal signaling encompasses several additional levels of complexity. Glia-driven purinergic signaling for example can induce down regulation of neuronal GABAA (Lalo et al., 2014) as well as NMDA receptors (Lalo et al., 2016), thereby potentially acting in both congruent and incongruent ways. Furthermore, astroglial Ca2+-sensitive Best-1 channels have been shown to release glutamate and GABA, in a region-dependent manner. While hippocampal astrocytes predominantly release glutamate (Woo et al., 2012), putatively due to a lack of GABA content (Yoon et al., 2011), cerebellar astrocytes do contain and release GABA (Lee et al., 2010).
Generalizing the gathered evidence, stimulation of the same astroglial receptor can lead to the release of different gliotransmitters. Stimulation of different receptors can have the same output and the same gliotransmitter can finally have different actions on the network. This paradoxical feature of astrocytes can be partially resolved by dissecting the information processing steps attributed to the astrocyte function itself or the context it is embedded in.
Astroglial Ca2+ Bottleneck: Upstream and Downstream Signal Discrimination and Processing
Astrocyte-mediated neuronal plasticity starts with the detection and discrimination of a stimulus. At the multipartite synapse, astroglial processes frequently interact with GABAergic interneurons and glutamatergic principal neurons. Specifically, cortical as well as hippocampal astroglia have the capacity to engage in interneuron-type specific interactions. Remarkably, they are more responsive to somatostatin compared to parvalbumin interneuron stimulation, based on the co-release of the neuropeptide somatostatin (Mariotti et al., 2018). Subsequently, the selective sensitivity to somatostatin interneurons is integrated and translated into an astroglia-mediated up-regulation of the synaptic inhibition of pyramidal neurons (Matos et al., 2018). Moreover, neuronal activity is also decoded in spatiotemporal patterns of circuit association, firing duration and frequency. Astrocytes are capable of discriminating synaptic activity of different neuronal circuits within the hippocampus, even if the same transmitter is released. This is likely due to distinct patterns of receptor expression and the existence of subcellular functional domains (Perea and Araque, 2005; Shigetomi et al., 2008). In the hippocampus, neuronal firing rates can be correlated with astroglial Ca2+ signals, i.e., high frequency synaptic activity depresses and low frequency increases Ca2+ signals (Perea and Araque, 2005). Moreover, neuronal firing patterns lead to distinct gliotransmitter release profiles, differentially affecting the neuronal network. While low or brief interneuron stimulation evokes glutamate release, high or sustained interneuron stimulation provokes the co-release of glutamate and purines (Covelo and Araque, 2018).
At the core of the astroglial black box, intracellular Ca2+-signals remain loosely defined. Even though a variety of Ca2+ signals have been mapped according to their spatiotemporal properties, still analysis and interpretation remain a major challenge (Nimmerjahn et al., 2009; Khakh and Sofroniew, 2015; Rusakov, 2015; Bazargani and Attwell, 2016; Bindocci et al., 2017; Stobart et al., 2018; Semyanov et al., 2020). So far, distinct Ca2+-signals have not been associated to specific intracellular signaling cascades or the nature of synaptic plasticity. The current consensus, however, states that spatially restrained Ca2+ signals will likely induce homosynaptic modulation while larger, propagating Ca2+ signals intervene in heterosynaptic and territorial synaptic plasticity (Araque et al., 2014). In particular, the integrative function of astrocytes, i.e., the computation of simultaneously converging signaling pathways in a probably non-linear fashion, remains to be elucidated (Durkee and Araque, 2019). Similarly, only few classes of Ca2+ signals could be clearly attributed to a functional correlate such as sensory stimulation (Wang et al., 2009; Stobart et al., 2018) or locomotion and startle responses (Paukert et al., 2014).
Downstream of intracellular Ca2+ signal integration, astrocytes can modulate gliotransmitter release and thereby determine the final impact on neuronal plasticity. Recent work supports the concept that the same astrocyte can release different gliotransmitters based on the existence of individual vesicle populations containing different gliotransmitters, operating through distinct v-SNARE proteins and oppositely regulating synaptic plasticity (Schwarz et al., 2017; Covelo and Araque, 2018). A further level of complexity arises from the coexistence of different mechanisms of gliotransmission with specific Ca2+-dependency. Vesicular release of gliotransmitters is threshold-based and requires relatively high intracellular Ca2+ elevations (Kreft et al., 2003; Parpura and Zorec, 2010). Ca2+-dependent opening of large conductance anion channels like Best1 is sigmoidal and has an EC50 for [Ca2+]i of about 150 nM (Lee et al., 2010), thus making it a suitable mechanism of gliotransmitter release even at resting conditions or in response to minute Ca2+ elevations (Clapham, 2007).
Finally, the net outcome of astroglial black box operations depends on the context of targeted neuronal receptors and the connectivity of the local circuitry. Especially in glutamatergic and purinergic transmission, the stimulus nature can be switched if presynaptic rather than postsynaptic receptors are activated (Serrano et al., 2006; Andersson et al., 2007; Hulme et al., 2014; Cavaccini et al., 2020). The connectivity of local circuits plays a major role when inhibitory transmission is potentiated by an excitatory transmitter (Kang et al., 1998; Crosby et al., 2018; Mederos and Perea, 2019), inhibitory transmission is inhibited, or excitatory transmission is disinhibited (Liu et al., 2004; Yarishkin et al., 2015). Associated to specific local circuits, distinct astroglial subpopulations (Zhang and Barres, 2010; Liddelow et al., 2017; Morel et al., 2017) interact with designated neuronal subtypes or circuits such as the striatal dopaminergic D1/D2 pathways (Martín et al., 2015; Martin-Fernandez et al., 2017). Given the kaleidoscopic complexity added to brain and network function, the astroglial syncytium holds a multitude of targets to be explored in health and disease.
Aberrant Ca2+ Signaling and Network Excitability Disruption in Epilepsy
The (im-) balance of E/I is a central element in the pathophysiology of epilepsy. More specifically, large populations of neurons become hyperexcitable and are more likely to engage in synchronous firing episodes. Accordingly, a great proportion of anti-epileptic drugs aim at enhancing GABA-mediated inhibition and decreasing glutamate-mediated excitation (Treiman, 2001; Czapiński et al., 2005). Astrocytes are portrayed as key players in epilepsy due to their diverse roles in the modulation of neuronal excitability and synchrony (Coulter and Steinhäuser, 2015; Robel and Sontheimer, 2016). A central property in this context is Ca2+-dependent astroglial glutamate release, driving NMDA receptor-induced neuronal excitation and favoring neuronal synchrony (Parri et al., 2001; Angulo et al., 2004; Fellin et al., 2004; Tian et al., 2005; Poskanzer and Yuste, 2011; Sasaki et al., 2014). Importantly, astroglial Ca2+ signals themselves are generally perturbed under neuropathological conditions (Kuchibhotla et al., 2009; Jiang et al., 2016; Mizuno et al., 2018; Shigetomi et al., 2019). Tonic long-lasting Ca2+ elevations result at least partially from excitotoxic spilling of glutamate, GABA and ATP from dying cells (Shigetomi et al., 2019).
Astrocyte (dys-) functions have been causally linked to the etiology of TLE (Bedner et al., 2015; Steinhäuser et al., 2016; Deshpande et al., 2020). On a cellular and molecular level, this is paralleled by a selective vulnerability of GABAergic interneurons (Sanon et al., 2005; Upadhya et al., 2019) and reduced glutamate decarboxylase (GAD) as well as glutamine synthetase (GS) activity in astrocytes (Robel and Sontheimer, 2016; Chan et al., 2019), leading to reduced vesicular GABA levels in neurons and subsequently reduced inhibitory input on hippocampal pyramidal neurons (Liang et al., 2006). However, glutamate levels also increase (Luna-Munguia et al., 2011) and a re-emergence of astroglial mGluR5 receptors can be observed (Umpierre et al., 2019). Recent lines of evidence also support a role for purinergic signaling in the etiology and progression of epilepsy and therefore further suggest that astrocytes actively contribute to this pathological scenario as modulators of the ATP/adenosine signaling (Boison, 2016; Beamer et al., 2017; Boison and Steinhäuser, 2018). Astroglial Ca2+ signals are pivotal for understanding the pathophysiology of epilepsy (Carmignoto and Haydon, 2012). Ex vivo, focal, seizure-like discharge onset and maintenance are associated with substantial astroglial Ca2+ transients, generating recurrent excitatory loops with neurons (Gómez-Gonzalo et al., 2010; Álvarez-Ferradas et al., 2015). In vivo, astroglial Ca2+ signals promote the propagation of epileptiform activity in the hippocampus. In fact, at seizure onset astrocytes display Ca2+ elevations prior to neurons and the suppression of those preceeding signals in Itpr2–/– mice reduces seizure activity (Heuser et al., 2018). These data suggest a modulatory, pro-epileptic effect of astroglial Ca2+ signals (Fellin et al., 2006; Heuser et al., 2018). Finally, seizure termination by spreading depolarization (SD) is accompanied by large Ca2+ waves in astrocytes, whose importance has been largely overlooked so far (Seidel et al., 2016; Heuser et al., 2018).
As it is for TLE, both glutamatergic- and GABAergic signaling are intimately involved in the generation and spreading of slow-wave discharges (SWDs) normally occurring in the thalamocortical network during sleep and pathologically in absence epilepsy (Tanaka et al., 1997; Tancredi et al., 2000; Crunelli and Leresche, 2002; Melø et al., 2006; Gould et al., 2014). In vivo astroglial Ca2+ activity temporally precedes the rhythmic and synchronized neocortical slow oscillations (∼1 Hz) which are typical for sleep. Indeed, optogenetic activation of astrocytes can induce this slow-oscillation state, possibly through glutamate transients (Poskanzer and Yuste, 2016). In line with this, neuronal firing reliably follows astroglial network synchronization during slow-wave activity. The fraction of astrocytes and neurons involved in the synchronous slow-wave state decreases both after astroglial uncoupling and intracellular Ca2+ chelation using EGTA (Szabó et al., 2017). Moreover, astroglial Ca2+ signaling is involved in the in vivo mGluR2-dependent disinhibition of neurons of the thalamic ventro-basal nucleus, thus playing a key role in sensory information processing (Copeland et al., 2017). On the other hand, dampening of astroglial Ca2+ oscillations in the striatum was linked to the upregulation of GAT3 expression and the resulting GABA uptake, thus reducing tonic inhibition and exacerbating neuronal excitability (Yu et al., 2018). The role of astrocytic Ca2+ transients in network synchronization and pathology is therefore still controversial.
Conclusion
Neuronal network plasticity can be simplified in terms of E/I, represented by glutamate and GABA, respectively. Including the discriminatory and integrative function of astrocytes into the equation reveals a profound entanglement of E/I, making these traditional labels insufficient. Astrocytes can preserve (congruence) or reverse (incongruence) neuronal inputs based on the engagement of distinct receptor arrays as well as the integration of converging Ca2+ signaling cascades and orchestrated gliotransmitter release. Furthermore, the association of astroglial subpopulations to specific neuronal circuits adds a further layer of complexity. To date, further efforts are required in order to understand astroglial black box operations and link them to Ca2+ signal heterogeneity. Advances in decoding astroglial Ca2+ signaling could reveal the untapped therapeutic potential in pathologies emerging from network excitability dysregulation, as suggested by the collectively acknowledged role of astroglial Ca2+ in epilepsy.
Author Contributions
LC and DG equally contributed to the review, screened the literature, wrote the first draft, conceptualized the table, designed the figure with AS, and finalized the manuscript. AS and FK reviewed and finalized manuscript and figure. All authors approved on the final version of the manuscript.
Funding
This project has received funding from the European Union’s Horizon 2020 Research and Innovation Programme under the Marie Skłodowska-Curie grant agreement No. 722053 and from the Deutsche Forschungsgemeinschaft DFG (SFB 894, SFB 1158, SPP 1757, FOR 2289, and Open Access Publishing funds).
Conflict of Interest
The authors declare that the research was conducted in the absence of any commercial or financial relationships that could be construed as a potential conflict of interest.
Acknowledgments
We thank the members of the department for fruitful discussions, particularly Wenhui Huang and Jens Grosche (Effigos AG) for his contribution to Figure 1A.
References
Abdullaev, I. F., Rudkouskaya, A., Schools, G. P., Kimelberg, H. K., and Mongin, A. A. (2006). Pharmacological comparison of swelling-activated excitatory amino acid release and Cl- currents in cultured rat astrocytes. J. Physiol. 572, 677–689. doi: 10.1113/jphysiol.2005.103820
Agarwal, A., Wu, P. H., Hughes, E. G., Fukaya, M., Tischfield, M. A., Langseth, A. J., et al. (2017). Transient opening of the mitochondrial permeability transition pore induces microdomain calcium transients in astrocyte processes. Neuron 93, 587–605.e7.
Álvarez-Ferradas, C., Morales, J. C., Wellmann, M., Nualart, F., Roncagliolo, M., Fuenzalida, M., et al. (2015). Enhanced astroglial Ca2+ signaling increases excitatory synaptic strength in the epileptic brain. Glia 63, 1507–1521. doi: 10.1002/glia.22817
Andersson, M., Blomstrand, F., and Hanse, E. (2007). Astrocytes play a critical role in transient heterosynaptic depression in the rat hippocampal CA1 region. J. Physiol. 585, 843–852. doi: 10.1113/jphysiol.2007.142737
Angulo, M. C., Kozlov, A. S., Charpak, S., and Audinat, E. (2004). Glutamate released from glial cells synchronizes neuronal activity in the hippocampus. J. Neurosci. 24, 6920–6927. doi: 10.1523/jneurosci.0473-04.2004
Araque, A., Carmignoto, G., Haydon, P. G., Oliet, S. H., Robitaille, R., and Volterra, A. (2014). Gliotransmitters travel in time and space. Neuron 81, 728–739. doi: 10.1016/j.neuron.2014.02.007
Araque, A., and Navarrete, M. (2010). Glial cells in neuronal network function. Philos. Trans. R. Soc. Lond. B Biol. Sci. 365, 2375–2381. doi: 10.1098/rstb.2009.0313
Araque, A., Parpura, V., Sanzgiri, R. P., and Haydon, P. G. (1999). Tripartite synapses: glia, the unacknowledged partner. Trends Neurosci. 22, 208–215. doi: 10.1016/s0166-2236(98)01349-6
Aronica, E., Gorter, J. A., Ijlst-Keizers, H., Rozemuller, A. J., Yankaya, B., Leenstra, S., et al. (2003). Expression and functional role of mGluR3 and mGluR5 in human astrocytes and glioma cells: opposite regulation of glutamate transporter proteins. Eur. J. Neurosci. 17, 2106–2118. doi: 10.1046/j.1460-9568.2003.02657.x
Barakat, L., and Bordey, A. (2002). GAT-1 and reversible GABA transport in Bergmann glia in slices. J. Neurophysiol. 88, 1407–1419. doi: 10.1152/jn.2002.88.3.1407
Bazargani, N., and Attwell, D. (2016). Astrocyte calcium signaling: the third wave. Nat. Neurosci. 19, 182–189. doi: 10.1038/nn.4201
Beamer, E., Fischer, W., and Engel, T. (2017). The ATP-Gated P2X7 receptor as a target for the treatment of drug-resistant epilepsy. Front. Neurosci. 11:21. doi: 10.3389/fnins.2017.00021
Bedner, P., Dupper, A., Hüttmann, K., Müller, J., Herde, M. K., Dublin, P., et al. (2015). Astrocyte uncoupling as a cause of human temporal lobe epilepsy. Brain 138, 1208–1222. doi: 10.1093/brain/awv067
Bezzi, P., Carmignoto, G., Pasti, L., Vesce, S., Rossi, D., Rizzini, B. L., et al. (1998). Prostaglandins stimulate calcium-dependent glutamate release in astrocytes. Nature 391, 281–285. doi: 10.1038/34651
Bezzi, P., Gundersen, V., Galbete, J. L., Seifert, G., Steinhäuser, C., Pilati, E., et al. (2004). Astrocytes contain a vesicular compartment that is competent for regulated exocytosis of glutamate. Nat. Neurosci. 7, 613–620. doi: 10.1038/nn1246
Bindocci, E., Savtchouk, I., Liaudet, N., Becker, D., Carriero, G., and Volterra, A. (2017). Three-dimensional Ca(2+) imaging advances understanding of astrocyte biology. Science 356:eaai8185. doi: 10.1126/science.aai8185
Boddum, K., Jensen, T. P., Magloire, V., Kristiansen, U., Rusakov, D. A., Pavlov, I., et al. (2016). Astrocytic GABA transporter activity modulates excitatory neurotransmission. Nat. Commun. 7:13572.
Bohmbach, K., Schwarz, M. K., Schoch, S., and Henneberger, C. (2018). The structural and functional evidence for vesicular release from astrocytes in situ. Brain Res. Bull. 136, 65–75. doi: 10.1016/j.brainresbull.2017.01.015
Boison, D. (2016). Adenosinergic signaling in epilepsy. Neuropharmacology 104, 131–139. doi: 10.1016/j.neuropharm.2015.08.046
Boison, D., and Steinhäuser, C. (2018). Epilepsy and astrocyte energy metabolism. Glia 66, 1235–1243. doi: 10.1002/glia.23247
Bowser, D. N., and Khakh, B. S. (2007). Vesicular ATP is the predominant cause of intercellular calcium waves in astrocytes. J. Gen. Physiol. 129, 485–491. doi: 10.1085/jgp.200709780
Carmignoto, G., and Haydon, P. G. (2012). Astrocyte calcium signaling and epilepsy. Glia 60, 1227–1233. doi: 10.1002/glia.22318
Cavaccini, A., Durkee, C., Kofuji, P., Tonini, R., and Araque, A. (2020). Astrocyte signaling gates long-term depression at corticostriatal synapses of the direct pathway. J. Neurosci. 40, 5757–5768. doi: 10.1523/jneurosci.2369-19.2020
Cavelier, P., and Attwell, D. (2005). Tonic release of glutamate by a DIDS-sensitive mechanism in rat hippocampal slices. J. Physiol. 564, 397–410. doi: 10.1113/jphysiol.2004.082131
Chan, F., Lax, N. Z., Voss, C. M., Aldana, B. I., Whyte, S., Jenkins, A., et al. (2019). The role of astrocytes in seizure generation: insights from a novel in vitro seizure model based on mitochondrial dysfunction. Brain 142, 391–411. doi: 10.1093/brain/awy320
Chen, J., Tan, Z., Zeng, L., Zhang, X., He, Y., Gao, W., et al. (2013). Heterosynaptic long-term depression mediated by ATP released from astrocytes. Glia 61, 178–191. doi: 10.1002/glia.22425
Copeland, C. S., Wall, T. M., Sims, R. E., Neale, S. A., Nisenbaum, E., Parri, H. R., et al. (2017). Astrocytes modulate thalamic sensory processing via mGlu2 receptor activation. Neuropharmacology 121, 100–110. doi: 10.1016/j.neuropharm.2017.04.019
Coulter, D. A., and Steinhäuser, C. (2015). Role of astrocytes in epilepsy. Cold Spring Harb. Perspect. Med. 5:a022434.
Covelo, A., and Araque, A. (2018). Neuronal activity determines distinct gliotransmitter release from a single astrocyte. eLife 7:e32237.
Crosby, K. M., Murphy-Royal, C., Wilson, S. A., Gordon, G. R., Bains, J. S., and Pittman, Q. J. (2018). Cholecystokinin switches the plasticity of GABA synapses in the dorsomedial hypothalamus via astrocytic ATP release. J. Neurosci. 38, 8515–8525. doi: 10.1523/jneurosci.0569-18.2018
Crunelli, V., and Leresche, N. (2002). Childhood absence epilepsy: genes, channels, neurons and networks. Nat. Rev. Neurosci. 3, 371–382. doi: 10.1038/nrn811
Czapiński, P., Blaszczyk, B., and Czuczwar, S. J. (2005). Mechanisms of action of antiepileptic drugs. Curr. Top. Med. Chem. 5, 3–14.
Das, A., Wallace, G. C., Holmes, C., McDowell, M. L., Smith, J. A., Marshall, J. D., et al. (2012). Hippocampal tissue of patients with refractory temporal lobe epilepsy is associated with astrocyte activation, inflammation, and altered expression of channels and receptors. Neuroscience 220, 237–246. doi: 10.1016/j.neuroscience.2012.06.002
D’Ascenzo, M., Fellin, T., Terunuma, M., Revilla-Sanchez, R., Meaney, D. F., Auberson, Y. P., et al. (2007). mGluR5 stimulates gliotransmission in the nucleus accumbens. Proc. Natl. Acad. Sci. U.S.A. 104, 1995–2000. doi: 10.1073/pnas.0609408104
Deshpande, T., Li, T., Henning, L., Wu, Z., Müller, J., Seifert, G., et al. (2020). Constitutive deletion of astrocytic connexins aggravates kainate-induced epilepsy. Glia 68, 2136–2147. doi: 10.1002/glia.23832
Ding, S., Fellin, T., Zhu, Y., Lee, S.-Y., Auberson, Y. P., Meaney, D. F., et al. (2007). Enhanced astrocytic Ca2+ signals contribute to neuronal excitotoxicity after status epilepticus. J. Neurosci. 27, 10674–10684. doi: 10.1523/jneurosci.2001-07.2007
Doengi, M., Hirnet, D., Coulon, P., Pape, H. C., Deitmer, J. W., and Lohr, C. (2009). GABA uptake-dependent Ca(2+) signaling in developing olfactory bulb astrocytes. Proc. Natl. Acad. Sci. U.S.A. 106, 17570–17575. doi: 10.1073/pnas.0809513106
Duan, S., Anderson, C. M., Keung, E. C., Chen, Y., and Swanson, R. A. (2003). P2X7 receptor-mediated release of excitatory amino acids from astrocytes. J. Neurosci. 23, 1320–1328. doi: 10.1523/jneurosci.23-04-01320.2003
Durkee, C. A., and Araque, A. (2019). Diversity and specificity of astrocyte-neuron communication. Neuroscience 396, 73–78. doi: 10.1016/j.neuroscience.2018.11.010
Durkee, C. A., Covelo, A., Lines, J., Kofuji, P., Aguilar, J., and Araque, A. (2019). Gi/o protein-coupled receptors inhibit neurons but activate astrocytes and stimulate gliotransmission. Glia 67, 1076–1093. doi: 10.1002/glia.23589
Fellin, T., Gomez-Gonzalo, M., Gobbo, S., Carmignoto, G., and Haydon, P. G. (2006). Astrocytic glutamate is not necessary for the generation of epileptiform neuronal activity in hippocampal slices. J. Neurosci. 26, 9312–9322. doi: 10.1523/jneurosci.2836-06.2006
Fellin, T., Pascual, O., Gobbo, S., Pozzan, T., Haydon, P. G., and Carmignoto, G. (2004). Neuronal synchrony mediated by astrocytic glutamate through activation of extrasynaptic NMDA receptors. Neuron 43, 729–743. doi: 10.1016/j.neuron.2004.08.011
Gómez-Gonzalo, M., Losi, G., Chiavegato, A., Zonta, M., Cammarota, M., Brondi, M., et al. (2010). An excitatory loop with astrocytes contributes to drive neurons to seizure threshold. PLoS Biol. 8:e1000352. doi: 10.1371/journal.pbio.1000352
Gómez-Gonzalo, M., Martin-Fernandez, M., Martínez-Murillo, R., Mederos, S., Hernández-Vivanco, A., Jamison, S., et al. (2017). Neuron-astrocyte signaling is preserved in the aging brain. Glia 65, 569–580. doi: 10.1002/glia.23112
Gould, T., Chen, L., Emri, Z., Pirttimaki, T., Errington, A. C., Crunelli, V., et al. (2014). GABA(B) receptor-mediated activation of astrocytes by gamma-hydroxybutyric acid. Philos. Trans. R. Soc. Lond. B Biol. Sci. 369:20130607. doi: 10.1098/rstb.2013.0607
Guerra-Gomes, S., Sousa, N., Pinto, L., and Oliveira, J. F. (2017). Functional roles of astrocyte calcium elevations: from synapses to behavior. Front. Cell Neurosci. 11:427. doi: 10.3389/fncel.2017.00427
Haustein, M. D., Kracun, S., Lu, X. H., Shih, T., Jackson-Weaver, O., Tong, X., et al. (2014). Conditions and constraints for astrocyte calcium signaling in the hippocampal mossy fiber pathway. Neuron 82, 413–429. doi: 10.1016/j.neuron.2014.02.041
Héja, L., Nyitrai, G., Kékesi, O., Dobolyi, A., Szabó, P., Fiáth, R., et al. (2012). Astrocytes convert network excitation to tonic inhibition of neurons. BMC Biol. 10:26. doi: 10.1186/1741-7007-10-26
Henneberger, C., Papouin, T., Oliet, S. H., and Rusakov, D. A. (2010). Long-term potentiation depends on release of D-serine from astrocytes. Nature 463, 232–236. doi: 10.1038/nature08673
Heuser, K., Nome, C. G., Pettersen, K. H., Åbjørsbråten, K. S., Jensen, V., Tang, W., et al. (2018). Ca2+ signals in astrocytes facilitate spread of epileptiform activity. Cereb. Cortex 28, 4036–4048. doi: 10.1093/cercor/bhy196
Huang, Y., and Thathiah, A. (2015). Regulation of neuronal communication by G protein-coupled receptors. FEBS Lett. 589, 1607–1619. doi: 10.1016/j.febslet.2015.05.007
Hulme, S. R., Jones, O. D., Raymond, C. R., Sah, P., and Abraham, W. C. (2014). Mechanisms of heterosynaptic metaplasticity. Philos. Trans. R. Soc. Lond. B Biol. Sci. 369:20130148. doi: 10.1098/rstb.2013.0148
Isaacson, J. S., and Scanziani, M. (2011). How inhibition shapes cortical activity. Neuron 72, 231–243. doi: 10.1016/j.neuron.2011.09.027
Jeftinija, S. D., Jeftinija, K. V., and Stefanovic, G. (1997). Cultured astrocytes express proteins involved in vesicular glutamate release. Brain Res. 750, 41–47. doi: 10.1016/s0006-8993(96)00610-5
Jennings, A., Tyurikova, O., Bard, L., Zheng, K., Semyanov, A., Henneberger, C., et al. (2017). Dopamine elevates and lowers astroglial Ca. Glia 65, 447–459. doi: 10.1002/glia.23103
Jiang, R., Diaz-Castro, B., Looger, L. L., and Khakh, B. S. (2016). Dysfunctional calcium and glutamate signaling in striatal astrocytes from Huntington’s disease model mice. J. Neurosci. 36, 3453–3470. doi: 10.1523/jneurosci.3693-15.2016
Jiménez-González, C., Pirttimaki, T., Cope, D. W., and Parri, H. R. (2011). Non-neuronal, slow GABA signalling in the ventrobasal thalamus targets δ-subunit-containing GABA(A) receptors. Eur. J. Neurosci. 33, 1471–1482. doi: 10.1111/j.1460-9568.2011.07645.x
Jo, S., Yarishkin, O., Hwang, Y. J., Chun, Y. E., Park, M., Woo, D. H., et al. (2014). GABA from reactive astrocytes impairs memory in mouse models of Alzheimer’s disease. Nat. Med. 20, 886–896. doi: 10.1038/nm.3639
Kang, J., Jiang, L., Goldman, S. A., and Nedergaard, M. (1998). Astrocyte-mediated potentiation of inhibitory synaptic transmission. Nat. Neurosci. 1, 683–692. doi: 10.1038/3684
Khakh, B. S., and Sofroniew, M. V. (2015). Diversity of astrocyte functions and phenotypes in neural circuits. Nat. Neurosci. 18, 942–952. doi: 10.1038/nn.4043
Kimelberg, H. K., Goderie, S. K., Higman, S., Pang, S., and Waniewski, R. A. (1990). Swelling-induced release of glutamate, aspartate, and taurine from astrocyte cultures. J. Neurosci. 10, 1583–1591. doi: 10.1523/jneurosci.10-05-01583.1990
Kirchhoff, F. (2017). Analysis of functional NMDA receptors in astrocytes. Methods Mol. Biol. 1677, 241–251. doi: 10.1007/978-1-4939-7321-7_13
Kofuji, P., and Araque, A. (2020). G-protein-coupled receptors in astrocyte-neuron communication. Neuroscience [Epub ahead of print],
Kozlov, A. S., Angulo, M. C., Audinat, E., and Charpak, S. (2006). Target cell-specific modulation of neuronal activity by astrocytes. Proc. Natl. Acad. Sci. U.S.A. 103, 10058–10063. doi: 10.1073/pnas.0603741103
Kreft, M., Stenovec, M., Rupnik, M., Grilc, S., Krzan, M., Milisav, I., et al. (2003). Calcium-dependent exocytosis in cultured astrocytes. Glia 46, 437–445.
Kuchibhotla, K. V., Lattarulo, C. R., Hyman, B. T., and Bacskai, B. J. (2009). Synchronous hyperactivity and intercellular calcium waves in astrocytes in Alzheimer mice. Science 323, 1211–1215. doi: 10.1126/science.1169096
Lalo, U., Palygin, O., North, R. A., Verkhratsky, A., and Pankratov, Y. (2011). Age-dependent remodelling of ionotropic signalling in cortical astroglia. Aging Cell 10, 392–402. doi: 10.1111/j.1474-9726.2011.00682.x
Lalo, U., Palygin, O., Rasooli-Nejad, S., Andrew, J., Haydon, P. G., and Pankratov, Y. (2014). Exocytosis of ATP from astrocytes modulates phasic and tonic inhibition in the neocortex. PLoS Biol. 12:e1001747. doi: 10.1371/journal.pbio.1001747
Lalo, U., Palygin, O., Verkhratsky, A., Grant, S. G., and Pankratov, Y. (2016). ATP from synaptic terminals and astrocytes regulates NMDA receptors and synaptic plasticity through PSD-95 multi-protein complex. Sci. Rep. 6:33609.
Lalo, U., Pankratov, Y., Kirchhoff, F., North, R. A., and Verkhratsky, A. (2006). NMDA receptors mediate neuron-to-glia signaling in mouse cortical astrocytes. J. Neurosci. 26, 2673–2683. doi: 10.1523/jneurosci.4689-05.2006
Le Meur, K., Mendizabal-Zubiaga, J., Grandes, P., and Audinat, E. (2012). GABA release by hippocampal astrocytes. Front. Comput. Neurosci. 6:59. doi: 10.3389/fncom.2012.00059
Lee, M., Schwab, C., and McGeer, P. L. (2011). Astrocytes are GABAergic cells that modulate microglial activity. Glia 59, 152–165. doi: 10.1002/glia.21087
Lee, S., Yoon, B. E., Berglund, K., Oh, S. J., Park, H., Shin, H. S., et al. (2010). Channel-mediated tonic GABA release from glia. Science 330, 790–796. doi: 10.1126/science.1184334
Letellier, M., Park, Y. K., Chater, T. E., Chipman, P. H., Gautam, S. G., Oshima-Takago, T., et al. (2016). Astrocytes regulate heterogeneity of presynaptic strengths in hippocampal networks. Proc. Natl. Acad. Sci. U.S.A. 113, E2685–E2694.
Liang, S. L., Carlson, G. C., and Coulter, D. A. (2006). Dynamic regulation of synaptic GABA release by the glutamate-glutamine cycle in hippocampal area CA1. J. Neurosci. 26, 8537–8548. doi: 10.1523/jneurosci.0329-06.2006
Liddelow, S. A., Guttenplan, K. A., Clarke, L. E., Bennett, F. C., Bohlen, C. J., Schirmer, L., et al. (2017). Neurotoxic reactive astrocytes are induced by activated microglia. Nature 541, 481–487.
Liu, H. T., Tashmukhamedov, B. A., Inoue, H., Okada, Y., and Sabirov, R. Z. (2006). Roles of two types of anion channels in glutamate release from mouse astrocytes under ischemic or osmotic stress. Glia 54, 343–357. doi: 10.1002/glia.20400
Liu, Q.-S., Xu, Q., Kang, J., and Nedergaard, M. (2004). Astrocyte activation of presynaptic metabotropic glutamate receptors modulates hippocampal inhibitory synaptic transmission. Neuron Glia Biol. 1, 307–316. doi: 10.1017/s1740925x05000190
Liu, Q. Y., Schaffner, A. E., Chang, Y. H., Maric, D., and Barker, J. L. (2000). Persistent activation of GABA(A) receptor/Cl(-) channels by astrocyte-derived GABA in cultured embryonic rat hippocampal neurons. J. Neurophysiol. 84, 1392–1403. doi: 10.1152/jn.2000.84.3.1392
Longuemare, M. C., and Swanson, R. A. (1997). Net glutamate release from astrocytes is not induced by extracellular potassium concentrations attainable in brain. J. Neurochem. 69, 879–882. doi: 10.1046/j.1471-4159.1997.69020879.x
Luna-Munguia, H., Orozco-Suarez, S., and Rocha, L. (2011). Effects of high frequency electrical stimulation and R-verapamil on seizure susceptibility and glutamate and GABA release in a model of phenytoin-resistant seizures. Neuropharmacology 61, 807–814. doi: 10.1016/j.neuropharm.2011.05.027
Mariotti, L., Losi, G., Lia, A., Melone, M., Chiavegato, A., Gómez-Gonzalo, M., et al. (2018). Interneuron-specific signaling evokes distinctive somatostatin-mediated responses in adult cortical astrocytes. Nat. Commun. 9:82.
Mariotti, L., Losi, G., Sessolo, M., Marcon, I., and Carmignoto, G. (2016). The inhibitory neurotransmitter GABA evokes long-lasting Ca(2+) oscillations in cortical astrocytes. Glia 64, 363–373. doi: 10.1002/glia.22933
Martín, R., Bajo-Grañeras, R., Moratalla, R., Perea, G., and Araque, A. (2015). Circuit-specific signaling in astrocyte-neuron networks in basal ganglia pathways. Science 349, 730–734. doi: 10.1126/science.aaa7945
Martin-Fernandez, M., Jamison, S., Robin, L. M., Zhao, Z., Martin, E. D., Aguilar, J., et al. (2017). Synapse-specific astrocyte gating of amygdala-related behavior. Nat. Neurosci. 20, 1540–1548. doi: 10.1038/nn.4649
Matos, M., Bosson, A., Riebe, I., Reynell, C., Vallée, J., Laplante, I., et al. (2018). Astrocytes detect and upregulate transmission at inhibitory synapses of somatostatin interneurons onto pyramidal cells. Nat. Commun. 9:4254.
Matthias, K., Kirchhoff, F., Seifert, G., Hüttmann, K., Matyash, M., Kettenmann, H., et al. (2003). Segregated expression of AMPA-type glutamate receptors and glutamate transporters defines distinct astrocyte populations in the mouse hippocampus. J. Neurosci. 23, 1750–1758. doi: 10.1523/jneurosci.23-05-01750.2003
Mederos, S., and Perea, G. (2019). GABAergic-astrocyte signaling: a refinement of inhibitory brain networks. Glia 67, 1842–1851. doi: 10.1002/glia.23644
Meier, S. D., Kafitz, K. W., and Rose, C. R. (2008). Developmental profile and mechanisms of GABA-induced calcium signaling in hippocampal astrocytes. Glia 56, 1127–1137. doi: 10.1002/glia.20684
Melø, T. M., Sonnewald, U., Touret, M., and Nehlig, A. (2006). Cortical glutamate metabolism is enhanced in a genetic model of absence epilepsy. J. Cereb. Blood Flow Metab. 26, 1496–1506. doi: 10.1038/sj.jcbfm.9600300
Mizuno, G. O., Wang, Y., Shi, G., Sun, J., Papadopoulos, S., Broussard, G. J., et al. (2018). Aberrant calcium signaling in astrocytes inhibits neuronal excitability in a human down syndrome stem cell model. Cell Rep. 24, 355–365. doi: 10.1016/j.celrep.2018.06.033
Mongin, A. A., and Kimelberg, H. K. (2005). ATP regulates anion channel-mediated organic osmolyte release from cultured rat astrocytes via multiple Ca2+-sensitive mechanisms. Am. J. Physiol. Cell Physiol. 288, C204–C213.
Montana, V., Malarkey, E. B., Verderio, C., Matteoli, M., and Parpura, V. (2006). Vesicular transmitter release from astrocytes. Glia 54, 700–715. doi: 10.1002/glia.20367
Morel, L., Chiang, M. S. R., Higashimori, H., Shoneye, T., Iyer, L. K., Yelick, J., et al. (2017). Molecular and functional properties of regional astrocytes in the adult brain. J. Neurosci. 37, 8706–8717. doi: 10.1523/jneurosci.3956-16.2017
Nagai, J., Rajbhandari, A. K., Gangwani, M. R., Hachisuka, A., Coppola, G., Masmanidis, S. C., et al. (2019). Hyperactivity with disrupted attention by activation of an astrocyte synaptogenic cue. Cell 177, 1280–1292.e20.
Nett, W. J., Oloff, S. H., and McCarthy, K. D. (2002). Hippocampal astrocytes in situ exhibit calcium oscillations that occur independent of neuronal activity. J. Neurophysiol. 87, 528–537. doi: 10.1152/jn.00268.2001
Nilsson, M., Eriksson, P. S., Rönnbäck, L., and Hansson, E. (1993). GABA induces Ca2+ transients in astrocytes. Neuroscience 54, 605–614. doi: 10.1016/0306-4522(93)90232-5
Nimmerjahn, A., Mukamel, E. A., and Schnitzer, M. J. (2009). Motor behavior activates Bergmann glial networks. Neuron 62, 400–412. doi: 10.1016/j.neuron.2009.03.019
Palygin, O., Lalo, U., Verkhratsky, A., and Pankratov, Y. (2010). Ionotropic NMDA and P2X1/5 receptors mediate synaptically induced Ca2+ signalling in cortical astrocytes. Cell Calcium 48, 225–231. doi: 10.1016/j.ceca.2010.09.004
Panatier, A., and Robitaille, R. (2016). Astrocytic mGluR5 and the tripartite synapse. Neuroscience 323, 29–34. doi: 10.1016/j.neuroscience.2015.03.063
Panatier, A., Vallée, J., Haber, M., Murai, K. K., Lacaille, J. C., and Robitaille, R. (2011). Astrocytes are endogenous regulators of basal transmission at central synapses. Cell 146, 785–798. doi: 10.1016/j.cell.2011.07.022
Pandit, S., Neupane, C., Woo, J., Sharma, R., Nam, M. H., Lee, G. S., et al. (2020). Bestrophin1-mediated tonic GABA release from reactive astrocytes prevents the development of seizure-prone network in kainate-injected hippocampi. Glia 68, 1065–1080. doi: 10.1002/glia.23762
Park, H., Oh, S. J., Han, K. S., Woo, D. H., Mannaioni, G., Traynelis, S. F., et al. (2009). Bestrophin-1 encodes for the Ca2+-activated anion channel in hippocampal astrocytes. J. Neurosci. 29, 13063–13073. doi: 10.1523/jneurosci.3193-09.2009
Parpura, V., Basarsky, T. A., Liu, F., Jeftinija, K., Jeftinija, S., and Haydon, P. G. (1994). Glutamate-mediated astrocyte neuron signaling. Nature 369, 744–747.
Parpura, V., Grubišiæ, V., and Verkhratsky, A. (2011). Ca(2+) sources for the exocytotic release of glutamate from astrocytes. Biochim. Biophys. Acta 1813, 984–991. doi: 10.1016/j.bbamcr.2010.11.006
Parpura, V., and Zorec, R. (2010). Gliotransmission: exocytotic release from astrocytes. Brain Res. Rev. 63, 83–92. doi: 10.1016/j.brainresrev.2009.11.008
Parri, H. R., Gould, T. M., and Crunelli, V. (2001). Spontaneous astrocytic Ca2+ oscillations in situ drive NMDAR-mediated neuronal excitation. Nat. Neurosci. 4, 803–812. doi: 10.1038/90507
Pascual, O., Casper, K. B., Kubera, C., Zhang, J., Revilla-Sanchez, R., Sul, J. Y., et al. (2005). Astrocytic purinergic signaling coordinates synaptic networks. Science 310, 113–116. doi: 10.1126/science.1116916
Pasti, L., Volterra, A., Pozzan, T., and Carmignoto, G. (1997). Intracellular calcium oscillations in astrocytes: a highly plastic, bidirectional form of communication between neurons and astrocytes in situ. J. Neurosci. 17, 7817–7830. doi: 10.1523/jneurosci.17-20-07817.1997
Paukert, M., Agarwal, A., Cha, J., Doze, V. A., Kang, J. U., and Bergles, D. E. (2014). Norepinephrine controls astroglial responsiveness to local circuit activity. Neuron 82, 1263–1270. doi: 10.1016/j.neuron.2014.04.038
Perea, G., and Araque, A. (2005). Properties of synaptically evoked astrocyte calcium signal reveal synaptic information processing by astrocytes. J. Neurosci. 25, 2192–2203. doi: 10.1523/jneurosci.3965-04.2005
Perea, G., Gómez, R., Mederos, S., Covelo, A., Ballesteros, J. J., Schlosser, L., et al. (2016). Activity-dependent switch of GABAergic inhibition into glutamatergic excitation in astrocyte-neuron networks. eLife 5:e20362.
Pirttimaki, T. M., Hall, S. D., and Parri, H. R. (2011). Sustained neuronal activity generated by glial plasticity. J. Neurosci. 31, 7637–7647. doi: 10.1523/jneurosci.5783-10.2011
Poskanzer, K. E., and Yuste, R. (2011). Astrocytic regulation of cortical UP states. Proc. Natl. Acad. Sci. U.S.A. 108, 18453–18458. doi: 10.1073/pnas.1112378108
Poskanzer, K. E., and Yuste, R. (2016). Astrocytes regulate cortical state switching in vivo. Proc. Natl. Acad. Sci. U.S.A. 113, E2675–E2684.
Ramos-Mandujano, G., Vázquez-Juárez, E., Hernández-Benítez, R., and Pasantes-Morales, H. (2007). Thrombin potently enhances swelling-sensitive glutamate efflux from cultured astrocytes. Glia 55, 917–925. doi: 10.1002/glia.20513
Reyes-Haro, D., Müller, J., Boresch, M., Pivneva, T., Benedetti, B., Scheller, A., et al. (2010). Neuron-astrocyte interactions in the medial nucleus of the trapezoid body. J. Gen. Physiol. 135, 583–594. doi: 10.1085/jgp.200910354
Richerson, G. B., and Wu, Y. (2003). Dynamic equilibrium of neurotransmitter transporters: not just for reuptake anymore. J. Neurophysiol. 90, 1363–1374. doi: 10.1152/jn.00317.2003
Robel, S., and Sontheimer, H. (2016). Glia as drivers of abnormal neuronal activity. Nat. Neurosci. 19, 28–33. doi: 10.1038/nn.4184
Rossi, D. J., Oshima, T., and Attwell, D. (2000). Glutamate release in severe brain ischaemia is mainly by reversed uptake. Nature 403, 316–321. doi: 10.1038/35002090
Rusakov, D. A. (2015). Disentangling calcium-driven astrocyte physiology. Nat. Rev. Neurosci. 16, 226–233. doi: 10.1038/nrn3878
Saab, A. S., Neumeyer, A., Jahn, H. M., Cupido, A., Šimek, A. A., Boele, H. J., et al. (2012). Bergmann glial AMPA receptors are required for fine motor coordination. Science 337, 749–753. doi: 10.1126/science.1221140
Sanon, N., Carmant, L., Emond, M., Congar, P., and Lacaille, J. C. (2005). Short-term effects of kainic acid on CA1 hippocampal interneurons differentially vulnerable to excitotoxicity. Epilepsia 46, 837–848. doi: 10.1111/j.1528-1167.2005.21404.x
Sasaki, T., Ishikawa, T., Abe, R., Nakayama, R., Asada, A., Matsuki, N., et al. (2014). Astrocyte calcium signalling orchestrates neuronal synchronization in organotypic hippocampal slices. J. Physiol. 592, 2771–2783. doi: 10.1113/jphysiol.2014.272864
Sasaki, T., Kuga, N., Namiki, S., Matsuki, N., and Ikegaya, Y. (2011). Locally synchronized astrocytes. Cereb. Cortex 21, 1889–1900. doi: 10.1093/cercor/bhq256
Savtchouk, I., and Volterra, A. (2018). Gliotransmission: beyond black-and-white. J. Neurosci. 38, 14–25. doi: 10.1523/jneurosci.0017-17.2017
Schipke, C. G., Ohlemeyer, C., Matyash, M., Nolte, C., Kettenmann, H., and Kirchhoff, F. (2001). Astrocytes of the mouse neocortex express functional N-methyl-D-aspartate receptors. FASEB J. 15, 1270–1272. doi: 10.1096/fj.00-0439fje
Schummers, J., Yu, H., and Sur, M. (2008). Tuned responses of astrocytes and their influence on hemodynamic signals in the visual cortex. Science 320, 1638–1643. doi: 10.1126/science.1156120
Schwarz, Y., Zhao, N., Kirchhoff, F., and Bruns, D. (2017). Astrocytes control synaptic strength by two distinct v-SNARE-dependent release pathways. Nat. Neurosci. 20, 1529–1539. doi: 10.1038/nn.4647
Seidel, J. L., Escartin, C., Ayata, C., Bonvento, G., and Shuttleworth, C. W. (2016). Multifaceted roles for astrocytes in spreading depolarization: a target for limiting spreading depolarization in acute brain injury? Glia 64, 5–20. doi: 10.1002/glia.22824
Semyanov, A., Henneberger, C., and Agarwal, A. (2020). Making sense of astrocytic calcium signals - from acquisition to interpretation. Nat. Rev. Neurosci. 21, 551–564. doi: 10.1038/s41583-020-0361-8
Serrano, A., Haddjeri, N., Lacaille, J. C., and Robitaille, R. (2006). GABAergic network activation of glial cells underlies hippocampal heterosynaptic depression. J. Neurosci. 26, 5370–5382. doi: 10.1523/jneurosci.5255-05.2006
Serrano, A., Robitaille, R., and Lacaille, J. C. (2008). Differential NMDA-dependent activation of glial cells in mouse hippocampus. Glia 56, 1648–1663. doi: 10.1002/glia.20717
Sherwood, M. W., Arizono, M., Hisatsune, C., Bannai, H., Ebisui, E., Sherwood, J. L., et al. (2017). Astrocytic IP. Glia 65, 502–513.
Shigetomi, E., Bowser, D. N., Sofroniew, M. V., and Khakh, B. S. (2008). Two forms of astrocyte calcium excitability have distinct effects on NMDA receptor-mediated slow inward currents in pyramidal neurons. J. Neurosci. 28, 6659–6663. doi: 10.1523/jneurosci.1717-08.2008
Shigetomi, E., Bushong, E. A., Haustein, M. D., Tong, X., Jackson-Weaver, O., Kracun, S., et al. (2013). Imaging calcium microdomains within entire astrocyte territories and endfeet with GCaMPs expressed using adeno-associated viruses. J. Gen. Physiol. 141, 633–647. doi: 10.1085/jgp.201210949
Shigetomi, E., Saito, K., Sano, F., and Koizumi, S. (2019). Aberrant calcium signals in reactive astrocytes: a key process in neurological disorders. Int. J. Mol. Sci. 20:996. doi: 10.3390/ijms20040996
Shigetomi, E., Tong, X., Kwan, K. Y., Corey, D. P., and Khakh, B. S. (2011). TRPA1 channels regulate astrocyte resting calcium and inhibitory synapse efficacy through GAT-3. Nat. Neurosci. 15, 70–80. doi: 10.1038/nn.3000
Smith, N. A., Bekar, L. K., and Nedergaard, M. (2020). Astrocytic endocannabinoids mediate hippocampal transient heterosynaptic depression. Neurochem. Res. 45, 100–108. doi: 10.1007/s11064-019-02834-0
Srinivasan, R., Huang, B. S., Venugopal, S., Johnston, A. D., Chai, H., Zeng, H., et al. (2015). Ca(2+) signaling in astrocytes from Ip3r2(-/-) mice in brain slices and during startle responses in vivo. Nat. Neurosci. 18, 708–717. doi: 10.1038/nn.4001
Steinhäuser, C., Grunnet, M., and Carmignoto, G. (2016). Crucial role of astrocytes in temporal lobe epilepsy. Neuroscience 323, 157–169. doi: 10.1016/j.neuroscience.2014.12.047
Stobart, J. L., Ferrari, K. D., Barrett, M. J. P., Glück, C., Stobart, M. J., Zuend, M., et al. (2018). Cortical circuit activity evokes rapid astrocyte calcium signals on a similar timescale to neurons. Neuron 98, 726–735.e4.
Sun, M. Y., Devaraju, P., Xie, A. X., Holman, I., Samones, E., Murphy, T. R., et al. (2014). Astrocyte calcium microdomains are inhibited by bafilomycin A1 and cannot be replicated by low-level Schaffer collateral stimulation in situ. Cell Calcium 55, 1–16. doi: 10.1016/j.ceca.2013.10.004
Sun, W., McConnell, E., Pare, J.-F., Xu, Q., Chen, M., Peng, W., et al. (2013). Glutamate-dependent neuroglial calcium signaling differs between young and adult brain. Science 339, 197–200. doi: 10.1126/science.1226740
Szabó, Z., Héja, L., Szalay, G., Kékesi, O., Füredi, A., Szebényi, K., et al. (2017). Extensive astrocyte synchronization advances neuronal coupling in slow wave activity in vivo. Sci. Rep. 7:6018.
Tanaka, K., Watase, K., Manabe, T., Yamada, K., Watanabe, M., Takahashi, K., et al. (1997). Epilepsy and exacerbation of brain injury in mice lacking the glutamate transporter GLT-1. Science 276, 1699–1702. doi: 10.1126/science.276.5319.1699
Tancredi, V., Biagini, G., D’Antuono, M., Louvel, J., Pumain, R., and Avoli, M. (2000). Spindle-like thalamocortical synchronization in a rat brain slice preparation. J. Neurophysiol. 84, 1093–1097. doi: 10.1152/jn.2000.84.2.1093
Tian, G. F., Azmi, H., Takano, T., Xu, Q. W., Peng, W. G., Lin, J., et al. (2005). An astrocytic basis of epilepsy. Nat. Med. 11, 973–981.
Treiman, D. M. (2001). GABAergic mechanisms in epilepsy. Epilepsia 42(Suppl. 3), 8–12. doi: 10.1046/j.1528-1157.2001.042suppl.3008.x
Umpierre, A. D., West, P. J., White, J. A., and Wilcox, K. S. (2019). Conditional knock-out of mGluR5 from astrocytes during epilepsy development impairs high-frequency glutamate uptake. J. Neurosci. 39, 727–742. doi: 10.1523/jneurosci.1148-18.2018
Unichenko, P., Dvorzhak, A., and Kirischuk, S. (2013). Transporter-mediated replacement of extracellular glutamate for GABA in the developing murine neocortex. Eur. J. Neurosci. 38, 3580–3588. doi: 10.1111/ejn.12380
Upadhya, D., Kodali, M., Gitai, D., Castro, O. W., Zanirati, G., Upadhya, R., et al. (2019). A model of chronic temporal lobe epilepsy presenting constantly rhythmic and robust spontaneous seizures, co-morbidities and hippocampal neuropathology. Aging Dis. 10, 915–936. doi: 10.14336/ad.2019.0720
Vargas, J. R., Takahashi, D. K., Thomson, K. E., and Wilcox, K. S. (2013). The expression of kainate receptor subunits in hippocampal astrocytes after experimentally induced status epilepticus. J. Neuropathol. Exp. Neurol. 72, 919–932. doi: 10.1097/nen.0b013e3182a4b266
Verkhratsky, A., and Kirchhoff, F. (2007). NMDA receptors in glia. Neuroscientist 13, 28–37. doi: 10.1177/1073858406294270
Verkhratsky, A., Reyes, R. C., and Parpura, V. (2014). TRP channels coordinate ion signalling in astroglia. Rev. Physiol. Biochem. Pharmacol. 166, 1–22. doi: 10.1007/112_2013_15
Verkhratsky, A., Rodríguez, J. J., and Parpura, V. (2012). Calcium signalling in astroglia. Mol. Cell Endocrinol. 353, 45–56. doi: 10.1016/j.mce.2011.08.039
Verkhratsky, A., and Steinhäuser, C. (2000). Ion channels in glial cells. Brain Res. Brain Res. Rev. 32, 380–412. doi: 10.1016/s0165-0173(99)00093-4
Wang, F., Smith, N. A., Xu, Q., Goldman, S., Peng, W., Huang, J. H., et al. (2013). Photolysis of caged Ca2+ but not receptor-mediated Ca2+ signaling triggers astrocytic glutamate release. J. Neurosci. 33, 17404–17412. doi: 10.1523/jneurosci.2178-13.2013
Wang, X., Takano, T., and Nedergaard, M. (2009). Astrocytic calcium signaling: mechanism and implications for functional brain imaging. Methods Mol. Biol. 489, 93–109. doi: 10.1007/978-1-59745-543-5_5
Woo, D. H., Han, K. S., Shim, J. W., Yoon, B. E., Kim, E., Bae, J. Y., et al. (2012). TREK-1 and Best1 channels mediate fast and slow glutamate release in astrocytes upon GPCR activation. Cell 151, 25–40. doi: 10.1016/j.cell.2012.09.005
Xin, W., Schuebel, K. E., Jair, K. W., Cimbro, R., De Biase, L. M., Goldman, D., et al. (2019). Ventral midbrain astrocytes display unique physiological features and sensitivity to dopamine D2 receptor signaling. Neuropsychopharmacology 44, 344–355. doi: 10.1038/s41386-018-0151-4
Xu, J., Peng, H., Kang, N., Zhao, Z., Lin, J. H., Stanton, P. K., et al. (2007). Glutamate-induced exocytosis of glutamate from astrocytes. J. Biol. Chem. 282, 24185–24197. doi: 10.1074/jbc.m700452200
Yarishkin, O., Lee, J., Jo, S., Hwang, E. M., and Lee, C. J. (2015). Disinhibitory action of astrocytic GABA at the perforant path to dentate gyrus granule neuron synapse reverses to inhibitory in Alzheimer’s disease model. Exp. Neurobiol. 24, 211–218. doi: 10.5607/en.2015.24.3.211
Ye, Z. C., Wyeth, M. S., Baltan-Tekkok, S., and Ransom, B. R. (2003). Functional hemichannels in astrocytes: a novel mechanism of glutamate release. J. Neurosci. 23, 3588–3596. doi: 10.1523/jneurosci.23-09-03588.2003
Yoon, B. E., Jo, S., Woo, J., Lee, J. H., Kim, T., Kim, D., et al. (2011). The amount of astrocytic GABA positively correlates with the degree of tonic inhibition in hippocampal CA1 and cerebellum. Mol. Brain 4:42. doi: 10.1186/1756-6606-4-42
Yoon, B. E., and Lee, C. J. (2014). GABA as a rising gliotransmitter. Front. Neural Circuits 8:141. doi: 10.3389/fncir.2014.00141
Yu, X., Taylor, A. M. W., Nagai, J., Golshani, P., Evans, C. J., Coppola, G., et al. (2018). Reducing astrocyte calcium signaling in vivo alters striatal microcircuits and causes repetitive behavior. Neuron 99, 1170–1187.e9.
Keywords: astrocyte, Ca2+, glutamate, γ-aminobutyric acid, epilepsy, gliotransmission, network plasticity
Citation: Caudal LC, Gobbo D, Scheller A and Kirchhoff F (2020) The Paradox of Astroglial Ca2 + Signals at the Interface of Excitation and Inhibition. Front. Cell. Neurosci. 14:609947. doi: 10.3389/fncel.2020.609947
Received: 24 September 2020; Accepted: 03 November 2020;
Published: 26 November 2020.
Edited by:
Wannan Tang, University of Oslo, NorwayReviewed by:
Yuriy Pankratov, University of Warwick, United KingdomHyungju Park, Korea Brain Research Institute, South Korea
Copyright © 2020 Caudal, Gobbo, Scheller and Kirchhoff. This is an open-access article distributed under the terms of the Creative Commons Attribution License (CC BY). The use, distribution or reproduction in other forums is permitted, provided the original author(s) and the copyright owner(s) are credited and that the original publication in this journal is cited, in accordance with accepted academic practice. No use, distribution or reproduction is permitted which does not comply with these terms.
*Correspondence: Frank Kirchhoff, ZnJhbmsua2lyY2hob2ZmQHVrcy5ldQ==
†These authors have contributed equally to this work