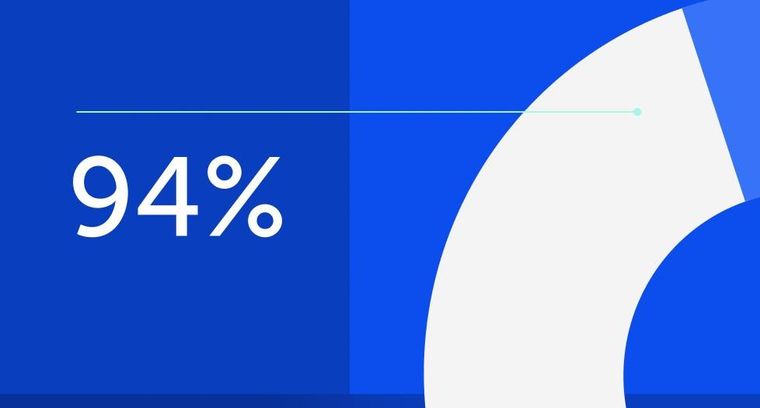
94% of researchers rate our articles as excellent or good
Learn more about the work of our research integrity team to safeguard the quality of each article we publish.
Find out more
REVIEW article
Front. Cell. Neurosci., 17 July 2020
Sec. Cellular Neurophysiology
Volume 14 - 2020 | https://doi.org/10.3389/fncel.2020.00213
This article is part of the Research TopicMechanisms Underlying Experience-dependent Plasticity of Cortical CircuitsView all 8 articles
Critical periods are postnatal, restricted time windows of heightened plasticity in cortical neural networks, during which experience refines principal neuron wiring configurations. Here, we propose a model with two distinct types of synapses, innate synapses that establish rudimentary networks with innate function, and gestalt synapses that govern the experience-dependent refinement process. Nascent gestalt synapses are constantly formed as AMPA receptor-silent synapses which are the substrates for critical period plasticity. Experience drives the unsilencing and stabilization of gestalt synapses, as well as synapse pruning. This maturation process changes synapse patterning and consequently the functional architecture of cortical excitatory networks. Ocular dominance plasticity (ODP) in the primary visual cortex (V1) is an established experimental model for cortical plasticity. While converging evidence indicates that the start of the critical period for ODP is marked by the maturation of local inhibitory circuits, recent results support our model that critical periods end through the progressive maturation of gestalt synapses. The cooperative yet opposing function of two postsynaptic signaling scaffolds of excitatory synapses, PSD-93 and PSD-95, governs the maturation of gestalt synapses. Without those proteins, networks do not progress far beyond their innate functionality, resulting in rather impaired perception. While cortical networks remain malleable throughout life, the cellular mechanisms and the scope of critical period and adult plasticity differ. Critical period ODP is initiated with the depression of deprived eye responses in V1, whereas adult ODP is characterized by an initial increase in non-deprived eye responses. Our model proposes the gestalt synapse-based mechanism for critical period ODP, and also predicts a different mechanism for adult ODP based on the sparsity of nascent gestalt synapses at that age. Under our model, early life experience shapes the boundaries (the gestalt) for network function, both for its optimal performance as well as for its pathological state. Thus, reintroducing nascent gestalt synapses as plasticity substrates into adults may improve the network gestalt to facilitate functional recovery.
Children learn differently than adults. They learn faster, and the skills that they acquire, stay with them for the rest of their lives. For example, children can achieve native-like proficiency in their second language if it is learned at young ages, but such ability is generally diminished after puberty. The learned second language in adults generally cannot shake off the accent. Thus, acquiring a language as a native depends on the age it is learned and less on the years experiencing it (Birdsong, 2018). The learning process in children is ultimately the acquisition and storage of information in the neural network for future reference, extraction, and modification. During so-called critical periods of restricted time windows in postnatal development, experience refines neural networks to set their foundation to optimally perform their dedicated function after continuous training later on. This process has been demonstrated across model systems and modalities. For example, songbirds learn to sing from early auditory experiences during critical periods, and if they do not have the chance to listen to a tutor of their species during that restricted time window, they will only acquire a rudimentary song (Brainard and Doupe, 2002; Prather et al., 2017). Experience can, however, also be maladaptive, e.g., if vision is compromised by an opaque lens, or if subjects are exposed to improper language in social contexts, early life experiences may lead to long-lasting impairments of vision or language skills, respectively (Grimshaw et al., 1998; Webber and Wood, 2005; Birch, 2013; Feldman et al., 2019).
In mammalian brains, the formation of cortical neural networks is initially guided by genetic programs, molecular pathfinding, and spontaneous neuronal activity patterns to establish innate functionality. These early networks are immature and rudimental and need a further experience-dependent specification of the excitatory network architecture for instructive functionality (Changeux and Danchin, 1976; Singer, 1995; O’Leary et al., 2007; Zipursky and Sanes, 2010). Experience-dependent cortical plasticity is broadly defined as the ability of cortical networks to encode information by long-lasting changes of their neural representations, and thus to adapt to ever-changing environments. The concept of critical period plasticity was pioneered by Hubel and Wiesel, who described experience-dependent plasticity of the functional architecture in the cat and rhesus monkey primary visual cortex (V1) (Hubel and Wiesel, 1963; Wiesel and Hubel, 1963a, b; LeVay et al., 1980). While zebra finch song learning (Aamodt et al., 1996; Roberts et al., 2010) and barn owl auditory localization (Feldman et al., 1996; Feldman and Knudsen, 1997) are also compelling experimental models, further molecular mechanistic driven studies are sparse due to technical limitations.
In the mammalian visual system, neurons in the binocular zone of V1 respond to visual inputs from both eyes (Mrsic-Flogel et al., 2007). Depriving one eye of vision by suturing it shut for a few days during postnatal development (monocular deprivation, MD), shifts the ocular dominance of binocular V1 neurons toward the non-deprived (experienced, also referred to as open) eye. This phenomenon is termed ocular dominance plasticity (ODP) and is the in vivo reference experimental model for studying cortical plasticity. ODP describes a network phenomenon that transcends from cats (Wiesel and Hubel, 1963b; Shatz and Stryker, 1978; Jones et al., 1984), monkeys (Hubel et al., 1977; Blakemore et al., 1978), and ferrets (Issa et al., 1999), to rodents such as rats (Rothblat et al., 1978) and mice (Dräger, 1978). ODP exploits the fact that visual experience can be reliably manipulated and leads to measurable modifications in V1-networks.
The visual impairments induced by MD before and throughout the critical period, mirror amblyopia in patients with impaired vision in one eye during their childhood (e.g., a cataract) (Prusky and Douglas, 2003; Webber and Wood, 2005; Birch, 2013). While the degree of recovery of visual features in animal species differs, it is generally accepted that full functional recovery is not achieved if binocular vision is only restored in adulthood. Therefore, understanding the cellular and molecular mechanisms underlying critical period plasticity is of utmost importance for developing causal therapeutic approaches to correct early network refinement defects. Furthermore, it is conceivable that those approaches might prove to be efficient also for inducing functional recovery in other neurodevelopmental disorders or after brain lesions caused by stroke or trauma.
BOX 1. Approaches to measure ocular dominance plasticity.
To analyze ODP, visual responses are assessed using various techniques that report different parameters of network activity. These techniques include single-unit recordings (Dräger, 1978; Bear and Singer, 1986; Gordon and Stryker, 1996; Hensch et al., 1998; Fagiolini and Hensch, 2000), intrinsic signal optical imaging (Kalatsky and Stryker, 2003; Cang et al., 2005) visually evoked potentials (VEPs) (Sawtell et al., 2003; Frenkel and Bear, 2004) and two-photon calcium imaging (Mrsic-Flogel et al., 2007; Kameyama et al., 2010; Kuhlman et al., 2013).
Single unit recordings assess the proportion of the recorded cells, responding preferentially to the deprived eye versus the non-deprived eye, and the contralateral bias index (CBI) or ocular dominance index (ODI) is used to quantify ocular dominance shifts by relating the difference in evoked spike numbers. This analysis measures the output, i.e., action potentials from the recorded V1-cells, and reports the proportion of the population preferring one eye over the other. The resulting ocular dominance shift towards the non-deprived eye after MD can be due to either a reduction of the deprived eye responses, or to an increase of the non-deprived eye responses, or to both. In an attempt to quantify changes specific to either eye, responses have been normalized to the firing rate (Bukhari et al., 2015) which is based upon the assumption that the baseline firing rate does not change between control and MD animals. However, single unit recordings typically report a relative change in the firing rate between non-deprived and deprived eye as the CBI.
Two-photon calcium imaging reports changes in calcium indicator fluorescence following action potentials as a proxy for neuronal activity. Similar to single unit recordings, this approach can report whether a neuron prefers one eye over the other, and the CBI is typically calculated. Additionally, the amplitude of the fluorescence change is used as a proxy to compare neuronal responses to either eye’s input in binocular V1 of control and MD animals after normalizing to baseline fluorescence intensity (Mrsic-Flogel et al., 2007; Kameyama et al., 2010; Kuhlman et al., 2013). In contrast to single unit recordings, calcium imaging samples a more unbiased population of neurons, whereas single unit recordings typically sample the most active neurons. Calcium imaging is typically limited to superficial layers of the cortex, and targets neurons in layers 2/3 of V1 (Mrsic-Flogel et al., 2007; Kameyama et al., 2010; Kuhlman et al., 2013). In contrast, single unit recordings allow measurements in all cortical layers, but results are often pooled across layers. It should be noted that the intracellular calcium rise in the neurons is an indirect consequence of action potential-driven membrane depolarization, thus is inherently delayed from the neuronal spike and often lacks single action potential resolution. Additionally, the calcium indicators and the fluorescence detection approaches also introduce further temporal delay of the signal measured versus the immediate neuronal responses. These technical differences need to be taken into consideration when interpreting MD-induced circuit plasticity as mechanistic differences between layers are apparent (Trachtenberg et al., 2000; Rao and Daw, 2004; Crozier et al., 2007; Liu et al., 2008; Fong et al., 2020).
VEPs are complex extracellular field potential recordings induced by visual stimulation. Using one-dimensional current source density analysis to track the signal along the cortical depth, the VEPs induced by visual stimulation are primarily driven through thalamocortical synaptic inputs to layer 4 neurons (Sawtell et al., 2003), but also include population spikes and local network activities in the later phase of the VEP waveform. Chronic VEP recordings have been used to longitudinally track the collective activities of the recorded cell population in rodents (Sawtell et al., 2003; Frenkel and Bear, 2004).
Intrinsic signal optical imaging exploits the fact that active neurons consume more oxygen than inactive ones, thereby locally increasing the concentration of deoxyhemoglobin, which absorbs more of the red light that is shone onto the cortical surface for monitoring the visually stimulated activity changes in mostly superficial V1 (Kalatsky and Stryker, 2003; Cang et al., 2005). Absolute values of intrinsic signals are tracked in individual mice before and after MD through chronic imaging (Kaneko et al., 2008b; Greifzu et al., 2014) or they allow quantitative eye-specific comparisons between control and experimental animals (Cang et al., 2005). Both VEP recordings and intrinsic signal optical imaging measure population responses induced by visual stimulations of either eye. Both methods are used to monitor the specific changes in responses to visual stimulation of the deprived or the non-deprived eye, i.e., whether MD induces either deprived eye depression or non-deprived eye potentiation, or both to cause ODP. However, both approaches lack resolution to monitor individual neuronal responses. Furthermore, given the complexity of the signals, when analyzing the population responses, using VEP recordings and intrinsic signal optical imaging, the underlying cellular and circuit mechanisms for the same measured responses may be different.
In particular, by investigating mouse models using an array of experimental approaches (Box 1), the field has gained mechanistic insights into the molecular and cellular mechanisms of ODP, summarized in Table 1. Emerging evidence implicates that cortical plasticity, such as ODP, is regulated and controlled by distinct mechanisms during the critical period, as opposed to that in adult (Hooks and Chen, 2007; Espinosa and Stryker, 2012; Levelt and Hübener, 2012; Hensch and Quinlan, 2018; Stryker and Löwel, 2018). Here, we specify the key features for critical period plasticity in the binocular region of mouse V1, outlining a new model with dedicated synapse categories and states: innate versus gestalt synapses, and silent, unsilenced, versus stabilized state. We will first outline our model for the refinement of excitatory neural networks during critical periods, and then elaborate on how the model is supported by our recent studies and the current body of literature. We will then describe the implications for the cellular mechanisms of ODP and perception. While our discussion is focused on ODP as an experimental model for cortical plasticity and critical periods, we also survey the different expression mechanisms of cortical plasticity at different developmental stages and address results that highlight the general applicability of the discussed principles.
We propose two categories of synapses that are developmentally and functionally defined: Innate synapses establish innate network functionality, and gestalt synapses primarily govern experience-dependent refinement of principal neuron connections during critical periods (Figure 1A and Table 2). The word “gestalt” (\gə-’stält\), is derived from German with the meaning: “An organized whole that is perceived as more than the sum of its parts” (Oxford University Press). It describes the functional role of these synapses after experience-dependent network maturation, namely providing a configuration of connections, a “gestalt,” that stores higher-order instructive information for future reference and modification, particularly the percept of the world created as a result of the individual’s early life experience.
Figure 1. Model of critical period excitatory neural network refinement. (A) Two categories of synapses that are developmentally and functionally defined: Innate synapses (blue) that establish innate functionality of the network, and gestalt synapses (dark magenta) that primarily govern the plasticity and experience-dependent changes in network configurations during critical periods. Innate synapses provide depolarization (dotted blue circle), while plasticity happens at the gestalt synapses (dotted dark magenta circle). (B) Experience-dependent network maturation is mediated by synaptogenesis, gestalt synapse maturation and synapse pruning. Triangles illustrate principal neurons. During the critical period, the immature network starts with less specific connections. Through many iterations of synaptogenesis, maturation and pruning, the network becomes increasingly more organized with selectively strengthened, stable connections. The refined configuration (illustrated as symmetry) in the mature network builds the gained gestalt to represent the higher functionality acquired through experience. It is the gestalt that determines the network’s ability to present the sensory information as the percept.
By containing α-amino-3-hydroxy-5-methyl-4-isoxazole propionic acid-type glutamate receptors (AMPARs), innate synapses depolarize the postsynaptic membrane upon glutamate binding and mediate fast excitatory synaptic transmission in neuronal networks. During early development, innate synapses thus provide basic functionality by maintaining rudimentary excitatory network activity. The nascent gestalt synapses have an AMPAR-silent state (commonly referred to as silent synapses) (Isaac et al., 1995; Liao et al., 1995) and thus constitute morphological synaptic connections that transmit little or no information between the connected neurons. Nascent gestalt synapses are constantly formed throughout the critical period, creating random synaptic opportunities between principal neurons. The activation of innate synapses depolarizes neurons to enable associative plasticity at silent gestalt synapses that need coincidence of depolarization and presynaptic release at the silent gestalt synapses (Figure 1A).
Nascent (silent) gestalt synapses can, therefore, undergo experience-dependent excitatory synapse maturation during critical periods: repeated correlated neuronal activity of the pre- and postsynaptic neurons induces long-term synaptic potentiation (LTP), and drives AMPARs into silent gestalt synapses, transforming them into unsilenced gestalt synapses which now also contain responsive AMPARs (AMPAR+). These unsilenced gestalt synapses then get either stabilized to become stable gestalt synapses, consolidating a favorable synaptic connection, or get pruned if not timely and sufficiently activated. Thus, at the beginning of the critical period, the excitatory network consists of both rudimentary functional connections with innate synapses and gestalt synapses as potential substrates for experience-dependent network refinement. The coordinated action of unsilencing and stabilization of silent gestalt synapses, and pruning of gestalt synapses in the silent as well as the unsilenced state (but not stable gestalt synapses), underlies excitatory network maturation. This coordinated process sets up the fundamental cortical network architecture (Figure 1B). We thus postulate that the network gains the gestalt that mediates its higher functionality via experience-dependent refinement for visual perception in adults. It is the gestalt that determines the network’s ability to present the sensory information as a percept.
Silent synapses have so far been considered to represent a nascent state of functionally indistinguishable synapses. Here, we refer to silent synapses as the silent state of gestalt synapses. Postsynaptically silent synapses are nascent synapses that express N-methyl-D-aspartate-type glutamate receptors (NMDARs), but lack AMPARs (Isaac et al., 1995; Liao et al., 1995). At the resting potential, NMDARs are largely blocked by Mg2+. Hence at silent synapses, presynaptically released glutamate alone does not evoke an excitatory postsynaptic current (EPSC) in the recipient neurons when the recipient neurons sit at the resting potential. During LTP induction, NMDARs at silent synapses are opened with simultaneous postsynaptic depolarization and presynaptic glutamate release. Ca2+ influx through NMDARs drives Ca2+-dependent signaling cascades that lead to AMPAR incorporation into silent synapses, establishing AMPAR-mediated synaptic transmission (AMPAR positive; AMPAR+) that subsequently responds to presynaptic glutamate release at resting potential (Isaac et al., 1995; Liao et al., 1995). During the critical period, silent synapses mature, by recruiting and stabilizing AMPARs into the postsynaptic membrane, so that the proportion of silent synapses decreases with age and experience (Isaac et al., 1997; Bottjer, 2005; Funahashi et al., 2013; Huang X. et al., 2015; Han et al., 2017; Favaro et al., 2018). The functional consequence of this process is the increase of synaptic potency at unitary neural connections (Figure 2A; Isaac et al., 1997; Favaro et al., 2018). In the mature cortex, one excitatory axon forms on average five synapses with a target pyramidal neuron (Feldmeyer et al., 2002). The maturation of the gestalt synapses from an initially silent to an unsilenced and finally stabilized state, therefore, strengthens the connectivity of functional ensembles, representing a specific functional output of neural networks (Hebb, 1949). Thus, silent synapses constitute synapse opportunities, providing morphological (anatomical) connections between two neurons, and act as substrates to establish instructive connections when unsilenced and stabilized.
Figure 2. Schematic diagram illustrating the hypothesized changes of gestalt synapses underlying experience-dependent network maturation in the primary visual cortex during the critical period for ODP. (A) Increase of synaptic potency as the result of gestalt synapse maturation. Specifically, the number of stabilized synapses increases between certain neuron pairs. Gestalt synapses that are not stabilized are pruned away (dotted line). (B) The transition of different states of gestalt synapses. LTP drives AMPARs into silent gestalt synapses transforming them to unsilenced gestalt synapses now containing AMPARs (AMPAR+). These unsilenced gestalt synapses then get either stabilized to become stable gestalt synapses, consolidating a favorable synaptic connection, or get pruned if not timely and sufficiently activated. If not unsilenced, silent gestalt synapses are pruned away after NMDAR-LTD. Molecularly, PSD-95 drives the maturation of silent synapses, whereas PSD-93 opposes this function. Both maturation and pruning are likely controlled through post-synaptic calcium signaling, and neurogranin (Ng) is indicated as a key switch for governing the fate of gestalt synapses.
While functional ensembles are strengthened, the total number of excitatory synapses decreases during the critical period, and AMPAR-mediated synaptic transmission remains at equilibrium. The maturation of silent synapses is counter-balanced, at least in part, by synapse pruning (Han et al., 2017). This pruning is likely mediated by a form of long-term synaptic depression (LTD) (Nägerl et al., 2004; Zhou et al., 2004). Thus, maturation of silent synapses may consolidate a nascent synaptic connection, while its pruning eliminates the connection, resulting in a change of the connection pattern between excitatory neurons (Figure 2B). Consequently, silent synapse-based excitatory network maturation contains the essential ingredients for experience-dependent neural network refinement (Changeux and Danchin, 1976; Katz and Crowley, 2002). This excitatory network maturation process is reminiscent of the earlier work about the development of long-range, tangential connections in cat V1. At the beginning of the critical period, the V1 horizontal fiber network is rather homogeneously connected. Connections that participated in synchronized activities are stabilized (i.e., neurons wire together if they fire together) (Löwel and Singer, 1992) and network refinement includes pruning as well as a further elaboration of distinct connections (Callaway and Katz, 1990).
A critical role of silent synapses in juvenile ODP was revealed by the analysis of knock-out (KO) mice of postsynaptic density protein-93 (PSD-93) and PSD-95 (Huang X. et al., 2015; Favaro et al., 2018). PSD-93 and PSD-95 belong to the protein family of signaling scaffolds of the postsynaptic density that mediate signaling specificity in synaptic plasticity (Xu et al., 2008; Liu et al., 2017). PSD-95 is required for the experience-dependent maturation of silent synapses. A loss-of-function results in the persistent presence of a high fraction of silent synapses (∼50%) from eye-opening into adulthood in V1, as well as lifelong juvenile ODP (Huang X. et al., 2015). Additionally, when silent synapses are reinstated after the closure of the critical period by knocking down PSD-95 in the adult visual cortex, both the juvenile fraction of silent synapses and juvenile-like ODP are restored, indicating that PSD-95 is necessary for both the initial maturation of silent synapses and the maintenance of the matured synaptic state (Huang X. et al., 2015). Conversely, PSD-93 inhibits experience-dependent maturation of silent synapses (Favaro et al., 2018). In mice with a knock-out or visual cortex specific knock-down of PSD-93, silent synapses mature precociously, and consequently, the critical period for ODP closes earlier than that of wild-type mice. Notably, silent synapse maturation is delayed by dark rearing in wild-type mice and the duration of the critical period is consequently extended (Gianfranceschi et al., 2003; Funahashi et al., 2013; Favaro et al., 2018). However, in PSD-93 KO mice, dark rearing did not prevent the accelerated silent synapse maturation, nor the precocious termination of the critical period for ODP (Favaro et al., 2018).
The developmental increase in the protein expression of PSD-93 in V1 leads that of PSD-95 after eye-opening, and peaks before the critical period, whereas the expression of PSD-95 peaks at the end of the critical period, a result consistent with the opposing yet cooperative interaction of these paralogs in regulating silent synapse maturation (Huang X. et al., 2015; Favaro et al., 2018). The strict correlation between the time course of PSD-93 and PSD-95 expression, silent synapse maturation, and the duration of the critical period for ODP strongly imply that silent synapses serve as instructive substrates for network refinement during critical periods. While PSD-95 drives the maturation of silent synapses, PSD-93 opposes this function (Figure 2B; Favaro et al., 2018). The decrease of silent synapses may thus mark the end of the critical period, linking excitatory network maturation with the availability of silent synapses. Consistent with this notion, the accelerated loss of silent synapses also leads to early termination of the critical period in the primary auditory cortex (Sun et al., 2018). The opposing function of PSD-95 and PSD-93 in silent synapse maturation appears universal in gestalt synapses of principal neurons, as any of those synapses so far investigated, exhibit the same phenotype as described for layer 2/3 pyramidal neurons in V1 with ∼50% of synapses being regulated by these paralogs (Béïque et al., 2006; Huang X. et al., 2015; Shukla et al., 2017; Favaro et al., 2018).
Thus, under physiological conditions during the critical period, silent gestalt synapse-based refinement alters principal neuron wiring and their progressive maturation ends critical periods.
The two different types of AMPAR+ synapses were indicated by the analysis of the role of PSD-95 in critical period plasticity. PSD-95 is not required for all stable AMPAR+ synapses. First, the excitatory drive onto PV+ interneurons is unaltered in PSD-95 KO mice (Huang X. et al., 2015), although PSD-95 is also expressed in PV+ interneurons and thus absent in the excitatory synapses of the mutant mice (Akgul and Wollmuth, 2010) indicating that the basal excitatory postsynaptic function in PV+ interneurons does not require PSD-95. Secondly, the maturational defect by loss of PSD-95 is limited to a subset of synapses in principal neurons. At eye-opening, in both WT and PSD-95 KO mice, ∼50% synapses are AMPAR+. Notably, at this developmental point, there is hardly any PSD-95 expressed in V1 (Huang X. et al., 2015). While in WT mice, PSD-95 protein levels increase and the fraction of silent synapses decreases after eye-opening, in PSD-95 KO mice, the fraction of silent synapses remains at ∼50%. Third, when knocking-down PSD-95 after silent synapses have matured, the fraction of silent synapses is restored to the level at eye-opening (∼50% synapses). The remaining ∼50% synapses are AMPAR+ synapses persisting in the absence of PSD-95, as we and others had observed in PSD-95 KO mice (Béïque et al., 2006; Huang X. et al., 2015). These results indicate that about half of the synapses onto principal neurons and all excitatory synapses onto PV+ interneurons do not require PSD-95 for synaptic AMPAR stabilization. We operationally define these PSD-95 independent synapses as innate synapses, which potentially provide constitutive excitatory drive onto neurons and build the rudimentary backbone network with innate function. In contrast, the PSD-95 dependent synapses are gestalt synapses.
The morphological identity of innate and gestalt synapses remains speculative. Dendritic spines are the typical morphological structures of glutamatergic synapses in the mature brain, categorized into thin, mushroom, and stubby spines (Juraska and Fifkova, 1979; Miller and Peters, 1981; Harris et al., 1992). Each spine generally contains one excitatory synapse, and hence the spine number has been widely used as a proxy for the number of glutamatergic synapses (LeVay, 1973; Harris et al., 1992). However, during early development, initial excitatory synapses are also formed onto the dendritic shaft of principal neurons of visual cortex, and other cortical and subcortical areas (Juraska and Fifkova, 1979; Miller and Peters, 1981; Boyer et al., 1998; Jang et al., 2015). Thus, shaft excitatory synapses might constitute part of the innate synapses. In layers 2/3 of the visual cortex of adult mice or rats, excitatory shaft synapses are rare, whereas stubby spines account for 20–30% of all spines (Miller and Peters, 1981; Majewska and Sur, 2003; Bukhari et al., 2015; Hodges et al., 2017). As the knock-down of PSD-95 revealed the presence of innate synapses in adult mice (Huang X. et al., 2015), we postulate that both shaft and stubby spines belong to the category of innate synapses. Based on dendritic compartmentalization theory, stubby synapses functionally resemble shaft synapses, as they are not electrically and morphologically separated from the dendritic shaft as the other types of spine synapses (Yuste, 2013).
Spine synapses start to form in the visual cortex after P7 in rats (Juraska and Fifkova, 1979; Miller and Peters, 1981) and the relative fraction of spine synapses increases during development (Harris et al., 1992). We postulate that synapses on thin and mushroom spines constitute the gestalt synapses that primarily govern the plasticity and changes in network configurations during critical periods. The observation that in some neurons, both shaft and spine synapses coexist throughout life, and that they do not convert into each other, further supports our two category concept with innate as shaft and gestalt as spine synapses, respectively (Reilly et al., 2011; Jang et al., 2015). This morphological differentiation might not be absolute and the main discriminator of the two synapse categories is the dependence of stable AMPAR incorporation by PSD-95.
PSD-95 mediates the maturation of silent gestalt synapses (Huang X. et al., 2015; Favaro et al., 2018) and thus the stable incorporation of AMPARs into gestalt synapses. This notion is further substantiated by the observations that first, loss of PSD-95 leads to ∼50% reduction in AMPAR EPSCs, corresponding to a reinstatement of ∼50% silent synapses (Nakagawa et al., 2004; Béïque et al., 2006; Elias et al., 2006; Schlüter et al., 2006; Ehrlich et al., 2007; Carlisle et al., 2008; Xu et al., 2008; Krüger et al., 2013) second, the increase in PSD-95 protein levels during the critical period; and third, gain of PSD-95 function increases AMPAR EPSCs by accelerated silent synapse maturation in developmentally young but not adult brains (Stein et al., 2003; Levy and Nicoll, 2017; Favaro et al., 2018).
The hypothesized two functional categories of synapses become apparent also in other KO mice. Loss of cadherin, EGF LAG seven-pass G-type receptor 3 (Celsr3) leads to a loss of ∼50% glutamatergic synapses (Thakar et al., 2017) a result consistent with our two synapse categories concept, if one of them strictly requires Celsr3. Furthermore, loss- and gain-of-function of the signaling scaffold membrane-associated guanylate kinase inverted-2 and atrophin interacting protein-1 (MAGI-2) leads to a shift in the fraction of stubby spines and mushroom spines (Danielson et al., 2012). Further analyses of the functional and molecular interactions between PSD-95 and Celsr3 or MAGI-2, respectively, might reveal more insights about the identity and function of these synaptic categories.
Thus, both morphological as well as functional characterizations, support at least two synaptic categories. Given that PSD-95 is expressed in shaft synapses of PV+ interneurons, the differentiation is not possible by the sole presence or absence of PSD-95, but might be rather determined by one of the PSD-95 interaction proteins, such as Celsr3 (Thakar et al., 2017).
Based upon studies investigating dendritic spines, the morphological proxy of excitatory synapses, we postulate that nascent gestalt synapses are constantly generated during the critical period and serve as synaptic substrates for experience-dependent refinement by being unsilenced and stabilized, or pruned. Consistent with our model, the density of dendritic spines in the cerebral cortex peaks during early childhood, and then slowly decreases toward adulthood in humans, non-human primates, and other mammalian brains (Huttenlocher, 1979; Juraska and Fifkova, 1979; Rakic et al., 1986; Munoz-Cueto et al., 1991; Bourgeois and Rakic, 1993; Huttenlocher and Dabholkar, 1997).
Previous models have focused on the motility of filopodia and spines in mediating structural plasticity during critical period network refinement (Ziv and Smith, 1996; Dunaevsky et al., 1999; Lendvai et al., 2000). Filopodia are thin, long protrusions of the dendritic shaft that form and disappear in minutes both in cultured neurons and in vivo (Dailey and Smith, 1996; Ziv and Smith, 1996; Lendvai et al., 2000). They lack postsynaptic specializations and are postulated to sample the surrounding for new synaptic connections (Ziv and Smith, 1996). Filopodia are particularly abundant during early development; in layer 2/3 of the visual cortex, they are evident between P3 and P12 (Miller and Peters, 1981). The number of filopodia decreases to a low level at the beginning of the critical period in visual cortex (Majewska and Sur, 2003; Konur and Yuste, 2004; Cruz-Martin et al., 2010). Sensory experience drives the motility of dendritic protrusions in dendrites of layer 2/3 pyramidal neurons in the somatosensory cortex during critical periods (Lendvai et al., 2000). However, dark rearing which prolongs the critical period for ODP does not prolong the time window of highly motile spines/filopodia in the visual cortex (Konur and Yuste, 2004). Hence, interrogations that extend critical periods and the motility of spines/filopodia appear disconnected.
Furthermore, the high numbers of filopodia in early postnatal (P3–P12) cortex is overlapping with the critical period of the somatosensory cortex (P11–13), but not with the later critical period in the visual cortex (P21–35) when filopodia numbers are low (Miller and Peters, 1981; Dunaevsky et al., 1999; Lendvai et al., 2000). While it is conceivable that filopodia are responsible for synapse increase before the critical period (Juraska and Fifkova, 1979; Munoz-Cueto et al., 1991), they are unlikely to play a major role for synaptogenesis during the critical period, at least in the visual cortex (Majewska and Sur, 2003; Konur and Yuste, 2004; Cruz-Martin et al., 2010). Nascent gestalt synapses in the visual cortex might thus rather be formed as thin spines that are unstable when lacking PSD-95, and that mature with PSD-95 incorporation (Cane et al., 2014).
While synaptogenesis already starts prenatally, the increase of synapse numbers continues postnatally, providing evidence for postnatal synaptogenesis (Huttenlocher, 1979; Juraska and Fifkova, 1979; Rakic et al., 1986). Postnatal synaptogenesis is also supported by more recent spine imaging studies using in vivo two-photon microscopy (Grutzendler et al., 2002; Trachtenberg et al., 2002). Spine formation is prominent in juvenile rodents during the critical period for ODP in V1 (Grutzendler et al., 2002; Holtmaat et al., 2005; Zhou et al., 2017) and also in somatosensory, motor and frontal cortices (Lendvai et al., 2000; Holtmaat et al., 2005; Zuo et al., 2005) likely replenishing the nascent gestalt synapse pool for further network refinement. However, little is known whether synaptogenesis or its kinetics are regulated by experience during the critical period.
Synapse elimination has been widely reported in the neuromuscular junction, the ganglia of the peripheral nervous system, cerebellum, and spinal motor neurons (Purves and Lichtman, 1980; Colman et al., 1997). Eliminating underutilized connections to refine the network structure has long been hypothesized to be the leading mechanism in the development of both the peripheral and central nervous systems (Shatz and Stryker, 1988; Simon et al., 1992; Walsh and Lichtman, 2003). At both the neuromuscular junction and the retino-thalamic connections, in a “winner takes it all” process, one neuron typically wins the competition among a group of neurons to connect to the target neuron/muscle, while the other connections are pruned (Kasthuri and Lichtman, 2003; Hooks and Chen, 2007).
The synaptic reorganization in the cortex appears to be different, not the least because neurons are innervated by thousands of afferents in complex dendritic trees. MD increases the structural dynamics of spines (Tropea et al., 2010; Yu et al., 2011) and accelerates spine elimination during the critical period in V1 (Yu et al., 2011; Zhou et al., 2017; Sun et al., 2019), indicating that pruning also contributes to experience-dependent structural plasticity in the cortex. However, in the cerebral cortex, the wiring configuration among principal neurons is more complex and silent gestalt synapses might rather operate under a process, subsumed as “use it or lose it,” a concept, theorized for bidirectional plasticity such as LTP and LTD (Hebb, 1949; Miller et al., 1989; Balice-Gordon and Lichtman, 1994; Katz and Shatz, 1996). In contrast to LTP and LTD, not only synaptic weights are changed during experience-dependent plasticity, but synaptic connections between principal neurons are gained with synaptogenesis and silent synapse maturation, or lost with synapse pruning.
While little is known about the pruning of (silent) gestalt synapses, their partly transient nature is indicated by the observation that the rate of dendritic spine turn-over is high during early cortical development and in PSD-95 deficient neurons, situations when silent synapse numbers are high (Zuo et al., 2005; Ehrlich et al., 2007). Given the equilibrium of AMPAR-mediated synaptic transmission during critical periods (Han et al., 2017), the model proposes that some AMPAR+ connections are weakened by uncorrelated neuronal activities, resulting in synaptic depression and ultimately spine pruning. In contrast, if repeatedly and successfully activated by experience, silent synapses may be unsilenced and consolidated into mature synapses between principal neurons to improve network function.
Recent findings provided new insights on mechanisms coordinating synapse maturation and synapse pruning in neurons. Neurogranin is a Ca2+/calmodulin (CaM) binding protein that regulates the availability of the Ca2+ signal mediator CaM and consequently regulates the activation of the downstream signaling proteins including CaM-dependent phosphatase and CaM-dependent kinase. Tipping the Ca-dependent signaling toward CaM-dependent phosphatase activation by genetically reducing or abolishing the function of neurogranin (Hwang et al., 2018), hinders the maturation of silent gestalt synapses and exacerbates synapse pruning (Han et al., 2017). Thus, both maturation and pruning are likely controlled through post-synaptic calcium signaling, and neurogranin is indicated as a key switch for governing the fate of gestalt synapses (Figure 2B).
Notably, both synaptogenesis and synapse pruning are also strongly influenced by astrocytes and microglia, reviewed by Clarke and Barres (2013); Chung et al. (2015), and Neniskyte and Gross (2017). While many astrocyte-secreted factors have been reported to drive synaptogenesis in vitro in the peripheral or central nervous systems (reviewed by Clarke and Barres, 2013), existing data support the involvement of thrombospondins in driving excitatory synaptogenesis in the cortex (Christopherson et al., 2005; Eroglu et al., 2009), and silent synapse generation after cocaine in the nucleus accumbens (Wang et al., 2020). Studies primarily from the retinogeniculate system and hippocampus implicate that glial cells are involved in synapse elimination (Paolicelli et al., 2011; Schafer et al., 2012), mediated via the complement pathway of microglia (Stevens et al., 2007; Schafer et al., 2012), and via Multiple Epidermal Growth Factor-like Domains 10 (MEGF10) and MER receptor tyrosine kinase (MERTK) phagocytic pathways of astrocytes (Chung et al., 2013). It is not known whether these pathways also contribute to the experience-dependent maturation of gestalt synapses and if so whether there exists any synapse subtype specificity, concerning the neuro-immune interaction and communication to control the highly specific network refinement. A major outstanding challenge, therefore, remains to identify the subtypes of synapses by e.g., identifying molecular markers for categorizing synapses, notwithstanding their functional categorization in governing refinement processes of cortical networks.
According to our model, gestalt synapses are the primary source for experience-dependent plasticity during critical periods, especially for the experience-dependent strengthening of functional ensembles. Nevertheless, innate synapses also appear to be targets of regulation. This prediction is based on two results: First, the same fraction of AMPAR+ and silent synapses was found after later loss of PSD-95 and at eye-opening (Huang X. et al., 2015), indicating that the fraction of innate synapses remains constant with age. Second, the spine density decreases toward the end of the critical period, indicating a decrease in total numbers of synapses (Han et al., 2017). To keep the same share of innate synapses, both innate synapses and gestalt synapses must be pruned during development at a similar rate. These observations also predict that both categories of synapses might be homeostatically linked despite their different requirements on PSD-95, so that changes in the number of gestalt synapses influence the number of innate synapses.
Thus, at the beginning of the critical period, the excitatory network starts with both innate synapses and silent gestalt synapses. Innate synapses provide the activity to maintain a backbone of rudimentary excitatory neural network activity, and silent gestalt synapses provide potential substrates for experience-dependent network refinement. Additional nascent gestalt synapses are continuously formed during the critical period, setting up repeating iterative cycles for changing and optimizing neural network configurations. The excitatory network matures based upon the coordinated action of unsilencing and stabilization of silent gestalt synapses, and pruning of gestalt synapses in the silent as well as the unsilenced state, but not stable gestalt synapses. This coordinated process sets up the fundamental functional cortical network architecture (the gestalt) during the critical period (Figure 1). We hypothesize that this silent synapse-based network maturation during critical periods is universal for the cerebral cortex. In addition to the visual cortex, it also takes place in the auditory cortex (Sun et al., 2018), the somatosensory cortex (Isaac et al., 1997), the medial prefrontal cortex, and the hippocampus of rodents (Favaro et al., 2018). Silent synapses have also been shown to be necessary for experience-dependent network development/maturation beyond the mammalian brain, namely for song learning in zebra finches during the sensitive period (Bottjer, 2005).
This silent synapse-based mechanism of critical period plasticity is consistent with the heightened excitatory synaptic plasticity during this time window. The high susceptibility of the visual cortex for LTP coincides with the critical period and can be prolonged by dark rearing (Kirkwood et al., 1995), which prevents silent synapse maturation (Funahashi et al., 2013; Favaro et al., 2018). Unsilencing silent synapses is a mechanism for LTP, especially at a young age (Isaac et al., 1995), thereby strengthening and stabilizing functional connections by increasing the number of AMPAR+ synapses at these connections. Similar to the increase in potency by silent synapse maturation in V1, LTP increases the potency in minimal stimulation recordings, consistent with the hypothesis that LTP mechanisms mature silent synapses in vivo (Liao et al., 1995; Isaac et al., 1996, 1997; Favaro et al., 2018).
The progressive maturation of silent gestalt synapses predicts that the number of AMPAR+ synapses should increase during development. However, the frequency of miniature (m) EPSCs remains unchanged throughout the critical period until adulthood (Corlew et al., 2007; Han et al., 2017). This equilibrium is at least partially explained by the observation that some existing AMPAR+ synapses go through experience-dependent elimination and thus counterbalance excitatory synapse maturation during the critical period, as spine density, the anatomical proxy of excitatory synapses also decreases during the critical period (Han et al., 2017). Mechanistically, this pruning is likely mediated by a form of LTD. In support of this notion, it was shown that synapse pruning results from a form of LTD (Nägerl et al., 2004; Zhou et al., 2004), and lowering the threshold for LTD also leads to a profound spine loss (Han et al., 2017).
While reducing neurogranin levels lowers the threshold for LTD (Han et al., 2017) and blocks LTP (Pak et al., 2000; Hwang et al., 2018), enhancing neurogranin levels lowers the threshold for LTP (Zhong and Gerges, 2012; Hwang et al., 2018). Thus, changes in neurogranin levels likely lead to a form of metaplasticity by sliding the plasticity threshold (Bienenstock et al., 1982; Abraham and Bear, 1996), which in turn will influence critical period ODP. According to both the metaplasticity theory (Abraham and Bear, 1996; Philpot et al., 2001) and the silent synapse mechanism, we predict that lowering neurogranin levels will extend the critical period for ODP while enhancing neurogranin levels will drive a faster maturation of the cortical circuit, and likely drive the adult form of plasticity earlier. Testing how changes in neurogranin levels affect ODP will likely provide more insight into this process.
It should be noted that both synapse stabilization and synapse pruning are cellular events lasting beyond the time scale of the initial NMDAR-dependent bi-directional plasticity, and thus likely depend on additional trophic factors which initiate inter-spine competition that will ultimately determine synapse fate (maturation or pruning) (Bian et al., 2015).
Before discussing how the concept of gestalt synapses integrates into the expression mechanisms of critical period ocular dominance plasticity, we review the current state of the art. MD shifts the ocular dominance of visual responses in the binocular segment of V1 toward the non-deprived eye. During the critical period, this shift is initially mediated by a depression of deprived-eye responses in cortical layer 4 and layer 2/3, measured with visually evoked potentials (VEPs) or calcium imaging, respectively (Box 1) (Frenkel and Bear, 2004; Mrsic-Flogel et al., 2007; Gandhi et al., 2008) or in superficial layers with intrinsic signal optical imaging (Cang et al., 2005; Kaneko et al., 2008a, b). When analyzing synaptic strength directly with in vivo patch-clamp recordings of layer 4 spiny stellate neurons in the binocular region, excitatory synaptic inputs are primarily driven by visual stimulation of the contralateral eye in rodents, and the ocular dominance shift resembles that measured with VEPs (Ma et al., 2013). The rapid ocular dominance shift initiated by 3–4 days of MD in juvenile rodents is primarily expressed at the thalamocortical glutamatergic feedforward synaptic connection, as pharmacological removal of intracortical polysynaptic inhibitory and excitatory synapses did not influence the magnitude of the ocular dominance shift (Khibnik et al., 2010). Despite the proposed expression of ODP at the glutamatergic synapses, plasticity in inhibitory neurons is expressed in parallel. The strength of the feed-forward inhibitory drive of either pathway is equilibrated to the excitatory drive, resulting in a similar excitatory/inhibitory ratio driven by either eye, echoing equilibration in layer 2/3 pyramidal neurons (Xue et al., 2014). MD causes a depression of the excitatory drive selectively of the deprived eye pathway, while the feed-forward inhibition of both deprived and non-deprived eye pathways is reduced. The resulting shift in the response of the layer 4 spiny stellate neurons is thus mediated by both a shift in the excitatory drive toward the non-deprived eye and a relative decrease of inhibition of the non-deprived eye drive (Ma et al., 2013). This reduction of the feed-forward inhibition of both pathways is also seen in layer 2/3 (Kuhlman et al., 2013), enabling the expression of critical period ODP. The depression of the excitatory synaptic drive reaches a maximum after 3-d MD. In contrast, the depression of feed-forward inhibition progresses further and after 6-day MD, the excitatory/inhibitory ratio of the non-deprived eye inputs is higher than that of the deprived contralateral eye input (Ma et al., 2013).
Mechanistically, multiple lines of evidence point toward NMDAR-dependent LTD to cause deprived eye depression (Bear et al., 1990; Rittenhouse et al., 1999; Sawtell et al., 2003; Yoon et al., 2009). First, NMDAR-dependent LTD is prevalent during critical periods, and second, MD occludes subsequent LTD ex vivo in visual cortex slices (Crozier et al., 2007). Third, the susceptibility of some forms of LTD declines in an age-dependent manner (Dudek and Friedlander, 1996; Kirkwood et al., 1997; Jiang et al., 2007), consistent with the absence of deprived eye depression in adult ODP of rodents raised in standard cages. Fourth, layer 4 activity is only depressed if the deprived eye sends spontaneous activity. If this activity is blocked by the sodium channel blocker tetrodotoxin, only the increase of the non-deprived eye pathway after longer MD is expressed (Frenkel and Bear, 2004). This result is consistent with the dependence of LTD on unsynchronized neural activity, rather than lack of activity, between pre- and postsynaptic neurons to induce NMDAR activity. Fifth, MD induces a phosphorylation pattern of AMPARs that resembles the one after LTD induction (Heynen et al., 2003). Sixth, deprived eye depression only occurs in neurons receiving synaptic inputs from both eyes and requires the competing input of both eyes (Rittenhouse et al., 1999; Frenkel and Bear, 2004; Mrsic-Flogel et al., 2007), indicating that during MD, the spontaneous activity of the deprived eye is likely unsynchronized with the visually evoked non-deprived eye inputs so that depression is induced at the deprived eye inputs. Pruning of synapses that occurs in both layer 1 and layer 2/3 after short MD was observed in the binocular region of V1, indicating that LTD is a step preceding pruning or even part of it (Mataga et al., 2004; Yoon et al., 2009; Zhou et al., 2017; Fong et al., 2020).
Despite the canonical feedforward information flow of sensory stimuli from the thalamus via layer 4 spiny stellate neurons to layer 2/3 pyramidal neurons (Gilbert and Wiesel, 1979; Constantinople and Bruno, 2013), critical period ODP is expressed independently in each layer (Trachtenberg et al., 2000; Liu et al., 2008). Notably, depression in layer 2/3 occurs before depression in layer 4 (Trachtenberg et al., 2000). The mechanisms underlying LTD also differ in each layer. While low-frequency stimulation-induced LTD in both layer 4 and layer 2/3 depends on postsynaptic NMDARs, LTD in layer 4 is mediated by AMPAR endocytosis, whereas LTD in layer 2/3 is independent of AMPAR endocytosis but dependent on endocannabinoid receptor 1 (CB1R) activation (Rao and Daw, 2004; Crozier et al., 2007). When blocking LTD and ODP in layer 2/3 by CB1R blockers, ODP in layer 4 is still expressed (Liu et al., 2008) indicating that ODP in layer 2/3 is not a prerequisite for ODP in layer 4, even though layer 4 plasticity lags layer 2/3 plasticity. This independence might be partly caused by direct innervation of layer 2/3 from the thalamus, as demonstrated in other primary sensory cortices in mice (Petreanu et al., 2009).
A longer duration MD during the critical period (≥ 7 days) leads to a delayed increase of V1-responses to the non-deprived eye (Frenkel and Bear, 2004; Sato and Stryker, 2008) a phenomenon hypothesized to be due to homeostatic regulation after a prolonged decrease of deprived eye inputs (Kaneko et al., 2008b; Smith et al., 2009; Espinosa and Stryker, 2012; Ranson et al., 2012). Thus, depending on the duration of MD, interdependent mechanisms operate at different stages in the binocular region of the primary visual cortex to reorganize cortical neuron connectivity.
How do gestalt synapses integrate into the LTD-based expression mechanism of ODP? On the one hand, the presence of silent synapses defines the duration of the critical period for ODP (Huang X. et al., 2015; Favaro et al., 2018), and on the other hand, LTD is a mechanism of deprived eye depression in ODP, expressed by AMPAR endocytosis at least in layer 4 (Crozier et al., 2007; Yoon et al., 2009). These two concepts of mechanisms underlying critical period ODP appear paradoxical, as silent synapses lack AMPARs, which apparently conflicts with their direct involvement as substrates of LTD for critical period ODP. Additionally, with PSD-95 deficiency, silent synapses prevail and critical period ODP lasts lifelong, while LTD, as tested in the hippocampus, is impaired, a finding that also appears to be at odds with the role of LTD in critical period ODP (Carlisle et al., 2008; Xu et al., 2008; Huang X. et al., 2015). In fact, in PSD-95 KO mice, the threshold for LTP in the hippocampus is lower compared to wild-type mice (Migaud et al., 1998; Béïque et al., 2006; Carlisle et al., 2008). While this observation is consistent with the role of silent synapses as substrates for LTP (Isaac et al., 1995; Liao et al., 1995), it appears at odds with the prevailing high fraction of silent synapses in adult PSD-95 KO mice. If LTP is easier to achieve, why aren’t there more and stronger AMPAR+ synapses instead of silent synapses in adult PSD-95 KO mice? Conversely, in PSD-93 KO mice, silent synapses mature precociously and the critical period for ODP closes earlier, whereas the threshold for LTP in the hippocampus is reported to be elevated (Carlisle et al., 2008; Favaro et al., 2018). If LTP is harder to achieve, why aren’t there more silent synapses in adult PSD-93 KO mice? Thus, it appears that there is a paradox between the prevalence of silent synapses as substrates for ODP and LTD as the mechanism for ODP, as well as between the pace of maturation of silent synapses and the susceptibility of LTP in PSD-95 and PSD-93 KO mice.
At first sight, one might ask for additional experiments, as e.g., the mechanism of LTD underlying ODP in V1 layers 2/3 is distinct from NMDAR-dependent LTD in the hippocampus (Crozier et al., 2007; Xu et al., 2008). It is not known whether LTD in layer 2/3 is impaired in PSD-95 KO mice. While further studies are needed to fully resolve the paradox, some plausible predictions already unravel most of it. One key prediction drawn from the current literature that helps disentangle this apparent paradox is that the functional maturation of gestalt synapses processes through two steps, first unsilencing and then stabilization of synaptic AMPARs to consolidate a connection between two principal neurons, and the MD-induced LTD may happen at the unsilenced, but not stable gestalt synapses.
This two steps maturation process is implicated by studies of PSD-95 KO mice. In PSD-95 KO mice, the threshold for LTP in the hippocampus is low (Béïque et al., 2006; Carlisle et al., 2008). Consequently, memory acquisition in PSD-95 KO mice is normal or even better compared to WT mice (Fitzgerald et al., 2015; Shukla et al., 2017). However, memory retention is impaired. This disconnection can be explained as follows: NMDAR-dependent LTP drives the unsilencing of silent gestalt synapses in the hippocampus, and likely also in the visual cortex (Isaac et al., 1995; Kirkwood et al., 1995; Liao et al., 1995). The prevalence of silent gestalt synapses leads to more substrates for unsilencing, contributing to the lower threshold for LTP. However, the unsilenced gestalt synapses in PSD-95 KO mice are apparently not stable and AMPARs driven into them do not persist for longer time periods, as the fraction of silent synapses does not change throughout development. Furthermore, removing PSD-95 in adults will reinstate silent gestalt synapses (Huang X. et al., 2015). Thus, it is conceivable that silent gestalt synapses are initially converted into the unsilenced state, i.e., incorporate AMPARs into the postsynaptic membrane, but only transiently. The second step during gestalt synapse maturation, namely the stabilization of postsynaptic AMPARs, requires PSD-95.
During the critical period, some AMPAR+ synapses are thus unsilenced gestalt synapses, in an AMPAR+, but yet transient state. While both unsilencing and stabilization steps require correlated neural activity to drive LTP signaling pathways, at this stage, any uncorrelated neuronal activity can drive unsilenced gestalt synapses to depression, followed by pruning, so that the connection is lost during refinement (Figure 3). It is the very nature of the critical period that the network remains plastic until the most favorable configuration of the excitatory network is achieved. Thus, the prevalent unsilenced gestalt synapses that still need rounds of evaluation, and are vulnerable to depression, are the substrates for LTD. Depression is mediated by LTD or depotentiation, which are mechanistically similar, and will eventually lead to the pruning of some of the unsilenced AMPAR+ gestalt synapses. During MD, the lack of correlated activity in afferents of the deprived eye prevents stabilization of gestalt synapses, and thus favors the depression and pruning of unsilenced gestalt synapses, ultimately leading to the depression of the deprived eye inputs. This process is hypothesized to be unique to the critical period, most likely due to the prevalence of silent and unsilenced gestalt synapses that are not yet stabilized. Both processes, stabilization, and pruning of gestalt synapses, collectively lead to the decrease of silent synapses, and thus the decrease in the capability for plastic changes in mature, adult networks using this mechanism. A revealing test for this prediction will be the preservation of an LTD or depotentiation mechanism in the visual cortex of adult PSD-95 KO mice, presumably coupled with synapse pruning. Consistent with this prediction, the turnover of spines in the hippocampus of PSD-95-deficient neurons is elevated (Ehrlich et al., 2007).
Figure 3. Schematic diagram illustrating the hypothesized changes in eye specific inputs underlying experience-dependent plasticity in the primary visual cortex during the critical period for ODP. During the critical period, postsynaptic neurons in binocular V1 receive inputs from both eyes with a strong overrepresentation of contralateral eye inputs, via both innate synapses and silent, and unsilenced gestalt synapses. Unsilencing of silent gestalt synapses, synaptogenesis, and pruning are ongoing: innate synapses, silent gestalt synapses, unsilenced gestalt synapses and newly generated silent gestalt synapses are present. After the critical period (post-critical period), some previously unsilenced gestalt synapses are consolidated, while others are pruned away, stabilizing the synapses continuously receiving correlated inputs after unsilencing. Contralateral eye deprivation drives depression and preferential pruning of deprived eye inputs, causing an OD-shift toward the open/non-deprived/experienced, ipsilateral eye.
Studies on the role of PSD-93 in plasticity were performed primarily in the hippocampus, and at adult ages (4–12 months) when developmental processes have ceased and plasticity follows different rules. In PSD-93 KO mice, LTP with a classical high-frequency train is similar to that in WT mice. Only with non-classical conditioning stimuli with 5 Hz, a reduction in potentiation in PSD-93 KO mice emerges (Carlisle et al., 2008). However, the mechanism of this form of potentiation was not further explored. Given the presence of inhibitory transmission, a distinction between disinhibition and potentiation cannot be made at present. A direct role of PSD-93 in developmental LTP for unsilencing silent synapses needs further research. Considering the precocious maturation of silent synapses in PSD-93 KO/KD mice (Favaro et al., 2018), PSD-93 more likely inhibits both the unsilencing of silent gestalt synapses by LTP and the stabilization of unsilenced gestalt synapses during early development.
Our survey of the apparent paradox about the mechanisms of critical period plasticity elucidates that synapses may go through stepwise transitions during the critical period, from silent gestalt synapses to unsilenced gestalt synapses that then either get stabilized or depressed and ultimately pruned if not further stabilized (Figure 2B). Which of the different possible scenarios happens depends on Hebbian plasticity, and the whole process goes beyond the time window of LTP and LTD (Figure 2B). During these stepwise transitions, discrete molecular events will take place, orchestrated via signaling scaffold proteins and calcium signaling. These molecular events include the insertion and stabilization of AMPARs, the exchange of NMDAR subtypes (Quinlan et al., 1999; Philpot et al., 2001; Bellone and Nicoll, 2007), and likely also the dynamic changes of synaptic scaffold composition that eventually determine the fate of a particular synapse. Although some key players that control the signaling scaffolds and the signaling cascade have already been identified, the overall molecular landscape regulating the process remains elusive. Further studies are required to identify the critical pathways and players, and to elucidate the exact sequence of molecular events leading to network maturation during critical periods.
While we propose that the expression of ODP is primarily mediated by excitatory gestalt synapses, the opening of the critical period is likely primarily mediated by the maturation of local inhibitory circuits in V1 (Hensch et al., 1998; Fagiolini and Hensch, 2000). The fraction of silent gestalt synapses among all synapses does not peak at the beginning of critical periods. In the rodent visual cortex, their fraction is highest at, or even before eye-opening (Rumpel et al., 2004; Funahashi et al., 2013; Favaro et al., 2018) while the critical period for ODP in V1 begins at P21 (Espinosa and Stryker, 2012). Hence, the sole appearance of silent gestalt synapses provides substrates for critical period plasticity but does not open critical periods. The opening of the critical period requires the maturation of the inhibitory circuit (Hensch et al., 1998; Fagiolini and Hensch, 2000), whereas the duration of the critical period is dictated by the availability of silent gestalt synapses (Huang X. et al., 2015; Favaro et al., 2018). With the beginning of the critical period in the visual cortex, the potency of minimally evoked synaptic responses increases, indicating excitatory synapse maturation by AMPAR incorporation (Favaro et al., 2018). However, the fraction of silent synapses decreases already before the onset of the critical period. Given that the mEPSC frequency increases steeply before the critical period while the synaptic potency doesn’t (Desai et al., 2002; Corlew et al., 2007; Han et al., 2017; Favaro et al., 2018), we postulate that unsilencing silent synapses before critical periods enhances the overall functional connectivity in the cortical network, whereas unsilencing silent synapses during the critical period increases the weight of connectivity.
The opening of the critical period for ODP in rodent V1 is marked by a steep increase in the expression of α1 type of GABA-A receptors at PV+ interneuron-innervated postsynaptic sites of pyramidal neurons (Klausberger et al., 2002; Fagiolini et al., 2004; Huang X. et al., 2015). Theoretic models imply that lateral inhibition is important for stimulus selectivity (Nass and Cooper, 1975), which is required for MD induced changes in cortical networks, as laid out in the BCM theory for cortical plasticity (Bienenstock et al., 1982). Consistent with this model, intracortical blockade of GABA transmission in the kitten striate cortex lowered orientation selectivity in neuronal responses, accompanied by abnormal receptive field properties and reduced ocular dominance shifts (Ramoa et al., 1988). Reduced GABAergic transmission as seen in the GAD65 knock-out mice correlates with the lack of the opening of the critical period for ODP (Hensch et al., 1998). The correlation between the maturation of the inhibitory system and the opening of the critical period for ODP is also seen in genetic models, targeting specific regulatory genes and pharmacological interrogations. Interventions that accelerate inhibitory system maturation often lead to a precocious opening of critical periods, whereas interrogations that delay inhibitory system maturation, delay the opening of critical periods, as has been reported for both the visual and auditory cortex (Hensch, 2005; Espinosa and Stryker, 2012; Levelt and Hübener, 2012; Takesian et al., 2018). The maturation of the inhibitory network is initiated by inhibitory neurons of the 5-HT3AR positive subgroup by Neuregulin/ErbB4 signaling (Sun et al., 2016; Takesian et al., 2018) while the maturation of the strength of α1-type GABA-A receptor-positive synapses, presumably from PV+ interneurons to pyramidal neurons, may initiate the onset of the critical period for ODP (Fagiolini et al., 2004). Diazepam, a positive allosteric modulator of GABA-A receptors, rescues the ODP deficit in GAD65 knock-out mice (Hensch et al., 1998) and is sufficient to initiate a premature opening of the critical period for ODP in WT mice (Fagiolini and Hensch, 2000; Chen et al., 2014). These results indicate that mechanisms for critical period cortical plasticity are already in place without proper inhibitory transmission and that the maturation of the inhibitory network likely opens the critical period for ODP. As a result, the maturation of the inhibitory system may allow the excitatory drive to guide the experience-dependent excitatory network maturation (Chen et al., 2014).
Paradoxically, diazepam treatment during dark-rearing impairs ODP, suggesting that experience or the lack thereof modifies the inhibitory involvement in cortical plasticity (Iwai et al., 2003). Recent studies have demonstrated that prior to the critical period, specific synapses within the cortical network undergo distinct changes. The inhibitory synaptic strength of PV+ interneurons onto pyramidal neurons and of somatostatin+ (SST+) interneurons onto PV+ interneurons increases, while the synaptic strength of SST+ interneurons onto pyramidal neurons decreases. The excitatory synapses within the local cortical network exhibit a similar decrease in short-term depression in different target cell types (Miao et al., 2016). Additionally, interrogations that affect the maturation of inhibitory systems also lead to changes in expression of particular subtypes of NMDAR subunits. In GAD65 KO mice, the protein level of the NMDAR GluN2A subunit is decreased, whereas diazepam treatment rescues the decreased GluN2A protein levels (Kanold et al., 2009). These findings indicate an intricate interplay between the inhibitory and excitatory synapses within the local cortical circuit during the maturation process that controls the opening of critical periods.
In layer 2/3 pyramidal neurons, the feedforward excitatory and inhibitory drive are equilibrated, resulting in a similar AMPAR EPSC/GABA inhibitory postsynaptic current ratio (Xue et al., 2014). How does the impaired silent synapse maturation in PSD-95 KO mice affect the developmental increase in inhibitory transmission? In PSD-95 KO mice, the maturation of the inhibitory tone (normalized to NMDAR EPSCs) onto layer 2/3 pyramidal neurons during the critical period is similar to that in WT mice (Huang X. et al., 2015). Furthermore, the inhibitory tone in V1 of PSD-95 KO mice increases similar to that in WT mice throughout the critical period. Thus, the prolonged juvenile ODP in PSD-95 KO mice is likely not due to changes in the inhibitory system. ODP in juvenile and adult PSD-95 KO mice is similar to the critical period ODP in WT mice (Fagiolini et al., 2003; Huang X. et al., 2015). Together, these analyses indicate that the increased level of inhibition at the beginning of the critical period is permissive for critical period plasticity (Hensch et al., 1998; Fagiolini and Hensch, 2000) but further increases in inhibition are not instructive and do not end critical periods, because critical period ODP is present lifelong in PSD-95 KO mice, even in the presence of a high “adult” inhibitory tone (Huang X. et al., 2015).
Since cortical plasticity is also observed in adulthood, it suggests that the refined cortical excitatory networks can further optimize their functional output through progressive training throughout life. At the cellular level, it is known that the rate of synapse turnover is lower in adults, compared to that in critical periods (Holtmaat et al., 2005; Zuo et al., 2005) and the fraction of silent synapses is low, indicating a different molecular and cellular mechanism underlying adult cortical plasticity (Greifzu et al., 2014; Huang X. et al., 2015). It is therefore worthwhile to discuss what cellular and molecular mechanisms govern ODP at different developmental stages.
Prior to the critical period, basic cortical topography has already become organized, and specific visual cortical response properties such as orientation selectivity and ocular dominance are also established, although not yet with their mature features. During the critical period, under physiological conditions, many features of visual function are fine-tuned in an experience-dependent manner, particularly the tuning of neuronal responses to binocular input. It is thought that this tuning is necessary to achieve binocular matching for optimized vision, including binocular vision (Wang et al., 2010; Espinosa and Stryker, 2012).
The initial evidence of the expression mechanism for critical period ODP came from chronic single-unit recordings in kittens (Mioche and Singer, 1989): a short period (6–24 h) of MD led to a decrease of the excitatory responses to the deprived eye, while the excitatory responses to the non-deprived eye remained stable. In rodents, during the critical period, after a short period of MD (1 – 4 days), visual stimulation of the deprived compared to the non-deprived eye results in decreased VEP responses or decreased deprived eye responses, recorded by intrinsic signal optical imaging in the binocular region of V1, contralateral to the deprived eye (Sawtell et al., 2003; Sato and Stryker, 2008). Longer durations of MD (≥7 days), additionally lead to a delayed increase of V1-responses to the non-deprived eye (Frenkel and Bear, 2004; Sato and Stryker, 2008).
After the end of the critical period for ODP, V1 of standard cage-reared mice becomes increasingly less plastic to MD (Lehmann and Löwel, 2008; Sato and Stryker, 2008) and longer MD durations are necessary to induce an ocular dominance shift (Sawtell et al., 2003; Hofer et al., 2006; Lehmann and Löwel, 2008; Sato and Stryker, 2008; Hosang et al., 2018). In clear contrast to the rapid depression of the deprived eye responses in V1 consistently observed in young mice during the critical period for ODP, a hallmark of adult ODP is that MD rather induces potentiation of non-deprived eye responses with no initial depression of the deprived eye responses in V1 (Sawtell et al., 2003; Hofer et al., 2006; Sato and Stryker, 2008). These phenotypic differences between critical period ODP and adult ODP indicate that the underlying mechanisms also differ at different developmental stages (Ranson et al., 2012).
In adults, the mature network and the stabilized synapses do not favor dynamic changes of the excitatory network, spine formation and elimination are at a low rate in layer 5 neuron apical dendrites (Grutzendler et al., 2002; Zuo et al., 2005). Moreover, MD does not induce changes in spine dynamics in layer 2/3 neurons in adult but does so during the critical period (Hofer et al., 2009; Zhou et al., 2017). Additionally, both silent synapses and forms of LTD associated with ODP, which are the two cellular correlates of deprived eye depression during critical period ODP, are diminished in adults (Kirkwood et al., 1997; Heynen et al., 2003; Huang X. et al., 2015; Han et al., 2017). Thus, the silent synapse based refinement of excitatory networks, associated with critical period plasticity, is unlikely to operate in adult standard cage reared rodents.
Depending on the age, MD with different duration leads to non-deprived eye potentiation in adult rodents (Sawtell et al., 2003; Lehmann and Löwel, 2008; Hosang et al., 2018). Different from the critical period ODP, the pre-existing, deprived eye inputs are likely not specifically affected during the initial phase of MD. Additionally, the non-deprived eye potentiation is inhibited by a phosphorylation site mutant of CaMKII T286A, indicating an LTP-like mechanism (Ranson et al., 2012). This is distinct from the non-deprived eye potentiation after prolonged MD (at least 7 days) in juvenile ODP, mediated via homeostatic mechanisms independent of CaMKII activity (Ranson et al., 2012). This homeostatic potentiation is absent in adult ODP. Furthermore, even though hypo-NMDAR function prevented ODP in young adults (Sawtell et al., 2003), the NMDAR signaling in layer 4 principal neurons in adults is dispensable (Fong et al., 2020), suggesting a cell-type specific or layer-specific role of NMDARs in mediating adult ODP. It will require further investigation to identify the underlying synaptic changes and examine whether and how the adult network can recover to the pre-MD condition if MD happens in adulthood, as opposed to the long-lasting deficits caused by MD during the critical period.
A leading hypothesis for the closure of the critical period for ODP so far has been, that a number of so-called “plasticity breaks” (Hensch and Quinlan, 2018), such as the extracellular matrix, Nogo receptor 1 (NgR1), Paired immunoglobulin-like receptor B (PirB), Lynx1, class I major histocompatibility complex (MHC) are expressed and thus prevent critical period ODP (Huh et al., 2000; Pizzorusso et al., 2002; McGee et al., 2005; Syken et al., 2006; Morishita et al., 2010). Loss of function of these proteins leaves the visual cortex in a more plastic state in terms of expressing ODP. However, except for Lynx1 (Bukhari et al., 2015) the mechanisms underlying ODP were not investigated in detail, and it is not yet known whether OD shifts were mediated by deprived eye depression and/or open eye potentiation. Thus, further studies are needed to identify the specific role of the plasticity breaks in critical period ODP. Notably, all these proteins constitute potential candidates to govern the stabilization process of unsilenced gestalt synapses. However, the relationship of these breaks with silent synapses remains unexplored as their role in cortical plasticity was described before the silent synapse-based mechanisms of critical period plasticity were evidenced (Huang X. et al., 2015; Favaro et al., 2018).
Since there is lifelong learning, probably with different expression at network level compared to juvenile plasticity, it remains to be explored how molecular and cellular mechanisms of cortical plasticity differ between critical period and mature networks. Nevertheless, a two-step process emerges: First, during the critical period, the foundation of cortical processing architectures, or the gestalt of the cortical network is established. Second, in adults, the established cortical gestalt enables continuous learning and adaptations of the mature network to ever-changing environments and challenges within the boundary set early on, and by using plasticity mechanisms that are distinct from the principles governing critical period plasticity.
Studies using animals raised in diverse environments exhibit different degrees of ODP, implicating malleability in the expression of cortical plasticity. Results from these studies also shed significant light on the distinct expression mechanisms between critical period versus adult cortical plasticity. Most laboratory rodents are born and raised with a small number of animals (up to 5) in so-called standard cages, which are relatively small, usually translucent cages with woodchip bedding, and water and food ad libitum. Essentially, these cages represent a rather deprived environment compared to the environment that rodents encounter in the wild, outside the laboratory setting. Enriched environment cages likely provide a more physiological state than the standard laboratory cages. They are bigger, house a larger number of animals, and are usually equipped with running wheels, regularly changed labyrinths and toys to provide a variety of physical, social and cognitive stimulation (Löwel et al., 2017; Stryker and Löwel, 2018).
Deprivation of visual experience is generally used to study the role of experience in cortical plasticity. Either animals are raised in the dark from birth until the experimental time point, termed dark rearing, or animals are put into the dark at various developmental stages and for various durations, termed dark exposure. Combining these manipulations with ODP at different ages, one can query how deprivation of visual input can influence experience-dependent plasticity in young and adult animals.
After around postnatal day 110, mice raised in standard cages are no longer susceptible to MD, even if the MD was prolonged over 20 days (Lehmann and Löwel, 2008; Sato and Stryker, 2008). It is known that giving animals the opportunity of enhanced physical, social, and cognitive stimulation strongly affects physiology, plasticity, and behavior (van Praag et al., 2000; Nithianantharajah and Hannan, 2006; Löwel et al., 2017). Raising mice or rats in an enriched environment alters the expression of several key signaling molecules involved in regulating brain excitability and plasticity (Cancedda et al., 2004), increases the volume of brain areas (Diamond et al., 1964; Beaulieu and Colonnier, 1987), and alters maternal behavior (Sale et al., 2004). Furthermore, enrichment extends ODP into adulthood (Baroncelli et al., 2010a; Greifzu et al., 2014), accelerates experience-dependent V1-activity changes (Kalogeraki et al., 2017), and allows ODP to persist even throughout life (Greifzu et al., 2016). Placing standard cage-reared rodents into an enriched environment as adults, restores ODP (Sale et al., 2007; Baroncelli et al., 2010b, 2012; Scali et al., 2012; Greifzu et al., 2014, 2016), while transferring mice from an enriched environment to standard cages might prevent ODP susceptibility (Kalogeraki et al., 2017).
Some mechanistic insights come from studies on behaviorally interrogated mice with an enriched environment and dark exposure. Both enriched environment and dark exposure, cause a decrease in the inhibitory tone (Huang et al., 2010; Greifzu et al., 2014) and both enable adult ODP. Importantly, with the enriched environment, as little as 4 days of MD in adult mice (>P90) already induced non-deprived eye potentiation (Kalogeraki et al., 2017), and thus accelerated the adult form of ODP, indicating that the decrease of the inhibitory tone facilitates plasticity of the adult cortical network.
We would like to stress that ODP of enriched mice after the critical period, is mediated through non-deprived eye potentiation in V1, a hallmark of adult ODP, already after 4 days of MD (Figure 4). Thus, the V1-activity changes in adult enriched mice resemble adult ODP (of standard cage housed-mice) with a faster onset but include an additional not yet characterized component of late depression. After 7 days of MD, reductions of both deprived and non-deprived eye responses in V1 have been observed in adult enriched mice (Kalogeraki et al., 2017). Notably, such reductions in stimulus-induced V1-activity were only seen in mice up to about P300, while beyond P400, only increases in non-deprived eye V1-responses were observed after 7 days of MD (Greifzu et al., 2016). In the earlier work (Greifzu et al., 2014, 2016) ODP was only measured after 7 days of MD, the initial non-deprived eye potentiation was missed, and the results were misinterpreted as providing evidence for “juvenile”-like ODP in adult enriched mice (Greifzu et al., 2014). Given the initial non-deprived eye potentiation after MD in adult enriched mice, the later deprived eye depression is likely mediated by other mechanisms than the juvenile-type of plasticity. Thus, deprived eye input may still exist, though weakened after 7 days of MD. Taken together, ODP in adult enriched mice is different from critical period ODP (Figure 4: open eye potentiation versus deprived eye depression with 4 days of MD), whereas mechanistically similar to ODP in adult standard cage housed-mice (non-deprived eye potentiation), but faster (Kalogeraki et al., 2017).
Figure 4. Difference in ODP between juvenile (critical period) and adult mice raised in an enriched environment (EE) (modified from Kalogeraki et al., 2017 use with permission): (A) Optically imaged ocular dominance index (ODI). Symbols represent individual ODI-values, means are marked by horizontal lines; values after MD are indicated by half-black squares. (B) V1-activation elicited by stimulation of the contralateral or ipsilateral eye without and with MD (black filled circle indicates the deprived eye). In critical period EE-mice, OD-shifts are mediated by a reduction of V1-activation via the deprived (contralateral = C) eye, as in standard cage raised critical period-mice (red arrow pointing down). In contrast, beyond the critical period, OD-shifts in adult EE-mice are mediated via a fast increase of V1-activation through the open (non-deprived, ipsilateral = I) eye (red arrow pointing up). Quantification of V1-activation after stimulation of the ipsilateral (I) or contralateral (C) eye, recorded by intrinsic signal optical imaging in EE-mice of two different age groups before and after MD. Intra- and intergroup comparisons were analyzed either by a two-tailed t-test or one-way ANOVA followed by multiple comparisons Bonferroni correction. The levels of significance were set as *p < 0.05; **p < 0.01; ***p < 0.001. Data are represented as means ± SEM.
Consistent with previous work in cats (Cynader and Mitchell, 1980; Timney et al., 1980; Cynader, 1983) and rats (Fagiolini et al., 1994), dark-reared mice display a delayed critical period (Chen et al., 2014), and potentially the window for ODP is prolonged into “adult” age (Fagiolini et al., 2003; Gianfranceschi et al., 2003; Favaro et al., 2018). Furthermore, when a period of dark exposure is started in adulthood, MD can again induce ODP in adult standard cage housed rodents (Guire et al., 1999; He et al., 2006; Stodieck et al., 2014). Remarkably, dark exposure after P70 leads to functional improvements in rats with amblyopia (He et al., 2007). After 3 days of MD, deprived eye responses are reduced as well as non-deprived eye responses increased (He et al., 2006). In mice, after dark exposure, ODP is mediated by an increase of non-deprived eye responses in V1 after 7 days of MD (Stodieck et al., 2014). The simultaneous expression of both, reduction of deprived eye responses and increase of non-deprived eye responses after short MD in dark exposed adults, is distinct from both critical period and adult ODP with an enriched environment.
Together, these results show that both enriched environment and dark exposure (deprivation of visual experience) enhance ODP compared to standard cage rodents. It is intriguing how the two seemingly opposite manipulations lead to a phenotypically similar outcome. While mechanisms, such as metaplasticity with experience-dependent modifications in NMDAR subunit composition by dark rearing (Philpot et al., 2001; Corlew et al., 2007), and changes in inhibitory tone with the enriched environment (Greifzu et al., 2014) have been proposed, further experiments will be necessary to elaborate the mechanistic differences and potential similarities in the plasticity after dark exposure and that in an enriched environment. Notably, no induction of silent gestalt synapses was observed with enriched environment exposure (Greifzu et al., 2014) indicating that at least environmental enrichment is not effective in rejuvenating the cortical network for critical period plasticity.
The process of critical period network refinement through the step-wise maturation of excitatory synapses is essential for proper perception in adulthood. For example, juvenile barn owls can learn abnormal associations between auditory cues and locations in visual space if their visual input is displaced by wearing prism goggles, and these abnormal associations are recalled in adulthood if the owls re-experience the displaced sensory inputs by again wearing prism goggles (Linkenhoker et al., 2005). However, this adaptation is hampered if the owls experience the abnormal association for the first time in adulthood when plasticity is limited. Thus experience during critical periods shapes the gestalt of neural networks which is essential for the ability to perceive certain sensory associations later on.
In the rodent visual system, visual perception, as measured by orientation discrimination, is impaired if critical periods close precociously as in PSD-93 KO mice, or if the closure is impaired as in PSD-95 KO mice (Huang X. et al., 2015; Favaro et al., 2018). In the absence of both PSD-93 and PSD-95, visual perception in mice is further compromised and only innate visual capabilities remain, indicating that either PSD-93 or PSD-95 is required for establishing gestalt synapses (Favaro et al., 2018). Further studies will be needed to explore the features of synapses lacking both PSD-93 and PSD-95, and whether only innate synapses are left in double KO mice. Furthermore, mice with a postnatal knock-down of neurogranin in the visual cortex were impaired in perceiving a visual cliff (Han et al., 2017). While it is not yet clear whether the loss of neurogranin specifically affects pruning of gestalt synapses, the impaired silent gestalt synapse maturation is consistent with their role in visual perception.
Binocular matching of orientation tuning and binocular vision require experience-dependent cortical plasticity during critical periods and if this is prevented by dark rearing or genetically impairing critical period refinement, these visual features are otherwise impaired in adulthood (Kaye et al., 1981; Mower et al., 1985; Crair et al., 1998; Wang et al., 2010; Han et al., 2017). However, maturation of visual acuity, a visual function thought to be controlled at the thalamic level, appears less sensitive to either genetic or sensory interrogations of the time course of critical periods, as it is unaffected by dark-rearing, or loss of either PSD-93 or PSD-95 (Rochefort et al., 2011; Kang et al., 2013; Huang X. et al., 2015; Favaro et al., 2018). While more detailed mechanistic studies are still needed, these results highlight the critical function of the signaling scaffolds PSD-93 and PSD-95, as well as neurogranin in coordinating the proper pace and balance of silent-synapse based cortical excitatory network maturation during critical periods (Huang X. et al., 2015; Favaro et al., 2018; Han et al., 2017). The specific impairments in this process set a premise to untangle the cellular mechanisms of critical period principal neuron network refinement.
Judging from the clinical experience in treating neurodevelopmental disorders, merely inducing or enhancing adult plasticity is unlikely sufficient for long-term modification of the network, as a potential pathway to correct network dysfunction caused by developmental deficits. Thus, one provocative idea is to try to rejuvenate networks by engaging critical period plasticity to reorganize/repair connectivity in adulthood. Hijacking the rejuvenation mechanism promises a potential approach that could lead to long-lasting reorganization of the cortical network to correct network dysfunction, such as amblyopia but also other neurodevelopmental disorders that are caused by developmental deficits. Knocking down PSD-95 in adult mice was already shown to restore the fraction of silent synapses to the eye-opening level, and also to reinstate critical period ODP (Huang X. et al., 2015). Together, these observations suggest that the network can be reverted to express critical period plasticity. Additionally, a rejuvenation of the excitatory network has also been successfully induced in the brain’s reward circuit under pathological conditions with drugs of abuse. Cocaine challenge induces newly formed silent synapses in this circuit, which leads to long-lasting network modifications that contribute to the obsessive drug-seeking behavior in a rodent model of addiction (Huang et al., 2009; Huang Y. H. et al., 2015; Wright et al., 2020). Both approaches have disadvantages due to the lack of perceptual maturation when PSD-95 levels are low, and the potential of drug addiction. Nevertheless, understanding the molecular underpinnings and cellular processes that guide excitatory network maturation during critical periods will help to figure out whether adult neural networks can be rejuvenated for network reorganization/repair.
Using the critical period for ODP in the primary visual cortex as a model system, the field has gained significant insight into understanding the experience-dependent refinement of cortical networks during postnatal development. This process builds the fundamental architecture for achieving optimal functional output after later progressive training (Figure 1). Both excitatory and inhibitory systems operate hand in hand to open the critical period, and to define the duration of critical period synaptic plasticity. In recent years, it also became clear that the plasticity mechanisms during the critical period are different from those in adults. We propose a model with two distinct types of synapses, innate synapses that establish rudimentary networks with innate function, and gestalt synapses that govern the experience-dependent refinement process. The maturation of silent gestalt synapses, transitioning via experience-dependent unsilencing and stabilization is a fundamental step in strengthening functional ensembles of the cortical network. The generation, maturation, or pruning of gestalt synapses governs experience-dependent network maturation, and is the essential mechanism for the developmental refinement of cortical principal neuron networks (Figures 2, 3). While this review focuses on the functional analyses of silent synapses in cortical layer 2/3 pyramidal neurons, silent synapses are prevalent at different developmental stages also in cortical layer 5 (Anastasiades and Butt, 2012), hippocampus (Isaac et al., 1995; Liao et al., 1995), and nucleus accumbens (Huang et al., 2009). Experience-dependent spine dynamics, as proxies of the plasticity of excitatory networks, have been observed in both cortical layer 2/3 and layer 5 (Holtmaat et al., 2005; Zuo et al., 2005). Potential differences in network dynamics in different layers and brain regions warrant further investigations. This silent synapse-based network refinement is likely generalizable to all excitatory networks in sensory cortices, as well as to higher-order cortical networks controlling cognition and executive functions, such as the prefrontal cortex. Despite extensive studies on critical period plasticity and the role of silent synapses in Hebbian plasticity, it is only recently that their defining role in critical period plasticity was revealed, opening the door for further studies to elucidate the underlying molecular mechanisms.
While our new working model raises many questions and challenges, it also provides testable hypotheses and may thus guide further research. First, although we deduced different functional categories of synapses and transitions through different states during experience-dependent network maturation, it remains unknown how the different synapse categories and states are defined at the molecular level. While lack of PSD-95 prevents the transition from unsilenced gestalt synapses to stable gestalt synapses, little is known about the key signaling cascades that control this transition. Our model postulates that unsilenced gestalt synapses are the substrates for LTD and pruning during critical period refinement, which in turn constitutes the expression mechanism of deprived eye depression during MD (Figures 2, 3). Based on this model, the unsilencing and stabilization of gestalt synapses are distinct processes. While LTP drives AMPARs into the gestalt synapses, the stabilization process goes beyond the LTP time window to finalize the maturation.
Our recent studies provide some initial insights into the molecular mechanisms governing experience-dependent maturation of excitatory synapses, and identified three key players: neurogranin, PSD-95, and PSD-93 (Figure 2B). While PSD-95 drives the maturation of silent synapses, PSD-93 opposes this function. Neurogranin is a key switch governing the fate of gestalt synapses for maturation or through regulating post-synaptic calcium/CaM-dependent signaling. Further investigations of the molecular landscape associated with these key players will help to further identify molecular mechanisms for the different state transitions during the experience-dependent synapse and network maturation process, such as synapse pruning and synaptogenesis of gestalt synapses during critical periods and how they interact with synapse maturation.
New players are emerging from recent studies, yet, it remains critical to apply the molecular approaches combined with functional analyses to further elucidate how the identified targets regulate excitatory network maturation and perceptual capabilities. Furthermore, cellular and molecular identities involved in glia and astrocyte signaling in regulating postnatal synaptogenesis and synapse elimination warrant further investigation. Finally, the intriguing array of proteins that put a break to critical period plasticity should be investigated in light of synaptic state transitions. Together, the new analyses may answer the key question whether it is possible to preserve or reactivate the generation of silent synapses in the adult brain, to rejuvenate the network gestalt for a critical period-like plasticity to “repair” abnormal configurations of the neuronal networks.
All authors listed have made a substantial, direct and intellectual contribution to the work, and approved it for publication.
SL (CRC889 “Cellular Mechanisms of Sensory Processing,” Project B5; Federal Ministry of Education and Research, Germany, Grant 01GQ0810); the Campus Institute for Dynamics of Biological Networks (CIDBN) is funded by the Ministry for Science and Education of Lower Saxony and the Volkswagen Foundation through the program “Niedersächsisches Vorab”; OS (NIH NS107604, Whitehall Foundation 2018-05-68, CRC889 “Cellular Mechanisms of Sensory Processing”; Project B3); WX (NIH MH118298, JPB Foundation, Broad Institute Stanley Center Neuropsychiatric Initiative, Whitehall Foundation).
The authors declare that the research was conducted in the absence of any commercial or financial relationships that could be construed as a potential conflict of interest.
We thank former and current members of our laboratories, and many colleagues for their insightful discussions and work, especially Dr. Mark F. Bear who helped shape these ideas, and Dr. Ben Philpot for his helpful comments.
Aamodt, S. M., Nordeen, E. J., and Nordeen, K. W. (1996). Blockade of NMDA receptors during song model exposure impairs song development in juvenile zebra finches. Neurobiol. Learn. Mem. 65, 91–98. doi: 10.1006/nlme.1996.0010
Abraham, W. C., and Bear, M. F. (1996). Metaplasticity: the plasticity of synaptic plasticity. Trends Neurosci. 19, 126–130. doi: 10.1016/s0166-2236(96)80018-x
Akgul, G., and Wollmuth, L. P. (2010). Expression pattern of membrane-associated guanylate kinases in interneurons of the visual cortex. J. Comp. Neurol. 518, 4842–4854. doi: 10.1002/cne.22491
Anastasiades, P. G., and Butt, S. J. (2012). A role for silent synapses in the development of the pathway from layer 2/3 to 5 pyramidal cells in the neocortex. J. Neurosci. 32, 13085–13099. doi: 10.1523/JNEUROSCI.1262-12.2012
Balice-Gordon, R. J., and Lichtman, J. W. (1994). Long-term synapse loss induced by focal blockade of postsynaptic receptors. Nature 372, 519–524. doi: 10.1038/372519a0
Baroncelli, L., Bonaccorsi, J., Milanese, M., Bonifacino, T., Giribaldi, F., Manno, I., et al. (2012). Enriched experience and recovery from amblyopia in adult rats: impact of motor, social and sensory components. Neuropharmacology 62, 2388–2397. doi: 10.1016/j.neuropharm.2012.02.010
Baroncelli, L., Braschi, C., Spolidoro, M., Begenisic, T., Sale, A., and Maffei, L. (2010a). Nurturing brain plasticity: impact of environmental enrichment. Cell Death Differ. 17, 1092–1103. doi: 10.1038/cdd.2009.193
Baroncelli, L., Sale, A., Viegi, A., Maya Vetencourt, J. F., De Pasquale, R., Baldini, S., et al. (2010b). Experience-dependent reactivation of ocular dominance plasticity in the adult visual cortex. Exp. Neurol. 226, 100–109. doi: 10.1016/j.expneurol.2010.08.009
Bear, M. F., Kleinschmidt, A., Gu, Q. A., and Singer, W. (1990). Disruption of experience-dependent synaptic modifications in striate cortex by infusion of an NMDA receptor antagonist. J. Neurosci. 10, 909–925.
Bear, M. F., and Singer, W. (1986). Modulation of visual cortical plasticity by acetylcholine and noradrenaline. Nature 320, 172–176. doi: 10.1038/320172a0
Beaulieu, C., and Colonnier, M. (1987). Effect of the richness of the environment on the cat visual cortex. J. Comp. Neurol. 266, 478–494. doi: 10.1002/cne.902660404
Béïque, J.-C., Lin, D.-T., Kang, M.-G., Aizawa, H., Takamiya, K., and Huganir, R. L. (2006). Synapse-specific regulation of AMPA receptor function by PSD-95. Proc. Natl. Acad. Sci. U.S.A. 103, 19535–19540. doi: 10.1073/pnas.0608492103
Bellone, C., and Nicoll, R. A. (2007). Rapid bidirectional switching of synaptic NMDA receptors. Neuron 55, 779–785. doi: 10.1016/j.neuron.2007.07.035
Bian, W. J., Miao, W. Y., He, S. J., Qiu, Z., and Yu, X. (2015). Coordinated spine pruning and maturation mediated by inter-spine competition for Cadherin/Catenin complexes. Cell 162, 808–822. doi: 10.1016/j.cell.2015.07.018
Bienenstock, E. L., Cooper, L. N., and Munro, P. W. (1982). Theory for the development of neuron selectivity: orientation specificity and binocular interaction in visual cortex. J. Neurosci. 2, 32–48.
Birch, E. E. (2013). Amblyopia and binocular vision. Prog. Retin. Eye Res. 33, 67–84. doi: 10.1016/j.preteyeres.2012.11.001
Birdsong, D. (2018). Plasticity, variability and age in second language acquisition and bilingualism. Front. Psychol. 9:81. doi: 10.3389/fpsyg.2018.00081
Blakemore, C., Garey, L. J., and Vital-Durand, F. (1978). The physiological effects of monocular deprivation and their reversal in the monkey’s visual cortex. J. Physiol. 283, 223–262. doi: 10.1113/jphysiol.1978.sp012498
Bottjer, S. W. (2005). Silent synapses in a thalamo-cortical circuit necessary for song learning in zebra finches. J. Neurophysiol. 94, 3698–3707. doi: 10.1152/jn.00282.2005
Bourgeois, J. P., and Rakic, P. (1993). Changes of synaptic density in the primary visual cortex of the macaque monkey from fetal to adult stage. J. Neurosci. 13, 2801–2820.
Boyer, C., Schikorski, T., and Stevens, C. F. (1998). Comparison of hippocampal dendritic spines in culture and in brain. J. Neurosci. 18, 5294–5300.
Brainard, M. S., and Doupe, A. J. (2002). What songbirds teach us about learning. Nature 417, 351–358. doi: 10.1038/417351a
Bukhari, N., Burman, P. N., Hussein, A., Demars, M. P., Sadahiro, M., Brady, D. M., et al. (2015). Unmasking proteolytic activity for adult visual cortex plasticity by the removal of Lynx1. J. Neurosci. 35, 12693–12702. doi: 10.1523/JNEUROSCI.4315-14.2015
Callaway, E. M., and Katz, L. C. (1990). Emergence and refinement of clustered horizontal connections in cat striate cortex. J. Neurosci. 10, 1134–1153.
Cancedda, L., Putignano, E., Sale, A., Viegi, A., Berardi, N., and Maffei, L. (2004). Acceleration of visual system development by environmental enrichment. J. Neurosci. 24, 4840–4848. doi: 10.1523/JNEUROSCI.0845-04.2004
Cane, M., Maco, B., Knott, G., and Holtmaat, A. (2014). The relationship between PSD-95 clustering and spine stability in vivo. J. Neurosci. 34, 2075–2086. doi: 10.1523/JNEUROSCI.3353-13.2014
Cang, J., Kalatsky, V. A., Löwel, S., and Stryker, M. P. (2005). Optical imaging of the intrinsic signal as a measure of cortical plasticity in the mouse. Vis. Neurosci. 22, 685–691. doi: 10.1017/S0952523805225178
Carlisle, H. J., Fink, A. E., Grant, S. G., and O’Dell, T. J. (2008). Opposing effects of PSD-93 and PSD-95 on long-term potentiation and spike timing-dependent plasticity. J. Physiol. 586, 5885–5900. doi: 10.1113/jphysiol.2008.163469
Changeux, J. P., and Danchin, A. (1976). Selective stabilisation of developing synapses as a mechanism for the specification of neuronal networks. Nature 264, 705–712. doi: 10.1038/264705a0
Chen, X. J., Rasch, M. J., Chen, G., Ye, C. Q., Wu, S., and Zhang, X. H. (2014). Binocular input coincidence mediates critical period plasticity in the mouse primary visual cortex. J. Neurosci. 34, 2940–2955. doi: 10.1523/JNEUROSCI.2640-13.2014
Christopherson, K. S., Ullian, E. M., Stokes, C. C., Mullowney, C. E., Hell, J. W., Agah, A., et al. (2005). Thrombospondins are astrocyte-secreted proteins that promote CNS synaptogenesis. Cell 120, 421–433. doi: 10.1016/j.cell.2004.12.020
Chung, W. S., Allen, N. J., and Eroglu, C. (2015). Astrocytes control synapse formation. function, and elimination. Cold Spring Harb. Perspect. Biol. 7:a020370. doi: 10.1101/cshperspect.a020370
Chung, W. S., Clarke, L. E., Wang, G. X., Stafford, B. K., Sher, A., Chakraborty, C., et al. (2013). Astrocytes mediate synapse elimination through MEGF10 and MERTK pathways. Nature 504, 394–400. doi: 10.1038/nature12776
Clarke, L. E., and Barres, B. A. (2013). Emerging roles of astrocytes in neural circuit development. Nat. Rev. Neurosci. 14, 311–321. doi: 10.1038/nrn3484
Colman, H., Nabekura, J., and Lichtman, J. W. (1997). Alterations in synaptic strength preceding axon withdrawal. Science 275, 356–361. doi: 10.1126/science.275.5298.356
Constantinople, C. M., and Bruno, R. M. (2013). Deep cortical layers are activated directly by thalamus. Science 340, 1591–1594. doi: 10.1126/science.1236425
Corlew, R., Wang, Y., Ghermazien, H., Erisir, A., and Philpot, B. D. (2007). Developmental switch in the contribution of presynaptic and postsynaptic NMDA receptors to long-term depression. J. Neurosci. 27, 9835–9845. doi: 10.1523/JNEUROSCI.5494-06.2007
Crair, M. C., Gillespie, D. C., and Stryker, M. P. (1998). The role of visual experience in the development of columns in cat visual cortex. Science 279, 566–570. doi: 10.1126/science.279.5350.566
Crozier, R. A., Wang, Y., Liu, C. H., and Bear, M. F. (2007). Deprivation-induced synaptic depression by distinct mechanisms in different layers of mouse visual cortex. Proc. Natl. Acad. Sci. U.S.A. 104, 1383–1388. doi: 10.1073/pnas.0609596104
Cruz-Martin, A., Crespo, M., and Portera-Cailliau, C. (2010). Delayed stabilization of dendritic spines in fragile X mice. J. Neurosci. 30, 7793–7803. doi: 10.1523/JNEUROSCI.0577-10.2010
Cynader, M. (1983). Prolonged sensitivity to monocular deprivation in dark-reared cats: effects of age and visual exposure. Brain Res. 284, 155–164. doi: 10.1016/0165-3806(83)90002-0
Cynader, M., and Mitchell, D. E. (1980). Prolonged sensitivity to monocular deprivation in dark-reared cats. J. Neurophysiol. 43, 1026–1040. doi: 10.1152/jn.1980.43.4.1026
Dailey, M. E., and Smith, S. J. (1996). The dynamics of dendritic structure in developing hippocampal slices. J. Neurosci. 16, 2983–2994.
Danielson, E., Zhang, N., Metallo, J., Kaleka, K., Shin, S. M., Gerges, N., et al. (2012). S-SCAM/MAGI-2 is an essential synaptic scaffolding molecule for the GluA2-containing maintenance pool of AMPA receptors. J. Neurosci. 32, 6967–6980. doi: 10.1523/JNEUROSCI.0025-12.2012
Desai, N. S., Cudmore, R. H., Nelson, S. B., and Turrigiano, G. G. (2002). Critical periods for experience-dependent synaptic scaling in visual cortex. Nat. Neurosci. 5, 783–789. doi: 10.1038/nn878
Diamond, M. C., Krech, D., and Rosenzweig, M. R. (1964). The Effects of an Enriched Environment on the Histology of the Rat Cerebral Cortex. J. Comp. Neurol. 123, 111–120. doi: 10.1002/cne.901230110
Dräger, U. C. (1978). Observations on monocular deprivation in mice. J. Neurophysiol. 41, 28–42. doi: 10.1152/jn.1978.41.1.28
Dudek, S. M., and Friedlander, M. J. (1996). Developmental down-regulation of LTD in cortical layer IV and its independence of modulation by inhibition. Neuron 16, 1097–1106. doi: 10.1016/s0896-6273(00)80136-1
Dunaevsky, A., Tashiro, A., Majewska, A., Mason, C., and Yuste, R. (1999). Developmental regulation of spine motility in the mammalian central nervous system. Proc. Natl. Acad. Sci. U.S.A. 96, 13438–13443. doi: 10.1073/pnas.96.23.13438
Ehrlich, I., Klein, M., Rumpel, S., and Malinow, R. (2007). PSD-95 is required for activity-driven synapse stabilization. Proc. Natl. Acad. Sci. U.S.A. 104, 4176–4181. doi: 10.1073/pnas.0609307104
Elias, G. M., Funke, L., Stein, V., Grant, S. G. N., Bredt, D. S., and Nicoll, R. A. (2006). Synapse-specific and developmentally regulated targeting of AMPA receptors by a family of MAGUK scaffolding proteins. Neuron 52, 307–320. doi: 10.1016/j.neuron.2006.09.012
Eroglu, C., Allen, N. J., Susman, M. W., O’Rourke, N. A., Park, C. Y., Ozkan, E., et al. (2009). Gabapentin receptor alpha2delta-1 is a neuronal thrombospondin receptor responsible for excitatory CNS synaptogenesis. Cell 139, 380–392. doi: 10.1016/j.cell.2009.09.025
Espinosa, J. S., and Stryker, M. P. (2012). Development and plasticity of the primary visual cortex. Neuron 75, 230–249. doi: 10.1016/j.neuron.2012.06.009
Fagiolini, M., Fritschy, J. M., Löw, K., Möhler, H., Rudolph, U., and Hensch, T. K. (2004). Specific GABAA circuits for visual cortical plasticity. Science 303, 1681–1683. doi: 10.1126/science.1091032
Fagiolini, M., and Hensch, T. K. (2000). Inhibitory threshold for critical-period activation in primary visual cortex. Nature 404, 183–186. doi: 10.1038/35004582
Fagiolini, M., Katagiri, H., Miyamoto, H., Mori, H., Grant, S. G., Mishina, M., et al. (2003). Separable features of visual cortical plasticity revealed by N-methyl-D-aspartate receptor 2A signaling. Proc. Natl. Acad. Sci. U.S.A. 100, 2854–2859. doi: 10.1073/pnas.0536089100
Fagiolini, M., Pizzorusso, T., Berardi, N., Domenici, L., and Maffei, L. (1994). Functional postnatal development of the rat primary visual cortex and the role of visual experience: dark rearing and monocular deprivation. Vision Res. 34, 709–720.
Favaro, P. D., Huang, X., Hosang, L., Stodieck, S., Cui, L., Liu, Y. Z., et al. (2018). An opposing function of paralogs in balancing developmental synapse maturation. PLoS Biol. 16:e2006838. doi: 10.1371/journal.pbio.2006838
Feldman, D. E., Brainard, M. S., and Knudsen, E. I. (1996). Newly learned auditory responses mediated by NMDA receptors in the owl inferior colliculus. Science 271, 525–528. doi: 10.1126/science.271.5248.525
Feldman, D. E., and Knudsen, E. I. (1997). An anatomical basis for visual calibration of the auditory space map in the barn owl’s midbrain. J. Neurosci. 17, 6820–6837.
Feldman, R., Braun, K., and Champagne, F. A. (2019). The neural mechanisms and consequences of paternal caregiving. Nat. Rev. Neurosci. 20, 205–224. doi: 10.1038/s41583-019-0124-6
Feldmeyer, D., Lübke, J., Silver, R. A., and Sakmann, B. (2002). Synaptic connections between layer 4 spiny neurone-layer 2/3 pyramidal cell pairs in juvenile rat barrel cortex: physiology and anatomy of interlaminar signalling within a cortical column. J. Physiol. 538(Pt 3), 803–822. doi: 10.1113/jphysiol.2001.012959
Fitzgerald, P. J., Pinard, C. R., Camp, M. C., Feyder, M., Sah, A., Bergstrom, H. C., et al. (2015). Durable fear memories require PSD-95. Mol. Psychiatry 20, 901–912. doi: 10.1038/mp.2014.161
Fong, M. F., Finnie, P. S., Kim, T., Thomazeau, A., Kaplan, E. S., Cooke, S. F., et al. (2020). Distinct laminar requirements for NMDA receptors in experience-dependent visual cortical plasticity. Cereb. Cortex. 30, 2555–2572. doi: 10.1093/cercor/bhz260
Frenkel, M. Y., and Bear, M. F. (2004). How monocular deprivation shifts ocular dominance in visual cortex of young mice. Neuron 44, 917–923. doi: 10.1016/j.neuron.2004.12.003
Funahashi, R., Maruyama, T., Yoshimura, Y., and Komatsu, Y. (2013). Silent synapses persist into adulthood in layer 2/3 pyramidal neurons of visual cortex in dark-reared mice. J. Neurophysiol. 109, 2064–2076. doi: 10.1152/jn.00912.2012
Gandhi, S. P., Yanagawa, Y., and Stryker, M. P. (2008). Delayed plasticity of inhibitory neurons in developing visual cortex. Proc. Natl. Acad. Sci. U.S.A. 105, 16797–16802. doi: 10.1073/pnas.0806159105
Gianfranceschi, L., Siciliano, R., Walls, J., Morales, B., Kirkwood, A., Huang, Z. J., et al. (2003). Visual cortex is rescued from the effects of dark rearing by overexpression of BDNF. Proc. Natl. Acad. Sci. U.S.A. 100, 12486–12491. doi: 10.1073/pnas.1934836100
Gilbert, C. D., and Wiesel, T. N. (1979). Morphology and intracortical projections of functionally characterised neurones in the cat visual cortex. Nature 280, 120–125. doi: 10.1038/280120a0
Gordon, J. A., and Stryker, M. P. (1996). Experience-dependent plasticity of binocular responses in the primary visual cortex of the mouse. J. Neurosci. 16, 3274–3286.
Greifzu, F., Parthier, D., Goetze, B., Schlüter, O. M., and Löwel, S. (2016). Ocular dominance plasticity after stroke was preserved in PSD-95 knockout mice. PLoS One 11:e0149771. doi: 10.1371/journal.pone.0149771
Greifzu, F., Pielecka-Fortuna, J., Kalogeraki, E., Krempler, K., Favaro, P. D., Schlüter, O. M., et al. (2014). Environmental enrichment extends ocular dominance plasticity into adulthood and protects from stroke-induced impairments of plasticity. Proc. Natl. Acad. Sci. U.S.A. 111, 1150–1155. doi: 10.1073/pnas.1313385111
Grimshaw, G. M., Adelstein, A., Bryden, M. P., and MacKinnon, G. E. (1998). First-language acquisition in adolescence: evidence for a critical period for verbal language development. Brain Lang. 63, 237–255. doi: 10.1006/brln.1997.1943
Grutzendler, J., Kasthuri, N., and Gan, W. B. (2002). Long-term dendritic spine stability in the adult cortex. Nature 420, 812–816. doi: 10.1038/nature01276
Guire, E. S., Lickey, M. E., and Gordon, B. (1999). Critical period for the monocular deprivation effect in rats: assessment with sweep visually evoked potentials. J. Neurophysiol. 81, 121–128. doi: 10.1152/jn.1999.81.1.121
Han, K.-S., Cooke, S. F., and Xu, W. (2017). Experience-dependent equilibration of AMPAR-mediated synaptic transmission during the critical period. Cell Rep. 18, 892–904. doi: 10.1016/j.celrep.2016.12.084
Harris, K. M., Jensen, F. E., and Tsao, B. (1992). Three-dimensional structure of dendritic spines and synapses in rat hippocampus (CA1) at postnatal day 15 and adult ages: implications for the maturation of synaptic physiology and long-term potentiation. J. Neurosci. 12, 2685–2705.
He, H. Y., Hodos, W., and Quinlan, E. M. (2006). Visual deprivation reactivates rapid ocular dominance plasticity in adult visual cortex. J. Neurosci. 26, 2951–2955. doi: 10.1523/JNEUROSCI.5554-05.2006
He, H. Y., Ray, B., Dennis, K., and Quinlan, E. M. (2007). Experience-dependent recovery of vision following chronic deprivation amblyopia. Nat. Neurosci. 10, 1134–1136. doi: 10.1038/nn1965
Hensch, T. K. (2005). Critical period plasticity in local cortical circuits. Nat. Rev. Neurosci. 6, 877–888. doi: 10.1038/nrn1787
Hensch, T. K., Fagiolini, M., Mataga, N., Stryker, M. P., Baekkeskov, S., and Kash, S. F. (1998). Local GABA circuit control of experience-dependent plasticity in developing visual cortex. Science 282, 1504–1508. doi: 10.1126/science.282.5393.1504
Hensch, T. K., and Quinlan, E. M. (2018). Critical periods in amblyopia. Vis. Neurosci. 35:E014. doi: 10.1017/S0952523817000219
Heynen, A. J., Yoon, B. J., Liu, C. H., Chung, H. J., Huganir, R. L., and Bear, M. F. (2003). Molecular mechanism for loss of visual cortical responsiveness following brief monocular deprivation. Nat. Neurosci. 6, 854–862. doi: 10.1038/nn1100
Hodges, J. L., Yu, X., Gilmore, A., Bennett, H., Tjia, M., Perna, J. F., et al. (2017). Astrocytic contributions to synaptic and learning abnormalities in a mouse model of fragile X syndrome. Biol. Psychiatry 82, 139–149. doi: 10.1016/j.biopsych.2016.08.036
Hofer, S. B., Mrsic-Flogel, T. D., Bonhoeffer, T., and Hübener, M. (2006). Prior experience enhances plasticity in adult visual cortex. Nat. Neurosci. 9, 127–132. doi: 10.1038/nn1610
Hofer, S. B., Mrsic-Flogel, T. D., Bonhoeffer, T., and Hübener, M. (2009). Experience leaves a lasting structural trace in cortical circuits. Nature 457, 313–317. doi: 10.1038/nature07487
Holtmaat, A. J., Trachtenberg, J. T., Wilbrecht, L., Shepherd, G. M., Zhang, X., Knott, G. W., et al. (2005). Transient and persistent dendritic spines in the neocortex in vivo. Neuron 45, 279–291. doi: 10.1016/j.neuron.2005.01.003
Hooks, B. M., and Chen, C. (2007). Critical periods in the visual system: changing views for a model of experience-dependent plasticity. Neuron 56, 312–326. doi: 10.1016/j.neuron.2007.10.003
Hosang, L., Yusifov, R., and Löwel, S. (2018). Long-term visual training increases visual acuity and long-term monocular deprivation promotes ocular dominance plasticity in adult standard cage-raised mice. eNeuro 5:ENEURO.0289-17.2017. doi: 10.1523/ENEURO.0289-17.2017
Huang, S., Gu, Y., Quinlan, E. M., and Kirkwood, A. (2010). A refractory period for rejuvenating GABAergic synaptic transmission and ocular dominance plasticity with dark exposure. J. Neurosci. 30, 16636–16642. doi: 10.1523/JNEUROSCI.4384-10.2010
Huang, X., Stodieck, S. K., Goetze, B., Cui, L., Wong, M. H., Wenzel, C., et al. (2015). Progressive maturation of silent synapses governs the duration of a critical period. Proc. Natl. Acad. Sci. U.S.A. 112, E3131–E3140. doi: 10.1073/pnas.1506488112
Huang, Y. H., Lin, Y., Mu, P., Lee, B. R., Brown, T. E., Wayman, G., et al. (2009). In vivo cocaine experience generates silent synapses. Neuron 63, 40–47. doi: 10.1016/j.neuron.2009.06.007
Huang, Y. H., Schlüter, O. M., and Dong, Y. (2015). Silent synapses speak up: updates of the neural rejuvenation hypothesis of drug addiction. Neuroscientist 21, 451–459. doi: 10.1177/1073858415579405
Hubel, D. H., and Wiesel, T. N. (1963). Receptive fields of cells in striate cortex of very young, visually inexperienced kittens. J. Neurophysiol. 26, 994–1002. doi: 10.1152/jn.1963.26.6.994
Hubel, D. H., Wiesel, T. N., and LeVay, S. (1977). Plasticity of ocular dominance columns in monkey striate cortex. Philos. Trans. R. Soc. Lond. B Biol. Sci. 278, 377–409. doi: 10.1098/rstb.1977.0050
Huh, G. S., Boulanger, L. M., Du, H., Riquelme, P. A., Brotz, T. M., and Shatz, C. J. (2000). Functional requirement for class I MHC in CNS development and plasticity. Science 290, 2155–2159. doi: 10.1126/science.290.5499.2155
Huttenlocher, P. R. (1979). Synaptic density in human frontal cortex - developmental changes and effects of aging. Brain Res. 163, 195–205. doi: 10.1016/0006-8993(79)90349-4
Huttenlocher, P. R., and Dabholkar, A. S. (1997). Regional differences in synaptogenesis in human cerebral cortex. J. Comp. Neurol. 387, 167–178.
Hwang, H., Szucs, M. J., Ding, L. J., Allen, A., Haensgen, H., Gao, F., et al. (2018). A schizophrenia risk gene, NRGN, bidirectionally modulates synaptic plasticity via regulating the neuronal phosphoproteome. bioRxiv [Preprint]. doi: 10.1101/481291
Isaac, J. T., Crair, M. C., Nicoll, R. A., and Malenka, R. C. (1997). Silent synapses during development of thalamocortical inputs. Neuron 18, 269–280. doi: 10.1016/s0896-6273(00)80267-6
Isaac, J. T., Hjelmstad, G. O., Nicoll, R. A., and Malenka, R. C. (1996). Long-term potentiation at single fiber inputs to hippocampal CA1 pyramidal cells. Proc. Natl. Acad. Sci. U.S.A. 93, 8710–8715. doi: 10.1073/pnas.93.16.8710
Isaac, J. T. R., Nicoll, R. A., and Malenka, R. C. (1995). Evidence for silent synapses: implications for the expression of LTP. Neuron 15, 427–434.
Issa, N. P., Trachtenberg, J. T., Chapman, B., Zahs, K. R., and Stryker, M. P. (1999). The critical period for ocular dominance plasticity in the Ferret’s visual cortex. J. Neurosci. 19, 6965–6978.
Iwai, Y., Fagiolini, M., Obata, K., and Hensch, T. K. (2003). Rapid critical period induction by tonic inhibition in visual cortex. J. Neurosci. 23, 6695–6702.
Jang, M., Um, K. B., Jang, J., Kim, H. J., Cho, H., Chung, S., et al. (2015). Coexistence of glutamatergic spine synapses and shaft synapses in substantia nigra dopamine neurons. Sci. Rep. 5:14773. doi: 10.1038/srep14773
Jiang, B., Trevino, M., and Kirkwood, A. (2007). Sequential development of long-term potentiation and depression in different layers of the mouse visual cortex. J. Neurosci. 27, 9648–9652. doi: 10.1523/JNEUROSCI.2655-07.2007
Jones, K. R., Spear, P. D., and Tong, L. (1984). Critical periods for effects of monocular deprivation: differences between striate and extrastriate cortex. J. Neurosci. 4, 2543–2552.
Juraska, J. M., and Fifkova, E. (1979). An electron microscope study of the early postnatal development of the visual cortex of the hooded rat. J. Comp. Neurol. 183, 257–267. doi: 10.1002/cne.901830204
Kalatsky, V. A., and Stryker, M. P. (2003). New paradigm for optical imaging: temporally encoded maps of intrinsic signal. Neuron 38, 529–545. doi: 10.1016/s0896-6273(03)00286-1
Kalogeraki, E., Greifzu, F., Haack, F., and Löwel, S. (2014). Voluntary physical exercise promotes ocular dominance plasticity in adult mouse primary visual cortex. J. Neurosci. 34, 15476–15481. doi: 10.1523/JNEUROSCI.2678-14.2014
Kalogeraki, E., Pielecka-Fortuna, J., and Löwel, S. (2017). Environmental enrichment accelerates ocular dominance plasticity in mouse visual cortex whereas transfer to standard cages resulted in a rapid loss of increased plasticity. PLoS One 12:e0186999. doi: 10.1371/journal.pone.0186999
Kameyama, K., Sohya, K., Ebina, T., Fukuda, A., Yanagawa, Y., and Tsumoto, T. (2010). Difference in binocularity and ocular dominance plasticity between GABAergic and excitatory cortical neurons. J. Neurosci. 30, 1551–1559. doi: 10.1523/JNEUROSCI.5025-09.2010
Kaneko, M., Hanover, J. L., England, P. M., and Stryker, M. P. (2008a). TrkB kinase is required for recovery, but not loss, of cortical responses following monocular deprivation. Nat. Neurosci. 11, 497–504. doi: 10.1038/nn2068
Kaneko, M., Stellwagen, D., Malenka, R. C., and Stryker, M. P. (2008b). Tumor necrosis factor-alpha mediates one component of competitive, experience-dependent plasticity in developing visual cortex. Neuron 58, 673–680. doi: 10.1016/j.neuron.2008.04.023
Kang, E., Durand, S., LeBlanc, J. J., Hensch, T. K., Chen, C., and Fagiolini, M. (2013). Visual acuity development and plasticity in the absence of sensory experience. J. Neurosci. 33, 17789–17796. doi: 10.1523/JNEUROSCI.1500-13.2013
Kanold, P. O., Kim, Y. A., GrandPre, T., and Shatz, C. J. (2009). Co-regulation of ocular dominance plasticity and NMDA receptor subunit expression in glutamic acid decarboxylase-65 knock-out mice. J. Physiol. 587(Pt 12), 2857–2867. doi: 10.1113/jphysiol.2009.171215
Kasthuri, N., and Lichtman, J. W. (2003). The role of neuronal identity in synaptic competition. Nature 424, 426–430. doi: 10.1038/nature01836
Katz, L. C., and Crowley, J. C. (2002). Development of cortical circuits: lessons from ocular dominance columns. Nat. Rev. Neurosci. 3, 34–42. doi: 10.1038/nrn703
Katz, L. C., and Shatz, C. J. (1996). Synaptic activity and the construction of cortical circuits. Science 274, 1133–1138. doi: 10.1126/science.274.5290.1133
Kaye, M., Mitchell, D. E., and Cynader, M. (1981). Depth perception, eye alignment and cortical ocular dominance of dark-related cats. Brain Res. 254, 37–53. doi: 10.1016/0165-3806(81)90057-2
Khibnik, L. A., Cho, K. K., and Bear, M. F. (2010). Relative contribution of feedforward excitatory connections to expression of ocular dominance plasticity in layer 4 of visual cortex. Neuron 66, 493–500. doi: 10.1016/j.neuron.2010.04.012
Kirkwood, A., Lee, H. K., and Bear, M. F. (1995). Co-regulation of long-term potentiation and experience-dependent synaptic plasticity in visual cortex by age and experience. Nature 375, 328–331. doi: 10.1038/375328a0
Kirkwood, A., Silva, A., and Bear, M. F. (1997). Age-dependent decrease of synaptic plasticity in the neocortex of alphaCaMKII mutant mice. Proc. Natl. Acad. Sci. U.S.A. 94, 3380–3383. doi: 10.1073/pnas.94.7.3380
Klausberger, T., Roberts, J. D., and Somogyi, P. (2002). Cell type- and input-specific differences in the number and subtypes of synaptic GABA(A) receptors in the hippocampus. J. Neurosci. 22, 2513–2521.
Konur, S., and Yuste, R. (2004). Developmental regulation of spine and filopodial motility in primary visual cortex: reduced effects of activity and sensory deprivation. J. Neurobiol. 59, 236–246. doi: 10.1002/neu.10306
Krüger, J. M., Favaro, P. D., Liu, M., Kitlinska, A., Huang, X., Raabe, M., et al. (2013). Differential roles of postsynaptic density-93 isoforms in regulating synaptic transmission. J. Neurosci. 33, 15504–15517. doi: 10.1523/JNEUROSCI.0019-12.2013
Kuhlman, S. J., Olivas, N. D., Tring, E., Ikrar, T., Xu, X., and Trachtenberg, J. T. (2013). A disinhibitory microcircuit initiates critical-period plasticity in the visual cortex. Nature 501, 543–546. doi: 10.1038/nature12485
Lehmann, K., and Löwel, S. (2008). Age-dependent ocular dominance plasticity in adult mice. PLoS One 3:e3120. doi: 10.1371/journal.pone.0003120
Lendvai, B., Stern, E. A., Chen, B., and Svoboda, K. (2000). Experience-dependent plasticity of dendritic spines in the developing rat barrel cortex in vivo. Nature 404, 876–881. doi: 10.1038/35009107
LeVay, S. (1973). Synaptic patterns in the visual cortex of the cat and monkey. Electron microscopy of Golgi preparations. J. Comp. Neurol. 150, 53–85. doi: 10.1002/cne.901500104
LeVay, S., Wiesel, T. N., and Hubel, D. H. (1980). The development of ocular dominance columns in normal and visually deprived monkeys. J. Comp. Neurol. 191, 1–51. doi: 10.1002/cne.901910102
Levelt, C. N., and Hübener, M. (2012). Critical-period plasticity in the visual cortex. Annu. Rev. Neurosci. 35, 309–330. doi: 10.1146/annurev-neuro-061010-113813
Levy, J. M., and Nicoll, R. A. (2017). Membrane-associated guanylate kinase dynamics reveal regional and developmental specificity of synapse stability. J. Physiol. 595, 1699–1709. doi: 10.1113/JP273147
Liao, D. S., Hessler, N. A., and Malinow, R. (1995). Activation of postsynaptically silent synapses during pairing-induced LTP in CA1 region of hippocampal slice. Nature 375, 400–404. doi: 10.1038/375400a0
Linkenhoker, B. A., von, der Ohe, C. G., and Knudsen, E. I. (2005). Anatomical traces of juvenile learning in the auditory system of adult barn owls. Nat. Neurosci. 8, 93–98. doi: 10.1038/nn1367
Liu, C. H., Heynen, A. J., Shuler, M. G., and Bear, M. F. (2008). Cannabinoid receptor blockade reveals parallel plasticity mechanisms in different layers of mouse visual cortex. Neuron 58, 340–345. doi: 10.1016/j.neuron.2008.02.020
Liu, Y., Cui, L., Schwarz, M. K., Dong, Y., and Schlüter, O. M. (2017). Adrenergic gate release for spike timing-dependent synaptic potentiation. Neuron 93, 394–408. doi: 10.1016/j.neuron.2016.12.039
Löwel, S., Kalogeraki, E., Dehmel, S., and Makowiecki, K. (2017). Environmental conditions strongly affect brain plasticity. e-Neuroforum 24, A19–A29. doi: 10.1515/nf-2017-A050
Löwel, S., and Singer, W. (1992). Selection of intrinsic horizontal connections in the visual cortex by correlated neuronal activity. Science 255, 209–212. doi: 10.1126/science.1372754
Ma, W. P., Li, Y. T., and Tao, H. W. (2013). Downregulation of cortical inhibition mediates ocular dominance plasticity during the critical period. J. Neurosci. 33, 11276–11280. doi: 10.1523/JNEUROSCI.5598-12.2013
Majewska, A., and Sur, M. (2003). Motility of dendritic spines in visual cortex in vivo: changes during the critical period and effects of visual deprivation. Proc. Natl. Acad. Sci. U.S.A. 100, 16024–16029. doi: 10.1073/pnas.2636949100
Mataga, N., Mizuguchi, Y., and Hensch, T. K. (2004). Experience-dependent pruning of dendritic spines in visual cortex by tissue plasminogen activator. Neuron 44, 1031–1041. doi: 10.1016/j.neuron.2004.11.028
McGee, A. W., Yang, Y., Fischer, Q. S., Daw, N. W., and Strittmatter, S. M. (2005). Experience-driven plasticity of visual cortex limited by myelin and Nogo receptor. Science 309, 2222–2226. doi: 10.1126/science.1114362
Miao, Q., Yao, L., Rasch, M. J., Ye, Q., Li, X., and Zhang, X. (2016). Selective maturation of temporal dynamics of intracortical excitatory transmission at the critical period onset. Cell Rep. 16, 1677–1689. doi: 10.1016/j.celrep.2016.07.013
Migaud, M., Charlesworth, P., Dempster, M., Webster, L. C., Watabe, A. M., Makhinson, M., et al. (1998). Enhanced long-term potentiation and impaired learning in mice with mutant postsynaptic density-95 protein. Nature 396, 433–439. doi: 10.1038/24790
Miller, K. D., Keller, J. B., and Stryker, M. P. (1989). Ocular dominance column development: analysis and simulation. Science 245, 605–615. doi: 10.1126/science.2762813
Miller, M., and Peters, A. (1981). Maturation of rat visual cortex. II. A combined Golgi-electron microscope study of pyramidal neurons. J. Comp. Neurol. 203, 555–573. doi: 10.1002/cne.902030402
Mioche, L., and Singer, W. (1989). Chronic recordings from single sites of kitten striate cortex during experience-dependent modifications of receptive-field properties. J. Neurophysiol. 62, 185–197. doi: 10.1152/jn.1989.62.1.185
Morishita, H., Miwa, J. M., Heintz, N., and Hensch, T. K. (2010). Lynx1, a cholinergic brake, limits plasticity in adult visual cortex. Science 330, 1238–1240. doi: 10.1126/science.1195320
Mower, G. D., Caplan, C. J., Christen, W. G., and Duffy, F. H. (1985). Dark rearing prolongs physiological but not anatomical plasticity of the cat visual cortex. J. Comp. Neurol. 235, 448–466. doi: 10.1002/cne.902350404
Mrsic-Flogel, T. D., Hofer, S. B., Ohki, K., Reid, R. C., Bonhoeffer, T., and Hübener, M. (2007). Homeostatic regulation of eye-specific responses in visual cortex during ocular dominance plasticity. Neuron 54, 961–972. doi: 10.1016/j.neuron.2007.05.028
Munoz-Cueto, J. A., Garcia-Segura, L. M., and Ruiz-Marcos, A. (1991). Regional sex differences in spine density along the apical shaft of visual cortex pyramids during postnatal development. Brain Res. 540, 41–47. doi: 10.1016/0006-8993(91)90490-m
Nägerl, U. V., Eberhorn, N., Cambridge, S. B., and Bonhoeffer, T. (2004). Bidirectional activity-dependent morphological plasticity in hippocampal neurons. Neuron 44, 759–767. doi: 10.1016/j.neuron.2004.11.016
Nakagawa, T., Futai, K., Lashuel, H. A., Lo, I., Okamoto, K., Walz, T., et al. (2004). Quaternary structure, protein dynamics, and synaptic function of SAP97 controlled by L27 domain interactions. Neuron 44, 453–467. doi: 10.1016/j.neuron.2004.10.012
Nass, M. M., and Cooper, L. N. (1975). A theory for the development of feature detecting cells in visual cortex. Biol. Cybern. 19, 1–18. doi: 10.1007/bf00319777
Neniskyte, U., and Gross, C. T. (2017). Errant gardeners: glial-cell-dependent synaptic pruning and neurodevelopmental disorders. Nat. Rev. Neurosci. 18, 658–670. doi: 10.1038/nrn.2017.110
Nithianantharajah, J., and Hannan, A. J. (2006). Enriched environments, experience-dependent plasticity and disorders of the nervous system. Nat. Rev. Neurosci. 7, 697–709. doi: 10.1038/nrn1970
O’Leary, D. D., Chou, S. J., and Sahara, S. (2007). Area patterning of the mammalian cortex. Neuron 56, 252–269. doi: 10.1016/j.neuron.2007.10.010
Pak, J. H., Huang, F. L., Li, J., Balschun, D., Reymann, K. G., Chiang, C., et al. (2000). Involvement of neurogranin in the modulation of calcium/calmodulin-dependent protein kinase II, synaptic plasticity, and spatial learning: a study with knockout mice. Proc. Natl. Acad. Sci. U.S.A. 97, 11232–11237.
Paolicelli, R. C., Bolasco, G., Pagani, F., Maggi, L., Scianni, M., Panzanelli, P., et al. (2011). Synaptic pruning by microglia is necessary for normal brain development. Science 333, 1456–1458. doi: 10.1126/science.1202529
Petreanu, L., Mao, T., Sternson, S. M., and Svoboda, K. (2009). The subcellular organization of neocortical excitatory connections. Nature 457, 1142–1145. doi: 10.1038/nature07709
Philpot, B. D., Sekhar, A. K., Shouval, H. Z., and Bear, M. F. (2001). Visual experience and deprivation bidirectionally modify the composition and function of NMDA receptors in visual cortex. Neuron 29, 157–169. doi: 10.1016/s0896-6273(01)00187-8
Pizzorusso, T., Medini, P., Berardi, N., Chierzi, S., Fawcett, J. W., and Maffei, L. (2002). Reactivation of ocular dominance plasticity in the adult visual cortex. Science 298, 1248–1251. doi: 10.1126/science.1072699
Prather, J. F., Okanoya, K., and Bolhuis, J. J. (2017). Brains for birds and babies: neural parallels between birdsong and speech acquisition. Neurosci. Biobehav. Rev. 81(Pt B), 225–237. doi: 10.1016/j.neubiorev.2016.12.035
Prusky, G. T., and Douglas, R. M. (2003). Developmental plasticity of mouse visual acuity. Eur. J. Neurosci. 17, 167–173. doi: 10.1046/j.1460-9568.2003.02420.x
Purves, D., and Lichtman, J. W. (1980). Elimination of synapses in the developing nervous system. Science 210, 153–157. doi: 10.1126/science.7414326
Quinlan, E. M., Philpot, B. D., Huganir, R. L., and Bear, M. F. (1999). Rapid, experience-dependent expression of synaptic NMDA receptors in visual cortex in vivo. Nat. Neurosci. 2, 352–357. doi: 10.1038/7263
Rakic, P., Bourgeois, J. P., Eckenhoff, M. F., Zecevic, N., and Goldman-Rakic, P. S. (1986). Concurrent overproduction of synapses in diverse regions of the primate cerebral cortex. Science 232, 232–235. doi: 10.1126/science.3952506
Ramoa, A. S., Paradiso, M. A., and Freeman, R. D. (1988). Blockade of intracortical inhibition in kitten striate cortex: effects on receptive field properties and associated loss of ocular dominance plasticity. Exp. Brain Res. 73, 285–296. doi: 10.1007/bf00248220
Ranson, A., Cheetham, C. E., Fox, K., and Sengpiel, F. (2012). Homeostatic plasticity mechanisms are required for juvenile, but not adult, ocular dominance plasticity. Proc. Natl. Acad. Sci. U.S.A. 109, 1311–1316. doi: 10.1073/pnas.1112204109
Rao, Y., and Daw, N. W. (2004). Layer variations of long-term depression in rat visual cortex. J. Neurophysiol. 92, 2652–2658. doi: 10.1152/jn.00298.2004
Reilly, J. E., Hanson, H. H., and Phillips, G. R. (2011). Persistence of excitatory shaft synapses adjacent to newly emerged dendritic protrusions. Mol. Cell. Neurosci. 48, 129–136. doi: 10.1016/j.mcn.2011.06.014
Rittenhouse, C. D., Shouval, H. Z., Paradiso, M. A., and Bear, M. F. (1999). Monocular deprivation induces homosynaptic long-term depression in visual cortex. Nature 397, 347–350. doi: 10.1038/16922
Roberts, T. F., Tschida, K. A., Klein, M. E., and Mooney, R. (2010). Rapid spine stabilization and synaptic enhancement at the onset of behavioural learning. Nature 463, 948–952. doi: 10.1038/nature08759
Rochefort, N. L., Narushima, M., Grienberger, C., Marandi, N., Hill, D. N., and Konnerth, A. (2011). Development of direction selectivity in mouse cortical neurons. Neuron 71, 425–432. doi: 10.1016/j.neuron.2011.06.013
Rothblat, L. A., Schwartz, M. L., and Kasdan, P. M. (1978). Monocular deprivation in the rat: evidence for an age-related defect in visual behavior. Brain Res. 158, 456–460. doi: 10.1016/0006-8993(78)90689-3
Rumpel, S., Kattenstroth, G., and Gottmann, K. (2004). Silent synapses in the immature visual cortex: layer-specific developmental regulation. J. Neurophysiol. 91, 1097–1101. doi: 10.1152/jn.00443.2003
Sale, A., Maya Vetencourt, J. F., Medini, P., Cenni, M. C., Baroncelli, L., De Pasquale, R., et al. (2007). Environmental enrichment in adulthood promotes amblyopia recovery through a reduction of intracortical inhibition. Nat. Neurosci. 10, 679–681. doi: 10.1038/nn1899
Sale, A., Putignano, E., Cancedda, L., Landi, S., Cirulli, F., Berardi, N., et al. (2004). Enriched environment and acceleration of visual system development. Neuropharmacology 47, 649–660. doi: 10.1016/j.neuropharm.2004.07.008
Sato, M., and Stryker, M. P. (2008). Distinctive features of adult ocular dominance plasticity. J. Neurosci. 28, 10278–10286. doi: 10.1523/JNEUROSCI.2451-08.2008
Sawtell, N. B., Frenkel, M. Y., Philpot, B. D., Nakazawa, K., Tonegawa, S., and Bear, M. F. (2003). NMDA receptor-dependent ocular dominance plasticity in adult visual cortex. Neuron 38, 977–985. doi: 10.1016/s0896-6273(03)00323-4
Scali, M., Baroncelli, L., Cenni, M. C., Sale, A., and Maffei, L. (2012). A rich environmental experience reactivates visual cortex plasticity in aged rats. Exp. Gerontol. 47, 337–341. doi: 10.1016/j.exger.2012.01.007
Schafer, D. P., Lehrman, E. K., Kautzman, A. G., Koyama, R., Mardinly, A. R., Yamasaki, R., et al. (2012). Microglia sculpt postnatal neural circuits in an activity and complement-dependent manner. Neuron 74, 691–705. doi: 10.1016/j.neuron.2012.03.026
Schlüter, O. M., Xu, W., and Malenka, R. C. (2006). Alternative N-terminal domains of PSD-95 and SAP97 govern activity-dependent regulation of synaptic AMPA receptor function. Neuron 51, 99–111. doi: 10.1016/j.neuron.2006.05.016
Shatz, C. J., and Stryker, M. P. (1978). Ocular dominance in layer IV of the cat’s visual cortex and the effects of monocular deprivation. J. Physiol. 281, 267–283. doi: 10.1113/jphysiol.1978.sp012421
Shatz, C. J., and Stryker, M. P. (1988). Prenatal tetrodotoxin infusion blocks segregation of retinogeniculate afferents. Science 242, 87–89. doi: 10.1126/science.3175636
Shukla, A., Beroun, A., Panopoulou, M., Neumann, P. A., Grant, S. G., Olive, M. F., et al. (2017). Calcium-permeable AMPA receptors and silent synapses in cocaine-conditioned place preference. EMBO J. 36, 458–474. doi: 10.15252/embj.201695465
Simon, D. K., Prusky, G. T., O’Leary, D. D., and Constantine-Paton, M. (1992). N-methyl-D-aspartate receptor antagonists disrupt the formation of a mammalian neural map. Proc. Natl. Acad. Sci. U.S.A. 89, 10593–10597. doi: 10.1073/pnas.89.22.10593
Singer, W. (1995). Development and plasticity of cortical processing architectures. Science 270, 758–764. doi: 10.1126/science.270.5237.758
Smith, G. B., Heynen, A. J., and Bear, M. F. (2009). Bidirectional synaptic mechanisms of ocular dominance plasticity in visual cortex. Philos. Trans. R. Soc. Lond. B Biol. Sci. 364, 357–367. doi: 10.1098/rstb.2008.0198
Stein, V., House, D. R., Bredt, D. S., and Nicoll, R. A. (2003). Postsynaptic density-95 mimics and occludes hippocampal long-term potentiation and enhances long-term depression. J. Neurosci. 23, 5503–5506.
Stevens, B., Allen, N. J., Vazquez, L. E., Howell, G. R., Christopherson, K. S., Nouri, N., et al. (2007). The classical complement cascade mediates CNS synapse elimination. Cell 131, 1164–1178. doi: 10.1016/j.cell.2007.10.036
Stodieck, S. K., Greifzu, F., Goetze, B., Schmidt, K. F., and Löwel, S. (2014). Brief dark exposure restored ocular dominance plasticity in aging mice and after a cortical stroke. Exp. Gerontol. 60, 1–11. doi: 10.1016/j.exger.2014.09.007
Stryker, M. P., and Löwel, S. (2018). Amblyopia: new molecular/pharmacological and environmental approaches. Vis. Neurosci. 35:E018. doi: 10.1017/S0952523817000256
Sun, H., Takesian, A. E., Wang, T. T., Lippman-Bell, J. J., Hensch, T. K., and Jensen, F. E. (2018). Early seizures prematurely unsilence auditory synapses to disrupt thalamocortical critical period plasticity. Cell Rep. 23, 2533–2540. doi: 10.1016/j.celrep.2018.04.108
Sun, Y., Ikrar, T., Davis, M. F., Gong, N., Zheng, X., Luo, Z. D., et al. (2016). Neuregulin-1/ErbB4 signaling regulates visual cortical plasticity. Neuron 92, 160–173. doi: 10.1016/j.neuron.2016.08.033
Sun, Y. J., Espinosa, J. S., Hoseini, M. S., and Stryker, M. P. (2019). Experience-dependent structural plasticity at pre- and postsynaptic sites of layer 2/3 cells in developing visual cortex. Proc. Natl. Acad. Sci. U.S.A. 116, 21812–21820. doi: 10.1073/pnas.1914661116
Syken, J., Grandpre, T., Kanold, P. O., and Shatz, C. J. (2006). PirB restricts ocular-dominance plasticity in visual cortex. Science 313, 1795–1800. doi: 10.1126/science.1128232
Takesian, A. E., Bogart, L. J., Lichtman, J. W., and Hensch, T. K. (2018). Inhibitory circuit gating of auditory critical-period plasticity. Nat. Neurosci. 21, 218–227. doi: 10.1038/s41593-017-0064-2
Thakar, S., Wang, L., Yu, T., Ye, M., Onishi, K., Scott, J., et al. (2017). Evidence for opposing roles of Celsr3 and Vangl2 in glutamatergic synapse formation. Proc. Natl. Acad. Sci. U.S.A. 114, E610–E618. doi: 10.1073/pnas.1612062114
Timney, B., Mitchell, D. E., and Cynader, M. (1980). Behavioral evidence for prolonged sensitivity to effects of monocular deprivation in dark-reared cats. J. Neurophysiol. 43, 1041–1054. doi: 10.1152/jn.1980.43.4.1041
Trachtenberg, J. T., Chen, B. E., Knott, G. W., Feng, G., Sanes, J. R., Welker, E., et al. (2002). Long-term in vivo imaging of experience-dependent synaptic plasticity in adult cortex. Nature 420, 788–794. doi: 10.1038/nature01273
Trachtenberg, J. T., Trepel, C., and Stryker, M. P. (2000). Rapid extragranular plasticity in the absence of thalamocortical plasticity in the developing primary visual cortex. Science 287, 2029–2032. doi: 10.1126/science.287.5460.2029
Tropea, D., Majewska, A. K., Garcia, R., and Sur, M. (2010). Structural dynamics of synapses in vivo correlate with functional changes during experience-dependent plasticity in visual cortex. J. Neurosci. 30, 11086–11095. doi: 10.1523/JNEUROSCI.1661-10.2010
van Praag, H., Kempermann, G., and Gage, F. H. (2000). Neural consequences of environmental enrichment. Nat. Rev. Neurosci. 1, 191–198. doi: 10.1038/35044558
Walsh, M. K., and Lichtman, J. W. (2003). In vivo time-lapse imaging of synaptic takeover associated with naturally occurring synapse elimination. Neuron 37, 67–73. doi: 10.1016/s0896-6273(02)01142-x
Wang, B. S., Sarnaik, R., and Cang, J. (2010). Critical period plasticity matches binocular orientation preference in the visual cortex. Neuron 65, 246–256. doi: 10.1016/j.neuron.2010.01.002
Wang, J., Li, K.-L., Shukla, A., Beroun, A., Ishikawa, M., Huang, X., et al. (2020). Cocaine triggers Glial-mediated synaptogenesis. bioRxiv [Preprint]. doi: 10.1101/2020.01.20.896233
Webber, A. L., and Wood, J. (2005). Amblyopia: prevalence, natural history, functional effects and treatment. Clin. Exp. Optom. 88, 365–375. doi: 10.1111/j.1444-0938.2005.tb05102.x
Wiesel, T. N., and Hubel, D. H. (1963a). Effects of visual deprivation on morphology and physiology of cells in the cats lateral geniculate body. J. Neurophysiol. 26, 978–993. doi: 10.1152/jn.1963.26.6.978
Wiesel, T. N., and Hubel, D. H. (1963b). Single-cell responses in striate cortex of kittens deprived of vision in one eye. J. Neurophysiol. 26, 1003–1017. doi: 10.1152/jn.1963.26.6.1003
Wright, W. J., Graziane, N. M., Neumann, P. A., Hamilton, P. J., Cates, H. M., Fuerst, L., et al. (2020). Silent synapses dictate cocaine memory destabilization and reconsolidation. Nat. Neurosci. 23, 32–46. doi: 10.1038/s41593-019-0537-6
Xu, W., Schlüter, O. M., Steiner, P., Czervionke, B. L., Sabatini, B. L., and Malenka, R. C. (2008). Molecular dissociation of the role of PSD-95 in regulating synaptic strength and LTD. Neuron 57, 248–262. doi: 10.1016/j.neuron.2007.11.027
Xue, M., Atallah, B. V., and Scanziani, M. (2014). Equalizing excitation-inhibition ratios across visual cortical neurons. Nature 511, 596–600. doi: 10.1038/nature13321
Yoon, B. J., Smith, G. B., Heynen, A. J., Neve, R. L., and Bear, M. F. (2009). Essential role for a long-term depression mechanism in ocular dominance plasticity. Proc. Natl. Acad. Sci. U.S.A. 106, 9860–9865. doi: 10.1073/pnas.0901305106
Yu, H., Majewska, A. K., and Sur, M. (2011). Rapid experience-dependent plasticity of synapse function and structure in ferret visual cortex in vivo. Proc. Natl. Acad. Sci. U.S.A. 108, 21235–21240. doi: 10.1073/pnas.1108270109
Yuste, R. (2013). Electrical compartmentalization in dendritic spines. Annu. Rev. Neurosci. 36, 429–449. doi: 10.1146/annurev-neuro-062111-150455
Zhong, L., and Gerges, N. Z. (2012). Neurogranin targets calmodulin and lowers the threshold for the induction of long-term potentiation. PLoS One 7:e41275. doi: 10.1371/journal.pone.0041275
Zhou, Q., Homma, K. J., and Poo, M. M. (2004). Shrinkage of dendritic spines associated with long-term depression of hippocampal synapses. Neuron 44, 749–757. doi: 10.1016/j.neuron.2004.11.011
Zhou, Y., Lai, B., and Gan, W. B. (2017). Monocular deprivation induces dendritic spine elimination in the developing mouse visual cortex. Sci. Rep. 7:4977. doi: 10.1038/s41598-017-05337-6
Zipursky, S. L., and Sanes, J. R. (2010). Chemoaffinity revisited: dscams, protocadherins, and neural circuit assembly. Cell 143, 343–353. doi: 10.1016/j.cell.2010.10.009
Ziv, N. E., and Smith, S. J. (1996). Evidence for a role of dendritic filopodia in synaptogenesis and spine formation. Neuron 17, 91–102. doi: 10.1016/s0896-6273(00)80283-4
Keywords: environmental enrichment, dark exposure, spine elimination, monocular deprivation, unsilencing, refinement, innate synapse, gestalt synapse
Citation: Xu W, Löwel S and Schlüter OM (2020) Silent Synapse-Based Mechanisms of Critical Period Plasticity. Front. Cell. Neurosci. 14:213. doi: 10.3389/fncel.2020.00213
Received: 10 March 2020; Accepted: 17 June 2020;
Published: 17 July 2020.
Edited by:
Lang Wang, Zhejiang University, ChinaReviewed by:
Kathryn M. Murphy, McMaster University, CanadaCopyright © 2020 Xu, Löwel and Schlüter. This is an open-access article distributed under the terms of the Creative Commons Attribution License (CC BY). The use, distribution or reproduction in other forums is permitted, provided the original author(s) and the copyright owner(s) are credited and that the original publication in this journal is cited, in accordance with accepted academic practice. No use, distribution or reproduction is permitted which does not comply with these terms.
*Correspondence: Weifeng Xu, d2VpZmVuZ194dUBicm93bi5lZHU=; Siegrid Löwel, c2xvZXdlbEBnd2RnLmRl; Oliver M. Schlüter, c2NobHV0ZXJAcGl0dC5lZHU=
†These authors have contributed equally to this work
Disclaimer: All claims expressed in this article are solely those of the authors and do not necessarily represent those of their affiliated organizations, or those of the publisher, the editors and the reviewers. Any product that may be evaluated in this article or claim that may be made by its manufacturer is not guaranteed or endorsed by the publisher.
Research integrity at Frontiers
Learn more about the work of our research integrity team to safeguard the quality of each article we publish.