- 1UCL School of Pharmacy, University College London, London, United Kingdom
- 2Centre for Perinatal Medicine and Health, Institute of Clinical Sciences, Sahlgrenska Academy, University of Gothenburg, Gothenburg, Sweden
- 3Department of Comparative Biomedical Sciences, Royal Veterinary College, London, United Kingdom
The perinatal period represents a time of great vulnerability for the developing brain. A variety of injuries can result in death or devastating injury causing profound neurocognitive deficits. Hypoxic-ischemic neonatal encephalopathy (HIE) remains the leading cause of brain injury in term infants during the perinatal period with limited options available to aid in recovery. It can result in long-term devastating consequences with neurologic complications varying from mild behavioral deficits to severe seizure, intellectual disability, and/or cerebral palsy in the newborn. Despite medical advances, the only viable option is therapeutic hypothermia which is classified as the gold standard but is not used, or may not be as effective in preterm cases, infection-associated cases or low resource settings. Therefore, alternatives or adjunct therapies are urgently needed. Ongoing research continues to advance our understanding of the mechanisms contributing to perinatal brain injury and identify new targets and treatments. Drugs used for the treatment of patients with type 2 diabetes mellitus (T2DM) have demonstrated neuroprotective properties and therapeutic efficacy from neurological sequelae following HIE insults in preclinical models, both alone, or in combination with induced hypothermia. In this short review, we have focused on recent findings on the use of diabetes drugs that provide a neuroprotective effect using in vitro and in vivo models of HIE that could be considered for clinical translation as a promising treatment.
Introduction
Hypoxic-ischemic encephalopathy (HIE) is the most common neonatal encephalopathy accounting for up to 85% of cases (Volpe, 2012). It is caused by of an inadequate oxygen supply and blood flow resulting in a variety of clinical manifestations (Ferriero, 2004; Allen and Brandon, 2011; Hagberg et al., 2015). These include developmental delays, epilepsy, cerebral palsy, and death (Dilenge et al., 2001; Shankaran, 2012; Hagberg et al., 2016). One to six babies per 1,000 live births in high-income countries and approximately 20 infants per 1,000 live births in low- and middle-income countries die or develop a life-long brain condition. This accounts for approximately one million deaths annually (Lee et al., 2013; Pauliah et al., 2013; Wu et al., 2014).
Currently, the standard care for neonates with HIE is therapeutic hypothermia (TH), which is able to reduce overall neurodevelopmental disability and mortality (Jacobs et al., 2013; Azzopardi et al., 2014; Silveira and Procianoy, 2015; Rao et al., 2017). However, while TH is very promising, up to 55% of treated neonates are not protected and still develop life-long neurodisabilities, including cerebral palsy (Jacobs et al., 2013; Davidson et al., 2015). Therefore, there is a need to develop therapies that are either more effective than hypothermia, can be used in combination with hypothermia to enhance its therapeutic efficacy, or which can be used alone in lower resource environments.
Over the past decade, a growing number of pre-clinical and now clinical studies have provided evidence of drugs licensed for the treatment of diabetes as having protective effects on the brain (Athauda et al., 2017; Rotermund et al., 2018; Mousa and Ayoub, 2019). These effects have been proven in different neurological conditions such as Alzheimer’s disease (AD) and Parkinson’s Disease (PD), traumatic brain injury (TBI), stroke and epilepsy (Figure 1). Given the need to develop effective treatments for neonatal HIE, researchers have investigated these diabetes drugs to assess their therapeutic efficacy for this indication.
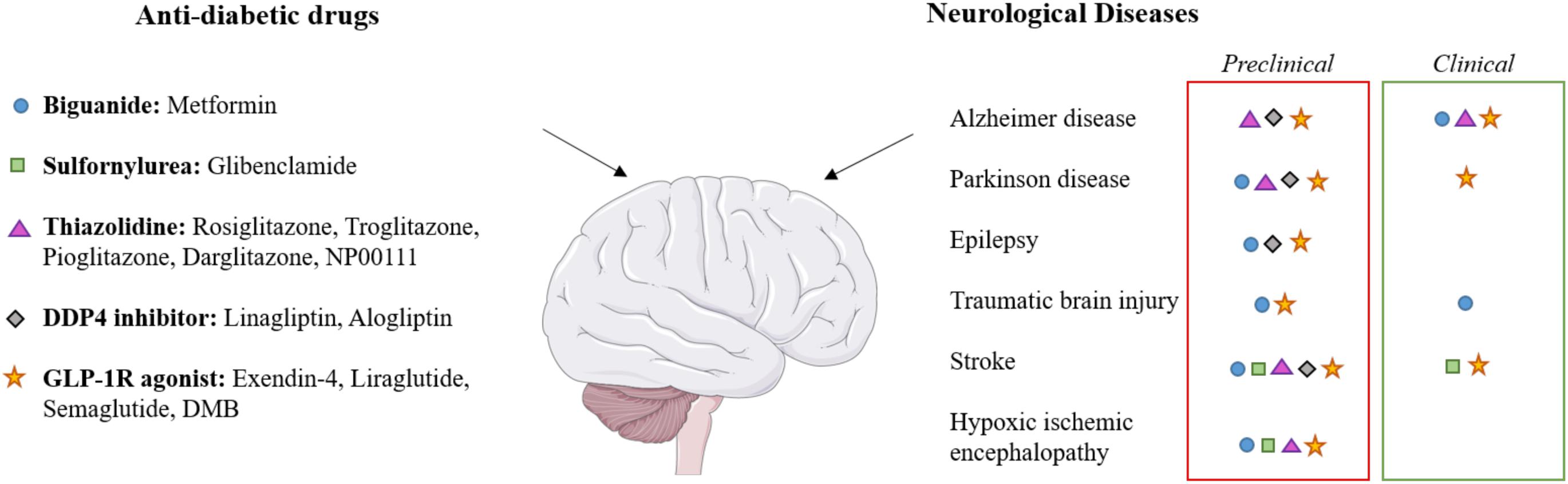
Figure 1. Effects of diabetes drugs on various neurological diseases. The applied color code indicates whether the effect on the target disease has been observed in preclinical studies (red boxes) or in clinical studies (green boxes). Each class of drugs corresponds to a symbol which is indicated in neurological diseases for which clinical and/or preclinical studies has been performed. Brain image taken from the SMART Servier Medical Art Library (https://smart.servier.com).
In this short review, we first describe the experimental and animal models of HIE that are used in preclinical studies to assess the therapeutic efficacy of candidate drugs (Table 1). We then highlight the studies that support the potential of commonly used diabetes medicines to ameliorate neurological damage from HIE. This includes recent data demonstrating that diabetes drugs can enhance the therapeutic effect of TH.
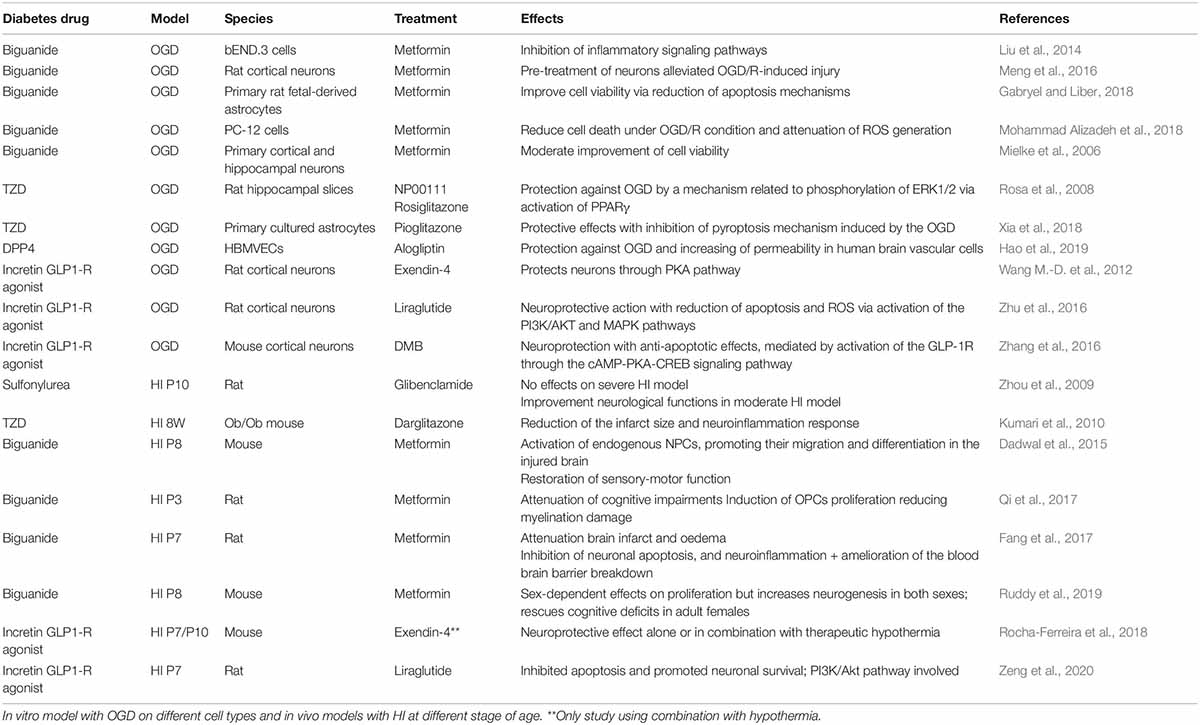
Table 1. Evidence supporting the neuroprotective properties of diabetes drugs use in the context of treatments for hypoxia-ischemia encephalopathy.
Experimental and Animal Models of HIE
Hypoxic-ischemic encephalopathy is an evolving process that involves distinct phases leading to a delayed cell death, including primary injury, latent phase, secondary phase, and tertiary phase (Wyatt et al., 1989; Fleiss and Gressens, 2012; Davidson et al., 2015). Understanding the characteristics observed during the different phases leading to neonatal encephalopathy are key to the development of new therapeutics, when they can be used to ameliorate HIE and the multiple possible subsequent sequela. The timing of the events following hypoxia-ischemia (HI) and the therapeutic window in rodent models is well defined at ∼6 h correlating with initiation of the secondary phase of brain injury (Nair and Kumar, 2018). Therefore, there is a narrow window within the first few hours of birth during which a therapy should be initiated for optimal outcomes (Silveira and Procianoy, 2015; Martinello et al., 2017). Furthermore, if the drug is administered systemically, then it should be able to reach the brain quickly and cross the blood brain barrier (BBB).
The neuroprotective properties of diabetes drugs were first recognized by positive neurological effects in type 2 diabetes mellitus (T2DM) patients under treatment (Grant et al., 2011) and now in various studies for the treatment of different neurological conditions (Hussien et al., 2018; Rotermund et al., 2018; Erbil et al., 2019). A number of studies have demonstrated that diabetes drugs are indeed capable of entering the brain following systemic administrations and mediating a physiological response, e.g., metformin (Lv et al., 2012), sulfonylurea (SUR) (Simard et al., 2012), thiazolidine (Grommes et al., 2013), dipeptidyl peptidase-4 (DPP-4) inhibitors (Mousa and Ayoub, 2019), and glucagon-like peptide-1 receptor (GLP1-R) agonists (Hunter and Hölscher, 2012).
Accurate and reliable in vitro and in vivo models of HIE are of utmost importance in determining the mechanisms of damage and also evaluating the efficacy of potential treatments. The development of a variety of in vitro and in vivo models of HIE have facilitated this process.
Oxygen Glucose Deprivation
Oxygen glucose deprivation is widely used as a relatively convenient in vitro model for ischemia, stroke or HIE, showing similarities with the in vivo models of brain ischemia (Tasca et al., 2015). This primary neural cell or immortalized cell culture model has been used extensively to examine the cellular mechanisms mediating ischemia–reperfusion injury (Rousset et al., 2015; Gao et al., 2019). The OGD model is a simple process that firstly involves changes to the cell culture medium to exclude glucose. The cells are incubated in a hypoxic incubator with decreased O2 and increased N2 levels with a saturated humidity atmosphere at 37°C over a specific period of time. Thus, cultured cells subjected to hypoxia, fuel deprivation and then reoxygenation mimic the scenario of ischemia–reperfusion.
Hypoxia-Ischemia Surgery
The rodent model of neonatal HIE was first validated by Rice et al. (1981) and has since been extensively used to identify mechanisms of brain injury resulting from perinatal HI (Vannucci and Vannucci, 2005). It is also used to test potential therapeutic interventions. The HIE model is a two-step process and involves the ligation of one common carotid artery followed by exposure to a hypoxic environment before restoration to normal atmospheric conditions. Traditional models of HIE have utilized rodents at postnatal day 7–10 as being roughly equivalent to a near-term or term human infant based on electrophysiological, neurochemical, cardiovascular, and metabolic criteria of brain development (Hagberg et al., 1997; Semple et al., 2013). There are a wide variety of HI animal models used to investigate different aspects of HIE. Examples of this include; rodents (Recker et al., 2009), rabbits (Derrick et al., 2004), term piglet (Rocha-Ferreira et al., 2016, 2017), preterm sheep (Nitsos et al., 2014), and non-human primates (Juul et al., 2007). To date, existing preclinical data using diabetes drugs as a treatment for HIE have only been performed on rodent models. Furthermore, rodents have limitations in simulating the range, accuracy, and physiology of clinical HI and the relevant systems neuropathology that contribute to the human brain injury pattern. Large animal models of perinatal HI can better replicate the conditions of human HIE (Koehler et al., 2018).Therefore, the availability of these larger animal models of HIE are an invaluable tool to evaluate the therapeutic efficacy of these candidate diabetes drugs prior to any clinical trials.
Diabetes Drugs and Neurological Diseases
Pharmacologic therapy of T2DM has changed dramatically in the last 10 years, with new drugs and drug classes becoming available. Among the different categories of therapies for T2DM, metformin serves as the first line drug whereas other hypoglycaemic agents (SUR, thiazolidine, DPP4 inhibitor, incretin) are used as second line therapies, or in combination with metformin. Over the past three decades, numerous epidemiological studies have shown a clear association between T2DM and an increased risk of developing neurological disorders (NDs) such as AD (Li et al., 2015), PD (Khan et al., 2014), epilepsy/seizure (Yun and Xuefeng, 2013), and stroke (Putaala et al., 2011).
Various studies suggest a comorbid association between NDs and T2DM indicating that there could be shared underlying pathophysiological mechanisms. Using comparative analysis, several putative “shared pathways” have been indentified and demonstrated how the insulin signaling pathway is related to other significant ND pathways. These include the signaling pathways for neurotrophin (Tong et al., 2009; Karki et al., 2017), PI3K/AKT (Gabbouj et al., 2019), mTOR (Bryan and Bowman, 2017; Sun et al., 2019), and mitogen-activated protein kinase (MAPK) (Santiago and Potashkin, 2013; Karki et al., 2017) and how these pathways cross-talk with each other. Consequently, studies started to investigate T2DM treatments as neuroprotective strategies for different types of ND, including perinatal HIE.
Metformin
Metformin is a biguanide drug widely used since the 1960s for the treatment of patients with T2DM. It enhances insulin sensitivity, induces glycolysis, and suppresses gluconeogenesis in the liver (Martin-Montalvo et al., 2013). Pre-clinical studies have also reported the promising therapeutic effect of metformin against neurodegeneration in conditions such as PD (Patil et al., 2014), epilepsy (Yimer et al., 2019), and cerebral ischaemia/reperfusion injury (Ge et al., 2017; Leech et al., 2019). In addition to its neuroprotective effects, metformin has been shown to promote neurogenesis by enhancing neural precursor self-renewal, proliferation, and differentiation (Potts and Lim, 2012; Wang J. et al., 2012). Increased neurogenesis upon metformin treatment resulted in improved memory formation in multiple experimental models of brain injury (Jin et al., 2014; Liu et al., 2014; Dadwal et al., 2015; Ge et al., 2017; Qi et al., 2017). In vitro studies using the OGD model demonstrated that metformin improves neuronal viability and regulates programmed cell death in a caspase-independent manner, thereby reducing ischemic reperfusion injuries (Mielke et al., 2006; Meng et al., 2016; Gabryel and Liber, 2018; Mohammad Alizadeh et al., 2018). Metformin treatment remarkably attenuated brain infarct volumes, brain oedema and restored behavior deficits in a neonatal rat model of HI (Qi et al., 2017). It also induced activation of endogenous neural precursor cells (NPCs) (Dadwal et al., 2015). A rodent model of neonatal HI injury showed better neuroprotection induced by metformin in females following early injury relative to males. Indeed, metformin treatment in mice increased the NPC pool in both sexes in neonates but only in females at the adult stage. Consequently, long-term metformin treatment leads to cognitive improvements in females, but not males following early HI injury (Ruddy et al., 2019). The mechanism could be linked to the sex hormones (Ruddy et al., 2019) but further exploration of the mechanism underlying this effect is required. Of note, females have an advantage following neonatal hypoxia ischemia; larger cognitive deficits and less functional recovery have been observed in males despite a comparable neuropathology across sexes (Smith et al., 2014). The neuroprotective properties of metformin were associated with inhibition of neuronal apoptosis, suppression of neuroinflammation and amelioration of the blood-brain barrier breakdown via downregulation of the NFκB signaling pathway (Fang et al., 2017). Overall, these studies have highlighted this drug as a promising potential treatment in childhood brain injury models.
Sulfonylurea
Sulfonylurea agents are the second oral hypoglycaemic drugs after metformin and they remain an imperative tool for glucose control (Thulé and Umpierrez, 2014). Recent studies demonstrated that sulfonylurea receptor 1 (SUR1) is involved in brain injury in rodent models of stroke (Hussien et al., 2018). The SUR drugs glibenclamide and glimepiride have neuroprotective effects (Ortega et al., 2013; Wang et al., 2019) and ameliorate cerebral stroke, spinal cord injury, premature encephalopathy, and TBI (Tosun et al., 2013). The neuroprotective effect of SUR agents is not fully understood but glibenclamide blocks SUR1, a regulatory subunit of the microglial KATP channel. This channel is overexpressed in rodent models of stroke and the effect of blocking SUR1 could inhibit microglia activation which release inflammatory cytokines and initiate downstream signaling pathways, resulting in neuronal cell loss and necrosis (Ortega et al., 2013). Glibenclamide is clinically effective in preventing oedema and improving outcome after focal ischemia (Sheth et al., 2014); clinical studies are being conducted to evaluate its efficacy in acute cerebral embolism and severe cerebral edema (NCT03284463, NCT02864953). In a rat model of HI injury, glibenclamide improved several neurological parameters but failed to attenuate brain edema, infarct volume or brain tissue loss (Zhou et al., 2009). This may be attributed to the significant reduction in blood glucose induced by the dose of glibenclamide used, which may exacerbate the ischemic brain injury. More investigations need to be performed around the dose and its full potential as a treatment for childhood brain injury models.
Thiazolidine
Thiazolidinedione [also called glitazone or peroxisome proliferator activated receptor-γ (PPARγ) agonists] are a group of oral anti-diabetic drugs designed to treat patients with T2DM. They enhance insulin sensitivity and reduce serum glucose in diabetic patients, without significant alterations in serum glucose of non-diabetic animals or humans (Plutzky, 2003). Rosiglitazone, troglitazone, and pioglitazone suppressed the activation and infiltration of macrophages and reduced the infarct size after cerebral ischemia in a middle cerebral artery occlusion (MCAO) model by reducing levels of proinflammatory cytokines (Sundararajan et al., 2005; Culman et al., 2007; Xia et al., 2018). Moreover, PPARγ agonists such as NP00111, rosiglitazone and pioglitazone treatments could relieve OGD-induced hypoxia injury in vitro and exert neuroprotective effects (Rosa et al., 2008; Xia et al., 2018). The neuroprotective effect of TZD requires further investigation. However, data suggests activation of PPARγ mediates suppression of NF-κB signaling pathway, inhibiting apoptosis and reducing neuronal loss (Zhang et al., 2011). In the context of adult HI injury, TZD has shown therapeutic efficacy in Ob/Ob mice, a model for T2DM and obesity. This model was chosen for its high risk factor of stroke and increased risk of brain damage. Darglitazone treatment in this adult diabetic mouse resulted in significant neuroprotection associated with a complete restoration of the initial microglial response and reduction of the infarct brain size at 24 h of recovery (Kumari et al., 2010). No studies have yet been conducted in a neonatal HI model but the proven neuroprotective properties and potent anti-ischemic effects of this class of diabetes drug could be a promising option.
Incretin / GLP1-Receptor Agonists
Glucagon-like peptide-1-receptor agonists are used in combination with diet and exercise in the therapy of T2DM, either alone or in combination with other antidiabetic agents. GLP1-R agonists have been found to enter the brain following systemic administration (Hunter and Hölscher, 2012; Athauda et al., 2017) and have neuroprotective properties when assessed in various rodent models of neurological disease and damage such as AD (Xu et al., 2015; Cai et al., 2018), PD (Yun et al., 2018), epilepsy (Wen et al., 2019), TBI (Glotfelty et al., 2019), and stroke. As a result, a number of clinical trials are already underway for some of these molecules such as exendin-4 (NCT03456687, NCT02829502, NCT03287076), liraglutide (NCT02953665, NCT01469351, NCT01843075, NCT03948347) or semaglutide (NCT03659682) to assess benefits to AD, PD, or stroke patients. A PD trial is already completed and has reported that patients on exendin-4 show a statistically significant improvement in clinical motor and cognitive measures compared to the control group (Athauda et al., 2017). Numerous experimental studies also demonstrated the potential of glucagon-like peptide-1 (GLP1) and analog, such as liraglutide or semaglutide, to reduce acute ischaemic damage in the brain (Wang M.-D. et al., 2012; Zhu et al., 2016; Basalay et al., 2019; Yang et al., 2019). Exendin-4, liraglutide and quinoxaline 6,7-dichloro-2-methylsulfonyl-3-N-tert-butylaminoquinoxaline (DMB, an agonist and allosteric modulator of the GLP-1R) have been shown to increase neuron survival under OGD in vitro by reducing reactive oxygen species (ROS), apoptotic and necrotic mechanisms (Wang M.-D. et al., 2012; Zhang et al., 2016; Zhu et al., 2016). The potential that GLP1-R agonists have for treating perinatal HIE has been further strengthened in two recent studies demonstrating: (1) that exendin-4 has significant therapeutic efficacy in the mouse model of neonatal HIE (Rocha-Ferreira et al., 2018), (2) and that liraglutide exerts neuroprotection via the PI3k/Akt pathway (Zeng et al., 2020). The study by Rocha-Ferreira and colleagues demonstrated that systemic administration either directly after HI injury, or even 2 h later significantly reduced the size of the brain infarct, the inflammatory response and the oxidative stress. Exendin-4 treatment was able to work synergistically with hypothermia to further enhance therapeutic efficacy (Rocha-Ferreira et al., 2018).
DPP-4 Inhibitor
Also called gliptins, DPP-4 inhibitors are a class of glucose-lowering agents for the treatment of T2DM. Their actions are mediated indirectly through preservation of GLP-1 incretins that are mainly metabolized by the key enzyme DPP-4 (Andersen et al., 2018). Preclinical studies have shown that DPP-4 inhibitors have neuroprotective effects (Darsalia et al., 2019; Mousa and Ayoub, 2019) but unlike GLP-1 receptor agonists (incretins), the ability of DPP-4 inhibitors to cross the blood-brain barrier are still unclear (Chen et al., 2015). However, they could indirectly increase levels of active GLP-1 in the brain that crosses from the blood (Yang et al., 2013). Several kinds of gliptins have been shown to be effective in different experimental models of neurological diseases such as AD (Wiciński et al., 2018; Dong et al., 2019), PD, epilepsy and stroke (Darsalia et al., 2019; Mousa and Ayoub, 2019). In the MCAO model mimicking stroke, linagliptin and alogliptin reduced infarct volume and neurological deficits (Chiazza et al., 2018; Hao et al., 2019). In the same study, alogliptin protectsed against oxygen glucose deprivation reperfusion (OGD/R) and has a neurovascular protective effect increasing permeability in human brain vascular cells (Hao et al., 2019). No data exists in an experimental neonatal HIE model but DPP-4 enzyme activity is known to increase in the blood serum of term and preterm neonates with cerebral ischemia (Yakovleva et al., 2015). Because DPP-4 inhibitors have shown neuroprotective properties and increase levels of GLP-1 in the brain, this could suggest that they have potential for treating HIE.
Summary
The neuroprotective properties of diabetes drugs were first recognized by improvements in the neuropathic aspects in T2DM patients under treatment (Grant et al., 2011). The role of insulin as a pro-survival neurotrophic factor, where its receptor is widely expressed in cognitive areas of the brain such as the hippocampus and in the dopaminergic system also helped to consolidate this hypothesis (Haas et al., 2016; Fiory et al., 2019). The emerging evidence has suggested a beneficial effect of diabetes drugs in the management of diabetic and non-diabetic NDs. Furthermore, data supporting their neuroprotective effects are supported by a growing number of preclinical studies in neurodegenerative disorders such as AD (Cai et al., 2018; Dong et al., 2019) and PD (Athauda and Foltynie, 2016; Ayoub et al., 2018). Importantly, therapeutic efficacy had also been demonstrated in a clinical trial in PD patients (Athauda et al., 2017). However, many developmental, functional, and injury time course differences exist between the neonatal and the adult brain (Ferriero, 2004). Drug delivery properties, dosage and use can also be complex to translate from a adult to neonatal setting.
Several proof-of-concept studies with different classes of diabetes drugs as a treatment in neonatal HIE have been identified with glibenclamide (Zhou et al., 2009), metformin (Dadwal et al., 2015; Fang et al., 2017; Qi et al., 2017) and exendin-4 (Rocha-Ferreira et al., 2018). These diabetes drugs act on a plethora of biological pathways (Rosa et al., 2008; Wang M.-D. et al., 2012; Zhang et al., 2016; Zhu et al., 2016) and the precise mechanisms of action of diabetes drugs for neuroprotection are still not fully understood. However, in the context of HIE several studies have demonstrated neuroprotective actions of the GLP1-R agonists (Kimura et al., 2009; Jiang et al., 2016; Zhao et al., 2018) and metformin (Khallaghi et al., 2016) through the PI3K/Akt signaling pathway. In cases of brain injury, diabetes drugs have shown to be able to help repair the brain by modulating cell death mechanisms (Mielke et al., 2006; Gabryel and Liber, 2018; Xia et al., 2018), reducing neuronal oxidative stress (Mohammad Alizadeh et al., 2018) and promoting growth of new neurons and cells (Dadwal et al., 2015; Qi et al., 2017).
In adults, diabetes drugs are generally well tolerated with a long track record in the clinic and demonstrable safety profiles. However, this requires to be established in new born babies. Therefore, the normal advantages of rapidly repurposing drugs at a lower cost than the drug development process (Ayoub et al., 2018; Mousa and Ayoub, 2019) may be diminished for application to HIE. Potential safety risks could be reduced since the administration of the drug would likely only be required during a short acute period following the HI insult over a 48 h period (Rocha-Ferreira et al., 2018). However, depending on the diabetes drug in question, an important consideration is the potential to induce a hypoglycaemic effects when perturbations in glucose metabolism (hypoglycaemia and hyperglycemia) are already common in newborn infants with HIE (Vannucci, 1992; Salhab et al., 2004; Basu et al., 2009). This issue of hypoglycaemia exacerbating brain injury has been highlighted in the previously mentioned pre-clinical study using glibenclamide (Zhou et al., 2009). Therefore, the evaluation of the safety of diabetes drugs must be conducted in preclinical neonatal models, including larger animals prior to clinical trials.
Conclusion
In conclusion, there is a growing body of evidence supporting the neuroprotective and anti-neuroinflammatory properties of specific diabetes drugs. Furthermore, the emerging proof of concept studies supporting their potential use as a treatment for HIE, either independently or in combination with hypothermia, is highly encouraging and warrants further investigation.
Author Contributions
LP-B, ER-F, CT, HH, and AR drafted the manuscript.
Funding
LP-B, ER-F, CT, HH, and AR are funded by Action Medical Research (GN2485). AR is also funded by UK Medical Research Council Grants (MR/R025134/1, MR/R015325/1, MR/S009434/1, MR/N026101/1, and MR/S036784/1) and the Wellcome Trust Institutional Strategic Support Fund/UCL Therapeutic Acceleration Support (TAS) Fund (204841/Z/16/Z). ER-F is funded by the Hasselblad Foundation (2020–2021) and the Åke Wibergs Foundation (M19-0660). HH is funded by governmental grants to Swedish University Hospital (ALF-GBG 432291), ERA-NET (Contract: 0755101), Swedish Medical Research Council (2019-01320), Brain Foundation (2015-0004), and EU (contract: 874721 Horizon 2020). CT is funded by UK Medical Research Council New Investigator Research Grant (MR/T014725/1), and by Action Medical Research (GN2796).
Conflict of Interest
The authors declare that the research was conducted in the absence of any commercial or financial relationships that could be construed as a potential conflict of interest.
Abbreviations
AD, Alzheimer’s disease; BBB, blood brain barrier; DPP-4, dipeptidyl peptidase-4; DMB, quinoxaline 6,7-dichloro-2-methylsulfonyl-3-N-tert-butylaminoquinoxaline; GLP1, glucagon-like peptide-1; GLP1-R, glucagon like peptide-1 receptor; HI, hypoxia-ischemia; HIE, hypoxic-ischemic encephalopathy; HBMVECs, primary human brain microvascular endothelial cells; MAPK, mitogen-activated protein kinase; MCAO, middle cerebral artery occlusion; ND, neurological disorder; NPCs, neural precursor cells; OGD, oxygen glucose deprivation; OGD/R, oxygen glucose deprivation reperfusion; OPCs, oligodendrocyte progenitor cells; PD, Parkinson’s disease; PPAR- γ, peroxisome proliferator activated receptor- γ; ROS, reactive oxygen species; SUR, sulfonylurea; T2DM, type 2 diabetes mellitus; TH, therapeutic hypothermia; TBI, traumatic brain injury; TZD, thiazolidinedione
References
Allen, K. A., and Brandon, D. H. (2011). Hypoxic ischemic encephalopathy: pathophysiology and experimental treatments. Newborn Infant Nurs. Rev. 11, 125–133. doi: 10.1053/j.nainr.2011.07.004
Andersen, E. S., Deacon, C. F., and Holst, J. J. (2018). Do we know the true mechanism of action of the DPP-4 inhibitors? Diab. Obes. Metab. 20, 34–41. doi: 10.1111/dom.13018
Athauda, D., and Foltynie, T. (2016). The glucagon-like peptide 1 (GLP) receptor as a therapeutic target in Parkinson’s disease: mechanisms of action. Drug Discov. Today 21, 802–818. doi: 10.1016/j.drudis.2016.01.013
Athauda, D., Maclagan, K., Skene, S. S., Bajwa-Joseph, M., Letchford, D., Chowdhury, K., et al. (2017). Exenatide once weekly versus placebo in Parkinson’s disease: a randomised, double-blind, placebo-controlled trial. Lancet 390, 1664–1675. doi: 10.1016/S0140-6736(17)31585-4
Ayoub, B. M., Mowaka, S., Safar, M. M., Ashoush, N., Arafa, M. G., Michel, H. E., et al. (2018). Repositioning of omarigliptin as a once-weekly intranasal Anti-parkinsonian agent. Sci. Rep. 8:8959. doi: 10.1038/s41598-018-27395-0
Azzopardi, D., Strohm, B., Marlow, N., Brocklehurst, P., Deierl, A., Eddama, O., et al. (2014). Effects of hypothermia for perinatal asphyxia on childhood outcomes. N. Engl. J. Med. 371, 140–149. doi: 10.1056/NEJMoa1315788
Basalay, M. V., Davidson, S. M., and Yellon, D. M. (2019). Neuroprotection in rats following ischaemia-reperfusion injury by GLP-1 analogues-liraglutide and semaglutide. Cardiovasc. Drugs Ther. 33, 661–667. doi: 10.1007/s10557-019-06915-8
Basu, P., Som, S., Choudhuri, N., and Das, H. (2009). Contribution of the blood glucose level in perinatal asphyxia. Eur. J. Pediatr. 168, 833–838. doi: 10.1007/s00431-008-0844-5
Bryan, M. R., and Bowman, A. B. (2017). Manganese and the insulin-IGF signaling network in Huntington’s disease and other neurodegenerative disorders. Adv. Neurobiol. 18, 113–142. doi: 10.1007/978-3-319-60189-2_6
Cai, H.-Y., Yang, J.-T., Wang, Z.-J., Zhang, J., Yang, W., Wu, M.-N., et al. (2018). Lixisenatide reduces amyloid plaques, neurofibrillary tangles and neuroinflammation in an APP/PS1/tau mouse model of Alzheimer’s disease. Biochem. Biophys. Res. Commun. 495, 1034–1040. doi: 10.1016/j.bbrc.2017.11.114
Chen, D.-Y., Wang, S.-H., Mao, C.-T., Tsai, M.-L., Lin, Y.-S., Su, F.-C., et al. (2015). Sitagliptin after ischemic stroke in type 2 diabetic patients: a nationwide cohort study. Medicine (Baltimore) 94, e1128. doi: 10.1097/MD.0000000000001128
Chiazza, F., Tammen, H., Pintana, H., Lietzau, G., Collino, M., Nyström, T., et al. (2018). The effect of DPP-4 inhibition to improve functional outcome after stroke is mediated by the SDF-1α/CXCR4 pathway. Cardiovasc. Diabetol. 17:60. doi: 10.1186/s12933-018-0702-3
Culman, J., Zhao, Y., Gohlke, P., and Herdegen, T. (2007). PPAR-gamma: therapeutic target for ischemic stroke. Trends Pharmacol. Sci. 28, 244–249. doi: 10.1016/j.tips.2007.03.004
Dadwal, P., Mahmud, N., Sinai, L., Azimi, A., Fatt, M., Wondisford, F. E., et al. (2015). Activating endogenous neural precursor cells using metformin leads to neural repair and functional recovery in a model of childhood brain injury. Stem Cell Rep. 5, 166–173. doi: 10.1016/j.stemcr.2015.06.011
Darsalia, V., Johansen, O. E., Lietzau, G., Nyström, T., Klein, T., and Patrone, C. (2019). Dipeptidyl peptidase-4 inhibitors for the potential treatment of brain disorders; a mini-review with special focus on linagliptin and stroke. Front. Neurol. 10:493. doi: 10.3389/fneur.2019.00493
Davidson, J. O., Wassink, G., van den Heuij, L. G., Bennet, L., and Gunn, A. J. (2015). Therapeutic hypothermia for neonatal hypoxic–ischemic encephalopathy–where to from here? Front. Neurol. 6:198. doi: 10.3389/fneur.2015.00198
Derrick, M., Luo, N. L., Bregman, J. C., Jilling, T., Ji, X., Fisher, K., et al. (2004). Preterm fetal hypoxia-ischemia causes hypertonia and motor deficits in the neonatal rabbit: a model for human cerebral palsy? J. Neurosci. 24, 24–34. doi: 10.1523/JNEUROSCI.2816-03.2004
Dilenge, M. E., Majnemer, A., and Shevell, M. I. (2001). Long-term developmental outcome of asphyxiated term neonates. J. Child Neurol. 16, 781–792. doi: 10.1177/08830738010160110201
Dong, Q., Teng, S.-W., Wang, Y., Qin, F., Li, Y., Ai, L.-L., et al. (2019). Sitagliptin protects the cognition function of the Alzheimer’s disease mice through activating glucagon-like peptide-1 and BDNF-TrkB signalings. Neurosci. Lett. 696, 184–190. doi: 10.1016/j.neulet.2018.12.041
Erbil, D., Eren, C. Y., Demirel, C., Küçüker, M. U., Solaroğlu, I., and Eser, H. Y. (2019). GLP-1’s role in neuroprotection: a systematic review. Brain Inj. 33, 734–819. doi: 10.1080/02699052.2019.1587000
Fang, M., Jiang, H., Ye, L., Cai, C., Hu, Y., Pan, S., et al. (2017). Metformin treatment after the hypoxia-ischemia attenuates brain injury in newborn rats. Oncotarget 8, 75308–75325. doi: 10.18632/oncotarget.20779
Ferriero, D. M. (2004). Neonatal brain injury. N. Engl. J. Med. 351, 1985–1995. doi: 10.1056/NEJMra041996
Fiory, F., Perruolo, G., Cimmino, I., Cabaro, S., Pignalosa, F. C., Miele, C., et al. (2019). The relevance of insulin action in the dopaminergic system. Front. Neurosci. 13:868. doi: 10.3389/fnins.2019.00868
Fleiss, B., and Gressens, P. (2012). Tertiary mechanisms of brain damage: a new hope for treatment of cerebral palsy? Lancet Neurol. 11, 556–566. doi: 10.1016/S1474-4422(12)70058-3
Gabbouj, S., Ryhänen, S., Marttinen, M., Wittrahm, R., Takalo, M., Kemppainen, S., et al. (2019). Altered insulin signaling in Alzheimer’s disease brain – special emphasis on PI3K-Akt pathway. Front. Neurosci. 13:629. doi: 10.3389/fnins.2019.00629
Gabryel, B., and Liber, S. (2018). Metformin limits apoptosis in primary rat cortical astrocytes subjected to oxygen and glucose deprivation. Folia Neuropathol. 56, 328–336. doi: 10.5114/fn.2018.80866
Gao, Y., Wang, Z., He, W., Ma, W., and Ni, X. (2019). Mild hypothermia protects neurons against oxygen glucose deprivation via poly (ADP-ribose) signaling. J. Matern. Fetal Neonatal Med. 32, 1633–1639. doi: 10.1080/14767058.2017.1413548
Ge, X.-H., Zhu, G.-J., Geng, D.-Q., Zhang, H.-Z., He, J.-M., Guo, A.-Z., et al. (2017). Metformin protects the brain against ischemia/reperfusion injury through PI3K/Akt1/JNK3 signaling pathways in rats. Physiol. Behav. 170, 115–123. doi: 10.1016/j.physbeh.2016.12.021
Glotfelty, E. J., Delgado, T., Tovar-Y-Romo, L. B., Luo, Y., Hoffer, B., Olson, L., et al. (2019). Incretin mimetics as rational candidates for the treatment of traumatic brain injury. ACS Pharmacol. Transl. Sci. 2, 66–91. doi: 10.1021/acsptsci.9b00003
Grant, P., Lipscomb, D., and Quin, J. (2011). Psychological and quality of life changes in patients using GLP-1 analogues. J. Diab. Complicat. 25, 244–246. doi: 10.1016/j.jdiacomp.2011.03.002
Grommes, C., Karlo, J. C., Caprariello, A., Blankenship, D., Dechant, A., and Landreth, G. E. (2013). The PPARγ agonist pioglitazone crosses the blood-brain barrier and reduces tumor growth in a human xenograft model. Cancer Chemother. Pharmacol. 71, 929–936. doi: 10.1007/s00280-013-2084-2
Haas, C. B., Kalinine, E., Zimmer, E. R., Hansel, G., Brochier, A. W., Oses, J. P., et al. (2016). Brain insulin administration triggers distinct cognitive and neurotrophic responses in young and aged rats. Mol. Neurobiol. 53, 5807–5817. doi: 10.1007/s12035-015-9494-6
Hagberg, H., Bona, E., Gilland, E., and Puka-Sundvall, M. (1997). Hypoxia-ischaemia model in the 7-day-old rat: possibilities and shortcomings. Acta Paediatr. Suppl. 86, 85–88.
Hagberg, H., David Edwards, A., and Groenendaal, F. (2016). Perinatal brain damage: the term infant. Neurobiol. Dis. 92, 102–112. doi: 10.1016/j.nbd.2015.09.011
Hagberg, H., Mallard, C., Ferriero, D. M., Vannucci, S. J., Levison, S. W., Vexler, Z. S., et al. (2015). The role of inflammation in perinatal brain injury. Nat. Rev. Neurol. 11, 192–208. doi: 10.1038/nrneurol.2015.13
Hao, F., Han, X., Wang, X., Zhao, Z., Guo, A., Lu, X., et al. (2019). The neurovascular protective effect of alogliptin in murine MCAO model and brain endothelial cells. Biomed. Pharmacother. 109, 181–187. doi: 10.1016/j.biopha.2018.10.064
Hunter, K., and Hölscher, C. (2012). Drugs developed to treat diabetes, liraglutide and lixisenatide, cross the blood brain barrier and enhance neurogenesis. BMC Neurosci. 13:33. doi: 10.1186/1471-2202-13-33
Hussien, N. R., Al-Naimi, M. S., Rasheed, H. A., Al-Kuraishy, H. M., and Al-Gareeb, A. I. (2018). Sulfonylurea and neuroprotection: the bright side of the moon. J. Adv. Pharm. Technol. Res. 9, 120–123. doi: 10.4103/japtr.JAPTR_317_18
Jacobs, S. E., Berg, M., Hunt, R., Tarnow-Mordi, W. O., Inder, T. E., and Davis, P. G. (2013). Cooling for newborns with hypoxic ischaemic encephalopathy. Cochrane Database Syst. Rev. 2013:CD003311. doi: 10.1002/14651858
Jiang, Y.-Q., Chang, G.-L., Wang, Y., Zhang, D.-Y., Cao, L., and Liu, J. (2016). Geniposide prevents hypoxia/reoxygenation-induced apoptosis in H9c2 cells: improvement of mitochondrial dysfunction and activation of GLP-1R and the PI3K/AKT signaling pathway. Cell. Physiol. Biochem. 39, 407–421. doi: 10.1159/000445634
Jin, Q., Cheng, J., Liu, Y., Wu, J., Wang, X., Wei, S., et al. (2014). Improvement of functional recovery by chronic metformin treatment is associated with enhanced alternative activation of microglia/macrophages and increased angiogenesis and neurogenesis following experimental stroke. Brain Behav. Immun. 40, 131–142. doi: 10.1016/j.bbi.2014.03.003
Juul, S. E., Aylward, E., Richards, T., McPherson, R. J., Kuratani, J., and Burbacher, T. M. (2007). Prenatal cord clamping in newborn Macaca nemestrina: a model of perinatal asphyxia. Dev. Neurosci. 29, 311–320. doi: 10.1159/000105472
Karki, R., Kodamullil, A. T., and Hofmann-Apitius, M. (2017). Comorbidity analysis between Alzheimer’s disease and type 2 diabetes mellitus (T2DM) based on shared pathways and the role of T2DM drugs. J. Alzheimers Dis. 60, 721–731. doi: 10.3233/JAD-170440
Khallaghi, B., Safarian, F., Nasoohi, S., Ahmadiani, A., and Dargahi, L. (2016). Metformin-induced protection against oxidative stress is associated with AKT/mTOR restoration in PC12 cells. Life Sci. 148, 286–292. doi: 10.1016/j.lfs.2016.02.024
Khan, N. M., Ahmad, A., Tiwari, R. K., Kamal, M. A., Mushtaq, G., and Ashraf, G. M. (2014). Current challenges to overcome in the management of type 2 diabetes mellitus and associated neurological disorders. CNS Neurol. Disord. Drug Targets 13, 1440–1457. doi: 10.2174/1871527313666141023160448
Kimura, R., Okouchi, M., Fujioka, H., Ichiyanagi, A., Ryuge, F., Mizuno, T., et al. (2009). Glucagon-like peptide-1 (GLP-1) protects against methylglyoxal-induced PC12 cell apoptosis through the PI3K/Akt/mTOR/GCLc/redox signaling pathway. Neuroscience 162, 1212–1219. doi: 10.1016/j.neuroscience.2009.05.025
Koehler, R. C., Yang, Z.-J., Lee, J. K., and Martin, L. J. (2018). Perinatal hypoxic-ischemic brain injury in large animal models: relevance to human neonatal encephalopathy. J. Cereb. Blood Flow Metab. 38, 2092–2111. doi: 10.1177/0271678X18797328
Kumari, R., Willing, L. B., Patel, S. D., Krady, J. K., Zavadoski, W. J., Gibbs, E. M., et al. (2010). The PPAR-γ agonist, darglitazone, restores acute inflammatory responses to cerebral hypoxia–ischemia in the diabetic ob/ob mouse. J. Cereb. Blood Flow Metab. 30, 352–360. doi: 10.1038/jcbfm.2009.221
Lee, A. C. C., Kozuki, N., Blencowe, H., Vos, T., Bahalim, A., Darmstadt, G. L., et al. (2013). Intrapartum-related neonatal encephalopathy incidence and impairment at regional and global levels for 2010 with trends from 1990. Pediatr. Res. 74(Suppl. 1), 50–72. doi: 10.1038/pr.2013.206
Leech, T., Chattipakorn, N., and Chattipakorn, S. C. (2019). The beneficial roles of metformin on the brain with cerebral ischaemia/reperfusion injury. Pharmacol. Res. 146:104261. doi: 10.1016/j.phrs.2019.104261
Li, X., Song, D., and Leng, S. X. (2015). Link between type 2 diabetes and Alzheimer’s disease: from epidemiology to mechanism and treatment. Clin. Interv. Aging 10, 549–560. doi: 10.2147/CIA.S74042
Liu, Y., Tang, G., Li, Y., Wang, Y., Chen, X., Gu, X., et al. (2014). Metformin attenuates blood-brain barrier disruption in mice following middle cerebral artery occlusion. J. Neuroinflamm. 11:177. doi: 10.1186/s12974-014-0177-4
Lv, W.-S., Wen, J.-P., Li, L., Sun, R.-X., Wang, J., Xian, Y.-X., et al. (2012). The effect of metformin on food intake and its potential role in hypothalamic regulation in obese diabetic rats. Brain Res. 1444, 11–19. doi: 10.1016/j.brainres.2012.01.028
Martinello, K., Hart, A. R., Yap, S., Mitra, S., and Robertson, N. J. (2017). Management and investigation of neonatal encephalopathy: 2017 update. Arch. Dis. Child. Fetal Neonatal Ed. 102, F346–F358. doi: 10.1136/archdischild-2015-309639
Martin-Montalvo, A., Mercken, E. M., Mitchell, S. J., Palacios, H. H., Mote, P. L., Scheibye-Knudsen, M., et al. (2013). Metformin improves healthspan and lifespan in mice. Nat. Commun. 4:2192. doi: 10.1038/ncomms3192
Meng, X., Chu, G., Yang, Z., Qiu, P., Hu, Y., Chen, X., et al. (2016). Metformin protects neurons against oxygen-glucose deprivation/reoxygenation -induced injury by down-regulating MAD2B. Cell. Physiol. Biochem. 40, 477–485. doi: 10.1159/000452562
Mielke, J. G., Taghibiglou, C., and Wang, Y. T. (2006). Endogenous insulin signaling protects cultured neurons from oxygen–glucose deprivation-induced cell death. Neuroscience 143, 165–173. doi: 10.1016/j.neuroscience.2006.07.055
Mohammad Alizadeh, E., Mahdavi, M., Jenani Fard, F., Chamani, S., Farajdokht, F., and Karimi, P. (2018). Metformin protects PC12 cells against oxygen-glucose deprivation/reperfusion injury. Toxicol. Mech. Methods 28, 622–629. doi: 10.1080/15376516.2018.1486495
Mousa, S. A., and Ayoub, B. M. (2019). Repositioning of dipeptidyl peptidase-4 inhibitors and glucagon like peptide-1 agonists as potential neuroprotective agents. Neural Regen. Res. 14, 745–748. doi: 10.4103/1673-5374.249217
Nair, J., and Kumar, V. H. S. (2018). Current and emerging therapies in the management of hypoxic ischemic encephalopathy in neonates. Children (Basel) 5:E99. doi: 10.3390/children5070099
Nitsos, I., Newnham, J. P., Rees, S. M., Harding, R., and Moss, T. J. M. (2014). The impact of chronic intrauterine inflammation on the physiologic and neurodevelopmental consequences of intermittent umbilical cord occlusion in fetal sheep. Reprod. Sci. 21, 658–670. doi: 10.1177/1933719111399928
Ortega, F. J., Jolkkonen, J., Mahy, N., and Rodríguez, M. J. (2013). Glibenclamide enhances neurogenesis and improves long-term functional recovery after transient focal cerebral ischemia. J. Cereb. Blood Flow Metab. 33, 356–364. doi: 10.1038/jcbfm.2012.166
Patil, S. P., Jain, P. D., Ghumatkar, P. J., Tambe, R., and Sathaye, S. (2014). Neuroprotective effect of metformin in MPTP-induced Parkinson’s disease in mice. Neuroscience 277, 747–754. doi: 10.1016/j.neuroscience.2014.07.046
Pauliah, S. S., Shankaran, S., Wade, A., Cady, E. B., and Thayyil, S. (2013). Therapeutic hypothermia for neonatal encephalopathy in low- and middle-income countries: a systematic review and meta-analysis. PLoS One 8:e58834. doi: 10.1371/journal.pone.0058834
Plutzky, J. (2003). The potential role of peroxisome proliferator–activated receptors on inflammation in type 2 diabetes mellitus and atherosclerosis. Am. J. Cardiol. 92, 34–41. doi: 10.1016/S0002-9149(03)00614-3
Potts, M. B., and Lim, D. A. (2012). An old drug for new ideas: metformin promotes adult neurogenesis and spatial memory formation. Cell Stem Cell 11, 5–6. doi: 10.1016/j.stem.2012.06.003
Putaala, J., Liebkind, R., Gordin, D., Thorn, L. M., Haapaniemi, E., Forsblom, C., et al. (2011). Diabetes mellitus and ischemic stroke in the young: clinical features and long-term prognosis. Neurology 76, 1831–1837. doi: 10.1212/WNL.0b013e31821cccc2
Qi, B., Hu, L., Zhu, L., Shang, L., Sheng, L., Wang, X., et al. (2017). Metformin attenuates cognitive impairments in hypoxia–ischemia neonatal rats via improving remyelination. Cell Mol. Neurobiol. 37, 1269–1278. doi: 10.1007/s10571-016-0459-8
Rao, R., Trivedi, S., Vesoulis, Z., Liao, S. M., Smyser, C. D., and Mathur, A. M. (2017). Safety and short-term outcomes of therapeutic hypothermia in preterm neonates 34-35 weeks gestational age with hypoxic-ischemic encephalopathy. J. Pediatr. 183, 37–42. doi: 10.1016/j.jpeds.2016.11.019
Recker, R., Adami, A., Tone, B., Tian, H. R., Lalas, S., Hartman, R. E., et al. (2009). Rodent neonatal bilateral carotid artery occlusion with hypoxia mimics human hypoxic-ischemic injury. J. Cereb. Blood Flow Metab. 29, 1305–1316. doi: 10.1038/jcbfm.2009.56
Rice, J. E., Vannucci, R. C., and Brierley, J. B. (1981). The influence of immaturity on hypoxic-ischemic brain damage in the rat. Ann. Neurol. 9, 131–141. doi: 10.1002/ana.410090206
Rocha-Ferreira, E., Kelen, D., Faulkner, S., Broad, K. D., Chandrasekaran, M., and Kerenyi, Á, et al. (2017). Systemic pro-inflammatory cytokine status following therapeutic hypothermia in a piglet hypoxia-ischemia model. J. Neuroinflamm. 14:44. doi: 10.1186/s12974-017-0821-x
Rocha-Ferreira, E., Poupon, L., Zelco, A., Leverin, A.-L., Nair, S., Jonsdotter, A., et al. (2018). Neuroprotective exendin-4 enhances hypothermia therapy in a model of hypoxic-ischaemic encephalopathy. Brain 141, 2925–2942. doi: 10.1093/brain/awy220
Rocha-Ferreira, E., Rudge, B., Hughes, M. P., Rahim, A. A., Hristova, M., and Robertson, N. J. (2016). Immediate remote ischemic postconditioning reduces brain nitrotyrosine formation in a piglet asphyxia model. Oxid. Med. Cell Longev. 2016:5763743. doi: 10.1155/2016/5763743
Rosa, A. O., Egea, J., Martínez, A., García, A. G., and López, M. G. (2008). Neuroprotective effect of the new thiadiazolidinone NP00111 against oxygen-glucose deprivation in rat hippocampal slices: implication of ERK1/2 and PPARgamma receptors. Exp. Neurol. 212, 93–99. doi: 10.1016/j.expneurol.2008.03.008
Rotermund, C., Machetanz, G., and Fitzgerald, J. C. (2018). The therapeutic potential of metformin in neurodegenerative diseases. Front. Endocrinol. (Lausanne) 9:400. doi: 10.3389/fendo.2018.00400
Rousset, C. I., Leiper, F. C., Kichev, A., Gressens, P., Carling, D., Hagberg, H., et al. (2015). A dual role for AMP-activated protein kinase (AMPK) during neonatal hypoxic-ischaemic brain injury in mice. J. Neurochem. 133, 242–252. doi: 10.1111/jnc.13034
Ruddy, R. M., Adams, K. V., and Morshead, C. M. (2019). Age- and sex-dependent effects of metformin on neural precursor cells and cognitive recovery in a model of neonatal stroke. Sci. Adv. 5:eaax1912. doi: 10.1126/sciadv.aax1912
Salhab, W. A., Wyckoff, M. H., Laptook, A. R., and Perlman, J. M. (2004). Initial hypoglycemia and neonatal brain injury in term infants with severe fetal acidemia. Pediatrics 114, 361–366. doi: 10.1542/peds.114.2.361
Santiago, J. A., and Potashkin, J. A. (2013). Shared dysregulated pathways lead to Parkinson’s disease and diabetes. Trends Mol. Med. 19, 176–186. doi: 10.1016/j.molmed.2013.01.002
Semple, B. D., Blomgren, K., Gimlin, K., Ferriero, D. M., and Noble-Haeusslein, L. J. (2013). Brain development in rodents and humans: identifying benchmarks of maturation and vulnerability to injury across species. Prog. Neurobiol. 106–107, 1–16. doi: 10.1016/j.pneurobio.2013.04.001
Shankaran, S. (2012). Hypoxic-ischemic encephalopathy and novel strategies for neuroprotection. Clin. Perinatol. 39, 919–929. doi: 10.1016/j.clp.2012.09.008
Sheth, K. N., Kimberly, W. T., Elm, J. J., Kent, T. A., Mandava, P., Yoo, A. J., et al. (2014). Pilot study of intravenous glyburide in patients with a large ischemic stroke. Stroke 45, 281–283. doi: 10.1161/STROKEAHA.113.003352
Silveira, R. C., and Procianoy, R. S. (2015). Hypothermia therapy for newborns with hypoxic ischemic encephalopathy. J. Pediatr. (Rio J) 91, S78–S83. doi: 10.1016/j.jped.2015.07.004
Simard, J. M., Woo, S. K., Schwartzbauer, G. T., and Gerzanich, V. (2012). Sulfonylurea receptor 1 in central nervous system injury: a focused review. J. Cereb. Blood Flow Metab. 32, 1699–1717. doi: 10.1038/jcbfm.2012.91
Smith, A. L., Alexander, M., Rosenkrantz, T. S., Sadek, M. L., and Fitch, R. H. (2014). Sex differences in behavioral outcome following neonatal hypoxia ischemia: insights from a clinical meta-analysis and a rodent model of induced hypoxic ischemic brain injury. Exp. Neurol. 254, 54–67. doi: 10.1016/j.expneurol.2014.01.003
Sun, Q., Wei, L.-L., Zhang, M., Li, T.-X., Yang, C., Deng, S.-P., et al. (2019). Rapamycin inhibits activation of AMPK-mTOR signaling pathway-induced Alzheimer’s disease lesion in hippocampus of rats with type 2 diabetes mellitus. Int. J. Neurosci. 129, 179–188. doi: 10.1080/00207454.2018.1491571
Sundararajan, S., Gamboa, J. L., Victor, N. A., Wanderi, E. W., Lust, W. D., and Landreth, G. E. (2005). Peroxisome proliferator-activated receptor-γ ligands reduce inflammation and infarction size in transient focal ischemia. Neuroscience 130, 685–696. doi: 10.1016/j.neuroscience.2004.10.021
Tasca, C. I., Dal-Cim, T., and Cimarosti, H. (2015). In vitro oxygen-glucose deprivation to study ischemic cell death. Methods Mol. Biol. 1254, 197–210. doi: 10.1007/978-1-4939-2152-2_15
Thulé, P. M., and Umpierrez, G. (2014). Sulfonylureas: a new look at old therapy. Curr. Diab. Rep. 14:473. doi: 10.1007/s11892-014-0473-5
Tong, M., Dong, M., and de la Monte, S. M. (2009). Brain insulin-like growth factor and neurotrophin resistance in Parkinson’s disease and dementia with Lewy bodies: potential role of manganese neurotoxicity. J. Alzheimers Dis. 16, 585–599. doi: 10.3233/JAD-2009-0995
Tosun, C., Koltz, M. T., Kurland, D. B., Ijaz, H., Gurakar, M., Schwartzbauer, G., et al. (2013). The protective effect of glibenclamide in a model of hemorrhagic encephalopathy of prematurity. Brain Sci. 3, 215–238. doi: 10.3390/brainsci3010215
Vannucci, R. C. (1992). Cerebral carbohydrate and energy metabolism in perinatal hypoxic-ischemic brain damage. Brain Pathol. 2, 229–234. doi: 10.1111/j.1750-3639.1992.tb00696.x
Vannucci, R. C., and Vannucci, S. J. (2005). Perinatal hypoxic-ischemic brain damage: evolution of an animal model. Dev. Neurosci. 27, 81–86. doi: 10.1159/000085978
Volpe, J. J. (2012). Neonatal encephalopathy: an inadequate term for hypoxic-ischemic encephalopathy. Ann. Neurol. 72, 156–166. doi: 10.1002/ana.23647
Wang, J., Gallagher, D., DeVito, L. M., Cancino, G. I., Tsui, D., He, L., et al. (2012). Metformin activates an atypical PKC-CBP pathway to promote neurogenesis and enhance spatial memory formation. Cell Stem Cell 11, 23–35. doi: 10.1016/j.stem.2012.03.016
Wang, M.-D., Huang, Y., Zhang, G.-P., Mao, L., Xia, Y.-P., Mei, Y.-W., et al. (2012). Exendin-4 improved rat cortical neuron survival under oxygen/glucose deprivation through PKA pathway. Neuroscience 226, 388–396. doi: 10.1016/j.neuroscience.2012.09.025
Wang, X., Chang, Y., He, Y., Lyu, C., Li, H., Zhu, J., et al. (2019). Glimepiride and glibenclamide have comparable efficacy in treating acute ischemic stroke in mice. Neuropharmacology 162, 107845. doi: 10.1016/j.neuropharm.2019.107845
Wen, Y., Wu, K., Xie, Y., Dan, W., Zhan, Y., and Shi, Q. (2019). Inhibitory effects of glucagon-like peptide-1 receptor on epilepsy. Biochem. Biophys. Res. Commun. 511, 79–86. doi: 10.1016/j.bbrc.2019.02.028
Wiciński, M., Wódkiewicz, E., Słupski, M., Walczak, M., Socha, M., Malinowski, B., et al. (2018). Neuroprotective activity of sitagliptin via reduction of neuroinflammation beyond the incretin effect: focus on Alzheimer’s disease. Biomed. Res. Int. 2018:6091014. doi: 10.1155/2018/6091014
Wu, T.-W., McLean, C., Friedlich, P., Wisnowski, J., Grimm, J., Panigrahy, A., et al. (2014). Brain temperature in neonates with hypoxic-ischemic encephalopathy during therapeutic hypothermia. J. Pediatr. 165, 1129–1134. doi: 10.1016/j.jpeds.2014.07.022
Wyatt, J. S., Edwards, A. D., Azzopardi, D., and Reynolds, E. O. (1989). Magnetic resonance and near infrared spectroscopy for investigation of perinatal hypoxic-ischaemic brain injury. Arch. Dis. Child. 64, 953–963.
Xia, P., Pan, Y., Zhang, F., Wang, N., Wang, E., Guo, Q., et al. (2018). Pioglitazone confers neuroprotection against ischemia-induced pyroptosis due to its inhibitory effects on HMGB-1/RAGE and Rac1/ROS pathway by activating PPAR-? CPB 45, 2351–2368. doi: 10.1159/000488183
Xu, W., Yang, Y., Yuan, G., Zhu, W., Ma, D., and Hu, S. (2015). Exendin-4, a glucagon-like peptide-1 receptor agonist, reduces Alzheimer disease-associated tau hyperphosphorylation in the hippocampus of rats with type 2 diabetes. J. Investig. Med. 63, 267–272. doi: 10.1097/JIM.0000000000000129
Yakovleva, A. A., Zolotov, N. N., Sokolov, O. Y., Kost, N. V., Kolyasnikova, K. N., and Micheeva, I. G. (2015). Dipeptidylpeptidase 4 (DPP4, CD26) activity in the blood serum of term and preterm neonates with cerebral ischemia. Neuropeptides 52, 113–117. doi: 10.1016/j.npep.2015.05.001
Yang, D., Nakajo, Y., Iihara, K., Kataoka, H., and Yanamoto, H. (2013). Alogliptin, a dipeptidylpeptidase-4 inhibitor, for patients with diabetes mellitus type 2, induces tolerance to focal cerebral ischemia in non-diabetic, normal mice. Brain Res. 1517, 104–113. doi: 10.1016/j.brainres.2013.04.015
Yang, X., Feng, P., Zhang, X., Li, D., Wang, R., Ji, C., et al. (2019). The diabetes drug semaglutide reduces infarct size, inflammation, and apoptosis, and normalizes neurogenesis in a rat model of stroke. Neuropharmacology 158:107748. doi: 10.1016/j.neuropharm.2019.107748
Yimer, E. M., Surur, A., Wondafrash, D. Z., and Gebre, A. K. (2019). The effect of metformin in experimentally induced animal models of epileptic seizure. Behav. Neurol. 2019:6234758. doi: 10.1155/2019/6234758
Yun, C., and Xuefeng, W. (2013). Association between seizures and diabetes mellitus: a comprehensive review of literature. Curr. Diab. Rev. 9, 350–354. doi: 10.2174/15733998113099990060
Yun, S. P., Kam, T.-I., Panicker, N., Kim, S., Oh, Y., Park, J.-S., et al. (2018). Block of A1 astrocyte conversion by microglia is neuroprotective in models of Parkinson’s disease. Nat. Med. 24, 931–938. doi: 10.1038/s41591-018-0051-5
Zeng, S., Bai, J., Jiang, H., Zhu, J., Fu, C., He, M., et al. (2020). Treatment with liraglutide exerts neuroprotection after hypoxic–ischemic brain injury in neonatal rats via the PI3K/AKT/GSK3β pathway. Front. Cell. Neurosci. 13:585. doi: 10.3389/fncel.2019.00585
Zhang, H., Liu, Y., Guan, S., Qu, D., Wang, L., Wang, X., et al. (2016). An orally active allosteric GLP-1 receptor agonist is neuroprotective in cellular and rodent models of stroke. PLoS One 11:e0148827. doi: 10.1371/journal.pone.0148827
Zhang, H.-L., Xu, M., Wei, C., Qin, A.-P., Liu, C.-F., Hong, L.-Z., et al. (2011). Neuroprotective effects of pioglitazone in a rat model of permanent focal cerebral ischemia are associated with peroxisome proliferator-activated receptor gamma-mediated suppression of nuclear factor-κB signaling pathway. Neuroscience 176, 381–395. doi: 10.1016/j.neuroscience.2010.12.029
Zhao, Y., Li, H., Fang, F., Qin, T., Xiao, W., Wang, Z., et al. (2018). Geniposide improves repeated restraint stress-induced depression-like behavior in mice by ameliorating neuronal apoptosis via regulating GLP-1R/AKT signaling pathway. Neurosci. Lett. 676, 19–26. doi: 10.1016/j.neulet.2018.04.010
Zhou, Y., Fathali, N., Lekic, T., Tang, J., and Zhang, J. H. (2009). Glibenclamide improves neurological function in neonatal hypoxia-ischemia in rats. Brain Res. 1270, 131–139. doi: 10.1016/j.brainres.2009.03.010
Keywords: hypoxic-ischemic encephalopathy, perinatal brain injury, cerebral palsy, neuroprotection, hypothermia, diabetes
Citation: Poupon-Bejuit L, Rocha-Ferreira E, Thornton C, Hagberg H and Rahim AA (2020) Neuroprotective Effects of Diabetes Drugs for the Treatment of Neonatal Hypoxia-Ischemia Encephalopathy. Front. Cell. Neurosci. 14:112. doi: 10.3389/fncel.2020.00112
Received: 14 February 2020; Accepted: 08 April 2020;
Published: 06 May 2020.
Edited by:
Chao Deng, University of Wollongong, AustraliaReviewed by:
Sandra E. Juul, University of Washington, United StatesHemmen Sabir, University Hospital Bonn, Germany
Copyright © 2020 Poupon-Bejuit, Rocha-Ferreira, Thornton, Hagberg and Rahim. This is an open-access article distributed under the terms of the Creative Commons Attribution License (CC BY). The use, distribution or reproduction in other forums is permitted, provided the original author(s) and the copyright owner(s) are credited and that the original publication in this journal is cited, in accordance with accepted academic practice. No use, distribution or reproduction is permitted which does not comply with these terms.
*Correspondence: Ahad A. Rahim, YS5yYWhpbUB1Y2wuYWMudWs=