- IRCCS SDN, Naples, Italy
Glial activation characterizes most neurodegenerative and psychiatric diseases, often anticipating clinical manifestations and macroscopical brain alterations. Although imaging techniques have improved diagnostic accuracy in many neurological conditions, often supporting diagnosis, prognosis prediction and treatment outcome, very few molecular imaging probes, specifically focused on microglial and astrocytic activation, have been translated to a clinical setting. In this context, hybrid positron emission tomography (PET)/magnetic resonance (MR) scanners represent the most advanced tool for molecular imaging, combining the functional specificity of PET radiotracers (e.g., targeting metabolism, hypoxia, and inflammation) to both high-resolution and multiparametric information derived by MR in a single imaging acquisition session. This simultaneity of findings achievable by PET/MR, if useful for reciprocal technical adjustments regarding temporal and spatial cross-modal alignment/synchronization, opens still debated issues about its clinical value in neurological patients, possibly incompliant and highly variable from a clinical point of view. While several preclinical and clinical studies have investigated the sensitivity of PET tracers to track microglial (mainly TSPO ligands) and astrocytic (mainly MAOB ligands) activation, less studies have focused on MR specificity to this topic (e.g., through the assessment of diffusion properties and T2 relaxometry), and only few exploiting the integration of simultaneous hybrid acquisition. This review aims at summarizing and critically review the current state about PET and MR imaging for glial targets, as well as the potential added value of hybrid scanners for characterizing microglial and astrocytic activation.
Introduction
Selective cortical or subcortical atrophy, neuronal death and shrinkage characterize pathological feature across different neurodegenerative diseases and brain disorders (Chi et al., 2018).
Nevertheless, exclusively neuron-centric approaches to neuropathological phenomena have not returned new breakthroughs in the prevention and therapy of brain disorders (Verkhratsky et al., 2014). This gap is due to the complexity of neuronal networks that cannot only be explained by neuronal activity, addressing research efforts to different actors in the central nervous system (CNS): the glia (Papa et al., 2014).
Glial cells, mainly represented by microglia and astrocytes, are key components for development and maintenance of brain functions and circuitry. Microglial cells correspond to the brain-resident macrophage playing an essential role for synaptic pruning, CNS repair, and mainly, as cellular mediators of neuroinflammation that characterizes different brain disorders (Colonna and Butovsky, 2017). Astrocytes instead, initially deputed to support neuronal activity, have now gained a central role in brain function, for example being part of the concept of tripartite synapse and gliotransmission for their effects on neuronal communication and plasticity (Pérez-Alvarez and Araque, 2013).
Increasing evidences support the role of an altered glial function as an underlying dynamic feature in psychiatric and neurological disorders (Garden and Campbell, 2016).
As pharmaceutical efforts begin to focus on glial specific targets, a limiting step in knowledge consists of the unavailability of validated biomarkers to assess and monitor gliosis longitudinally, supporting clinical management and potentially identifying best responders to cells-specific drugs (Garden and Campbell, 2016).
Accordingly, the interest in the development of novel methods to investigate glial activation and, more in general, neuroinflammation, has surged over the past 15 years. Neuroimaging offers a wide panel of non- or minimally invasive techniques to characterize neuroinflammatory processes.
Among different imaging techniques, non-invasive brain functional measurements using positron emission tomography (PET) and detailed morpho-functional information provided by magnetic resonance imaging (MRI) appeared the more appealing for translational purposes, being both used in preclinical and clinical settings.
In this context, hybrid PET/MR scanner represent the most advanced tool for molecular imaging, combining the functional specificity of PET radiotracers (e.g., targeting metabolism, hypoxia, inflammation, and specific membrane receptors) to both high-resolution and multiparametric information derived by MR in a single imaging acquisition session.
Aims of this review are: to summarize preclinical and clinical studies employing both PET and MRI techniques to investigate glial contribute in brain disorders; to critically review the main evidences raised up by PET glial tracers in neurodegenerative disorders and their feasibility in a clinical context; to investigate the potential added value of hybrid PET/MRI for characterizing microglial and astrocytic activation in neurological and psychiatric diseases.
Hybrid PET/MR Scanner
Compared to other more widespread and validated hybrid imaging techniques, like PET/computed tomography (CT), PET/MR scanner constitutes the first real effort to effectively integrate two modalities by simultaneous acquisition, going beyond the serial PET/CT, assuring naturally co-registered multimodal images (Monti et al., 2017). The integration and coregistration of multimodal information achieved by different imaging techniques is essential for a complete understanding of the brain processes, representing a crucial step both for visual qualitative assessment and for multiparametric quantitative analysis. The retrospective coregistration of complex diagnostic datasets serially acquired on different scanners is typically achieved via software, through transformation algorithms based on the anatomical information of the CT component of PET/CT. Despite of the cost effectiveness of this approach compared to hybrid solutions, retrospective coregistration could be particularly challenging and technically demanding in un-collaborative patients (Monti et al., 2017). This problem is intrinsically overcome by hybrid scanners that allow you to simultaneously acquire images that share the same coordinate system (Zaidi and Guerra, 2011).
This innovation has been possible after the development of dedicated hardware components for each modality, and not mutually influenced by the magnetic field or photon pathway (Aiello et al., 2018).
Nowadays, integrated PET/MRI represents the most advanced tool for molecular imaging, opening new insights for the characterization of neurological and psychiatric disorders, and possibly for a multifaceted patient management (Aiello et al., 2015, 2016; Cavaliere et al., 2018a). Indeed, this innovative clinical diagnostic scanner allows to combine the functional specificity of PET radiotracers (e.g., targeting metabolism, hypoxia, inflammation, specific ligands, or receptors) to both high-resolution and multiparametric information derived by MR in a single imaging acquisition session (Herzog et al., 2010; Aiello et al., 2019). This simultaneity of findings achievable by PET/MR, if useful for reciprocal technical adjustments regarding temporal and spatial cross-modal alignment/synchronization, opens still debated insights about its clinical value in neurological patients, possibly incompliant and highly variable from a clinical point of view (Zaidi and Guerra, 2011; Cavaliere et al., 2018b).
Nuclear medicine imaging techniques, like PET, offer the unique opportunity to investigate in vivo and in a mini- or not-invasively manner, a plethora of molecular mechanisms, depending on the selectivity of the radio-tracer used. This potentiality has pushed radio-chemists and drug industries to test and investigate new potentially innovative tracers able to specifically target pathophysiological pathways, such as neuroinflammation, glial cells or membrane receptors to disentangle neurodegenerative phenomena (Herzog et al., 2010). However, stand-alone PET imaging is still limited by low spatial resolution, that obliges it to be co-registered to an higher resolution imaging techniques, such as CT and MR. Compared to CT, MRI provides different quantitative information (e.g., water and metabolites diffusion, metabolites concentrations, regional perfusion, and activation), simply modifying sequences’ parameters, representing the gold standard for soft tissue and brain study and providing complementary information compared to PET imaging (Cavaliere et al., 2018b; Marchitelli et al., 2018). Moreover, the higher contrast resolution provided by MR can be more suitable for the segmentation of regions of reference useful to normalize PET signal in order to extract quantitative uptake parameters.
While, similarly to PET/CT scanners, findings derived by MRI and PET can be sequentially achieved in two different times, for highly dynamic systems like the brain this temporal gap could bias intermodality comparison. Indeed, sequential approach supposes that no significant modifications have occurred in the underlying state of the subject between two imaging sessions — a statement that could be ineffective both in physiological terms (e.g., changes in cognitive states or alertness) and in pathological conditions (e.g., psychiatric or neurological disorders) or as a result of medical interventions through psychological counseling, drugs or electrical/magnetic brain stimulations (Aiello et al., 2018; Cavaliere et al., 2018b). For these reasons, hybrid PET/MR scanners allow to overcome these limitations by simultaneously acquiring morphological, functional, molecular or metabolic information derived by both MRI and PET in a single shot.
PET and MR Imaging of Microglial Activation
Neuroinflammation is a dynamic flogistic response which involve a complex multicellular cascade including the activation of both microglia and astrocytes, and the release of different neuroactive compounds.
Previous experimental studies have provided overwhelming evidence of the pivotal involvement of microglia-related molecular networks in the pathophysiology of many neurodegenerative and psychiatric diseases (Condello et al., 2018). However, the precise mechanisms by which microglia affect the disease’s progression and modify neuropathology remain poorly understood.
Different activation phenotypes seem characterize chronic brain pathologies, ranging from a neuroprotective and anti-inflammatory M2 phenotype, mainly represented in an early stage, to the shift into the pro-inflammatory M1 phenotype, typical of later stages and contributing to neuronal dysfunction, injury, and disease progression (Figure 1) (Shen et al., 2018).
Therefore, the development of non-invasive molecular imaging methods able to dynamically characterize the spatio-temporal profile of neuroinflammatory biomarkers is widely attracting scientific and clinical interest, also for the application of immunomodulatory therapies both in clinical and preclinical settings (Narayanaswami et al., 2018).
Positron emission tomography imaging of microglia has emerged over recent years, through the development of target-specific radiotracers and, among these, mainly the translocator protein-18 kDa (TSPO) (Table 1) (Figure 2).
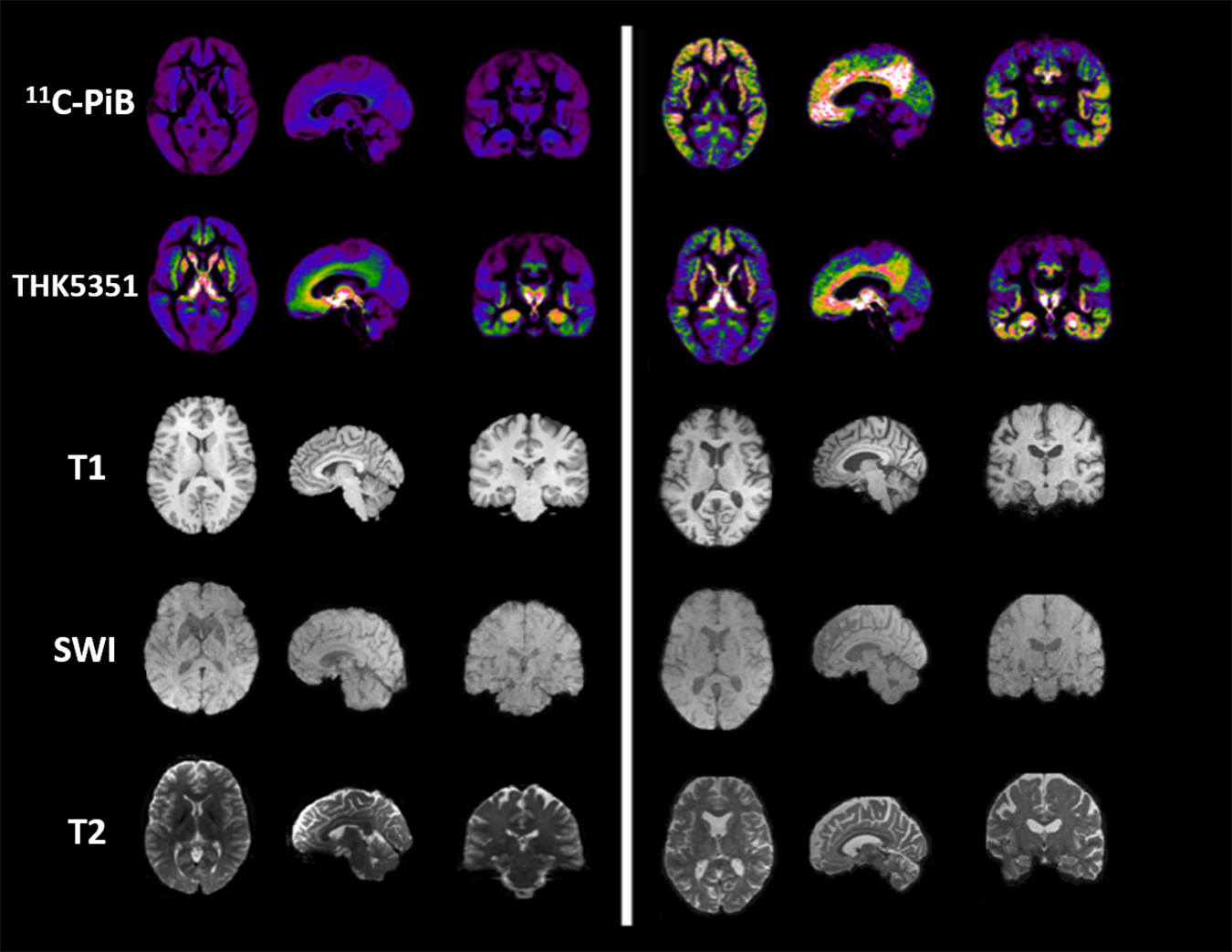
Figure 2. Imaging examples in PET and MR imaging. The figure depicts, from left to right, axial, sagittal and coronal projections of healthy (Left) and AD spectrum (Right) brain for different PET (Shigemoto et al., 2018) and MR contrasts. From top to bottom row: microglial uptake as revealed by 11C-PiB PET tracer; astrocytic uptake as revealed by 18F-THK5351 PET tracer; structural 3D T1-weighted MR used as anatomical reference for PET and to highlight cortical atrophy and ventricular enlargement; 3D Susceptibility-weighted imaging MR linked to iron deposition and microgliosis; T2-weighted MR scan used for T2 mapping and astrogliosis.
The first-generation radioligand [11C](R)-PK11195 has been the most widely studied in clinical studies and different neurodegenerative diseases, including multiple sclerosis (Airas et al., 2018), Creutzfeldt-Jakob disease (Iaccarino et al., 2018), and also in psychiatric disorders such as schizophrenia (Di Biase et al., 2017). Although the findings of an increased uptake of this tracer in these pathologies, limitations including low signal-to-noise ratio and high non-specific binding has addressed for the synthesis of second- and third-generation TSPO-specific radiopharmaceuticals, linked to [11C] or [18F] and including [11C]-PBR28, [18F]-DPA-714, [18F]-PBR06, [18F]-FEPPA, and [18F]-GE-180 (all used in a clinical context, except for [18F]-DPA-714) (Luo et al., 2018; Singhal et al., 2018; Best et al., 2019). Similarly, different studies have reported selective microglial uptake of these tracers in multiple sclerosis animal models and patients (Hagens et al., 2018; Herranz et al., 2019; Nack et al., 2019), amyotrophic lateral sclerosis (Zürcher et al., 2015; Datta et al., 2017), Alzheimer’s disease (Alam et al., 2017; Keller et al., 2018; Focke et al., 2019), and Lyme disease on humans (Coughlin et al., 2018), and stroke experimental models (Miyajima et al., 2018), with more discordant results for psychiatric patients, suffering from schizophrenia (Di Biase et al., 2017; Hafizi et al., 2017; Ottoy et al., 2018; Selvaraj et al., 2018) and major depression (Li et al., 2018), probably due to the different stage of disease. Interestingly, one study on fibromyalgia subjects attempts to demonstrate specificity of TSPO tracers for microglia, considering that an high expression of this molecule was also detected in activated astrocytes (Albrecht et al., 2019). The authors, using [11C]PBR28, which binds to the TSPO, and [11C]-DED thought to primarily reflect astrocytic (but not microglial) signal, demonstrated although in a small size sample, a selective cortical uptake of microglial tracer but not of the astrocytic one (Albrecht et al., 2019).
Nevertheless, the application of TSPO tracers is affected by significant inter-subjects variability, essentially due to a rs6971 polymorphism that affects TSPO binding mainly in first- and second-generation radioligands (Hafizi et al., 2017), and more importantly, they are not able to differentiate microglial phenotype, distinguishing between conservative and detrimental activation. Moreover, although TSPO is upregulated in activated glial cells, its function and role in the immunity response is still unclear. Finally, considering that major evidences emerged by TSPO uptake in multiple sclerosis patients, and the very low brain uptake in healthy subjects, several doubts have been raised for the blood–brain barrier permeability of these tracers in physiological conditions (Albert et al., 2019).
For this reason, other microglial targets have been identified for specific radiotracers in animal models, such as the cannabinoid receptor type 2 ([18F]-D8-THC) (Ni et al., 2019; Gifford et al., 2002), the P2X7 receptor ([11C]-GSK1482160) (Han et al., 2017), the cyclo-oxygenase 1 ([11C]-Ketoprofen methyl ester) (Shukuri et al., 2011), the cyclo-oxygenase 2 ([11C]-Celecoxib) (Kumar et al., 2018), and the macrophage colony-stimulating factor 1 ([11C]CPPC [5-cyano-N-(4-(4-[11C]methylpiperazin-1-yl)-2-(piperidin-1-yl) phenyl)furan-2-carboxamide]) (Horti et al., 2019).
Regarding the use of MRI for microgliosis identification, less studies have tried to directly characterize microglial activation in specific brain regions. Changes of iron deposition, quantified using quantitative susceptibility mapping in MR, have been correlated with activated microglia/macrophages at edges of some chronic demyelinated lesions in patients suffering from multiple sclerosis (Dal-Bianco et al., 2017; Gillen et al., 2018; Hametner et al., 2018). Advanced diffusion models, based on neurite orientation dispersion and density imaging (NODDI) in MR, have been proved to be sensitive to microglial density and to the cellular changes associated with microglial activation in a preclinical setting (Yi et al., 2019). Finally, in amyotrophic lateral sclerosis patients, a technique based on diffusion spectroscopy has been applied to identify the increase of the predominantly glial metabolites (unspecific for microglia and astrocytes) tCr (creatine + phosphocreatine) and tCho (choline-containing compounds) in the primary motor cortex (Reischauer et al., 2018).
Despite the proved potential of both PET and MRI for the microglial imaging, very few studies have integrated both the modalities in humans and, generally, the majority use MRI to simply colocalize TSPO-uptake with multiple sclerosis plaques identified by MRI (Colasanti et al., 2014; Kang et al., 2018; Unterrainer et al., 2018; Kaunzner et al., 2019). Up to now, only three studies performed a simultaneous PET/MRI to investigate microglial activation in neurodegenerative diseases. The first one, using a selective P2X7R radiotracers in patients affected by Parkinson’s disease, was not able to identify significant differences in PET uptake between patients and control subjects (van Weehaeghe et al., 2019). The second one, using a second-generation TSPO radiotracer in patients affected by multiple sclerosis, demonstrated microglial activation in both normal appearing cerebellum and segmented lesions (Barletta et al., 2019). The latter combines magnetic resonance spectroscopy of glial metabolites to TSPO-radiotracer uptake in patients affected by amyotrophic lateral sclerosis demonstrating a positive correlation between MR and PET biomarkers of neuroinflammation (Ratai et al., 2018) (Figure 2).
PET and MR Imaging of Astrocytic Activation
Astrocytes are the most abundant cell type in the brain, and participate to complex neuronal-glial interactions to assure synaptic homeostasis and metabolic sustainment for neurons (Miller, 2018). As for microglial cells, different phenotypes seem characterize astrocytic activation in physiological and pathological conditions, ranging from a pro-inflammatory A1 phenotype to a protective and anti-inflammatory A2 phenotype (Figure 1) (Miller, 2018).
Previously considered only as a supporting neuronal cells, astrocytic contributions to different neurodegenerative and psychiatric diseases has been recently revised (Papa et al., 2014).
Compared to microglial visualization, PET radiotracers specific for astrocytic function have been only recently applied for clinical purpose, mainly binding the mono-amine oxidase type B (MAOB) enzyme, highly expressed by activated astrocytes (Table 1) (Figure 2) (Ishibashi et al., 2019). PET studies using the MAOB radiotracers 11C-DED or [18F]THK5351 in patients affected by Alzheimer’s disease have demonstrated an high correlation between brain tau deposition and astrocytic uptake (Harada et al., 2018) and between cortical glucose hypometabolism, detected by 18F-fluorodeoxyglucose [18F]-FDG PET, and longitudinal decline in astrocytic function detected by 11C-DED (Carter et al., 2019). These findings are in agreement with another clinical paper that demonstrate an increase of FDG uptake following selective activation of astrocytic glutamate transport (Zimmer et al., 2017). A specific regional increase of astrocytic function was also detected in two cases of progressive sopranuclear palsy studied with [18F]THK5351 PET (Ishiki et al., 2018).
Regarding the use of MRI to highlight astrocytic involvement in neurological and psychiatric diseases, few research groups have stressed MR potentiality to achieve this aim (Figure 2). Several preclinical studies proposed magnetic resonance spectroscopy to highlight lactate (Blanc et al., 2019) or acetate (Dehghani et al., 2017) peaks changes as surrogate markers specific for astrocytic metabolic homeostasis. However, magnetic field strength of clinically available scanner is not able to spectrally resolve these metabolites by surrounding peaks. In another clinical study (Alshelh et al., 2018), regional alterations in T2 relaxation times have been speculated as indicative of astroglial activation in patients suffered of neuropathic pain. More recently, the origin of signal used by functional MRI to investigate large-group of neuronal activations has been debated, considering preclinical evidences that evoked astrocytic Ca2+ waves can correlate with increased EEG power signal (Wang et al., 2018), astrocyte-evoked BOLD fluctuations (Takata et al., 2018), and transcranial direct current stimulation response (Monai and Hirase, 2018). These findings render furtherly more appealing the use of hybrid scanner that can acquire simultaneously PET, fMRI, and EEG signal (Mele et al., 2019).
Anyway, evidences derived by MRI on astrocytic activation are still confined to few groups, and often on limited samples. Few studies have serially used both PET and MRI with this purpose, and employing MR findings to colocalize PET uptake. In a longitudinal study on patients affected by corticobasal syndrome, [18F]THK5351 binding have been demonstrated to detect dynamical astrogliosis in specific cortical regions (Ezura et al., 2019). In a case of multiple sclerosis, instead, the authors demonstrated the coregistration of [18F]THK5351 uptake with demyelinating plaques (Ishibashi et al., 2019). Another study compared the spatial uptake patterns of [18F]THK5351 and fMRI network alterations in patients with early AD and healthy controls, showing a similar pattern for the precuneus, a region crucial for Alzheimer progression (Yokoi et al., 2018).
Finally, in patients suffering of semantic variant primary progressive aphasia, several authors demonstrated that the MAOB tracers can be more sensitive to detective neurodegenerative alterations compared to MRI (Kobayashi et al., 2018).
Glial PET Radiotracers: a Critical Point of View
Despite the remarkable development of radiotracers for numerous molecular targets implicated in the process of neuroinflammation, only a few have been used on patients successfully.
TSPO PET Tracers
The main problems of TSPO tracers are that they cannot distinguish pro- and anti-inflammatory responses, they have a low signal-to-noise ratio and high non-specific binding, low dynamic response variation during neurodegenerative pathologies (Figure 2). Furthermore the main problem in the clinical use of these PET tracers is given the presence of polymorphism (SNP) in TSPO gene. These problems have been reduced and/or eliminated with second and third generation TSPO PET tracers, however, there is still no possible differentiation between the M1 (neurotoxic) and M2 (neuroprotective) genotypes. Moreover, even if the 3D pentameric structure of the TSPO has been revealed, the role of this receptor does not yet appear to be clear, since the influence of receptor upregulation as an immune reaction is not clear. Currently [18F] -GE180 is used as a third-generation tracer in clinical trials. Although [18F] -GE180 is able to pass in CNS only in case of blood–brain barrier breakdown, with low BBB permeability in human healthy brain, this radiotracer is a very important neuroinflammation imaging agent in various CNS diseases (Albert et al., 2019).
Cannabinoid Receptor Type 2 PET Tracers
Due to its high brain density, this receptor has been subjected to in vivo imaging using PET techniques using radioligands, such as Δ8-Tetrahydrocannabinol (Δ8-THC) labeled with fluorine-18. However, the PET images obtained after injection of [18F]-Δ8-THC in primates do not show a particular specific region of cerebral localization, so much so that the radiotracer appears to be widely diffused in all regions of the brain (Gifford et al., 2002). Some of the synthetic derivatives of classic cannabinoids, which show nano affinity for CB2 receptors, may prove to be successful candidates for in vivo imaging of these receptors, including pyrazole derivatives and aminoalkylindole derivatives. Since the level of expression of CB2 receptors in the healthy brain is low and there is an increase of these receptors in pathological conditions, much research has focused on these receptors. To date only the radiotracer [11C] -NE40 has been used in humans for biodistribution studies on healthy patients, to verify the uptake and washout (Ahmad et al., 2013). CB2 ligands are still in the preclinical evaluation phase, however, they can represent a valid alternative in the evaluation of microglial activation (Yamagishi et al., 2019).
Cyclooxygenase-2 PET Tracers
Despite the potential given by the study of the increase in cyclooxygenases (COX1 and COX2) in inflammation, actually only very few radiotracers have been synthesized and studied. For neuroinflammation studies it is mandatory to use selective radioligands for the respective isoforms COX1 and COX2. The advantage in the use of [11C]-celecoxib is certainly represented by the ability to pass the blood–brain barrier, even when it is not damaged (Kumar et al., 2018).
P2X7 Receptor PET Tracers
P2X7 receptor is an adenosine triphosphate ATP-gated purinoreceptor that is widely expressed in microglia and astrocytes. The activation of P2X7 receptor leads to the release of the proinflammatory mediators such as cytokine IL-1β in the brain. In fact the increase in the expression of this receptor leads to the activation of microglia, more than its over-expression is a consequence of the activation of microglia. Over the last years, research has focused on the synthesis and study of ligands of this receptor. The most promising are certainly: [11C]-GSK1482160, [11C]-SMW139, and [18F]-JNJ-64413739. The first [11C]-GSK1482160 has been studied in healthy volunteer patients for the estimation of radiopharmaceutical radiation dosimetry and biodistribution (Green et al., 2018). The first PET/MRI study, even if preliminary, on patients affected by multiple sclerosis was conducted in 2019 with the administration of [11C]-SMW139 (Hagens et al., 2019). The Janssen compound [18F]-JNJ-64413739 is labeled with fluorine-18, which certainly represents an advantage from a clinical point of view considering the longer radioisotope half-life (Kolb et al., 2019). [18F]-JNJ-64413739 is still under evaluation in preclinical study phase and data on patients are not yet available, however, the first studies on animals show its potential as a PET tracer for neuroinflammation.
Macrophage Colony-Stimulating Factor 1 Receptor (CSF1R) PET Tracer
CSF1R is a surface receptor (tyrosine kinase family receptors) mainly expressed by microglia. Recently a specific radioligand of this receptor was synthesized and studied, [11C]-CPPC (Horti et al., 2019). Even if this PET ligand is still in a preclinical phase of animal experiments, this new class of radioligands could be very promising for the study of microglial PET/MRI activation.
Monoamine Oxidase-B PET Tracers
During neuroinflammation, astrocytic increased MAO-B (amine oxidases) activity which leads to an oxidative stress due to the formation of hydrogen peroxides (Gulyás et al., 2011). Among PET ligands MAO-B studied and synthesized over the years, two radiotracers were successfully used in patients, one of which containing carbon-11([11C]-L-deprenyl-D2) (Arakawa et al., 2017), the other containing fluorine-18 ([18F]-THK5351) (Ishibashi et al., 2019). The last one was used in a patient with relapsing-remitting multiple sclerosis (MS), it was seen that [18F]-THK5351 accumulation corresponded to sites identified in MRI where there were MS plaques, in lesions undergoing astrogliosis.
Bimodal Probes as Future Perspectives
Although PET/MRI has been often addressed as the new frontier of molecular imaging (Chen and Chen, 2010), very few studies have employed this technique in glial imaging, and often limiting MR to a structural reference for PET signal. One possible innovation in this field could be represented by the development of an efficient and reliable PET/MR dual imaging probe, that if challenging for chemists and neuroscientists (Bouziotis et al., 2013; Vecchione et al., 2017), can help to identify killer applications for PET/MRI.
A general issue for new single- or multi-modal imaging probes that target the brain and that have to be taken into account is the permeability of the blood–brain barrier, a complex multicellular structure that protect CNS by external neurotoxic substances and which functions are assured also by astrocytic endfeet (Poupot et al., 2018). A possible solution is represented by the use of physiological transfer existing across the barrier, like the use of specific carriers or the transcytosis phenomenon (Pulgar, 2019).
Among novel imaging tools, different nanoparticles (usually smaller than 100 nm) have been extensively used in preclinical settings as MRI contrast agent combined with a PET tracer, mainly for oncological and more recently cardiovascular imaging (Aime et al., 2002; Uppal et al., 2011; Lewis et al., 2015; Kirschbaum et al., 2016; Vecchione et al., 2017; Grimaldi et al., 2019). Recently, a potential multimodal PET/MRI probe has been described to target microglia and neuroinflammation in a mouse model (Tang et al., 2018). The proposed nanoparticle selectively binds to the scavenger receptor class A (SR-A) expressed on activated microglia and is iron-oxide coated and so detectable by T2∗ -weighted MRI.
Conclusion
In this review main PET and MR imaging biomarkers for microglial and astrocytic activation have been summarized. Furthermore, these studies also demonstrate potential benefits for the integration of findings achievable by simultaneous PET/MRI scanners, although still few employed in the literature. While the technological challenges seem to be overcome with new powerful scanners able to in vivo characterize molecular processes, more efforts to identify selective glial targets and efficient multimodal imaging probes, potentially useful for tailored treatments targeting glial activation, are needed.
Author Contributions
CC designed and wrote the manuscript. LT, DF, and VA wrote the manuscript and prepared figures/tables. MA and MS revised and approved the text.
Conflict of Interest
The authors declare that the research was conducted in the absence of any commercial or financial relationships that could be construed as a potential conflict of interest.
References
Ahmad, R., Koole, M., Evens, N., Serdons, K., Verbruggen, A., Bormans, G., et al. (2013). Whole-body biodistribution and radiation dosimetry of the cannabinoid type 2 receptor ligand [11C]-NE40 in healthy subjects. Mol. Imaging Biol. 15, 384–390. doi: 10.1007/s11307-013-0626-y
Aiello, M., Cavaliere, C., Fiorenza, D., Duggento, A., Passamonti, L., and Toschi, N. (2019). Neuroinflammation inneurodegenerative diseases: current multi-modal imaging studies and future opportunities for hybridPET/MRI. Neuroscience 403, 125–135. doi: 10.1016/j.neuroscience.2018.07.033
Aiello, M., Cavaliere, C., Marchitelli, R., d’Albore, A., De Vita, E., and Salvatore, M. (2018). Hybrid PET/MRIMethodology. Int. Rev. Neurobiol. 141, 97–128. doi: 10.1016/bs.irn.2018.07.026
Aiello, M., Cavaliere, C., and Salvatore, M. (2016). Hybrid PET/MR imaging and brain connectivity. Front. Neurosci. 10:64. doi: 10.3389/fnins.2016.00064
Aiello, M., Monti, S., Inglese, M., Forte, E., Cavaliere, C., Catalano, O. A., et al. (2015). A multimodalfusion scheme for the enhancement of PET/MR viewing. Ejnmmi. Phys. 2(Suppl.1):A32. doi: 10.1186/2197-7364-2-S1-A32
Aime, S., Frullano, L., and Crich, S. G. (2002). Compartmentalization of a gadolinium complex in the apoferritin cavity: a route to obtain high relaxivity contrast agents for magnetic resonance imaging. Angewandte Chem. Int. Ed. 41, 1017–1019. doi: 10.1002/1521-3773(20020315)41:6<1017::aid-anie1017>3.0.co;2-p
Airas, L., Nylund, M., and Rissanen, E. (2018). Evaluation of microglial activation in multiple sclerosis patients using positron emission tomography. Front. Neurol. 9:181. doi: 10.3389/fneur.2018.00181
Alam, M. M., Lee, J., and Lee, S. Y. (2017). Recent progress in the development of TSPO PET ligands for neuroinflammation imaging in neurological diseases. Nucl. Med. Mol. Imaging 51, 283–296. doi: 10.1007/s13139-017-0475-8
Albert, N. L., Unterrainer, M., Brendel, M., Kaiser, L., Zweckstetter, M., Cumming, P., et al. (2019). In response to: the validity of 18F-GE180 as a TSPO imaging agent. Eur, J. Nucl. Med. Mol. Imaging 46, 1208–1211. doi: 10.1007/s00259-019-04294-8
Albrecht, D. S., Forsberg, A., Sandström, A., Bergan, C., Kadetoff, D., Protsenko, E., et al. (2019). Brain glial activation in fibromyalgia - A multi-site positron emission tomography investigation. Brain Behav. Immun. 75, 72–83. doi: 10.1016/j.bbi.2018.09.018
Alshelh, Z., Di Pietro, F., Mills, E. P., Vickers, E. R., Peck, C. C., Murray, G. M., et al. (2018). Altered regional brain T2 relaxation times in individuals with chronic orofacial neuropathic pain. Neuroimage Clin. 19, 167–173. doi: 10.1016/j.nicl.2018.04.015
Arakawa, R., Stenkrona, P., Takano, A., Nag, S., Maior, R. S., and Halldin, C. (2017). Test-retest reproducibility of [11C]-L-deprenyl-D2 binding to MAO-B in the human brain. Ejnmmi Res. Dec 7:54. doi: 10.1186/s13550-017-0301-4
Barletta, V. T., Herranz, E., Treaba, C. A., Ouellette, R., Mehndiratta, A., Loggia, M., et al. (2019). Evidence of diffuse cerebellar neuroinflammation in multiple sclerosis by 11C-PBR28 MR-PET. Mult. Scler. doi: 10.1177/1352458519843048 [Epub ahead of print].
Best, L., Ghadery, C., Pavese, N., Tai, Y. F., and Strafella, A. P. (2019). New and old TSPO PET radioligands for imaging brain microglial activation in neurodegenerative disease. Curr. Neurol. Neurosci. Rep 19:24. doi: 10.1007/s11910-019-0934-y
Blanc, J., Roumes, H., Mazuel, L., Massot, P., Raffard, G., Biran, M., et al. (2019). Functional magnetic resonance spectroscopy at 7 T in the rat barrel cortex during whisker activation. J. Vis. Exp. 8:e58912. doi: 10.3791/58912
Bouziotis, P., Psimadas, D., Tsotakos, T., Stamopoulos, D., and Tsoukalas, C. (2013). Radiolabeled iron oxide nanoparticles as dual-modality SPECT/MRI and PET/MRI agents. Curr. Top. Med. Chem. 12, 2694–2702. doi: 10.2174/1568026611212230007
Carter, S. F., Chiotis, K., Nordberg, A., and Rodriguez-Vieitez, E. (2019). Longitudinal association between astrocyte function and glucose metabolism in autosomal dominant Alzheimer’s disease. Eur. J. Nucl. Med. Mol. Imaging 46, 348–356. doi: 10.1007/s00259-018-4217-7
Cavaliere, C., Kandeepan, S., Aiello, M., Ribeiro de Paula, D., Marchitelli, R., Fiorenza, S., et al. (2018a). Multimodal neuroimaging approach to variability of functional connectivity in disorders ofconsciousness: a PET/MRI pilot study. Front. Neurol. 9:861. doi: 10.3389/fneur.2018.00861
Cavaliere, C., Longarzo, M., Orsini, M., Aiello, M., and Grossi, D. (2018b). Fronto-temporal circuits in musicalhallucinations: a PET-MR case study. Front. Hum. Neurosci. 12:385. doi: 10.3389/fnhum.2018.00385
Chen, K., and Chen, X. (2010). Design and development of molecular imaging probes. Curr. Top. Med. Chem. 10, 1227–1236. doi: 10.2174/156802610791384225
Chi, H., Chang, H. Y., and Sang, T. K. (2018). Neuronal cell death mechanisms in major neurodegenerative diseases. Int. J. Mol. Sci. 19:3082. doi: 10.3390/ijms19103082
Colasanti, A., Guo, Q., Muhlert, N., Giannetti, P., Onega, M., Newbould, R. D., et al. (2014). In vivo assessment of brain white matter inflammation in multiple sclerosis with (18)F-PBR111 PET. J. Nucl Med. 55, 1112–1118. doi: 10.2967/jnumed.113.135129
Colonna, M., and Butovsky, O. (2017). Microglia function in the central nervous system during health and neurodegeneration. Annu. Rev. Immunol. 35, 441–468. doi: 10.1146/annurev-immunol-051116-052358
Condello, C., Yuan, P., and Grutzendler, J. (2018). Microglia-mediated neuroprotection, trem2, and alzheimer’s disease: evidence from optical imaging. Biol. Psychiatry 83, 377–387. doi: 10.1016/j.biopsych.2017.10.007
Coughlin, J. M., Yang, T., Rebman, A. W., Bechtold, K. T., Du, Y., Mathews, W. B., et al. (2018). Imaging glial activation in patients with post-treatment Lyme disease symptoms: a pilot study using [11C]DPA-713 PET. J. Neuroinflammation 15:346. doi: 10.1186/s12974-018-1381-1384
Dal-Bianco, A., Grabner, G., Kronnerwetter, C., Weber, M., Höftberger, R., Berger, T., et al. (2017). Slow expansion of multiple sclerosis iron rim lesions: pathology and 7 T magnetic resonance imaging. Acta Neuropathol. 133, 25–42. doi: 10.1007/s00401-016-1636-z
Datta, G., Colasanti, A., Kalk, N., Owen, D., Scott, G., Rabiner, E. A., et al. (2017). 11C-PBR28 and 18F-PBR111 detect white matter inflammatory heterogeneity in multiple sclerosis. J. Nucl. Med. 58, 1477–1482. doi: 10.2967/jnumed.116.187161
Dehghani, M., Kunz, N., Lanz, B., Yoshihara, H. A. I., and Gruetter, R. (2017). Diffusion-weighted MRS of acetate in the rat brain. NMR Biomed 30:e3768. doi: 10.1002/nbm.3768
Di Biase, M. A., Zalesky, A., O’keefe, G., Laskaris, L., Baune, B. T., Weickert, C. S., et al. (2017). PET imaging of putative microglial activation in individuals at ultra-high risk for psychosis, recently diagnosed and chronically ill with schizophrenia. Transl. Psychiatry 7:e1225. doi: 10.1038/tp.2017.193
Ezura, M., Kikuchi, A., Ishiki, A., Okamura, N., Hasegawa, T., Harada, R., et al. (2019). Longitudinal changes in 18 F-THK5351 positron emission tomography in corticobasal syndrome. Eur. J. Neurol. 26, 1205–1211. doi: 10.1111/ene.13966
Focke, C., Blume, T., Zott, B., Shi, Y., Deussing, M., Peters, F., et al. (2019). Early and Longitudinal microglial activation but not amyloid accumulation predicts cognitive outcome in PS2APP mice. J. Nucl. Med. 60, 548–554. doi: 10.2967/jnumed.118.217703
Garden, G. A., and Campbell, B. M. (2016). Glial biomarkers in human central nervous system disease. Glia 64, 1755–1771. doi: 10.1002/glia.22998
Gifford, A. N., Makriyannis, A., Volkow, N. D., and Gatley, S. J. (2002). In vivo imaging of the brain cannabinoid receptor. Chem.Phys. Lipids 121, 65–72. doi: 10.1016/s0009-3084(02)00148-2
Gillen, K. M., Mubarak, M., Nguyen, T. D., and Pitt, D. (2018). Significance and in vivo detection of iron-laden microglia in white matter multiple sclerosis lesions. Front. Immunol. 9:255. doi: 10.3389/fimmu.2018.00255
Green, M., Hutchins, G., Fletcher, J., Territo, W., Polson, H., Trussell, H., et al. (2018). Distribution of the P2X7-receptor-targeted [11C]GSK1482160 radiopharmaceutical in normal human subjects. J. Nucl. Med. 59(Suppl. 1):1009.
Grimaldi, A. M., Forte, E., Infante, T., Cavaliere, C., Salvatore, M., Cademartiri, F., et al. (2019). Future perspectives of nanoparticle-based contrast agents for cardiac magnetic resonance in myocardial infarction. Nanomedicine 17, 329–341. doi: 10.1016/j.nano.2019.02.003
Gulyás, B., Pavlova, E., Kása, P., Gulya, K., Bakota, L., and Várszegi, S. (2011). Activated MAO-B in the brain of Alzheimer patients, demonstrated by [11C]-L-deprenyl using whole hemisphere autoradiography. Neurochem. Int. 58, 60–68. doi: 10.1016/j.neuint.2010.10.013
Hafizi, S., Da Silva, T., Gerritsen, C., Kiang, M., Bagby, R. M., Prce, I., et al. (2017). Imaging Microglial activation in individuals at clinical high risk for psychosis: an In Vivo PET Study with [18F]FEPPA. Neuropsychopharmacology 42, 2474–2481. doi: 10.1038/npp.2017.111
Hagens, M. H. J., Golla, S. S. V., Janssen, B., Vugts, D. J., Beaino, W., and Windhorst, A. D. (2019). The P2X7 receptor tracer [11C]SMW139 as an in vivo marker of neuroinflammation in multiple sclerosis: a first-in man study. Eur. J. Nucl. Med. Mol. Imaging 47, 379–389. doi: 10.1007/s00259-019-04550-x
Hagens, M. H. J., Golla, S. V., Wijburg, M. T., Yaqub, M., Heijtel, D., Steenwijk, M. D., et al. (2018). In vivo assessment of neuroinflammation in progressive multiple sclerosis: a proof of concept study with [18F]DPA714 PET. J. Neuroinflammation 15:314. doi: 10.1186/s12974-018-1352-9
Hametner, S., Dal Bianco, A., Trattnig, S., and Lassmann, H. (2018). Iron related changes in MS lesions and their validity to characterize MS lesion types and dynamics with Ultra-high field magnetic resonance imaging. Brain Pathol. 28, 743–749. doi: 10.1111/bpa.12643
Han, J., Liu, H., Liu, C., Jin, H., Perlmutter, J. S., Egan, T. M., et al. (2017). Pharmacologic characterizations of a P2X7 receptor-specific radioligand, [11C]GSK1482160 for neuroinflammatory response. Nucl. Med. Commun. 38, 372–382. doi: 10.1097/MNM.0000000000000660
Harada, R., Ishiki, A., Kai, H., Sato, N., Furukawa, K., Furumoto, S., et al. (2018). Correlations of 18F-THK5351 PET with Postmortem Burden of Tau and Astrogliosis in Alzheimer Disease. J. Nucl. Med. 59, 671–674. doi: 10.2967/jnumed.117.197426
Herranz, E., Louapre, C., Treaba, C. A., Govindarajan, S. T., Ouellette, R., Mangeat, G., et al. (2019). Profiles of cortical inflammation in multiple sclerosis by 11C-PBR28 MR-PET and 7 Tesla imaging. MultScler 1:1352458519867320. doi: 10.1177/1352458519867320
Herzog, H., Pietrzyk, U., Shah, N. J., and Ziemons, K. (2010). The current state, challenges and perspectives of MR-PET. NeuroImage 49, 2072–2082. doi: 10.1016/j.neuroimage.2009.10.036
Horti, A. G., Naik, R., Foss, C. A., Minn, I., Misheneva, V., Du, Y., et al. (2019). PET imaging of microglia by targeting macrophage colony-stimulating factor 1 receptor (CSF1R). Proc. Natl. Acad. Sci. U.S.A. 116, 1686–1691. doi: 10.1073/pnas.1812155116
Iaccarino, L., Moresco, R. M., Presotto, L., Bugiani, O., Iannaccone, S., Giaccone, G., et al. (2018). An In Vivo 11C-(R)-PK11195 PET and in vitro pathology study of microglia activation in creutzfeldt-jakob disease. Mol. Neurobiol. 55, 2856–2868. doi: 10.1007/s12035-017-0522-6
Ishibashi, K., Miura, Y., Hirata, K., Toyohara, J., and Ishii, K. (2019). 18F-THK5351 PET can identify astrogliosis in multiple sclerosis plaques. Clin. Nucl. Med. 2019:24. doi: 10.1097/RLU.0000000000002751
Ishiki, A., Harada, R., Kai, H., Sato, N., Totsune, T., Tomita, N., et al. (2018). Neuroimaging-pathological correlations of [18F]THK5351 PET in progressive supranuclear palsy. Acta Neuropathol. Commun. 6:53. doi: 10.1186/s40478-018-0556-7
Kang, Y., Schlyer, D., Kaunzner, U. W., Kuceyeski, A., Kothari, P. J., and Gauthier, S. A. (2018). Comparison of two different methods of image analysis for the assessment of microglial activation in patients with multiple sclerosis using (R)-[N-methyl-carbon-11]PK11195. PLoS One 13:e0201289. doi: 10.1371/journal.pone.0201289
Kaunzner, U. W., Kang, Y., Zhang, S., Morris, E., Yao, Y., Pandya, S., et al. (2019). Quantitative susceptibility mapping identifies inflammation in a subset of chronic multiple sclerosis lesions. Brain 142, 133–145. doi: 10.1093/brain/awy296
Keller, T., López-Picón, F. R., Krzyczmonik, A., Forsback, S., Kirjavainen, A. K., Takkinen, J. S., et al. (2018). [18F]F-DPA for the detection of activated microglia in a mouse model of Alzheimer’s disease. Nucl. Med. Biol. 67, 1–9. doi: 10.1016/j.nucmedbio.2018.09.001
Kirschbaum, K., Sonner, J. K., Zeller, M. W., Deumelandt, K., Bode, J., Sharma, R., et al. (2016). In vivo nanoparticle imaging of innate immune cells can serve as a marker of disease severity in a model of multiple sclerosis. Proc. Natl. Acad. Sci. U.S.A. 113, 13227–13232. doi: 10.1073/pnas.1609397113
Kobayashi, R., Hayashi, H., Kawakatsu, S., Ishiki, A., Okamura, N., Arai, H., et al. (2018). [18F]THK-5351 PET imaging in early-stage semantic variant primary progressive aphasia: a report of two cases and a literature review. BMC Neurol. 18:109. doi: 10.1186/s12883-018-1115-3
Kolb, H. C., Barret, O., Bhattacharya, A., Chen, G., Constantinescu, C., and Huang, C. (2019). Preclinical evaluation and nonhuman primate receptor occupancy study of 18F-JNJ-64413739, a PET radioligand for P2X7 receptors. J. Nucl. Med. 60, 1154–1159. doi: 10.2967/jnumed.118.212696
Kumar, J. S. D., Bai, B., Zanderigo, F., DeLorenzo, C., Prabhakaran, J., Parsey, R. V., et al. (2018). In vivo brain imaging, biodistribution, and radiation dosimetry estimation of [11C]Celecoxib, a COX-2 PET ligand, in nonhuman primates. Molecules 23:E1929. doi: 10.3390/molecules23081929
Lewis, C. M., Graves, S. A., Hernandez, R., Valdovinos, H. F., Barnhart, T. E., Cai, W., et al. (2015). 52Mn production for PET/MRI tracking of human stem cells expressing divalent metal transporter 1 (DMT1). Theranostics 5, 227–239. doi: 10.7150/thno.10185
Li, H., Sagar, A. P., and Kéri, S. (2018). Translocator protein (18kDa TSPO) binding, a marker of microglia, is reduced in major depression during cognitive-behavioral therapy. Prog. Neuropsychopharmacol. Biol. Psychiatry 83, 1–7. doi: 10.1016/j.pnpbp.2017.12.011
Luo, S., Kong, X., Wu, J. R., Wang, C. Y., Tian, Y., Zheng, G., et al. (2018). Neuroinflammation in acute hepatic encephalopathy rats: imaging and therapeutic effectiveness evaluation using 11C-PK11195 and 18F-DPA-714 micro-positron emission tomography. Metab. Brain Dis. 33, 1733–1742. doi: 10.1007/s11011-018-0282-7
Marchitelli, R., Aiello, M., Cachia, A., Quarantelli, M., Cavaliere, C., Postiglione, A., et al. (2018). Simultaneous resting- state FDG-PET/fMRI in Alzheimer disease: relationship between glucose metabolism and intrinsic activity. NeuroImage 176, 246–258. doi: 10.1016/j.neuroimage.2018.04.048
Mele, G., Cavaliere, C., Alfano, V., Orsini, M., Salvatore, M., and Aiello, M. (2019). Simultaneous EEG-fMRI for functional neurological assessment. Front. Neurol. 10:848. doi: 10.3389/fneur.2019.00848
Miller, S. J. (2018). Astrocyte heterogeneity in the adult central nervous system. Front. Cell Neurosci. 2018:15. doi: 10.3389/fncel.2018.00401
Miyajima, N., Ito, M., Rokugawa, T., Iimori, H., Momosaki, S., Omachi, S., et al. (2018). Detection of neuroinflammation before selective neuronal loss appearance after mild focal ischemia using [18F]DPA-714 imaging. EJNMMI Res. 8:43. doi: 10.1186/s13550-018-0400-x
Monai, H., and Hirase, H. (2018). Astrocytes as a target of transcranial direct current stimulation (tDCS) to treat depression. Neurosci. Res. 126, 15–21. doi: 10.1016/j.neures.2017.08.012
Monti, S., Cavaliere, C., Covello, M., Nicolai, E., Salvatore, M., and Aiello, M. (2017). An evaluation of the benefits of simultaneous acquisition on PET/MR coregistration in head/neck imaging. J. Healthcare Eng. 2017:2634389. doi: 10.1155/2017/2634389
Nack, A., Brendel, M., Nedelcu, J., Daerr, M., Nyamoya, S., Beyer, C., et al. (2019). Expression of translocator protein and [18F]-ge180 ligand uptake in multiple sclerosis animal models. Cells 8, E94. doi: 10.3390/cells8020094
Narayanaswami, V., Dahl, K., Bernard-Gauthier, V., Josephson, L., Cumming, P., and Vasdev, N. (2018). Emerging PET radiotracers and targets for imaging of neuroinflammation in neurodegenerative diseases: outlook beyond TSPO. Mol. Imaging 17:1536012118792317. doi: 10.1177/1536012118792317
Ni, R., Mu, L., and Ametamey, S. (2019). Positron emission tomography of type 2 cannabinoid receptors for detecting inflammation in the central nervous system. Acta Pharmacol. Sin. 40, 351–357. doi: 10.1038/s41401-018-0035-5
Ottoy, J., De Picker, L., Verhaeghe, J., Deleye, S., Wyffels, L., Kosten, L., et al. (2018). 18F-PBR111 PET imaging in healthy controls and schizophrenia: test-retest reproducibility and quantification of neuroinflammation. J. Nucl. Med. 59, 1267–1274. doi: 10.2967/jnumed.117.203315
Papa, M., De Luca, C., Petta, F., Alberghina, L., and Cirillo, G. (2014). Astrocyte-neuron interplay in maladaptive plasticity. Neurosci. Biobehav. Rev. 42, 35–54. doi: 10.1016/j.neubiorev.2014.01.010
Pérez-Alvarez, A., and Araque, A. (2013). Astrocyte-neuron interaction at tripartite synapses. Curr. Drug Targets 14, 1220–1224. doi: 10.2174/13894501113149990203
Poupot, R., Bergozza, D., and Fruchon, S. (2018). Nanoparticle-based strategies to treat Neuro-inflammation. Materials 11:270. doi: 10.3390/ma11020270
Pulgar, V. M. (2019). Transcytosis to cross the blood brain barrier, new advancements and challenges. Front. Neurosci. 12, 1019.
Ratai, E. M., Alshikho, M. J., Zürcher, N. R., Loggia, M. L., Cebulla, C. L., Cernasov, P., et al. (2018). Integrated imaging of [11C]-PBR28 PET, MR diffusion and magnetic resonance spectroscopy 1H-MRS in amyotrophic lateral sclerosis. Neuroimage Clin. 20, 357–364. doi: 10.1016/j.nicl.2018.08.007
Reischauer, C., Gutzeit, A., Neuwirth, C., Fuchs, A., Sartoretti-Schefer, S., Weber, M., et al. (2018). In-vivo evaluation of neuronal and glial changes in amyotrophic lateral sclerosis with diffusion tensor spectroscopy. Neuroimage Clin. 20, 993–1000. doi: 10.1016/j.nicl.2018.10.001
Selvaraj, S., Bloomfield, P. S., Cao, B., Veronese, M., Turkheimer, F., and Howes, O. D. (2018). Brain TSPO imaging and gray matter volume in schizophrenia patients and in people at ultra high risk of psychosis: an [11C]PBR28 study. Schizophr Res. 195, 206–214. doi: 10.1016/j.schres.2017.08.063
Shen, Z., Bao, X., and Wang, R. (2018). Clinical PET imaging of microglial activation: implications for microglial therapeutics in Alzheimer’s Disease. Front. Aging Neurosci. 10:314. doi: 10.3389/fnagi.2018.00314
Shigemoto, Y., Sone, D., Maikusa, N., Okamura, O., Furumoto, S., Kudo, Y., et al. (2018). Association of deposition of tau and amyloid-β proteins with structural connectivity changes in cognitively normal older adults and alzheimer’s disease spectrum patients. Brain Behav. 8:e01145. doi: 10.1002/brb3.1145
Shukuri, M., Takashima-Hirano, M., Tokuda, K., Takashima, T., Matsumura, K., Inoue, O., et al. (2011). In vivo expression of cyclooxygenase-1 in activated microglia and macrophages during neuroinflammation visualized by PET with 11C-ketoprofen methyl ester. J. Nucl. Med. 52, 1094–1101. doi: 10.2967/jnumed.110.084046
Singhal, T., O’Connor, K., Dubey, S., Belanger, A. P., Hurwitz, S., Chu, R., et al. (2018). 18F-PBR06 Versus 11C-PBR28 PET for assessing white matter translocator protein binding in multiple sclerosis. Clin. Nucl. Med. 43, e289–e295. doi: 10.1097/RLU.0000000000002179
Takata, N., Sugiura, Y., Yoshida, K., Koizumi, M., Hiroshi, N., Honda, K., et al. (2018). Optogenetic astrocyte activation evokes BOLD fMRI response with oxygen consumption without neuronal activity modulation. Glia. 66, 2013–2023. doi: 10.1002/glia.23454
Tang, T., Valenzuela, A., Petit, F., Chow, S., Leung, K., Gorin, F., et al. (2018). In Vivo MRI of functionalized iron oxide nanoparticles for brain inflammation. Contrast Media Mol. Imaging 25:3476476. doi: 10.1155/2018/3476476
Unterrainer, M., Mahler, C., Vomacka, L., Lindner, S., Havla, J., Brendel, M., et al. (2018). TSPO PET with [18F]GE-180 sensitively detects focal neuroinflammation in patients with relapsing-remitting multiple sclerosis. Eur. J. Nucl. Med. Mol. Imaging 45, 1423–1431. doi: 10.1007/s00259-018-3974-7
Uppal, R., Catana, C., Ay, I., Benner, T., Sorensen, A. G., and Caravan, P. (2011). Bimodal thrombus imaging: simultaneous PET/MR imaging with a fibrin-targeted dual PET/MR probe—Feasibility study in rat model. Radiology 258, 812–820. doi: 10.1148/radiol.10100881
van Weehaeghe, D., Koole, M., Schmidt, M. E., Deman, S., Jacobs, A. H., Souche, E., et al. (2019). [11C]JNJ54173717, a novel P2X7 receptor radioligand as marker for neuroinflammation: human biodistribution, dosimetry, brain kinetic modelling and quantification of brain P2X7 receptors in patients with Parkinson’s disease and healthy volunteers. Eur. J. Nucl. Med. Mol. Imaging 46, 2051–2064. doi: 10.1007/s00259-019-04369-6
Vecchione, D., Aiello, M., Cavaliere, C., Nicolai, E., Netti, P. A., and Torino, E. (2017). Hybrid core shell nanoparticles entrapping Gd-DTPA and 18F-FDG for simultaneous PET/MRI acquisitions. Nanomedicine 12, 2223–2231. doi: 10.2217/nnm-2017-0110
Verkhratsky, A., Parpura, V., Pekna, M., Pekny, M., and Sofroniew, M. (2014). Glia in the pathogenesis of neurodegenerative diseases. Biochem. Soc. Trans. 42, 1291–1301. doi: 10.1042/BST20140107
Wang, M., He, Y., Sejnowski, T. J., and Yu, X. (2018). Brain-state dependent astrocytic Ca2+ signals are coupled to both positive and negative BOLD-fMRI signals. Proc. Natl. Acad. Sci. U.S.A. 115, E1647–E1656. doi: 10.1073/pnas.1711692115
Yamagishi, S., Iga, Y., Nakamura, M., Takizawa, C., Fukumoto, D., and Kakiuchi, T. (2019). Upregulation of cannabinoid receptor type 2, but not TSPO, in senescence-accelerated neuroinflammation in mice: a positron emission tomography study. J. Neuroinflammation.10 16:208. doi: 10.1186/s12974-019-1604-3
Yi, S. Y., Barnett, B. R., Torres-Velázquez, M., Zhang, Y., Hurley, S. A., Rowley, P. A., et al. (2019). Detecting microglial density with quantitative multi-compartment diffusion MRI. Front. Neurosci. 13:81. doi: 10.3389/fnins.2019.00081
Yokoi, T., Watanabe, H., Yamaguchi, H., Bagarinao, E., Masuda, M., Imai, K., et al. (2018). Involvement of the precuneus/posterior cingulate cortex is significant for the development of Alzheimer’s Disease: a PET (THK5351, PiB) and resting fMRI Study. Front. Aging Neurosci. 10:304. doi: 10.3389/fnagi.2018.00304
Zaidi, H., and Guerra, A. D. (2011). An outlook on future design of hybrid PET/MRI systems. Med. Phys. 38, 5667–5689. doi: 10.1118/1.3633909
Zimmer, E. R., Parent, M. J., Souza, D. G., Leuzy, A., Lecrux, C., Kim, H. I., et al. (2017). [18F]FDG PET signal is driven by astroglial glutamate transport. Nat. Neurosci. 20, 393–395. doi: 10.1038/nn.4492
Keywords: PET, MRI, gliosis, microglia, astrocytes, hybrid imaging, dual PET/MR agents, molecular imaging
Citation: Cavaliere C, Tramontano L, Fiorenza D, Alfano V, Aiello M and Salvatore M (2020) Gliosis and Neurodegenerative Diseases: The Role of PET and MR Imaging. Front. Cell. Neurosci. 14:75. doi: 10.3389/fncel.2020.00075
Received: 23 September 2019; Accepted: 13 March 2020;
Published: 02 April 2020.
Edited by:
Giovanni Cirillo, Universitá degli Studi della Campania Luigi Vanvitelli, ItalyReviewed by:
Jorge Matias-Guiu, Complutense University of Madrid, SpainMathias Hoehn, Max-Planck-Gesellschaft (MPG), Germany
Copyright © 2020 Cavaliere, Tramontano, Fiorenza, Alfano, Aiello and Salvatore. This is an open-access article distributed under the terms of the Creative Commons Attribution License (CC BY). The use, distribution or reproduction in other forums is permitted, provided the original author(s) and the copyright owner(s) are credited and that the original publication in this journal is cited, in accordance with accepted academic practice. No use, distribution or reproduction is permitted which does not comply with these terms.
*Correspondence: Carlo Cavaliere, Y2NhdmFsaWVyZUBzZG4tbmFwb2xpLml0; Y2FybG9jYXZhbGllcmUxOTgzQHlhaG9vLml0
†These authors have contributed equally to this work