- 1Department of Surgical Oncology, The Second Affiliated Hospital, School of Medicine, Zhejiang University, Hangzhou, China
- 2Department of Neurosurgery, The Second Affiliated Hospital, School of Medicine, Zhejiang University, Hangzhou, China
Traumatic brain injury (TBI) is an important cause of human mortality and morbidity, which can induce serious neurological damage. At present, clinical treatments for neurological dysfunction after TBI include hyperbaric oxygen, brain stimulation and behavioral therapy, but the therapeutic effect is not satisfactory. Recent studies have found that exogenous stem cells can migrate to damaged brain tissue, then participate in the repair of damaged brain tissue by further differentiation to replace damaged cells, while releasing anti-inflammatory factors and growth factors, thereby significantly improving neurological function. This article will mainly review the effects, deficiencies and related mechanisms of different types of stem cells in TBI.
Introduction
Traumatic brain injury (TBI) is a common and frequently occurring disease. According to the World Health Organization, TBI will become the main cause of human mortality and morbidity after 2020, which brings a heavy economic burden to patients and families (Maas et al., 2017). TBI is a disease which causes the destruction of normal brain function, and leads to serious physical, cognitive and emotional disorders. The pathophysiology of TBI mainly includes the break of the blood brain barrier (BBB), extensive neuroinflammation, diffuse axonal injury, and neurodegenerative lesions (Xiong et al., 2008). The pathological changes of brain injury are mainly the loss of normal tissue structure, destruction of neuronal cells and internal environment disturbance, among which neuronal cells injury is the key point. There is no effective drug treatment so far. At present, the main treatment includ hyperbaric oxygen, non-invasive brain stimulation, task-oriented functional electrical stimulation and behavioral therapy (Dang et al., 2017).
In recent years, study (Cox, 2018) have found that a variety of stem cells can treat neurological impairment after TBI, including mesenchymal stem cells (MSCs), neural stem cells (NSCs), multipotent adult progenitor cells (MAPCs), and endothelial progenitor cells (EPCs) (Table 1). In this article, we review the literature on the role, progress, major deficiencies and related mechanisms of different types of stem cell therapy in TBI.
Therapeutic Effect of Different Types of Stem Cells
Mesenchymal Stem Cells
Mesenchymal stem cells are heterogeneous multipotent adult cells that can be isolated from bone marrow, and perivascular tissues (da Silva Meirelles et al., 2006; Wong et al., 2015), with the ability of directional differentiation into mesenchymal and non-mesenchymal tissues, including nerve cells (Sanchez-Ramos et al., 2000). MSCs play an important role in tissue regeneration, which can promote the regeneration of damaged tissues by inhibiting inflammation, secreting trophic factors, and recruiting local progenitor cells to replace lost cells. Study (Adibi et al., 2016) has shown that MSCs can down-regulate the expression of inflammatory proteins and accelerate the repair of intracranial aneurysms. Moreover, study (Wang et al., 2013) has found that in addition to its secretory ability, MSCs can selectively migrate to the injured brain tissue of the TBI rat, and then differentiate into neurons and astrocytes to repair damaged brain tissue, thereby improving the motion function after TBI. Zhang et al. (2013) used the TBI rat model to study the anti-inflammatory and immunomodulatory properties of MSCs. Compared with the control group, the neurological function of TBI animals in the MSCs treatment group was significantly improved from 3 to 28 days, with the brain water content decreased significantly. In addition, they found that MSCs treatment could reduce the number of microglia, macrophages, neutrophils, CD3+ lymphocytes, apoptotic cells and pro-inflammatory cytokines in the injured cortex, thereby inhibiting the inflammatory response after TBI (Figure 1) (Zhang et al., 2013). Similarly, Guo S. et al. (2017) studied the treatment effect by injecting bone marrow-derived MSCs into TBI mice. Compared with the control group, MSCs treatment could promote the recovery of neurological function in TBI mice, improve learning and memory ability, and reduce neuronal apoptosis. The mechanism may be that MSCs promote the expression of VEGF and Ang-1, and microangiogenesis. Despite simply transfecting MSCs into the body, injecting MSCs with proliferation and anti-oxidative effects enhanced through overexpression of specific gene in vitro is also an efficient treatment for TBI. Furthermore, superoxide dismutase 2 (SOD2) plays a critical role against oxidative stress, Shi et al. (2018) found that SOD2 could reduce the neuroinflammatory response and maintain the integrity of BBB in TBI mice; and by intravenously transfecting MSCs, in which the SOD2 gene is overexpressed, into TBI mice, the neurological function could be ultimately improved. Although basic research (Djouad et al., 2003) has confirmed that MSCs can improve the neurological function and prognosis of TBI, MSCs using in clinical practice is still difficult, as treatment with MSCs has the potential to promote brain tumor growth. To date, there have been two clinical studies on the efficacy and safety of MSCs in the treatment of TBI. In one of the clinical studies (Zhang et al., 2008), seven patients were transplanted with MSCs during craniocerebral surgery. The surgeons injected MSCs directly to the injured brain tissue, and venously injected 108 to 1010 MSCs into TBI patients; neurological function improved significantly at 6 months follow-up, and no evidence of toxicity was found. However, the sample size of this study was too small and there was no control group, and the intervals between MCSs transplantation and injury of direct infusion and intravenous infusion were different, while the interval has an important correlation with homing effect. Moreover, the numbers of MSCs injected into each patient were very different, without regularity or explanation. No quantitative or qualitative analysis of the aggregation effect of MSCs on the injury site were recorded. The second one was Phase I clinical study (Wang et al., 2017) including 10 patients with severe brain trauma. MSCs were injected intravenously or intrathecally. The improvement of neurological function was mainly determined by NIHSS scale, GCS score and GOS score. After the cells were transplanted, the serum nerve growth factor and brain-derived neurotrophic factor were significantly increased, and during the 6 months follow-up, the patients’ neurological function was improved, and no death or adverse events occurred. However, the limitation of, this clinical study is similar to the clinical study mentioned above as the sample is small and there is no control group, and is not as comprehensive and rigorous in the evaluation of the adverse effect. Although MSCs have achieved good therapeutic effects in both basic and clinical research, their ability to survive for long periods on the TBI model and the possible presence of immunological rejection are important issues that warrant further investigation (Gold et al., 2013). Currently, cell replacement, long-term transplantation-mediated nutritional support or immune regulation are the main mechanisms of the effect of MSCs. However, low cell viability, immune rejection, and inability to quantify cell viability may affect the accurate assessment of the ability of MSCs for long-term repair and recovery of neurological function (Haus et al., 2016).
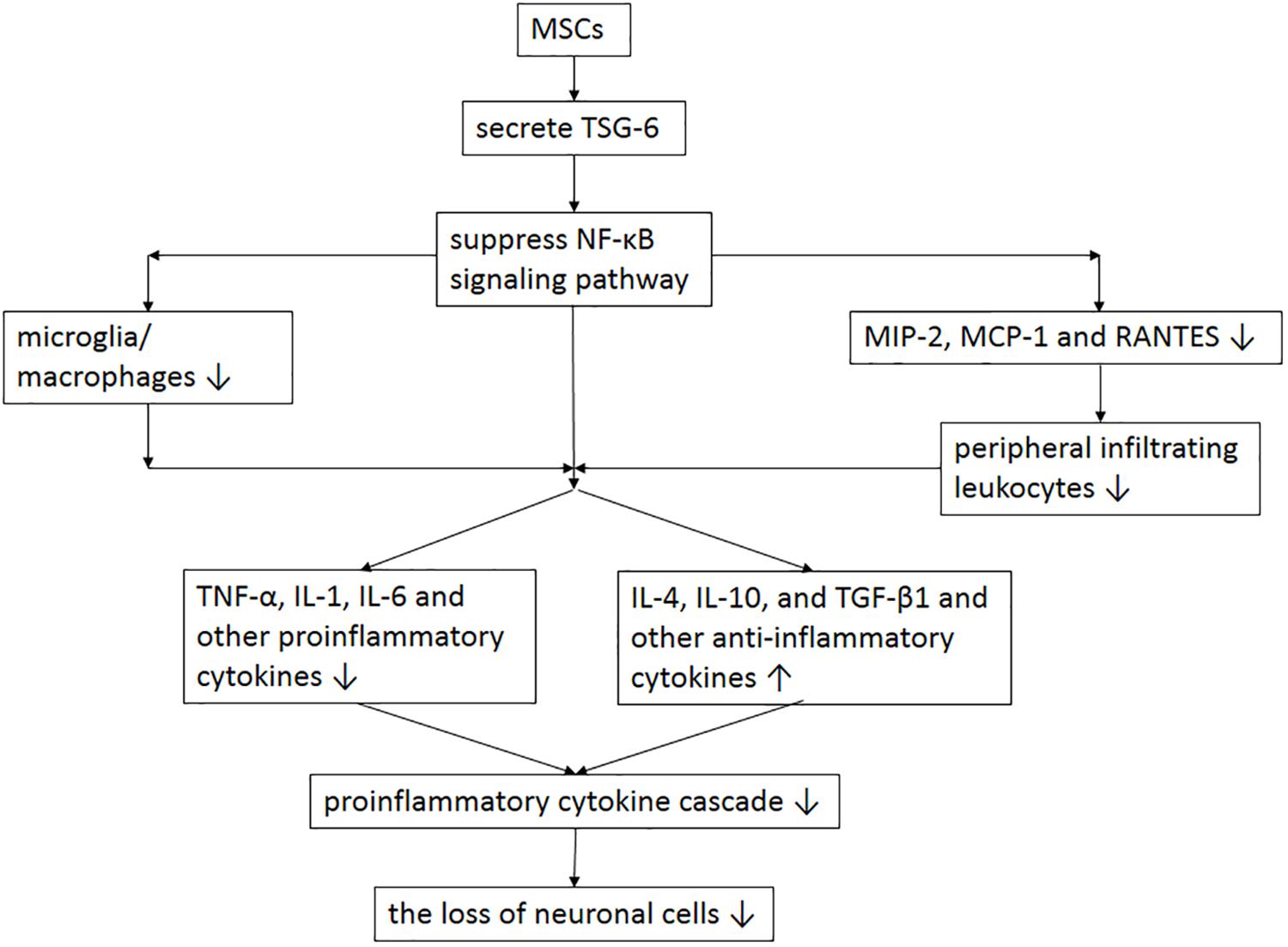
Figure 1. The potential mechanism of MSCs involved in anti-inflammatory and immunomodulatory after TBI. MSC transplantation enhances the expression of TSG-6 and then suppresses the activation of NF-κB signaling pathway, resulting in the reduction of microglia, macrophages, peripheral infiltrating leukocytes and proinflammatory cytokines and the increase of anti-inflammatory cytokines, which thereby alleviate the initiation of proinflammatory cytokine cascade and the loss of neuronal cells. MSCs = mesenchymal stem cells; TBI = traumatic brain injury; TNF = tumor necrosis factor; TSG-6 = TNF-α stimulated gene/protein 6; NF-κB = nuclear factor-κB; IL = interleukin; MCP = macrophage chemotactic protein; MIP = macrophage inflammatory protein; TGF = transforming growth factor; RANTES = regulated on activation in normal T-cell expressed and secreted.
In vitro exosomes are small vesicles containing various RNAs (mRNAs, miRNAs, etc.) and proteins with a diameter of 30–100 nm, which can be released from multiple cells under normal or pathological conditions (Barteneva et al., 2013). Exosomes have important clinical value, as their surface markers and molecules can be used as potential diagnostic markers for some diseases (Escudero et al., 2016), which not only have low immunity and long half-life in the peripheral circulation, but also have the ability to cross the BBB (Escudero et al., 2016). Compared with other types of cells, MSCs can produce a large number of exosomes, and the extracted exosomes are not significantly different from other cell-derived exosomes in morphological characteristics, isolation and storage methods (Yeo et al., 2013). In addition to transplantation of MSCs into TBI animal models by different routes, Zhang et al. (2015) extracted exosomes from MSCs and then injected exosomal proteins through the tail vein to the TBI animal. Results showed that neurological function in the TBI group could be significantly improved, and the mechanism might be related to the promoting endogenous angiogenesis and neurogenesis, as well as reducing inflammatory response post-TBI. Then, by comparing the difference in the efficacy of exosome cultured in two-dimensional and three-dimensional collagen scaffolds, they found that exosomes cultured in three-dimensional collagen had better effects in improving neurological recovery and spatial learning ability after TBI (Zhang et al., 2017). Similarly, a recent study (Yeo et al., 2013) found that treated with exosomes from MSCs could alleviate the damage of cognitive function after TBI. Xin et al. (2013) found that exosomes could significantly promote the recovery of neurological function in rat stroke models. Further studies showed that compared with the control group, exosomes can enhance axonal reconstruction, neurogenesis, and angiogenesis. Compared with transplantation of exogenous stem cells, MSCs-derived exosomal transplantation has several advantages in repairing damaged brain tissue, for example:no ethical problems, less invasiveness, lower or no immunogenicity, and low or no tumorigenicity (Xiong et al., 2017). So far, there is no clinical study on the treatment of TBI using MSCs-derived exosomes. The underlying mechanism of TBI treatment of exosomes is still not fully understood, so the mechanism of exosome repairing damaged brain tissue after TBI need further study. It is a necessary step in its application to the clinic.
Neural Stem Cells
Neural stem cells are self-renewing stem cells that can be further differentiated into neurons, glial cells and oligodendrocytes. Long-term survival of human cells transplanted into TBI animal models is difficult to achieve due to host immune rejection, and Haus et al. (2016) produced a new immunodeficient TBI rat model and transplanted human NSCs to the rat model, the recovery of cognitive function after brain injury was observed for a long time (greater than or equal to 2 months), and 9 to 25% of NSCs transplanted into the TBI model survived for at least 5 months, and differentiated into mature neurons, astrocytes, and oligodendrocytes; this study suggests that transplanted human NSCs may be an effective, long-term treatment for neurological recovery after brain injury. In addition, transfection of genes that promote growth into NSCs could enhance the proliferation, differentiation and other functions of NSCs, and then transplantation of these NSCs into the TBI model is an effective method for the treatment of TBI. In a study by Philips et al. (2001), injection of NSCs transfected with a virus carrying a nerve growth factor gene into the TBI model significantly increased pyramidal cell survival in the hippocampus, and enhanced the ability of cognitive, learning, and motor function. At present, NSCs are transplanted into animal models mainly through stereotactic injection and lateral ventricle injection, and NSCs injected through the lateral ventricle have higher survival rates in vivo (Wallenquist et al., 2009). Despite the NSCs transplantation method, the time of transplantation is also a key factor affecting the efficacy of NSCs treatment of TBI. The effect of injecting NSCs 2 days and 1 week after TBI is significantly better than that after two weeks, and injecting NSCs only 1 month after TBI has no significant effect for the recovery of motor and cognitive function (Zhang et al., 2005). At present, the application of NSCs to clinical trials is mainly limited by the difficulty in large-scale cultivation and production of NSCs. Recently, there is a clinical study on the treatment of chronic cervical spinal cord injury using human NSCs (Ghobrial et al., 2017). Although the study found that neurological function was generally restored after treatment with NSCs, it was necessary to increase the sample and add control group to further clarify its efficacy.
Multipotent Adult Progenitor Cells
Multipotent adult progenitor cells were first reported in 2002, and received attention because of their characteristics of differentiation into mesenchymal cells, visceral mesoderm, neuroectoderm and endoderm (Reis et al., 2017). Their differentiation potential is not sustained (Reis et al., 2017). MAPCs are a distinct group of cells that differ from MSCs because of the low expression of MHC class I surface proteins and their ability to differentiate into endothelial cells (Roobrouck et al., 2011). To date, only one research team has used SD rats (Walker et al., 2010; Bedi et al., 2013a) and mice (Walker et al., 2012) to study human MAPCs for TBI. They injected 106 MAPCs into the TBI animals at 2 and 24 h after TBI. It was found that MAPCs could improve their spatial learning, information retention, memory retrieval and dyskinesia after 120 days of brain injury, and could maintain the integrity of the BBB in the acute phase of TBI (Bedi et al., 2013b). The main mechanism may be counteracting the inflammatory response caused by injury by up-regulating the expression of anti-inflammatory response factors (Bedi et al., 2013a).
Induced Pluripotent Stem Cells
Induced pluripotent stem cells (iPSCs) were first reported in 2006. Two Japanese scientists used viral vectors to transfer the transcription factors Oct3/4, Sox2, c-Myc and Klf4 into differentiated somatic cells and reprogram the cells into a class of cells resembling embryonic stem cells (Takahashi and Yamanaka, 2006). Such cells can be extracted from patients and then reprogrammed in vitro to generate iPSCs, which are then returned to the patient, thereby avoiding ethical problems and immune rejection. They have the ability to self-renew and differentiate into various types of cells, so have promising clinical application prospects. Recently, Cary et al. (2015) obtained iPSCs by taking a dura mater from a patient with severe cognitive impairment after TBI, and then extracted fibroblasts from the tissue, finally iPSCs can be obtained by transfection of Sendai virus carrying non-conformity. For patients with brain trauma requiring surgery, taking the dura mater for culture is a good way to obtain iPSCs. Kobayashi et al. (2012) used the spinal cord injury marmoset model, the authors found that transplanted human iPSCs could survive in animals and differentiate into three neural cell lines, which promoted axonal regeneration and prevented brain tissue damage. Gao et al. (2016) used retrovirus to reprogram four transcription factors Oct4, Sox2, Klf4 and c-Myc and reactive glial cells into iPSCs, and found that such iPSCs could differentiate into a large number of NSCs and could be further differentiated into neurons and glial cells, repairing TBI damaged brain tissue. Lyu et al. (2017) used the TBI model and found that iPSCs-derived A2B5+ cells could effectively improve neurological dysfunction after transplantation into the brain injury zone. The mechanism is mainly related to the change of lncRNA and mRNA expression. Similarly, Wei et al. (2016) and Dunkerson et al. (2014) used animal TBI models and found that iPSCs transplantation could significantly improve cognitive and motor function after TBI. In addition to promoting the recovery of neurological function after trauma, iPSCs is also an important choice for the treatment of Huntington’s disease. An et al. (2012) used the human Huntington’s disease cell model to study and extracted fibroblast reprogramming from Huntington’s disease patients to finally obtain iPSCs, and found that iPSCs could change the phenotype of Huntington’s disease cell model, and could further differentiate into striatum neurons. Although iPSCs have many advantages, there are still many shortcomings (Dekmak et al., 2018). First, since this cell is reprogrammed by infection with a virus, it has certain tumorigenicity, and its efficiency from reprogramming by somatic cells is low. Furthermore, as such cells generated by reprogramming have an unknown genetic and epigenetic background, the safety issues need to be carefully evaluated before using iPSCs in the clinic.
Endothelial Progenitor Cells
Endothelial progenitor cells are precursor cells of vascular endothelial cells, mainly in the bone marrow. They are progenitor cells with migratory properties that can be further differentiated into vascular endothelial cells. They can participate in embryonic angiogenesis and postnatal angiogenesis. Under the stimulation of physiological or pathological factors, EPCs can be mobilized from the bone marrow into peripheral blood by certain chemokines and adhesion molecules, spontaneously recruited to the site of endothelial injury, and participate in endothelial repair (Malinovskaya et al., 2016), especially in the brain after trauma (Guo et al., 2009). Changes in the number of peripheral blood EPCs can be used as markers of whether the BBB is destroyed (Huang S. H. et al., 2013). In addition, EPCs were significantly elevated within 24 h after TBI, whereas patients with relatively low levels of EPCs in peripheral blood could suggest a poor prognosis (Lin et al., 2017). BBB is mainly composed of microvascular endothelial cells. Boyer-Di Ponio et al. (2014) found that certain conditions can induce EPCs to differentiate into BBB. Endothelial colony-forming cells (ECFCs), also known as “late endothelial progenitor cells” or “endothelial outward-growth cells,” are a subtype of EPCs, first reported by Lin et al. (2000). In the brain injury model, ECFCs injected into the umbilical cord blood through the ventricle were found to be able to home to the brain injury area to participate in the repair of BBB and enhance neovascularization (Huang X. T. et al., 2013). Activation of the Notch pathway enhances the migration and lumen formation of EPCs, and activation of the Notch pathway of EPCs in the TBI animal model promotes the repair of damaged blood vessels and brain tissue (Ran et al., 2015). In order to track the distribution of EPCs in injured brain tissue and to clarify whether EPCs actually migrated to damaged brain tissue for repair, some scholars used GFP and BrdU double-labeled EPCs, find that EPCs can home to damaged brain tissue after intravenous injection, and promote hippocampal neurogenesis and angiogenesis, ultimately improving neurological function after TBI (Guo X. B. et al., 2017). Xue et al. (2010) studied EPCs isolated from adipose tissue and found that EPCs can accumulate in damaged brain tissue and participate in the capillary formation, reduce astrocyte proliferation and inflammation. Similarly, Chen et al. (2013) injected SPIO-labeled EPCs into the TBI model 6 and 12 h after TBI, respectively, and found that after 1 week, the cerebral blood perfusion recovered and the microvessels increased significantly in the damaged brain tissue area. Because the number of EPCs in peripheral blood is extremely small, transplantation of EPCs in vitro may be an effective method to promote neurogenesis and neurological recovery in patients with TBI. In addition, exogenous drugs enhance the migration ability and lumen formation of peripheral blood EPCs, can promote the recovery of neurological function after TBI, such as erythropoietin (Wang et al., 2015) and progesterone (Yu et al., 2016). The reduction of white matter after TBI is an important indicator of survival and prognosis of patients (Carmeliet and Tessier-Lavigne, 2005). Blood vessels provide structural support for the growth and development of axons, as well as transporting oxygen and removing metabolic waste. In view of the important role of vascular cells in axonal development and homeostasis, some scholars suggested that EPCs played a role in mediating local angiogenesis in the brain, and used TBI animal models to confirm that EPCs could maintain the integrity of white matter after TBI and reduce capillary damage (Park et al., 2014). At present, although a large number of basic experiments have confirmed that EPCs transplantation therapy can significantly improve neurological function after TBI, its efficacy and safety remain to be further studied.
Conclusion and Prospects
Although there is a large volume of basic research into TBI, especially on the complexity of pathophysiology and the application of stem cell therapy, there are still many problems that need to be solved, to determine the best method for brain function recovery. Although there have been clinical studies on stem cell treatment of TBI, and all have achieved good therapeutic results, the sample size is not large enough, and there is no control group. Therefore, all studies and interventions that may affect the efficacy of TBI treatment require multi-center long-term follow-up and randomized prospective trials, which will have a huge impact on our decision to develop appropriate treatment options for different TBI populations. Although a large number of basic studies have confirmed that stem cells have good effect in the craniocerebral injury, the safety of stem cells, the route of injection, the time of injection and the specific mechanism are all factors that affect the clinical application of stem cells., and are the important research point in the future study.
Author Contributions
YZ, AS, WX, and HW reviewed the literature and drafted the manuscript. AS and YD finalized the manuscript and provided suggestions to improve it. YZ and AS critically revised the texts and figures. All authors participated in the designing of the concept of the manuscript, and read and approved the final manuscript.
Funding
This work was funded by China Postdoctoral Science Foundation (2017M612010) and National Natural Science Foundation of China (81701144).
Conflict of Interest Statement
The authors declare that the research was conducted in the absence of any commercial or financial relationships that could be construed as a potential conflict of interest.
References
Adibi, A., Sen, A., and Mitha, A. P. (2016). Cell therapy for intracranial aneurysms: a review. World Neurosurg. 86, 390–398. doi: 10.1016/j.wneu.2015.10.082
An, M. C., Zhang, N., Scott, G., Montoro, D., Wittkop, T., Mooney, S., et al. (2012). Genetic correction of Huntington’s disease phenotypes in induced pluripotent stem cells. Cell Stem Cell 11, 253–263. doi: 10.1016/j.stem.2012.04.026
Barteneva, N. S., Maltsev, N., and Vorobjev, I. A. (2013). Microvesicles and intercellular communication in the context of parasitism. Front. Cell Infect. Microbiol. 3:49. doi: 10.3389/fcimb.2013.00049
Bedi, S. S., Hetz, R., Thomas, C., Smith, P., Olsen, A. B., Williams, S., et al. (2013a). Intravenous multipotent adult progenitor cell therapy attenuates activated microglial/macrophage response and improves spatial learning after traumatic brain injury. Stem Cells Transl. Med. 2, 953–960. doi: 10.5966/sctm.2013-0100
Bedi, S. S., Walker, P. A., Shah, S. K., Jimenez, F., Thomas, C. P., Smith, P., et al. (2013b). Autologous bone marrow mononuclear cells therapy attenuates activated microglial/macrophage response and improves spatial learning after traumatic brain injury. J. Trauma Acute Care Surg. 75, 410–416. doi: 10.1097/TA.0b013e31829617c6
Boyer-Di Ponio, J., El-Ayoubi, F., Glacial, F., Ganeshamoorthy, K., Driancourt, C., Godet, M., et al. (2014). Instruction of circulating endothelial progenitors in vitro towards specialized bloodbrain barrier and arterial phenotypes. PLoS One 9:e84179. doi: 10.1371/journal.pone.0084179
Carmeliet, P., and Tessier-Lavigne, M. (2005). Common mechanisms of nerve and blood vessel wiring. Nature 436, 193–200. doi: 10.1038/nature03875
Cary, W. A., Hori, C. N., Pham, M. T., Nacey, C. A., McGee, J. L., Hamou, M., et al. (2015). Efficient generation of induced pluripotent stem and neural progenitor cells from acutely harvested dura mater obtained during ventriculoperitoneal shunt surgery. World Neurosurg. 84, 1256–1266. doi: 10.1016/j.wneu.2015.05.076
Chen, X., Yin, J., Wu, X., Li, R., Fang, J., Chen, R., et al. (2013). Effects of magnetically labeled exogenous endothelial progenitor cells on cerebral blood perfusion and microvasculature alterations after traumatic brain injury in rat model. Acta Radiol. 54, 313–323. doi: 10.1258/ar.2012.120605
Cox, C. S. Jr. (2018). Cellular therapy for traumatic neurological injury. Pediatr. Res. 83, 325–332. doi: 10.1038/pr.2017.253
da Silva Meirelles, L., Chagastelles, P. C., and Nardi, N. B. (2006). Mesenchymal stem cells reside in virtually all post-natal organs and tissues. J. Cell Sci. 119(Pt 11), 2204–2213. doi: 10.1242/jcs.02932
Dang, B., Chen, W., He, W., and Chen, G. (2017). Rehabilitation treatment and progress of traumatic brain injury dysfunction. Neural Plast. 2017:1582182. doi: 10.1155/2017/1582182
Dekmak, A., Mantash, S., Shaito, A., Toutonji, A., Ramadan, N., Ghazale, H., et al. (2018). Stem cells and combination therapy for the treatment of traumatic brain injury. Behav. Brain Res. 340, 49–62. doi: 10.1016/j.bbr.2016.12.039
Djouad, F., Plence, P., Bony, C., Tropel, P., Apparailly, F., Sany, J., et al. (2003). Immunosuppressive effect of mesenchymal stem cells favors tumor growth in allogeneic animals. Blood 102, 3837–3844. doi: 10.1182/blood-2003-04-1193
Dunkerson, J., Moritz, K. E., Young, J., Pionk, T., Fink, K., Rossignol, J., et al. (2014). Combining enriched environment and induced pluripotent stem cell therapy results in improved cognitive and motor function following traumatic brain injury. Restor Neurol. Neurosci. 32, 675–687. doi: 10.3233/RNN-140408
Escudero, C. A., Herlitz, K., Troncoso, F., Acurio, J., Aguayo, C., Roberts, J. M., et al. (2016). Role of extracellular vesicles and microRNAs on dysfunctional angiogenesis during preeclamptic pregnancies. Front. Physiol. 7:98. doi: 10.3389/fphys.2016.00098
Gao, X., Wang, X., Xiong, W., and Chen, J. (2016). In vivo reprogramming reactive glia into iPSCs to produce new neurons in the cortex following traumatic brain injury. Sci. Rep. 6:22490. doi: 10.1038/srep22490
Ghobrial, G. M., Anderson, K. D., Dididze, M., Martinez-Barrizonte, J., Sunn, G. H., Gant, K. L., et al. (2017). Human neural stem cell transplantation in chronic cervical spinal cord injury: functional outcomes at 12 months in a Phase II clinical trial. Neurosurgery 64, 87–91. doi: 10.1093/neuros/nyx242
Gold, E. M., Su, D., López-Velázquez, L., Haus, D. L., Perez, H., Lacuesta, G. A., et al. (2013). Functional assessment of long-term deficits in rodent models of traumatic brain injury. Regen. Med. 8, 483–516. doi: 10.2217/rme.13.41
Guo, S., Zhen, Y., and Wang, A. (2017). Transplantation of bone mesenchymal stem cells promotes angiogenesis and improves neurological function after traumatic brain injury in mouse. Neuropsychiatr. Dis. Treat 13, 2757–2765. doi: 10.2147/NDT.S141534
Guo, X. B., Deng, X., and Wei, Y. (2017). Homing of cultured endothelial progenitor cells and their effect on traumatic brain injury in rat model. Sci. Rep. 7:4164. doi: 10.1038/s41598-017-04153-2
Guo, X., Liu, L., Zhang, M., Bergeron, A., Cui, Z., Dong, J. F., et al. (2009). Correlation of CD34+cells with tissue angiogenesis after traumatic brain injury in a rat model. J. Neurotrauma 26, 1337–1344. doi: 10.1089/neu.2008-0733
Haus, D. L., López-Velázquez, L., Gold, E. M., Cunningham, K. M., Perez, H., Anderson, A. J., et al. (2016). Transplantation of human neural stem cells restores cognition in an immunodeficient rodent model of traumatic brain injury. Exp. Neurol. 281, 1–16. doi: 10.1016/j.expneurol.2016.04.008
Huang, S. H., Wang, L., Chi, F., Wu, C. H., Cao, H., Zhang, A., et al. (2013). Circulating brain microvascular endothelial cells (cBMECs) as potential biomarkers of the bloodbrain barrier disorders caused by microbial and non-microbial factors. PLoS One 8:e62164. doi: 10.1371/journal.pone.0062164
Huang, X. T., Zhang, Y. Q., Li, S. J., Li, S. H., Tang, Q., Wang, Z. T., et al. (2013). Intracerebroventricular transplantation of Ex vivo expanded endothelial colony-forming cells restores blood–brain barrier integrity and promotes angiogenesis of mice with traumatic brain injury. J. Neurotrauma 30, 2080–2088. doi: 10.1089/neu.2013.2996
Kobayashi, Y., Okada, Y., Itakura, G., Iwai, H., Nishimura, S., Yasuda, A., et al. (2012). Pre-evaluated safe human i PSC-derived neural stem cells promote functional recovery after spinal cord injury in common marmoset without tumorigenicity. PLoS One 7:e52787. doi: 10.1371/journal.pone.0052787
Lin, Y., Luo, L. L., Sun, J., Gao, W., Tian, Y., Park, E., et al. (2017). Relationship of circulating CXCR4+EPC with prognosis of mild traumatic brain injury patients. Aging Dis. 8, 115–127. doi: 10.14336/AD.2016.0610
Lin, Y., Weisdorf, D. J., Solovey, A., and Hebbel, R. P. (2000). Origins of circulating endothelial cells and endothelial outgrowth from blood. J. Clin. Invest. 105, 71–77. doi: 10.1172/jci8071
Lyu, Q., Zhang, Z. B., Fu, S. J., Xiong, L. L., Liu, J., and Wang, T. H. (2017). Microarray expression profile of lncrnas and mrnas in rats with traumatic brain injury after A2B5+cell transplantation. Cell Transplant 26, 1622–1635. doi: 10.1177/0963689717723014
Maas, A. I. R., Menon, D. K., Adelson, P. D., Andelic, N., Bell, M. J., Belli, A., et al. (2017). Traumatic brain injury: integrated approaches to improve prevention, clinical care, and research. Lancet Neurol. 16, 987–1048.
Malinovskaya, N. A., Komleva, Y. K., Salmin, V. V., Morgun, A. V., Shuvaev, A. N., Panina, Y. A., et al. (2016). Endothelial progenitor cells physiology and metabolic plasticity in brain angiogenesis and blood-brain barrier modeling. Front. Physiol. 7:599. doi: 10.3389/fphys.2016.00599
Park, K. J., Park, E., Liu, E., and Baker, A. J. (2014). Bone marrow-derived endothelial progenitor cells protect postischemic axons after traumatic brain injury. J. Cereb. Blood Flow Metab. 34, 357–366. doi: 10.1038/jcbfm.2013.216
Philips, M. F., Mattiasson, G., Wieloch, T., Björklund, A., Johansson, B. B., Tomasevic, G., et al. (2001). Neuroprotective and behavioral efficacy of nerve growth factor-transfected hippocampal progenitor cell transplants after experimental traumatic brain injury. J. Neurosurg. 94, 765–774. doi: 10.3171/jns.2001.94.5.0765
Ran, Q. S., Yu, Y. H., Fu, X. H., and Wen, Y. C. (2015). Activation of the Notch signaling pathway promotes neurovascular repair after traumatic brain injury. Neural Regen. Res. 10, 1258–1264. doi: 10.4103/1673-5374.162758
Reis, C., Gospodarev, V., Reis, H., Wilkinson, M., Gaio, J., Araujo, C., et al. (2017). Traumatic brain injury and stem cell:pathophysiology and update on recent treatment modalities. Stem Cells Int. 2017, 1–13. doi: 10.1155/2017/6392592
Roobrouck, V. D., Clavel, C., Jacobs, S. A., Ulloa-Montoya, F., Crippa, S., Sohni, A., et al. (2011). Differentiation potential of human postnatal mesenchymal stem cells, mesoangioblasts, and multipotent adult progenitor cells reflected in their transcriptome and partially influenced by the culture conditions. Stem Cells 29, 871–882. doi: 10.1002/stem.633
Sanchez-Ramos, J., Song, S., Cardozo-Pelaez, F., Hazzi, C., Stedeford, T., Willing, A., et al. (2000). Adult bone marrow stromal cells differentiate into neural cells in vitro. Exp. Neurol. 164, 247–256. doi: 10.1006/exnr.2000.7389
Shi, X., Bai, Y., Zhang, G., Liu, Y., Xiao, H., Liu, X., et al. (2018). Effects of over-expression of SOD2 in bone marrow-derived mesenchymal stem cells on traumatic brain injury. Cell Tissue Res. 372, 67–75. doi: 10.1007/s00441-017-2716-7
Takahashi, K., and Yamanaka, S. (2006). Induction of pluripotent stem cells from mouse embryonic and adult fibroblast cultures by defined factors. Cell 126, 663–676. doi: 10.1016/j.cell.2006.07.024
Walker, P. A., Bedi, S. S., Shah, S. K., Jimenez, F., Xue, H., Hamilton, J. A., et al. (2012). Intravenous multipotent adult progenitor cell therapy after traumatic brain injury:modulation of the resident microglia population. J. Neuroinflammation 9:228. doi: 10.1186/1742-2094-9-228
Walker, P. A., Shah, S. K., Jimenez, F., Gerber, M. H., Xue, H., Cutrone, R., et al. (2010). Intravenous multipotent adult progenitor cell therapy for traumatic brain injury: preserving the blood brain barrier via an interaction with splenocytes. Exp. Neurol. 225, 341–352. doi: 10.1016/j.expneurol.2010.07.005
Wallenquist, U., Brännvall, K., Clausen, F., Lewén, A., Hillered, L., Forsberg-Nilsson, K., et al. (2009). Grafted neural progenitors migrate and form neurons after experimental traumatic brain injury. Restor Neurol. Neurosci. 27, 323–334. doi: 10.3233/RNN-2009-0481
Wang, L., Wang, X., Su, H., Han, Z., Yu, H., Wang, D., et al. (2015). Recombinant human erythropoietin improves the neurofunctional recovery of rats following traumatic brain injury via an increase in circulating endothelial progenitor cells. Transl. Stroke Res. 6, 50–59. doi: 10.1007/s12975-014-0362-x
Wang, S., Kan, Q., Sun, Y., Han, R., Zhang, G., Peng, T., et al. (2013). Caveolin-1 regulates neural differentiation of rat bone mesenchymal stem cells into neurons by modulating Notch signaling. Int. J. Dev. Neurosci. 31, 30–35. doi: 10.1016/j.ijdevneu.2012.09.004
Wang, Z., Luo, Y., Chen, L., and Liang, W. (2017). Safety of neural stem cell transplantation in patients with severe traumatic brain injury. Exp. Ther. Med. 13, 3613–3618. doi: 10.3892/etm.2017.4423
Wei, Z. Z., Lee, J. H., Zhang, Y., Zhu, Y. B., Deveau, T. C., Gu, X., et al. (2016). Intracranial transplantation of hypoxia-preconditioned i PSC-derived neural progenitor cells alleviates neuropsychiatric defects after traumatic brain injury in juvenile rats. Cell Transplant 25, 797–809. doi: 10.3727/096368916x690403
Wong, S. P., Rowley, J. E., Redpath, A. N., Tilman, J. D., Fellous, T. G., and Johnson, J. R. (2015). Pericytes, mesenchymal stem cells and their contributions to tissue repair. Pharmacol. Ther. 151, 107–120. doi: 10.1016/j.pharmthera.2015.03.006
Xin, H., Li, Y., Cui, Y., Yang, J. J., Zhang, Z. G., and Chopp, M. (2013). Systemic administration of exosomes released from mesenchymal stromal cells promote functional recovery and neurovascular plasticity after stroke in rats. J. Cereb. Blood Flow Metab. 33, 1711–1715. doi: 10.1038/jcbfm.2013.152
Xiong, Y., Mahmood, A., and Chopp, M. (2017). Emerging potential of exosomes for treatment of traumatic brain injury. Neural Regen. Res. 12, 19–22. doi: 10.4103/1673-5374.198966
Xiong, Y., Mahmood, A., Lu, D., Qu, C., Kazmi, H., Goussev, A., et al. (2008). Histological and functional outcomes after traumatic brain injury in mice null for the erythropoietin receptor in the central nervous system. Brain Res. 1230, 247–257. doi: 10.1016/j.brainres.2008.06.127
Xue, S., Zhang, H. T., Zhang, P., Luo, J., Chen, Z. Z., Jang, X. D., et al. (2010). Functional endothelial progenitor cells derived from adipose tissue show beneficial effect on cell therapy of traumatic brain injury. Neurosci. Lett. 473, 186–191. doi: 10.1016/j.neulet.2010.02.035
Yeo, R. W., Lai, R. C., Zhang, B., Tan, S. S., Yin, Y., Teh, B. J., et al. (2013). Mesenchymal stem cell: an efficient mass producer of exosomes for drug delivery. Adv. Drug Deliv. Rev. 65, 336–341. doi: 10.1016/j.addr.2012.07.001
Yu, P., Zhang, Z., Li, S., Wen, X., Quan, W., Tian, Q., et al. (2016). Progesterone modulates endothelial progenitor cell (EPC) viability through the CXCL12/CXCR4/PI3K/Akt signalling pathway. Cell Prolif. 49, 48–57. doi: 10.1111/cpr.12231
Zhang, C., Saatman, K. E., Royo, N. C., Soltesz, K. M., Millard, M., Schouten, J. W., et al. (2005). Delayed transplantation of human neurons following brain injury in rats:a long-term graft survival and behavior study. J. Neurotrauma 22, 1456–1474. doi: 10.1089/neu.2005.22.1456
Zhang, R., Liu, Y., Yan, K., Chen, L., Chen, X. R., Li, P., et al. (2013). Anti-inflammatory and immunomodulatory mechanisms of mesenchymal stem cell transplantation in experimental traumatic brain injury. J. Neuroinflammation 10:106. doi: 10.1186/1742-2094-10-106
Zhang, Y., Chopp, M., Meng, Y., Katakowski, M., Xin, H., Mahmood, A., et al. (2015). Effect of exosomes derived from multipluripotent mesenchymal stromal cells on functional recovery and neurovascular plasticity in rats after traumatic brain injury. J. Neurosurg. 122, 856–867. doi: 10.3171/2014.11.JNS14770
Zhang, Y., Chopp, M., Zhang, Z. G., Katakowski, M., Xin, H., Qu, C., et al. (2017). Systemic administration of cell-free exosomes generated by human bone marrow derived mesenchymal stem cells cultured under 2D and 3D conditions improves functional recovery in rats after traumatic brain injury. Neurochem. Int. 111, 69–81. doi: 10.1016/j.neuint.2016.08.003
Keywords: traumatic brain injury, stem cell, mechanism, treatment, review
Citation: Zhou Y, Shao A, Xu W, Wu H and Deng Y (2019) Advance of Stem Cell Treatment for Traumatic Brain Injury. Front. Cell. Neurosci. 13:301. doi: 10.3389/fncel.2019.00301
Received: 20 December 2018; Accepted: 19 June 2019;
Published: 13 August 2019.
Edited by:
Hung-Ming Chang, Taipei Medical University, TaiwanReviewed by:
Gourav Roy Choudhury, Texas Biomedical Research Institute, United StatesZoltan Molnar, University of Oxford, United Kingdom
Copyright © 2019 Zhou, Shao, Xu, Wu and Deng. This is an open-access article distributed under the terms of the Creative Commons Attribution License (CC BY). The use, distribution or reproduction in other forums is permitted, provided the original author(s) and the copyright owner(s) are credited and that the original publication in this journal is cited, in accordance with accepted academic practice. No use, distribution or reproduction is permitted which does not comply with these terms.
*Correspondence: Anwen Shao, MjExMTgxMTZAemp1LmVkdS5jbg==; YW53ZW5zaGFvQHNpbmEuY29t; Yongchuan Deng, ZHljMDAxQHpqdS5lZHUuY24=
†These authors have contributed equally to this work