- Institute of Neuroregeneration and Neurorehabilitation, Qingdao University, Qingdao, China
Natural killer T (NKT) cells are a unique subset of T lymphocytes with the expression of T cell receptor (TCR) and NK cell lineage receptors. These cells can rapidly release large quantities of cytokines and function as a bridge between innate and adaptive immunity. To date, multiple reports have investigated the role of NKT cells under various pathological conditions, such as cancer, autoimmune disease, and infection. Knowledge about NKT cells in neurological diseases is increasing, albeit limited. Here, we review evidence for the involvement of NKT cells in neurological diseases, and discuss immunotherapeutic potential and future study goals. As the development and function of NKT cells become increasingly well understood, the next few years should yield many new insights into NKT cell function, and mechanistic regulation in neurological disorders.
Introduction
Historically, the brain has long been regarded as an immune-privileged area as a result of the presence of a blood-brain barrier (BBB) and the lack of lymphatic drainage. Researchers focused mainly on neurons or glial cells when investigating the underlying molecular mechanism of neurological disorders (Price et al., 1987; Almad and Maragakis, 2012). However, mounting evidence highlights the importance of neuroinflammation in neurological diseases (Schwartz and Deczkowska, 2016; Fung et al., 2017; Skaper et al., 2018). In addition, the recently discovered glymphatic system and meningeal lymphatics uncovers a way for peripheral immune cells to enter the brain and communicate with resident cells (Da Mesquita et al., 2018; Sun et al., 2018). Thus, the function of peripheral immune cells in neurological diseases should motivate and be investigated by more researchers.
Natural killer T cells are unique CD1d-restricted T lymphocytes that function as a bridge between innate and adaptive immunity. Based on their TCR usage and lipid antigen specificity, NKT cells have been divided into two subpopulations, type I and type II. Both of these subpopulations recognize lipids antigens presented by CD1d (Bendelac et al., 2007; Godfrey et al., 2010; Nishioka et al., 2018). NKT cells account for a small percentage of lymphocytes, but have profound immunomodulatory roles in a variety of diseases, as these cells show both innate, and adaptive immunological features (Taniguchi et al., 2003; Brennan et al., 2013). Given the abundant existence of glycosphingolipids in the brain (Hirabayashi, 2012), we wondered whether and how NKT cells functioned in neurological diseases. In this review, we described NKT cell properties, summarized current reports on the functions of NKT cells in neurological disorders, including ischemic stroke, brain tumor, multiple sclerosis (MS), neurodegenerative disease and other neurological disorders, and discussed immunotherapeutic potential of these cells and the goals of future studies.
NKT Cell Classification and Effector Function
Natural killer T cells are a specialized subset of T cells that express TCR and NK cell lineage markers, such as NK1.1, NKG2D, and Ly49A. There are two broad categories of NKT cells, type I, and type II. Type I NKT cells, known as invariant NKT cells (iNKT cells) typically express an invariant Vα14-Jα18 TCRα chain and a limited number of non-invariant TCRβ chains that recognize α-galactosylceramide (α-GalCer), a glycosphingolipid isolated from the marine sponge, presented by CD1d. In addition, an increasing number of endogenous antigens, such as isoglobotrihexosylceramide (iGb3) and disialoganglioside (GD3), have also been discovered. Conversely, type II NKT cells use diverse TCRα and β chains that are reactive to more broad antigens, such as glycolipids, phospholipids, and hydrophobic antigens (Bendelac et al., 2007; Godfrey et al., 2010; Nishioka et al., 2018; Figure 1A). Genetic tools have been generated to study NKT cell development and function based on the TCR composition of type I and type II NKT cells. Vα14Jα18 transgenic mice and Jα18−/− mice were used to enrich and delete type I NKT cells, respectively (Bendelac et al., 1996; Cui et al., 1997). In contrast to the Jα18−/− mouse, CD1d−/− mice lack both type I and type II NKT cells, as CD1d is essential for the positive selection of both subsets in the thymus (Chen et al., 1997). Unlike type I NKT cells, whose development and function have been well investigated, the functional role of type II NKT cells is less clear due to the lack of universal and specific staining antibodies, although these cells are more prevalent in humans than type I NKT cells are (Dhodapkar and Kumar, 2017).
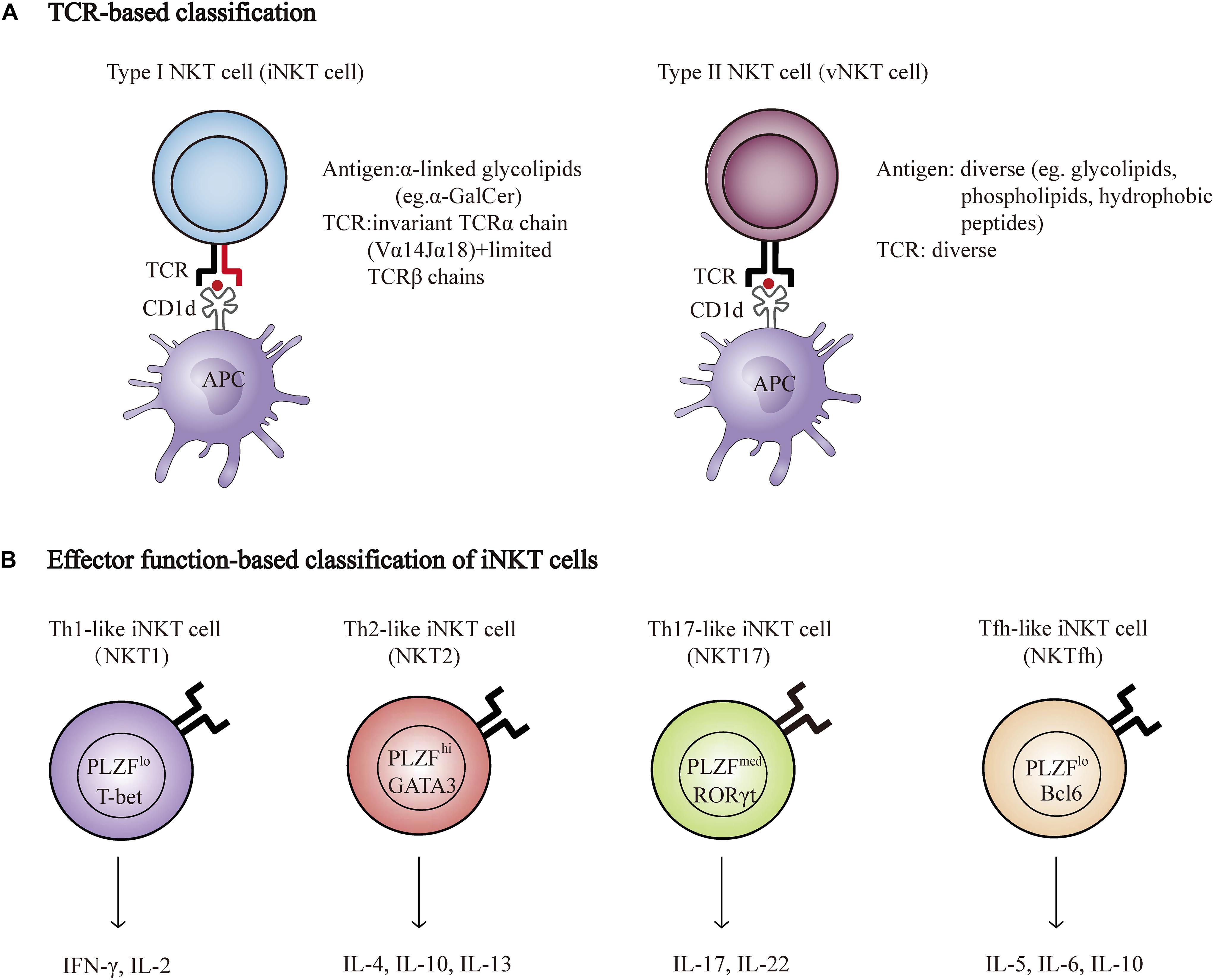
Figure 1. NKT cell classification. (A) Properties of Type I (iNKT) and type II NKT cell (vNKT) subpopulations based on the composition of TCR. (B) Major subsets of iNKT cells. Transcription factors and cytokines associated with each subset are shown. T-bet, T-box transcription factor; GATA3, GATA-binding protein 3; RORγt, retinoic acid receptor-related orphan receptor-γt; PLZF, promyelocytic leukemia zinc finger protein; Bcl6, B-Cell Lymphoma 6; IFNγ, interferon-γ; IL-2, Interleukin 2.
Natural killer T cells can quickly secrete large quantities of cytokines, such as interferon-γ (IFN-γ), IL-17, IL-4, and IL-10, which are important for the initiation and regulation of various immune responses (Brennan et al., 2013). Researchers have recently described a new classification system according to the cytokines and transcription factors expressed by type I NKT cells, namely, Th1-like iNKT cell (iNKT1), Th2-like iNKT cell (iNKT2), Th17-like iNKT cell (iNKT17), and Tfh-like iNKT cell (iNKTfh) (Chang et al., 2011; Engel et al., 2016; Figure 1B). The effector function of NKT cells is determined by the differentiation potential of these effector subsets induced via distinct mechanisms of activation. For example, activation with IL-12 and TLR agonists results in the production of Th1-type cytokines instead of Th2-type cytokines (Brigl et al., 2003; Nagarajan and Kronenberg, 2007). In addition, different lipid antigens influence not only the magnitude but also the quality of NKT cell activation (Im et al., 2009).
Natural killer T cells have been reported to participate in a variety of diseases, such as infection, autoimmune disease, and cancer (Dhodapkar and Kumar, 2017). Upon infection, antigenic microbial lipids can be presented by CD1d and then lead to activation-induced cytokine production, which subsequently facilitates the recruitment and activation of other innate, and adaptive immune cells that promote bacterial or viral infection (Brigl et al., 2003). Type I NKT cells have been implicated in antitumor immunity by direct or indirect ways (Godfrey et al., 2018). Notably, chimeric antigen receptor expressing NKT (CAR-NKT) cells and α-Galcer-type I NKT cell-based immunotherapy are being explored (Kriegsmann et al., 2018; Pyaram and Yadav, 2018). During the autoimmune response, certain “self” lipid antigens are presented by antigen presenting cells, which then activate NKT cells to promote inflammatory cytokine production, and aggravate the disease (Wu and Van Kaer, 2009). Despite the classical CD1d-mediated activation of NKT cells in disease progression, non-cognate interactions-related stimuli, including cytokines, damage-associated molecular patterns (DAMPs), and pathogen-associated molecular patterns (PAMPs), also contribute to NKT cell activation, which functions in many diseases (Shimizu et al., 2002; Paget et al., 2007). The detailed molecular mechanism of NKT cell-mediated disease development and progression requires further investigation.
NKT Cells and Neurological Diseases
NKT Cells and Ischemic Stroke
Ischemic stroke is one of the leading causes of disability and mortality worldwide, and its incidence is increasing. Early reperfusion remains the effective treatment but can result in secondary damage; thus understanding and alleviating brain damage has become the focus of research (Pan et al., 2007). Typically, most studies have focused on neurons, the cells most sensitive to ischemic-induced injury (Baron et al., 2014). However, mounting evidence has elucidated the critical and diverse roles of immune cells during ischemic stroke (Iadecola and Anrather, 2011; Chamorro et al., 2012), which may offer a novel perspective on immunotherapy for resolving this devastating condition.
Several studies have shown that type I NKT cells accumulate in the ischemic hemisphere in mice or rats by transient occlusion of the middle cerebral artery (tMCAO) (Gelderblom et al., 2009; Lehmann et al., 2014). Similarly, when a cerebral ischemia model was induced by permanent occlusion of the middle cerebral artery (pMCAO), type I NKT cells were observed to infiltrate the blood and brain of induced mice at 24 and 48 h, and this infiltration occurred at an earlier time-point when α-Galcer was administered (Wang et al., 2016). The effects of NKT cells on cerebral stroke severity have also been studied. Wang et al. (2016) discovered that brain water content, cerebral infarct volume, neurological deficit scores and brain edema were significantly increased at 24 h in the pMCAO plus α-Galcer group compared with the pMCAO plus vehicle group. In addition, the production of the proinflammatory cytokines TNFα and IFNγ was increased in the pMCAO plus α-Galcer group. These data suggest that activated type I NKT cells may contribute to brain infarction in cerebral ischemia. In contrast, Kleinschnitz et al. (2010) demonstrated that type I and type II NKT cells are probably of minor importance during the early phase of injury in a model of tMCAO by using CD1d-deficient mice. The reasons for the discrepant effects may result from different NKT cell manipulating methods and mouse models. In the study by Wang et al. (2016) the function of activated type I NKT cells was examined by using a pMCAO mouse model through administration of α-Galcer, whereas in the study by Kleinschnitz et al. (2010) the effects of both type I NKT and type II NKT cell deficiency on brain infarction volume were tested by using a tMCAO model induced in CD1d−/− mice. Further studies discriminating the role of different NKT cell subsets in ischemic stroke are needed.
Infectious complications, particularly pneumonia and urinary tract infections, were reported to be leading causes of death in ischemic stroke patients. The suppression of immune responses after brain ischemia increases the susceptibility to infections, and the underlying molecular mechanisms are largely unknown (Meisel and Meisel, 2011; Chamorro et al., 2012). Emerging evidence indicates that NKT cells function in stroke-induced suppression of the immune response. Wong et al. (2011) discovered that NKT cell-deficient mice (CD1d−/−) showed a similar brain infarct size after tMCAO but were more susceptible to poststroke pulmonary infection with greater pulmonary damage, more prominent neutrophil infiltration and decreased survival rate. NKT cells showed restricted crawling and produced more Th2-type cytokines, including IL-10 and IL-5, and less Th1-type cytokines, such as IFN-γ and IL-12 after tMCAO. Selective immunomodulation of type I NKT cells with the specific activator α-Galcer or through the blockade of noradrenergic neurotransmitters protected mice from poststroke infections. The same group conducted another study by delineating the systemic immune profile of stroke patients over time. Consistent with observations in mouse models, a profound activation of type I NKT cells was observed after stroke, which positively correlated with IL-10 production in patients with stroke. The authors proposed that stroke-induced activation of type I NKT cells mediated an immunosuppressive response via the release of IL-10, rendering the patients more susceptible to infections (Wong et al., 2017). These results suggest that the modulation of NKT cell activation, especially enhanced IL-10 production, may prevent poststroke-associated infections, although other explanations of poststroke immunosuppression may exist.
NKT Cells and Multiple Sclerosis
Multiple sclerosis is a chronic autoimmune disorder of the central nervous system (CNS) in young adults. The pathological hallmarks of MS are extensive demyelination and formation of inflammatory plaques in the spinal cord and brain, which causes irreversible neurological injuries (Frohman et al., 2006). Although the cause of this disease is not entirely clear, autoreactive T cells are considered to be the main attackers on the CNS (Kurschus, 2015). Other immune cells, by affecting myelin-reactive T cell function, may also participate in this pathological condition (Herz et al., 2017).
Numerous studies have highlighted the important role of NKT cells during experimental autoimmune encephalomyelitis (EAE), the most commonly used mouse model of MS. Basically, these studies can be divided into two categories according to the tools used to manipulate NKT cells: genetic tools, such as NKT cell TCR-specific transgenic mice or NKT cell-deficient mice, to enrich or delete NKT cells and NKT cell ligands to trigger the activation of NKT cells. Vα14-Jα281 transgenic mice are protected from EAE in the non-diabetic (NOD) background, and this protection is conferred by an inhibited Th1 response (Mars et al., 2002). However, deletion of NKT cells exhibits conflicting effects on EAE, with some studies showing no effects (Singh et al., 2001; Furlan et al., 2003) and other studies showing disease exacerbation in CD1d-deficient mice (Teige et al., 2004) and in Jα18-deficient mice (Furlan et al., 2003; Oh and Chung, 2011; Denney et al., 2012). These contradictory observations may result from different mouse backgrounds or EAE models (Van Kaer et al., 2015).
Similar to NKT cell enrichment or deletion, the activation of NKT cells through α-GalCer or its analog also has contrasting effects on EAE outcome. Most studies have shown that the activation of type I NKT cells with α-GalCer or its analog improved EAE outcome (Miyamoto et al., 2001; Singh et al., 2001; Furlan et al., 2003; Kojo et al., 2005; Denney et al., 2012). These improvements are achieved either through indirectly enhancing the Th2 response and/or reducing the Th1 response (Miyamoto et al., 2001; Singh et al., 2001; Furlan et al., 2003) or potentiating the differentiation of immunosuppressive myeloid cell, such as regulatory dendritic cells (DCs) (Kojo et al., 2005), myeloid-derived suppressor cells (MDSCs) (Parekh et al., 2013), and M2 (alternatively activated) macrophages (Denney et al., 2012; Figure 2). Unexpectedly, Qian et al. (2010) demonstrated that high doses of α-GalCer could selectively interacted with CD1d2 expressed on memory T cells instead of activating type I NKT cells, which mainly required CD1d1 expression on APCs, and worsen EAE by directly enhancing Th17 and Th1 differentiation through phosphorylation of STAT3 and activation of NF-κB. Furthermore, another study found that coimmunization of α-GalCer with myelin antigens potentiated EAE in B10. PL mice and prevented EAE in C57BL/6 mice, while prior immunization prevented EAE in both strains. Exacerbation was mediated by promoting Th1 response, and protection was dependent on IL-4 production (Jahng et al., 2001). For type II NKT cells, Jahng et al. discovered that administration of sulfatide, which is only recognized by a subset of type II NKT cells, protected mice from EAE in a CD1d-dependent manner (Jahng et al., 2004). These differences may be due to different mouse backgrounds, as NKT cell populations differ greatly between strains. It is also worth considering that the differences may result from the activation magnitude and treatment time during disease. Regardless of the reason, one conclusion we draw is that the function of NKT cells on EAE mainly depends on the cytokines secreted or factors expressed by NKT cells which can further determine activation or inhibition of peripheral adaptive or innate immune cells, and eventually determine disease outcome.
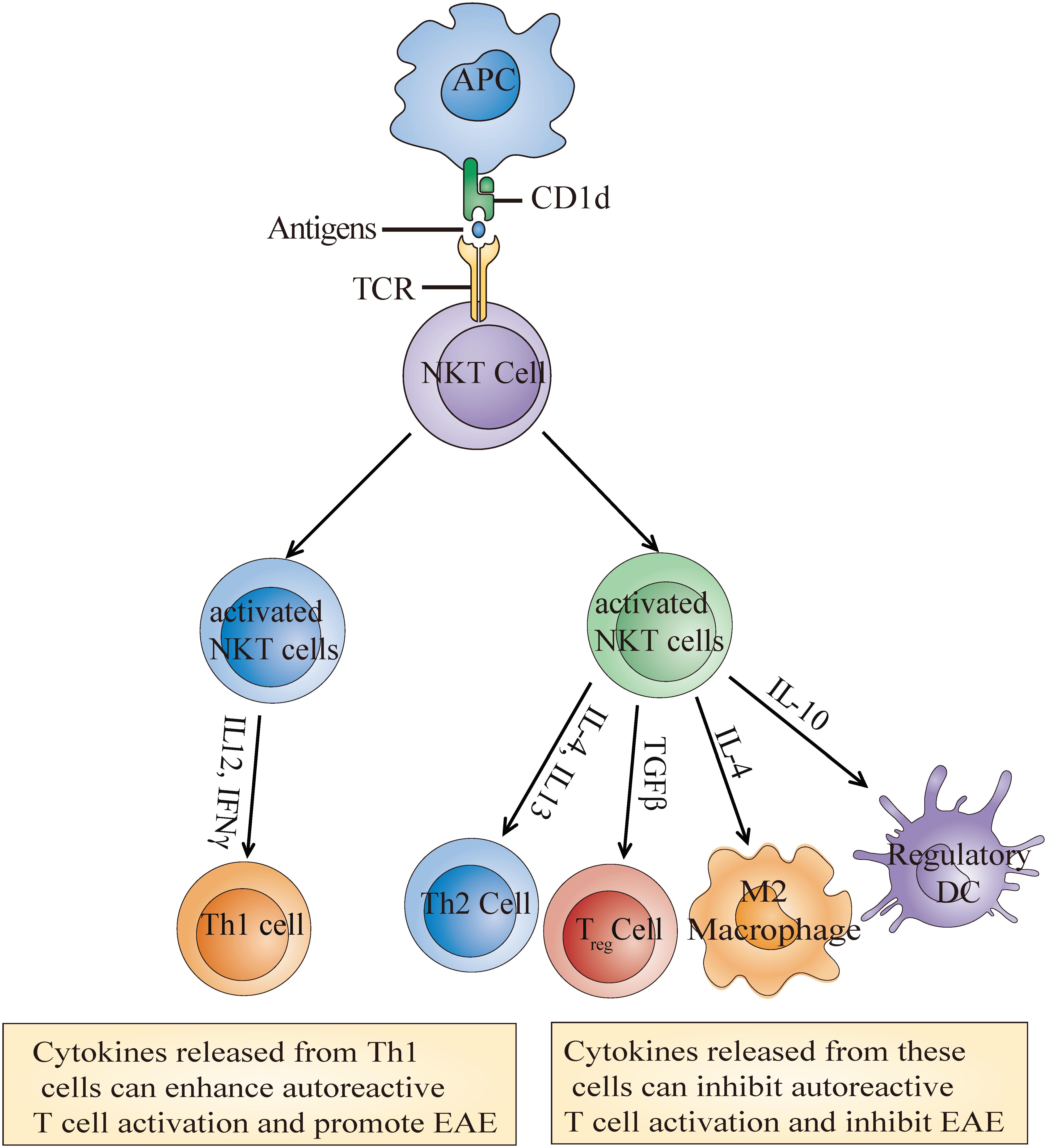
Figure 2. Molecular mechanisms of the NKT cell activation-mediated effects on EAE. Recognition of α-Galcer or its analog presented by CD1d leads to the activation of NKT cells which then secrete multiple cytokines such as IL-4, IL-10, and TGFβ. These cytokines further induce the generation of some immunosuppressive cells or Th1 cells to inhibit or enhance autoreactive T cell activation and eventually alleviate or promote EAE development.
Several studies have investigated NKT cell number and function in MS patients. Some studies have reported that NKT cell number is decreased in MS patients (Illes et al., 2000; van der Vliet et al., 2001; Demoulins et al., 2003). O’Keeffe et al. (2008) observed an increased frequency of circulating NKT cells in MS patients, while Gausling et al. (2001) did not observe any difference. NKT cells also display distinct cytokine production profiles in MS patients. A study revealed that IL-4 production was increased by CD4+ NKT cell clones in RRMS (relapsing-remitting) patients compared to progressive MS patients and control subjects (Araki et al., 2003). Sara et al. found that NKT cells in secondary progressive MS patients displayed pro-inflammatory profiles (De Biasi et al., 2016). These conflicting findings may result from different forms of MS or distinct stages of disease progression. Notably, Stax et al. (2017) reported the surprising finding that sulfatide from myelin or cerebrospinal fluid apoE could directly activate human, but not mouse, type I NKT cells. These findings indicate the important roles of NKT cells in MS, although the detailed molecular mechanism requires further investigation.
Several studies mentioned that the current available drugs targeting MS may exert their actions through targeting NKT cells. It was reported that a great reduction of type I NKT cells in the peripheral blood was associated with the remission state of MS (Araki et al., 2003), and patients who were orally given a low dose of corticosteroid restored the frequency of type I NKT cells (Sakuishi et al., 2010). In addition, type 1 interferon-β (T1IFN-β), the first choice of therapy for MS, have been reported to promote the expansion and function of type I NKT cells to prevent autoimmune disease in pre-clinical models of MS (Gigli et al., 2007). These studies raised the possibility that modulating NKT cell activity might be an alternative way to treat MS.
Altogether, the above findings indicate that NKT cells play diverse roles in MS owing to their cytokine production pattern in certain scenarios. However, some questions remain poorly understood. Instead of investigating how NKT cell activation triggers T cell response, it would be important to determine whether other autoreactive lipids exist in the brain that can activate NKT cells to directly attack the CNS. What are the different roles of type I and type II of NKT cells during MS initiation and progression? Resolving these questions may provide a comprehensive understanding of the effects exerted by NKT cells on MS and expand our strategies for the rational design of NKT-cell-based immunotherapies.
NKT Cells and Brain Tumors
Brain tumors remain one of the most serious diseases that pose a significant public health threat. Most primary brain tumors are of glial-cell origin, such as glioblastoma, astrocytomas, and oligodendrogliomas (Fu et al., 2014; Gao, 2017). Unlike other kinds of tumors, the treatment of brain tumors is especially difficult due to the blood brain barrier, which restricts drugs from gaining access to the tumors. Although immunotherapies have been considered an effective treatment for tumors, the translation of these strategies to brain tumors is still limited (Brown et al., 2018).
Recent approaches to harness antitumor T cell responses using antibodies of immune checkpoint inhibitors and chimeric antigen receptor (CAR)-T cell immunotherapy have yielded some success (Sanmamed and Chen, 2018). However, due to the complexity of the tumor microenvironment, the application of cancer immunotherapy is limited (Liu et al., 2019). Revealing the functional properties and regulatory mechanisms of immune cells in the tumor microenvironment is critical to overcome the limitations of existing cancer immunotherapy and explore new approaches for cancer immunotherapy. NKT cells, as the bridging system between innate immunity and adaptive immunity, have been implicated in tumor recognition and destruction (Berzins et al., 2011; Godfrey et al., 2018). Type I NKT cells target tumor cells by diverse effective strategies. First, these cells can kill tumor cells by secreting perforin and granzyme B via cytolysis (Nair and Dhodapkar, 2017). Second, activated type I NKT cells secrete various kinds of cytokines to modulate the function of other immune cells such as T cells, B cells and DCs. Third, immunosuppressive cells, including tumor-associated macrophages (TAMs) and MDSCs, could also be targeted by type I NKT cells to indirectly attack tumor cells (McEwen-Smith et al., 2015; Pyaram and Yadav, 2018). Unlike type I NKT cells, type II NKT cells have been reported to inhibit antitumor immunity, although only a few investigations into the role of type II NKT cells in cancer have been published (Godfrey et al., 2018).
Type I NKT cell number and functional properties have been evaluated in glioma patients. Both the number and expanding potential of type I NKT cells in the blood of glioma patients was comparable to those of healthy controls, and these cells could kill glioma in a CD1d-dependent manner, indicating their normal function (Dhodapkar et al., 2004; Teo et al., 2014). Moreover, CD1d expression has been observed in some kinds of brain tumors including medulloblastoma and glioblastoma (Liu et al., 2013; Pyaram and Yadav, 2018). These results suggest that enhancing type I NKT cell function may potentiate antitumor immunity. In support of this notion, studies have observed tumor killing potential by using strategies to induce type I NKT cell activation. Vaccination with irradiated tumor cells pulsed with α-Galcer significantly induced the activation of type I NKT cells and was effective for the treatment of glioma (Hunn et al., 2012). Intracranial administration of NKT cells with the α-Galcer analog 7DW8-5 resulted in an obvious anti-tumor effect (Liu et al., 2013). In addition, co-delivery of tumor-derived exosomes with α-Galcer-loaded DCs improved priming of CD8 T cells and elicited strong anti-tumor immune responses (Liu et al., 2017). To date, the function of type II NKT cells in brain tumors is not understood. These results highlight the necessity of further research to clarify the effects and molecular mechanism of NKT cell subsets in regulating brain tumor immunity, which may offer new therapeutic targets for brain tumor immunotherapy.
NKT Cells and Neurodegenerative Disease
The increased frequency of neurodegenerative disease can reduce human life expectancy. The most common neurodegenerative diseases are Alzheimer’s disease (AD), Parkinson’s disease (PD), Huntington’s disease (HD), and amyotrophic lateral sclerosis (ALS). Understanding the causes and molecular mechanisms of each disease is desperately required for the effective treatment of neurodegeneration (Heemels, 2016; Gitler et al., 2017).
Despite the well-established role of neuroinflammation in neurodegenerative disease, the function of NKT cells in neurodegeneration is limited (Chen et al., 2016). Notably, Finkelstein et al. reported the role of type I NKT cells in amyotrophic lateral sclerosis (ALS). Type I NKT cells were dramatically accumulated in the spinal cord, spleen and liver of the induced mouse ALS model. Immunomodulation of NKT cells using an analog of α-GalCer delayed motor neuron death and reduced astrogliosis in the spinal cord. Mechanistically, modulation of NKT cells induced a cytokine shift in the liver and facilitated T cell recruitment into the affected spinal cord, leading to a significant prolongation of the life span of mSOD1 mice (Finkelstein et al., 2011). Clearly, more research is required to understand the role of NKT cells in other neurodegenerative diseases considering the high abundance of glycolipids in the brain (Hirabayashi, 2012).
NKT Cells and CNS Viral Infection
The CNS is classically considered an immune-privileged area. The presence of pathogens can activate tissue resident immune cells via the recognition of PAMPs with pattern recognition receptors (PRRs), resulting in neuroinflammation and inducing subsequent T cell infiltration. Infection of the CNS usually generates efficient immune responses(Ransohoff and Brown, 2012). Mars et al. (2014) demonstrated that type I NKT cells resided in the CNS in the absence of inflammation and their presence dramatically augmented after local viral infection. The infiltrating NKT cells expressed a pro-inflammatory cytokine profile and inhibited the anti-viral responses of CD8 T cells. Accordingly, treatment with exogenous type I NKT cell ligands inhibited the viral-specific CD8 T cell response. These results indicate the involvement of type I NKT cells in viral infection of the CNS.
NKT Cells and X-Linked Adrenoleukodystrophy (X-ALD)
X-linked adrenoleukodystrophy is a metabolic disorder with the accumulation of very long-chain fatty acids (VLCFA) in plasma and tissues caused by impaired peroxisomal beta-oxidation. The disease is characterized by progressive demyelination within the CNS and adrenal insufficiency (Engelen et al., 2012). In ALD patients, type I NKT cell frequency and CD1d expression on the surface of B cells were slightly decreased (Gautron et al., 2010). Considering the correlation between NKT cell activation ligands and fatty acid metabolism, the function of NKT cells in ALD progression deserves further investigation in mouse models.
NKT Cells and Myasthenia Gravis
Myasthenia gravis (MG) is an autoimmune disorder of the neuromuscular junction that causes weakness in the skeletal muscles (Thiruppathi et al., 2012). Increased mature NKT cells were observed in the blood of MG patients with thymic hyperplasia, but not in those with thymoma (Suzuki et al., 2005). Immunization with α-GalCer activated type I NKT cells and protected mice from the occurrence of experimental autoimmune myasthenia gravis (EAMG), an animal model of human myasthenia gravis, which may be caused by the increased expansion of Treg cells (Liu et al., 2005; Wang et al., 2015).
NKT Cells and Neurological Antiphospholipid Syndrome (APS)
Antiphospholipid syndrome is an autoimmune disorder characterized by the presence of antiphospholipid antibodies, such as anticardiolipin antibodies, anti-β2-glycoprotein 1 antibodies, and lupus anticoagulant (Schreiber et al., 2018). APS neurological manifestations have been reported to be associated with some neurological diseases including ischemic stroke and MS (Ricarte et al., 2018; D’Angelo et al., 2019). Of note, cardiolipin is among the reported lipids antigens recognized by CD1d and this recognition may explain the increased number of NKT cells in some autoimmune diseases (Cox et al., 2009; D’Angelo et al., 2019). Wermeling et al. (2010) demonstrated that injection of apoptotic cells decreased the ability of type I NKT cells to produce cytokines, which further increased autoreactive B cells to produce autoreactive antibodies, such as anticardiolipin antibodies and antiphosphorylcholine, although this contrasts with the demonstration that NKT cells could promote B cell responses, and antibody production (Doherty et al., 2018). Considering the close association of antiphospholipid antibodies and some neurological diseases, it will be interesting to modulate NKT cell activity to regulate APS, although a better understanding of NKT cell and APS is needed.
Conclusion
Recent studies have elucidated novel and striking aspects of NKT cell function and its modulatory roles in disease progression. NKT cell-modulated neurological diseases, particularly ischemic stroke, MS and glioblastoma have been described (Table 1). Despite major advances in the understanding of NKT cell effector function in neurological disorders, there is still much to learn about. First, as more is learned about NKT heterogeneity, different NKT cell subsets should be discriminated in pathological conditions. There is very little knowledge of the role of type II NKT cells in neurological disease which should be the goal of future studies. Second, NKT cell function should be investigated in different disease progression stages. For ischemic stroke, NKT cells do not affect infarct volume but function in poststroke immunosuppression, which is also a very important factor that determines the life span of patients. Third, given the critical role of inflammation in neurodegenerative disease, the function of NKT cells in PD and AD should be explored. Finally, despite the well-established role of cognate recognition-induced activation of NKT cells, the non-cognate interaction-induced activation of NKT cells in disease progression deserves further investigation. If a thorough understanding of the roles and molecular mechanisms of NKT cells in neurological disease is gained, new avenues might be opened in the treatment of neurological disorders.
Author Contributions
YC was involved in the writing, reading literature, and design of the figures. QW was involved in the overall supervision of the review and editing of the manuscript. All authors gave approval before submission.
Funding
This work was supported by the Natural Science Foundation of China (NSFC; 81470599).
Conflict of Interest Statement
The authors declare that the research was conducted in the absence of any commercial or financial relationships that could be construed as a potential conflict of interest.
Acknowledgments
We thank Dr. Zhang Zhaolong for his critical reading and discussion.
References
Almad, A. A., and Maragakis, N. J. (2012). Glia: an emerging target for neurological disease therapy. Stem Cell Res. Ther. 3:37. doi: 10.1186/scrt128
Araki, M., Kondo, T., Gumperz, J. E., Brenner, M. B., Miyake, S., and Yamamura, T. (2003). Th2 bias of CD4+ NKT cells derived from multiple sclerosis in remission. Int. Immunol. 15, 279–288. doi: 10.1093/intimm/dxg029
Baron, J. C., Yamauchi, H., Fujioka, M., and Endres, M. (2014). Selective neuronal loss in ischemic stroke and cerebrovascular disease. J. Cereb. Blood Flow Metab. 34, 2–18. doi: 10.1038/jcbfm.2013.188
Bendelac, A., Hunziker, R. D., and Lantz, O. (1996). Increased interleukin 4 and immunoglobulin E production in transgenic mice overexpressing NK1 T cells. J. Exp. Med. 184, 1285–1293. doi: 10.1084/jem.184.4.1285
Bendelac, A., Savage, P. B., and Teyton, L. (2007). The biology of NKT cells. Annu. Rev. Immunol. 25, 297–336.
Berzins, S. P., Smyth, M. J., and Baxter, A. G. (2011). Presumed guilty: natural killer T cell defects and human disease. Nat. Rev. Immunol. 11, 131–142. doi: 10.1038/nri2904
Brennan, P. J., Brigl, M., and Brenner, M. B. (2013). Invariant natural killer T cells: an innate activation scheme linked to diverse effector functions. Nat. Rev. Immunol. 13, 101–117. doi: 10.1038/nri3369
Brigl, M., Bry, L., Kent, S. C., Gumperz, J. E., and Brenner, M. B. (2003). Mechanism of CD1d-restricted natural killer T cell activation during microbial infection. Nat. Immunol. 4, 1230–1237. doi: 10.1038/ni1002
Brown, N. F., Carter, T. J., Ottaviani, D., and Mulholland, P. (2018). Harnessing the immune system in glioblastoma. Br. J. Cancer 119, 1171–1181. doi: 10.1038/s41416-018-0258-8
Chamorro, A., Meisel, A., Planas, A. M., Urra, X., van de Beek, D., and Veltkamp, R. (2012). The immunology of acute stroke. Nat. Rev. Neurol. 8, 401–410. doi: 10.1038/nrneurol.2012.98
Chang, P. P., Barral, P., Fitch, J., Pratama, A., Ma, C. S., Kallies, A., et al. (2011). Identification of Bcl-6-dependent follicular helper NKT cells that provide cognate help for B cell responses. Nat. Immunol. 13, 35–43. doi: 10.1038/ni.2166
Chen, W. W., Zhang, X., and Huang, W. J. (2016). Role of neuroinflammation in neurodegenerative diseases (Review). Mol. Med. Rep. 13, 3391–3396. doi: 10.3892/mmr.2016.4948
Chen, Y. H., Chiu, N. M., Mandal, M., Wang, N., and Wang, C. R. (1997). Impaired NK1+ T cell development and early IL-4 production in CD1-deficient mice. Immunity 6, 459–467. doi: 10.1016/s1074-7613(00)80289-7
Cox, D., Fox, L., Tian, R., Bardet, W., Skaley, M., Mojsilovic, D., et al. (2009). Determination of cellular lipids bound to human CD1d molecules. PLoS One 4:e5325. doi: 10.1371/journal.pone.0005325
Cui, J., Shin, T., Kawano, T., Sato, H., Kondo, E., Toura, I., et al. (1997). Requirement for Valpha14 NKT cells in IL-12-mediated rejection of tumors. Science 278, 1623–1626. doi: 10.1126/science.278.5343.1623
Da Mesquita, S., Louveau, A., Vaccari, A., Smirnov, I., Cornelison, R. C., Kingsmore, K. M., et al. (2018). Functional aspects of meningeal lymphatics in ageing and Alzheimer’s disease. Nature 560, 185–191. doi: 10.1038/s41586-018-0368-8
D’Angelo, C., Franch, O., Fernandez-Paredes, L., Oreja-Guevara, C., Nunez-Beltran, M., Comins-Boo, A., et al. (2019). Antiphospholipid antibodies overlapping in isolated neurological syndrome and multiple sclerosis: neurobiological insights and diagnostic challenges. Front. Cell Neurosci. 13:107. doi: 10.3389/fncel.2019.00107
De Biasi, S., Simone, A. M., Nasi, M., Bianchini, E., Ferraro, D., Vitetta, F., et al. (2016). iNKT cells in secondary progressive multiple sclerosis patients display pro-inflammatory profiles. Front. Immunol. 7:555.
Demoulins, T., Gachelin, G., Bequet, D., and Dormont, D. (2003). A biased Valpha24+ T-cell repertoire leads to circulating NKT-cell defects in a multiple sclerosis patient at the onset of his disease. Immunol. Lett. 90, 223–228. doi: 10.1016/j.imlet.2003.09.014
Denney, L., Kok, W. L., Cole, S. L., Sanderson, S., McMichael, A. J., and Ho, L. P. (2012). Activation of invariant NKT cells in early phase of experimental autoimmune encephalomyelitis results in differentiation of Ly6Chi inflammatory monocyte to M2 macrophages and improved outcome. J. Immunol. 189, 551–557. doi: 10.4049/jimmunol.1103608
Dhodapkar, K. M., Cirignano, B., Chamian, F., Zagzag, D., Miller, D. C., Finlay, J. L., et al. (2004). Invariant natural killer T cells are preserved in patients with glioma and exhibit antitumor lytic activity following dendritic cell-mediated expansion. Int. J. Cancer 109, 893–899. doi: 10.1002/ijc.20050
Dhodapkar, M. V., and Kumar, V. (2017). Type II NKT cells and their emerging role in health and disease. J. Immunol. 198, 1015–1021. doi: 10.4049/jimmunol.1601399
Doherty, D. G., Melo, A. M., Moreno-Olivera, A., and Solomos, A. C. (2018). Activation and regulation of B cell responses by invariant natural killer T cells. Front. Immunol. 9:1360. doi: 10.3389/fimmu.2018.01360
Engel, I., Seumois, G., Chavez, L., Samaniego-Castruita, D., White, B., Chawla, A., et al. (2016). Innate-like functions of natural killer T cell subsets result from highly divergent gene programs. Nat. Immunol. 17, 728–739. doi: 10.1038/ni.3437
Engelen, M., Kemp, S., de Visser, M., van Geel, B. M., Wanders, R. J., Aubourg, P., et al. (2012). X-linked adrenoleukodystrophy (X-ALD): clinical presentation and guidelines for diagnosis, follow-up and management. Orph. J. Rare Dis. 7:51. doi: 10.1186/1750-1172-7-51
Finkelstein, A., Kunis, G., Seksenyan, A., Ronen, A., Berkutzki, T., Azoulay, D., et al. (2011). Abnormal changes in NKT cells, the IGF-1 axis, and liver pathology in an animal model of ALS. PLoS One 6:e22374. doi: 10.1371/journal.pone.0022374
Frohman, E. M., Racke, M. K., and Raine, C. S. (2006). Multiple sclerosis–the plaque and its pathogenesis. N. Engl. J. Med. 354, 942–955. doi: 10.1056/nejmra052130
Fu, R., Shen, Q., Xu, P., Luo, J. J., and Tang, Y. (2014). Phagocytosis of microglia in the central nervous system diseases. Mol. Neurobiol. 49, 1422–1434. doi: 10.1007/s12035-013-8620-6
Fung, T. C., Olson, C. A., and Hsiao, E. Y. (2017). Interactions between the microbiota, immune and nervous systems in health and disease. Nat. Neurosci. 20, 145–155. doi: 10.1038/nn.4476
Furlan, R., Bergami, A., Cantarella, D., Brambilla, E., Taniguchi, M., Dellabona, P., et al. (2003). Activation of invariant NKT cells by alphaGalCer administration protects mice from MOG35-55-induced EAE: critical roles for administration route and IFN-gamma. Eur. J. Immunol. 33, 1830–1838. doi: 10.1002/eji.200323885
Gao, H. (2017). Perspectives on dual targeting delivery systems for brain tumors. J. Neuroimm. Pharmacol. 12, 6–16. doi: 10.1007/s11481-016-9687-4
Gausling, R., Trollmo, C., and Hafler, D. A. (2001). Decreases in interleukin-4 secretion by invariant CD4(-)CD8(-)V alpha 24J alpha Q T cells in peripheral blood of patientswith relapsing-remitting multiple sclerosis. Clin. Immunol. 98, 11–17. doi: 10.1006/clim.2000.4942
Gautron, A. S., Giquel, B., Beaudoin, L., Autrusseau, E., Speak, A., Platt, F., et al. (2010). Invariant NKT cells in adrenoleukodystrophy patients and mice. J. Neuroimmunol. 229, 204–211. doi: 10.1016/j.jneuroim.2010.09.003
Gelderblom, M., Leypoldt, F., Steinbach, K., Behrens, D., Choe, C. U., Siler, D. A., et al. (2009). Temporal and spatial dynamics of cerebral immune cell accumulation in stroke. Stroke 40, 1849–1857. doi: 10.1161/STROKEAHA.108.534503
Gigli, G., Caielli, S., Cutuli, D., and Falcone, M. (2007). Innate immunity modulates autoimmunity: type 1 interferon-beta treatment in multiple sclerosis promotes growth and function of regulatory invariant natural killer T cells through dendritic cell maturation. Immunology 122, 409–417. doi: 10.1111/j.1365-2567.2007.02655.x
Gitler, A. D., Dhillon, P., and Shorter, J. (2017). Neurodegenerative disease: models, mechanisms, and a new hope. Dis. Model Mech. 10, 499–502. doi: 10.1242/dmm.030205
Godfrey, D. I., Le Nours, J., Andrews, D. M., Uldrich, A. P., and Rossjohn, J. (2018). Unconventional T cell targets for cancer immunotherapy. Immunity 48, 453–473. doi: 10.1016/j.immuni.2018.03.009
Godfrey, D. I., Stankovic, S., and Baxter, A. G. (2010). Raising the NKT cell family. Nat. Immunol. 11, 197–206. doi: 10.1038/ni.1841
Herz, J., Filiano, A. J., Smith, A., Yogev, N., and Kipnis, J. (2017). Myeloid cells in the central nervous system. Immunity 46, 943–956. doi: 10.1016/j.immuni.2017.06.007
Hirabayashi, Y. (2012). A world of sphingolipids and glycolipids in the brain–novel functions of simple lipids modified with glucose. Proc. Jpn. Acad. Ser. B Phys. Biol. Sci. 88, 129–143. doi: 10.2183/pjab.88.129
Hunn, M. K., Farrand, K. J., Broadley, K. W., Weinkove, R., Ferguson, P., Miller, R. J., et al. (2012). Vaccination with irradiated tumor cells pulsed with an adjuvant that stimulates NKT cells is an effective treatment for glioma. Clin. Cancer Res. 18, 6446–6459. doi: 10.1158/1078-0432.CCR-12-0704
Iadecola, C., and Anrather, J. (2011). The immunology of stroke: from mechanisms to translation. Nat. Med. 17, 796–808. doi: 10.1038/nm.2399
Illes, Z., Kondo, T., Newcombe, J., Oka, N., Tabira, T., and Yamamura, T. (2000). Differential expression of NK T cell V alpha 24J alpha Q invariant TCR chain in the lesions of multiple sclerosis and chronic inflammatory demyelinating polyneuropathy. J. Immunol. 164, 4375–4381. doi: 10.4049/jimmunol.164.8.4375
Im, J. S., Arora, P., Bricard, G., Molano, A., Venkataswamy, M. M., Baine, I., et al. (2009). Kinetics and cellular site of glycolipid loading control the outcome of natural killer T cell activation. Immunity 30, 888–898. doi: 10.1016/j.immuni.2009.03.022
Jahng, A., Maricic, I., Aguilera, C., Cardell, S., Halder, R. C., and Kumar, V. (2004). Prevention of autoimmunity by targeting a distinct, noninvariant CD1d-reactive T cell population reactive to sulfatide. J. Exp. Med. 199, 947–957. doi: 10.1084/jem.20031389
Jahng, A. W., Maricic, I., Pedersen, B., Burdin, N., Naidenko, O., Kronenberg, M., et al. (2001). Activation of natural killer T cells potentiates or prevents experimental autoimmune encephalomyelitis. J. Exp. Med. 194, 1789–1799. doi: 10.1084/jem.194.12.1789
Kleinschnitz, C., Schwab, N., Kraft, P., Hagedorn, I., Dreykluft, A., Schwarz, T., et al. (2010). Early detrimental T-cell effects in experimental cerebral ischemia are neither related to adaptive immunity nor thrombus formation. Blood 115, 3835–3842. doi: 10.1182/blood-2009-10-249078
Kojo, S., Seino, K., Harada, M., Watarai, H., Wakao, H., Uchida, T., et al. (2005). Induction of regulatory properties in dendritic cells by Valpha14 NKT cells. J. Immunol. 175, 3648–3655. doi: 10.4049/jimmunol.175.6.3648
Kriegsmann, K., Kriegsmann, M., von Bergwelt-Baildon, M., Cremer, M., and Witzens-Harig, M. (2018). NKT cells - new players in CAR cell immunotherapy? Eur. J. Haematol. 101, 750–757. doi: 10.1111/ejh.13170
Kurschus, F. C. (2015). T cell mediated pathogenesis in EAE: molecular mechanisms. Biomed. J. 38, 183–193. doi: 10.4103/2319-4170.155590
Lehmann, J., Hartig, W., Seidel, A., Fuldner, C., Hobohm, C., Grosche, J., et al. (2014). Inflammatory cell recruitment after experimental thromboembolic stroke in rats. Neuroscience 279, 139–154. doi: 10.1016/j.neuroscience.2014.08.023
Liu, D., Jenkins, R. W., and Sullivan, R. J. (2019). Mechanisms of resistance to immune checkpoint blockade. Am. J. Clin. Dermatol. 20, 41–54. doi: 10.1007/s40257-018-0389-y
Liu, D. F., Song, L. P., Brawley, V. S., Robison, N., Wei, J., Gao, X. H., et al. (2013). Medulloblastoma expresses CD1d and can be targeted for immunotherapy with NKT cells. Clin. Immunol. 149, 55–64. doi: 10.1016/j.clim.2013.06.005
Liu, H., Chen, L., Liu, J., Meng, H., Zhang, R., Ma, L., et al. (2017). Co-delivery of tumor-derived exosomes with alpha-galactosylceramide on dendritic cell-based immunotherapy for glioblastoma. Cancer Lett. 411, 182–190. doi: 10.1016/j.canlet.2017.09.022
Liu, R., La Cava, A., Bai, X. F., Jee, Y., Price, M., Campagnolo, D. I., et al. (2005). Cooperation of invariant NKT cells and CD4+CD25+ T regulatory cells in the prevention of autoimmune myasthenia. J. Immunol. 175, 7898–7904. doi: 10.4049/jimmunol.175.12.7898
Mars, L. T., Laloux, V., Goude, K., Desbois, S., Saoudi, A., Van Kaer, L., et al. (2002). Cutting edge: V alpha 14-J alpha 281 NKT cells naturally regulate experimental autoimmune encephalomyelitis in nonobese diabetic mice. J. Immunol. 168, 6007–6011. doi: 10.4049/jimmunol.168.12.6007
Mars, L. T., Mas, M., Beaudoin, L., Bauer, J., Leite-de-Moraes, M., Lehuen, A., et al. (2014). Invariant NKT cells regulate the CD8 T cell response during theiler’s virus infection. PLoS One 9:e87717. doi: 10.1371/journal.pone.0087717
McEwen-Smith, R. M., Salio, M., and Cerundolo, V. (2015). The regulatory role of invariant NKT cells in tumor immunity. Cancer Immunol. Res. 3, 425–435. doi: 10.1158/2326-6066.CIR-15-0062
Meisel, C., and Meisel, A. (2011). Suppressing immunosuppression after stroke. N. Engl. J. Med. 365, 2134–2136. doi: 10.1056/nejmcibr1112454
Miyamoto, K., Miyake, S., and Yamamura, T. (2001). A. synthetic glycolipid prevents autoimmune encephalomyelitis by inducing TH2 bias of natural killer T cells. Nature 413, 531–534. doi: 10.1038/35097097
Nagarajan, N. A., and Kronenberg, M. (2007). Invariant NKT cells amplify the innate immune response to lipopolysaccharide. J. Immunol. 178, 2706–2713. doi: 10.4049/jimmunol.178.5.2706
Nair, S., and Dhodapkar, M. V. (2017). Natural killer T cells in cancer immunotherapy. Front. Immunol. 8:1178. doi: 10.3389/fimmu.2017.01178
Nishioka, Y., Masuda, S., Tomaru, U., and Ishizu, A. (2018). CD1d-restricted type II NKT cells reactive with endogenous hydrophobic peptides. Front. Immunol. 9:548. doi: 10.3389/fimmu.2018.00548
Oh, S. J., and Chung, D. H. (2011). Invariant NKT cells producing IL-4 or IL-10, but not IFN-gamma, inhibit the Th1 response in experimental autoimmune encephalomyelitis, whereas none of these cells inhibits the Th17 response. J. Immunol. 186, 6815–6821. doi: 10.4049/jimmunol.1003916
O’Keeffe, J., Gately, C. M., Counihan, T., Hennessy, M., Leahy, T., Moran, A. P., et al. (2008). T-cells expressing natural killer (NK) receptors are altered in multiple sclerosis and responses to alpha-galactosylceramide are impaired. J. Neurol. Sci. 275, 22–28. doi: 10.1016/j.jns.2008.07.007
Paget, C., Mallevaey, T., Speak, A. O., Torres, D., Fontaine, J., Sheehan, K. C., et al. (2007). Activation of invariant NKT cells by toll-like receptor 9-stimulated dendritic cells requires type I interferon and charged glycosphingolipids. Immunity 27, 597–609. doi: 10.1016/j.immuni.2007.08.017
Pan, J., Konstas, A. A., Bateman, B., Ortolano, G. A., and Pile-Spellman, J. (2007). Reperfusion injury following cerebral ischemia: pathophysiology, MR imaging, and potential therapies. Neuroradiology 49, 93–102. doi: 10.1007/s00234-006-0183-z
Parekh, V. V., Wu, L., Olivares-Villagomez, D., Wilson, K. T., and Van Kaer, L. (2013). Activated invariant NKT cells control central nervous system autoimmunity in a mechanism that involves myeloid-derived suppressor cells. J. Immunol. 190, 1948–1960. doi: 10.4049/jimmunol.1201718
Price, D. L., Cork, L. C., Struble, R. G., Kitt, C. A., Walker, L. C., Powers, R. E., et al. (1987). Dysfunction and death of neurons in human degenerative neurological diseases and in animal models. Ciba Found. Symp. 126, 30–48. doi: 10.1002/9780470513422.ch3
Pyaram, K., and Yadav, V. N. (2018). Advances in NKT cell immunotherapy for glioblastoma. J. Cancer Sci. Ther. 10:533.
Qian, G., Qin, X., Zang, Y. Q., Ge, B., Guo, T. B., Wan, B., et al. (2010). High doses of alpha-galactosylceramide potentiate experimental autoimmune encephalomyelitis by directly enhancing Th17 response. Cell Res. 20, 480–491. doi: 10.1038/cr.2010.6
Ransohoff, R. M., and Brown, M. A. (2012). Innate immunity in the central nervous system. J. Clin. Invest. 122, 1164–1171. doi: 10.1172/jci58644
Ricarte, I. F., Dutra, L. A., Abrantes, F. F., Toso, F. F., Barsottini, O. G. P., Silva, G. S., et al. (2018). Neurologic manifestations of antiphospholipid syndrome. Lupus 27, 1404–1414. doi: 10.1177/0961203318776110
Sakuishi, K., Miyake, S., and Yamamura, T. (2010). Role of NK cells and invariant NKT cells in multiple sclerosis. Results Probl. Cell Differ. 51, 127–147. doi: 10.1007/400_2009_11
Sanmamed, M. F., and Chen, L. (2018). A paradigm shift in cancer immunotherapy: from enhancement to normalization. Cell 175, 313–326. doi: 10.1016/j.cell.2018.09.035
Schreiber, K., Sciascia, S., de Groot, P. G., Devreese, K., Jacobsen, S., Ruiz-Irastorza, G., et al. (2018). Antiphospholipid syndrome. Nat. Rev. Dis. Primers 4:18005. doi: 10.1038/nrdp.2018.5
Schwartz, M., and Deczkowska, A. (2016). Neurological disease as a failure of brain-immune crosstalk: the multiple faces of neuroinflammation. Trends Immunol. 37, 668–679. doi: 10.1016/j.it.2016.08.001
Shimizu, H., Matsuguchi, T., Fukuda, Y., Nakano, I., Hayakawa, T., Takeuchi, O., et al. (2002). Toll-like receptor 2 contributes to liver injury by Salmonella infection through Fas ligand expression on NKT cells in mice. Gastroenterology 123, 1265–1277. doi: 10.1053/gast.2002.36006
Singh, A. K., Wilson, M. T., Hong, S., Olivares-Villagomez, D., Du, C., Stanic, A. K., et al. (2001). Natural killer T cell activation protects mice against experimental autoimmune encephalomyelitis. J. Exp. Med. 194, 1801–1811. doi: 10.1084/jem.194.12.1801
Skaper, S. D., Facci, L., Zusso, M., and Giusti, P. (2018). An inflammation-centric view of neurological disease: beyond the neuron. Front. Cell Neurosci. 12:72. doi: 10.3389/fncel.2018.00072
Stax, A. M., Tuengel, J., Girardi, E., Kitano, N., Allan, L. L., Liu, V., et al. (2017). Autoreactivity to sulfatide by human invariant NKT Cells. J. Immunol. 199, 97–106. doi: 10.4049/jimmunol.1601976
Sun, B. L., Wang, L. H., Yang, T., Sun, J. Y., Mao, L. L., Yang, M. F., et al. (2018). Lymphatic drainage system of the brain: a novel target for intervention of neurological diseases. Prog. Neurobiol. 16, 118–143. doi: 10.1016/j.pneurobio.2017.08.007
Suzuki, Y., Onodera, H., Tago, H., Saito, R., Ohuchi, M., Shimizu, M., et al. (2005). Altered populations of natural killer cell and natural killer T cell subclasses in myasthenia gravis. J. Neuroimmunol. 167, 186–189. doi: 10.1016/j.jneuroim.2005.06.015
Taniguchi, M., Seino, K., and Nakayama, T. (2003). The NKT cell system: bridging innate and acquired immunity. Nat. Immunol. 4, 1164–1165. doi: 10.1038/ni1203-1164
Teige, A., Teige, I., Lavasani, S., Bockermann, R., Mondoc, E., Holmdahl, R., et al. (2004). CD1-dependent regulation of chronic central nervous system inflammation in experimental autoimmune encephalomyelitis. J. Immunol. 172, 186–194. doi: 10.4049/jimmunol.172.1.186
Teo, W. Y., Elghetany, M. T., Shen, J., Man, T. K., Li, X., Chintagumpala, M., et al. (2014). Therapeutic implications of CD1d expression and tumor-infiltrating macrophages in pediatric medulloblastomas. J. Neurooncol. 120, 293–301. doi: 10.1007/s11060-014-1572-5
Thiruppathi, M., Rowin, J., Li Jiang, Q., Sheng, J. R., Prabhakar, B. S., and Meriggioli, M. N. (2012). Functional defect in regulatory T cells in myasthenia gravis. Ann. N.Y. Acad. Sci. 1274, 68–76. doi: 10.1111/j.1749-6632.2012.06840.x
van der Vliet, H. J., von Blomberg, B. M., Nishi, N., Reijm, M., Voskuyl, A. E., van Bodegraven, A. A., et al. (2001). Circulating V(alpha24+) Vbeta11+ NKT cell numbers are decreased in a wide variety of diseases that are characterized by autoreactive tissue damage. Clin. Immunol. 100, 144–148. doi: 10.1006/clim.2001.5060
Van Kaer, L., Wu, L., and Parekh, V. V. (2015). Natural killer T cells in multiple sclerosis and its animal model, experimental autoimmune encephalomyelitis. Immunology 146, 1–10. doi: 10.1111/imm.12485
Wang, Y. H., Jia, J. C., Liu, G., and Wang, Y. F. (2015). Research on the influence of alpha-GalCer activating experimental autoimmune myasthenia gravis mice NKT cells at different times on myasthenia gravis. J. Biol. Regul. Homeost. Agents 29, 195–200.
Wang, Z. K., Xue, L., Wang, T., Wang, X. J., and Su, Z. Q. (2016). Infiltration of invariant natural killer T cells occur and accelerate brain infarction in permanent ischemic stroke in mice. Neurosci. Lett. 633, 62–68. doi: 10.1016/j.neulet.2016.09.010
Wermeling, F., Lind, S. M., Jordo, E. D., Cardell, S. L., and Karlsson, M. C. (2010). Invariant NKT cells limit activation of autoreactive CD1d-positive B cells. J. Exp. Med. 207, 943–952. doi: 10.1084/jem.20091314
Wong, C. H., Jenne, C. N., Lee, W. Y., Leger, C., and Kubes, P. (2011). Functional innervation of hepatic iNKT cells is immunosuppressive following stroke. Science 334, 101–105. doi: 10.1126/science.1210301
Wong, C. H., Jenne, C. N., Tam, P. P., Leger, C., Venegas, A., Ryckborst, K., et al. (2017). Prolonged activation of invariant natural killer T cells and TH2-skewed immunity in stroke patients. Front. Neurol. 8:6. doi: 10.3389/fneur.2017.00006
Keywords: nature killer T cells, ischemic stroke, multiple sclerosis, brain tumor, neurodegenerative disease
Citation: Cui Y and Wan Q (2019) NKT Cells in Neurological Diseases. Front. Cell. Neurosci. 13:245. doi: 10.3389/fncel.2019.00245
Received: 06 April 2019; Accepted: 16 May 2019;
Published: 29 May 2019.
Edited by:
Silvia Sánchez-Ramón, Complutense University of Madrid, SpainReviewed by:
Luc Van Kaer, Vanderbilt University, United StatesRicardo Constantino Ginestal, Hospital Clínico San Carlos, Spain
Copyright © 2019 Cui and Wan. This is an open-access article distributed under the terms of the Creative Commons Attribution License (CC BY). The use, distribution or reproduction in other forums is permitted, provided the original author(s) and the copyright owner(s) are credited and that the original publication in this journal is cited, in accordance with accepted academic practice. No use, distribution or reproduction is permitted which does not comply with these terms.
*Correspondence: Qi Wan, cXdhbndoQGhvdG1haWwuY29t