- 1Department of Biosystems Science and Engineering, ETH Zürich, Basel, Switzerland
- 2MaxWell Biosystems AG, Basel, Switzerland
Axons convey information in neuronal circuits via reliable conduction of action potentials (APs) from the axon initial segment (AIS) to the presynaptic terminals. Recent experimental findings increasingly evidence that the axonal function is not limited to the simple transmission of APs. Advances in subcellular-resolution recording techniques have shown that axons display activity-dependent modulation in spike shape and conduction velocity, which influence synaptic strength and latency. We briefly review here, how recent methodological developments facilitate the understanding of the axon physiology. We included the three most common methods, i.e., genetically encoded voltage imaging (GEVI), subcellular patch-clamp and high-density microelectrode arrays (HD-MEAs). We then describe the potential of using HD-MEAs in studying axonal physiology in more detail. Due to their robustness, amenability to high-throughput and high spatiotemporal resolution, HD-MEAs can provide a direct functional electrical readout of single cells and cellular ensembles at subcellular resolution. HD-MEAs can, therefore, be employed in investigating axonal pathologies, the effects of large-scale genomic interventions (e.g., with RNAi or CRISPR) or in compound screenings. A combination of extracellular microelectrode arrays (MEAs), intracellular microelectrodes and optical imaging may potentially reveal yet unexplored repertoires of axonal functions.
Introduction
Intricate operations, performed by neuronal networks, emerge from the orchestrated interplay of individual neurons. Neurons use action potentials (APs) as a means to encode and relay information from the soma to the presynaptic terminal via reliable conduction through the axon. The three functional compartments of the axon include the axon initial segment (AIS), the axon proper, and the presynaptic terminal. Somato-dendritic integration of a number of synaptic inputs at the AIS are thought to shape the AP firing patterns. The axon proper is often conceived as a simple cable, whose function is the faithful transmission of the AP to distant presynaptic terminals in a digital (all or none) fashion. However, with the development of modern techniques that can directly access small axonal structures, an increasing body of work has emerged that challenges the traditional view on the role of the axon being purely limited to the transmission of the AP(Debanne et al., 2011; Sasaki et al., 2011; Sasaki, 2013; Bucher, 2016). It has been shown that the shape of the presynaptic AP can be modulated by subthreshold potentials, which, in turn, modulate the spike-evoked transmission through so-called “analog-digital facilitation” (Debanne, 2004; Alle and Geiger, 2008; Kress and Mennerick, 2009; Bucher and Goaillard, 2011; Debanne et al., 2011; Sasaki, 2013; Bucher, 2016). As AP propagation and synaptic transmission might undergo substantial modulation, the computational repertoires of single axons in the neuronal circuit may be more complex than commonly assumed.
Axonal membrane excitability and conduction velocity can change substantially with repeated activation. This can potentially alter the temporal patterns of spikes during propagation from the AIS to presynaptic sites. Such changes in temporal spike patterns may be an important feature of neural coding strategies (Izhikevich, 2006; Bucher and Goaillard, 2011; Bucher, 2016). Axonal conduction velocity in unmyelinated axons depends on several biophysical factors, such as ion-channel densities and kinetics, membrane capacitance, axial resistance, axon geometry, and, for myelinated axons, myelin thickness and internodal distances (Hodgkin, 1954; Manor et al., 1991; Shepherd and Harris, 1998; Ganguly et al., 2000; Fields, 2005; Cai et al., 2011). Axonal conduction velocity per se provides little information about the functional aspects of neuronal communication. On the other hand, axonal conduction delay, which depends on both, conduction velocity and axonal length, may have important functional implications in the integration of sensory information (Konishi, 2003). A plethora of diverse neurological disorders is associated with impaired axonal functionality (Suter and Scherer, 2003; Waxman, 2006; Krarup and Moldovan, 2009; Kullmann, 2010; Egawa et al., 2017; Khalilpour et al., 2017). Axonal dysfunction can be caused by missing or reduced myelination (e.g., multiple sclerosis) (Steinman, 1996; Trapp et al., 1999). Acute axonal damage (e.g., traumatic injury) (Smith and Meaney, 2000; Johnson V.E. et al., 2013), toxic entities, aggregated proteins, microgliosis and disrupted axonal transport (e.g., prion disease, Parkinson’s disease, Alzheimer’s disease) (Liberski and Budka, 1999; Millecamps and Julien, 2013) may directly affect axonal physiology. Lastly, abnormalities in the composition or function of ion channels (in channelopathies, e.g., in certain forms of migraine and epilepsy) are known to alter the conduction properties of axons (Goadsby et al., 2017; Oyrer et al., 2018; Pietrobon, 2018).
Recent advances in the understanding of axon physiology and pathophysiology have been driven by technological developments, such as optical imaging of the membrane potentials using genetically encoded voltage indicators (GEVI), subcellular patch-clamp recordings from thin axons and boutons, and high-density microelectrode array recordings (HD-MEA) (Figure 1; Shu et al., 2006; Kole et al., 2007; Sasaki et al., 2011; Bakkum et al., 2013; Novak et al., 2013; Hoppa et al., 2014; Kawaguchi and Sakaba, 2015; Müller et al., 2015; Rama et al., 2015a; Radivojevic et al., 2017). These techniques stand apart from other classical electrophysiological methods in their ability to monitor and interactively control subcellular components of single neurons at high spatial and microsecond temporal resolution. The above-mentioned techniques and devices were reviewed in-depth elsewhere (Hierlemann et al., 2011; Sasaki, 2013; Spira and Hai, 2013; Obien et al., 2015; Inagaki and Nagai, 2016; Ohura and Kamiya, 2016; Yang and St-Pierre, 2016; Xu et al., 2017; Zeck et al., 2017; Platisa and Pieribone, 2018; Rama et al., 2018; Wang et al., 2019). Here, we will briefly introduce these technologies with the focus on studying axonal signals, while we describe - in a bit more detail - recent investigations in axonal neurobiology by using HD-MEAs.
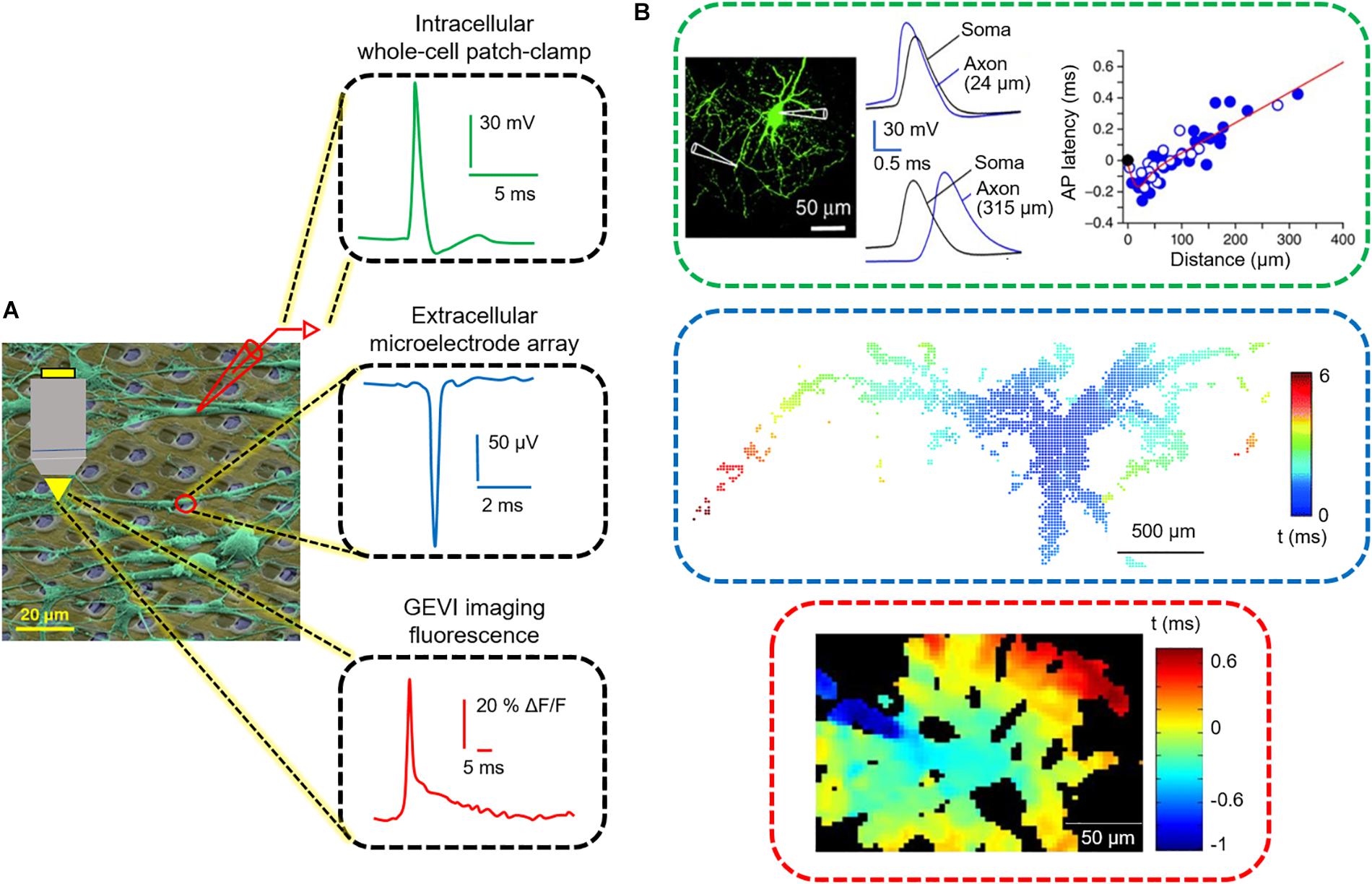
Figure 1. Three functional readouts for measuring neuronal and axonal activity. (A) Colored scanning electron micrograph of neurons (green) cultured on a CMOS HD-MEA chip. The readouts include, from top to bottom, patch pipettes, microelectrodes and fluorescence microscopy. At the right side, an intracellular action potential (AP), recorded by using the patch-clamp technique, an extracellular AP recorded by an HD-MEA, and an AP-induced fluorescence signal using GEVIs are displayed. (B) Tracking of AP propagation in neuronal processes performed by the three techniques displayed in (A). Modified with permissions from Hochbaum et al., 2014; Hu and Jonas, 2014; Müller et al., 2015.
Technological Approaches
In the following, we provide a brief overview of the three most commonly used recording modalities for measuring axonal signals. We describe the parameters that govern to the detection of neuronal signals and outline recent advances, including potential advantages and limitations. Table 1 lists the key specifications of each methodology. Since several publications are available on each technology, we pooled the data from the most advanced and most recent publications. Given the plethora of applications for each methodology, we will restrict our comparison to the detection and measurements of AP propagation. A schematic overview on the different methodologies along with representative signals is displayed in Figure 1.
Voltage Imaging
In order to monitor neural activity at single-cell resolution, optical methods, such as voltage-sensitive dyes and GEVI, make use of fluorescence signals to detect alterations in voltage (Peterka et al., 2011; Storace et al., 2016). Voltage-sensitive dyes have provided important insights into neuronal electrical signaling ranging from individual neurons to population dynamics (Grinvald et al., 1981; Gross and Loew, 1989; Petersen et al., 2003; Miller et al., 2012; Popovic et al., 2015). Yet, major limitations of voltage-sensitive dyes include cell toxicity, phototoxicity, indiscriminate neuronal and glial staining, and small signal-to-noise ratio (SNR) (Knöpfel et al., 2006; Mennerick et al., 2010).
In the last two decades, considerable efforts have been made to overcome these limitations, which have led to the development of GEVIs. The three main molecular designs of GEVIs – inserted into the plasma membrane – are (1) the fusion of fluorescent proteins (FP) to voltage-sensing domains, (2) the use of opsins, and (3) hybrid opsin-FP pairs (rhodopsin-FRET sensor) (Boyden et al., 2005; Kralj et al., 2011; Akemann et al., 2012; Jin et al., 2012; Tsutsui et al., 2013; Hochbaum et al., 2014; St-Pierre et al., 2014; Gong et al., 2015). Voltage sensitivity (dynamic range, %) is an important parameter of fluorescence indicators, expressed as ΔF/F per 100 mV (−70 to 30 mV), which represents linear changes in fluorescence in response to voltage fluctuations. To detect neuronal activity with high SNR, a combination of key features of voltage indicators, such as bright fluorescence, fast kinetics (rapid response to changes in voltage), large dynamic range, photostability, and efficient plasma membrane localization is desired (Lee et al., 2017; Xu et al., 2017; Piatkevich et al., 2018; Wang et al., 2019).
While there is constant development of new constructs, a recent work by Bando et al. (2019) provided a temporal snapshot of state-of-the-art GEVIs and compared their performances. The authors report that QuasAr2, a rhodopsin-based GEVI, outperforms other GEVIs concerning the optical detection of single APs of neurons in vitro, featuring high signal amplitude, fast kinetics and high SNR. In addition, Ace-2N-4AA-mNeon, a rhodopsin-FRET sensor, was shown to resolve individual spikes in single trials without averaging, while suffering from fast photobleaching.
A combination of a red-light-excited QuasAr2 with a spectrally compatible blue-light-activated channelrhodopsin (CheRiff) was co-expressed in neurons via a vector called “Optopatch,” which was targeted at enabling simultaneous all-optical electrophysiology in neuronal cultures or organotypic brain slice cultures (Hochbaum et al., 2014; Kiskinis et al., 2018). The use of this construct enabled mapping of the dynamics of AP initiation and propagation across dendritic and axonal structures at high spatiotemporal resolution (Figure 1). However, significant multi-trial averaging (200–17,000 trials) was required for attaining good enough signal-to-noise characteristics (Hochbaum et al., 2014).
Subcellular Patch-Clamp Recordings
Patch-clamping is the gold standard technique for studying electrical properties of neurons at unprecedented resolution. The patch-clamp technique uses a glass micropipette that presses against the cell membrane to form a tight gigaohm seal resistance between the cell membrane and the rim of the glass micropipette. In the original cell-attached configuration, activity of single ion channels in the tiny patch of membrane surrounded by the tip of the pipette can be studied. If the patch of membrane under the pipette tip is ruptured by applying pressure, the electrode accesses the inside of the cell in the so-called whole-cell configuration, where the trans-membrane voltage and currents can be directly recorded (Neher and Sakmann, 1976; Ogden and Stanfield, 1994).
Most patch-clamp studies have been conducted on the soma, which is the largest compartment of a neuron (8–30 μm in diameter). One of the limitations of the conventional patch-clamp technique is that the studies on axons encountered technical difficulties due to the thin axonal structure (∼200 nm in diameter). Accordingly, axonal recordings have been mostly restricted to giant axon terminals, such as hippocampal mossy fiber boutons (Geiger and Jonas, 2000; Bischofberger et al., 2006; Boudkkazi et al., 2011) and the Calyx of Held (Forsythe, 1994; Borst et al., 1995; Awatramani et al., 2005). Recordings from thin axons have been obtained from axonal blebs (3–6 μm), which are resealed swellings at the cut ends of axons after brain slicing procedures (Shu et al., 2006, 2007; Kole et al., 2007; Kim, 2014; Rama et al., 2015a). Recently, recordings from intact thin axons have been made possible using a fluorescence-guided patch-clamp technique (Ishikawa et al., 2010; Sasaki et al., 2011, 2012; Hu and Jonas, 2014; Kawaguchi and Sakaba, 2015). Cell-attached extracellular recordings of APs in intact unmyelinated axons (∼1 μm in diameter) have been made using pipettes coated with fluorescently conjugated albumin. However, stable recording was possible for only less than 60 min with ∼50% success rate. Simultaneous whole-cell recordings have been performed from the soma and axon shaft of hippocampal basket cells in acute slices (Hu and Jonas, 2014) as well as in the presynaptic terminals in cerebellar Purkinje cells in cultures (Kawaguchi and Sakaba, 2015) to examine the fidelity of AP propagation.
In recent years, several studies were conducted using paired recordings from two distinct sites along a single axon or from a presynaptic axon terminal and a postsynaptic neuron. These experiments have made considerable contributions to understanding the mechanism of analog-digital facilitation, compartmentalized distribution of ion channels and gating properties, as well as the modulation of short- and long-term synaptic plasticity (Engel and Jonas, 2005; Alle and Geiger, 2008; Sasaki et al., 2011; Hu and Jonas, 2014; Kawaguchi and Sakaba, 2015; Rama et al., 2015b; Rowan et al., 2016). However, due to the limitation in simultaneously recording from multiple sites along the axon, the patch-clamp technique is not capable of tracking the modulation of AP propagation throughout the axon proper.
CMOS HD-MEAs
The electrical activity of neurons can also be detected extracellularly by means of metal electrodes, arranged in large arrays and known as MEAs. Microelectrodes can record changes in the electric field generated by the moving ions in the extracellular space during the electrical activity of a nearby neuron (Buzsáki et al., 2012; Anastassiou et al., 2013). During an AP, the fast Na+ current flows away from the electrode into the cell and results in a negative peak in the extracellular action potential (EAP). Thereafter, a slower current of K+ ions flows out of the cell toward the electrode resulting in a positive peak. Most axonal signals show a stereotypical positive-first, triphasic shape. The first, small amplitude positive peak corresponds to a capacitive current, the large negative peak to the Na+ current, and the final positive peak to the K+ current (Gold et al., 2006). In general, EAPs show heterogeneity in signal shapes and amplitudes depending on the magnitude, polarity, and the distance from the recording site (Nunez and Srinivasan, 2006). In addition, the relative positioning of cells with respect to the location of electrodes has a strong influence on the amplitude of the EAP (Gold et al., 2006). EAPs signal amplitudes are in the range of μV and are usually around three orders of magnitude lower than intracellularly measured signals (mV) (Buzsáki et al., 2012).
Commercially available standard MEAs are an established technology for investigating neuronal network activity. However, they do not allow for targeting individual neurons in a network due to the limited number of electrodes (<300), arranged at a comparably large pitch (>30 μm) (Gross et al., 1993; Jimbo and Robinson, 2000; Stett et al., 2003; Rutten et al., 2007). In order to investigate the properties of individual neurons, CMOS (complementary metal oxide semiconductor)-technology-based, planar, HD-MEAs can be used that enable simultaneous recording from a large number of sites at high spatiotemporal resolution (Eversmann et al., 2003; Berdondini et al., 2009; Frey et al., 2009; Huys et al., 2012; Johnson B. et al., 2013; Bertotti et al., 2014; Jackel et al., 2017; Ogi et al., 2017; Tsai et al., 2017). In contrast to a full-frame readout that is also used with CMOS cameras, our lab has developed a flexible readout approach, where a matrix of switches below the electrodes (total number: 26,000–59,000 electrodes) routes arbitrarily selectable subsets of 1024 or 2048 electrodes to a high-end readout circuitry placed outside the electrode array. This flexible readout approach enables (i) high spatial resolution with electrode densities of 3,000 to 5,000 electrodes per mm2 at (ii) good signal quality. However, not all the electrodes can be simultaneously read out, but only subsets in a sequential approach [for technical details, cf. (Ballini et al., 2014; Müller et al., 2015; Dragas et al., 2017; Viswam et al., 2018; Yuan et al., 2018)].
The main advantage of the HD-MEAs is the high spatiotemporal resolution, which allows for detection of signals from thin axons (∼200 nm diameter) and the ability to record APs at microsecond temporal resolution. This degree of resolution helps to efficiently assign detected extracellular spikes to units or neurons through spike sorting (Einevoll et al., 2012; Diggelmann et al., 2018). HD-MEAs enable, owing to the high spatial resolution and non-invasive detection of EAPs, the simultaneous recording at EAPs at hundreds of sites simultaneously along the axonal arbor for up to several days (Bakkum et al., 2013; Radivojevic et al., 2017). CMOS-based HD-MEAs have been used with many in vitro preparations, such as dissociated cell cultures (Maccione et al., 2012; Bakkum et al., 2013; Yada et al., 2016; Amin et al., 2017; Radivojevic et al., 2017), cultures of induced pluripotent stem cells (iPSCs) (Amin et al., 2016; Fiscella et al., 2018), acute retinae (Menzler and Zeck, 2011; Fiscella et al., 2012; Jones et al., 2015), acute brain slices (Egert et al., 2002; Frey et al., 2009; Ferrea et al., 2012; Medrihan et al., 2014; Obien et al., 2019), and organotypic brain slice cultures (Gong et al., 2016). A disadvantage of HD-MEAs is that inferences with respect to analog signaling are difficult, as the subthreshold signals are not directly measurable.
Studies of Axonal Neurobiology Using HD-MEAs
The possibility to stimulate and record from a single axon, simultaneously at multiple spatial locations, enables to study axonal electrical properties in great detail. Capitalizing on this capability, our group investigated the possibilities to study neuronal cultures by using a combination of HD-MEA recordings, electrical extracellular stimulations, live staining of neurons directly on the HD-MEA, and patch-clamping of targeted individual neurons. For a long time, the soma and dendrites have been considered the main contributors to the EAP landscape, since most electrophysiological measurements, e.g., through whole-cell patch-clamp, have been done at the soma. Although the initiation of APs has been known to occur at the distal AIS (Kole et al., 2008; Hu et al., 2009; Foust et al., 2010; Popovic et al., 2011; Baranauskas et al., 2013; Debanne and Garrido, 2018; Leterrier, 2018), the contribution of axons to the EAP landscape has been assumed to be small, if not negligible due to the small dimensions of axonal structures – in contrast to the soma and dendrites. In order to investigate the contribution of different neuronal compartments to the EAP spatial landscape in detail, our group has used HD-MEAs to electrically image EAPs of cultured cortical neurons and of Purkinje cells in acute cerebellar slices (Bakkum et al., 2018). By using spike-triggered averaging, the EAP landscapes of more than 50 neurons were measured and compared to fluorescence images of the respective neuronal cells (Bakkum et al., 2013). We found that the largest measured EAP signal amplitudes originated from the AIS, instead of the soma. The dominant EAP signals, found at the AIS, featured negative polarity (charges entering the cell), while some EAP signals found in nearby dendrites had positive polarity (return currents or charges exiting the cell) (Figures 2A,B). These findings are relevant in interpreting results obtained with extracellular recording schemes (in vitro and in vivo), for setting up compartmental neuron models, and for developing methods to study the function of the AIS in healthy and diseased cellular cultures.
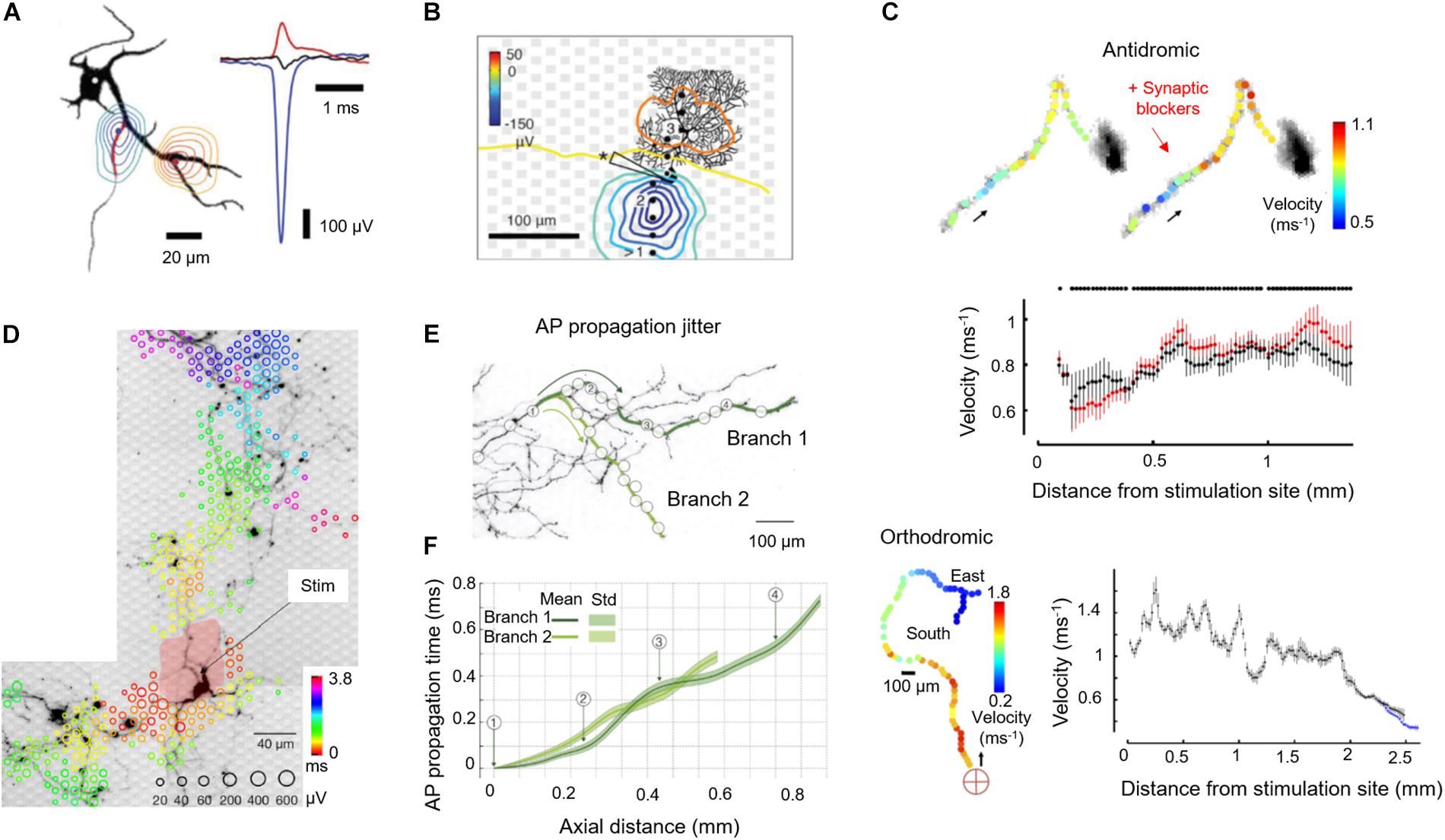
Figure 2. Application of HD-MEAs to study axon neurobiology. (A) (Left) Contour plots of the minimum (blue) or maximum (red) extracellular-action-potential (EAP) signal within ± 500 μs of the negative peak. The AIS has been marked on the MAP2 fluorescence image in the background (black) with a red line (ankyrin-G staining). The contours have been normalized to the largest negative signal (blue-to-green) or the largest positive signal (red-to-yellow), see right panel. (Right) The largest negative (blue) and positive EAP signals (red) along with the somatic potentials (black) are shown as peaks. (B) Spatial distribution of the averaged spontaneous EAP of a Purkinje cell (PC). The largest EAP amplitudes were found along the axon of the PC. (C) (Top) Velocity profiles (color) along the propagation pathway without (left) and with (right) application of synaptic blockers. Arrows indicate the antidromic propagation direction. (Middle) Velocities without (black) and with (red) application of synaptic blockers calculated by using a bootstrapping procedure. (Bottom) The same analysis was performed for an orthodromic action potential. The red cross indicates the stimulation electrode located near the soma. Propagation continued into two branches (“East” and “South”). (D) Stimulation-triggered EAP footprint superimposed with neuronal morphology, revealed by live-cell imaging using lipofection. Circle sizes indicate logarithmically scaled amplitudes of triggered APs, whereas colors indicate the occurrence times of the negative AP peaks relative to the stimulation time. The black arrow points to the stimulation electrode for orthodromic stimulation, whereas the pale red patch indicates the area affected by the stimulation artifact. (E) Two axonal branches, labeled “Branch 1” and “Branch 2,” are marked by dark-green and light-green lines on a fluorescence image. White circles indicate the positions of the used recording electrodes. (F) AP propagation times obtained from the two branches: average propagation times are presented by solid lines; the standard deviations of the propagation times are represented by the pale bands in the background. Except Panel B, which is an acute cerebellar slice preparation, all other panels refer to cortical neuronal cultures. Images have been adapted with permissions from Bakkum et al., 2013, 2018 (A–C), Radivojevic et al., 2016, 2017 (D–F).
A characteristic parameter of axons is the conduction velocity, which determines how fast information is transferred between neurons. Detecting fluctuations or deviations in conduction velocity along the axon can provide an understanding of factors that affect conduction success or failure. Such detection poses a major challenge, as it requires a method to directly measure AP propagation at several points along the axon. Several groups have utilized PDMS tunnels, combined with MEAs, to confine the axons, to increase SNR (higher electrical resistance along the channels) and to track the AP propagation along axons and axonal bundles (Shimba et al., 2014; Lewandowska et al., 2015; Habibey et al., 2017). Our group used stimulus-triggered averaging of EAPs to precisely measure the propagation of APs and quantify the conduction velocity along axonal branches (Bakkum et al., 2013). In general, the velocity of AP propagation along axons of wild-type primary rodent neuron cultures increases with age, as observed in our experiments and reported by other authors (Bakkum et al., 2013; Habibey et al., 2017). Both, antidromic (toward the soma) and orthodromic (away from the soma) AP propagations featured variations in conduction velocity along the axon (Figure 2C). The variations persisted upon application of synaptic blockers (100 μM APV, 10 μM CNQX, and 50 μM bicuculline methiodide), suggesting that variations in ion-channel properties and densities influence the conduction properties of axons, among others factors. Moreover, higher conduction velocities were observed in axonal segments closer to the soma as compared to the putatively thinner distal branches, which is in agreement with the theory that the action-potential propagation velocity is inversely proportional to the axon diameter (Goldstein and Rall, 1974). Pathological conditions affecting the axon may cause conduction delays, so that the capability to measure axonal signal propagation may allow for phenotyping cell cultures or brain slices that are characteristic of brain disorders and for identifying pharmacological effects.
The electrical properties of neurons, including their susceptibility to extracellular electrical stimulation, are highly variable across their morphology, so that stimulation efficiency with extracellular electrodes will strongly depend on where the neuron is stimulated. By combining optical imaging and electrically multisite stimulation, we could determine the electrical stimulation profiles of single neurons (Radivojevic et al., 2016). The AIS, the axonal arbor, and proximal somatodendritic compartments could be identified as prime stimulation targets (Figure 2D). Stimulation at the AIS required low voltages and provided immediate, selective and reliable neuronal activation, whereas stimulation at the soma required high voltages and produced delayed and unreliable responses. Subthreshold stimulation at the soma depolarized the somatic membrane potential without eliciting APs. These findings provided a strategy to stimulate individual neurons with high specificity, by first measuring their EAP footprint to determine the likely location of their AIS (region of highest signal amplitudes) for subsequent electrical stimulation with low voltages.
A property of axons, which is of high biological relevance but is very hard to experimentally investigate, is the temporal precision with which axons conduct APs. The dendritic integration depends on the timing of incoming postsynaptic potentials. However, determining the temporal precision of axonal conduction, again, requires experimental access to a single axon at multiple locations and requires resolving single APs. As discussed previously, most recording modalities lack either the spatial or temporal resolution, or they rely on averaging many APs. Averaging, however, is not an option, when the timing of individual APs needs to be estimated. We demonstrated a method to non-invasively and directly record individual APs propagating along axons at microsecond temporal resolution using HD-MEA recordings and a template-matching technique relying on multi-electrode templates. We were able to detect individual APs propagating across entire neurons including axonal terminals, which were hundreds of micrometers away from the AIS by using optimized matched filters (Radivojevic et al., 2017). We found that cortical axons conduct single APs with high temporal precision and reliability. Individual APs travel along 1 mm of axons with a fixed travel time ± 100 μs, and we did not observe any conduction or branch-point failure in more than 8,000,000 recorded APs (Figures 2E,F).
Discussion and Outlook
Based on the studies reviewed above, we think that HD-MEAs constitute a versatile tool to investigate neuronal information processing and axonal signaling. The possibility to conduct long-term, simultaneous, multisite recordings at high spatiotemporal resolution renders HD-MEAs an ideal technology for detailed characterizations of neurons and axons. HD-MEAs can be used to study alterations in axonal signal propagation and the effects of brain disorders on axonal signaling, as they provide a direct functional readout. Examples include the assessment of the effects of mutations in voltage-gated ion channels (e.g., in the case of channelopathies) on signal propagation velocity. The possibility to reliably electrically stimulate neurons at high frequencies can be used to study the modulation of axonal APs and the mechanisms of axonal conduction failures during repetitive neuronal activation (Geiger and Jonas, 2000; Debanne, 2004; Boudkkazi et al., 2011). Furthermore, spatiotemporal aspects of analog-digital integration in axonal signals can be investigated (Alle and Geiger, 2006; Shu et al., 2006).
HD-MEAs can be arranged in a multi-well-plate format to realize high-throughput as required, e.g., for large-scale genomic interventions (RNAi or CRISPR) or compound screenings, using human iPSC-derived neurons to model neurological diseases. HD-MEAs enable access to a variety of electrophysiological parameters, including axonal properties, which can be used for characterizing functional phenotypes of neurological disease models. Therefore, HD-MEAs can be used as a platform for drug screening, pre-clinical diagnostics and will find applications in the evolving landscape of precision medicine.
From a technological perspective, it is conceivable that only the combination of different recording modalities will substantially increase the number of applications. Extracellular, intracellular and optical readouts can be combined to determine how they can complement each other. For example, subthreshold voltage distributions can be optically visualized while simultaneously measuring axonal signals throughout the axonal arbors using HD-MEAs. Such an approach will allow for deciphering effects of axonal and synaptic plasticity in neuronal networks and provide functional insights into axon physiology and, possibly, pathophysiology. In particular, it may become possible in the future to better understand the functional underpinnings of clinically heterogeneous diseases that arise from axonal disturbances.
Author Contributions
VE, FF, MO, and AH wrote the manuscript. VE built the figures.
Funding
This work was supported by the European Community through the European Research Council Advanced Grants 694829 “neuroXscales” (Horizon 2020) and the Swiss National Science Foundation Grant 205321_157092/1 (“Axons”). FF received individual support by the Swiss National Science Foundation “Ambizione” Grant PZ00P3_167989. The funders had no role in study design, data collection and analysis, decision to publish, or preparation of the manuscript.
Conflict of Interest Statement
The authors declare that the research was conducted in the absence of any commercial or financial relationships that could be construed as a potential conflict of interest.
Acknowledgments
M. Emmenegger and E. J. Rushing, both at the University of Zurich, are acknowledged for their help with the manuscript and proofreading.
References
Akemann, W., Mutoh, H., Perron, A., Park, Y. K., Iwamoto, Y., and Knöpfel, T. (2012). Imaging neural circuit dynamics with a voltage-sensitive fluorescent protein. J. Neurophysiol. 108, 2323–2337. doi: 10.1152/jn.00452.2012
Alle, H., and Geiger, J. R. P. (2006). Combined analog and action potential coding in hippocampal mossy fibers. Science 311, 1290–1293. doi: 10.1126/science.1119055
Alle, H., and Geiger, J. R. P. (2008). Analog signalling in mammalian cortical axons. Curr. Opin. Neurobiol. 18, 314–320. doi: 10.1016/j.conb.2008.08.006
Amin, H., Maccione, A., Marinaro, F., Zordan, S., Nieus, T., and Berdondini, L. (2016). Electrical responses and spontaneous activity of human iPS-derived neuronal networks characterized for 3-month culture with 4096-electrode arrays. Front. Neurosci. 10:121. doi: 10.3389/fnins.2016.00121
Amin, H., Nieus, T., Lonardoni, D., Maccione, A., and Berdondini, L. (2017). High-resolution bioelectrical imaging of Abeta-induced network dysfunction on CMOS-MEAs for neurotoxicity and rescue studies. Sci. Rep. 7:2460. doi: 10.1038/s41598-017-02635-x
Anastassiou, C. A., Buzsáki, G., and Koch, C. (2013). “Biophysics of extracellular spikes,” in Principles of Neural Coding, Vol. 15, eds R. Q. Quiroga and S. Panzeri (Boca Raton, FL: CRC Press), 146.
Awatramani, G. B., Price, G. D., and Trussell, L. O. (2005). Modulation of transmitter release by presynaptic resting potential and background calcium levels. Neuron 48, 109–121. doi: 10.1016/j.neuron.2005.08.038
Bakkum, D. J., Frey, U., Radivojevic, M., Russell, T. L., Muller, J., Fiscella, M., et al. (2013). Tracking axonal action potential propagation on a high-density microelectrode array across hundreds of sites. Nat. Commun. 4:2181. doi: 10.1038/ncomms3181
Bakkum, D. J., Obien, M. E. J., Radivojevic, M., Jäckel, D., Frey, U., Takahashi, H., et al. (2018). The axon initial segment is the dominant contributor to the neuron’s extracellular electrical potential landscape. Adv. Biosyst. 3:1800308. doi: 10.1002/adbi.201800308
Ballini, M., Müller, J., Livi, P., Chen, Y., Frey, U., Stettler, A., et al. (2014). A 1024-channel CMOS microelectrode array with 26,400 electrodes for recording and stimulation of electrogenic cells in vitro. IEEE J. Solid State Circuits 49, 2705–2719. doi: 10.1109/JSSC.2014.2359219
Bando, Y., Sakamoto, M., Kim, S., Ayzenshtat, I., and Yuste, R. (2019). Comparative evaluation of genetically encoded voltage indicators. Cell Rep. 26, 802–813.e4. doi: 10.1016/j.celrep.2018.12.088
Baranauskas, G., David, Y., and Fleidervish, I. A. (2013). Spatial mismatch between the Na+ flux and spike initiation in axon initial segment. Proc. Natl. Acad. Sci. U.S.A. 110, 4051–4056. doi: 10.1073/pnas.1215125110
Berdondini, L., Massobrio, P., Chiappalone, M., Tedesco, M., Imfeld, K., Maccione, A., et al. (2009). Extracellular recordings from locally dense microelectrode arrays coupled to dissociated cortical cultures. J. Neurosci. Methods 177,386–396. doi: 10.1016/j.jneumeth.2008.10.032
Bertotti, G., Velychko, D., Dodel, N., Keil, S., Wolansky, D., Tillak, B., et al. (2014). “A CMOS-based sensor array for in-vitro neural tissue interfacing with 4225 recording sites and 1024 stimulation sites,” in Proceedings of the Biomedical Circuits and Systems Conference (BioCAS), (Cleveland, OH: IEEE), 304–307.
Bischofberger, J., Engel, D., Li, L., Geiger, J. R. P., and Jonas, P. (2006). Patch-clamp recording from mossy fiber terminals in hippocampal slices. Nat. Protoc. 1, 2075–2081. doi: 10.1038/nprot.2006.312
Borst, J. G., Helmchen, F., and Sakmann, B. (1995). Pre-and postsynaptic whole-cell recordings in the medial nucleus of the trapezoid body of the rat. J. Physiol. 489, 825–840. doi: 10.1113/jphysiol.1995.sp021095
Boudkkazi, S., Fronzaroli-Molinieres, L., and Debanne, D. (2011). Presynaptic action potential waveform determines cortical synaptic latency. J. Physiol. 589, 1117–1131. doi: 10.1113/jphysiol.2010.199653
Boyden, E. S., Zhang, F., Bamberg, E., Nagel, G., and Deisseroth, K. (2005). Millisecond-timescale, genetically targeted optical control of neural activity. Nat. Neurosci. 8, 1263–1268. doi: 10.1038/nn1525
Bucher, D. (2016). “Contribution of axons to short-term dynamics of neuronal communication,” in Axons and Brain Architecture, ed. K. S. Rockland (San Diego, CA: Academic Press), 245–263. doi: 10.1016/b978-0-12-801393-9.00012-8
Bucher, D., and Goaillard, J. M. (2011). Beyond faithful conduction: short-term dynamics, neuromodulation, and long-term regulation of spike propagation in the axon. Prog. Neurobiol. 94, 307–346. doi: 10.1016/j.pneurobio.2011.06.001
Buzsáki, G., Anastassiou, C. A., and Koch, C. (2012). The origin of extracellular fields and currents—EEG, ECoG, LFP and spikes. Nat. Rev. Neurosci. 13, 407–420. doi: 10.1038/nrn3241
Cai, Q., Davis, M. L., and Sheng, Z. H. (2011). Regulation of axonal mitochondrial transport and its impact on synaptic transmission. Neurosci. Res. 70, 9–15. doi: 10.1016/j.neures.2011.02.005
Debanne, D. (2004). Information processing in the axon. Nat. Rev. Neurosci. 5, 304–316. doi: 10.1038/nrn1397
Debanne, D., Campanac, E., Bialowas, A., Carlier, E., and Alcaraz, G. (2011). Axon physiology. Physiol. Rev. 91, 555–602. doi: 10.1152/physrev.00048.2009
Debanne, D., and Garrido, J. J. (eds). (2018). “Axon initial segment,” in eLS, (Chichester: John Wiley & Sons, Ltd).
Diggelmann, R., Fiscella, M., Hierlemann, A., and Franke, F. (2018). Automatic spike sorting algorithm for high-density microelectrode arrays. J. Neurophysiol. 120, 3155–3171. doi: 10.1152/jn.00803.2017
Dragas, J., Viswam, V., Shadmani, A., Chen, Y., Bounik, R., Stettler, A., et al. (2017). A multi-functional microelectrode array featuring 59760 electrodes, 2048 electrophysiology channels, stimulation, impedance measurement and neurotransmitter detection channels. IEEE J Solid State Circuits 52, 1576–1590. doi: 10.1109/JSSC.2017.2686580
Egawa, N., Lok, J., Washida, K., and Arai, K. (2017). Mechanisms of axonal damage and repair after central nervous system injury. Transl. Stroke Res. 8, 14–21. doi: 10.1007/s12975-016-0495-1
Egert, U., Heck, D., and Aertsen, A. (2002). Two-dimensional monitoring of spiking networks in acute brain slices. Exp. Brain Res. 142, 268–274. doi: 10.1007/s00221-001-0932-5
Einevoll, G. T., Franke, F., Hagen, E., Pouzat, C., and Harris, K. D. (2012). Towards reliable spike-train recordings from thousands of neurons with multielectrodes. Curr. Opin. Neurobiol. 22, 11–17. doi: 10.1016/j.conb.2011.10.001
Engel, D., and Jonas, P. (2005). Presynaptic action potential amplification by voltage-gated Na+ channels in hippocampal mossy fiber boutons. Neuron 45, 405–417. doi: 10.1016/j.neuron.2004.12.048
Eversmann, B., Jenkner, M., Hofmann, F., Paulus, C., Brederlow, R., Holzapfl, B., et al. (2003). A 128/spl times/128 CMOS biosensor array for extracellular recording of neural activity. IEEE J. Solid State Circuits 38, 2306–2317. doi: 10.1109/jssc.2003.819174
Ferrea, E., Maccione, A., Medrihan, L., Nieus, T., Ghezzi, D., Baldelli, P., et al. (2012). Large-scale, high-resolution electrophysiological imaging of field potentials in brain slices with microelectronic multielectrode arrays. Front. Neural Circuits 6:80. doi: 10.3389/fncir.2012.00080
Fields, R. D. (2005). Myelination: an overlooked mechanism of synaptic plasticity? Neuroscientist 11, 528–531. doi: 10.1177/1073858405282304
Fiscella, M., Farrow, K., Jones, I. L., Jäckel, D., Müller, J., Frey, U., et al. (2012). Recording from defined populations of retinal ganglion cells using a high-density CMOS-integrated microelectrode array with real-time switchable electrode selection. J. Neurosci. Methods 211, 103–113. doi: 10.1016/j.jneumeth.2012.08.017
Fiscella, M., Leary, N., Acun, E., Ronchi, S., and Hierlemann, A. (2018). Electrophysiological phenotype characterization of human iPSC-derived dopaminergic neuronal lines by means of high-resolution microelectrode arrays. Front. Cell. Neurosci. 12:14. doi: 10.3389/conf.fncel.2018.38.00014
Forsythe, I. D. (1994). Direct patch recording from identified presynaptic terminals mediating glutamatergic EPSCs in the rat CNS, in vitro. J. Physiol. 479, 381–387. doi: 10.1113/jphysiol.1994.sp020303
Foust, A., Popovic, M., Zecevic, D., and Mccormick, D. A. (2010). Action potentials initiate in the axon initial segment and propagate through axon collaterals reliably in cerebellar Purkinje neurons. J. Neurosci. 30, 6891–6902. doi: 10.1523/JNEUROSCI.0552-10.2010
Frey, U., Egert, U., Heer, F., Hafizovic, S., and Hierlemann, A. (2009). Microelectronic system for high-resolution mapping of extracellular electric fields applied to brain slices. Biosens. Bioelectron. 24, 2191–2198. doi: 10.1016/j.bios.2008.11.028
Ganguly, K., Kiss, L., and Poo, M.-M. (2000). Enhancement of presynaptic neuronal excitability by correlated presynaptic and postsynaptic spiking. Nat. Neurosci. 3, 1018–1026. doi: 10.1038/79838
Geiger, J. R. P., and Jonas, P. (2000). Dynamic control of presynaptic Ca2+ inflow by fast-inactivating K+ channels in hippocampal mossy fiber boutons. Neuron 28, 927–939. doi: 10.1016/s0896-6273(00)00164-1
Goadsby, P. J., Holland, P. R., Martins-Oliveira, M., Hoffmann, J., Schankin, C., and Akerman, S. (2017). Pathophysiology of migraine: a disorder of sensory processing. Physiol. Rev. 97, 553–622. doi: 10.1152/physrev.00034.2015
Gold, C., Henze, D. A., Koch, C., and Buzsaki, G. (2006). On the origin of the extracellular action potential waveform: a modeling study. J. Neurophysiol. 95, 3113–3128. doi: 10.1152/jn.00979.2005
Goldstein, S. S., and Rall, W. (1974). Changes of action potential shape and velocity for changing core conductor geometry. Biophys. J. 14, 731–757. doi: 10.1016/s0006-3495(74)85947-3
Gong, W., Senčar, J., Bakkum, D. J., Jäckel, D., Obien, M. E. J., Radivojevic, M., et al. (2016). Multiple single-unit long-term tracking on organotypic hippocampal slices using high-density microelectrode arrays. Front. Neurosci. 10:537. doi: 10.3389/fnins.2016.00537
Gong, Y., Huang, C., Li, J. Z., Grewe, B. F., Zhang, Y., Eismann, S., et al. (2015). High-speed recording of neural spikes in awake mice and flies with a fluorescent voltage sensor. Science 350, 1361–1366. doi: 10.1126/science.aab0810
Grinvald, A., Cohen, L. B., Lesher, S., and Boyle, M. B. (1981). Simultaneous optical monitoring of activity of many neurons in invertebrate ganglia using a 124-element photodiode array. J. Neurophysiol. 45, 829–840. doi: 10.1152/jn.1981.45.5.829
Gross, D., and Loew, L. M. (1989). Fluorescent indicators of membrane potential: microspectrofluorometry and imaging. Methods Cell Biol. 30, 193–218. doi: 10.1016/s0091-679x(08)60980-2
Gross, G. W., Rhoades, B. K., Reust, D. L., and Schwalm, F. U. (1993). Stimulation of monolayer networks in culture through thin-film indium-tin oxide recording electrodes. J. Neurosci. Methods 50, 131–143. doi: 10.1016/0165-0270(93)90001-8
Habibey, R., Latifi, S., Mousavi, H., Pesce, M., Arab-Tehrany, E., and Blau, A. (2017). A multielectrode array microchannel platform reveals both transient and slow changes in axonal conduction velocity. Sci. Rep. 7:8558. doi: 10.1038/s41598-017-09033-3
Hierlemann, A., Frey, U., Hafizovic, S., and Heer, F. (2011). Growing cells atop microelectronic chips: interfacing electrogenic cells in vitro with CMOS-based microelectrode arrays. Proc. IEEE 99, 252–284. doi: 10.1109/jproc.2010.2066532
Hochbaum, D. R., Zhao, Y., Farhi, S. L., Klapoetke, N., Werley, C. A., Kapoor, V., et al. (2014). All-optical electrophysiology in mammalian neurons using engineered microbial rhodopsins. Nat. Methods 11, 825–833. doi: 10.1038/nmeth.3000
Hodgkin, A. L. (1954). A note on conduction velocity. J. Physiol. 125, 221–224. doi: 10.1113/jphysiol.1954.sp005152
Hoppa, M. B., Gouzer, G., Armbruster, M., and Ryan, T. A. (2014). Control and plasticity of the presynaptic action potential waveform at small CNS nerve terminals. Neuron 84, 778–789. doi: 10.1016/j.neuron.2014.09.038
Hu, H., and Jonas, P. (2014). A supercritical density of Na(+) channels ensures fast signaling in GABAergic interneuron axons. Nat. Neurosci. 17, 686–693. doi: 10.1038/nn.3678
Hu, W., Tian, C., Li, T., Yang, M., Hou, H., and Shu, Y. (2009). Distinct contributions of Na v 1.6 and Na v 1.2 in action potential initiation and backpropagation. Nat. Neurosci. 12, 996–1002. doi: 10.1038/nn.2359
Huys, R., Braeken, D., Jans, D., Stassen, A., Collaert, N., Wouters, J., et al. (2012). Single-cell recording and stimulation with a 16k micro-nail electrode array integrated on a 0.18 μm CMOS chip. Lab Chip 12, 1274–1280. doi: 10.1039/c2lc21037a
Inagaki, S., and Nagai, T. (2016). Current progress in genetically encoded voltage indicators for neural activity recording. Curr. Opin. Chem. Biol. 33, 95–100. doi: 10.1016/j.cbpa.2016.05.023
Ishikawa, D., Takahashi, N., Sasaki, T., Usami, A., Matsuki, N., and Ikegaya, Y. (2010). Fluorescent pipettes for optically targeted patch-clamp recordings. Neural Netw. 23, 669–672. doi: 10.1016/j.neunet.2010.02.004
Izhikevich, E. M. (2006). Polychronization: computation with spikes. Neural Comput. 18, 245–282. doi: 10.1162/089976606775093882
Jackel, D., Bakkum, D. J., Russell, T. L., Muller, J., Radivojevic, M., Frey, U., et al. (2017). Combination of high-density microelectrode array and patch clamp recordings to enable studies of multisynaptic integration. Sci. Rep. 7:978. doi: 10.1038/s41598-017-00981-4
Jimbo, Y., and Robinson, H. P. C. (2000). Propagation of spontaneous synchronized activity in cortical slice cultures recorded by planar electrode arrays. Bioelectrochemistry 51, 107–115. doi: 10.1016/s0302-4598(99)00083-5
Jin, L., Han, Z., Platisa, J., Wooltorton, J. R., Cohen, L. B., and Pieribone, V. A. (2012). Single action potentials and subthreshold electrical events imaged in neurons with a fluorescent protein voltage probe. Neuron 75, 779–785. doi: 10.1016/j.neuron.2012.06.040
Johnson, B., Peace, S. T., Cleland, T. A., and Molnar, A. (2013). “A 50μm pitch, 1120-channel, 20kHz frame rate microelectrode array for slice recording,” in Proceedings of the IEEE Biomedical Circuits and Systems Conference (BioCAS), Rotterdam, 109–112.
Johnson, V. E., Stewart, W., and Smith, D. H. (2013). Axonal pathology in traumatic brain injury. Exp. Neurol. 246, 35–43. doi: 10.1016/j.expneurol.2012.01.013
Jones, I. L., Russell, T. L., Farrow, K., Fiscella, M., Franke, F., Müller, J., et al. (2015). A method for electrophysiological characterization of hamster retinal ganglion cells using a high-density CMOS microelectrode array. Front. Neurosci. 9:360. doi: 10.3389/fnins.2015.00360
Kawaguchi, S. Y., and Sakaba, T. (2015). Control of inhibitory synaptic outputs by low excitability of axon terminals revealed by direct recording. Neuron 85, 1273–1288. doi: 10.1016/j.neuron.2015.02.013
Khalilpour, S., Latifi, S., Behnammanesh, G., Majid, A. M. S. A., Majid, A. S. A., and Tamayol, A. (2017). Ischemic optic neuropathy as a model of neurodegenerative disorder: a review of pathogenic mechanism of axonal degeneration and the role of neuroprotection. J. Neurol. Sci. 375, 430–441. doi: 10.1016/j.jns.2016.12.044
Kim, S. (2014). Action potential modulation in CA1 pyramidal neuron axons facilitates OLM interneuron activation in recurrent inhibitory microcircuits of rat hippocampus. PLoS One 9:e113124. doi: 10.1371/journal.pone.0113124
Kiskinis, E., Kralj, J. M., Zou, P., Weinstein, E. N., Zhang, H., Tsioras, K., et al. (2018). All-optical electrophysiology for high-throughput functional characterization of a human iPSC-derived motor neuron model of ALS. Stem Cell Rep. 10, 1991–2004. doi: 10.1016/j.stemcr.2018.04.020
Knöpfel, T., Díez-García, J., and Akemann, W. (2006). Optical probing of neuronal circuit dynamics: genetically encoded versus classical fluorescent sensors. Trends Neurosci. 29, 160–166. doi: 10.1016/j.tins.2006.01.004
Kole, M. H., Ilschner, S. U., Kampa, B. M., Williams, S. R., Ruben, P. C., and Stuart, G. J. (2008). Action potential generation requires a high sodium channel density in the axon initial segment. Nat. Neurosci. 11, 178–186. doi: 10.1038/nn2040
Kole, M. H., Letzkus, J. J., and Stuart, G. J. (2007). Axon initial segment Kv1 channels control axonal action potential waveform and synaptic efficacy. Neuron 55, 633–647. doi: 10.1016/j.neuron.2007.07.031
Kralj, J. M., Douglass, A. D., Hochbaum, D. R., Maclaurin, D., and Cohen, A. E. (2011). Optical recording of action potentials in mammalian neurons using a microbial rhodopsin. Nat. Methods 9, 90–95. doi: 10.1038/nmeth.1782
Krarup, C., and Moldovan, M. (2009). Nerve conduction and excitability studies in peripheral nerve disorders. Curr. Opin. Neurol. 22, 460–466. doi: 10.1097/WCO.0b013e3283304c9d
Kress, G. J., and Mennerick, S. (2009). Action potential initiation and propagation: upstream influences on neurotransmission. Neuroscience 158, 211–222. doi: 10.1016/j.neuroscience.2008.03.021
Kullmann, D. M. (2010). Neurological channelopathies. Annu. Rev. Neurosci. 33, 151–172. doi: 10.1146/annurev-neuro-060909-153122
Lee, S., Geiller, T., Jung, A., Nakajima, R., Song, Y. K., and Baker, B. J. (2017). Improving a genetically encoded voltage indicator by modifying the cytoplasmic charge composition. Sci. Rep. 7:8286. doi: 10.1038/s41598-017-08731-2
Leterrier, C. (2018). The axon initial segment: an updated viewpoint. J. Neurosci. 38, 2135–2145. doi: 10.1523/JNEUROSCI.1922-17.2018
Lewandowska, M. K., Bakkum, D. J., Rompani, S. B., and Hierlemann, A. (2015). Recording large extracellular spikes in microchannels along many axonal sites from individual neurons. PLoS One 10:e0118514. doi: 10.1371/journal.pone.0118514
Liberski, P. P., and Budka, H. (1999). Neuroaxonal pathology in Creutzfeldt-Jakob disease. Acta Neuropathol. 97, 329–334. doi: 10.1007/s004010050995
Maccione, A., Garofalo, M., Nieus, T., Tedesco, M., Berdondini, L., and Martinoia, S. (2012). Multiscale functional connectivity estimation on low-density neuronal cultures recorded by high-density CMOS micro electrode arrays. J. Neurosci. Methods 207, 161–171. doi: 10.1016/j.jneumeth.2012.04.002
Manor, Y., Koch, C., and Segev, I. (1991). Effect of geometrical irregularities on propagation delay in axonal trees. Biophys. J. 60, 1424–1437. doi: 10.1016/s0006-3495(91)82179-8
Medrihan, L., Ferrea, E., Greco, B., Baldelli, P., and Benfenati, F. (2014). Asynchronous GABA release is a key determinant of tonic inhibition and controls neuronal excitability: a study in the Synapsin II-/- mouse. Cereb. Cortex 25, 3356–3368. doi: 10.1093/cercor/bhu141
Mennerick, S., Chisari, M., Shu, H.-J., Taylor, A., Vasek, M., Eisenman, L. N., et al. (2010). Diverse voltage-sensitive dyes modulate GABAA Receptor function. J. Neurosci. 30, 2871–2879. doi: 10.1523/JNEUROSCI.5607-09.2010
Menzler, J., and Zeck, G. (2011). Network oscillations in rod-degenerated mouse retinas. J. Neurosci. 31, 2280–2291. doi: 10.1523/JNEUROSCI.4238-10.2011
Millecamps, S., and Julien, J.-P. (2013). Axonal transport deficits and neurodegenerative diseases. Nat. Rev. Neurosci. 14, 161–176. doi: 10.1038/nrn3380
Miller, E. W., Lin, J. Y., Frady, E. P., Steinbach, P. A., Kristan, W. B., and Tsien, R. Y. (2012). Optically monitoring voltage in neurons by photo-induced electron transfer through molecular wires. Proc. Natl. Acad. Sci. U.S.A. 109, 2114–2119. doi: 10.1073/pnas.1120694109
Müller, J., Ballini, M., Livi, P., Chen, Y., Radivojevic, M., Shadmani, A., et al. (2015). High-resolution CMOS MEA platform to study neurons at subcellular, cellular, and network levels. Lab Chip 15, 2767–2780. doi: 10.1039/c5lc00133a
Neher, E., and Sakmann, B. (1976). Single-channel currents recorded from membrane of denervated frog muscle fibres. Nature 260, 799–802. doi: 10.1038/260799a0
Novak, P., Gorelik, J., Vivekananda, U., Shevchuk, A. I., Ermolyuk, Y. S., Bailey, R. J., et al. (2013). Nanoscale-targeted patch-clamp recordings of functional presynaptic ion channels. Neuron 79, 1067–1077. doi: 10.1016/j.neuron.2013.07.012
Nunez, P. L., and Srinivasan, R. (2006). Electric Fields of the Brain: the Neurophysics of EEG. New York, NY: Oxford University Press.
Obien, M. E., Deligkaris, K., Bullmann, T., Bakkum, D. J., and Frey, U. (2015). Revealing neuronal function through microelectrode array recordings. Front. Neurosci. 8:423. doi: 10.3389/fnins.2014.00423
Obien, M. E. J., Hierlemann, A., and Frey, U. (2019). Accurate signal-source localization in brain slices by means of high-density microelectrode arrays. Sci. Rep. 9:788. doi: 10.1038/s41598-018-36895-y
Ogden, D., and Stanfield, P. (1994). “Patch clamp techniques for single channel and whole-cell recording,” in Microelectrode Techniques: the Plymouth Workshop Handbook, ed. D. Ogden (Cambridge: Company of Biologists), 53–78.
Ogi, J., Kato, Y., Matoba, Y., Yamane, C., Nagahata, K., Nakashima, Y., et al. (2017). Twenty-four-micrometer-pitch microelectrode array with 6912-channel readout at 12 kHz via highly scalable implementation for high-spatial-resolution mapping of action potentials. Biointerphases 12:05F402. doi: 10.1116/1.4997358
Ohura, S., and Kamiya, H. (2016). Excitability tuning of axons in the central nervous system. J. Physiol. Sci. 66, 189–196. doi: 10.1007/s12576-015-0415-2
Oyrer, J., Maljevic, S., Scheffer, I. E., Berkovic, S. F., Petrou, S., and Reid, C. A. (2018). Ion channels in genetic epilepsy: from genes and mechanisms to disease-targeted therapies. Pharmacol. Rev. 70, 142–173. doi: 10.1124/pr.117.014456
Peterka, D. S., Takahashi, H., and Yuste, R. (2011). Imaging voltage in neurons. Neuron 69, 9–21. doi: 10.1016/j.neuron.2010.12.010
Petersen, C. C. H., Grinvald, A., and Sakmann, B. (2003). Spatiotemporal dynamics of sensory responses in layer 2/3 of rat barrel cortex measured in vivo by voltage-sensitive dye imaging combined with whole-cell voltage recordings and neuron reconstructions. J. Neurosci. 23, 1298–1309. doi: 10.1523/jneurosci.23-04-01298.2003
Piatkevich, K. D., Jung, E. E., Straub, C., Linghu, C., Park, D., Suk, H. J., et al. (2018). A robotic multidimensional directed evolution approach applied to fluorescent voltage reporters. Nat. Chem. Biol. 14, 352–360. doi: 10.1038/s41589-018-0004-9
Pietrobon, D. (2018). Ion channels in migraine disorders. Curr. Opin. Physiol. 2, 98–108. doi: 10.1016/j.cophys.2018.02.001
Platisa, J., and Pieribone, V. A. (2018). Genetically encoded fluorescent voltage indicators: are we there yet? Curr. Opin. Neurobiol. 50, 146–153. doi: 10.1016/j.conb.2018.02.006
Popovic, M. A., Carnevale, N., Rozsa, B., and Zecevic, D. (2015). Electrical behaviour of dendritic spines as revealed by voltage imaging. Nat. Commun. 6:8436. doi: 10.1038/ncomms9436
Popovic, M. A., Foust, A. J., Mccormick, D. A., and Zecevic, D. (2011). The spatio-temporal characteristics of action potential initiation in layer 5 pyramidal neurons: a voltage imaging study. J. Physiol. 589, 4167–4187. doi: 10.1113/jphysiol.2011.209015
Radivojevic, M., Franke, F., Altermatt, M., Muller, J., Hierlemann, A., and Bakkum, D. J. (2017). Tracking individual action potentials throughout mammalian axonal arbors. eLife 6:e30198. doi: 10.7554/eLife.30198
Radivojevic, M., Jackel, D., Altermatt, M., Muller, J., Viswam, V., Hierlemann, A., et al. (2016). Electrical identification and selective microstimulation of neuronal compartments based on features of extracellular action potentials. Sci. Rep. 6:31332. doi: 10.1038/srep31332
Rama, S., Zbili, M., Bialowas, A., Fronzaroli-Molinieres, L., Ankri, N., Carlier, E., et al. (2015a). Presynaptic hyperpolarization induces a fast analogue modulation of spike-evoked transmission mediated by axonal sodium channels. Nat. Commun. 6:10163. doi: 10.1038/ncomms10163
Rama, S., Zbili, M., and Debanne, D. (2015b). Modulation of spike-evoked synaptic transmission: the role of presynaptic calcium and potassium channels. Biochim. Biophys. Acta 1853, 1933–1939. doi: 10.1016/j.bbamcr.2014.11.024
Rama, S., Zbili, M., and Debanne, D. (2018). Signal propagation along the axon. Curr. Opin. Neurobiol. 51, 37–44. doi: 10.1016/j.conb.2018.02.017
Rowan, M. J., Delcanto, G., Yu, J. J., Kamasawa, N., and Christie, J. M. (2016). Synapse-level determination of action potential duration by K(+) channel clustering in axons. Neuron 91, 370–383. doi: 10.1016/j.neuron.2016.05.035
Rutten, W. L. C., Ruardij, T. G., Marani, E., and Roelofsen, B. H. (2007). “Neural networks on chemically patterned electrode arrays: towards a cultured probe,” in Operative Neuromodulation, eds D. E. Sakas and B. A. Simpson (Vienna: Springer), 547–554. doi: 10.1007/978-3-211-33081-4_63
Sasaki, T. (2013). The axon as a unique computational unit in neurons. Neurosci. Res. 75, 83–88. doi: 10.1016/j.neures.2012.12.004
Sasaki, T., Matsuki, N., and Ikegaya, Y. (2011). Action-potential modulation during axonal conduction. Science 331, 599–601. doi: 10.1126/science.1197598
Sasaki, T., Matsuki, N., and Ikegaya, Y. (2012). Targeted axon-attached recording with fluorescent patch-clamp pipettes in brain slices. Nat. Protoc. 7, 1228–1234. doi: 10.1038/nprot.2012.061
Shepherd, G. M. G., and Harris, K. M. (1998). Three-dimensional structure and composition of CA3? CA1 axons in rat hippocampal slices: implications for presynaptic connectivity and compartmentalization. J. Neurosci. 18, 8300–8310. doi: 10.1523/jneurosci.18-20-08300.1998
Shimba, K., Sakai, K., Isomura, T., Kotani, K., and Jimbo, Y. (2014). Axonal conduction slowing induced by spontaneous bursting activity in cortical neurons cultured in a microtunnel device. Integr. Biol. 7, 64–72. doi: 10.1039/c4ib00223g
Shu, Y., Hasenstaub, A., Duque, A., Yu, Y., and Mccormick, D. A. (2006). Modulation of intracortical synaptic potentials by presynaptic somatic membrane potential. Nature 441, 761–765. doi: 10.1038/nature04720
Shu, Y., Yu, Y., Yang, J., and Mccormick, D. A. (2007). Selective control of cortical axonal spikes by a slowly inactivating K+ current. Proc. Natl. Acad. Sci. U.S.A. 104, 11453–11458. doi: 10.1073/pnas.0702041104
Smith, D. H., and Meaney, D. F. (2000). Axonal damage in traumatic brain injury. Neuroscientist 6, 483–495. doi: 10.1177/107385840000600611
Spira, M. E., and Hai, A. (2013). Multi-electrode array technologies for neuroscience and cardiology. Nat. Nanotechnol. 8, 83–94. doi: 10.1038/nnano.2012.265
Steinman, L. (1996). Multiple sclerosis: a coordinated immunological attack against myelin in the central nervous system. Cell 85, 299–302. doi: 10.1016/s0092-8674(00)81107-1
Stett, A., Egert, U., Guenther, E., Hofmann, F., Meyer, T., Nisch, W., et al. (2003). Biological application of microelectrode arrays in drug discovery and basic research. Anal. Bioanal. Chem. 377, 486–495. doi: 10.1007/s00216-003-2149-x
Storace, D., Sepehri Rad, M., Kang, B., Cohen, L. B., Hughes, T., and Baker, B. J. (2016). Toward better genetically encoded sensors of membrane potential. Trends Neurosci. 39, 277–289. doi: 10.1016/j.tins.2016.02.005
St-Pierre, F., Marshall, J. D., Yang, Y., Gong, Y., Schnitzer, M. J., and Lin, M. Z. (2014). High-fidelity optical reporting of neuronal electrical activity with an ultrafast fluorescent voltage sensor. Nat. Neurosci. 17, 884–889. doi: 10.1038/nn.3709
Suter, U., and Scherer, S. S. (2003). Disease mechanisms in inherited neuropathies. Nat. Rev. Neurosci. 4, 714–726. doi: 10.1038/nrn1196
Trapp, B. D., Ransohoff, R., and Rudick, R. (1999). Axonal pathology in multiple sclerosis: relationship to neurologic disability. Curr. Opin. Neurol. 12, 295–302. doi: 10.1097/00019052-199906000-00008
Tsai, D., Sawyer, D., Bradd, A., Yuste, R., and Shepard, K. L. (2017). A very large-scale microelectrode array for cellular-resolution electrophysiology. Nat. Commun. 8:1802. doi: 10.1038/s41467-017-02009-x
Tsutsui, H., Jinno, Y., Tomita, A., Niino, Y., Yamada, Y., Mikoshiba, K., et al. (2013). Improved detection of electrical activity with a voltage probe based on a voltage-sensing phosphatase. J. Physiol. 591, 4427–4437. doi: 10.1113/jphysiol.2013.257048
Viswam, V., Bounik, R., Shadmani, A., Dragas, J., Urwyler, C., Boos, J. A., et al. (2018). Impedance spectroscopy and electrophysiological imaging of cells with a high-density CMOS microelectrode array system. IEEE Trans. Biomed. Circuits Syst. 12, 1356–1368. doi: 10.1109/TBCAS.2018.2881044
Wang, W., Kim, C. K., and Ting, A. Y. (2019). Molecular tools for imaging and recording neuronal activity. Nat. Chem. Biol. 15, 101–110. doi: 10.1038/s41589-018-0207-0
Waxman, S. G. (2006). Axonal conduction and injury in multiple sclerosis: the role of sodium channels. Nat. Rev. Neurosci. 7, 932–941. doi: 10.1038/nrn2023
Xu, Y., Zou, P., and Cohen, A. E. (2017). Voltage imaging with genetically encoded indicators. Curr. Opin. Chem. Biol. 39, 1–10. doi: 10.1016/j.cbpa.2017.04.005
Yada, Y., Kanzaki, R., and Takahashi, H. (2016). State-dependent propagation of neuronal sub-population in spontaneous synchronized bursts. Front. Syst. Neurosci. 10:28. doi: 10.3389/fnsys.2016.00028
Yang, H. H., and St-Pierre, F. (2016). Genetically encoded voltage indicators: opportunities and challenges. J. Neurosci. 36, 9977–9989. doi: 10.1523/JNEUROSCI.1095-16.2016
Yuan, X., Emmenegger, V., Obien, M. E. J., Hierlemann, A., and Frey, U. (2018). “Dual-mode microelectrode array featuring 20k electrodes and high SNR for extracellular recording of neural networks,” in Proceedings of the IEEE Biomedical Circuits and Systems Conference (BioCAS), Cleveland, OH, 1–4.
Keywords: axon, action potential propagation, patch-clamp technique, genetically encoded voltage indicators, high-density microelectrode arrays
Citation: Emmenegger V, Obien MEJ, Franke F and Hierlemann A (2019) Technologies to Study Action Potential Propagation With a Focus on HD-MEAs. Front. Cell. Neurosci. 13:159. doi: 10.3389/fncel.2019.00159
Received: 28 February 2019; Accepted: 08 April 2019;
Published: 26 April 2019.
Edited by:
Dominique Debanne, INSERM U1072 Neurobiologie des Canaux Ioniques et de la Synapse, FranceReviewed by:
Zbili Mickael, Institut National de la Santé et de la Recherche Médicale (INSERM), FranceMichael Blake Hoppa, Dartmouth College, United States
Copyright © 2019 Emmenegger, Obien, Franke and Hierlemann. This is an open-access article distributed under the terms of the Creative Commons Attribution License (CC BY). The use, distribution or reproduction in other forums is permitted, provided the original author(s) and the copyright owner(s) are credited and that the original publication in this journal is cited, in accordance with accepted academic practice. No use, distribution or reproduction is permitted which does not comply with these terms.
*Correspondence: Vishalini Emmenegger, dmlzaGFsaW5pLmVtbWVuZWdnZXJAYnNzZS5ldGh6LmNo