- 1GIGA-Stem Cells, Interdisciplinary Cluster for Applied Genoproteomics (GIGA-R), University of Liège, Liège, Belgium
- 2Institut Pasteur, Biology of Infection Unit, Paris, France
- 3INSERM U1117, Biologie des Infections, Paris, France
- 4Paris Descartes University, Division of Infectious Diseases and Tropical Medicine, Necker-Enfants Malades Hospital, Institut Imagine, Sorbonne Paris Cité, Paris, France
Zika virus (ZIKV) is a mosquito-borne virus that belongs to the Flaviviridae family, together with dengue, yellow fever, and West Nile viruses. In the wake of its emergence in the French Polynesia and in the Americas, ZIKV has been shown to cause congenital microcephaly. It is the first arbovirus which has been proven to be teratogenic and sexually transmissible. Confronted with this major public health challenge, the scientific and medical communities teamed up to precisely characterize the clinical features of congenital ZIKV syndrome and its underlying pathophysiological mechanisms. This review focuses on the critical impact of the unfolded protein response (UPR) on ZIKV-associated congenital microcephaly. ZIKV infection of cortical neuron progenitors leads to high endoplasmic reticulum (ER) stress. This results in both the stalling of indirect neurogenesis, and UPR-dependent neuronal apoptotic death, and leads to cortical microcephaly. In line with these results, the administration of molecules inhibiting UPR prevents ZIKV-induced cortical microcephaly. The discovery of the link between ZIKV infection and UPR activation has a broader relevance, since this pathway plays a crucial role in many distinct cellular processes and its induction by ZIKV may account for several reported ZIKV-associated defects.
Overview of the Zika Epidemics
Zika virus (ZIKV) was first isolated in 1947 from the Zika forest in Uganda, East Africa (Dick et al., 1952), and identified as a member of the Flaviviridae genus (as yellow fever, West Nile, Japanese encephalitis, and dengue viruses). However, it remained until recently largely unknown, with fewer than 20 confirmed human infections reported over the 60 years prior to 2007 (Musso and Gubler, 2016). It has likely, however, circulated undetected during this time. This may result from (i) clinical or serological misdiagnosis with life threatening arboviral infections (such as dengue), (ii) the absence of known severe outcome favoring silent transmission, and (iii) occurrence in places where epidemiological and medical infrastructure are scarce and which are overwhelmed by more severe medical threats (Musso and Gubler, 2016). After ZIKV emergence in Western Pacific in Yap Island in 2007 (Lanciotti et al., 2008; Duffy et al., 2009), and in French Polynesia, South Pacific in 2013–2014 (Oehler et al., 2014), ZIKV reached the Americas, particularly Brazil in 2015, with 440,000–1,300,000 reported cases (Bogoch et al., 2016).
In Brazil, soon after the emergence of ZIKV, microcephalic newborns from ZIKV-infected mothers were reported (Coelho and Crovella, 2017; Saiz et al., 2017), as well as children with postnatal neural and non-neural disorders following congenital ZIKV infection (McCarthy, 2016; Moore et al., 2017; Mavigner et al., 2018). This led to the identification of ZIKV as a teratogenic agent (Baud et al., 2017; Schaub et al., 2017b). Additionally, ZIKV has been shown to be transmitted sexually (Matusali et al., 2018; Sakkas et al., 2018) and more likely transmitted from men to women than the converse (Counotte et al., 2018), with an increased risk of male infertility (Caswell and Manavi, 2018). Furthermore, ZIKV infection of adults has been causally linked to Guillain-Barré syndrome (GBS) (Cao-Lormeau et al., 2016). The emergence of ZIKV in the Pacific and the Americas, and the identification of severe neurological complications led the declaration of ZIKA as a Public Health Emergency of International Concern (PHEIC) (WHO, 20161). The concurrence of this epidemic with the 2016 Summer Olympic Games in Rio de Janeiro further crystallized the global scrutiny on this virus.
Open Questions on Zika Pathogenesis
Toward understanding the underlying mechanisms and pathogenesis of ZIKV infections, molecular studies were performed with cutting edge tools, such as human induced pluripotent stem cells (hiPSCs), as well as human cell-derived neurospheres and organoids (Cugola et al., 2016; Tang et al., 2016; Garcez et al., 2017; Himmelsbach and Hildt, 2018). These tools shed some light on the ZIKV pathophysiological mechanisms on human-derived samples, and these experiments were further corroborated by studies performed in vivo in animals and in immortalized cell lines. Indeed, the initial reports from different groups unveiled a strong tropism of ZIKV for neuronal precursors of the cerebral cortex (Cugola et al., 2016; Li et al., 2016; Tang et al., 2016; Gladwyn-Ng et al., 2018). In combination, these findings supported that ZIKV impairs cell cycle progression, and triggers a cascade of apoptotic and autophagic cell deaths that ultimately lead to microcephaly. A recent study reported that ZIKV-induced transcriptional effects in human neural progenitors that were similar to those elicited by numerous genetic mutations underlying severe microcephaly in mice (Ghouzzi et al., 2016; Zhang et al., 2016). The massive cell loss observed in ZIKV-infected brains is likely to account for cortical lesions with impaired neuronal connectivity, able to induce postnatal neurological dysfunctions such as epilepsy, secondary microcephaly, cognitive defects, that constitute frequent outcomes of congenital ZIKV syndrome (CZS) (Franca et al., 2018; Subissi et al., 2018; van der Linden et al., 2018). These reports supported a causal link between ZIKV infection and the neurological impairments observed in utero-infected infants.
Zika Infection Induces Endoplasmic Reticulum Stress and Activation of the Unfolded Protein Response
Zika virus, as a single stranded RNA virus replicates in the endoplasmic reticulum (ER) of host cells, which was recently reported to be targeted by the interferon (IFN) response (Richardson et al., 2018). ZIKV infection causes ultrastructural changes of the ER and ER-derived structures in nerve cells in mouse brains infected after birth in vivo and hepatocarcinoma cells in vitro (Bell et al., 1971; Cortese et al., 2017). In addition, ZIKV induces massive vacuolization (which are derived from the ER) followed by cell death in human astrocytes and others such as primary skin fibroblasts and epithelial cells (Monel et al., 2017). ZIKV infection also perturbs ER-related pathways that are connected to other relevant pathways such as apoptosis and innate immunity (Blazquez et al., 2014). Stressors of ER physiological functions have led to the selection of an evolutionarily conserved cell response that comprises multiple effector pathways, collectively known as the unfolded protein response (UPR). Although the UPR has been described as a homeostatic compensation for cell stress, it can potentially trigger cell death after severe or prolonged ER dysfunction (reviewed in Xu et al., 2005). UPR is modulated by three critical ER-membrane bound receptors: PKR-like endoplasmic reticulum kinase (PERK), Inositol-requiring enzyme 1 (IRE1α) and activating transcription factor 6 (ATF6) (see Figure 1; Godin et al., 2016). Collectively, these pathways constitute a finely tuned sensor of ER activity during altered protein translation and folding (resulting for example from oxidative stress, hypoxia, infections), which mainly act by (i) reducing the workload of the ER through the PERK-mediated pathway, which mainly slows down ribosomal activity and (ii) modulating the transcription of several effectors regulating different cellular events through IRE1α- and ATF6-mediated pathways (such as cell cycle to cell survival, from autophagy to cytoskeletal remodeling). The pharmacological modulation of UPR has been explored as a potential therapeutic strategy against a diverse gamut of pathologies such as cancer, neurodegenerative and metabolic diseases (e.g., diabetes) as well as age-related dysfunctions (e.g., age-related retinopathies) (Scheper and Hoozemans, 2015; Galmiche et al., 2017). Interestingly, many of the cellular dysfunctions observed in ZIKV-infected cells (such as deregulation of homeostasis and cell cycle) would fit with the induction of ER stress and the ensuing UPR.
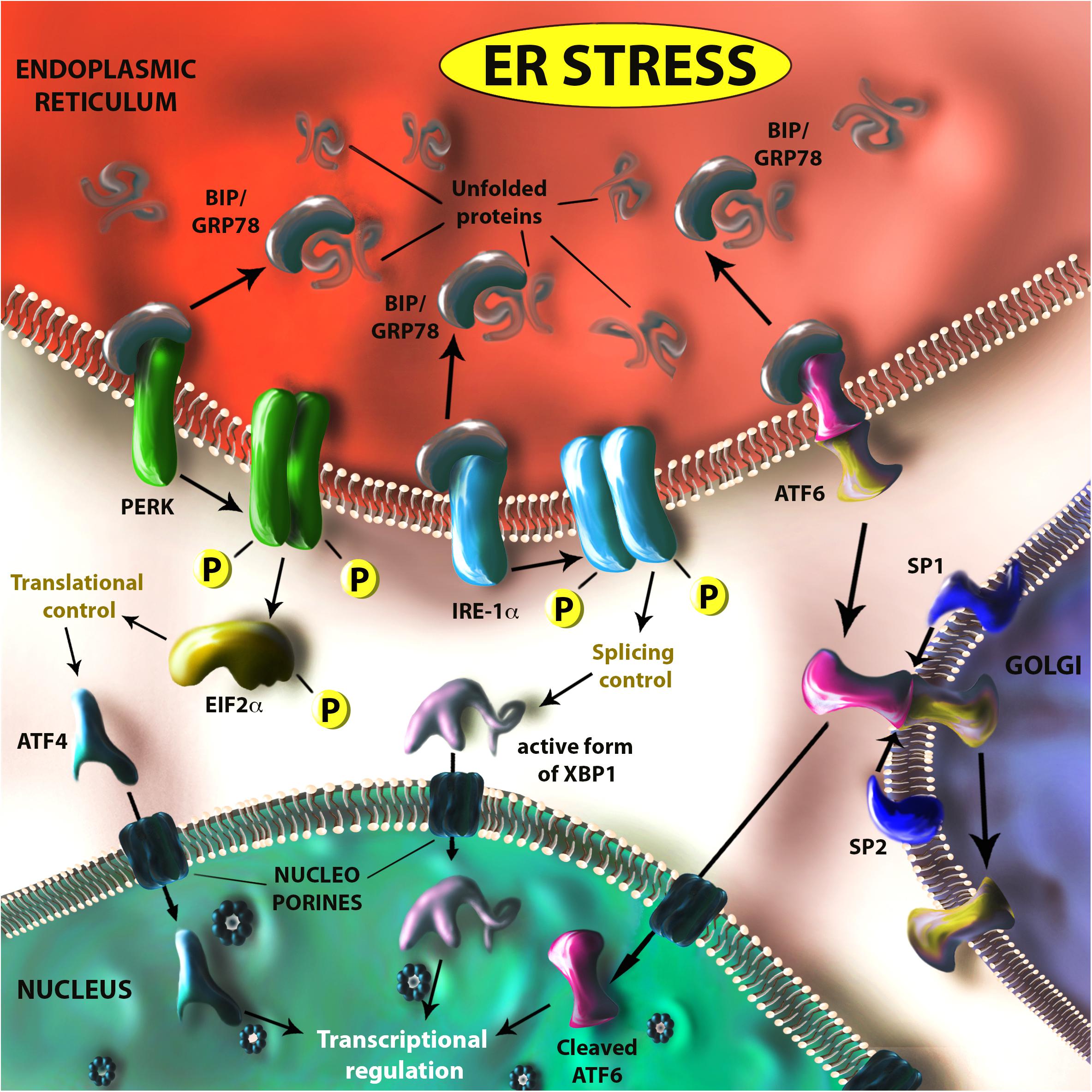
Figure 1. Schematic representation of the unfolded protein response (UPR). In case of ER stress (due to viral infection, metabolic impairments, inflammation, cancer, hypoxia, etc.) translated proteins improperly folded are recognized by the endoplasmic reticulum (ER) chaperon GRP78. This protein is usually bound to three ER receptors (PERK, IRE-1α, and ATF6), which are maintained in an inactive form. When GRP78 binds to unfolded proteins it releases these three receptors, which are activated either through dimerization and auto-phosphorilation (PERK and IRE-1α) or by translocation and cleavage (through SP1 and SP2 proteases) on Golgi apparatus membrane (ATF6). The PERK-controlled pathway is mainly deputed to the control of translational activity in the ER through EIF2α phosphorilation, but has also an active role in transcriptional control by regulating ATF4 translation. IRE-1α and ATF6, together with ATF4, have an important role in the transcriptional control of genes involved in UPR regulated processes such as apoptosis, autophagy, inflammation, and angiogenesis, etc. IRE-1α regulates XBP1 activity by modulating its RNA-splicing.
A Crucial Link Between UPR and Neurogenesis
The UPR pathway was first shown to be fundamental for temporal regulation neurogenesis in the mammalian cerebral cortex during development (Laguesse et al., 2015a). Neural progenitors [also called apical progenitors (AP)], located at the luminal surface of the rostral neural tube (telencephalon), predominately generate neurons that migrate basally during the earlier stages of cortical development. This process is termed direct neurogenesis and gives rise to the primordial cerebral cortex (Figure 2, left). Toward later stages of cortical development, AP sequentially give rise to a second and third pool of progenitors known as the basal progenitors (BP) and outer radial glia (oRG), respectively. These progenitors may divide either symmetrically producing either two identical daughter progenitors or two newborn neurons (indirect neurogenesis), thus amplifying the neuronal output from each AP [reviewed in Laguesse et al. (2015b)] (Figure 2, left). This transition from direct to indirect neurogenesis during cortical development occurred relatively recently in vertebrate evolution, and favored the increasing complexification of mammalian cerebral cortices when compared to other vertebrates (Dugas-Ford and Ragsdale, 2015). Laguesse et al. (2015b) first demonstrated that this gradual shift from direct to indirect neurogenesis is regulated by a progressive decrease in the UPR basal activity within AP, whereby its impairment leads to microcephaly. Taken together, these reports posit the following: (i) how does Zika induce microcephaly?; and more specifically (ii) does ZIKV perturb ER function or induce UPR response in ZIKV-infected fetuses?; (iii) does ZIKV unhinge the transition from direct to indirect neurogenesis?; (iv) what are the neurological sequelae of disrupted neurogenesis and corticogenesis in infants with congenital ZIKV? We launched a research program to answer these questions, and elucidate the molecular bases of ZIKV-associated microcephaly (Gladwyn-Ng et al., 2018).
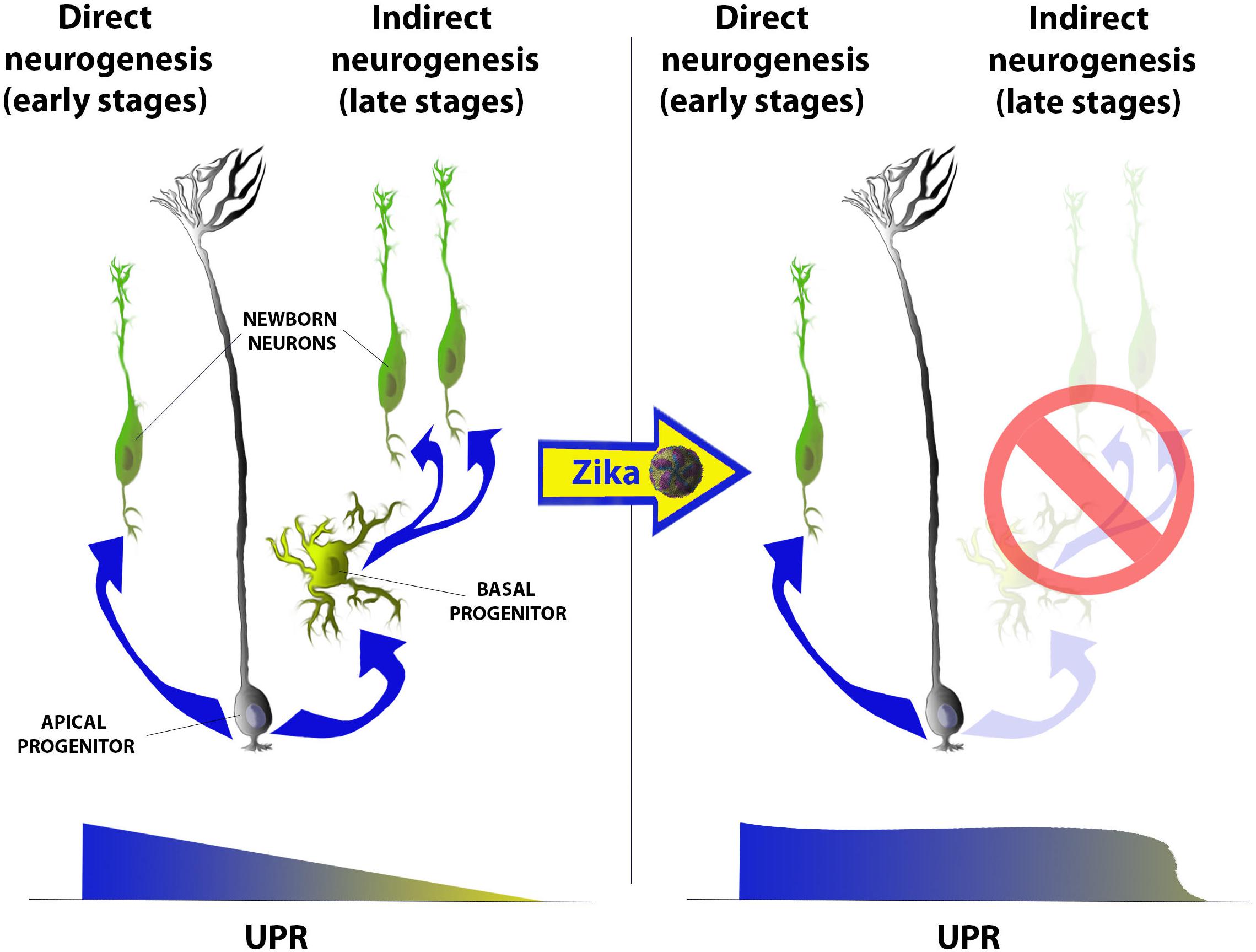
Figure 2. Schematic representation of the gradual shift from direct (left) to indirect (right) neurogenesis and of its imbalance after Zika infection in mammalian cerebral cortices. Briefly, at early stages of corticogenesis (from E10.5 to E13.5 in mice) apical progenitors (AP) populating the ventricular zone (VZ) of the rostral neural tube start producing first neurons, which will give rise to the primordial cortical plate (CP). At these stages the level of UPR is higher then later stages and gradually decreases as development proceeds. From E13.5 onward (in mice) AP start producing at least other two progenitor populations: basal progenitors and outer radial glia (not in the scheme) and, as UPR decreases, this neurogenic mode (called indirect) becomes gradually predominant. Indirect neurogenesis is only present in mammals (among all vertebrates) and mainly contributes to the amplification of the neuronal output during corticogenesis. Upon Zika infection, instead, UPR levels remain high also at later stages of development and the predominant mode of neurogenesis remains the direct one leading to a decrease in the total neuronal output and, hence, to a microcephalic cortex.
Zika Infection Deregulates UPR During Cortical Neurogenesis
We first performed transcriptomic and histological analyses of ZIKV-infected human fetal microcephalic cortices (median age of 22 gestational weeks), and uncovered a distinct up-regulation of UPR effectors. These data were further confirmed by the analysis of transcripts from both induced human neural progenitor cells (hNPCs) and mouse cortical cells that had been experimentally infected with ZIKV. Interestingly both PERK and IRE-1α downstream effectors were also strongly up-regulated. By using intraplacental (IPL) and intracerebroventricular (ICV) infections in combination with in utero electroporation (IUE), we were able to model ZIKV infection in wild-type embryonic outbred mice (Tuttle et al., 2018). These methodologies allowed further interrogation of the putative links between ZIKV infection, ZIKV-induced defects of corticogenesis and neurogenesis, as well as UPR deregulation (Gladwyn-Ng et al., 2018). Firstly, we observed ZIKV-infected embryonic cortices to be microcephalic (reduced both in tangential and radial dimensions), with disorganized cortical layering, as well as the tropism of ZIKV for neuronal progenitors and neurons, all of which are consistent with previous studies (Cugola et al., 2016; Li et al., 2016; Wu et al., 2016). We further demonstrated that neuronal progenitors within ZIKV-infected brains exhibited ER stress markers (e.g., upregulation of Calreticulin and Calnexin), and were disinclined to transit from direct to indirect neurogenesis prior to massive apoptosis of their progeny daughter cells (projection neurons) (Figure 2, right). These findings are supported by subsequent reports of transcriptomics analyses of ZIKV-infected cells that revealed a distinct up-regulation of UPR effectors (Rolfe et al., 2016; Kozak et al., 2017; Gurumayum et al., 2018). These conclusions were later supported by multiple investigations of the ZIKV in immunodeficient mice (Garcez et al., 2018; Kum et al., 2018), such as the intraperitoneal injection of ZIKV into mice lacking Type I and II IFN receptors that subsequently exhibited significant up-regulation of the expression of key markers of ER stress in the neural cells (Tan et al., 2018). To investigate the observed Zika tropism for progenitors and neurons, we infected embryonic mouse brains with two other flaviviruses [West Nile and yellow fever (vaccine 17D strain) viruses]and demonstrated that defects in corticogenesis and neurogenesis were specific to ZIKV.
We next utilized GSK2656157 and 4μ8C, which are specific inhibitors of the PERK and IRE-1α, respectively, to investigate if these compounds could ameliorate ZIKV-induced UPR-mediated cortical defects. Of note, oral administration of GSK2656157 has been reported to cross the blood-brain barrier and possess preventive properties against tau-mediated neurodegeneration in a mouse model of frontotemporal dementia (Radford et al., 2015) and clinical disease in prion-infected mice (Moreno et al., 2013), while 4μ8C is undergoing preclinical trials in the treatment of multiple myeloma (Cross et al., 2012). We were able to significantly rescue cortical dimensions, neurogenic defects by modulating cortical progenitors’ specification as well as to prevent the excessive cell death observed in infected mouse brains that were co-administered with either of these UPR inhibitors. Moreover, the genetic suppression of C/EBP homologs protein (Chop) (a pro-apoptotic factor induced by protracted UPR up-regulation, and a common effector gene of PERK and IRE-1α) by co-in utero electroporation of a specific siRNA reduced the apoptotic events within ZIKV-infected mouse cerebral cortices. These findings also directly implicated the terminal UPR pathway in the cell death observed in ZIKV-infected brains in addition to compromised direct neurogenesis.
ZIKV Induces ER Stress and Cell Death Via Cell-Autonomous and Non-Cell-Autonomous Ways
The disrupted neurogenesis and elevated cell death of positively and negatively ZIKV-immunolabelled cells within infected brains could be rescued by co-administration of the above-stated UPR inhibitors. These findings further indicated that ZIKV also alters cortical cell behavior and survival in a non-cell-autonomous way, which have been further substantiated in other reports (Olmo et al., 2017), and ZIKV might induce UPR deregulation in a direct and indirect manner. This suggests that ZIKV-infected cells may release signaling molecules that influence UPR activity of neighboring cells, either as an anti-viral defense mechanism or to favor ZIKV replication. Correspondingly, ZIKV infection was recently reported to induce a strong release of glutamate and cytokines (e.g., IL-8, IL-1ß, IL-6, CXCL10, and TNF-alpha) that have a cytotoxic effect on both infected and uninfected neurons. Specifically, high doses of interleukins are known to induce ER stress and cell death (Zhang and Kaufman, 2008; Smith, 2018), while ZIKV induces chemokine CXCL10 that stimulates the NMDA receptor (NMDAR) (Ye et al., 2013; Li et al., 2016; Olmo et al., 2017) and exacerbates NMDAR-mediated cytotoxicity through activation of the interferon-gamma (IFN-γ) regulated pathways (van Weering et al., 2011; Chaudhary et al., 2017). The combinatorial release of interleukins and chemokines also possesses the indirect ability to attract and activate microglia, the resident immune cells in the CNS, to the site of infection (Hanisch, 2002). The activation of microglia upon ZIKV infection, via the cell-surface receptor tyrosine kinase (encoded by the AXL gene) (Lum et al., 2017; Meertens et al., 2017; Diop et al., 2018; Wang et al., 2018), may be due to a direct up-regulation of the ATF6 pathway (Ta et al., 2016). ZIKV induces the secretion of chemokines and other cytotoxic molecules from infected microglia by activating both UPR and toll-like receptor 3 (TLR3) signaling (Hosoi et al., 2013; Kim et al., 2015; Wen et al., 2017). Hence, the infection of microglia by ZIKV may promote viral replication by inducing cell cycle arrest and cell differentiation (Wang et al., 2018). In addition, microglia is a potential reservoir of viruses in the CNS (biorxiv, https://doi.org/10.1101/142497) and, by releasing TNF-α and other molecules, microglia induces the apoptosis of neural precursors (Ghosh and Basu, 2009; Guadagno et al., 2013). Furthermore, ZIKV can infect astrocytes during perinatal stages and adulthood (Limonta et al., 2018; Stefanik et al., 2018) and probably induces the production of several chemokines and cytokines mainly through UPR activation, since IFN-modulated pathways regulating cytokines production, such as the Jak1/Stat3 signaling, are efficiently inhibited by Zika infection (O’Shea and Plenge, 2012; Cao et al., 2017). Microglia, in turn, releases IL-6 cytokines, which synergize with ER stress within astrocytes, to further promote inflammatory responses (Meares et al., 2014). This feed-forward loop is most probably at the basis of CD8+ T cell infiltration in CNS, since high levels of IL-6 and CXCL10 release and ER stress induction in astrocytes might be the main cause of breakdown of the brain blood barrier and lymphocyte chemoattraction/activation (Lunardi et al., 2015; Lee et al., 2016; Manangeeswaran et al., 2016; Jurado et al., 2018). Upon infiltration of the immune privileged CNS and/or PNS (e.g., the dorsal root ganglion) by these activated CD8+ T cells and other lymphocytes, their cellular activities may contribute to the neurological sequelae of perinatal and adult ZIKV infection (Manangeeswaran et al., 2016; Huang et al., 2017; Zukor et al., 2018). Interestingly, interleukin-induced UPR activation might underlie lymphocyte-mediated neuronal damages since this pathway also has important roles in the activation of the adaptive immune pathway (Kamimura and Bevan, 2008; Grootjans et al., 2016). Thus, direct and/or indirect induction of UPR responses by ZIKV may regulate both innate and adaptive immune responses (Smith, 2014; Sprenkle et al., 2017) and be crucial for the insurgence of ZIKV pathogenesis and co-morbidities.
Further exploration of this signaling system by delineating the temporal dynamics between viral infection, replication, and dissemination with the co-administration of UPR inhibitors would be useful toward elucidating the relationships between UPR, cell proliferation and death to construct a more exhaustive understanding of interactions of flaviviruses with host cells and uncovering novel therapeutic targets.
Perspectives
Our work has improved the understanding of ZIKV-induced defects by providing a more comprehensive perspective to other molecular studies that implicate different pathways in the cellular response to ZIKV infection. For example, the observed up-regulation of the pro-apoptotic factor p53 in PC12 cells overexpressing different ZIKV structural proteins and the consequent activation of a caspase-9- and caspase-3-mediated apoptotic pattern (Liu et al., 2018) might be attributable to a consistent and prolonged UPR activity. Indeed, Lin et al. (2012) demonstrated that UPR regulates p53 expression through modulation of NF-kB, which in turn, has a crucial role in several processes such as cell proliferation, differentiation, apoptosis, and inflammation and immune responses. Another report demonstrated that ZIKV acts, at least in part, by inhibiting the Akt-mTOR pathway, hence inducing strong autophagy and a block of neurogenesis (Liang et al., 2017). Previous work clearly showed that UPR up-regulation modulates autophagy through the same pathway (Qin et al., 2010). Finally, it was recently proposed that a ZIKV-induced increase in p53 expression and a deregulation of the mTOR pathway might induce early shifting from glycolysis to oxidative phosphorylation (OXPHOS) in mature myelin-producing cells notably in Schwann cells of peripheral nervous system. These recent reports suggest that activation of p53 is an early, albeit specific event in ZIKV infection that may result from cell- or non-cell- autonomous mechanisms (Devhare et al., 2017; Liu et al., 2018). These cellular events could also unsettle the homeostatic demands on cell proliferation, differentiation and metabolism, and potentially perturb cellular activities that lead to neurological diseases such as Guillain-Barre syndrome (Rothan et al., 2018). Thus, ZIKV-induced UPR deregulation may account for many of the defects observed in infected brains such as brain calcification, cell cycle and neurogenic impairments, cell death increase and cortical laminar defects.
Whilst clinicians and epidemiologists continue to investigate the distribution of ZIKV amongst different human populations in order to identify potential biological and socio-economic co-acting risk factors (Campos et al., 2015), clinical case studies remain sparse in comparison to molecular studies in animal and cellular models in vivo and in vitro, respectively. Recent gynecological reports demonstrate that the dramatic presentation of microcephaly in ZIKV-infected fetuses is not the most prominent clinical presentation, and is overshadowed by radiological findings of ventriculomegaly, cortical atrophy, calcifications (particularly located at the cortico-subcortical junction), and anomalies of the corpus callosum (Netto et al., 2017; Schaub et al., 2017a). Furthermore, emerging pediatric case reports of congenitally ZIKV-infected infants support that the spectrum of fetal brain and other anomalies is broader and more complex than microcephaly alone and includes subtle fetal brain injuries (Walker et al., 2018), ocular and auditory defects (Peloggia et al., 2018), as well as histopathological findings of viral dissemination and detection within extra-CNS tissues of post-mortem samples (Valdespino-Vazquez et al., 2018).
Conclusion
With the global increase in arthropod- and mosquito-borne diseases, it remains imperative to better understand the epidemiology and pathophysiology underlying the teratogenic effects ZIKV and other related arboviruses [reviewed in Charlier et al. (2017)]. The combined efforts of the public with clinical, translational, and fundamental research will hopefully help limit the health and economic burden associated with emerging arboviruses and help limit their associated morbidity.
Author Contributions
CA and IG-N wrote the manuscript with the help of the other authors. All authors edited the manuscript.
Funding
This work was supported by the European Union’s Horizon 2020 Research and Innovation Programme under ZIKAlliance Grant Agreement N° 734548 (to LN and ML) and by the European Virus Archives goes Global (EVAg) project under grant agreement N° 653316. ML was also funded by Institut Pasteur, INSERM, and LabEx IBEID. LN was funded by F.R.S.-F.N.R.S., the Fonds Léon Fredericq, the Fondation Médicale Reine Elisabeth, the Fondation Simone et Pierre Clerdent, the Belgian Science Policy (IAP-VII network P7/20), the ARC (ARC11/16-01), the EU H2020 ZIKAlliance (#734548), the ERANET Neuron STEM-MCD, and NeuroTalk. LN and IG-N are, respectively senior research associate and postdoctoral fellows from F.R.S- F.N.R.S.
Conflict of Interest Statement
The authors declare that the research was conducted in the absence of any commercial or financial relationships that could be construed as a potential conflict of interest.
Footnotes
References
Baud, D., Gubler, D. J., Schaub, B., Lanteri, M. C., and Musso, D. (2017). An update on Zika virus infection. Lancet 390, 2099–2109. doi: 10.1016/S0140-6736(17)31450-2
Bell, T. M., Field, E. J., and Narang, H. K. (1971). Zika virus infection of the central nervous system of mice. Arch. Gesamte Virusforsch. 35, 183–193. doi: 10.1007/BF01249709
Blazquez, A. B., Escribano-Romero, E., Merino-Ramos, T., Saiz, J. C., and Martin-Acebes, M. A. (2014). Stress responses in flavivirus-infected cells: activation of unfolded protein response and autophagy. Front. Microbiol. 5:266. doi: 10.3389/fmicb.2014.00266
Bogoch, I. I., Brady, O. J., Kraemer, M. U. G., German, M., Creatore, M. I., Kulkarni, M. A., et al. (2016). Anticipating the international spread of Zika virus from Brazil. Lancet 387, 335–336. doi: 10.1016/S0140-6736(16)00080-5
Campos, G. S., Bandeira, A. C., and Sardi, S. I. (2015). Zika virus outbreak, Bahia, Brazil. Emerg. Infect. Dis. 21, 1885–1886. doi: 10.3201/eid2110.150847
Cao, B., Diamond, M. S., and Mysorekar, I. U. (2017). Maternal-fetal transmission of Zika Virus: routes and signals for infection. J Interferon Cytokine Res. 37, 287–294. doi: 10.1089/jir.2017.0011
Cao-Lormeau, V. M., Blake, A., Mons, S., Lastere, S., Roche, C., Vanhomwegen, J., et al. (2016). Guillain-Barre syndrome outbreak associated with Zika virus infection in French Polynesia: a case-control study. Lancet 387, 1531–1539. doi: 10.1016/S0140-6736(16)00562-6
Caswell, R. J., and Manavi, K. (2018). Emerging sexually transmitted viral infections: 2. Review of Zika virus disease. Int. J. Std. Aids 1:956462418779465. doi: 10.1177/0956462418779465
Charlier, C., Beaudoin, M. C., Couderc, T., Lortholary, O., and Lecuit, M. (2017). Arboviruses and pregnancy: maternal, fetal, and neonatal effects. Lancet Child Adolesc. Health 1, 134–146. doi: 10.1016/S2352-4642(17)30021-4
Chaudhary, V., Yuen, K. S., Chan, J. F., Chan, C. P., Wang, P. H., Cai, J. P., et al. (2017). Selective activation of type II interferon signaling by Zika Virus NS5 Protein. J. Virol. 91:e00163–17. doi: 10.1128/JVI.00163-17
Coelho, A. V. C., and Crovella, S. (2017). Microcephaly prevalence in infants born to Zika Virus-infected women: a systematic review and meta-analysis. Int J Mol Sci. 18:E1714 doi: 10.3390/ijms18081714
Cortese, M., Goellner, S., Acosta, E. G., Neufeldt, C. J., Oleksiuk, O., Lampe, M., et al. (2017). Ultrastructural characterization of Zika Virus replication factories. Cell Rep. 18, 2113–2123. doi: 10.1016/j.celrep.2017.02.014
Counotte, M. J., Kim, C. R., Wang, J., Bernstein, K., Deal, C. D., Broutet, N. J. N., et al. (2018). Sexual transmission of Zika virus and other flaviviruses: a living systematic review. PLoS Med. 15:e1002611. doi: 10.1371/journal.pmed.1002611
Cross, B. C., Bond, P. J., Sadowski, P. G., Jha, B. K., Zak, J., Goodman, J. M., et al. (2012). The molecular basis for selective inhibition of unconventional mRNA splicing by an IRE1-binding small molecule. Proc. Natl. Acad. Sci. U.S.A. 109, E869–E878. doi: 10.1073/pnas.1115623109
Cugola, F. R., Fernandes, I. R., Russo, F. B., Freitas, B. C., Dias, J. L., Guimaraes, K. P., et al. (2016). The Brazilian Zika virus strain causes birth defects in experimental models. Nature 534, 267–271. doi: 10.1038/nature18296
Devhare, P., Meyer, K., Steele, R., Ray, R. B., and Ray, R. (2017). Zika virus infection dysregulates human neural stem cell growth and inhibits differentiation into neuroprogenitor cells. Cell Death Dis. 8:e3106. doi: 10.1038/cddis.2017.517
Dick, G. W., Kitchen, S. F., and Haddow, A. J. (1952). Zika virus. I. Isolations and serological specificity. Trans. R. Soc. Trop. Med. Hygiene 46, 509–520. doi: 10.1016/0035-9203(52)90042-4
Diop, F., Vial, T., Ferraris, P., Wichit, S., Bengue, M., Hamel, R., et al. (2018). Zika virus infection modulates the metabolomic profile of microglial cells. PLoS One 13:e0206093. doi: 10.1371/journal.pone.0206093
Duffy, M. R., Chen, T. H., Hancock, W. T., Powers, A. M., Kool, J. L., Lanciotti, R. S., et al. (2009). Zika virus outbreak on Yap Island, Federated States of Micronesia. N. Engl. J. Med. 360, 2536–2543. doi: 10.1056/NEJMoa0805715
Dugas-Ford, J., and Ragsdale, C. W. (2015). Levels of homology and the problem of neocortex. Annu. Rev. Neurosci. 38, 351–368. doi: 10.1146/annurev-neuro-071714-033911
Franca, T. L. B., Medeiros, W. R., Souza, N. L., Longo, E., Pereira, S. A., Franca, T. B. O., et al. (2018). Growth and development of children with microcephaly associated with congenital Zika Virus Syndrome in Brazil. Int. J. Environ. Res. Public Health 15:1990. doi: 10.3390/ijerph15091990
Galmiche, A., Sauzay, C., Chevet, E., and Pluquet, O. (2017). Role of the unfolded protein response in tumor cell characteristics and cancer outcome. Curr. Opin. Oncol. 29, 41–47. doi: 10.1097/CCO.0000000000000339
Garcez, P. P., Nascimento, J. M., de Vasconcelos, J. M., Madeiro da Costa, R., Delvecchio, R., Trindade, P., et al. (2017). Zika virus disrupts molecular fingerprinting of human neurospheres. Sci. Rep. 7:40780. doi: 10.1038/srep40780
Garcez, P. P., Stolp, H. B., Sravanam, S., Christoff, R. R., Ferreira, J., Dias, A. A., et al. (2018). Zika virus impairs the development of blood vessels in a mouse model of congenital infection. Sci. Rep. 8:12774. doi: 10.1038/s41598-018-31149-3
Ghosh, D., and Basu, A. (2009). Japanese encephalitis-a pathological and clinical perspective. PLoS Negl. Trop. Dis. 3:e437. doi: 10.1371/journal.pntd.0000437
Ghouzzi, V. E., Bianchi, F. T., Molineris, I., Mounce, B. C., Berto, G. E., Rak, M., et al. (2016). ZIKA virus elicits P53 activation and genotoxic stress in human neural progenitors similar to mutations involved in severe forms of genetic microcephaly. Cell Death Dis. 7:e2440. doi: 10.1038/cddis.2016.266
Gladwyn-Ng, I., Cordon-Barris, L., Alfano, C., Creppe, C., Couderc, T., Morelli, G., et al. (2018). Stress-induced unfolded protein response contributes to Zika virus-associated microcephaly. Nat. Neurosci. 21, 63–71. doi: 10.1038/s41593-017-0038-4
Godin, J. D., Creppe, C., Laguesse, S., and Nguyen, L. (2016). Emerging roles for the unfolded protein response in the developing nervous system. Trends Neurosci. 39, 394–404. doi: 10.1016/j.tins.2016.04.002
Grootjans, J., Kaser, A., Kaufman, R. J., and Blumberg, R. S. (2016). The unfolded protein response in immunity and inflammation. Nat. Rev. Immunol. 16, 469–484. doi: 10.1038/nri.2016.62
Guadagno, J., Xu, X., Karajgikar, M., Brown, A., and Cregan, S. P. (2013). Microglia-derived TNFalpha induces apoptosis in neural precursor cells via transcriptional activation of the Bcl-2 family member Puma. Cell Death Dis. 4:e538. doi: 10.1038/cddis.2013.59
Gurumayum, S., Brahma, R., Naorem, L. D., Muthaiyan, M., Gopal, J., and Venkatesan, A. (2018). ZikaBase: an integrated ZIKV- human interactome Map database. Virology 514, 203–210. doi: 10.1016/j.virol.2017.11.007
Hanisch, U. K. (2002). Microglia as a source and target of cytokines. Glia 40, 140–155. doi: 10.1002/glia.10161
Himmelsbach, K., and Hildt, E. (2018). Identification of various cell culture models for the study of Zika virus. World J. Virol. 7, 10–20. doi: 10.5501/wjv.v7.i1.10
Hosoi, T., Honda, M., Oba, T., and Ozawa, K. (2013). ER stress upregulated PGE(2)/IFNgamma-induced IL-6 expression and down-regulated iNOS expression in glial cells. Sci. Rep. 3:3388. doi: 10.1038/srep03388
Huang, H., Li, S., Zhang, Y., Han, X., Jia, B., Liu, H., et al. (2017). CD8(+) T cell immune response in immunocompetent mice during Zika Virus infection. J. Virol. 15:91. doi: 10.1128/JVI.00900-17
Jurado, K. A., Yockey, L. J., Wong, P. W., Lee, S., Huttner, A. J., and Iwasaki, A. (2018). Antiviral CD8 T cells induce Zika-virus-associated paralysis in mice. Nat. Microbiol. 3, 141–147. doi: 10.1038/s41564-017-0060-z
Kamimura, D., and Bevan, M. J. (2008). Endoplasmic reticulum stress regulator XBP-1 contributes to effector CD8+ T cell differentiation during acute infection. J. Immunol. 181, 5433–5441. doi: 10.4049/jimmunol.181.8.5433
Kim, S., Joe, Y., Kim, H. J., Kim, Y. S., Jeong, S. O., Pae, H. O., et al. (2015). Endoplasmic reticulum stress-induced IRE1alpha activation mediates cross-talk of GSK-3beta and XBP-1 to regulate inflammatory cytokine production. J. Immunol. 194, 4498–4506. doi: 10.4049/jimmunol.1401399
Kozak, R. A., Majer, A., Biondi, M. J., Medina, S. J., Goneau, L. W., Sajesh, B. V., et al. (2017). MicroRNA and mRNA dysregulation in astrocytes infected with Zika Virus. Viruses 9:E297. doi: 10.3390/v9100297
Kum, D. B., Mishra, N., Boudewijns, R., Gladwyn-Ng, I., Alfano, C., Ma, J., et al. (2018). A yellow fever-Zika chimeric virus vaccine candidate protects against Zika infection and congenital malformations in mice. NPJ Vaccines 3:56. doi: 10.1038/s41541-018-0092-2
Laguesse, S., Creppe, C., Nedialkova, D. D., Prevot, P. P., Borgs, L., Huysseune, S., et al. (2015a). A dynamic unfolded protein response contributes to the control of cortical neurogenesis. Dev. Cell 35, 553–567. doi: 10.1016/j.devcel.2015.11.005
Laguesse, S., Peyre, E., and Nguyen, L. (2015b). Progenitor genealogy in the developing cerebral cortex. Cell Tissue Res. 359, 17–32. doi: 10.1007/s00441-014-1979-5
Lanciotti, R. S., Kosoy, O. L., Laven, J. J., Velez, J. O., Lambert, A. J., Johnson, A. J., et al. (2008). Genetic and serologic properties of Zika virus associated with an epidemic, Yap State, Micronesia, 2007. Emerg. Infect. Dis. 14, 1232–1239. doi: 10.3201/eid1408.080287
Lee, Y. J., Won, T. J., Hyung, K. E., Jang, Y. W., Kim, S. J., Lee do, I., et al. (2016). IL-6 induced proliferation and cytotoxic activity of CD8(+) T cells is elevated by SUMO2 overexpression. Arch. Pharm. Res. 39, 705–712. doi: 10.1007/s12272-016-0736-6
Li, C., Xu, D., Ye, Q., Hong, S., Jiang, Y., Liu, X., et al. (2016). Zika virus disrupts neural progenitor development and leads to microcephaly in mice. Cell Stem Cell 19, 120–126. doi: 10.1016/j.stem.2016.04.017
Liang, Y., Zhou, T., Chen, Y., Lin, D., Jing, X., Peng, S., et al. (2017). Rifampicin inhibits rotenone-induced microglial inflammation via enhancement of autophagy. Neurotoxicology 63, 137–145. doi: 10.1016/j.neuro.2017.09.015
Limonta, D., Jovel, J., Kumar, A., Airo, A. M., Hou, S., Saito, L., et al. (2018). Human fetal astrocytes infected with Zika Virus exhibit delayed apoptosis and resistance to interferon: implications for persistence. Viruses 10:E646. doi: 10.3390/v10110646
Lin, W. C., Chuang, Y. C., Chang, Y. S., Lai, M. D., Teng, Y. N., Su, I. J., et al. (2012). Endoplasmic reticulum stress stimulates p53 expression through NF-kappaB activation. PLoS One 7:e39120. doi: 10.1371/journal.pone.0039120
Liu, J., Li, Q., Li, X., Qiu, Z., Li, A., Liang, W., et al. (2018). Zika Virus envelope protein induces G2/M cell cycle arrest and apoptosis via an intrinsic cell death signaling pathway in neuroendocrine PC12 cells. Int. J. Biol. Sci. 14, 1099–1108. doi: 10.7150/ijbs.26400
Lum, F. M., Low, D. K., Fan, Y., Tan, J. J., Lee, B., Chan, J. K., et al. (2017). Zika Virus infects human fetal brain microglia and induces inflammation. Clin. Infect. Dis. 64, 914–920. doi: 10.1093/cid/ciw878
Lunardi, S., Lim, S. Y., Muschel, R. J., and Brunner, T. B. (2015). IP-10/CXCL10 attracts regulatory T cells: implication for pancreatic cancer. Oncoimmunology 4:e1027473. doi: 10.1080/2162402X.2015.1027473
Manangeeswaran, M., Ireland, D. D., and Verthelyi, D. (2016). Zika (PRVABC59) infection is associated with T cell infiltration and neurodegeneration in CNS of immunocompetent neonatal C57Bl/6 mice. PLoS Pathog. 12:e1006004. doi: 10.1371/journal.ppat.1006004
Matusali, G., Houzet, L., Satie, A. P., Mahe, D., Aubry, F., Couderc, T., et al. (2018). Zika virus infects human testicular tissue and germ cells. J. Clin. Invest. 128, 4697–4710. doi: 10.1172/JCI121735
Mavigner, M., Raper, J., Kovacs-Balint, Z., Gumber, S., O’Neal, J. T., Bhaumik, S. K., et al. (2018). Postnatal Zika virus infection is associated with persistent abnormalities in brain structure, function, and behavior in infant macaques. Sci. Trans. Med. 10:eaao6975. doi: 10.1126/scitranslmed.aao6975
McCarthy, M. (2016). Severe eye damage in infants with microcephaly is presumed to be due to Zika virus. BMJ 352:i855. doi: 10.1136/bmj.i855
Meares, G. P., Liu, Y., Rajbhandari, R., Qin, H., Nozell, S. E., Mobley, J. A., et al. (2014). PERK-dependent activation of JAK1 and STAT3 contributes to endoplasmic reticulum stress-induced inflammation. Mol. Cell Biol. 34, 3911–3925. doi: 10.1128/MCB.00980-14
Meertens, L., Labeau, A., Dejarnac, O., Cipriani, S., Sinigaglia, L., Bonnet-Madin, L., et al. (2017). Axl mediates ZIKA Virus entry in human glial cells and modulates innate immune responses. Cell Rep. 18, 324–333. doi: 10.1016/j.celrep.2016.12.045
Monel, B., Compton, A. A., Bruel, T., Amraoui, S., Burlaud-Gaillard, J., Roy, N., et al. (2017). Zika virus induces massive cytoplasmic vacuolization and paraptosis-like death in infected cells. EMBO J. 36, 1653–1668. doi: 10.15252/embj.201695597
Moore, C. A., Staples, J. E., Dobyns, W. B., Pessoa, A., Ventura, C. V., Fonseca, E. B., et al. (2017). Characterizing the pattern of anomalies in congenital zika syndrome for pediatric clinicians. JAMA Pediatr. 171, 288–295. doi: 10.1001/jamapediatrics.2016.3982
Moreno, J. A., Halliday, M., Molloy, C., Radford, H., Verity, N., Axten, J. M., et al. (2013). Oral treatment targeting the unfolded protein response prevents neurodegeneration and clinical disease in prion-infected mice. Sci. Trans. Med. 5:206ra138. doi: 10.1126/scitranslmed.3006767
Musso, D., and Gubler, D. J. (2016). Zika Virus. Clin. Microbiol. Rev. 29, 487–524. doi: 10.1128/CMR.00072-15
Netto, E. M., Moreira-Soto, A., Pedroso, C., Hoser, C., Funk, S., Kucharski, A. J., et al. (2017). High zika virus seroprevalence in salvador, northeastern brazil limits the potential for further outbreaks. mBio 8:e01390-17. doi: 10.1128/mBio.01390-17
Oehler, E., Watrin, L., Larre, P., Leparc-Goffart, I., Lastere, S., Valour, F., et al. (2014). Zika virus infection complicated by guillain-barre syndrome–case report, French Polynesia, December 2013. Euro Surveill. 19:20720. doi: 10.2807/1560-7917.ES2014.19.9.20720
Olmo, I. G., Carvalho, T. G., Costa, V. V., Alves-Silva, J., Ferrari, C. Z., Izidoro-Toledo, T. C., et al. (2017). Zika Virus promotes neuronal cell death in a non-cell autonomous manner by triggering the release of neurotoxic factors. Front. Immunol. 8:1016. doi: 10.3389/fimmu.2017.01016
O’Shea, J. J., and Plenge, R. (2012). JAK and STAT signaling molecules in immunoregulation and immune-mediated disease. Immunity 36, 542–550. doi: 10.1016/j.immuni.2012.03.014
Peloggia, A., Ali, M., Nanda, K., and Bahamondes, L. (2018). Zika virus exposure in pregnancy and its association with newborn visual anomalies and hearing loss. Int. J. Gynaecol. Obstet. 143, 277–281. doi: 10.1002/ijgo.12663
Qin, L., Wang, Z., Tao, L., and Wang, Y. (2010). ER stress negatively regulates AKT/TSC/mTOR pathway to enhance autophagy. Autophagy 6, 239–247. doi: 10.4161/auto.6.2.11062
Radford, H., Moreno, J. A., Verity, N., Halliday, M., and Mallucci, G. R. (2015). PERK inhibition prevents tau-mediated neurodegeneration in a mouse model of frontotemporal dementia. Acta Neuropathol. 130, 633–642. doi: 10.1007/s00401-015-1487-z
Richardson, R. B., Ohlson, M. B., Eitson, J. L., Kumar, A., McDougal, M. B., Boys, I. N., et al. (2018). A CRISPR screen identifies IFI6 as an ER-resident interferon effector that blocks flavivirus replication. Nat. Microbiol. 3, 1214–1223. doi: 10.1038/s41564-018-0244-1
Rolfe, A. J., Bosco, D. B., Wang, J., Nowakowski, R. S., Fan, J., and Ren, Y. (2016). Bioinformatic analysis reveals the expression of unique transcriptomic signatures in Zika virus infected human neural stem cells. Cell Biosci. 6:42. doi: 10.1186/s13578-016-0110-x
Rothan, H. A., Fang, S., Mahesh, M., and Byrareddy, S. N. (2018). Zika virus and the metabolism of neuronal cells. Mol. Neurobiol. doi: 10.1007/s12035-018-1263-x [Epub ahead of print].
Saiz, J. C., Martin-Acebes, M. A., Bueno-Mari, R., Salomon, O. D., Villamil-Jimenez, L. C., Heukelbach, J., et al. (2017). Zika Virus: what have we learnt since the start of the recent epidemic? Front. Microbiol. 8:1554. doi: 10.3389/fmicb.2017.01554
Sakkas, H., Bozidis, P., Giannakopoulos, X., Sofikitis, N., and Papadopoulou, C. (2018). An update on sexual transmission of Zika Virus. Pathogens 7:E66. doi: 10.3390/pathogens7030066
Schaub, B., Gueneret, M., Jolivet, E., Decatrelle, V., Yazza, S., Gueye, H., et al. (2017a). Ultrasound imaging for identification of cerebral damage in congenital Zika virus syndrome: a case series. Lancet Child Adolesc. Health 1, 45–55. doi: 10.1016/S2352-4642(17)30001-9
Schaub, B., Vouga, M., Najioullah, F., Gueneret, M., Monthieux, A., Harte, C., et al. (2017b). Analysis of blood from Zika virus-infected fetuses: a prospective case series. Lancet Infect. Dis. 17, 520–527. doi: 10.1016/S1473-3099(17)30102-0
Scheper, W., and Hoozemans, J. J. (2015). The unfolded protein response in neurodegenerative diseases: a neuropathological perspective. Acta Neuropathol. 130, 315–331. doi: 10.1007/s00401-015-1462-8
Smith, J. A. (2014). A new paradigm: innate immune sensing of viruses via the unfolded protein response. Front. Microbiol. 5:222. doi: 10.3389/fmicb.2014.00222
Smith, J. A. (2018). Regulation of cytokine production by the unfolded protein response; implications for infection and autoimmunity. Front. Immunol. 9:422. doi: 10.3389/fimmu.2018.00422
Sprenkle, N. T., Sims, S. G., Sanchez, C. L., and Meares, G. P. (2017). Endoplasmic reticulum stress and inflammation in the central nervous system. Mol. Neurodegener. 12:42. doi: 10.1186/s13024-017-0183-y
Stefanik, M., Formanova, P., Bily, T., Vancova, M., Eyer, L., Palus, M., et al. (2018). Characterisation of Zika virus infection in primary human astrocytes. BMC Neurosci. 19:5. doi: 10.1186/s12868-018-0407-2
Subissi, L., Dub, T., Besnard, M., Mariteragi-Helle, T., Nhan, T., Lutringer-Magnin, D., et al. (2018). Zika Virus Infection during Pregnancy and Effects on Early Childhood Development, French Polynesia, 2013-2016. Emerg. Infect. Dis. 24, 1850–1858. doi: 10.3201/eid2410.172079
Ta, H. M., Le, T. M., Ishii, H., Takarada-Iemata, M., Hattori, T., Hashida, K., et al. (2016). Atf6alpha deficiency suppresses microglial activation and ameliorates pathology of experimental autoimmune encephalomyelitis. J. Neurochem. 139, 1124–1137. doi: 10.1111/jnc.13714
Tan, Z., Zhang, W., Sun, J., Fu, Z., Ke, X., Zheng, C., et al. (2018). ZIKV infection activates the IRE1-XBP1 and ATF6 pathways of unfolded protein response in neural cells. J. Neuroinflammation 15:275. doi: 10.1186/s12974-018-1311-5
Tang, H., Hammack, C., Ogden, S. C., Wen, Z., Qian, X., Li, Y., et al. (2016). Zika virus infects human cortical neural progenitors and attenuates their growth. Cell Stem Cell 18, 587–590. doi: 10.1016/j.stem.2016.02.016
Tuttle, A. H., Philip, V. M., Chesler, E. J., and Mogil, J. S. (2018). Comparing phenotypic variation between inbred and outbred mice. Nat. Methods 15, 994–996. doi: 10.1038/s41592-018-0224-7
Valdespino-Vazquez, M. Y., Sevilla-Reyes, E. E., Lira, R., Yocupicio-Monroy, M., Piten-Isidro, E., Boukadida, C., et al. (2018). Congenital Zika syndrome and extra-CNS detection of Zika virus in a pre-term newborn in Mexico. Clin. Infect. Dis. doi: 10.1093/cid/ciy616 [Epub ahead of print].
van der Linden, H. Jr., Carvalho, M. D., van der Linden, V., Lacerda, K. M., Pessoa, A., Carneiro, M. L., et al. (2018). Epilepsy Profile in Infants with Congenital Zika Virus Infection. N. Engl. J. Med. 379, 891–892. doi: 10.1056/NEJMc1716070
van Weering, H. R., Boddeke, H. W., Vinet, J., Brouwer, N., de Haas, A. H., van Rooijen, N., et al. (2011). CXCL10/CXCR3 signaling in glia cells differentially affects NMDA-induced cell death in CA and DG neurons of the mouse hippocampus. Hippocampus 21, 220–232. doi: 10.1002/hipo.20742
Walker, C. L., Little, M. E., Roby, J. A., Armistead, B., Gale, M. Jr., Rajagopal, L., et al. (2018). Zika virus and the non-microcephalic fetus: why we should still worry. Am. J. Obstet. Gynecol. 220, 45–56. doi: 10.1016/j.ajog.2018.08.035
Wang, J., Liu, J., Zhou, R., Ding, X., Zhang, Q., Zhang, C., et al. (2018). Zika virus infected primary microglia impairs NPCs proliferation and differentiation. Biochem. Biophys. Res. Commun. 497, 619–625. doi: 10.1016/j.bbrc.2018.02.118
Wen, Z., Song, H., and Ming, G. L. (2017). How does Zika virus cause microcephaly? Genes Dev. 31, 849–861. doi: 10.1101/gad.298216.117
Wu, K. Y., Zuo, G. L., Li, X. F., Ye, Q., Deng, Y. Q., Huang, X. Y., et al. (2016). Vertical transmission of Zika virus targeting the radial glial cells affects cortex development of offspring mice. Cell Res. 26, 645–654. doi: 10.1038/cr.2016.58
Xu, C., Bailly-Maitre, B., and Reed, J. C. (2005). Endoplasmic reticulum stress: cell life and death decisions. J. Clin. Invest. 115, 2656–2664. doi: 10.1172/JCI26373
Ye, L., Huang, Y., Zhao, L., Li, Y., Sun, L., Zhou, Y., et al. (2013). IL-1beta and TNF-alpha induce neurotoxicity through glutamate production: a potential role for neuronal glutaminase. J. Neurochem. 125, 897–908. doi: 10.1111/jnc.12263
Zhang, F., Hammack, C., Ogden, S. C., Cheng, Y., Lee, E. M., Wen, Z., et al. (2016). Molecular signatures associated with ZIKV exposure in human cortical neural progenitors. Nucleic Acids Res. 44, 8610–8620. doi: 10.1093/nar/gkw765
Zhang, K., and Kaufman, R. J. (2008). From endoplasmic-reticulum stress to the inflammatory response. Nature 24, 455–462. doi: 10.1038/nature07203
Keywords: unfolded protein response, ER stress, cortical progenitors, cerebral cortex, microcephaly, Zika virus
Citation: Alfano C, Gladwyn-Ng I, Couderc T, Lecuit M and Nguyen L (2019) The Unfolded Protein Response: A Key Player in Zika Virus-Associated Congenital Microcephaly. Front. Cell. Neurosci. 13:94. doi: 10.3389/fncel.2019.00094
Received: 31 October 2018; Accepted: 26 February 2019;
Published: 26 March 2019.
Edited by:
Yannick Simonin, Université de Montpellier, FranceReviewed by:
Juarez Antonio Simões Quaresma, Instituto Evandro Chagas, BrazilAna Belen Blazquez, Instituto Nacional de Investigación y Tecnología Agraria y Alimentaria (INIA), Spain
Copyright © 2019 Alfano, Gladwyn-Ng, Couderc, Lecuit and Nguyen. This is an open-access article distributed under the terms of the Creative Commons Attribution License (CC BY). The use, distribution or reproduction in other forums is permitted, provided the original author(s) and the copyright owner(s) are credited and that the original publication in this journal is cited, in accordance with accepted academic practice. No use, distribution or reproduction is permitted which does not comply with these terms.
*Correspondence: Marc Lecuit, bWFyYy5sZWN1aXRAcGFzdGV1ci5mcg== Laurent Nguyen, bG5ndXllbkB1bGllZ2UuYmU=
†These authors have contributed equally to this work